- 1Colegio de Postgraduados Campus Córdoba, Amatlán de los Reyes, Veracruz, Mexico
- 2Colegio de Postgraduados Campus Montecillo, Texcoco, Mexico
Diacylglycerol kinases (DGKs) are pivotal signaling enzymes that phosphorylate diacylglycerol (DAG) to yield phosphatidic acid (PA). The biosynthesis of PA from phospholipase D (PLD) and the coupled phospholipase C (PLC)/DGK route is a crucial signaling process in eukaryotic cells. Next to PLD, the PLC/DGK pathway is the second most important generator of PA in response to biotic and abiotic stresses. In eukaryotic cells, DGK, DAG, and PA are implicated in vital processes such as growth, development, and responses to environmental cues. A plethora of DGK isoforms have been identified so far, making this a rather large family of enzymes in plants. Herein we performed a comprehensive phylogenetic analysis of DGK isoforms in model and crop plants in order to gain insight into the evolution of higher plant DGKs. Furthermore, we explored the expression profiling data available in public data bases concerning the regulation of plant DGK genes in response to beneficial elements and other metal and metalloid ions, including silver (Ag), aluminum (Al), arsenic (As), cadmium (Cd), chromium (Cr), mercury (Hg), and sodium (Na). In all plant genomes explored, we were able to find DGK representatives, though in different numbers. The phylogenetic analysis revealed that these enzymes fall into three major clusters, whose distribution depends on the composition of structural domains. The catalytic domain conserves the consensus sequence GXGXXG/A where ATP binds. The expression profiling data demonstrated that DGK genes are rapidly but transiently regulated in response to certain concentrations and time exposures of beneficial elements and other ions in different plant tissues analyzed, suggesting that DGKs may mediate signals triggered by these elements. Though this evidence is conclusive, further signaling cascades that such elements may stimulate during hormesis, involving the phosphoinositide signaling pathway and DGK genes and enzymes, remain to be elucidated.
Introduction
Plants have the remarkable capability of responding to environmental cues thanks to different signal transduction cascades, which are triggered by signaling molecules that perceive and expand external and internal signals, resulting in plant adaptation reactions (Arisz et al., 2009; Sparks et al., 2013; Abd-El-Haliem et al., 2016). Some of the most important signaling molecules in plants involve lipids, including phosphoinositides (PPI), sphingolipids, lysophospholipids, oxylipins, N-acylethanolamines, and free fatty acids (Wang and Chapman, 2013). Though most of the leaf structural lipids in plant cells are galactolipids (approximately 70%), phospholipids, including phosphatidic acid (PA), play important roles in signal perception and transduction (Testerink and Munnik, 2005).
PA has been proposed to be a pivotal second messenger in plants and its synthesis has been reported to be induced in response to ethylene (Munnik, 2001), abscisic acid (Zhang et al., 2004), wounding and Nod factor (Munnik, 2001), osmotic pressure (Munnik et al., 2000; Testerink et al., 2004), cold (Ruelland et al., 2002), salinity (Zhang et al., 2012), temperature changes (Arisz et al., 2013), pathogen attack (Zhang and Xiao, 2015), and drought (Li et al., 2015). In all of those cases, PA synthesis has been associated with plant cell adjustments to overcome such stress events. Nevertheless, some positive-strand RNA viruses use PA in order to stimulate their replication (Hyodo et al., 2015), which has to be taken into consideration when designing strategies to apply PA as a potential biostimulator of adaptive responses in crop plants. Importantly, PA is a precursor to all phosphoglycerolipids as well as triacylglycerols and galactolipids, and its turnover is crucial in determining lipid metabolic fluxes and membrane compositions (Arisz et al., 2009, 2013).
In plant cells, PA can be generated in the plasma membrane from two different metabolic pathways: (1) as a product of the hydrolysis of structural phospholipids, such as phosphatidylcholine and phosphatidylethanolamine by the action of different isoforms of phospholipase D (PLD; Hong et al., 2014); or (2) through the combined activity of phospholipase C (PLC) and diacylglycerol kinase (DGK). There are two types of PLCs in plant cells: those that take PPI as substrate, the so called phosphatidylinositol (PI)-PLCs, and those that hydrolyze structural phospholipids, known as non-specific PLCs (NPCs). In any case, PLCs yield diacylglycerol (DAG) and inositol 1,4,5-trisphosphate (IP3). After phosphatidylinositol 4,5-bisphosphate hydrolysis, IP3 diffuses into the cytosol and acts as a second messenger implicated in calcium (Ca2+) mobilization from intracellular compartments such as the vacuole, while DAG remains in the plasma membrane and may activate other physiological processes. DAG can then be phosphorylated by DGK to yield PA, which in turn can be further metabolized to PPI. Additionally, enzymes that dephosphorylate PA include lipid phosphate phosphatases and PA hydrolases (lipins). Moreover, PA can be further phosphorylated to diacylglycerol pyrophosphate by PA kinase, or metabolized to lyso-PA through PLA2 activity (Testerink and Munnik, 2011). Most of these lipids and enzymes have been proved to have signaling functions, and the synthesis of signaling lipids in response to different environmental cues and stressors is essentially transient (Testerink and Munnik, 2011; Ge et al., 2012).
In eukaryotic cells, PA levels are typically low, corresponding to 0.67% of total phospholipids (Arisz et al., 2000) and PA formation depends on the extracellular stimuli perceived.
In the green unicellular algae Chlamydomonas moewusii, hyperosmotic stress triggers the activation of both PA biosynthetic pathways (i.e., PLD and PLC/DGK; Munnik et al., 2000). Indeed, with the activation of mastoparan (a potent activator of PLC and PLD signaling in plants), 5–17% of PA is generated by PLD, while the rest is assumed to be generated by the PLC/DGK (Munnik et al., 1998). In cold-shock Arabidopsis stimulated cells (0°C), during the first 10 min up to 80% of PA is produced via the coordinated PLC/DGK pathway, whereas afterward the PLD pathway dominates (Ruelland et al., 2002). In Arabidopsis, the induction of programmed cell death due to the accumulation of H2O2 caused by UV irradiation or drought-induced dehydration is drastically reduced as a consequence of an overproduction of PA via PLD (Zhang et al., 2003). Interestingly, oxidative stress and wounding boost PA synthesis via the PLD pathway too (Munnik, 2001; Testerink and Munnik, 2005). This pathway (PLD) is also responsible for the total production of PA in Arabidopsis cells exposed to pathogens (de Torres Zabela et al., 2002). Nevertheless, in tomato cells exposed to the elicitors CH4 and flg22, PA biosynthesis is stimulated by PLC/DGK, whereas PLD does not show activity (van der Luit et al., 2000).
Based on significant contributions by a number of plant biologists (Munnik et al., 1998; Munnik, 2001; Gómez-Merino et al., 2004; Cai et al., 2009; Kirik and Mudgett, 2009; Xue et al., 2009; Gonorazky et al., 2010; Arisz and Munnik, 2011; Dubots et al., 2011; Shin and Loewen, 2011; Boss and Im, 2012; Arisz et al., 2013; Villasuso et al., 2013; Logothetis et al., 2015; Saucedo-García et al., 2015; Singh et al., 2015; Hou et al., 2016), Figure 1 shows the main metabolic pathways involving PLD, PLC, and DGK in the biosynthesis and turnover of PA in plant cells.
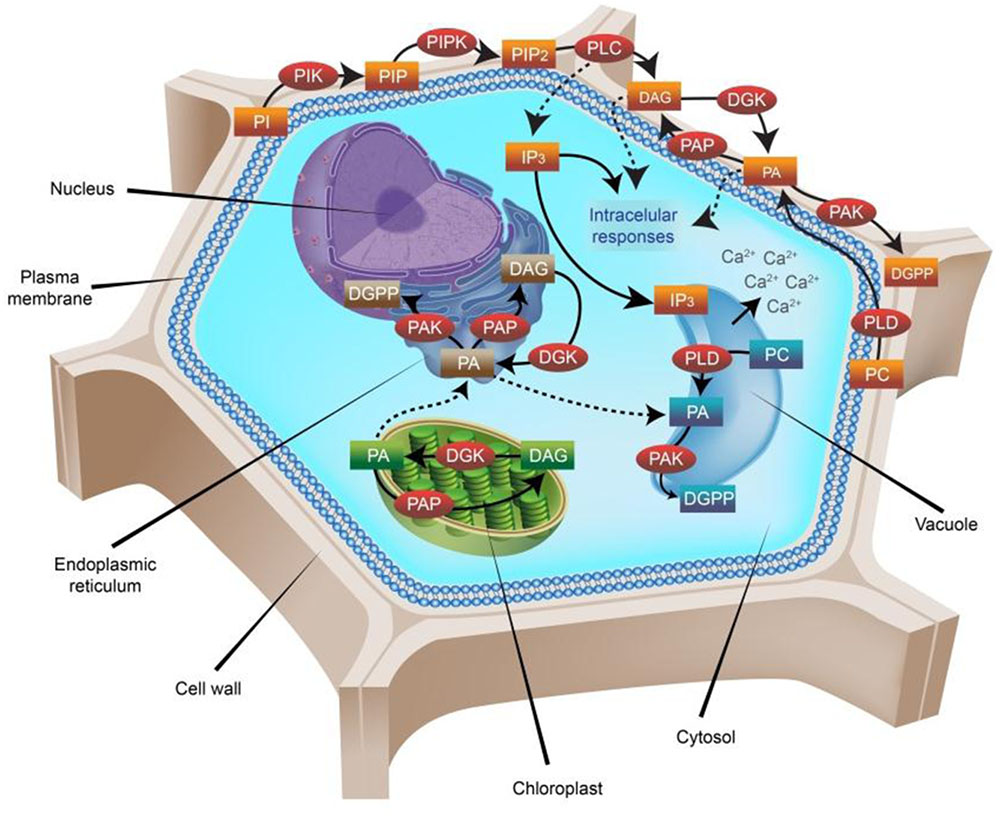
FIGURE 1. Phosphatidic acid (PA) metabolic pathways in higher plants. Nearly 30% of the plasma membrane in plants is composed of phospholipids, which can form lipid bilayers because of their amphiphilic properties. All phospholipids based on phosphatidylinositol (PI) are referred to as phosphoinositides (PPI). PIs are formed by two fatty-acid chains bounded to an inositol ring through a phosphodiester linkage. Phosphorylations of the inositol ring are carried out by specific PI kinases (PIK), including phosphatidylinositol 3-kinase (PI3K), phosphatidylinositol 4-kinase (PI4K) and phosphatidylinositol 5-kinase (PI5K) at the D-3, D-4, or D-5 positions, yielding phosphatidylinositol 3-phosphate (PI3P), phosphatidylinositol 4-phosphate (PI4P) or phosphatidylinositol 5-phosphate (PI5P), respectively. Sequential phosphorylation by phosphatidylinositol 5-phosphate 4-kinase (PIP4K) or phosphatidylinositol 4-phosphate 5-kinase (PIP5K) forms phosphatidylinositol 4,5-bisphosphate (PIP2), which is then hydrolyzed by phospholipase C (PLC), resulting in the production of two important second messengers: inositol trisphosphate (IP3) and diacylglycerol (DAG). The hydrophilic IP3 diffuses into the cytosol where it triggers Ca2+ release from intracellular stores, whereas the lipophilic DAG molecule remains in the membrane and participates in other signaling processes. DAG can be further phosphorylated by the enzyme diacylglycerol kinase (DGK), giving rise to phosphatidic acid (PA). Additionally, PLD hydrolyses phospholipids at the terminal phosphodiester bond and generates PA too. Further phosphorylation of PA by the enzyme PA kinase (PAK) generates diacylglycerol pyrophosphate (DGPP), which is also a signaling molecule in plant cells. Dephosphorylation of PA by the enzyme PA phosphatase (PAP) generates DAG again. DGK enzymes are present not only in the plasmalemma, but also in the endoplasmic reticulum (ER) and the chloroplast membranes. The PA produced in the chloroplast is transported to the ER, where it renders either DGPP (by a further phosphorylation carried out by PAK) or DAG (by the activity of PAP). In plasma membranes, the most abundant PPI is PIP2, where it comprises ∼1% of the total phospholipid pool present. Importantly, not all PPI signaling molecules are involved in signal perception and transduction all the time. Indeed, most of them are constantly participating in the biosynthesis and turnover of other phospholipids, and are recruited for signaling proposes just when needed. PA itself is not always expressed, and the magnitude of its variations looks crucial for plants. In the diagram, enzymes are represented in red circles, whereas substrates and products in rectangles are colored according to the localization of their origin: brown, ER; blue, vacuole; green, chloroplast; orange, plasma membrane.
Eukaryotic DGKs are a widespread family of enzymes, well conserved among most multicellular organisms. Plant DGK isoforms have a conserved catalytic domain with an ATP-binding site (consensus GXGXXG/A) required for kinase activity (Gómez-Merino et al., 2004). DGK activity is likely to be tightly regulated to control DAG and PA levels coordinately, enabling the cell to perform its physiological functions appropriately (Gómez-Merino et al., 2004). In higher plants, DGK enzymes are present in different isoforms and their activity has been proved in tomato (Solanum lycopersicum; SlDGK; Snedden and Blumwald, 2000), Arabidopsis (AtDGK; Gómez-Merino et al., 2004, 2005a), maize (Zea mays; ZmDGK; Sui et al., 2008), wheat (Triticum aestivum; TaDGK; Zhang et al., 2008), rice (Oryza sativa; OsDGK; Ge et al., 2012), and apple (Malus prunifolia; MpDGK; Li et al., 2015), among others. To date, cumulative evidence points to a crucial role of DGK enzymes in vital processes like growth, development and responses to environmental stimuli and stresses. Phylogenetic analyses have been performed for some plant species, including Arabidopsis and rice DGKs, though a more robust evolution analysis of DGKs in higher plants is still lacking, and their implications in response to nutrients, including potential and well-known beneficial elements, await further studies. Herewith we describe the physiological roles of characterized DGK from higher plants isolated so far and present a comprehensive phylogenetic analysis of DGK isoforms identified in the proteomes of important model and cultivated plants. Furthermore, based on the Genevestigator platform (Zimmermann et al., 2014) and experimental data, we also report a detailed expression profiling analysis of DGK genes in response to known beneficial elements such as aluminum (Al) and sodium (Na), as well as other metal and metalloid ions, including silver (Ag), arsenic (As), cadmium (Cd), chromium (Cr), and mercury (Hg). Beneficial elements are not required by all plants, but may be essential for certain taxa depending on environmental conditions, element concentration and plant species. When applied at low concentrations, they may elicit positive responses in growth, yield, and responses to environmental stresses (Pilon-Smits et al., 2009; Poschenrieder et al., 2013). Indeed, beneficial elements can prompt hormesis, a stimulatory effect of a low dose of a non-essential element (Calabrese, 2014). Herewith we provide evidence for the first time that the expression of some DGK genes may be modified when plants are exposed to some of those hormesis-inducing elements. Furthermore, we explored the expression profiling data of such genes in different plant tissues.
Materials and Methods
Phylogenetic Analysis of DGKs in Higher Plants
To identify DGK gene homologs in plant species, searches were performed based on the reported sequences of AtDGK (Gómez-Merino et al., 2004) using the BLAST software1 (Altschul et al., 1990) with a Block Substitution Matrix 62 (BLOSUM62), considering the non-redundant sequences deposited in the National Center for Biotechnology Information (NCBI)2 and the UniProtKB server3. According to Henikoff and Henikoff (1992), this matrix is more appropriate for searches and alignments than are matrices derived by extrapolation from mutation rates. For the first analysis reported herein, results were filtered, selecting only the DGK sequences previously characterized or included in other phylogenetic studies and next extracted in FASTA format. Subsequently, we carried out a multiple sequence alignment of the Arabidopsis DGK protein collected in the previous stage with the Clustal-omega1.2.1 software (Sievers et al., 2011). This software performs a multiple sequence alignment using the Hidden Markov Model (HMM; Yoon, 2009), for later constructing a tree guide using Muscle fast unweighted pair group method with arithmetic mean (UPGMA) implementation (Edgar, 2004). We used three iterations of this algorithm to refine the alignment. Final alignment was then transformed to NXS format, which was used as entry to construct the final phylogenetic tree. This tree was built using an evolutive method implemented in the MrBayes3.2.5 software4 (Altekar et al., 2004). This method is robust, and it is based on Markov Chain Monte Carlo simulation methods. When constructing the phylogenetic tree we integrated 26 taxa considering the following analytical parameters: a priority matrix of fixed amino acids (aamodel = mixed); the evolutive WAG method with variations described using a gamma-type distribution (rates = invgamma) and default hyperparameters; three million generations (ngen = 3000000); frequency of tree sampling each 100 generations (samplefreq = 1000); another 750 samples of trees in the diagnostic phase (burnin = 750) were discarded. The rest of the trees were used to infer the further probabilities of the individual clades. In order to assess the convergence of the model, we ran several parallel chains, keeping the standard deviation from the result to a value below 0.01 (Ronquist et al., 2005). For that, we tested the software parameters, resulting in the following: number of generations: 3000000; temperature of chains: above 8°C.
Expression Profiling Analyses of DGK Genes from Genevestigator
Tissue-specific expression patterns of available plant DGK gene probes were retrieved from the Genevestigator software package5 (Zimmermann et al., 2014). Furthermore, we were able to retrieve the expression profiling of Arabidopsis (AtDGK), rice (OsDGK), tomato (SlDGK), soybean (GmDGK), wheat (TaDGK) and barley (HvDGK) DGK genes in response to Ag, Al, As, Cd, Cr, Hg, and Na from Genevestigator as well.
Results
Phylogenetic Analysis of DGK Enzymes in Higher Plants
In order to reconstruct the DGK phylogeny from plants, a protein database search in the NCBI and UniProtKB was carried out. The queries were DGK sequences homolog to Arabidopsis DGKs using the BLAST tool. Subsequently, we added to our analysis all homolog DGK sequences previously analyzed phylogenetically and referred to in articles. From Arabidopsis, we took into consideration AtDGK1, AtDGK2, AtDGK3, AtDGK4, AtDGK5a, AtDGK5b, AtDGK6, and AtDGK7 (Gómez-Merino et al., 2004, 2005a); from rice, OsDGK1, OsDGK2, OsDGK3, OsDGK4, OsDGK5, OsDGK6, OsDGK7, and OsDGK8 (Ge et al., 2012); from maize, ZmDGK1, ZmDGK2, and ZmDGK3 (Sui et al., 2008); from wheat, TaDGK (Zhang et al., 2008); from tomato, SlDGK1a-b (Snedden and Blumwald, 2000); and from apple, MdGK1, MdDGK2, MdDGK5, and MdDGK7 (Li et al., 2015). Finally, each sequence was used as reference to perform the following searches of all DGK isoforms present in most crop plant proteomes, with a wider percentage of coverage.
After having completed the searches (47 DGK isoforms found in 22 plant species; Table 1), we aligned the sequences through Clustal-omega1.2.1 software (Sievers et al., 2011), using three iterations in the procedure to gain precision in the alignment. In our study, we performed a preliminary alignment of plant DGK against the Saccharomyces cerevisiae Dgk1p (renamed ScDGK1 in our analysis) sequence and demonstrated that the ATP-binding site is conserved in all DGK catalytic domains in plants. Conversely, the consensus sequence in ScDGK1 has been replaced by the sequence H58LKSHE63 in the catalytic CTP domain. This catalytic domain exhibits a much simpler and less varied amino-terminal regulatory domain than its ATP-dependent counterpart (Xie et al., 2015). Importantly, ScDGK1 is a unique CTP-dependent nuclear/endoplasmic reticulum membrane-associated enzyme that catalyzes the formation of PA from DAG in yeast (Fakas et al., 2011).
The sequence alignment results were used as input for the construction of the phylogenetic tree, using MrBayes3.2.5 software (Altekar et al., 2004) to perform it. To have an evolutionary perspective of the DGK phylogeny, we added PLC proteins as an external group in our analysis (Supplementary Material 1). Figure 2 shows the Bayesian phylogenetic tree of our results.
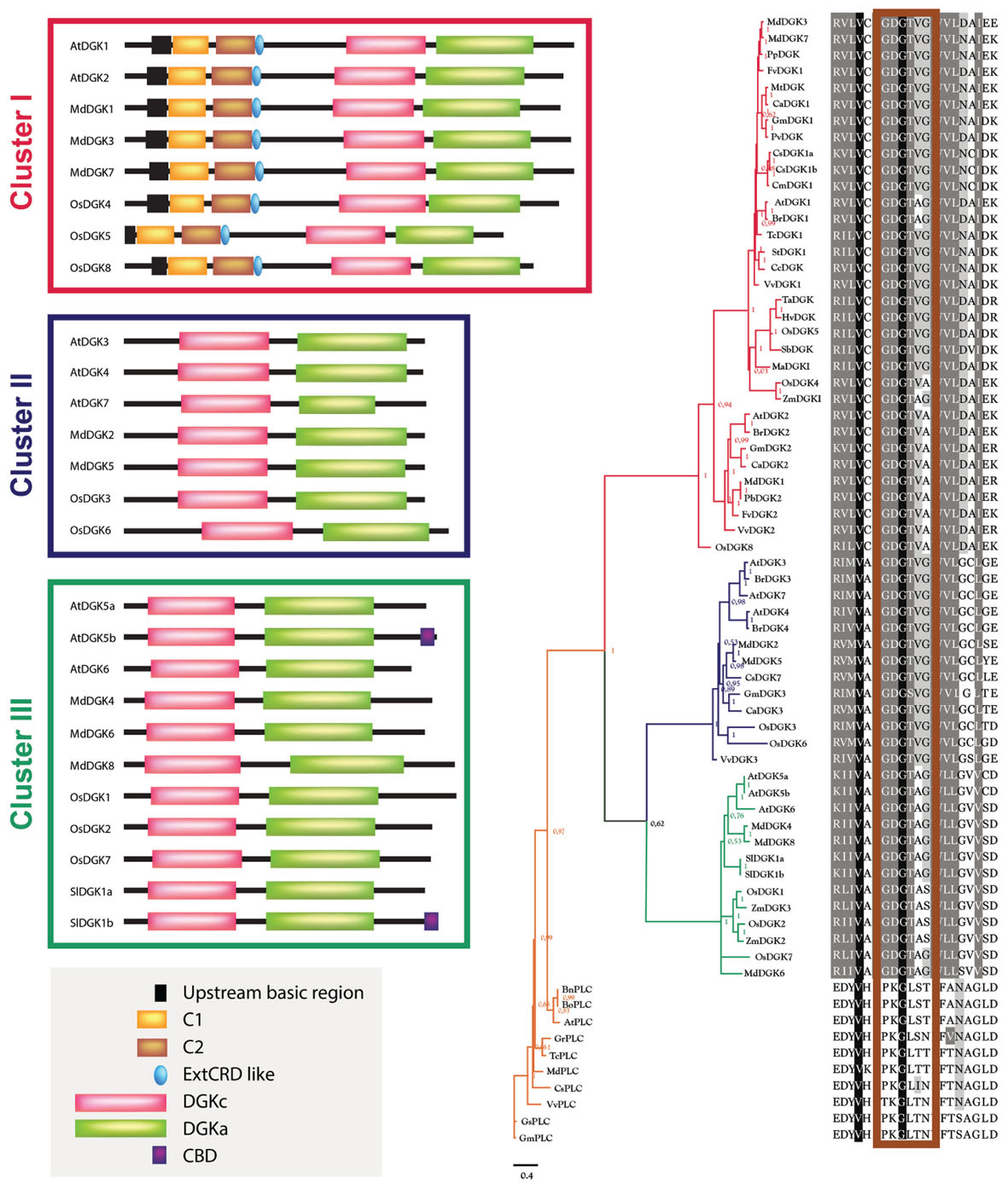
FIGURE 2. Phylogenetic analysis of DGK and PLC enzymes from higher plants. The phylogenetic analysis was built using the evolutive WAG method with variations described using a gamma-type distribution (rates = invgamma), default hyperparameters and three million generations (ngen = 3000000) in MrBayes3.2.5 software (Altekar et al., 2004). The subsequent probability values are indicated in the base of each clade. The sequence composition regarding the catalytic domain in each taxon in the multiple alignment is indicated in the right. The corresponding alignment was performed using Clustal-Omega1.2.1 software (Sievers et al., 2011), which uses HMM and a tree guide, constructed by the UPGMA method and a substitution matrix. This algorithm was iterated three times in order to avoid spurious results. The resulting clusters have been colored: red, Cluster I; blue, Cluster II; green, Cluster III; orange, External Group (PLCs). On the left of each DGK cluster, the domain distribution of some representative members is included and its structural composition is according to the studies carried out in tomato (Snedden and Blumwald, 2000), Arabidopsis (Gómez-Merino et al., 2004, 2005a; Vaultier et al., 2008; Arisz et al., 2009), rice (Ge et al., 2012), and apple (Li et al., 2015). The phylogenetic tree was visualized using the FigTree1.4.2 program (http://tree.bio.ed.ac.uk/). In the right of the tree, the ATP-binding site is indicated in the rectangle depicted in maroon over the alignment. The multiple sequence alignment result was visualized with GeneDoc 2.7 (Nicholas and Nicholas, 1997). The gray box in the bottom left corner includes the following domains: an upstream basic region; one or two DAG-binding domains (C1 and C2); an extended cysteine-rich domain (extCRD); a diacylglycerol kinase accessory domain (DGKa); a diacylglycerol kinase catalytic domain (DGKc); and calmodulin-binding domain (CBD).
In Figure 2, the isoforms AtDGK1, AtDGK2, BrDGK1, BrDGK2, CaDGK1, CaDGK2, CcDGK, CsDGK1a, CsDGK1b, CmDGK1, FvDGK1, FvDGK2, GmDGK1, GmDGK2, HvDGK, MaDGK1, MdDGK3, MdDGK7, MtDGK, PvDGK, TcDGK1, StDGK1, TaDGK, OsDGK5, PpDGK, SbDGK, MdDGK1, OsDGK4, OsDGK8, PbDGK2, VvDGK1, VvDGK2, and ZmDGK1 encompass Cluster I; Cluster II consists of the isoforms AtDGK3, AtDGK4, AtDGK7, BrDGK3, BrDGK4, MdDGK2, MdDGK5, CsDGK7, GmDGK3, CaDGK3, OsDGK3, OsDGK6, and VvDGK3; and the isoforms AtDGK5a, AtDGK5b, AtDGK6, MdDGK4, MdDGK6, MdDGK8, SlDGK1a, SlDGK1b, OsDG1, OsDGK2, OsDGK7, ZmDGK2, and ZmDGK3 comprise Cluster III. The sequences BnPLC, BoPLC, AtPLC, GrPLC, TcPLC, MdPLC, CsPLC, VvPLC, GsPLC, and GmPLC belong to an external group of the phylogenetic tree. Considering this external PLC group, the first event of diversification occurred between DGKs of Cluster I and the rest of the sequences. After that, the second diversification event took place between proteins included in Clusters I and II.
In order to test whether our phylogenetic analysis was consistent with those previously reported, we constructed an additional rooted tree (Figure 3). Our results confirm that plant DGKs fall into three phylogenetic clusters, which is in full agreement with other studies reported by Gómez-Merino et al. (2004), Zhang et al. (2008), Ge et al. (2012), and Li et al. (2015). This second phylogenetic tree is also consistent with that presented in Figure 2.
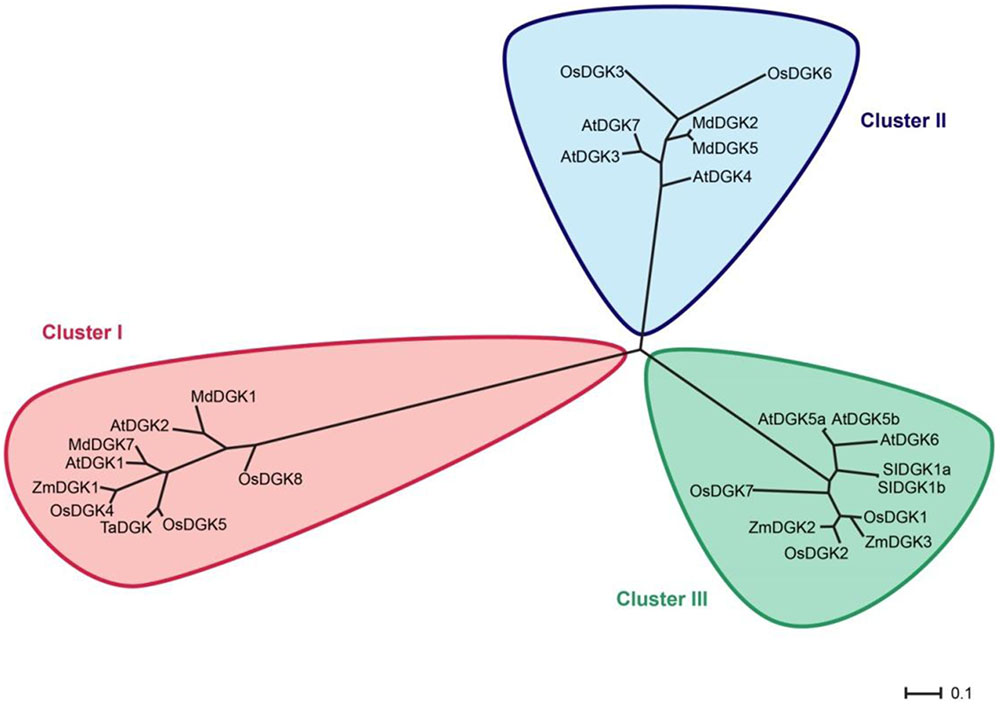
FIGURE 3. Rooted phylogenetic tree of plant DGK isoforms. For this analysis we used only DGK isoforms that had been reported in previous studies. In red, Cluster I; in blue, Cluster II; in green, Cluster III. The phylogenetic tree was visualized using the FigTree1.4.2 program (http://tree.bio.ed.ac.uk/). Protein names are according to Table 1.
Expression Profiling Analysis of DGK Genes in Different Plant Tissues
Genevestigator6 represents a high-performance bioinformatics search tool for gene expression analyses. It integrates a plethora of manually curated, well-described public experiments and accurately displays gene expression in response to diverse environmental contexts. We took advantage of this tool and analyzed transcriptional expression of DGKs genes in different tissues when available (Figure 4). Importantly, we were able to retrieve crucial expression data of DGK genes when Arabidopsis, barley, soybean, tomato, rice, or wheat plants were exposed to Ag, Al, As, Cd, Cr, Hg, or Na.
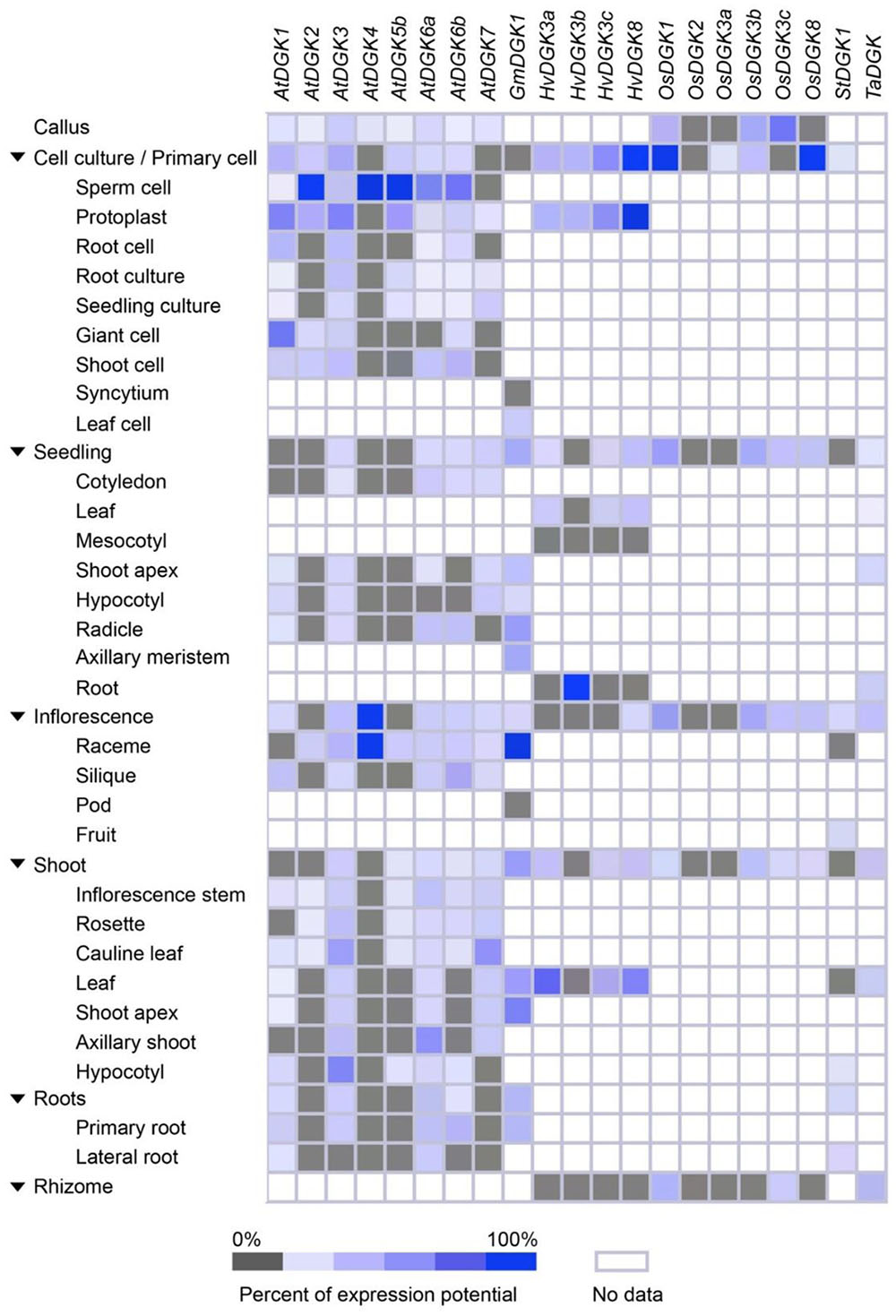
FIGURE 4. Expression patterns of selected plant DGK genes, based on plant tissue types. Tissue-specific expression in callus, cell culture, seedling, inflorescence, shoot, roots, and rhizome were retrieved from Genevestigator (https://genevestigator.com/gv/). Mean values were obtained from at least three replicates. Colors represent the intensity of expression (percentage of expression potential), from gray (0%) to dark blue (100%). Empty (colorless) boxes indicate that no data could be retrieved from the platform.
We found that DGK genes were overexpressed during cell culture, seedling, and inflorescent stages. At cell culture, induced expression of AtDGK2, AtDGK4, and AtDGK5b was observed in sperm cell; HvDGK8 in protoplast; OsDGK1 and OsDGK8 in the whole cell culture and primary cell. In seedlings the gene HvDGK3c was found to be induced in roots. During inflorescence, the genes AtDGK4 and GmDGK1 were overexpressed in raceme. In general, all genes analyzed were expressed in all tissues, though at different levels. This is consistent with the expression data reported by Gómez-Merino et al. (2004), especially concerning AtDGK2, since such gene was found to be highly expressed in young tissues and flowers. In a study performed in reproductive organs of Arabidopsis, the published microarray data revealed that AtDGK1, AtDGK3, and AtDGK5 are primarily expressed in pistils, stamens, and petals, while AtDGK4 is highly expressed only in stamens. Instead, AtDGK5 is slightly higher expressed in stamens and petals than in pistils (Yunus et al., 2015). Their qRT-PCR analyses showed that the expression of AtDGK7 was the highest among DGK isoforms, while AtDGK4 and AtDGK6 were the lowest. Accordingly, Gómez-Merino et al. (2004) and Arana-Ceballos (2006) reported that the AtDGK2 and AtDGK7 genes, respectively, were indeed expressed in flower tissues. Moreover, six apple DGK genes (DGK1, DGK2, DGK4, DGK5, DGK7, and DGK8) were found to be highly expressed in stems and most of them in the flower as a whole (Li et al., 2015), which is consistent with the results retrieved from Genevestigator.
All together, these findings demonstrate that eight DGK gene probes from Arabidopsis, one from soybean, four from barley, six from rice, one from tomato, and one from wheat, displayed ubiquitous expression in most tissues analyzed. In general, all genes showed high expression in the young and reproductive tissues, pointing to a role of DGK and its enzymatic product, PA, in development and functions of floral organs.
Expression Profiling Analysis of DGK Genes in Response to Beneficial Elements and Other Ions
We explored the gene expression profiling data deposited in the Genevestigator platform6 and found that various DGK genes are differentially regulated by Ag, Al, As, Cd, Cr, Hg, and Na (Figure 5).
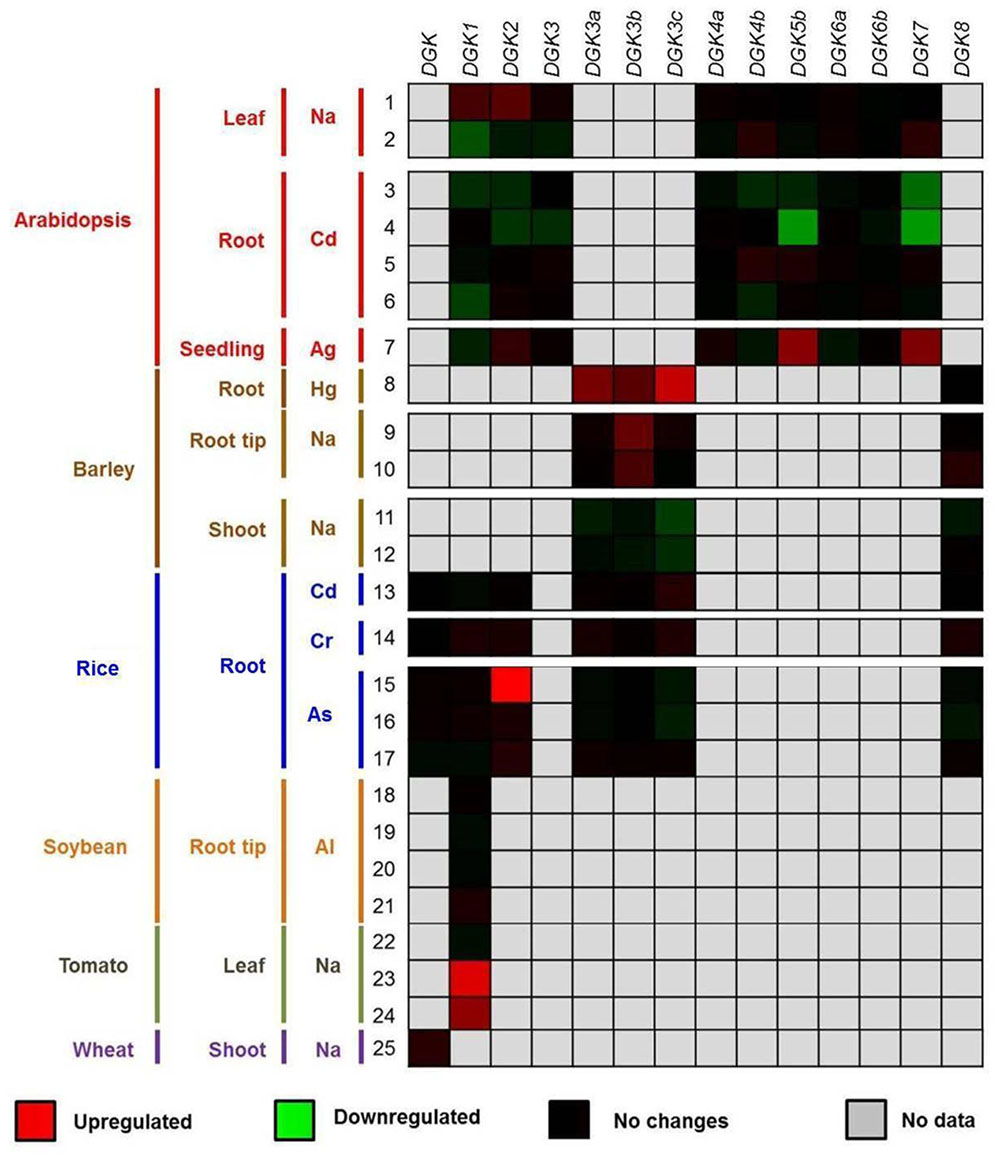
FIGURE 5. Expression profiling data of DGK genes in response to beneficial elements, metal, and metalloid ions. The up- or downregulation of the expression levels of DGK genes in the presence of Ag, Al, As, Cd, Cr, Hg, and Na are represented through red and green saturation colors, respectively. Experiment details are described in Supplementary Materials 2 and 3, as well as in Datasheet 1, while gene probe analyses are listed in Supplementary Material 4. Data were retrieved from the Genevestigator platform (https://genevestigator.com/gv/; Zimmermann et al., 2014). The experiments (1–25) were conducted in the presence of low concentrations of chemical elements. 1–7: Na (1–2), Cd (3–6), and Ag (7) in leaves (1–2), roots (3–6) and seedlings (7) of Arabidopsis; 8–12: Ag (7), Hg (8), and Na (9–12) in roots (8), root tips (9–10), and shoots (11–12) of barley; 13–17: Cd (13), Cr (14), and As (15–17) in rice roots; 18–21: Al in soybean root tips; 22–24: Na in tomato leaves; 25: Na in wheat shoots. In all cases, control experiments (where a determined element was not applied) were run in parallel.
Beneficial elements are not essential in most plant species. Instead, they may elicit hormetic responses by stimulatory effects when applied at low concentrations. Although up to now still largely unexplained, the plant growth enhancing effects of beneficial elements is gaining increased attention in relation to enhancing crop production (Belz and Cedergreen, 2010; Calabrese, 2014). In order to attain deeper knowledge on the effects of such elements on plant biology, we explored the transcript profiling data of DGK genes available in Genevestigator.
We have previously observed that AtDGK2 transcript levels are induced in response to Al, one of the beneficial elements referred to in the literature (Blamey et al., 2015; Gómez-Merino et al., 2005b). Herein we report that some other plant DGK genes are indeed activated by beneficial elements and other ions (Figure 5). When barley plants were exposed to ∼1 μM Hg the expression of HvDGK3a, HvDGK3b, and HvDGK3c was significantly induced in root tissues. Similarly, in Arabidopsis roots the genes AtDGK5b and AtDGK7 were highly transcribed in the presence of 10 μM Ag. In rice, plants exposed to 13.3 μM dibasic sodium arsenate (NaHAsO4) exhibited induced expression of OsDGK2 in leaves, whereas in tomato the application of 200 mM Na enhanced the expression of SlDGK1 also in leaves. Conversely, lower expression levels were detected in shoots, especially those of AtDGK5b and AtDGK7 in response to 200 μM Cd.
Discussion
Plants have evolved effective response strategies to react against environmental stimuli and protect themselves from various stress factors. One of the most important signaling pathways that mediate these responses is represented by the PPI, in which one of the emerging components is PA (Arisz et al., 2013; Saucedo-García et al., 2015). In the plasma membrane, PA can be produced principally by the PLD and PLC/DGK pathways. Interestingly, most of the PA synthetized in plants stimulated over a prolonged period is through the PLD pathway (Testerink and Munnik, 2011). Instead, a rapid PA accumulation in response to low temperature stress is generated through the PLC/DGK pathway (Arisz et al., 2013).
DGK activity has been reported in several plant species, including Catharanthus roseus, tobacco (Nicotiana tabacum), wheat, tomato, Arabidopsis, rice, and apple (Gómez-Merino et al., 2005a; Zhang et al., 2008; Li et al., 2015), and the molecular data bases reveal that they are present in a number of other crop plants such as maize, grape, sweet orange, and cotton. Nonetheless, detailed analysis of transcriptional activation of DGK genes and functional analysis of their corresponding protein products are still fragmentary. Two DGK cDNAs, LeDGK1 and LeCBDGK, have been cloned from tomato and found to be derived from the same gene via alternative splicing. LeCBDGK, the protein product of LeCBDGK, contains a calmodulin (CaM)-binding domain (CBD). The two proteins lack the cysteine-rich domain (CRD) present in other eukaryotic DGKs, but are active in vitro. LeCBDGK (SlDGK1b in Table 1) is found both in associations with membranes and in soluble cell extracts. By contrast, LeDGK1 (SlDGK1a in Table 1) only associates with the membrane fraction, via a Ca2+/CaM-independent mechanism, which might represent a means of encoding specificity in cellular responses by alternative splicing (Snedden and Blumwald, 2000). In Arabidopsis, the AtDGK1 cDNA has been isolated and reported to be mainly expressed in roots, shoots, and leaves, but its enzyme product was not active in vitro (Katagiri et al., 2001). However, Vaultier et al. (2008) found DGK activity in most membrane compartments, and speculated that AtDGK1 and AtDGK2 contribute to this activity. Two Arabidopsis DGK cDNAs (AtDGK2 and AtDGK7) were cloned and their encoded enzymes were catalytically active in in vitro assays. AtDGK2 transcripts are found in the whole plant except in stems and are induced by exposure to cold (4°C), pointing to a role in cold signal transduction (Gómez-Merino et al., 2004), whereas the AtDGK7 gene is mainly found in flowers, young seedlings, and cauline leaves (Gómez-Merino et al., 2005a). In rice, it has been reported that the overexpression of the DGK gene OsBIDK1 enhances disease resistance in transgenic tobacco (Zhang et al., 2008). Importantly, the inhibition of DGK activity drastically reduces root elongation and plant growth (Gómez-Merino et al., 2005a), indicating that DGKs may play a pivotal role not only in stress responses but also in developmental processes in plants. Whether this inhibition impact on plant development is related to an effect on the nutrient status of the plants remains to be elucidated. Nonetheless, with the highest DGK inhibitor dose used, plants exhibited a general chlorosis and died earlier as the inhibitor concentrations increased in the growth medium, which indeed points to a role of DGK enzymatic activity on nutrient status of the plant (Gómez-Merino et al., 2005a).
In terms of plant nutrition, essential elements are classified into macronutrients and micronutrients, according to the concentrations in which they are found in plant tissues. Apart from C, N, and O, the first group encompasses N, P, K, Ca, Mg, and S, which are found in plant tissues in concentrations of approximately 1000 mg kg-1 dry biomass weight. Instead, plant micronutrients, which are represented by Cl, Cu, Fe, Mg, Mo, Ni, and Zn, are found in concentrations close to 500 mg kg-1 of biomass in dry bases (Alcántar-González et al., 2016). Beneficial elements are not essential for plants, but when applied at low dosages, they may improve yield and quality parameters of some plant species of agricultural importance (Pilon-Smits et al., 2009; Trejo-Téllez et al., 2016). To date, the proposed beneficial elements include Al, Ce, Co, Fl, La, Na, Se, Si, Ti, V, and W (Pilon-Smits et al., 2009; Poschenrieder et al., 2013; Trejo-Téllez et al., 2016). Furthermore, other chemical elements such as Ag, As, Cd, and Hg may trigger hormetic effects in plants. Herewith we demonstrated that some DGK genes are induced in response to Ag, Al, Cd, Cr, Hg, and Na, suggesting a possible role of these genes and their protein products on plant nutrition.
We implemented an evolutive method in order to construct a consensus phylogenetic tree of DGKs from higher plants. In doing so, we took into consideration all DGK sequences in which enzymatic activity had been previously demonstrated, or that had been considered in other phylogenetic analyses. We found that plant DGK enzymes were distributed into three major clusters, as previously reported for Arabidopsis, rice, maize, wheat, tomato, and apple (Snedden and Blumwald, 2000; Gómez-Merino et al., 2004; Sui et al., 2008; Zhang et al., 2008; Li et al., 2015).
So far, all plant DGKs exhibit a bipartite catalytic region composed of a catalytic domain (DGKc; Pfam accession number PF00781) followed by an accessory domain (DGKa; Pfam accession number PF00609) at the C-terminus. Since DGKa is associated with DGKc, the former may contribute to the functionality of the catalytic domain. Whereas mammalian DGK enzymes can be classified into five groups according to sequence homology (Topham and Prescott, 2010), plant DGKs only form three phylogenetic clusters, and exhibit a simpler domain organization. Though less varied and complex, plant DGKs included in Cluster I resemble the closest sequence orthologous of metazoan enzymes with proteins that display one or two DAG-binding domains (C1), an upstream basic region and an extended CRD in their N-terminus (Gómez-Merino et al., 2004; Vaultier et al., 2008; Cacas et al., 2016). Conversely, Cluster II DGKs just harbor the DGKa and DGKc domains. Interestingly, Cluster III DGKs may exhibit a C-terminal CBD generated by alternative splicing (Arisz et al., 2009; Cacas et al., 2016).
The yeast S. cerevisiae was initially thought to lack a DGK, though Dgk1p, a novel type of DGK, utilizes CTP rather than the common ATP was discovered (Han et al., 2008a). This enzyme does not exhibit sequence similarity to DAG kinases from other species (Han et al., 2008b), and contains a short motif identified in a family of CTP-dependent phytol and dolichol kinases (Shridas and Waechter, 2006). Together with PA phosphatase, this enzyme controls the levels of PA and DAG for phospholipid synthesis, membrane growth, and lipid droplet formation (Qiu et al., 2013). The fact that the yeast DGK enzyme utilizes CTP, instead of ATP, as the phosphate donor in the reaction, explains why an ATP-dependent DAG kinase activity or a putative gene encoding a DAG kinase enzyme had not been identified in S. cerevisiae before (Han et al., 2008a).
Both experimental data previously reported and microarray analyses revealed that some DGK genes are expressed more prominently in young tissues, whereas their expression declines in older tissues. Importantly, most DGK transcripts are detectable in all tissues throughout all developmental stages, but their abundance decreases in tissues when plants become older.
Expression of DGK genes in response to both biotic and abiotic stimuli and stress factors has been proved (Gómez-Merino et al., 2004; Arisz et al., 2009; Pleskot et al., 2012; Ruelland et al., 2015). Accordingly, our survey revealed that some DGK genes are indeed regulated by Ag, Al, As, Cd, Cr, Hg, and Na, which may display hormetic dose–response curves.
According to Pilon-Smits et al. (2009), Ag can be considered as a potentially beneficial element, while information on the cellular basis for the positive effects of this element in plants is still fragmentary. Currently, silver nanoparticles (AgNPs) have remarkable uses in agricultural and environmental systems. Sharma et al. (2009) have pointed to a crucial role of AgNPs as antimicrobial agents. In plants, AgNPs impact oxidative stress-related gene expression, seed germination, and root elongation, leading to both positive and negative effects on plant growth (Cox et al., 2016). The final effects of AgNPs depend on nanoparticle (NP) size, shape, surface coating, and concentration used. Likewise, plant genotypes differ in their responses to NP exposure. In our survey, when Ag was applied to Arabidopsis plants, inducible gene expression was strongly evident for AtDGK5b and AtDGK7. Indeed, AgNPs have been implicated in signal transduction pathways involving kinases within the PI signaling pathway in eukaryotic cells (Kang et al., 2012), which suggests that Ag might influence other components of this pathway, including PLC and DGK in plants. This topic will be an interesting area for future study.
In the case of Al, Gómez-Merino et al. (2005b) reported induction of AtDGK2 gene expression in hydroponically growing Arabidopsis plants exposed to 200 μM Al in the nutrient solution. In this study, Al induced the expression of the DGK gene rapidly, within 30 min after exposure, and it returned to the pretreated level 20 h after treatment (Gómez-Merino et al., 2005b). Likewise, Martínez-Estévez et al. (2001b) reported that Al entered coffee cells 30 min after treatment, and the signal was retained for up to 2 h. Furthermore, it was found that Al increases phosphorylation of particular proteins in cellular suspension cultures of coffee (Martínez-Estévez et al., 2001a). Subsequently, it was demonstrated that Al quickly (in 1 min) increased enzymatic activity of PLC as well as PI4K, PIP5K, and DGK (Martínez-Estévez et al., 2003), supporting the hypothesis that DGK genes might be implicated in the production of PA in response to Al. Nevertheless, this issue awaits further research at the molecular level. Just recently, Bojórquez-Quintal et al. (2014) reported that Al effects on primary root growth in coffee plants were dose-dependent: 100–300 μM Al stimulated primary root growth, while 500 μM induced damage to the root tips and inhibited primary root growth. Furthermore, 100 μM Al also increased the K and Ca contents by around 33 and 35% in roots, as compared to the control, which demonstrates the beneficial effects of Al when applied at low concentrations. Indeed, Al may improve nutrient status in the Al-resistant wheat cultivar Yecora, since this genotype retained larger concentrations of Ca2+ and Mg2+ in the leaves (Moustaka et al., 2016). Nonetheless, we have to keep in mind that Al exhibits hormetic effects in plants, which means that low dosages may induce beneficial responses in some genotypes, and higher concentrations are likely to cause toxicity in most plant species, interfering with cytoskeleton structure and function, disrupting Ca homeostasis, hampering P metabolism, and inducing oxidative stress (Blamey et al., 2015). In our survey, only the soybean GmDGK1 gene was tested, showing no significant changes upon Al exposure. Whether other DGK genes are indeed induced by Al is still an open question that remains to be answered in future plant signaling studies.
Arsenic (As) is a metalloid occurring in natural environments in some abundance (1.2–1.4 ppm) in the Earth’s crust and in small quantities in rocks, soils, water bodies, and air. Hence, plants have evolved in the presence of this element, and it has been postulated that As might induce hormetic effects on plant growth. Although the mechanism of action is still unknown, it has been suggested that the growth benefit triggered by As arises from As stimulation of Pi uptake (Tu and Ma, 2003; Finnegan and Chen, 2012). However, because of its general toxic effects in living organisms, As is of considerable concern, and special care has to be taken in order to take advantage of its potential use in agriculture. In our study, As (in the form NaHAsO4) induced the expression of rice OsDGK2, but slightly downregulated that of OsDGK3a and OsDGK8. This fact, as well, points to a possible role of As in the PI signaling pathway.
In some plant species, Cd has been proved to trigger beneficial protective effects at low doses, in an hormetic dose–response manner (Liu et al., 2015). In fact, hormesis was found during the growth of switchgrass (Panicum virgatum) under hydroponic conditions, since plants were capable of developing better in the nutrient solution containing 100–175 μM Cd at pH 4.1–5.9, showing enlargement of the root length and surface, enhanced absorption of essential nutrients and increased biomass (Wang et al., 2015). Similarly, Liu et al. (2015) reported higher concentrations of chlorophylls (a, b, and total) and carotenoids, as well as relative water contents in leaves of Lonicera japonica plants receiving 0.5–5.0 mg L-1 Cd in the nutrient solution, as compared to the control. In our analysis, Cd repressed the transcriptional activity of AtDGK1, AtDGK2, AtDGK3, AtDGK4b, AtDGK5b, and AtDGK7. In rice, Cd slightly enhanced the expression of OsDGK8. It has been previously demonstrated that Cd affects the content of PPI in mammalian cells (Borikov and Kaliman, 1999). Just recently, Rajakumar et al. (2016) reported that Cd disrupts lipid metabolism in S. cerevisiae. As a hormetic factor, Cd is also a hazardous metal for plants when certain threshold concentrations in their cells are exceeded (Trejo-Téllez et al., 2014), as a result of its great toxicity inducing oxidative stress, genotoxicity, disruption of the photosynthetic apparatus, and inhibition of root metabolism (López-Millán et al., 2009; Han et al., 2012; Andresen and Küpper, 2013; Liu et al., 2015). Whether Cd interferes with the PI signaling pathway, and especially with the activity of PLC and DGK, is a question worthy of further study.
Cr has been generally referred to as an essential element for animals (Thorvaldsson and Jónsdóttir, 2005). In fact, Ma and Hooda (2010) established that trivalent chromium Cr(III) is an essential/beneficial nutrient that in trace amounts regulates the sugar and cholesterol metabolism in human and animal cells, though its hexavalent form Cr(VI) is a potent carcinogen and extremely toxic for those biological systems. Importantly, a recent report by the European Food Safety Association’s Panel on Dietetic Products, Nutrition and Allergies (EFSA NDA Panel, 2014) determined that there is no evidence of beneficial effects associated with Cr intake in human health, and setting of an adequate Cr intake level is also not appropriate. Concerning the plant system, though its abundance in the Earth’s crust ranges from 100 to 350 ppm (which is higher than that of essential elements like Ni, Zn, and Cu), Cr displays low solubility in soils and plants absorb just small amounts of this element. Hence, normal concentrations of Cr in plant tissues are between 0.02 and 1.0 mg kg-1, which depends on soil concentrations of this element and plant species (Ma and Hooda, 2010). Similar to humans and animals, Cr(VI) is much more toxic to plants than Cr(III). Just recently, Kabir (2016) reported that Cr stress tolerance in rice cv. Pokkali is not related to metal sequestration but is associated with reduced Fe transport and increased antioxidant defense. As an hormetic element, González et al. (2015) reported that low dosages of Cr (i.e., 2 and 4 mg L-1) enhanced defense response and maintained photosynthetic activity in Eichhornia crassipes, implying that the antioxidant defense system enzymes attempted to ensure the redox homeostasis. In our analysis, Cr slightly induced the expression of most OsDGK isoforms. Bovykin et al. (1999) found that Cr(III) ions are adsorbed on the bilayer lipid membrane surface, changing the intramembrane potential difference. As pH increases, the adsorption of those ions decreases. Furthermore, McCarty (2006) reported that bioactive Cr can induce the plasmalemmal Ca2+-ATPase in mammals, which is connected with the regulation of phospholipid levels (Govindaraju et al., 1989). In fact, it has been well documented that Cr alters phospholipid metabolism in animals (Tandon, 1982), but its implications on this pathway in plants is still at issue.
Low levels of Hg salts induce stress-dependent increase in glucose uptake in mammalian cells (Stearns, 2007). Furthermore, the antimicrobial properties of Hg are well documented (Lemire et al., 2013), though its use for agricultural proposes has been restricted since the 1990s (EPA, 2002). Hormetic effects of Hg have been proved in different organisms, including mammals (Schmidt et al., 2004), Caenorhabditis elegans (Helmcke and Aschner, 2010), Anas platyrhynchos (Heinz et al., 2012), and plants. In Lemna minor and Allium cepa, Subhadra et al. (1991) reported that low levels of aquatic Hg (0.001–1.0 mg L-1) accelerated catalase and peroxidase activities. In our survey, Hg application in barley resulted in a strong induction of all three HvDGK3 genes (a, b, and c). Nevertheless, additional experimental evidence demonstrating the connection between Hg and the PI signaling pathway remains to be elucidated.
Na has been proved to be an essential element for halophytes (i.e., salt-tolerant plants), including C4 or CAM species that use phosphoenolpyruvate (PEP) to fix CO2 during photosynthesis, since Na mediates the regeneration of PEP from pyruvate. Additionally, Na has been widely reported to trigger hormetic responses on most plant taxa, including C3 plants (Maathuis, 2013). Importantly, in hydrated form, Na+ and K+ are chemically and structurally very similar. Consequently, in environments with low K+ conditions, Na+ can be useful for plants. Hence, several functions carried out by K+ plants, including some of the physiological and metabolic ones (i.e., osmotic regulation, guard cell movement, and cell expansion), can be fulfilled by Na+. Nevertheless, plant species significantly vary in their capacity to replace K+ with Na+ (Pilon-Smits et al., 2009). Na is also an excellent accompanying cation for long-distance transport (Subbarao et al., 2003). In our survey, we could observe that Na slightly induced the expression of AtDGK1 and AtDGK2 in shoots. On the other hand, the activity of both genes was slightly repressed in roots. In wheat, Na hardly induced the expression of TaDGK in shoots, while it differentially repressed its expression in roots, depending on the time of exposure and the dosage used. In barley, Na induced the expression of HvDGK3b, but repressed that of HvDGK3c. In tomato, Na strongly induced transcriptional activity of SlDGK1. Interestingly, Peters et al. (2002) reported that the non-specific phospholipase C5 (NPC5) and its derived lipid mediator DAG regulate lateral root development induced by sodium chloride (NaCl) in Arabidopsis thaliana. Moreover, another non-specific phospholipase C, NPC4, promotes responses to abscisic acid and tolerance to hyperosmotic stress induced by NaCl in Arabidopsis (Peters et al., 2010). These findings suggest a connection between Na and the PLC/DGK pathway in plants, which has to be further investigated.
Beneficial elements are emerging as potential biostimulators for agricultural proposes, since they can induce desirable plant responses in a hormetic manner. In light of the growing need for sustainable agriculture, the general global challenges related to climate change and an increasing population demanding more food, beneficial elements open up new possibilities to attain food security. Though cumulative evidence points to beneficial elements like Al and Na having a role in the regulation of DGK gene expression, the possible impact of dosages, chemical forms, time of application, and genotype responses remains to be further investigated. Additionally, understanding the roles of beneficial elements and other hormesis-inducing ions in different plant species under stress conditions may be a possible area of further study. Herewith we provide evidence for the first time that DGK genes encoding enzymes implicated in the synthesis of PA, an emerging second messenger, are responsive to Ag, Al, As, Cd, Cr, Hg, and Na. Importantly, we found most DGK genes ubiquitously expressed in plant tissues, and encoded proteins widely distributed in higher plants. PA, the protein product of DGK activity, has been proved to be a crucial second messenger. Its potential role as signaling mediator in response to beneficial elements and other ions could be of crucial significance for plants, providing the cell with spatial and transient information to perform better under limiting environment conditions, or simply to improve plant production and productivity. The discovery and description of the molecular bases of such relationships would be a noteworthy contribution to the field of agriculture.
Author Contributions
HE-S and FG-M developed and designed the experiments and the survey strategies. LT-T supervised the physiological and plant nutrition experiments. PP-R and JH-C supervised the bioinformatics analyses. HE-S and FG-M wrote the manuscript. LT-T, PP-R, and JH-C revised the manuscript.
Funding
The Secretariat for Foreign Affairs (SRE) of Mexico granted a M.Sc. scholarship to HE-S.
Conflict of Interest Statement
The authors declare that the research was conducted in the absence of any commercial or financial relationships that could be construed as a potential conflict of interest.
Acknowledgments
We are especially thankful to Dr. Diego Mauricio Riaño-Pachón (CTBE-Brazil) for the advice and technical support given to improve this study. We also acknowledge the infrastructure facilities and financial support provided by the Córdoba and Montecillo campuses of the Colegio de Postgraduados.
Supplementary Material
The Supplementary Material for this article can be found online at: http://journal.frontiersin.org/article/10.3389/fpls.2017.00129/full#supplementary-material
Footnotes
- ^www.ncbi.nih.gov/blast
- ^www.ncbi.nih.gov
- ^http://www.uniprot.org/
- ^http://mrbayes.sourceforge.net
- ^https://genevestigator.com/gv/index.jsp
- ^https://genevestigator.com/gv/
References
Abd-El-Haliem, A., Vossen, J., van Zeijil, A., Dezhsetan, S., Testerink, C., Seidl, M., et al. (2016). Biochemical characterization of the tomato phosphatidylinositol-specific phospholipase C (PI-PLC) family and its role in plant immunity. Biochim. Biophys. Acta 1861(9 Pt. B), 1365–1378. doi: 10.1016/j.bbalip.2016.01.017
Alcántar-González, G., Trejo-Téllez, L. I., Fernández-Pavía, L., and Rodríguez-Mendoza, M. N. (2016). “Elementos esenciales,” in Nutrición de Cultivos. Segunda Edición, eds G. Alcántar-González, L. I. Trejo-Téllez, and F. C. G. ómez-Merino (Montecillo: Ed. Colegio de Postgraduados), 23–55.
Altekar, G., Dwarkadas, S., Huelsenbeck, J., and Ronquist, F. (2004). Parallel metropolis coupled Markov chain Monte Carlo for Bayesian phylogenetic inference. Bioinformatics 20, 407–415. doi: 10.1093/bioinformatics/btg427
Altschul, S. F., Gish, W., Miller, W., Myers, E. W., and Lipman, D. J. (1990). Basic local alignment search tool. J. Mol. Biol. 215, 403–410. doi: 10.1016/S0022-2836(05)80360-2
Andresen, E., and Küpper, H. (2013). Cadmium toxicity in plants. Metal Ions Life Sci. 11, 395–413. doi: 10.1007/978-94-007-5179-8_13
Arana-Ceballos, F. A. (2006). Biochemical and Physiological Studies of Arabidopsis thaliana Diacylglycerol Kinase 7 (AtDGK7). Ph.D. Thesis. University of Potsdam, Potsdam.
Arisz, S., and Munnik, T. (2011). The salt stress-induced LPA response in Chlamydomonas is produced via PLA 2 hydrolysis of DGK-generated phosphatidic acid. J. Lipid Res. 52, 2012–2020. doi: 10.1194/jlr.M016873
Arisz, S. A., Testerink, C., and Munnik, T. (2009). Plant PA signaling via diacylglycerol kinase. Biochim. Biophys. Acta 1791, 869–875. doi: 10.1016/j.bbalip.2009.04.006
Arisz, S. A., van Himbergen, J. A. J., Musgrave, A., van den Ende, H., and Munnik, T. (2000). Polar glycerolipids of Chlamydomonas moewusii. Phytochemistry 53, 265–270. doi: 10.1016/S0031-9422(99)00505-1
Arisz, S. A., van Wijk, R., Roels, W., Zhu, J., Haring, M. A., and Munnik, T. (2013). Rapid phosphatidic acid accumulation in response to low temperature stress in Arabidopsis is generated through diacylglycerol kinase. Front. Plant Sci. 4:1. doi: 10.3389/fpls.2013.00001
Belz, R. G., and Cedergreen, N. (2010). Parthenin hormesis in plants depends on growth conditions. Environ. Exp. Bot. 69, 293–301. doi: 10.1016/j.envexpbot.2010.04.010
Blamey, F. P. C., Kopittke, P. M., Wehr, J. B., and Menzies, N. W. (2015). “Beneficial elements. Aluminum,” in Handbook of Plant Nutrition, 2nd Edn, eds A. V. Barker and D. J. Pilbeam (Boca Raton, FL: CRC Press), 567–606.
Bojórquez-Quintal, J., Sánchez-Cach, L. A., Ku-González, A., de los Santos-Briones, C., Medina-Lara, M. F., Echevarría-Machado, I., et al. (2014). Differential effects of aluminum on in vitro primary root growth, nutrient content and phospholipase C activity in coffee seedlings (Coffea arabica). J. Inorg. Biochem. 134, 39–48. doi: 10.1016/j.jinorgbio.2014.01.018
Borikov, A., and Kaliman, P. A. (1999). Influence of cadmium chloride and hydrogen peroxide on the content of phosphoinositides in isolated hepatocytes of rats. Ukr. Biochem. J. 76, 104–109.
Boss, W. F., and Im, I. J. (2012). Phosphoinositide signaling. Annu. Rev. Plant Biol. 63, 409–429. doi: 10.1146/annurev-arplant-042110-103840
Bovykin, B. A., Kilivnik, K. E., and Zegzhda, G. D. (1999). Effect of chromium (III) ions and a cysteine complex of it on lipid bilayer membranes. Biofizika 44, 461–464.
Cacas, J.-L., Gerbeau-Pissot, P., Fromentin, J., Cantrel, C., Thomas, D., Jeannette, E., et al. (2016). Diacylglycerol kinases activate tobacco NADPH oxidase-dependent oxidative burst in response to cryptogein. Plant Cell Environ. doi: 10.1111/pce.12771 [Epub ahead of print].
Cai, J., Abramovici, H., Gee, S., and Topham, M. (2009). Diacylglycerol kinases as sources of phosphatidic acid. Biochim. Biophys. Acta Mol. Cell Biol. Lipids 1791, 942–948. doi: 10.1016/j.bbalip.2009.02.010
Calabrese, E. (2014). Hormesis: a fundamental concept in biology. Microbial Cell 1, 145–149. doi: 10.1242/jeb.067470
Cox, A., Venkatachalam, P., Sahi, S., and Sharma, N. (2016). Silver and titanium dioxide nanoparticle toxicity in plants: a review of current research. Plant Physiol. Biochem. 107, 147–163. doi: 10.1016/j.plaphy.2016.05.022
de Torres Zabela, M., Fernandez-Delmond, I., Niittyla, T., Sanchez, P., and Grant, M. (2002). Differential expression of genes encoding Arabidopsis phospholipases after challenge with virulent or avirulent Pseudomonas isolates. Mol. Plant Microbe Interact. 15, 808–816. doi: 10.1094/MPMI.2002.15.8.808
Dubots, E., Botté, C., Boudière, L., Yamaryo-Botté, Y., Jouhet, J., Maréchal, E., et al. (2011). Role of phosphatidic acid in plant galactolipid synthesis. Biochimie 94, 86–93. doi: 10.1016/j.biochi.2011.03.012
Edgar, R. C. (2004). MUSCLE: multiple sequence alignment with high accuracy and high throughput. Nucleic Acids Res. 32, 1792–1797. doi: 10.1093/nar/gkh340
EFSA NDA Panel (2014). EFSA panel on dietetic products, nutrition and allergies. scientific opinion on dietary reference values for chromium. EFSA J. 12:3845. doi: 10.2903/j.efsa.2014.3845
EPA (2002). Six-Year Review of National Primary Drinking Water Regulation. Washington, DC: Environmental Protection Agency, 453.
Fakas, S., Konstantinou, C., and Carman, G. M. (2011). DGK1-encoded Diacylglycerol kinase activity is required for phospholipid synthesis during growth resumption from stationary phase in Saccharomyces cerevisiae. J. Biol. Chem. 286, 1464–1474. doi: 10.1074/jbc.M110.194308
Finnegan, P. M., and Chen, W. (2012). Arsenic toxicity: the effects on plant metabolism. Front. Physiol. 3:182. doi: 10.3389/fphys.2012.00182
Ge, H., Chen, C., Jing, W., Zhang, Q., Wang, H., Wang, R., et al. (2012). The rice diacylglycerol kinase family: functional analysis using transient RNA interference. Front. Plant Sci. 3:60. doi: 10.3389/fpls.2012.00060
Gómez-Merino, F., Arana-Ceballos, F., Trejo-Téllez, L., Skirycz, A., Brearley, C., Dörmann, P., et al. (2005a). Arabidopsis AtDGK7, the smallest member of plant diacylglycerol kinases (DGKs), displays unique biochemical features and saturates at low substrate concentration. J. Biol. Chem. 280, 34888–34899. doi: 10.1074/jbc.M5068592004
Gómez-Merino, F., Brearley, C., Ornatowska, M., Abdel-Haliem, M., Zanor, M., and Mueller-Roeber, B. (2004). AtDGK2, a novel diacylglycerol kinase from Arabidopsis thaliana, phosphorylates 1-stearoyl-2-arachidonoyl-sn-glycerol and 1,2-Dioleoyl-sn-glycerol and exhibits cold-inducible gene expression. J. Biol. Chem. 279, 8230–8241. doi: 10.1074/jbc.M312187200
Gómez-Merino, F. C., Trejo-Téllez, L. I., Arana-Cerballos, F. A., Skirycz, A., and Mueller-Roeber, B. (2005b). “Aluminium modifies Diacylglicerol kinase gene expression in Arabidopsis thaliana,” in Proceedings of the First International Congress on Crop Plants Nutrition, eds L. I. Trejo-Téllez, M. Sandoval-Villa, and F. C. Gómez-Merino (Texcoco: Colegio de Postgraduados-Mexico), 26–29.
Gonorazky, G., Laxalt, A., and de la Canal, L. (2010). Involvement of phospholipase C in the responses triggered by extracellular phosphatidylinositol 4-phosphate. J. Plant Physiol. 167, 411–415. doi: 10.1016/j.jplph.2009.10.003
González, C. I., Maine, M. A., Cazenave, J., Sanchez, G. C., and Benavides, M. P. (2015). Physiological and biochemical responses of Eichhornia crassipes exposed to Cr (III). Environ. Sci. Pollut. Res. 22, 3739–3747. doi: 10.1007/s11356-014-3558-4
Govindaraju, K., Ramasami, T., and Ramaswamy, D. (1989). Chromium(III)-insulin derivatives and their implication in glucose metabolism. J. Inorg. Biochem. 35, 37–47. doi: 10.1016/0162-0134(89)80006-6
Han, C. L., Wu, L. H., Tan, W. N., Zhong, D. X., Huang, Y. J., Luo, Y. M., et al. (2012). Cadmium distribution in rice plants grown in three different soils after application of pig manure with added cadmium. Environ. Geochem. Health 34, 481–492. doi: 10.1007/s10653-011-9442-y
Han, G., O’Hara, L., Carman, G., and Siniossoglou, S. (2008a). An unconventional diacylglycerol kinase that regulates phospholipid synthesis and nuclear membrane growth. J. Biol. Chem. 283, 20433–20442. doi: 10.1074/jbc.M802903200
Han, G., O’Hara, L., Siniossoglou, S., and Carman, G. (2008b). Characterization of the yeast DGK1-encoded CTP-dependent diacylglycerol kinase. J. Biol. Chem. 283, 20443–20453. doi: 10.1074/jbc.M802866200
Heinz, G. H., Hoffman, D. J., Klimstra, J. D., Stebbins, K. R., Kondrad, S. L., and Erwin, C. A. (2012). Hormesis associated with a low dose of methylmercury injected into mallard eggs. Arch. Environ. Contam. Toxicol. 62, 141–144. doi: 10.1007/s00244-011-9680-0
Helmcke, K. J., and Aschner, M. (2010). Hormetic effect of methylmercury on Caenorhabditis elegans. Toxicol. Appl. Pharmacol. 248, 156–164. doi: 10.1016/j.taap.2010.07.023
Henikoff, S., and Henikoff, J. G. (1992). Amino acid substitution matrices from protein blocks. Proc. Natl. Acad Sci. U.S.A. 89, 10915–11919. doi: 10.1073/pnas.89.22.10915
Hong, Y., Devaiah, S. P., Bahn, S. C., Thamasandra, B. N., Li, M., Wetli, R., et al. (2014). Phospholipase D𝜀 and phosphatidic acid enhance Arabidopsis growth. Plant J. 58, 376–387. doi: 10.1111/j.1365-313X.2009.03788.x
Hou, Q., Ufer, G., and Bartels, D. (2016). Lipid signalling in plant responses to abiotic stress. Plant Cell Environ. 39, 1029–1048. doi: 10.1111/pce.12666
Hyodo, K., Taniguchi, T., Manabe, Y., Kaido, M., Mise, K., Sugawara, T., et al. (2015). Phosphatidic acid produced by phospholipase D promotes RNA replication of a plant RNA virus. PLoS Pathog. 11:e1004909. doi: 10.1371/journal.ppat.1004909
Kabir, A. H. (2016). Biochemical and molecular changes in rice seedlings (Oryza sativa L.) to cope with chromium stress. Plant Biol. 18, 710–719. doi: 10.1111/plb.12436
Kang, S. J., Ryoo, I. G., Lee, Y. J., and Kwak, M. K. (2012). Role of the Nrf2-heme oxygenase-1 pathway in silver nanoparticle-mediated cytotoxicity. Toxicol. Appl. Pharmacol. 258, 89–98. doi: 10.1016/j.taap.2011.10.011
Katagiri, T., Takahashi, S., and Shinozaki, K. (2001). Involvement of a novel Arabidopsis phospholipase D, AtPLDδ, in dehydration-inducible accumulation of phosphatidic acid in stress signaling. Plant J. 26, 595–605. doi: 10.1046/j.1365-313x.2001.01060.x
Kirik, A., and Mudgett, B. (2009). SOBER1 phospholipase activity suppresses phosphatidic acid accumulation and plant immunity in response to bacterial effector AvrBsT. Proc. Natl. Acad. Sci. U.S.A. 106, 20532–20537. doi: 10.1073/pnas.0903859106
Lemire, J. A., Harrison, J. J., and Turner, R. J. (2013). Antimicrobial activity of metals: mechanisms, molecular targets and applications. Nat. Rev. Microbiol. 11, 371–384. doi: 10.1038/nrmicro3028
Li, Y., Tan, Y., Shao, Y., Li, M., and Ma, F. (2015). Comprehensive genomic analysis and expression profiling of diacylglycerol kinase gene family in Malus prunifolia (Willd.) Borkh. Gene 561, 225–234. doi: 10.1016/j.gene.2015.02.029
Liu, Z., Chen, W., He, X., Jia, L., Yu, S., and Zhao, Z. (2015). Hormetic responses of Lonicera japonica Thunb. to cadmium stress. Dose Response 13:1. doi: 10.2203/dose-response.14-033.He
Logothetis, D. E., Petrou, V. I., Zhang, M., Mahajan, R., Meng, X.-Y., Adney, S. K., et al. (2015). Phosphoinositide control of membrane protein function: a frontier led by studies on ion channels. Annu. Rev. Physiol. 77, 81–104. doi: 10.1146/annurev-physiol-021113-170358
López-Millán, A. F., Sagardoy, R., Solanas, M., Abadía, A., and Abadía, J. (2009). Cadmium toxicity in tomato (Lycopersicon esculentum) plants grown in hydroponics. Environ. Exp. Bot. 65, 376–385. doi: 10.1016/j.envexpbot.2008.11.010
Ma, Y., and Hooda, P. S. (2010). “Chromium, nickel and cobalt,” in Trace Elements in Soils, ed. P. S. Hooda (Chichester: Wiley), 461–480.
Maathuis, F. J. M. (2013). Sodium in plants: perception, signalling, and regulation of sodium fluxes. J. Exp. Bot. 65, 849–858. doi: 10.1093/jxb/ert326
Martínez-Estévez, M., Loyola-Vargas, V., and Hernández-Sotomayor, S. (2001a). Aluminum increases phosphorylation of particular proteins in cellular suspension cultures of coffee (Coffea arabica). J. Plant Physiol. 158, 1375–1379. doi: 10.1078/0176-1617-00623
Martínez-Estévez, M., Muñoz-Sánchez, J., Loyola-Vargas, V., and Hernández-Sotomayor, S. (2001b). Modification of the culture medium to produce aluminum toxicity in cell suspensions of coffee (Coffea arabica L.). Plant Cell 20, 469–474. doi: 10.1007/s002990100332
Martínez-Estévez, M., Racagni-Di, G., Muñóz-Sánchez, J., Brito-Argáez, L., Loyola-Vargas, V., and Hernández-Sotomayor, S. (2003). Aluminium differentially modifies lipid metabolism from the phosphoinositide pathway in Coffea arabica cells. J. Plant Physiol. 160, 1297–1303. doi: 10.1078/0176-1617-01168
McCarty, M. F. (2006). PKC-mediated modulation of L-type calcium channels may contribute to fat-induced insulin resistance. Med. Hypotheses 66, 824–831. doi: 10.1016/j.mehy.2004.08.034
Moustaka, J., Ouzounidou, G., Bayçu, G., and Moustakas, M. (2016). Aluminum resistance in wheat involves maintenance of leaf Ca2+ and Mg2+ content, decreased lipid peroxidation and Al accumulation, and low photosystem II excitation pressure. Biometals 29, 611–623. doi: 10.1007/s10534-016-9938-0
Munnik, T. (2001). Phosphatidic acid: an emerging plant lipid second messenger. Trends Plant Sci. 6, 227–233. doi: 10.1016/S1360-1385(01)01918-5
Munnik, T., Meijer, H. J. G., ter Riet, B., Hirt, H., Frank, W., Bartels, D., et al. (2000). Hyperosmotic stress stimulates phospholipase D activity and elevates the levels of phosphatidic acid and diacylglycerol pyrophosphate. Plant J. 22, 147–154. doi: 10.1046/j.1365-313x.2000.00725.x
Munnik, T., van Himbergen, J. A. J., ter Riet, B., Braun, F., Irvine, R. F., van den Ende, H., et al. (1998). Detailed analysis of the turnover of polyphosphoinositides and phosphatidic acid upon activation of phospholipases C and D in Chlamydomonas cells treated with non-permeabilizing concentrations of mastoparan. Planta 207, 133–145. doi: 10.1007/s004250050465
Nicholas, K., and Nicholas, H. (1997). GeneDoc: A Tool for Editing and Annotating Multiple Sequence Alignment. Available at: http://www.psc.edu/biomed/genedoc
Peters, C., Kim, S. C., Devaiah, S., Li, M., and Wang, X. (2002). Non-specific phospholipase C5 and diacylglycerol promote lateral root development under mild salt stress in Arabidopsis. Plant Cell Environ. 37, 2002–2013. doi: 10.1111/pce.12334
Peters, C., Li, M., Narasimhan, R., Roth, M., Welti, R., and Wang, X. (2010). Nonspecific phospholipase C NPC4 promotes responses to abscisic acid and tolerance to hyperosmotic stress in Arabidopsis. Plant Cell 22, 2642–2659. doi: 10.1105/tpc.109.071720
Pilon-Smits, E. A., Quinn, C. F., Tapken, W., Malagoli, M., and Schiavon, M. (2009). Physiological functions of beneficial elements. Curr. Opin. Plant Biol. 12, 267–274. doi: 10.1016/j.pbi.2009.04.009
Pleskot, R., Pejchar, P., Bezvoda, R., Lichtscheidl, I. K., Wolters-Arts, M., Marc, J., et al. (2012). Turnover of phosphatidic acid through distinct signaling pathways affects multiple aspects of pollen tube growth in tobacco. Front. Plant Sci. 3:54. doi: 10.3389/fpls.2012.00054
Poschenrieder, C., Cabot, C., Martos, S., Gallego, B., and Barceló, J. (2013). Do toxic ions induce hormesis in plants? Plant Sci. 212, 15–25. doi: 10.1016/j.plantsci.2013.07.012
Qiu, Y., Fakas, S., Han, G. S., Barbosa, A. D., Siniossoglou, S., and Carman, G. M. (2013). Transcription factor Reb1p regulates DGK1-encoded diacylglycerol kinase and lipid metabolism in Saccharomyces cerevisiae. J. Biol. Chem. 288, 29124–29133. doi: 10.1074/jbc.M113.507392
Rajakumar, S., Bhanupriya, N., Ravi, C., and Nachiappan, V. (2016). Endoplasmic reticulum stress and calcium imbalance are involved in cadmium-induced lipid aberrancy in Saccharomyces cerevisiae. Cell Stress Chaperones 21, 895–906. doi: 10.1007/s12192-016-0714-4
Ronquist, F., Huelsenbeck, J. P., and van der Mark, P. (2005). MrBayes, Version 3.1 Manual. Available at: http://mrbayes.csit.fsu.edu/manual.php
Ruelland, E., Cantrel, C., Gawer, M., Kader, J., and Zachowski, A. (2002). Activation of phospholipases C and D is an early response to a cold exposure in Arabidopsis suspension cells. Plant Physiol. 130, 999–1007. doi: 10.1104/pp.006080
Ruelland, E., Kravets, V., Derevyanchuk, M., Martinec, J., Zachowski, A., and Pokotylo, I. (2015). Role of phospholipid signaling in plant environmental responses. Environ. Exp. Bot. 114, 129–143. doi: 10.1016/j.envexpbot.2014.08.009
Saucedo-García, M., Gavilanes-Ruíz, M., and Arce-Cervantes, O. (2015). Long-chain bases, phosphatidic acid, MAPKs, and reactive oxygen species as nodal signal transducers in stress responses in Arabidopsis. Front. Plant Sci. 6:55. doi: 10.3389/fpls.2015.00055
Schmidt, C. M., Cheng, C. N., Marino, A., Konsoula, R., and Barile, F. A. (2004). Hormesis effect of trace metals on cultured normal and immortal human mammary cells. Toxicol. Ind. Health 20, 57–68. doi: 10.1191/0748233704th192oa
Sharma, V. K., Yngard, R. A., and Lin, Y. (2009). Silver nanoparticles: Green synthesis and their antimicrobial activities. Adv. Colloid Interface Sci. 145, 83–96. doi: 10.1016/j.cis.2008.09.002
Shin, J., and Loewen, C. (2011). Putting the pH into phosphatidic acid signalling. BMC Biol. 9:85. doi: 10.1186/1741-7007-9-85
Shridas, P., and Waechter, C. J. (2006). Human Dolichol Kinase, a polytopic endoplasmic reticulum membrane protein with a cytoplasmically oriented CTP-binding site. J. Biol. Chem. 281, 31696–31704. doi: 10.1074/jbc.M604087200
Sievers, F., Wilm, A., Dineen, D., Gibson, T., Karplus, K., Li, W., et al. (2011). Fast, scalable generation of high-quality protein multiple sequence alignment using Clustal Omega. Mol. Syst. Biol. 7:539. doi: 10.1038/msb.2011.75
Singh, A., Bhatnagar, N., Pandey, A., and Pandey, G. (2015). Plant phospholipase C family: Regulation and functional role in lipid signaling. Cell Calcium 58, 139–146. doi: 10.1016/j.ceca.2015.04.003
Snedden, W., and Blumwald, W. (2000). Alternative splicing of a novel diacylglycerol kinase in tomato leads to a calmodulin-binding isoform. Plant J. 24, 317–326. doi: 10.1046/j.1365-313x.2000.00877.x
Sparks, E., Wachsman, G., and Benfey, P. N. (2013). Spatiotemporal signalling in plant development. Nat. Rev. Genet. 14, 631–644. doi: 10.1038/nrg3541
Stearns, D. M. (2007). “Multiple hypotheses of chromium(III) biochemistry. Why the essentiality of chromium(III) is still questioned,” in The Nutritional Biochemistry of Chromium, ed. J. B. Vincent (Oxford: Elsevier), 57–70.
Subbarao, G. V., Ito, O., Berry, W. L., and Wheeler, R. M. (2003). Sodium. A functional plant nutrient. Crit. Rev. Plant Sci. 22, 391–416. doi: 10.1080/713989756
Subhadra, A. V., Nanda, A. K., Behera, P. K., and Panda, B. B. (1991). Acceleration of catalase and peroxidase activities in Lemna minor L. and Allium cepa L. in response to low levels of aquatic mercury. Environ. Pollut. 69, 169–179. doi: 10.1016/0269-7491(91)90141-I
Sui, Z., Niu, L., Yue, G., Yang, A., and Zhang, J. (2008). Cloning and expression analysis of some genes involved in the phosphoinositide and phospholipid signaling pathways from maize (Zea mays L.). Gene 426, 47–56. doi: 10.1016/j.gene.2008.09.004
Tandon, S. K. (1982). “Organ toxicity of chromium in animals,” in Biological and Environmental Aspects of Chromium, ed. S. Langard (Amsterdam: Elsevier), 209–220.
Testerink, C., Dekker, H., Lim, Z., Johns, M., Holmes, A., de Koster, C., et al. (2004). Isolation and identification of phosphatidic acid targets from plants. Plant J. 39, 527–536. doi: 10.1111/j.1365-313X.2004.02152.x
Testerink, C., and Munnik, T. (2005). Phosphatidic acid: a multifunctional stress signaling lipid in plants. Trends Plant Sci. 10, 368–375. doi: 10.1016/j.tplants.2005.06.002
Testerink, C., and Munnik, T. (2011). Molecular, cellular, and physiological responses to phosphatidic acid formation in plants. J. Exp. Bot. 62, 2349–2361. doi: 10.1093/jxb/err079
Thorvaldsson, G., and Jónsdóttir, R. S. (2005). Essential Trace Elements for Plants, Animals and Humans. Borgarnes: Agricultural University of Iceland.
Topham, M. K., and Prescott, S. M. (2010). “Diacylglycerol kinases,” in Handbook of Cell Signaling, 2nd Edn, Vol. I, eds R. A. Bradshaw and E. A. Dennis (New York, NY: Elsevier), 1177–1182.
Trejo-Téllez, L. I., Gómez-Merino, F. C., and Alcántar-González, G. (2016). “Elementos benéficos: potencialidades y limitaciones,” in Nutrición de Cultivos. Segunda Edición, eds G. Alcántar-González, L. I. Trejo-Téllez, and F. C. G. ómez-Merino (Montecillo: Ed. Colegio de Postgraduados),k59–101.
Trejo-Téllez, L. I., Gómez-Merino, F. C., Rivera-Olivares, L. Y., and Tejeda-Sartorius, O. (2014). Cadmium-induced changes in leaf nutrient concentrations in sugarcane. J. Food Agric. Environ. 12, 879–885.
Tu, C., and Ma, L. Q. (2003). Interactive effects of pH, arsenic and phosphorus on uptake of As and P and growth of the arsenic hyperaccumulator Pteris vittata L. under hydroponic conditions. Environ. Exp. Bot. 50, 243–251. doi: 10.1016/S0098-8472(03)00040-6
van der Luit, A., Piatti, T., van Doorn, A., Musgrave, A., Felix, G., Boller, T., et al. (2000). Elicitation of suspension-cultured tomato cells triggers the formation of phosphatidic acid and diacylglycerol pyrophosphate. Plant Physiol. 123, 1507–1516. doi: 10.1104/pp.123.4.1507
Vaultier, M., Cantrel, C., Guerbette, F., Boutte, Y., Vernogolle, C., Cicek, D., et al. (2008). The hydrophobic segment of Arabidopsis thaliana cluster I diacylglycerol kinases is sufficient to target the proteins to cell membranes. FEBS Lett. 582, 1743–1748. doi: 10.1016/j.febslet.2008.04.042
Villasuso, A., Palma, M., Aveldaño, M., Pasquaré, S., Racagni, G., Giusto, N., et al. (2013). Differences in phosphatidic acid signalling and metabolism between ABA and GA treatments of barley aleurone cells. Plant Physiol. Biochem. 65, 1–8. doi: 10.1016/j.plaphy.2013.01.005
Wang, Q., Gu, M., Ma, X., Zhang, H., Wang, Y., Cui, J., et al. (2015). Model optimization of cadmium and accumulation in switchgrass (Panicum virgatum L.): potential use for ecological phytoremediation in Cd-contaminated soils. Environ. Sci. Pollut. Res. 22, 16758–16771. doi: 10.1007/s11356-015-4878-8
Wang, X., and Chapman, K. D. (2013). Lipid signaling in plants. Front. Plant Sci. 4:216. doi: 10.3389/fpls.2013.00216
Xie, S., Naslavsky, N., and Caplan, S. (2015). Diacylglycerol kinases in membrane trafficking. Cell. Logist. 5:e1078431. doi: 10.1080/21592799.2015.1078431
Xue, H., Chen, X., and Mei, Y. (2009). Function and regulation of phospholipid signalling in plants. Biochem. J. 421, 145–156. doi: 10.1042/BJ20090300
Yoon, B. J. (2009). Hidden Markov Models and their applications in biological sequence analysis. Curr. Gen. 10, 402–415. doi: 10.2174/138920209789177575
Yunus, I. S., Cazenave-Gassiot, A., Liu, Y. C., Lin, Y.-C., Wenk, M. R., and Nakamura, Y. (2015). Phosphatidic acid is a major phospholipid class in reproductive organs of Arabidopsis thaliana. Plant Signal Behav. 10:e1049790. doi: 10.1080/15592324.2015.1049790
Zhang, Q., Lin, F., Mao, T., Nie, J., Yan, M., Yuan, M., et al. (2012). Phosphatidic acid regulates microtubule organization by interacting with MAP65-1 in response to salt stress in Arabidopsis. Plant Cell 24, 4555–4576. doi: 10.1105/tpc.112.104182
Zhang, W., Chen, J., Zhang, H., and Song, F. (2008). Overexpression of a rice diacylglycerol kinase gene OsBIDK1 enhances disease resistance in transgenic Tobacco. Mol. Cells 26, 258–264.
Zhang, W., Qln, C., Zhao, J., and Wang, X. (2004). Phospholipase Dα1-derived phosphatidic acid interacts with ABI1 phosphatase 2C and regulates abscisic acid signaling. Proc. Natl. Acad. Sci. U.S.A. 101, 9508–9513. doi: 10.1073/pnas.0402112101
Zhang, W., Wang, C., Qin, C., Wood, T., Olafsdottir, G., Welti, R., et al. (2003). The oleate-stimulated phospholipase D, PLDδ, and phosphatidic acid decrease H2O2-induced cell death in Arabidopsis. Plant Cell 15, 2285–2295. doi: 10.1105/tpc.013961
Zhang, W., and Xiao, Z. (2015). Lipid in salicylic acid-mediated defense in plants: focusing on the roles of phosphatidic acid and phosphatidylinositol 4-phosphate. Front. Plant Sci. 6:387. doi: 10.3389/fpls.2015.00387
Keywords: phospholipids, glycerol, plant signaling, hormesis, beneficial elements, Genevestigator
Citation: Escobar -Sepúlveda HF, Trejo-Téllez LI, Pérez-Rodríguez P, Hidalgo-Contreras JV and Gómez-Merino FC (2017) Diacylglycerol Kinases Are Widespread in Higher Plants and Display Inducible Gene Expression in Response to Beneficial Elements, Metal, and Metalloid Ions. Front. Plant Sci. 8:129. doi: 10.3389/fpls.2017.00129
Received: 27 August 2016; Accepted: 21 January 2017;
Published: 07 February 2017.
Edited by:
Marta Wilton Vasconcelos, Catholic University of Portugal, PortugalReviewed by:
Manuel Martinez-Estevez, Centro de Investigacion Cientifica de Yucatan, MexicoEmanuel Bojorquez Quintal, Laboratorio de Análisis y Diagnóstico del Patrimonio (CONACYT), Mexico
Teresa Hernandez-Sotomayor, Centro de Investigación Científica de Yucatán, Mexico
Copyright © 2017 Escobar-Sepúlveda, Trejo-Téllez, Pérez-Rodríguez, Hidalgo-Contreras and Gómez-Merino. This is an open-access article distributed under the terms of the Creative Commons Attribution License (CC BY). The use, distribution or reproduction in other forums is permitted, provided the original author(s) or licensor are credited and that the original publication in this journal is cited, in accordance with accepted academic practice. No use, distribution or reproduction is permitted which does not comply with these terms.
*Correspondence: Fernando C. Gómez-Merino, fernandg@colpos.mx