- Department of Materials Science and Engineering, National Taiwan University of Science and Technology, Taipei, Taiwan
Hydrogen gas sensors are important because of the significant use of hydrogen in industrial and commercial applications. In this study, we synthesized a novel CsxWO3/MoS2 nanocomposite using a solvothermal method. The samples were spin-coated on Si/SiO2 substrates, and the sensors were fabricated with interdigital electrodes. The hydrogen gas sensing properties of the sensor were investigated. CsxWO3/MoS2 exhibited an outstanding hydrogen gas sensing ability at room temperature. In particular, the nanocomposite comprising 15 wt.% MoS2 (15% CsxWO3/MoS2) showed a 51% response to hydrogen gas at room temperature. Further, it exhibited an excellent cyclic stability for hydrogen gas sensing, which is crucial for practical applications. Therefore, this study facilitates the development of effective and efficient hydrogen gas sensors operable at room temperature.
Introduction
A polluted atmosphere endangers the health of living organisms. It comprises various gases that exist in nature and those released from industries (Masteghin et al., 2019; Pisarkiewicz et al., 2020; Duc et al., 2020; Kim et al., 2021). These odorless, tasteless, colorless gases are toxic when they exceed the safe limits (Ha et al., 2018; Miglietta et al., 2020; Pisarkiewicz et al., 2020). Hydrogen (H2), carbon dioxide (CO2), carbon monoxide (CO), and ammonia (NH3) present in the environment have adverse effects on human health when their concentrations exceed the permissible levels (Annanouch et al., 2021; Hübert et al., 2014; Saravanan et al., 2017). Therefore, the amount of these gases in the environment must be monitored, and the development of effective and efficient detection methods is essential.
Hydrogen is one of the most hazardous gas and regulating its concentration with adequate monitoring devices is crucial (Hübert et al., 2014). Currently it is extensively used as a major ecological energy source and is used in semiconductor processing, chemical industry, nuclear reactors, and petroleum extraction. Hydrogen is a potential candidate for next-generation energy requirements in power plants, automobile transportation, and aerospace industries. However, the storage and regulated supply of hydrogen are challenging owing to the potential dangers of leakage (Saravanan et al., 2017). Therefore, developing long-lasting and cost-effective hydrogen sensing devices that are highly reliable for the accurate detection of the hazardous hydrogen gas is compelling (Hienuki et al., 2021; Liang et al., 2021; Sridhar et al., 2021).
In recent years, tungsten-based materials have gained a lot of interest owing to their nanostructures and unique chemical and physical properties (Chala et al., 2017; Motora and Wu, 2020; Wu et al., 2019). Because of their good selectivity and stability, they are the most suitable for fabricating high-grade sensors. In particular, tungsten trioxide has been extensively used in sensors for the detection of hazardous gases, such as hydrogen, ammonia, nitrous oxide, and hydrogen sulfide (Wang et al., 2000; Rydosz et al., 2014; Castillo et al., 2020; Dong et al., 2020). However, most of the WO3-based gas sensors function at high temperatures or have high response/recovery times, which hinder their practical application (Esfandiar et al., 2014; Chang et al., 2020). Therefore, the development of tungsten oxide-based gas sensor materials that can circumvent these limitations is important. In our previous study, we proposed a new tungsten bronze material-cesium tungsten oxide (CsxWO3) for hydrogen gas sensors, which exhibited significant hydrogen gas sensing properties at room temperature owing to its mixed-valence state and unique properties (Wu et al., 2021). However, its sensor response and response time need to be enhanced to detect hydrogen gas within a short duration, which are crucial for practical applications. Therefore, to circumvent this limitation, a suitable sensor material should be selected.
In contrast, molybdenum disulfide is a transition metal dichalcogenide (TMD), which is used in various applications, such as photocatalysis, sensing, disinfection, contaminant adsorption, and membrane-based separation (Barua et al., 2017; Li et al., 2018; Bello et al., 2020). It has been applied in the field of sensors as photochemical sensors, gas sensors, glucose sensors, photodetectors, electrochemical sensors, and DNA sensors (Zhang et al., 2015; Akbari et al., 2018). Among the different types of gases, MoS2 nanostructures are usually selective toward NH3 gas at room temperature, which hinders their applicability as gas sensors. Although MoS2 is a good sensing material, it has limitations, such as a low structural strength and conductivity (Huaning et al., 2021). However, few studies have shown that its selective behavior can change while forming hybrids with different materials; this can be ascribed to the charge-transfer-based modulation of the channel conductivity of the gas sensor mechanism (Gottam et al., 2020). Therefore, combining MoS2 with unique material is important for achieving an enhanced sensing performance. For instance, WO3/MoS2 composites have been developed, and the nanocomposite exhibits excellent ammonia and hydrogen sulfide sensing properties (Singh et al., 2020, Singh et al., 2021). However, these sensor devices function only at temperatures higher than 200°C. Besides, most of the previously developed hydrogen gas sensor exhibited low sensor response. It is very important to develop hydrogen gas sensor with high sensitivity, selectivity, and stability for practical applications. Therefore, the development of MoS2 composites with excellent hydrogen sensing performance at room temperature is crucial.
Herein, we developed CsxWO3@MoS2 nanocomposites for application in hydrogen gas sensors through a solvothermal method by taking advantage of the low-temperature sensing properties of CsxWO3 and the high sensor response of MoS2. The hydrogen gas sensing properties of the developed CsxWO3@MoS2 nanocomposite gas sensor at room temperature were systematically investigated. The proposed gas-sensing mechanism of the sensor was also explained.
Materials and Methods
Materials
The tungsten hexachloride (WCl6), cesium hydroxide (CsOHH2O), anhydrous ethanol, and acetic acid were purchased from Kanto Chemical Co., Inc. Molybdenum disulfide powder (<2 μm, 98%) and N, N-dimethylformamide (DMF) were purchased from Sigma Aldrich.
All reagents and chemicals used in this study were of analytical grade and were used without further purification.
Synthesis of CsxWO3 Nanorods
Tungsten hexachloride (0.2976 g) was added to anhydrous ethanol (40 ml) under intense stirring, resulting in the formation of a yellowish solution. Thereafter, 0.065 g of CsOH·H2O was introduced into the yellowish solution. After the resultant solution became homogeneous, 10 ml of acetic acid was added. Subsequently, the mixture was transferred into a 100 ml Teflon-lined autoclave, followed by hydrothermal treatment at 240°C for 20 h. Afterward, it was cooled to room temperature naturally, and the blue product was centrifuged, washed with ethanol several times, and finally dried in a vacuum oven at 60°C for 8 h (Wu et al., 2021).
Exfoliation of MoS2 Nanosheets
The MoS2 nanosheets were exfoliated by ultrasonication according to a previously reported method (Bera and sciences, 2017). Bulk MoS2 (0.64 g) was added to DMF (20 ml) in a flat-bottomed beaker (100 ml) and ultrasonicated for 60 min. The temperature of the sonication was controlled by a cooling system during ultrasonication. Afterward, the sample was centrifuged, washed, and dried at 60°C for 8 h.
Preparation of CsxWO3/MoS2 Nanocomposites
The CsxWO3/MoS2 nanocomposite was prepared using a hydrothermal method. The material required for the synthesis—0.1 M aqueous solution of MoS2 (30 ml) was stirred for 1 h. Simultaneously, 0.2976 g of tungsten hexachloride was mixed with 40 ml of anhydrous ethanol under intense stirring, which resulted in the formation of a yellowish solution. Thereafter, 0.065 g of CsOH·H2O was added to the yellowish solution. Subsequently, 10 ml of acetic acid was added to the resultant solution when it became homogeneous. Afterward, different amounts of the prepared MoS2 solution were added to form composites with different ratios. Subsequently, the mixture was transferred into a 100 ml Teflon-lined autoclave, followed by a hydrothermal process at 240°C for 20 h. The obtained dark blue product was centrifuged, washed with ethanol several times, and finally dried in a vacuum oven at 60°C. In this study, three different composites were prepared by changing the amount of MoS2 and labeled as 5%, 15%, and 30%.
Characterization of CsxWO3/MoS2 Nanocomposites
Field emission scanning electron microscopy (FESEM, JSM6500F, JEOL, Tokyo, Japan), transmission electron microscopy (TEM), and high-resolution TEM (TEM, Philips-6500F) were used to study the surface morphologies of the samples. The crystal structures of the CsxWO3, MoS2, and CsxWO3/MoS2 nanocomposites were obtained by X-ray diffraction (XRD; D2 Phaser X-ray diffractometer, Bruker, Karlsruhe, Germany) analysis. The chemical compositions of the prepared samples were determined by Raman spectroscopy using an HR800 instrument (Jobin Yvon, Taipei, Taiwan). The N2 adsorption/desorption isotherm and Brunauer, Emmett, and Teller (BET) surface area analyses of the samples were conducted at 77 K using a Quanta chrome iQ-MP gas adsorption analyzer (BEL JAPAN, INC.).
Sensor Fabrication and Hydrogen Gas Sensing Measurements
To fabricate the gas sensor, the as-prepared CsxWO3, MoS2, and CsxWO3/MoS2 nanocomposites were diluted with isopropanol (IPA) and spin-coated on Si/SiO2 substrates, which were then sputtered with multi-finger Pt interdigitated electrodes (Figure 1). The H2 gas sensing properties of the prepared sensors were measured in a compact vacuum chamber with an H2 flow of 99.9% (diluted with dry air) via a mass flow controller. The properties were measured systematically from the electrical output response recorded using a Keithley source meter unit (SMU, Keithley 2,400, Tektronix, Beaverton, OR, USA) connected to a computer.
Electrochemical Measurements of the Sensors
The electrochemical properties of the developed sensor materials were investigated by electrochemical impedance spectroscopy (EIS) using a 5,000 electrochemical workstation (Jiehan Technology Corp., Taiwan), wherein a conventional three-electrode configuration was used. In this measurement, 0.1 M aqueous solution of KCl was used as the electrolyte and platinum and Ag/AgCl were used as the counter and reference electrodes, respectively; a titanium substrate was used as the working electrode (Phuruangrat et al., 2009). To prepare the working electrode for EIS measurements, 4 mg of CsxWO3, MoS2, and 15% CsxWO3/MoS2 were added to 80 µL of Nafion solution and 920 µL of absolute ethanol, and the mixture was ultrasonicated for approximately 90 min to obtain a slurry. Thereafter, 40 µL of the slurry was drop-cast onto a 1 × 1 cm2 titanium substrate electrode and dried at room temperature.
Results and Discussion
Morphological and Structural Properties of CsxWO3/MoS2 Nanocomposites
The surface morphologies of the prepared samples were studied by FESEM, and the FESEM image in Figure 2A shows that MoS2 has a sheet-like morphology, mostly smaller than 2 µm in size. The FESEM image in Figure 2B depicts that the surface morphology of CsxWO3 comprises a nanorod-like structure with an average diameter of 40–50 nm. The FESEM image of the 15% CsxWO3/MoS2 nanocomposite in Figure 2C shows the coexistence of nanorods and nanosheets, owing to surface morphologies of CsxWO3 and MoS2, confirming the successful synthesis of the intended nanocomposites. Furthermore, the corresponding EDS spectrum confirmed the existence of Mo and S from MoS2; Cs, O, and W from CsxWO3. The actual elemental composition of the 15% CsxWO3/MoS2 nanocomposite was also investigated and the result as shown in the inset of Figure 2D. It can be seen from the actual mass percentage and theoretical mass percentage of each element in the nanocomposites that they are almost the same. These results reveal that the CsxWO3/MoS2 nanocomposite was successfully prepared.
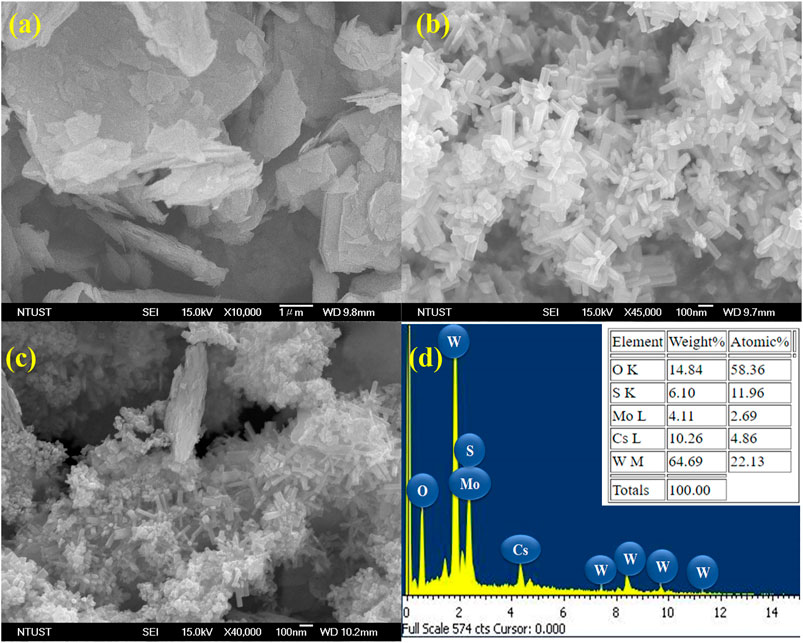
FIGURE 2. FESEM images of (A) MoS2 nanosheets; (B) CsxWO3 nanorods; (C) CsxWO3/MoS2 composites and (D) EDS Spectrum of 15% CsxWO3/MoS2 nanocomposites.
The FESEM image in Figure 3A and elemental mapping results of the 15% CsxWO3/MoS2 nanocomposites presented in Figures 3B–F show the homogeneous distribution of Mo, S, Cs, O, and W elements in the nanocomposite.
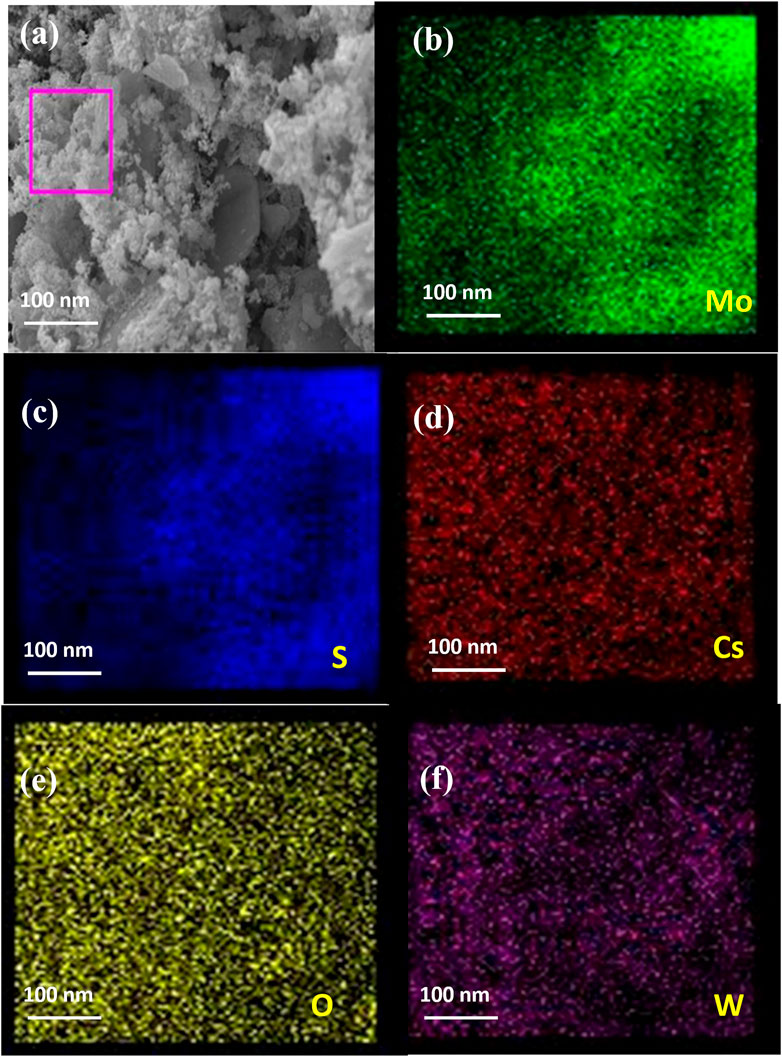
FIGURE 3. (A) FESEM image and elemental mapping of (B) Mo, (C) S, (D) Cs, (E) O, and (F) W of 15% CsxWO3/MoS2 nanocomposite.
The surface morphology of the synthesized 15% CsxWO3/MoS2 nanocomposite was further studied by TEM and HRTEM. The TEM image of 15% CsxWO3/MoS2 nanocomposite shown in Figure 4A can be ascribed to CsxWO3 nanorods and MoS2 nanosheets. The coexistence of the MoS2 nanosheets and CsxWO3 nanorods can be seen in the HRTEM image of the nanocomposite, as shown in Figure 4B. The lattice spacing of 0.32 nm corresponds to the (002) crystal plane of CsxWO3 and that of 0.27 nm corresponds to the (100) crystal plane of MoS2. The corresponding selected area electron diffraction (SAED) pattern in Figure 4C confirms the combination of both CsxWO3 and MoS2, where the dots forming the hexagonal shape are attributed to the MoS2 nanosheets and those forming the triangle are ascribed to CsxWO3 (Ahmadi et al., 2014). However, the SAED dots in Figure 4C form hexagonal and triangular shapes with minimal changes than those of individual CsxWO3 and MoS2. This can be attributed to the nanocomposite formation of CsxWO3/MoS2. These results confirm that the nanocomposite was successfully prepared, and the CsxWO3 nanorods and MoS2 nanosheets were in good contact, which would enhance the gas sensing property.
Figure 5 shows the XRD patterns of the CxWO3 nanorods, MoS2 nanosheets, and their nanocomposites. The XRD pattern of the CsxWO3 nanorods can be ascribed to the hexagonal phase of Cs0.33WO3 (JCPDS No. 83-1334) (Wu et al., 2021). The absence of additional diffraction peaks confirmed the successful synthesis of pure Cs0.33WO3. The XRD peaks of the MoS2 nanosheets can be indexed to hexagonal phase of MoS2 (JCPDS No. 65-1951) (Zhang et al., 2014). The XRD peaks of the CsxWO3/MoS2 nanocomposites comprise characteristic peaks of both CsxWO3 and MoS2, confirming the successful preparation of the nanocomposite. In addition, the peak intensity of CsxWO3 at (200) diffraction peak decreases as the MoS2 content increases. The crystallite size of the CsxWO3 and MoS2 in the synthesized 15% CsxWO3/MoS2 nanocomposite was determined by using the Scherrer equation as shown below:
where
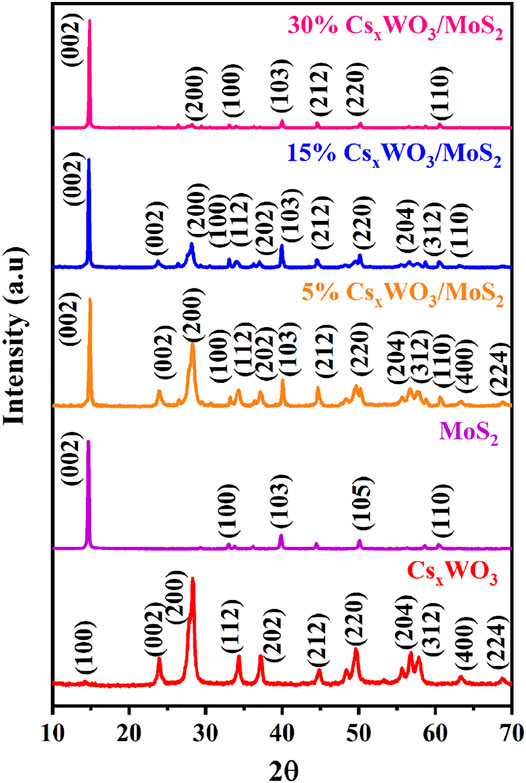
FIGURE 5. XRD results of pure CsxWO3, pure MoS2, and different ratios of CsxWO3/MoS2 nanocomposites.
The as-prepared samples were further characterized by Raman spectroscopy, and the Raman spectra are shown in Figure 6A. The spectra show that two major peaks were observed for the MoS2 nanosheets, which be indexed to the 2H phase of MoS2 and E2g (308 cm−1) and A1g (408 cm−1) activation modes (Vadivelmurugan et al., 2021). The E2g mode corresponds to the plane vibration of S and Mo atoms, whereas the A1g mode is attributed to the relative vibration of the S atom, which is in the plane direction (Yao et al., 2019). Further, the two weak peaks at 451 and 1599 cm−1 indicate E1g and the longitudinal acoustic phonon modes, respectively. In the case of CsxWO3, the Raman peak at 731 cm−1 corresponds to monoclinic Gama WO3 and that at 935 cm−1 corresponds to nanocrystalline CsxWO3 (Okada et al., 2019). These peaks in CsxWO3 confirm the nanocrystalline formation of WO3 with cesium. The characteristic peaks of both MoS2 and CsxWO3 were observed for CsxWO3/MoS2 composite, indicating the successful preparation of the nanocomposites. In addition, there is a slight shift in the WO3 peaks, at 872 and 993 cm−1, which indicates the presence of different organizations of elemental WO6 octahedral.
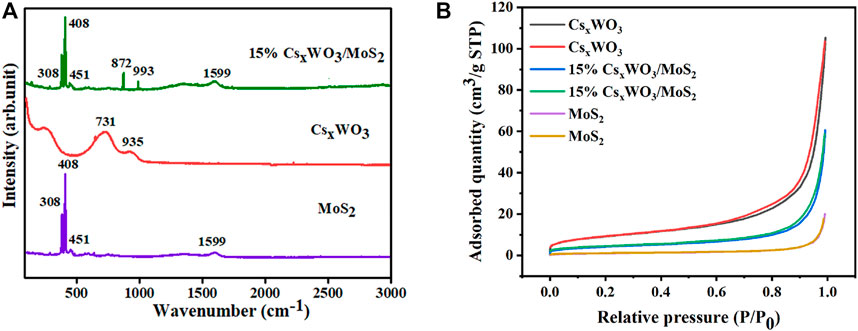
FIGURE 6. (A) Raman spectra and (B) N2 adsorption-desorption isotherms of the pure CsxWO3, pure MoS2, and 15% CsxWO3/MoS2 nanocomposite.
The BET specific surface areas of the materials were investigated by N2 adsorption analysis, and the results in Figure 6B show that all the samples exhibited N2 adsorption-desorption isotherms similar to type IV curves according to the Brunauer-Deming-Deming-Teller classification (Wang et al., 2018). The specific surface areas of the pure CsxWO3, pure MoS2, and 15% CsxWO3/MoS2 nanocomposites were calculated from the linear region of the multipoint plot. The BET specific surface areas of CsxWO3, MoS2, and 15% CsxWO3/MoS2 nanocomposites were 31.1, 3.3, and 15.0 m2g−1, respectively. Herein, the surface area of 15% CsxWO3/MoS2 was lower than that of pure CsxWO3 because of the encompassing of the nanorods by the MoS2 nanosheets. Compared to MoS2 nanosheets, the 15% CsxWO3/MoS2 nanocomposites possessed a larger surface area, which is important for the adsorption of a higher amount of hydrogen gas, and would aid in enhancing the gas sensing properties of the sensor.
Hydrogen Gas Sensing Properties of CsxWO3/MoS2 Composites-Based Gas Sensors
The sensing properties of metal oxide-based gas sensors are usually measured in terms of their sensitivity and repeatability by varying the resistance values of gas and vacuum/air vs. time. The H2 gas detection properties of the CsxWO3/MoS2 nanocomposites were tested at a gas concentration of 10–500 ppm at 25°C ( ∼ 35 RH %). The sensor response of the samples is calculated using Equation 2 for oxidizing gases and vice versa for reducing gases.
where R is the resistance; a is the air atmosphere, and g is the gas atmosphere of the samples.
The gas-sensing performance of a sensor can be determined using the current-voltage (I-V) curve recorded before and after the adsorption of the gas. Herein, we also evaluated the H2 gas sensing performance of 15% CsxWO3/MoS2 nanocomposites using I-V characteristics before and after the adsorption of hydrogen gas, and the results in Figure 7A show the change in the electrical performance in the presence of H2 when compared with that of no hydrogen gas. Figure 7B shows the sensor response of the developed 15% CsxWO3/MoS2 nanocomposite gas sensor at different concentrations (500, 250, 100, and 10 ppm) of hydrogen gas. The developed sensor exhibited outstanding H2 gas sensing properties with a 50.6% sensor response toward 500 ppm of H2 gas with 60 and 120 s response and recovery time, respectively. These recovery time and response time are better than that of reported Pd/CNT/Ni H2 gas sensor that has 312 s response time and 173 s recovery and Ni/Pd-graphene H2 gas, which has 180 s response time and 720 s recover time. (Lin and Huang, 2012; Phan and Chung, 2014). This sensor response is superior to that of the H2 gas sensor of pure CsxWO3 (31.3%) (Wu et al., 2021), which indicates that nanocomposite formation has enhanced the sensitivity. Figure 7C presents the sensor response vs. concentration curve, which shows a linear increase in the sensor response with an increase in concentration, revealing that the developed sensor can be applied to detect a wide range of concentrations of H2 gas. Figure 7D shows that the fabricated 15% CsxWO3/MoS2 nanocomposite H2 gas sensor demonstrates excellent cyclic stability for the five consecutive cycles that are important for practical applications. These findings reveal the potential applicability of the developed 15% CsxWO3/MoS2 nanocomposite sensor for the detection of H2 gas.
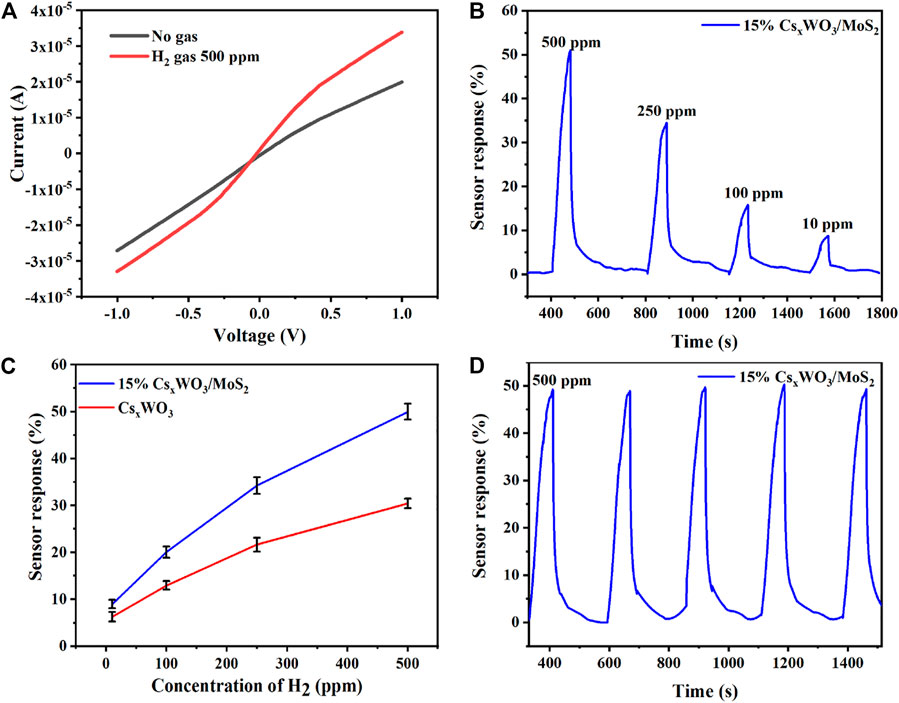
FIGURE 7. (A) I-V curves, (B) sensor response with respect to the H2 gas concentration of 15% CsxWO3/MoS2-based hydrogen gas sensor, (C) variation of the sensing response of CsxWO3 and 15% CsxWO3/MoS2-based hydrogen gas sensor at room temperature when exposed to different ppm hydrogen, and (D) repeatability of 15% CsxWO3/MoS2-based hydrogen gas sensor at 500 ppm.
Long-term stability of sensor is very important for practical application. Herein, the long-term stability of the developed sensor was investigated. The result as shown in Figure 8A shows that it has excellent long-term stability during the 11-days evaluation period. Moreover, selectivity of the fabricated 15% CsxWO3/MoS2 nanocomposite hydrogen gas sensor was also studied. The finding in Figure 8B indicates that the 15% CsxWO3/MoS2 nanocomposite sensor presented excellent selectivity towards hydrogen gas by showing only 4 and 5% sensor response toward ammonia and carbon dioxide, respectively. The sensor response of the developed sensor toward CO2 and NH3 is neglible when compared with that of H2 (50.6%). These findings confirm that developed sensor has potential applicability for real application for H2 gas sensing. The sensing property of the developed sensor with respect to current versus time was also investigated. As it can be seen in Supplementary Figure S1, the current response of the fabricated sensor increases with increasing H2 gas concentration, which proves the behavior of the n-type semiconductor sensor (Wisitsoraat et al., 2009; Mohamad et al., 2010). Moreover, the pure CsxWO3 and MoS2 also have the same properties (Saravanan et al., 2019; Wu et al., 2021).
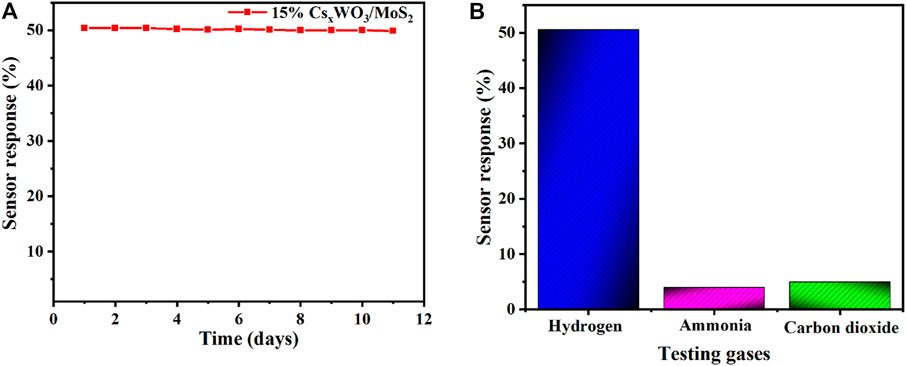
FIGURE 8. (A) long-term stability, and (B) selectivity of the 15% CsxWO3/MoS2-based hydrogen gas sensor.
Figure 9 shows the sensor response of CsxWO3/MoS2 nanocomposites against different amount of MoS2 (5%, 15%, and 30%) added to CsxWO3. The sensor response of the nanocomposite initially increased with an increase in the amount of MoS2 up to 15% MoS2 addition and then decreased till 30% MoS2 addition. This could be because of the encompassing of CsxWO3 nanorods by MoS2 nanosheets with low surface areas, which influences the adsorption of H2 gas and consequently the sensor response (Supplementary Figure S2). The photograph of the prepared samples dispersed in IPA shown in Supplementary Figure S3 also confirms that the intensity of the dark color of the nanocomposite increases with an increase in the concentration of MoS2 nanosheets, which confirms the presence of the high amount of MoS2 in 30% MoS2. Therefore, 15% CsxWO3/MoS2 nanocomposite was selected for further studies.
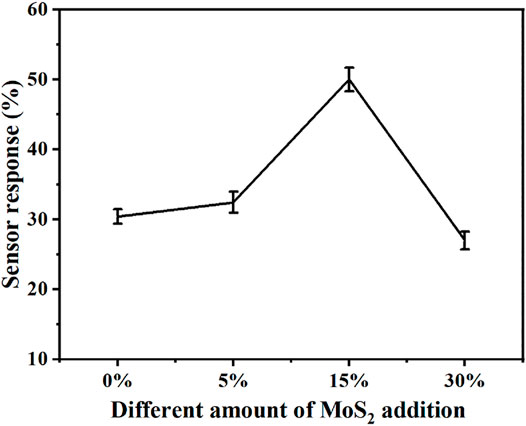
FIGURE 9. Sensor response of the CsxWO3/MoS2 nanocomposite sensor with respect to amounts of MoS2 added to CsxWO3.
Possible Hydrogen Gas Sensing Mechanisms of CsxWO3/MoS2 Nanocomposites
Metal oxide-based gas sensors, which sense hydrogen gas, are usually explained based on the variation of the electron depletion layer (EDL) and oxygen adsorption (Sun et al., 2011; Park et al., 2015; Wang et al., 2018). Herein, we propose a sensing mechanism for the developed sensor based on EDL. CsxWO3 has oxygen defects in its structure, and MoS2 is tuned to have a high bandgap after the exfoliation of bulk MoS2. Therefore, the CsxWO3 nanorods in the nanocomposite can adsorb oxygen from the air because of its oxygen vacancies, and the adsorbed oxygen extracts electrons from the conduction band and generates oxygen species and increases the width of the EDL (Figure 10A). In contrast, when the sensor is exposed to H2 gas, the adsorbed oxygen species react with H2 gas and produce extra electrons in the conduction band of CsxWO3, which increase the electrical performance of the material and consequently the sensing property (Figure 10B).
Further, the electrochemical properties of the samples were investigated, and the results in Figure 11 show that the EIS semicircle radius of the 15% CsxWO3/MoS2 nanocomposite is lower than those of pure CsxWO3 and pure MoS2, confirming that the nanocomposite has a higher electrical conductivity than those of pure materials, which contributed to the enhancement in the hydrogen gas sensor response.
The hydrogen gas sensing response of the developed 15% CsxWO3/MoS2 nanocomposite was also compared with those of reported hydrogen gas sensors, and the results in Table 1 indicate that the 15% CsxWO3/MoS2 nanocomposite exhibited a higher sensor response than those of other sensors (Esfandiar et al., 2012; Huang and Lin, 2012; Anand et al., 2014; Liu et al., 2015; Yang et al., 2015; Zhang et al., 2018; Sharma and Kim, 2018; Xiao et al., 2018; Wu et al., 2021). Further, some of the reported sensors can only operate at high temperatures. Therefore, the developed 15% CsxWO3/MoS2 nanocomposite H2 gas sensor is a promising candidate for practical application in the detection of hydrogen gas.
Conclusion
In this study, CsxWO3/MoS2 nanocomposites were successfully prepared using the solvothermal method. The developed CsxWO3/MoS2 nanocomposite-based sensor exhibited outstanding hydrogen gas sensing properties with 51% response to 500 ppm H2 gas. It also exhibits excellent cyclic stability. Further, the developed 15% CsxWO3/MoS2 nanocomposite demonstrated a superior H2 gas sensing response at room temperature compared to reported H2 gas sensors. The enhanced sensing response could be because of the improved electrical properties of the 15% CsxWO3/MoS2 nanocomposite. Therefore, this study facilitates the development of an effective and efficient H2 gas sensor at room temperature, which will play a crucial role in the detection of hydrogen gas in the environment.
Data Availability Statement
The original contributions presented in the study are included in the article/Supplementary Material, further inquiries can be directed to the corresponding author.
Author Contributions
C-MW: Supervision, conceptualization and reviewing and editing. Shrisha: Methodology, data curation and writing. KM: Review and editing. G-YC: Methodology and data curation. D-HK: Resources. NG: Methodology.
Conflict of Interest
The authors declare that the research was conducted in the absence of any commercial or financial relationships that could be construed as a potential conflict of interest.
Publisher’s Note
All claims expressed in this article are solely those of the authors and do not necessarily represent those of their affiliated organizations, or those of the publisher, the editors and the reviewers. Any product that may be evaluated in this article, orclaim that may be made by its manufacturer, is not guaranteed or endorsed by the publisher.
Acknowledgments
We would like to acknowledge the Ministry of Science and Technology of Taiwan, ROC for financially supporting part of this work, under contract numbers: MOST 110-2622-E-011-023.
Supplementary Material
The Supplementary Material for this article can be found online at: https://www.frontiersin.org/articles/10.3389/fmats.2022.831725/full#supplementary-material
References
Ahmadi, M., Younesi, R., and Guinel, M. J.-F. (2014). Synthesis of Tungsten Oxide Nanoparticles Using a Hydrothermal Method at Ambient Pressure. J. Mater. Res. 29, 1424–1430. doi:10.1557/jmr.2014.155
Akbari, E., Jahanbin, K., Afroozeh, A., Yupapin, P., and Buntat, Z. (2018). Brief Review of Monolayer Molybdenum Disulfide Application in Gas Sensor. Physica B: Condensed Matter 545, 510–518. doi:10.1016/j.physb.2018.06.033
Anand, K., Singh, O., Singh, M. P., Kaur, J., and Singh, R. C. (2014). Hydrogen Sensor Based on graphene/ZnO Nanocomposite. Sensors Actuators B: Chem. 195, 409–415. doi:10.1016/j.snb.2014.01.029
Annanouch, F. E., Martini, V., Fiorido, T., Lawson, B., Aguir, K., and Bendahan, M. (2021). Embedded Transdermal Alcohol Detection via a Finger Using SnO2 Gas Sensors. Sensors 21, 6852. doi:10.3390/s21206852
Barua, S., Dutta, H. S., Gogoi, S., Devi, R., and Khan, R. (2017). Nanostructured MoS2-Based Advanced Biosensors: A Review. ACS Appl. Nano Mater. 1, 2–25. doi:10.1021/acsanm.7b00157
Bekena, F. T., Abdullah, H., Kuo, D.-H., and Zeleke, M. A. (2019). Photocatalytic Reduction of 4-nitrophenol Using Effective Hole Scavenger over Novel Mg-Doped Zn(O,S) Nanoparticles. J. Ind. Eng. Chem. 78, 116–124. doi:10.1016/j.jiec.2019.06.029
Bello, I. T., Oladipo, A. O., Adedokun, O., and Dhlamini, S. M. (2020). Recent Advances on the Preparation and Electrochemical Analysis of MoS2-Based Materials for Supercapacitor Applications: A Mini-Review. Mater. Today Commun. 25, 101664. doi:10.1016/j.mtcomm.2020.101664
Castillo, C., Cabello, G., Chornik, B., Huentupil, Y., and Buono-Core, G. E. (2020). Characterization of Photochemically Grown Pd Loaded WO3 Thin Films and its Evaluation as Ammonia Gas Sensor. J. Alloys Compd. 825, 154166. doi:10.1016/j.jallcom.2020.154166
Chala, T., Wu, C.-M., Chou, M.-H., Gebeyehu, M., and Cheng, K.-B. (2017). Highly Efficient Near Infrared Photothermal Conversion Properties of Reduced Tungsten Oxide/polyurethane Nanocomposites. Nanomaterials 7, 191. doi:10.3390/nano7070191
Chang, C.-H., Chou, T.-C., Chen, W.-C., Niu, J.-S., Lin, K.-W., Cheng, S.-Y., et al. (2020). Study of a WO3 Thin Film Based Hydrogen Gas Sensor Decorated with Platinum Nanoparticles. Sensors Actuators B: Chem. 317, 128145. doi:10.1016/j.snb.2020.128145
Dong, C., Zhao, R., Yao, L., Ran, Y., Zhang, X., and Wang, Y. (2020). A Review on WO3 Based Gas Sensors: Morphology Control and Enhanced Sensing Properties. J. Alloys Compd. 820, 153194. doi:10.1016/j.jallcom.2019.153194
Duc, C., Boukhenane, M.-L., Wojkiewicz, J.-L., and Redon, N. (2020). Hydrogen Sulfide Detection by Sensors Based on Conductive Polymers: a Review. Front. Mater. 7, 215. doi:10.3389/fmats.2020.00215
Esfandiar, A., Ghasemi, S., Irajizad, A., Akhavan, O., and Gholami, M. R. (2012). The Decoration of TiO2/reduced Graphene Oxide by Pd and Pt Nanoparticles for Hydrogen Gas Sensing. Int. J. Hydrogen Energ. 37, 15423–15432. doi:10.1016/j.ijhydene.2012.08.011
Esfandiar, A., Irajizad, A., Akhavan, O., Ghasemi, S., and Gholami, M. R. (2014). Pd-WO3/reduced Graphene Oxide Hierarchical Nanostructures as Efficient Hydrogen Gas Sensors. Int. J. Hydrogen Energ. 39, 8169–8179. doi:10.1016/j.ijhydene.2014.03.117
Gottam, S. R., Tsai, C.-T., Wang, L.-W., Wang, C.-T., Lin, C.-C., and Chu, S.-Y. (2020). Highly Sensitive Hydrogen Gas Sensor Based on a MoS2-Pt Nanoparticle Composite. Appl. Surf. Sci. 506, 144981. doi:10.1016/j.apsusc.2019.144981
Ha, N. H., Thinh, D. D., Huong, N. T., Phuong, N. H., Thach, P. D., and Hong, H. S. (2018). Fast Response of Carbon Monoxide Gas Sensors Using a Highly Porous Network of ZnO Nanoparticles Decorated on 3D Reduced Graphene Oxide. Appl. Surf. Sci. 434, 1048–1054. doi:10.1016/j.apsusc.2017.11.047
Hienuki, S., Mitoma, H., Ogata, M., Uchida, I., and Kagawa, S. (2021). Environmental and Energy Life Cycle Analyses of Passenger Vehicle Systems Using Fossil Fuel-Derived Hydrogen. Int. J. Hydrogen Energ. 46, 36569–36580. doi:10.1016/j.ijhydene.2021.08.135
Huang, B.-R., and Lin, J.-C. (2012). Core-shell Structure of Zinc Oxide/indium Oxide Nanorod Based Hydrogen Sensors. Sensors Actuators B: Chem. 174, 389–393. doi:10.1016/j.snb.2012.08.065
Huaning, J., Huaizhang, W., and Ting, L. (2021). Research Progress of MoS2 Composite rGO Material in Gas Sensor. E3S Web Conferences: EDP Sci. 267, 02048. doi:10.1051/e3sconf/202126702048
Hübert, T., Boon-Brett, L., Palmisano, V., and Bader, M. A. (2014). Developments in Gas Sensor Technology for Hydrogen Safety. Int. J. Hydrogen Energ. 39, 20474–20483. doi:10.1016/j.ijhydene.2014.05.042
Kim, T., Lee, S., Cho, W., Kwon, Y. M., Baik, J. M., and Shin, H. (2021). Development of a Novel Gas-Sensing Platform Based on a Network of Metal Oxide Nanowire Junctions Formed on a Suspended Carbon Nanomesh Backbone. Sensors 21, 4525. doi:10.3390/s21134525
Li, Z., Meng, X., and Zhang, Z. (2018). Recent Development on MoS2-Based Photocatalysis: A Review. J. Photochem. Photobiol. C: Photochem. Rev. 35, 39–55. doi:10.1016/j.jphotochemrev.2017.12.002
Liang, L., Yang, Q., Zhao, S., Wang, L., and Liang, F. (2021). Excellent Catalytic Effect of LaNi5 on Hydrogen Storage Properties for Aluminium Hydride at Mild Temperature. Int. J. Hydrogen Energ. 46, 38733–38740. doi:10.1016/j.ijhydene.2021.09.130
Lin, T.-C., and Huang, B.-R. (2012). Palladium Nanoparticles Modified Carbon Nanotube/nickel Composite Rods (Pd/CNT/Ni) for Hydrogen Sensing. Sensors Actuators B: Chem. 162, 108–113. doi:10.1016/j.snb.2011.12.044
Liu, Y., Hao, L., Gao, W., Wu, Z., Lin, Y., Li, G., et al. (2015). Hydrogen Gas Sensing Properties of MoS2/Si Heterojunction. Sensors Actuators B: Chem. 211, 537–543. doi:10.1016/j.snb.2015.01.129
Masteghin, M. G., Godoi, D. R. M., and Orlandi, M. O. (2019). Heating Method Effect on SnO Micro-disks as NO2 Gas Sensor. Front. Mater. 6, 171. doi:10.3389/fmats.2019.00171
Miglietta, M., Alfano, B., Santos, J., Sayago, I., Polichetti, T., Massera, E., et al. (2020). “Outstanding NO2 Sensing Performance of Sensors Based on TiO2/Graphene Hybrid,” in Proceeding of the AISEM Annual Conference on Sensors and Microsystems, February 2020 (Springer), 349–355.
Mohamad, M., Mustafa, F., Abidin, M. S. Z., Abd Rahman, S. F., Al-Obaidi, N. K. A., Hashim, A. M., et al. (2010). “The Sensing Performance of Hydrogen Gas Sensor Utilizing Undoped-AlGaN/GaN HEMT,” in Proceeding of the 2010 IEEE International Conference on Semiconductor Electronics (ICSE2010), Malacca, Malaysia, June 2010 (IEEE). doi:10.1109/smelec.2010.5549369
Motora, K. G., and Wu, C.-M. (2020). Magnetically Separable Highly Efficient Full-Spectrum Light-Driven WO2.72/Fe3O4 Nanocomposites for Photocatalytic Reduction of Carcinogenic Chromium (VI) and Organic Dye Degradation. J. Taiwan Inst. Chem. Eng. 117, 123–132. doi:10.1016/j.jtice.2020.12.006
Okada, M., Ono, K., Yoshio, S., Fukuyama, H., and Adachi, K. (2019). Oxygen Vacancies and Pseudo Jahn-Teller Destabilization in Cesium-Doped Hexagonal Tungsten Bronzes. J. Am. Ceram. Soc. 102, 5386–5400. doi:10.1111/jace.16414
Park, S., Kim, S., Kheel, H., Park, S. E., and Lee, C. (2015). Synthesis and Hydrogen Gas Sensing Properties of TiO2-Decorated CuO Nanorods. Bull. Korean Chem. Soc. 36, 2458–2463. doi:10.1002/bkcs.10473
Phan, D.-T., and Chung, G.-S. (2014). Reliability of Hydrogen Sensing Based on Bimetallic Ni-Pd/graphene Composites. Int. J. Hydrogen Energ. 39, 20294–20304. doi:10.1016/j.ijhydene.2014.10.006
Phuruangrat, A., Ham, D. J., Thongtem, S., and Lee, J. S. (2009). Electrochemical Hydrogen Evolution over MoO3 Nanowires Produced by Microwave-Assisted Hydrothermal Reaction. Electrochemistry Commun. 11, 1740–1743. doi:10.1016/j.elecom.2009.07.005
Pisarkiewicz, T., Maziarz, W., Małolepszy, A., Stobiński, L., Michoń, D., and Rydosz, A. (2020). Multilayer Structure of Reduced Graphene Oxide and Copper Oxide as a Gas Sensor. Coatings 10, 1015. doi:10.3390/coatings10111015
Rydosz, A., Maziarz, W., Pisarkiewicz, T., Wincza, K., and Gruszczynski, S. (2014). “Deposition of Nanocrystalline Wo3 and CuO Thin Film in View of Gas Sensor Applications,” in Proceedings the Second International Conference on Technological Advances in Electrical, Electronics and Computer Engineering, Kuala Lumpur, Malaysia, March 2014.
Saravanan, A., Huang, B.-R., Kathiravan, D., and Prasannan, A. (2017). Natural Biowaste-Cocoon-Derived Granular Activated Carbon-Coated ZnO Nanorods: A Simple Route to Synthesizing a Core-Shell Structure and its Highly Enhanced UV and Hydrogen Sensing Properties. ACS Appl. Mater. Inter. 9, 39771–39780. doi:10.1021/acsami.7b11051
Saravanan, A., Huang, B.-R., Chu, J. P., Prasannan, A., and Tsai, H.-C. (2019). Interface Engineering of Ultrananocrystalline diamond/MoS2-ZnO Heterostructures and its Highly Enhanced Hydrogen Gas Sensing Properties. Sensors Actuators B: Chem. 292, 70–79. doi:10.1016/j.snb.2019.04.108
Sharma, B., and Kim, J.-S. (2018). Graphene Decorated Pd-Ag Nanoparticles for H2 Sensing. Int. J. Hydrogen Energ. 43, 11397–11402. doi:10.1016/j.ijhydene.2018.03.026
Singh, S., Deb, J., Sarkar, U., and Sharma, S. (2021). MoS2/WO3 Nanosheets for Detection of Ammonia. ACS Appl. Nano Mater. 4, 2594–2605. doi:10.1021/acsanm.0c03239
Singh, S., Dogra, N., and Sharma, S. (2020). A Sensitive H2S Sensor Using MoS2/WO3 Composite. Mater. Today Proc. 28, 8–10. doi:10.1016/j.matpr.2019.12.104
Sridhar, A., Ponnuchamy, M., Kumar, P. S., Kapoor, A., and Xiao, L. (2021). Progress in the Production of Hydrogen Energy from Food Waste: A Bibliometric Analysis. Int. J. Hydrogen Energ. doi:10.1016/j.ijhydene.2021.09.258
Sun, P., Sun, Y., Ma, J., You, L., Lu, G., Fu, W., et al. (2011). Synthesis of Novel SnO2/ZnSnO3 Core-Shell Microspheres and Their Gas Sensing Properities. Sensors Actuators B: Chem. 155, 606–611. doi:10.1016/j.snb.2011.01.017
Vadivelmurugan, A., Anbazhagan, R., Lai, J.-Y., and Tsai, H.-C. (2021). Paramagnetic Properties of Manganese Chelated on Glutathione-Exfoliated MoS2. Colloids Surf. A: Physicochemical Eng. Aspects 608, 125432. doi:10.1016/j.colsurfa.2020.125432
Wang, Z., Huang, S., Men, G., Han, D., and Gu, F. (2018). Sensitization of Pd Loading for Remarkably Enhanced Hydrogen Sensing Performance of 3DOM WO3. Sensors Actuators B: Chem. 262, 577–587. doi:10.1016/j.snb.2018.02.041
Wang, X., Miura, N., and Yamazoe, N. (2000). Study of WO3-Based Sensing Materials for NH3 and NO Detection. Sensors Actuators B: Chem. 66, 74–76. doi:10.1016/s0925-4005(99)00410-4
Wisitsoraat, A., Tuantranont, A., Comini, E., Sberveglieri, G., and Wlodarski, W. (2009). Characterization of N-type and P-type Semiconductor Gas Sensors Based on NiOx Doped TiO2 Thin Films. Thin Solid Films 517, 2775–2780. doi:10.1016/j.tsf.2008.10.090
Wu, C.-M., Naseem, S., Chou, M.-H., Wang, J.-H., and Jian, Y.-Q. (2019). Recent Advances in Tungsten-Oxide-Based Materials and Their Applications. Front. Mater. 6, 49. doi:10.3389/fmats.2019.00049
Wu, C.-M., Shrisha, C.-M., Motora, K. G., Kuo, D.-H., Lai, C.-C., Huang, B.-R., et al. (2021). Cesium Tungsten Bronze Nanostructures and Their Highly Enhanced Hydrogen Gas Sensing Properties at Room Temperature. Int. J. Hydrogen Energ. 46, 25752–25762. doi:10.1016/j.ijhydene.2021.05.064
Xiao, S., Liu, B., Zhou, R., Liu, Z., Li, Q., and Wang, T. (2018). Room-temperature H2 Sensing Interfered by CO Based on Interfacial Effects in Palladium-Tungsten Oxide Nanoparticles. Sensors Actuators B: Chem. 254, 966–972. doi:10.1016/j.snb.2017.07.169
Yang, X., Wang, W., Xiong, J., Chen, L., and Ma, Y. (2015). ZnO:Cd Nanorods Hydrogen Sensor with Low Operating Temperature. Int. J. Hydrogen Energ. 40, 12604–12609. doi:10.1016/j.ijhydene.2015.07.086
Yao, Y., Ao, K., Lv, P., and Wei, Q. (2019). MoS2 Coexisting in 1T and 2H Phases Synthesized by Common Hydrothermal Method for Hydrogen Evolution Reaction. Nanomaterials 9, 844. doi:10.3390/nano9060844
Zhang, X., Tang, H., Xue, M., and Li, C. (2014). Facile Synthesis and Characterization of Ultrathin MoS2 Nanosheets. Mater. Lett. 130, 83–86. doi:10.1016/j.matlet.2014.05.078
Zhang, W., Zhang, P., Su, Z., and Wei, G. (2015). Synthesis and Sensor Applications of MoS2-Based Nanocomposites. Nanoscale 7, 18364–18378. doi:10.1039/c5nr06121k
Keywords: CsxWO3/MoS2 nanocomposites, hydrogen gas, room temperature, sensors, metal oxide gas sensor
Citation: Wu C-M, Shrisha , Motora KG, Chen G-Y, Kuo D-H and Gultom NS (2022) Highly Efficient MoS2/CsxWO3 Nanocomposite Hydrogen Gas Sensors. Front. Mater. 9:831725. doi: 10.3389/fmats.2022.831725
Received: 08 December 2021; Accepted: 06 January 2022;
Published: 25 January 2022.
Edited by:
Mohammed M. Rahman, King Abdulaziz University, Saudi ArabiaReviewed by:
Raid Ismail, University of Technology, Iraq, IraqSanjit Manohar Majhi, United Arab Emirates University, United Arab Emirates
Franz Dickert, University of Vienna, Austria
Copyright © 2022 Wu, Shrisha, Motora, Chen, Kuo and Gultom. This is an open-access article distributed under the terms of the Creative Commons Attribution License (CC BY). The use, distribution or reproduction in other forums is permitted, provided the original author(s) and the copyright owner(s) are credited and that the original publication in this journal is cited, in accordance with accepted academic practice. No use, distribution or reproduction is permitted which does not comply with these terms.
*Correspondence: Chang-Mou Wu, Y213dUBtYWlsLm50dXN0LmVkdS50dw==