- Departamento de Genética, Facultad de Biología, Universidad Complutense de Madrid, Madrid, Spain
Chromatin Assembly Factor 1 (CAF-1) is an evolutionary conserved heterotrimeric chaperone complex that facilitates the incorporation of histones H3 and H4 onto newly synthesized DNA. We demonstrate here that the mutant deficient for the large subunit of the complex, fas1-4, and in minor extent, the mutant deficient for the middle subunit, fas2-1, display chromosome abnormalities throughout Arabidopsis mitosis. Among them, we observed multicentromeric chromosomes at metaphase, and chromatid bridges and acentric fragments at anaphase-telophase. 45S rDNA and telomeric sequences were frequently involved in bridges and fragments. Gene expression analysis by real-time qPCR has revealed that several genes related to homologous recombination (HR) and alternative non-homologous end-joining (aNHEJ) are overexpressed in fas1-4. These results concur with previous studies which have indicated that HR may be involved in the progressive loss of 45S rDNA and telomeres displayed by fas mutants. However, increased expression of PARP1, PARP2, and LIG6 in fas1-4, and the phenotype shown by the double mutant fas1 rad51 suggest that aNHEJ should also be responsible for the chromosomal aberrations observed. The activity of different DNA repair pathways in absence of CAF-1 is discussed.
Introduction
Histone chaperones are key regulators that participate in distinct steps of nucleosome assembly and histone exchange. They display essential roles during DNA replication and DNA repair (Eitoku et al., 2008; Das et al., 2010; Ransom et al., 2010; Burgess and Zhang, 2013). The Chromatin Assembly Factor 1 (CAF-1) is a heterotrimeric chaperone complex which facilitates the association and deposition of (H3-H4)2 histone tetramers onto newly synthesized DNA (Kaufman et al., 1995; Gaillard et al., 1996). Although CAF-1 is highly conserved, the consequences of its disruption are not identical among different organisms (Kaufman et al., 1997; Nabatiyan and Krude, 2004; Song et al., 2007). The complex is essential during the development in mouse and Drosophila, but yeast cells and plants with mutations affecting this complex are viable (Kaufman et al., 1997; Exner et al., 2006; Houlard et al., 2006; Song et al., 2007).
In Arabidopsis thaliana, FASCIATA 1 (FAS1) encodes the large subunit of CAF-1. It interacts directly with histone tetramers, and also with the DNA polymerase processivity factor namely PROLIFERATING CELL NUCLEAR ANTIGEN (PCNA). FASCIATA 2 (FAS2) and MULTICOPY SUPRESSOR OF IRA (MSI1) encode the other two subunits of the complex (Ramirez-Parra and Gutierrez, 2007a). fasciata mutants exhibit pleiotropic phenotypes that include fasciated stems, defective shoot apical meristems, disrupted leaf phyllotaxy, altered trichome differentiation, narrow and dentate leaves, and short roots (Leyser and Furner, 1992; Kaya et al., 2001; Exner et al., 2006). Cytological studies revealed that fas1 and fas2 mutants show an open chromatin conformation, as well as reduced heterochromatin content and dispersed pericentromeric DNA (Kirik et al., 2006; Schönrock et al., 2006). The mutants are hypersensitive to genotoxic agents and display an enhanced frequency of homologous recombination (HR) and T-DNA integration. They also present high levels of H2AX phosphorylation, a marker of DNA double-strand breaks (DSBs), and increased RAD51 expression compared with wild-type (WT) (Takeda et al., 2004; Endo et al., 2006; Kirik et al., 2006; Ono et al., 2006; Schönrock et al., 2006; Ramirez-Parra and Gutierrez, 2007b). Recently, it has been reported that in fas mutants there is also a specific and transgenerational loss of 45S rDNA and telomeric sequences (Mozgová et al., 2010; Jaške et al., 2013; Muchová et al., 2015; Pavlištová et al., 2016). The loss of these sequences is produced during the cell division and it does not occur during meiosis (Muchová et al., 2015; Varas et al., 2015). Additionally, CAF-1 deficiency probably leads to the activation of a G2 checkpoint that triggers the endocycle program (Ramirez-Parra and Gutierrez, 2007b). In fas mutants cell cycle arrest only occurs during post-meiotic pollen development resulting in formation of one sperm cell (Chen et al., 2008).
fas1-4 displays 3% of FAS1 mRNA expression levels and the truncated protein generated is unable to interact with PCNA and other subunits of the CAF-1 complex (Ramirez-Parra and Gutierrez, 2007a). It appears to be the strongest allele described to date since it exhibits the most severe developmental phenotype and ∼96-fold more intrachromosomal HR events than WT (Kaya et al., 2001; Kirik et al., 2006). This HR rate has also consequences in the repair of the DSBs generated during meiosis (Varas et al., 2015). Here, we have mainly focused our attention on the expression profiles of genes involved in different DNA repair pathways and the mitotic consequences derived from the absence of FAS1. Our results highlight that, in addition to HR, the non-homologous end-joining pathway (NHEJ) could be also involved in the enhanced genome instability displayed by fas1-4.
Materials and Methods
Plant Materials and Growth Conditions
The majority of the mutants analyzed corresponds to T-DNA insertion lines and belong to Columbia accession (Col-0). The exceptions are rad51-2, a T-DNA insertion line generated in Wassilewskija (Ws) background (Pradillo et al., 2012), and fas2-1, which has been generated by EMS in Landsberg erecta (Ler) background (Endo et al., 2006). The mutant alleles rad51-3 (SAIL_873_C08) and dmc1-2 (SAIL_170_F08) were obtained from the Salk Institute Genomic Analysis Laboratory (SiGnAL1; Alonso et al., 2003), and provided by the Nottingham Arabidopsis Stock Centre (NASC). Dr. Crisanto Gutiérrez kindly donated fas1-4 (SAIL_662_D10) and fas2-1 (Ramirez-Parra and Gutierrez, 2007b) mutant lines. Since there is a progressive transgenerational loss of some sequences in fas1-4 it is important to point out that the fas1-4 plants analyzed in this study were obtained from homozygous plants propagated during nine generations by selfing (G9). Plants were cultivated on a soil mixture of vermiculite and commercial soil (3:1) and grown in a green-house under a 16 h light/8 h dark photoperiod, at 18–20°C with 70% humidity. Plants were genotyped by PCR using primers listed in Supplementary Table S1.
Cytological Analysis
Fixation of flower buds, spreading preparations of somatic cells, and fluorescence in situ hybridization (FISH) were carried out according to Sánchez-Morán et al. (2001). The DNA probes used were: 45S rDNA (pTa71; Gerlach and Bedbrook, 1979), 5S rDNA (pCT4.2; Campell et al., 1992), centromeres (pAL1; Martínez-Zapater et al., 1986), and telomeres (pLT11; Richards and Ausubel, 1988). An Olympus BX-60 microscope equipped with an Olympus DP71 digital camera was used for cytological analysis. Images were processed with Adobe Photoshop CS5.
RNA Extraction and Real-Time Quantitative PCR
Total RNA was isolated from 10-day-old fas1-4 seedlings by RNeasy kit (Qiagen). Quantitative PCR was performed with the FastStart TaqMan Probe Master using Universal Probe Library (UPL) probes and specific primers designed by the UPL Assay Design Center2. Details about the primers and UPL probes are given in Supplementary Table S2. Relative quantification of mRNA was calculated over a calibrator, after normalization to ACTIN 2 by the standard curve method (Larionov et al., 2005). Three experimental replicates were carried out for each target gene.
DNA Damage Sensitivity Assays
Cisplatin [cis-diamminedichloroplatinum (II), CDDP] is a chemotherapy drug that causes intra-strands crosslinks in the DNA (Eastman, 1985). During DNA replication, some of these links produce DSBs, which are preferentially repaired by HR (de Silva et al., 2002; Sasaki et al., 2004). To test the sensitivity to CDDP, surface-sterilized fas1-4 and Col-0 seeds were maintained in sterile water at 4°C for approximately 24 h, and then were sown on plates containing MS agar medium with different CDDP concentrations (0, 30, and 50 μM; Sigma). Values corresponding to number of leaves and percentage of germination were evaluated 14 days after sowing.
Most of the oxidative damage caused by hydrogen peroxide, H2O2, occurs around the sugar-phosphate backbone in the DNA structure and base excision repair (BER) is the major pathway involved in the repair of this damage (Slupphaug et al., 2003). To check the sensitivity to oxidative damage, 10-day-old fas1-4 seedlings were immersed in MS liquid medium with increasing concentrations of H2O2 (0, 2.5, 5, 10, and 20 mM). The fresh weight of the plants was quantified 6 days after treatment.
Statistical Analyses
Statistical analyses were managed with the software SPSS Statistics 17.0. Qualitative variables are shown as percentages and quantitative variables as mean ± standard error. Chi-squared and Student’s t-test were used to compare qualitative and quantitative variables, respectively. To evaluate the relative gene expression differences between mutant and WT plants, 95% confidence intervals were defined for the average expression of each gene.
Results
Seed Germination and Flowering Are Delayed in fas1-4
Differences in developmental transitions between fas1-4 and WT plants were exemplified by analyzing seed germination and flowering (Table 1). Differences in germination were evident 4 days after sowing, and at the ninth day there were about 20% of non-germinated fas1-4 seeds (n = 300). About 80% of WT plants showed the first inflorescences 31 days after sowing, whereas only 42% of mutant plants present them at this time. Since seed germination and flowering are two critical processes coordinately regulated by genetic and environmental factors, we wonder whether the delay showed by fas1-4 in the parameters mentioned was related to alterations during the mitotic division.
fas1-4 Displays Chromosomal Aberrations during Mitotic Division
To investigate possible defects during mitosis in fas1-4, this division was cytologically analyzed in DAPI-stained chromosome spreads of somatic cells from immature flower buds. This material provides a high metaphase index and is more suitable than root tips in which there are often less than 10 metaphases. In WT plants, mitotic chromosomes are individualized at prometaphase (Figure 1A), and their morphology is clearly defined at metaphase (Figure 1B). Chromatids segregate to opposite poles at anaphase (Figure 1C), and full migration is achieved at telophase (Figure 1D). However, altered mitotic stages were observed in fas1-4 cells. Chromosomes at prometaphase appeared less condensed (Figure 1E), 26.31% of the metaphases displayed interchromosomal connections (Figure 1F) and chromosome bridges were subsequently observed at anaphase-telophase (Figures 1G,H and Table 2). To gain insight into these mitotic defects we applied FISH with probes to localize centromeres, telomeres, 45S rDNA, and 5S rDNA sequences. The most relevant results obtained were: (i) there is an intercellular variation in the number of multicentromeric chromosomes at metaphase in fas1-4; (ii) chromosome fusions generate mostly dicentric chromosomes; (iii) 45S rDNA and telomeres are frequently involved in the bridges and fragments observed at anaphase (Figures 1I–P and Supplementary Figures S1, S2). To determine whether the mitotic phenotype observed in fas1-4 appears in other mutants deficient for the CAF-1 complex, we also analyzed mitotic cells in fas2-1 and observed similar abnormalities to those described above (Table 2 and Supplementary Figure S3). The fact that fas mutants suffer ongoing genome destabilization may contribute to the delay observed in the vegetative growth.
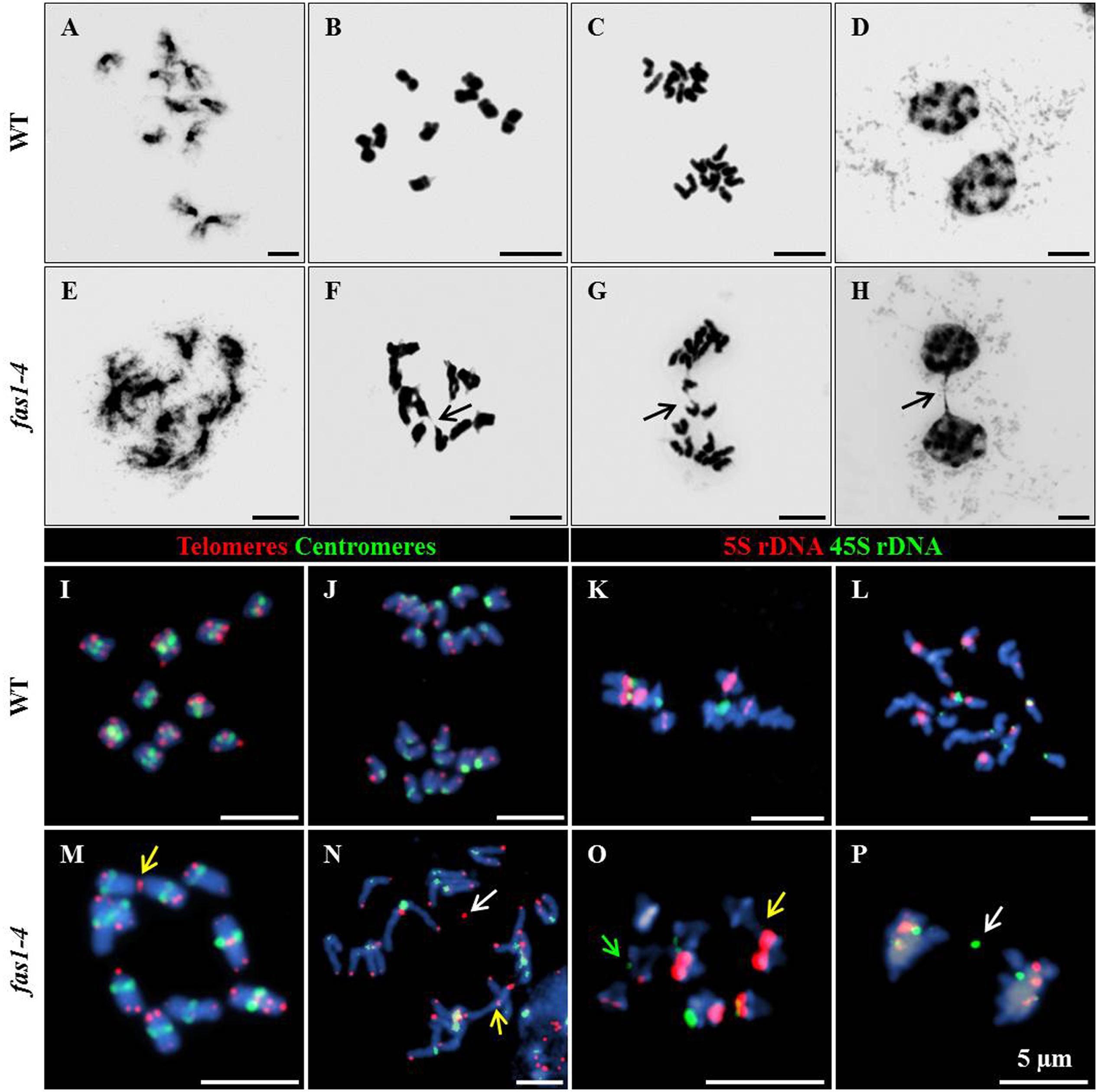
FIGURE 1. fas1-4 cells display defects during mitosis. DAPI-stained chromosome spreads and FISH were performed in somatic cells from young buds of WT (A–D,I–L) and fas1-4 (E–H,M–P). The images show the different mitotic stages: (A,E) prometaphase; (B,F,I,M,K,O) metaphase; (C,G,J,L,N,P) anaphase; (D,H) telophase. Arrows in F–H point to (F) sticky chromosomes, and (G,H) chromosome bridges. FISH probes detect (I,J,M,N) telomeres (red) and centromeres (green) or (K,L,O,P) 5S rDNA (red) and 45S rDNA (green). Yellow arrows denote end-to-end chromosome fusions, white arrows indicate chromosome fragments and the green arrow marks a reduced 45S rDNA region. Scale bars represent 5 μm.
In order to characterize the different DNA repair pathways that could be induced in fas1-4 and to get possible explanations for the mitotic abnormalities mentioned above, we conducted different methodological approaches: expression analyses of genes involved in different DNA repair pathways; genetic analyses by crossing fas1-4 with mutants defective for the recombinases RAD51 and the meiotic-specific DMC1; and DNA damage sensitivity assays.
Genes Involved in Different DNA Repair Pathways Are Differentially Expressed in fas1-4
Pivotal genes in HR such as RAD50 (involved in the resection of DSBs), ATM (kinase that produces a phosphorylation-mediated signal transduction cascade that leads to the repair of DSBs), BRCA1 (essential for RAD51 recruitment to sites of DNA damage), RAD51C (a RAD51 paralog), RAD51 (the main recombinase involved in HR), MND1 (assists RAD51 in the strand exchange) and SMC6A and SMC6B (both promote chromatid cohesion after DNA breakage and facilitates HR) were significantly overexpressed in fas1-4. Specifically, mRNA levels presented the following fold-changes: 1.42 ± 0.08 (RAD50), 1.62 ± 0.09 (ATM), 1.73 ± 0.10 (BRCA1), 1.64 ± 0.11 (RAD51C), 2.59 ± 0.08 (RAD51), 1.76 ± 0.06 (MND1), 1.20 ± 0.04 (SMC6A), and 2.05 ± 0.15 (SMC6B). Other genes involved in this DNA repair pathway (MRE11, NBS1, COM1, ATR, BRCA2B, and AHP2) showed high mRNA levels in fas1-4 seedlings, but they were not statistically significant from those observed in WT seedlings (Figure 2).
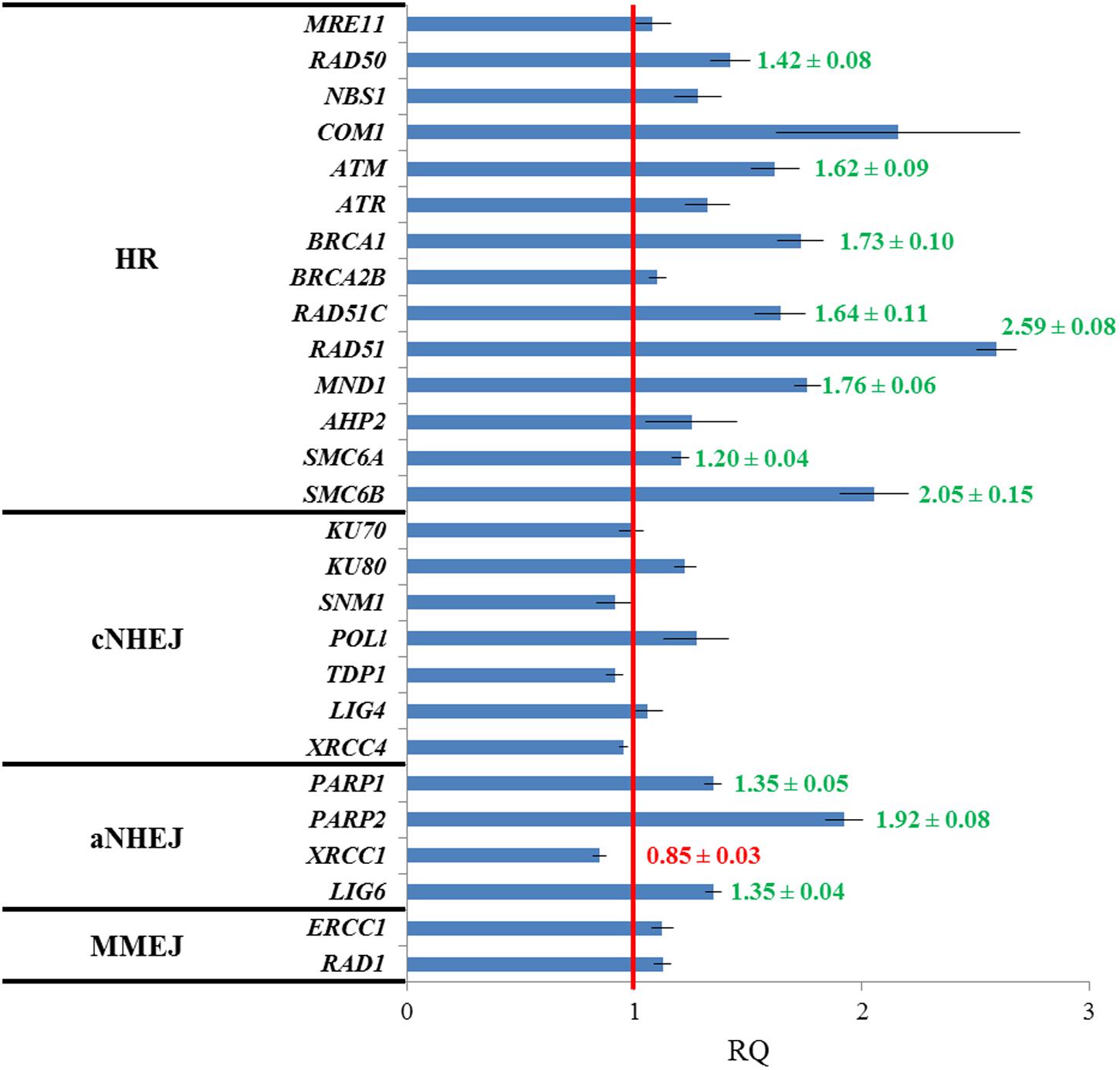
FIGURE 2. Genes involved in HR and aNHEJ display different expression profiles in fas1-4 respect to WT seedlings. Values are the average of three technical replicates. The red line is the reference for the fold-change respect to the WT after normalization to ACTIN 2 expression. Genes analyzed have been grouped into four categories: HR, cNHEJ, aNHEJ, and MMEJ. Overexpressed genes are highlighted in green and the underexpressed gene appears in red. Numbers corresponding to fold-changes are only displayed for significant differences. RQ, relative quantity.
Neither genes involved in the classical NHEJ (cNHEJ) nor in microhomology-mediated DNA end-joining (MMEJ), a pathway in which DNA repair is achieved using microhomologies localized at both sides of the break (McVey and Lee, 2008; Mladenov and Iliakis, 2011), displayed alterations in their expression. However, we detected significant overexpression of some of the genes involved in an alternative KU-independent pathway, alternative NHEJ (aNHEJ), which does not require the presence of pre-existing microhomologies and may rather rely on LIG1 and LIG6. Main actors in aNHEJ are the poly (ADP-ribose) polymerases PARP1 and PARP2 and XRCC1, which encodes a protein that acts as scaffold to other DNA repair proteins (Audebert et al., 2008; Charbonnel et al., 2010; Jia et al., 2013; Tallis et al., 2014). We detected statistically higher mRNA levels for PARP1 (1.35 ± 0.05), PARP2 (1.92 ± 0.08) and LIG6 (1.35 ± 0.04), although XRCC1 was slightly underexpressed (0.85 ± 0.03) (Figure 2).
Mitotic Aberrations in fas1-4 Are Aggravated in Combination with rad51 Deficient Alleles
To investigate a possible contribution of HR to the mentioned fas1-4 mitotic defects, three fas1-4 double mutants were analyzed: fas1-4 rad51-2 (rad51-2 is a knockdown allele, KD), fas1-4 rad51-3 (rad51-3 is a knockout allele, KO) and fas1-4 dmc1-2 (dmc1-2 is a KO allele). RAD51 is an essential protein involved in both mitotic and meiotic DSB repair by HR, whereas DMC1 is a recombinase involved exclusively in meiotic HR. The results obtained confirmed a more drastic phenotype in double mutants involving rad51 alleles, with smaller leaves and a more pronounced developmental delay respect to the single mutant fas1-4 (Figures 3A,E). The percentage of chromosome aberrations of fas1-4 dmc1-2 cells at metaphase and anaphase-telophase was similar to that of fas1-4 (Table 2 and Supplementary Figure S3, Tables S3–S6). This was an expected result since DMC1 is the meiotic-specific recombinase. However, the percentage of mitotic abnormalities increased significantly in both fas1-4 rad51-3 (Figures 3F,G,J,K,N,O and Supplementary Figure S4) and fas1-4 rad51-2 respect to fas1-4 (Figures 3H,I,L,M,P,Q, Table 2, and Supplementary Figure S5, Tables S3–S6). Surprisingly, the phenotype of the double mutant with the KD allele rad51-2 was more pronounced than that of the double mutant with the KO allele rad51-3 (Figures 3D,E), which could be attributed to the different genetic backgrounds (the mutant lines rad51-2 and rad51-3 belong to Ws and Col-0, respectively). In 20% of the anaphases analyzed in these double mutants, 45S rDNA and telomeric sequences were present in bridges and acentric fragments (Figures 3O–Q).
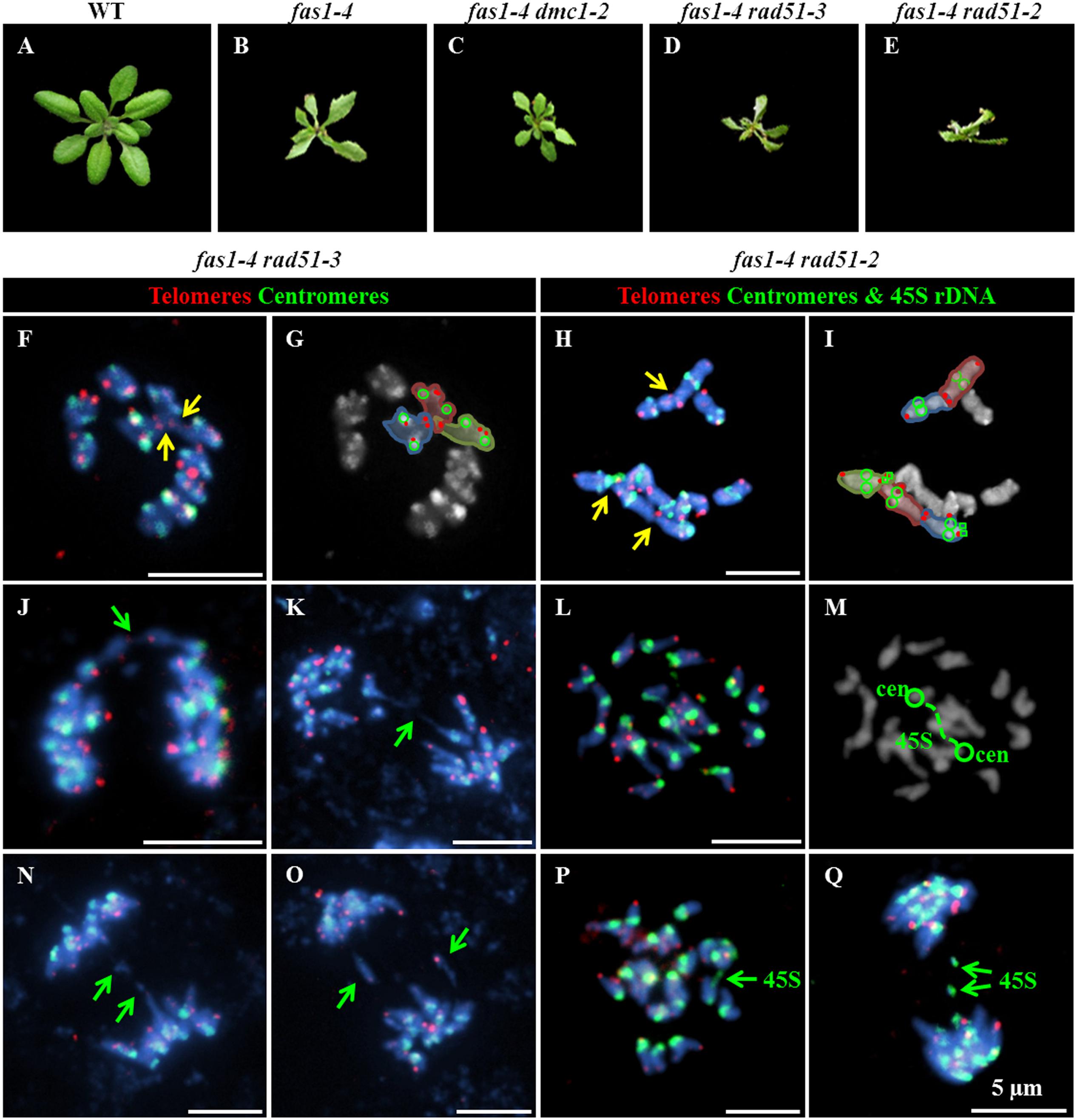
FIGURE 3. Absence of RAD51 aggravates the phenotype of fas1-4. Basal rosettes 30 days after sowing in (A) WT; (B) fas1-4; (C) fas1-4 dmc1-2; (D) fas1-4 rad51-3; and (E) fas1-4 rad1-2 plants. FISH using telomeric (red), centromeric (green) and 45S rDNA (green) probes in (F–I) metaphase and (J–Q) anaphase cells from (F,G,J,K,N,O) fas1-4 rad51-3; and (H,I,L,M,P,Q) fas1-4 rad1-2 plants. Yellow arrows point to end-to-end chromosome fusions; and green arrows denote either chromosome fragments or anaphase bridges. Scale bars represent 5 μm.
DNA Damage Sensitivity Assays
The increase in the percentage of mitotic alterations observed when the HR pathway was non-functional could be explained by the overexpression of some aNHEJ genes. Since PARP genes participate in both aNHEJ and BER (Gottlich et al., 1998), we decided to perform two genotoxicity assays to induce either DSBs or single-strand DNA nicks in both fas1-4 and WT plants. As fas1-4 showed a slower development and lower germination rate than WT (Table 1), we relativized the values of these parameters in relation to those shown by untreated plants. Results obtained revealed that fas1-4 was more sensitive to CDDP than WT (Figure 4A). This difference was higher at enhanced doses for both parameters evaluated: germination rate (Figure 4B; 30 μM: = 5.005, P = 0.025; 50 μM: = 12.297, P < 0.0001) and number of leaves per plant (Figure 4C; 30 μM: = 10.793, P = 0.001; 50 μM: = 5.623; P = 0.017). However, fas1-4 seedlings were not hypersensitive to H2O2 (Figures 4D,E).
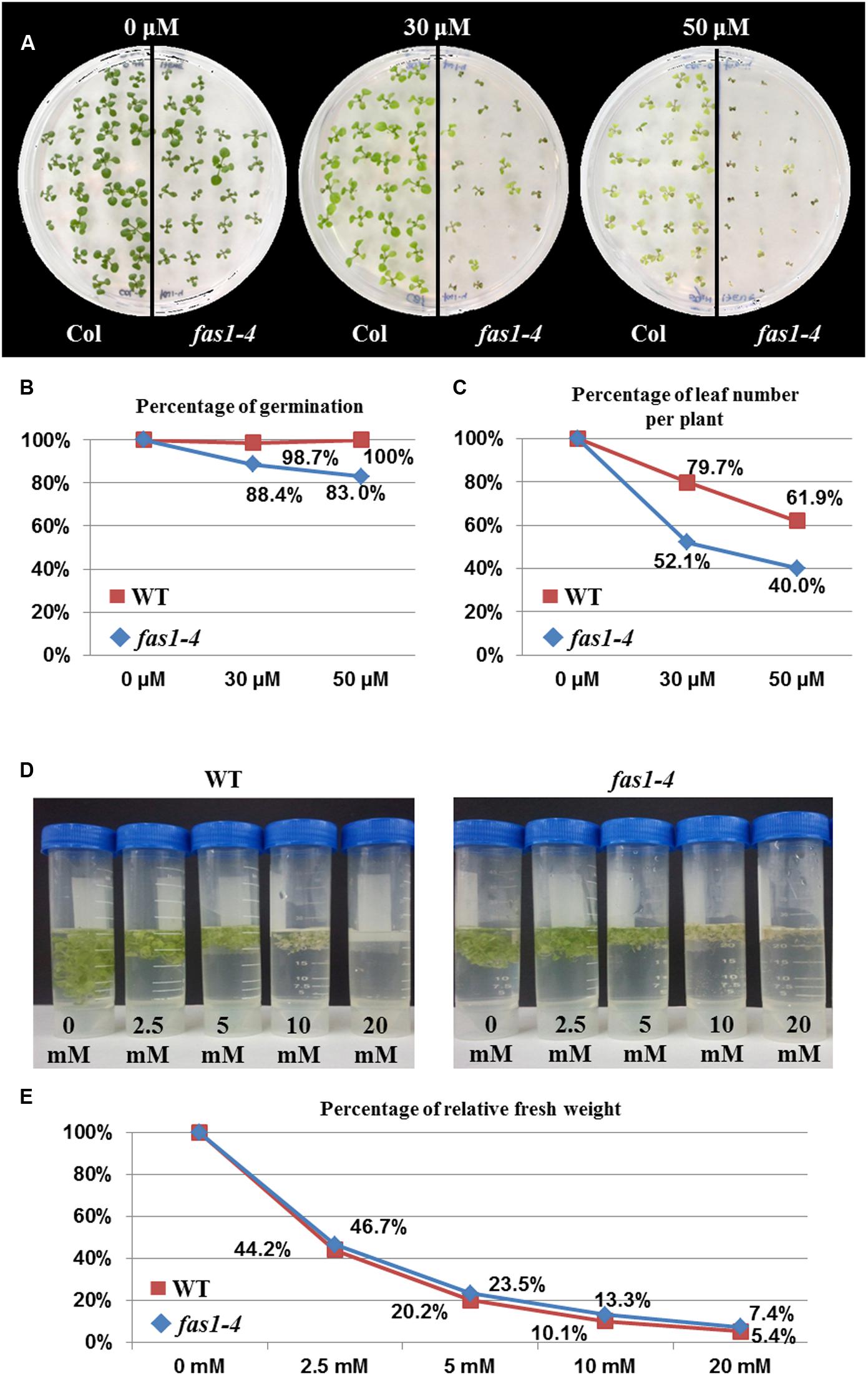
FIGURE 4. Absence of FAS1 increases sensitivity to CDDP but not to H2O2. (A) Phenotypes of 14-day-old seedlings (fas1-4 and WT) sown on media containing different concentrations of CDDP. (B) Percentages of germination. (C) Leaf number per plant after treatments with different concentrations of CDDP. The numbers at each dose were scored and put into relation to the leaf numbers of the untreated plantlets of the same line. (D) Phenotypes of 16-day-old seedlings (fas1-4 and WT) 6 days after immersion on liquid media containing different concentrations of H2O2. (E) Percentage of relative fresh weight of the plants 6 days after treatments with different concentrations of H2O2.
Discussion
Somatic Development in fas1-4 Mutants
The results presented here stress in the generalized somatic developmental delay and growth defects of fas1-4 mutants and reveal that they could be partially due to mitotic abnormalities (Figures 1, 3A,B and Table 2). Additionally, it has been reported that the absence of CAF-1 produces an accumulation of γH2AX histone variant, which marks DSBs (Endo et al., 2006; Amiard et al., 2013). Indeed, fas mutants have hypersensitivity to DNase I, γ rays, Zeocin, MMS, UV radiation, mitomycin C and bleomycin (Lowndes and Toh, 2005; Endo et al., 2006; Ono et al., 2006; Ramirez-Parra and Gutierrez, 2007b). Here we add new evidence of enhanced sensitivity of fas1-4 to cisplatin (Figure 4A). This increase in genome instability should be specific to the presence of DSBs and not to single-stranded breaks, since the mutant does not differ from WT in its sensitivity to H2O2 (Figures 4D,E). Consistent with this hypothesis, the absence of the main recombinase involved in HR, RAD51, exacerbates the developmental problems displayed by fas1-4 (Figure 3). Surprisingly, this does not occur when other HR proteins, as RAD51B, are depleted (Muchová et al., 2015).
Reparation of DSBs Generated as Consequence of Deficient CAF-1 Activity
In eukaryotes, DSBs are mainly repaired by two major pathways: HR and canonical NHEJ. HR predominates in the mid-S and G2 cell cycle phases and requires DNA sequence homology (San Filippo et al., 2008). On the contrary, cNHEJ occurs throughout the cell cycle although is dominant in G0/G1 and G2, and contributes to the repair of DSBs by blunt end ligation independently of sequence homology (Lieber, 2010). Absence of CAF-1 activity leads to genomic instability as consequence of nucleosome assembly failures. In this scenario, chromatin fibers would be more susceptible to mechanical stress and DNA damage (Kaya et al., 2001). Specifically, the increase in somatic HR produced in fas1-4 would be mainly due to high levels of DSBs derived from stalled DNA replication forks (Gao et al., 2012). Alternatively, it has been proposed that histone loss enhances chromatin dynamics and recombination rates (Hauer et al., 2017).
The importance of HR in the repair of DSBs generated in fas1-4 is evidenced by two facts. Firstly, by the overexpression of several genes with functions in this pathway (Figure 2). Indeed, the highest mRNA levels correspond to RAD51, the main gene involved in HR. Secondly, by the increase in the frequencies of mitotic alterations observed in fas1-4 rad51 (where RAD51 is partially or completely absent) respect to fas1-4 (Table 2 and Supplementary Tables S3–S5). In this sense, the RAD51 overexpression generated in fas1-4 is not enough to solve the genomic instability produced by the absence of the chaperone, since mitotic alterations are observed (Figure 3). It is noteworthy the difference in the somatic phenotype between fas1-4 rad51-2 and fas1-4 rad51-3 (Figure 3). The presence of a small amount of RAD51 protein in fas1-4 rad51-2 has more drastic consequences that the complete blocking of HR in fas1-4 rad51-3 (Figures 4D,E). This reveals that other DNA pathways (either alternative to HR or negatively regulated by HR) could be contributing to the repair of DNA intermediates generated in fas1-4.
Classical non-homologous end-joining, a pathway in which the DSB is repaired without much sequence loss and without microhomologies at the junction, might constitute a potential candidate to explain the presence of multicentromeric chromosomes that will lead to the formation of anaphase bridges. However, genes involved in this pathway such as KU70, KU80 and LIG4 did not show overexpression respect to WT in fas1-4. On the contrary, genes with a role in aNHEJ such as PARP1, PARP2, and LIG6 were overexpressed (Figure 2). Through this pathway broken ends are processed until a small number of identical nucleotides are complementary in both strands. The overexpression of PARP1 was reported previously by Schönrock et al. (2006), but was only related to the activation of a G2 checkpoint. Both PARP genes are also potentially involved in BER (Gottlich et al., 1998). However, this pathway seems to be unaffected in fas1-4 plants (Figures 4C,D).
Additionally, Charbonnel et al. (2010) described the existence of a third NHEJ pathway, XRCC1-dependent, named microhomology-mediated end-joining or MMEJ that operates in Arabidopsis. This pathway relies on pre-existing microhomologies around the DSB and is likely to operate through a mechanism related to single strand-annealing (SSA). The same authors have proposed the existence of a hierarchical organization of DSB repair in G2/M nuclei in such a way that cNHEJ acts prior aNHEJ, which can also inhibit MMEJ as seems to occur. In this context, the overexpression of genes involved in aNHEJ together with XRCC1 underexpression (Figure 2) could reflect the disruption of this hierarchical organization of DSB repair in fas1-4.
Deficient CAF-1 Activity and Loss of Repetitive Sequences
In human cells, it has been demonstrated that 45S rDNA repeats but not 5S rDNA repeats are highly sensitive to breaks produced by endonucleases (Warmerdam et al., 2016). NHEJ is the major pathway involved in the repair of these breaks generated in 45S repeat sequences (Harding et al., 2015), although HR is also implicated (van Sluis and McStay, 2015). In these regions, HR could act as an error-prone mechanism if there is an incorrect alignment that originates gain or loss of repeats and structural maintenance chromosome proteins (SMCs) would contribute to this HR-mediated repair (Warmerdam et al., 2016). In this sense, it has been proposed that the progressive loss of 45S rDNA that happens in successive generations in the Arabidopsis fas1-4 mutant is also HR-mediated, presumably by SSA, since fas1 rad51b mutants show a decrease in the rate of rDNA loss (Muchová et al., 2015). However, our results have revealed that fas1-4 rad51-2 and fas1-4 rad51-3 present an increase in the number of mitotic abnormalities, with the subsequent loss of rDNA 45S, and more severe developmental defects than the single mutant fas1-4 (Figure 3). These results highlight that other HR-independent DNA repair pathways could also be involved in the progressive loss of rDNA 45S sequences. According to RT qPCR analyses, aNHEJ could be one of these mechanisms (Figure 2). Curiously, this pathway is reminiscent of the SSA pathway of HR, since a particular amount of 3′-resection of the broken ends occurs, although the exonucleolytic enzyme complexes implicated are different. Regarding a possible role of SMC proteins in the repair of the breaks generated in the repeats, we have detected overexpression of both SMC6A and SMC6B in fas1-4 plants (Figure 2). In this context, it has been reported that SMC6A contribute to repair DSBs generated in mutants deficient for NHEJ (Kozak et al., 2008).
In relation to the loss of other repeats, 5S rDNA regions are unaffected in fas1-4. However, there is a loss of telomere sequences, although it is produced by a different mechanism from that responsible for the loss of 45S rDNA repeats (Muchová et al., 2015). Jaške et al. (2013), after analyzing single tert (telomerase reverse transcriptase) and fas mutants, and the corresponding double mutants, concluded that the progressive loss of telomeric DNA along generations in fas mutants is partially due to a suboptimal function of telomerase, but they also assumed that a further mechanism that contributes to telomere shortening in fas mutants must exist. The existence of telomeric acentric fragments in fas1-4 anaphases (Figure 1N) and the enhanced frequency of these fragments in the double mutant fas1-4 rad51 (Figure 3) suggest the involvement of a RAD51-independent mechanism in telomere shortening.
Conclusion
Our results suggest that the genome instability produced in fas1-4 is counteracted by, at least, two different DNA repair pathways: one RAD51-dependent that uses the sister chromatid as template, and another one that is error-prone and dependent on PARP proteins (aNHEJ). This error prone repair pathway could lead to the formation of multicentromeric chromosomes, which are clearly observed at mitotic metaphase in the mutant. Further studies would be required to decipher the specific contribution of these DNA repair pathways, not only in situations in which nucleosome dynamics is affected but also when chromatin conformation is unaffected.
Author Contributions
JV completed the experiments and performed data analyses. All authors conceived and designed the experiments, and wrote and reviewed the manuscript.
Funding
Authors acknowledge the support of the Ministry of Economy and Competitiveness of Spain (by grants AGL2012-38852 and AGL2015-67349-P) and of the European Union (Marie Curie ITN, COMREC 606956).
Conflict of Interest Statement
The authors declare that the research was conducted in the absence of any commercial or financial relationships that could be construed as a potential conflict of interest.
Acknowledgments
We would like to thank Bianca Martín, M. Carmen Moreno, and José Barrios for technical assistance, and Crisanto Gutiérrez and Elena Ramírez Parra for providing fas seeds. We also would like to thank Charles White and María E. Gallego for sharing the H2O2 genotoxicity protocol and for their helpful opinions and constructive criticisms.
Supplementary Material
The Supplementary Material for this article can be found online at: http://journal.frontiersin.org/article/10.3389/fpls.2017.00525/full#supplementary-material
Footnotes
References
Alonso, J. M., Stepanova, A. N., Leisse, T. J., Kim, C. J., Chen, H., Shinn, P., et al. (2003). Genome-wide insertional mutagenesis of Arabidopsis thaliana. Science 301, 653–657. doi: 10.1126/science.1086391
Amiard, S., Gallego, M. E., and White, C. I. (2013). Signaling of double strand breaks and deprotected telomeres in Arabidopsis. Front. Plant Sci. 4:405. doi: 10.3389/fpls.2013.00405
Audebert, M., Salles, B., and Calsou, P. (2008). Effect of double-strand break DNA sequence on the PARP-1 NHEJ pathway. Biochem. Biophys. Res. Commun. 369, 982–988. doi: 10.1016/j.bbrc.2007.11.132
Burgess, R. J., and Zhang, Z. (2013). Histone chaperones in nucleosome assembly and human disease. Nat. Struct. Mol. Biol. 20, 14–22. doi: 10.1038/nsmb.2461
Campell, B. R., Song, Y., Posch, T. E., Cullis, C. A., and Town, C. D. (1992). Sequence and organization of 5S ribosomal RNA-encoding genes of Arabidopsis thaliana. Gene 112, 225–228. doi: 10.1016/0378-1119(92)90380-8
Charbonnel, C., Gallego, M. E., and White, C. (2010). Xrcc1-dependent and Ku-dependent DNA double-strand break repair kinetics in Arabidopsis plants. Plant J. 64, 280–290. doi: 10.1111/j.1365-313X.2010.04331.x
Chen, Z., Tan, J. L. H., Ingouff, M., Sundaresan, V., and Berger, F. (2008). Chromatin assembly factor 1 regulates the cell cycle but not cell fate during male gametogenesis in Arabidopsis thaliana. Development 135, 65–73. doi: 10.1242/dev.010108
Das, C., Tyler, J. K., and Churchill, M. E. A. (2010). The histone shuffle: histone chaperones in an energetic dance. Trends Biochem. Sci. 35, 476–489. doi: 10.1016/j.tibs.2010.04.001
de Silva, I. U., McHugh, P. J., Clingen, P. H., and Hartley, J. A. (2002). Defects in interstrand cross-link uncoupling do not account for the extreme sensitivity of ERCC1 and XPF cells to cisplatin. Nucleic Acids Res. 30, 3848–3856. doi: 10.1093/nar/gkf479
Eastman, A. (1985). Interstrand cross-links and sequence specificity in the reaction of cis-dichloro(ethylenediamine)platinum(II) with DNA. Biochemistry 24, 5027–5032. doi: 10.1021/bi00340a011
Eitoku, M., Sato, L., Senda, T., and Horikoshi, M. (2008). Histone chaperones: 30 years from isolation to elucidation of the mechanisms of nucleosome assembly and disassembly. Cell Mol. Life. Sci. 65, 414–444. doi: 10.1007/s00018-007-7305-6
Endo, M., Ishikawa, Y., Osakabe, K., Najayama, S., Kaya, H., Araki, T., et al. (2006). Increased frequency of homologous recombination and T-DNA integration in Arabidopsis CAF-1 mutants. EMBO J. 25, 5579–5590. doi: 10.1038/sj.emboj.7601434
Exner, V., Taranto, P., Schönrock, N., Gruissem, W., and Hennig, L. (2006). Chromatin assembly factor CAF-1 is required for cellular differentiation during plant development. Development 133, 4163–4172. doi: 10.1242/dev.02599
Gaillard, P. H., Martini, E. M., Kaufman, P. D., Stillman, B., Moustacchi, E., and Almouzni, G. (1996). Chromatin assembly coupled to DNA repair: a new role for chromatin assembly factor I. Cell 86, 887–896. doi: 10.1016/S0092-8674(00)80164-6
Gao, J., Zhu, Y., Zhou, W., Molinier, J., Dong, A., and Shen, W. H. (2012). NAP1 family histone chaperones are required for somatic homologous recombination in Arabidopsis. Plant Cell 24, 1437–1447. doi: 10.1105/tpc.112.096792
Gerlach, W. L., and Bedbrook, J. R. (1979). Cloning and characterization of ribosomal RNA genes from wheat and barley. Nucleic Acids Res. 7, 1869–1885. doi: 10.1093/nar/7.7.1869
Gottlich, B., Reincherberger, S., Feldmann, E., and Pleiffer, P. (1998). Rejoining of DNA double-strand breaks in vitro by single-strand annealing. Eur. J. Biochem. 258, 387–395. doi: 10.1046/j.1432-1327.1998.2580387.x
Harding, S. M., Boiarsky, J. A., and Greenberg, R. A. (2015). ATM dependent silencing links nucleolar chromatin reorganization to DNA damage recognition. Cell Rep. 13, 251–259. doi: 10.1016/j.celrep.2015.08.085
Hauer, M. H., Seeber, A., Singh, V., Thierry, R., Sack, R., Amitai, A., et al. (2017). Histone degradation in response to DNA damage enhances chromatin dynamics and recombination rates. Nat. Struct. Mol. Biol. 24, 99–107. doi: 10.1038/nsmb.3347
Houlard, M., Berlivet, S., Probst, A. V., Quivy, J. P., Hery, P., Almouzni, G., et al. (2006). CAF-1 is essential for heterochromatin organization in pluripotent embryonic cells. PLoS Genet. 2:e181. doi: 10.1371/journal.pgen.0020181
Jaške, K., Mokros, P., Mozgová, I., Fojtová, M., and Fajkus, J. (2013). A telomerase –independent component of telomere loss in chromatin assembly factor 1 mutants of Arabidopsis thaliana. Chromosoma 122, 285–293. doi: 10.1007/s00412-013-0400-6
Jia, Q., den Duclk-Ras, A., Shen, H., Hooykaas, P. J., and de Pater, S. (2013). Poly(ADP-ribose)polymerases are involved in microhomology mediated back-upnon-homologous end joining in Arabidopsis thaliana. Plant Mol. Biol. 82, 339–351. doi: 10.1007/s11103-013-0065-9
Kaufman, P., Kobayashi, R., and Stillman, B. (1997). Ultraviolet radiation sensitivity and reduction of telomere silencing Saccharomyces cerevisiae cells lacking chromatin assembly factor-I. Genes Dev. 11, 345–357. doi: 10.1101/gad.11.3.345
Kaufman, P. D., Kobayashi, R., Kessler, N., and Stillman, B. (1995). The p150 and p60 subunits of chromatin assembly factor 1: a molecular link between newly synthesized histones and DNA replication. Cell 81, 1105–1114. doi: 10.1016/S0092-8674(05)80015-7
Kaya, H., Sibahara, K. I., Taoka, M., Iwabuchi, B., Stillman, T., and Araki, T. (2001). FASCIATA genes for chromatin assembly factor-1 in Arabidopsis maintain the cellular organization of apical meristems. Cell 104, 131–142. doi: 10.1016/S0092-8674(01)00197-0
Kirik, A., Pecinka, A., Wendeler, E., and Reiss, B. (2006). The chromatin assembly factor subunit FASCIATA1 is involved in homologous recombination in plants. Plant Cell 18, 2431–2442. doi: 10.1105/tpc.106.045088
Kozak, J., West, C. E., White, C., da Costa-Nunes, J. A., and Angelis, K. J. (2008). Rapid repair of DNA double strand breaks in Arabidopsis thaliana is dependent on proteins involved in chromosome structure maintenance. DNA Repair 8, 413–419. doi: 10.1016/j.dnarep.2008.11.012
Larionov, A., Krause, A., and Miller, W. (2005). A standard curve based method for relative real time PCR data processing. BMC Bioinformatics 6:62. doi: 10.1186/1471-2105-6-62
Leyser, H. M. O., and Furner, I. J. (1992). Characterisation of three shoot apical meristem mutants of Arabidopsis thaliana. Development 116, 397–403.
Lieber, M. R. (2010). The mechanism of double-strand DNA break repair by the nonhomologous DNA end-joining pathway. Annu. Rev. Biochem. 79, 181–211. doi: 10.1146/annurev.biochem.052308.093131
Lowndes, N. F., and Toh, G. W. (2005). DNA repair: the importance of phosphorylating histone H2AX. Curr. Biol. 15, R99–R102. doi: 10.1016/j.cub.2005.01.029
Martínez-Zapater, J. M., Estelle, M. A., and Somerville, C. (1986). A highly repeated DNA sequence in Arabidopsis thaliana. Mol. Gen. Genet. 204, 417–423. doi: 10.1007/BF00331018
McVey, M., and Lee, S. E. (2008). MMEJ repair of double-strand breaks (director’s cut): deleted sequences and alternative endings. Trends Genet. 24, 529–538. doi: 10.1016/j.tig.2008.08.007
Mladenov, E., and Iliakis, G. (2011). Induction and repair of DNA double strand breaks: the increasing spectrum of non-homologous end joining pathways. Mutat. Res. 711, 61–72. doi: 10.1016/j.mrfmmm.2011.02.005
Mozgová, I., Mokros, P., and Fajkus, J. (2010). Disfunction of chromatin assembly factor 1 induces shortening of telomeres and loss of 45S rDNA in Arabidopsis thaliana. Plant Cell 22, 2768–2780. doi: 10.1105/tpc.110.076182
Muchová, V., Amiard, S., Mozgová, I., Dvoráckova, M., Gallego, M. E., White, C., et al. (2015). Homology-dependent repair is involved in 45S rDNA loss in plant CAF-1 mutants. Plant J. 81, 198–209. doi: 10.1111/tpj.12718
Nabatiyan, A., and Krude, T. (2004). Silencing of chromatin assembly factor in human cells leads to cell death and loss of chromatin assembly during DNA synthesis. Mol. Cell Biol. 24, 2853–2862. doi: 10.1128/MCB.24.7.2853-2862.2004
Ono, T., Kaya, H., Takeda, S., Abe, M., Ogawa, Y., Kato, M., et al. (2006). Chromatin assembly factor 1 ensures the stable maintenance of silent chromatin states in Arabidopsis. Genes Cells 11, 153–162. doi: 10.1111/j.1365-2443.2006.00928.x
Pavlištová, V., Dvořáčková, M., Jež, M., Mozgová, I., Mokroš, P., and Fajkus, J. (2016). Phenotypic reversion in fas mutants of Arabidopsis thaliana by reintroduction of FAS genes: variable recovery of telomeres with major spatial rearrangements and transcriptional reprogramming of 45S rDNA genes. Plant J. 88, 411–424. doi: 10.1111/tpj.13257
Pradillo, M., López, E., Linacero, R., Romero, C., Cuñado, N., Sánchez-Morán, E., et al. (2012). Together yes, but not coupled: new insights into the roles of RAD51 and DMC1 in plant meiotic recombination. Plant J. 69, 921–933. doi: 10.1111/j.1365-313X.2011.04845.x
Ramirez-Parra, E., and Gutierrez, C. (2007a). The many faces of chromatin assembly factor 1. Trends Plant Sci. 12, 570–576.
Ramirez-Parra, E., and Gutierrez, C. (2007b). E2F regulates FASCIATA1, a chromatin assembly gene whose loss switches on the endocycle and activates gene expression by changing the epigenetic status. Plant Physiol. 144, 105–120.
Ransom, M., Dennehey, B. K., and Tyler, J. K. (2010). Chaperoning histones during DNA replication and repair. Cell 140, 183–189. doi: 10.1016/j.cell.2010.01.004
Richards, E. J., and Ausubel, F. M. (1988). Isolation of a higher eukaryotic telomere from Arabidopsis thaliana. Cell 53, 127–136. doi: 10.1016/0092-8674(88)90494-1
San Filippo, J., Sung, P., and Klein, H. (2008). Mechanism of eukaryotic homologous recombination. Annu. Rev. Biochem. 77, 229–257. doi: 10.1146/annurev.biochem.77.061306.125255
Sánchez-Morán, E., Armstrong, S. J., Santos, J. L., Franklin, F. C., and Jones, G. H. (2001). Chiasma formation in Arabidopsis thaliana accession Wassileskija and in two meiotic mutants. Chromosome Res. 9, 121–128. doi: 10.1023/A:1009278902994
Sasaki, M. S., Takata, M., Sonoda, E., Tachibana, A., and Takeda, S. (2004). Recombination repair pathway in the maintenance of chromosomal integrity against DNA interstrand crosslinks. Cytogenet. Genome Res. 104, 28–34. doi: 10.1159/000077463
Schönrock, N., Exner, V., Probst, A., Gruissen, L., and Hennig, I. (2006). Functional genomic analysis of CAF-1 mutants in Arabidopsis thaliana. J. Biol. Chem. 281, 9560–9568. doi: 10.1074/jbc.M513426200
Slupphaug, G. B., Kavli, H., and Krokan, E. (2003). The interacting pathways for prevention and repair of oxidative DNA damage. Mutat. Res. 531, 231–251. doi: 10.1016/j.mrfmmm.2003.06.002
Song, Y., He, F., Xie, G., Guo, X., Xu, Y., Chen, Y., et al. (2007). CAF-1 is essential for Drosophila development and involved in the maintenance of epigenetic memory. Dev. Biol. 311, 213–222. doi: 10.1016/j.ydbio.2007.08.039
Takeda, S., Tadele, Z., Hofmann, I., Probst, A. V., Angelis, K. J., Kaya, H., et al. (2004). BRU1, a novel link between responses to DNA damage and epigenetic gene silencing in Arabidopsis. Genes Dev. 18, 782–793. doi: 10.1101/gad.295404
Tallis, M., Morra, R., Barkauskaite, E., and Ahel, I. (2014). Poly(ADP-ribosyl)ation in regulation of chromatin structure and the DNA damage response. Chromosoma 123, 79–90. doi: 10.1007/s00412-013-0442-9
van Sluis, M., and McStay, B. (2015). A localized nucleolar DNA damage response facilitates recruitment of the homology-directed repair machinery independent of cell cycle stage. Genes Dev. 29, 1151–1163. doi: 10.1101/gad.260703.115
Varas, J., Sánchez-Morán, E., Copenhaver, G. P., Santos, J. L., and Pradillo, M. (2015). Analysis of the relationships between DNA double-strand breaks, synaptonemal complex and crossovers using the Atfas1-4 mutant. PLoS Genetics 11:e1005301. doi: 10.1371/journal.pgen.1005301
Keywords: Arabidopsis thaliana, DNA double-strand breaks, DNA repair, homologous recombination, non-homologous end-joining
Citation: Varas J, Santos JL and Pradillo M (2017) The Absence of the Arabidopsis Chaperone Complex CAF-1 Produces Mitotic Chromosome Abnormalities and Changes in the Expression Profiles of Genes Involved in DNA Repair. Front. Plant Sci. 8:525. doi: 10.3389/fpls.2017.00525
Received: 09 November 2016; Accepted: 24 March 2017;
Published: 11 April 2017.
Edited by:
Dao-Xiu Zhou, Université Paris-Sud, FranceCopyright © 2017 Varas, Santos and Pradillo. This is an open-access article distributed under the terms of the Creative Commons Attribution License (CC BY). The use, distribution or reproduction in other forums is permitted, provided the original author(s) or licensor are credited and that the original publication in this journal is cited, in accordance with accepted academic practice. No use, distribution or reproduction is permitted which does not comply with these terms.
*Correspondence: Mónica Pradillo, pradillo@bio.ucm.es