Synergetic effect of combining PEF treatments with sublethal doses of SO2 on the inactivation of Saccharomyces bayanus and Brettanomyces bruxellensis in red wine
- Food Technology, Veterinary Faculty, Agri-food Institute of Aragón-IA2, University of Zaragoza-CITA, Zaragoza, Spain
Certain microorganisms are capable of proliferating in wine despite its low pH and high ethanol content. The yeasts of the Saccharomyces genus responsible for alcoholic fermentation can alter wines with residual sugars; the proliferation of Brettanomyces bruxellensis brings about thoroughly unpleasant sensory changes. The main strategy currently applied in wineries for microbial control is the addition of sulfites (SO2). However, sulfites are being researched due to the symptoms they can cause in allergic individuals. Pulsed electric field (PEF) technology has the capability of inactivating vegetative cells of microorganisms at non-lethal temperatures and could thus prove to be an alternative to SO2. In this study, the resistance of Saccharomyces bayanus and B. bruxellensis suspended in wine to a series of different PEF treatments (10–25 kV/cm; 25–1000 µs; 40–170 kJ/kg) combined with sublethal concentrations of SO2 (10, 25, and 50 ppm) was evaluated. The results showed that even the least intense PEF treatments (10 kV/cm; 115 kJ/kg) inactivated more than 4.0 Log10 cycles in both types of yeasts immediately after treatment. The subsequent incubation of the treated yeasts for 24 h in wine managed to increase inactivation by 3.0 Log10 cycles. The combination of a moderate PEF treatment with sublethal doses of SO2 had a synergistic lethal effect on the two yeasts under study after 24 h of incubation in wine, leading to counts lying below the detection limit (>5.0 Log10 cycles). This synergistic effect was attributed to the existence of a portion of the population that had been sublethally damaged by PEF and in which SO2 could more easily penetrate the cytoplasm. These results demonstrate the capacity of PEF technology for microbial control of spoilage yeasts in wine. PEF could thus represent an alternative with the potential of eliminating or reducing SO2 levels in the winemaking process.
1 Introduction
Yeasts are key microorganisms in the winemaking process because they are responsible for transforming grape sugars into ethanol and CO2 (a role primarily played by Saccharomyces cerevisiae strains). The presence of ethanol (>8% v/v) in wine and its low pH (3.0–3.9 are two factors that usually prevent the proliferation of most microorganisms. However, in an uncompetitive environment such as this environment, certain microbial groups can still proliferate and spoil wines. In particular, certain fermentative or spoiling yeasts, as well as certain lactic acid bacteria (LAB) and acetic acid bacteria (AAB), constitute the main spoilage groups in wine (Campaniello and Sinigaglia, 2017). The presence and proliferation of Saccharomyces strains can spoil wine by uncontrolled refermentation, causing off-flavors and bottle explosion in sweet wines and sediment/cloudiness in dry wines. On the other hand, the yeast B. bruxellensis can produce devastating effects on wine quality (even at low concentrations) due to the production of 4-ethylphenol, which causes a “horse sweat” odor. B. bruxellensis is one of the most harmful microorganisms in wineries due to its ability to survive in environmental conditions lacking in nutrients and its capacity to contaminate cellar equipment; moreover, prior to the manifestation of spoilage, B. bruxellensis is difficult to detect (Wedral et al., 2010; Cibrario et al., 2020; Tedesco et al., 2022).
Microbial wine spoilage leads to pronounced economic losses coupled with rejection on the part of consumers. Therefore, apart from consistently applying adequate hygienic procedures, further inhibitory or lethal agents are required to prevent spoilage in winemaking. The most common strategy for microbial control in wineries is the addition of sulfur dioxide (SO2) at different steps of the wine production process. This antioxidant and antimicrobial compound has become indispensable in wineries due to its conveniently broad spectrum of action. However, in recent years, concern regarding the use of sulfites has increased. Certain authors have reported that SO2 can create unpleasant flavors in wine: moreover, it can be hazardous for human health due to allergic reactions observed in sensitive patients. Already in 2009, the World Health Organization (WHO) published an alert regarding excessive cumulative intake of SO2 in the general population, specifying that wine was one of the main factors in its intake in adults (WHO, 2009).
S. cerevisiae and B. bruxellensis have per se a considerable tolerance to SO2 (Malfeito-Ferreira, 2011); moreover, the extensive use of SO2 can increase this tolerance until they become strain-resistant to sulfites (Valdetara et al., 2020). This might explain how measurements in marketed red wine bottles revealed a concentration of 4-ethylphenol lying 25% above the preference threshold (Wedral et al., 2010). The wine industry is therefore searching for effective alternative methods to reduce or eliminate SO2 for purposes of microbial control. Given an increasing overall consumer rejection of chemicals as food preservatives (Mesías et al., 2021), physical preservation methods such as pulsed electric fields (PEFs) might present an adequate alternative.
PEF technology consists in the intermittent application of high-voltage pulses (kV) of short duration (microseconds to milliseconds) to a product placed between two electrodes. The increment in lipid bilayer permeability brought about by the electric field is referred to as electroporation (Kotnik et al., 2019). Electroporation of the cytoplasmic membrane of microbial cells can trigger a homeostatic imbalance that leads to cell death (Teissie et al., 2005). The proven efficacy of PEF for microbial inactivation, and its applicability in continuous flow and the current availability of flexible commercial devices are all factors that highlight this technology’s considerable potential as an appropriate method for the decontamination of liquid foods (Timmermans et al., 2022). Previous studies performed on wine have demonstrated the inactivating capability of PEF against the most common wine-associated microbiota (yeast and bacteria), either in a batch (Puértolas et al., 2009) or in a continuous process (González-Arenzana et al., 2015; 2019). Although several studies have been previously conducted on the subject of microbial inactivation in wine by PEF, there is still a lack of fundamental, systematic studies of microbial resistance to PEF that define appropriate PEF process parameters for microbial decontamination in wineries (Delso et al., 2022). Furthermore, most of these studies did not report the SO2 concentration in wines on which PEF inactivation experiments were performed, although this concentration could have potentially exerted a certain impact on the final lethal outcome.
The current study aimed to investigate the combined effect of moderate PEF treatments applied in continuous flow along with sublethal doses of SO2 on the inactivation of Saccharomyces bayanus and Brettanomyces bruxellensis suspended in red wine.
2 Material and methods
2.1 Strains, media, and culture conditions
Saccharomyces bayanus (CECT 1969) and Brettanomyces bruxellensis (CECT 11045) strains used in this study were supplied by the Spanish Type Culture Collection (CECT) and kept on slants of potato dextrose agar (PDA) (Oxoid, Basingstoke, Hampshire, United Kingdom) and Brettanomyces agar (Scharlab, Barcelona, Spain), respectively. Precultures were obtained by inoculating a single colony into a 10-mL test tube containing 5 mL of Sabouraud Dextrose (SD) broth (Oxoid), followed by incubation for 24 h at 25°C.
To obtain a S. bayanus suspension, a commercial red grape must (GREIP, PepsiCo, Vitoria, Spain) supplemented with 70 g/L of sucrose (Oxoid) was subjected to an alcoholic fermentation procedure for 9 days at 25°C to achieve a final concentration of 220 g of sucrose per liter, which corresponds to a potential ethanol content of 12.9% (v/v). The supplemented grape must was inoculated with S. bayanus at a concentration of 2 × 104 UFC/mL. PEF inactivation experiments on S. bayanus were conducted in the same wine after fermentation. On the other hand, to obtain B. bruxellensis cells for PEF inactivation experiments, 50 mL of SD was incubated 5 days at 25°C under agitation after inoculation with the B. bruxellensis preculture (1.8 × 104 UFC/mL). Cells destined for PEF inactivation experiments were recovered by centrifugation (10.000 g for 4 min) and suspended in the wine after removing S. bayanus cells by centrifugation (10.000 g–10 min). The wine’s pH and conductivity were 3.1 and 1.3 m/cm, respectively.
2.2 PEF treatments
A commercial VITAVE PEF generator (VPGU 20/500/9, Vitave, Prague, Czech Republic) with a maximum voltage of 20 kV and a current of 500 A was used. For inactivation experiments, wine containing the yeast strains was pumped through a parallel titanium electrode treatment chamber (0.4 cm gap × 3 cm length × 0.5 cm width) at a flow of 5 L/h, corresponding to a residence time of 0.43 s. Wine was tempered to 20°C using a heat exchanger located immediately before the treatment chamber. A cooling system located after the treatment chamber cooled the wine’s temperature to under 20°C in less than 5 s. Actual voltage during treatments was measured using a high-voltage probe (Tektronix, P6015A, Wilsonville, Oregon, United States) connected to an oscilloscope (Tektronix, TDS 220). Inlet and outlet temperatures were measured using type K thermocouples inserted in the circuit (Ahlborn, Holzkirchen, Germany).
Square pulses of 5 µs width at different electric field strengths (10, 15, 20, and 25 kV/cm) were delivered at different repetition rates up to 200 Hz to apply treatments of different duration (number of pulses x pulse width) ranging from 25 to 750 µs, which corresponded to outlet temperatures of 30, 35, 40, 45, 50, 55, and 60ºC ± 2.0°C. Total specific energies of the applied treatments ranged from 19.5 to 156 kJ/kg. The total specific energy (W) of treatments was estimated from the temperature increase during pulses delivering assuming adiabatic conditions (Heinz et al., 2001) and according to the average values of the specific heat capacity of wine obtained from Genc et al. (2017).
2.3 Sulfite addition
A stock solution of 25 g/L of SO2 was prepared from potassium bisulfite (Sigma, Burlington, MA, United States). Immediately after the PEF treatments, the corresponding volume of the stock solution was added to the untreated and PEF-treated wine samples to obtain final concentrations of 10, 25, and 50 ppm of SO2.
2.4 Microbial viability
The viability of S. bayanus and B. bruxellensis was evaluated by plate counting 30 min and 24 h after the application of PEF treatments. S. bayanus was recovered for 48 h at 25°C onto PDA. On the other hand, B. bruxellensis was recovered after 5 days at 25°C onto Brettanomyces agar. The number of colonies counted after incubation corresponded to the number of viable microorganisms expressed as colony forming units per milliliter (CFU/mL). The survival fraction was calculated by dividing the number of microorganisms that survived the treatment (Nt) by the initial number of viable cells (N0). Survival curves were obtained by plotting Log10 of the survival fraction vs. treatment time for each electric field strength.
2.5 Mathematical modeling
Mathematical modeling was used to evaluate the effect and interactions of the total specific energy (from 0 to 156 kJ/kg) of PEF treatments, the concentration of SO2 (0, 10, 25, and 50 ppm), and the incubation period (0, 24 h) on the inactivation of B. bruxellensis. The obtained inactivation data obtained after applying the treatment conditions described in Section 2.2 were fitted to the following polynomial equation:
where Y is the predicted response, and β0, βi, βii, and βij are the intercept, linear, quadratic, and cross-product coefficients, respectively. Xi and Xj represent the independent factors, and n is the total number of independent factors. Data analysis was performed with Design-Expert software (version 10.0, Stat-Ease, Inc., Minneapolis MN, United States). To determine the model’s significant parameters and to remove the non-significant effects (p > 0.05), backward regression was applied.
2.6 Experimental validation
For validation experiments, red wine supplemented with 20 g/L of sucrose was contaminated with B. bruxellensis to an initial concentration of 102 CFU/mL. After 2 months of incubation, the wine containing 105 CFU/mL of B. bruxellensis was subjected to PEF treatments (15 kV/cm, 5 µs) of varying duration and specific energies. PEF-treated wines were added with different SO2 doses, and the number of survivors was determined after 30 min and 24 h of incubation.
These independent data were used to validate the model obtained for the prediction of microbial inactivation of B. bruxellensis. The root mean square error (RMSE) between estimated and the actual experimental data was calculated.
2.7 Statistical analyses
At least three samples were analyzed for each condition. Data are expressed as the mean ± standard deviation. One-way analysis of variance (ANOVA) and Tukey tests using GraphPad Prism (GraphPad Software, San Diego, California, United States) were performed to evaluate the significance of differences among the mean values. Differences were considered significant at p < 0.05. Multiple regression analysis was conducted to fit Eq. 1 to the experimental data, and Design-Expert software (version 10.0, Stat-Ease Inc., Minneapolis, MN, United States) was used to determine the model’s significant terms (p > 0.005) by ANOVA.
3 Results and discussion
3.1 PEF resistance of Saccharomyces bayanus and Brettanomyces bruxellensis suspended in red wine
The survival curves of S. bayanus and B. bruxellensis in wine at different electric field strengths are shown in Figure 1. Residence time in the treatment chamber (0.43 s) and pulse width (5 µs) were kept constant. Therefore, to modify the treatment time (number of pulses × pulse width) at the different electric field strengths tested, the number of pulses delivered in the treatment chamber was increased by raising the frequency of the treatments (number of pulses delivered per second). For a given electric field strength, an increase in the number of pulses delivered to the treatment chamber leads to a rise in the total specific energy supplied to the sample. Consequently, the sample’s temperature increases due to the Joule effect. The outlet temperature of samples subjected to PEF treatments of various durations applied at different electric field strengths is indicated in Figure 1 next to the corresponding symbol.
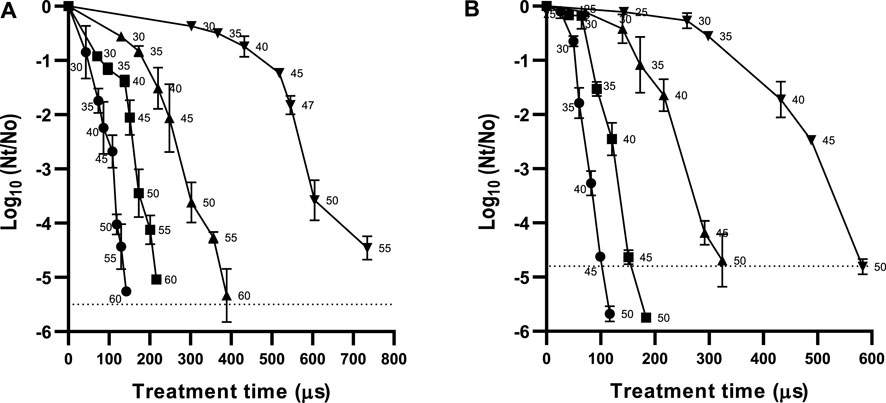
FIGURE 1. Influence of treatment time (µs) on the inactivation of Saccharomyces bayanus (A) or Brettanomyces bruxellensis (B) suspended in red wine at different electric field strengths 10 (▼), 15 (▲), 20 (■), and 25 kV/cm (●). Inactivation obtained immediately after PEF application. Numbers near the dots indicate the outlet temperature achieved during treatment. Outlet temperatures of 25, 30, 35, 40, 45, 50, 55, and 60 °C correspond with total specific energies of 19.5, 38.9, 58.3, 77.8, 97.2, 116.7, 136, and 155.6 kJ/kg, respectively. Dotted lines represent the quantification limit (<30 CFU/mL).
The survival curves of both microorganisms (S. bayanus and B. bruxellensis) showed an initial shoulder whose width was greater at lower electric field strengths. As the electric field strength increased, the shoulder decreased in size and the survival curves became progressively steeper. The survival curves’ shape can be explained by the increase in the lethal effect of PEF treatments at higher temperatures, as demonstrated in several previous studies (Saldaña et al., 2012; Timmermans et al., 2019). The existence of a shoulder in the survival curves implies the presence of a time threshold that appears to depend on the electric field strength and the exit temperature (total specific energy). At lower electric field strengths, longer treatments are necessary to deliver the total specific energy required to reach the exit temperature, from which inactivation increases rapidly with treatment time.
In terms of efficacy, more pulses were required to achieve a given level of inactivation at lower electric field strengths. For example, the treatment time required to achieve an inactivation of 3.0 Log10 cycles in S. bayanus and B. bruxellensis was three times longer at 15 kV/cm than at 25 kV/cm. However, a comparison of treatments at the same outlet temperature but with varying electric field strengths shows that the increment in terms of electric field strength had little effect on microbial inactivation. For instance, an inactivation rate lying between 3.6 and 4.0 Log10 cycles was achieved in S. bayanus at 15, 20, and 25 kV/cm for an outlet temperature of 50°C (117 kJ/kg). Meanwhile, for an outlet temperature of 45°C (97 kJ/kg), the inactivation of B. bruxellensis ranged between 4.1 and 4.6 Log10 cycles when the electric field strength was increased from 15 to 25 kV/cm. In studies of this nature, it is therefore essential to specify the electric field strength, treatment time, and the total specific energy applied when describing PEF treatments, especially in continuous processing where the energy introduced into the system increases the temperature of the sample and the efficacy of the PEF treatment.
Regarding the resistance of the two yeast strains to PEF, B. bruxellensis was generally more sensitive to PEF treatments than S. bayanus. For instance, PEF treatments of the same intensity (20 kV/cm, 150 µs, outlet temperature 45°C) inactivated 4.8 Log10 cycles of B. bruxellensis but only 2.0 Log10 cycles of S. bayanus. Previous studies have likewise observed a greater PEF resistance of Saccharomyces strains in wine compared to Brettanomyces (Puértolas et al., 2009; González-Arenzana et al., 2015). Differences in the composition of the cytoplasmic membrane could explain the observed differences among the two yeast strains in terms of resistance, given that they are of similar shape and size. Saccharomyces has a well-known capacity to perform alcoholic fermentation without suppression of metabolic activity due to the presence of ethanol. Certain studies have suggested that this ethanol tolerance of the Saccharomyces genus is directly related to changes in the cytoplasmic membrane composition triggered by the presence of ethanol (Aguilera et al., 2006; Henderson and Block, 2014).
Our study found that PEF treatments were highly effective in inactivating S. bayanus and B. bruxellensis, with inactivation levels of around 4.0 Log10 cycles or even higher, achieved at total specific energies ranging from 97 to 117 kJ/kg. These values are lower than those reported by previous studies, in which treatments of 121 kJ/kg at 50 kV/cm or 300 kJ/kg at 20 kV/cm were required (Puértolas et al., 2009; Delsart et al., 2015; van Wyk et al., 2018). The higher degree of lethality observed in our study may be due to the improved efficacy of PEF treatments when applied at moderate temperatures (30°C–60°C) (Saldaña et al., 2012; Timmermans et al., 2019). Although PEF is often viewed as a non-thermal technology, our findings suggest that PEF treatments at moderate temperatures may enhance lethality. However, this strategy may only be feasible for the food industry if the increase in temperature during the short PEF treatment duration does not negatively affect food’s sensory or nutritional quality. In the wine industry, maintaining quality properties is of utmost importance and thermal treatments for microbial inactivation tend to be avoided altogether. However, recent research by our team has shown that PEF treatments of up to 156 kJ/kg (resulting in an outlet temperature of 60°C) did not have a negative impact on the sensory profile of a high-value commercial wine (Delso et al., 2023).
3.2 Evaluation of the viable population of Saccharomyces bayanus and Brettanomyces bruxellensis treated by PEF after 24 h of incubation in wine
The efficacy of PEF treatments on S. bayanus and B. bruxellensis yeasts in wine after 24 h of incubation was also evaluated. Figure 2 shows the total inactivation of S. bayanus and B. bruxellensis, evaluating the number of survivors to the PEF treatments after 24 h. The dark gray area of the column corresponds to the inactivation obtained immediately after PEF, and the light gray area corresponds to the additional inactivation produced after 24 h of incubation in wine. Differences between inactivation achieved immediately after the PEF treatment and after 24 h of incubation are indicated by dots joined by a black line.
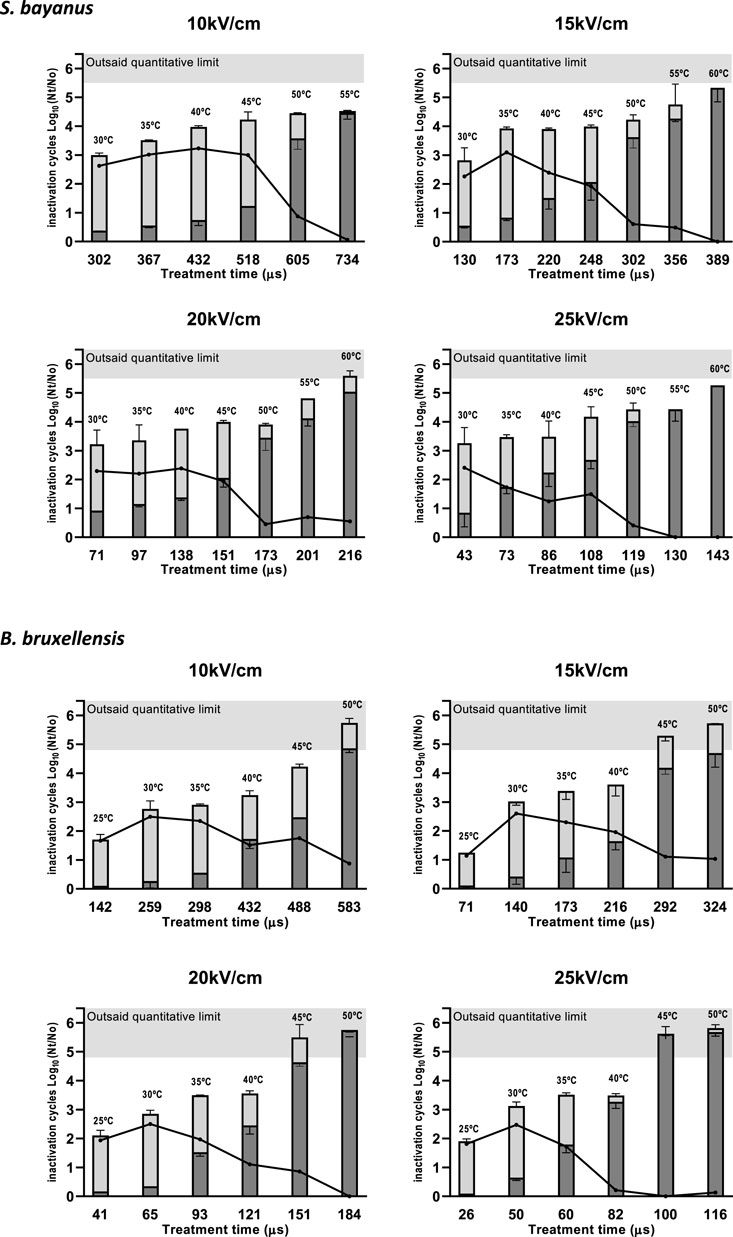
FIGURE 2. Effect of the incubation time in wine after PEF treatment on the inactivation of Saccharomyces bayanus or Brettanomyces bruxellensis at different electric field strengths and treatment times. Bars represent the total inactivation: the dark area corresponds to the inactivation obtained immediately after PEF, and the light area corresponds to the additional inactivation obtained after 24 h. The difference between the inactivation achieved after PEF or after 24 h of incubation is also represented (●).
A significant increase in efficacy against both yeasts was observed after 24 h at different electric field strengths, although the effect tended to disappear in the case of PEF treatments that achieved a greater degree of efficacy immediately after application. For example, PEF treatment with an electric field strength of 15 kV/cm, 173 µs, and 35°C of the outlet temperature that showed very low efficacy immediately after treatment (<1.0 Log10 reduction) exhibited an increase in inactivation of 3.0 and 2.3 Log10 for S. bayanus and B. bruxellensis, respectively, after 24 h of incubation. In contrast, PEF treatments of higher intensity and efficacy (4.0 Log10 cycles or higher) showed little improvement in inactivation after 24 h of incubation.
The observed differences between immediate inactivation and 24-h inactivation may be due to the presence of a sublethally injured population, the recovery of which may depend on environmental conditions. Electroporation triggered by PEF treatments is a purely physical process in which stable pores in the cytoplasmic membrane can be created if the induced transmembrane voltage lies above the cell’s threshold (Kotnik et al., 2019). However, recovery of cell viability after PEF treatment depends on intrinsic microbial features coupled with external factors that affect membrane integrity repair (Saulis, 2010). Recovery is more likely to occur when cells are transferred to an agar medium immediately after treatment, but the low pH (3.1) and high ethanol content (12.9% v/v) in wine may impair this recovery process, resulting in a lower number of survivors after 24 h of incubation. These results confirm previous findings obtained by Somolinos et al. (2007, 2008) on the subject of sublethal damage in PEF-treated S. cerevisiae and B. bruxellensis cells.
The loss of viability in a significant proportion of PEF-treated cells after 24 h of incubation allowed for a 3.0–4.0 Log10 reduction with very moderate treatments (58 kJ/kg, 35°C outlet temperature) at all the electric field strengths we studied. These results suggest that on account of the additional inactivation that takes place in wine even after moderate PEF treatments (10–15 kV/cm), the total specific energy required for a 3.0–4.0 Log10 reduction of the yeast population can be halved, making PEF technology more feasible to apply in wineries.
3.3 Inactivation of Saccharomyces bayanus and Brettanomyces bruxellensis in wine by combining PEF and sulfites
The addition of sulfur dioxide (SO2) is the method most commonly applied for controlling the proliferation of yeast in wine. After confirming that PEF caused sublethal damages in yeasts suspended in wine, we performed a further experiment combining PEF with different doses of SO2 with the aim of finding a way to further increase the lethal outcome of PEF treatments. For this purpose, 0, 10, 25, or 50 ppm of SO2 was combined with two moderate PEF treatments that had a low lethal effect but which managed to sublethally damage a high proportion of the yeast population: these treatments were PEF1 (15 kV/cm, 39 kJ/kg, 30°C outlet temp.) and PEF2 (15 kV/cm, 78 kJ/kg, 40°C outlet temp.). Figure 3 shows the inactivation of S. bayanus and B. bruxellensis obtained by applying these combined treatments immediately after the PEF treatment and after 24 h of incubation in the same wine. For comparison, we also dosed untreated wine samples with the same varying concentrations of SO2.
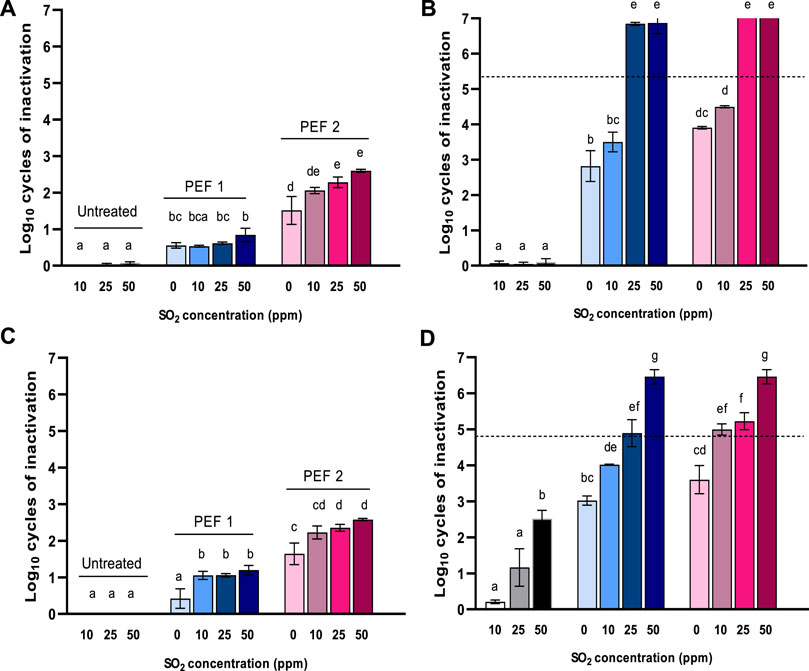
FIGURE 3. Inactivation of Saccharomyces bayanus (A and B) and Brettanomyces bruxellensis (C and D) when combining PEF1 (39 kJ/kg, 30°C of outlet temp.) or PEF2 (78 kJ/kg, 40°C of outlet temp.) treatment at 15 kV/cm with 0, 10, 25, or 50 ppm of sulfites immediately after PEF (A and C) and incubated for 24 h (C and D). Dotted lines represent the quantification limit (<30 CFU/mL).
The addition of sulfur dioxide did not affect the population of untreated S. bayanus cells, even after 24 h of incubation. For B. bruxellensis, a loss of viability of 1.1 and 2.4 Log10 cycles was only observed when 25 and 50 ppm of SO2 were added, respectively, after 24 h of incubation. The presence of sulfites scarcely increased the efficacy of the PEF treatments when the number of survivors was determined just after processing. However, a pronounced increase in inactivation was observed after 24 h of incubation when 25 and 50 ppm of SO2 were present. In all cases, the combination of the two procedures had a synergistic effect rather than an additive effect. Somolinos et al. (2007) reported a similar synergistic effect on S. cerevisiae and B. bruxellensis by combining a PEF treatment with sublethal doses of sorbic acid. The combination of PEF with further antimicrobial compounds such as nisin, lysozyme, and organic acids has also resulted in synergistic effects in the inactivation of different bacteria (Smith et al., 2002; Mosqueda-Melgar et al., 2008; Saldaña et al., 2012). These synergistic effects have been mainly attributed to the easier accessibility these compounds gain into the electroporated cells, whose target is located in the cytoplasm; another explanation is that the added compound facilitates the electroporation of the cytoplasmic membrane. SO2 needs to reach the cytoplasm, where it reacts with a number of molecules such as coenzymes, cofactors, vitamins, nucleic acid, and the enzymatic system. PEF treatment could therefore sensitize a proportion of the cells that were able to survive sublethal amounts of SO2 in the wine after the PEF treatment. The great tolerance of yeast to SO2 has been chiefly attributed to a sulfite efflux pumping system through the plasma membrane or to the microorganism’s massive production of acetaldehyde, which binds and blocks intracellular SO2 (García-Ríos and Guillamón, 2019). A lack of lipid membrane integrity in an electroporated cell could directly cancel the efflux pump effect. Furthermore, stress mechanisms triggered by homeostatic imbalance in permeabilized cells (García et al., 2006) could also be responsible for hindering intracellular acetaldehyde production or fixation.
3.4 Comparison of the inactivation of Brettanomyces bruxellensis inoculated or cultivated in wine by combined treatments of PEF with sulfites
Inactivation of B. bruxellensis was conducted on cells cultivated in a culture medium and then inoculated in red wine. However, growth conditions can influence the physiology of microorganisms and their resistance to different lethal effects. We, therefore, decided to investigate whether the data on resistance of B. bruxellensis cells inoculated in wine could be used to predict the inactivation of B. bruxellensis cells grown in wine. To accomplish this, we developed a multiple regression model featuring the total specific energy of the PEF treatment and sulfite concentration as independent variables. To develop the model, we used the data for B. bruxellensis obtained after the application of PEF treatments (from 0 to 168 kJ/kg), SO2 concentration (0, 10, 25, and 50 ppm) and incubation time (0, 24 h). This model was validated with inactivation data on the same microorganism after growth in wine for 2 months. The culture of B. bruxellensis in wine was extended during that time to reach a sufficiently large concentration of cells sufficient to evaluate the effectiveness of inactivation treatment.
Table 1 shows the terms of the equations with their coefficients after fitting the experimental data obtained immediately after the PEF treatment and after 24 h of incubation to a quadratic equation after removing non-significant terms (p < 0.05). The table also shows the results of analysis of variance (ANOVA) for the model’s significant terms and the statistics used to test its adequacy. F-value calculation indicates that the overall model was significant (p < 0.05): the model’s terms thus have a significant effect on the response. The model’s R2 value was 0.90, indicating that only 10% of the total response variation could not be explained by the model. The RMSE value confirms the model’s fitness for describing the experimental inactivation data. According to the F-values, total specific energy was the most significant variable with an F-value of 170.7, followed by the linear term of the sulfite concentration.
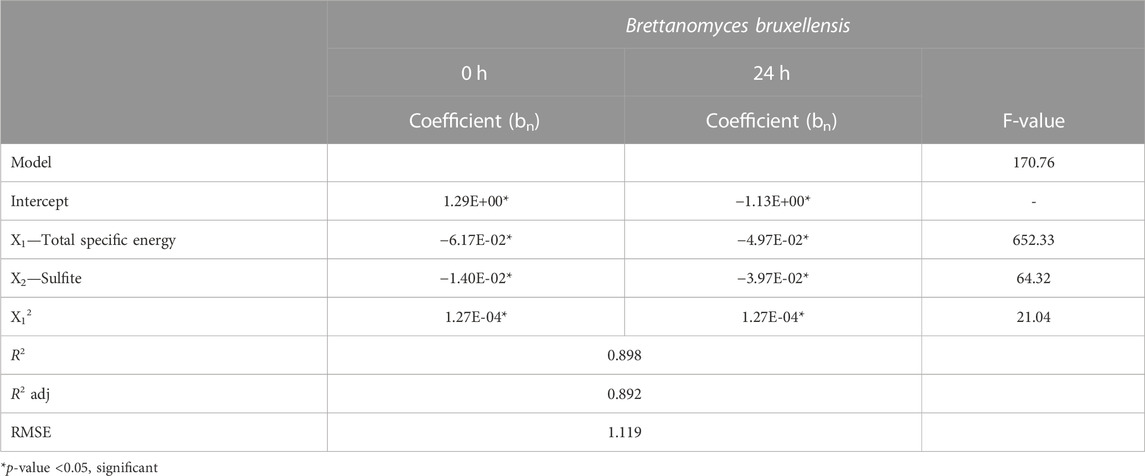
TABLE 1. Coefficients and F-values of the mathematical equation obtained after multiple regression modeling of Brettanomyces bruxellensis data.
Figure 4 shows the comparison of the inactivation data predicted by the model with actual data obtained from the observed inactivation of B. bruxellensis cells grown in wine. For data obtained immediately after the PEF treatment (closed symbols), the model predicted lower inactivation levels than those achieved in the B. bruxellensis cells grown in wine. These results indicate that the cells cultivated in the same wine were more sensitive to the PEF treatments than those cultivated in a culture medium and subsequently inoculated in the wine. However, after comparison of the results obtained after 24 h of incubation (open symbols), a close correspondence between predicted and actual inactivation can be observed. Remarkably, for inactivation levels higher than 2.0 Log10 cycles, most of the points lie below the equivalency line, thereby indicating that the model is reliable as it predicts less inactivation than that obtained with the cells grown in the wine.
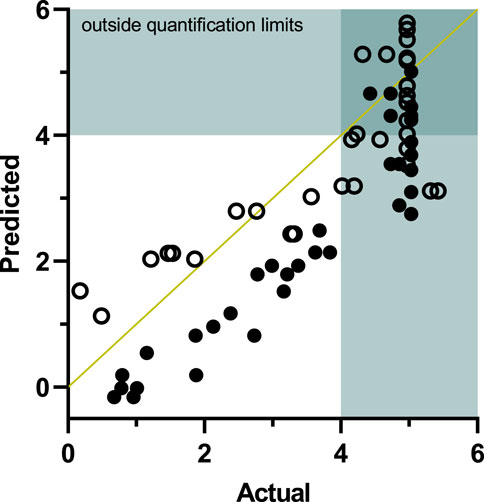
FIGURE 4. Validation of the model for the prediction of Brettanomyces bruxellensis inactivation by PEF and SO2 in wine (Table 1). Correlation of the predicted and the experimental data obtained independently without incubation (●) and with 24 h of incubation (○) after treatment.
Our results, therefore, reinforce the potential of applying PEF in combination with reduced concentrations of SO2 to decontaminate yeast in wine. This could contribute to an overall reduction of the final concentration of sulfites in winemaking and improve other processing stages, such as mutage or the implantation of lactic acid bacteria for the performance of malolactic fermentation after alcoholic fermentation.
4 Conclusion
Our evaluation of the PEF resistance of S. bayanus and B. bruxellensis in wine revealed that moderate PEF treatments can generate a significant proportion of sublethally damaged cells. Although many of these injured cells may not survive in the wine after PEF processing, those that do survive may be sensitized to SO2, resulting in a synergetic effect of PEF with reduced concentrations of SO2. As a result, significant inactivation rates were achieved in the two yeast strains under study by applying moderate PEF or by combining PEF treatments with sublethal doses of SO2. This study demonstrates the potential of moderate PEF treatments (15 kV/cm, <100 kJ/kg) for general application on an industrial scale in wineries. Such treatments can be implemented as a strategy to reduce SO2 concentrations with the overall purpose of controlling yeast in winemaking, thereby preventing refermentation and wine spoilage, as well as eventually facilitating the growth of lactic acid for the performance of malolactic fermentation.
Data availability statement
The raw data supporting the conclusion of this article will be made available by the authors, without undue reservation.
Author contributions
Conception and design of the study: CD, IÁ, and JR. Acquisition of data: CD, AB, and JS. Analysis and interpretation of the data: CD, IÁ, and JR. Drafting of the article: CD, AB, and JS. Critical revision of the article: IÁ and JR. All authors contributed to the article and approved the submitted version.
Acknowledgments
CD gratefully acknowledges the financial support for her doctoral studies provided by the Department of Innovation, Investigation and University of the Government of Aragón.
Conflict of interest
The authors declare that the research was conducted in the absence of any commercial or financial relationships that could be construed as a potential conflict of interest.
The author JR declared that they were an editorial board member of Frontiers, at the time of submission. This had no impact on the peer review process and the final decision.
Publisher’s note
All claims expressed in this article are solely those of the authors and do not necessarily represent those of their affiliated organizations, or those of the publisher, the editors, and the reviewers. Any product that may be evaluated in this article, or claim that may be made by its manufacturer, is not guaranteed or endorsed by the publisher.
References
Aguilera, F., et al. (2006). Relationship between ethanol tolerance, H+-ATPase activity and the lipid composition of the plasma membrane in different wine yeast strains. Int. J. Food Microbiol. 110 (1), 34–42. doi:10.1016/J.IJFOODMICRO.2006.02.002
Campaniello, D., and Sinigaglia, M. (2017). “Wine spoiling phenomena,” in The microbiological quality of food (China: Elsevier), 237–255. doi:10.1016/B978-0-08-100502-6.00013-3
Cibrario, A., Miot-Sertier, C., Paulin, M., Bullier, B., Riquier, L., Perello, M. C., et al. (2020). Brettanomyces bruxellensis phenotypic diversity, tolerance to wine stress and wine spoilage ability. Food Microbiol. 87, 103379. doi:10.1016/J.FM.2019.103379
Delsart, C., Grimi, N., Boussetta, N., Miot Sertier, C., Ghidossi, R., Vorobiev, E., et al. (2015). Impact of pulsed-electric field and high-voltage electrical discharges on red wine microbial stabilization and quality characteristics. J. Appl. Microbiol. 120, 152–164. doi:10.1111/jam.12981
Delso, C., Berzosa, A., Sanz, J., Álvarez, I., and Raso, J. (2023). Microbial decontamination of red wine by pulsed electric fields (PEF) after alcoholic and malolactic fermentation: effect on Saccharomyces cerevisiae, oenococcus oeni, and oenological parameters during storage’, foods. Multidiscip. Digit. Publ. Inst. 12 (2), 278. doi:10.3390/foods12020278
Delso, C., et al. (2022). “Microbial decontamination by pulsed electric fields (PEF) in winemaking,” in Grapes and wine (IntechOpen, 137–144. doi:10.5772/intechopen.101112
García, D., Mañas, P., Gómez, N., Raso, J., and Pagán, R. (2006). Biosynthetic requirements for the repair of sublethal membrane damage in Escherichia coli cells after pulsed electric fields. J. Appl. Microbiol. 100 (3), 428–435. doi:10.1111/j.1365-2672.2005.02795.x
García-Ríos, E., and Guillamón, J. M. (2019). Sulfur dioxide resistance in Saccharomyces cerevisiae: beyond SSU1. Microb. Cell. 6 (12), 527–530. doi:10.15698/mic2019.12.699
Genc, M., Genc, S., and Goksungur, Y. (2017). Exergy analysis of wine production: red wine production process as a case study. Appl. Therm. Eng. 117, 511–521. doi:10.1016/J.APPLTHERMALENG.2017.02.009
González-Arenzana, L., et al. (2019). Continuous pulsed electric field treatments’ impact on the microbiota of red Tempranillo wines aged in oak barrels. Food Biosci. 27, 54–59. doi:10.1016/J.FBIO.2018.10.012
González-Arenzana, L., et al. (2015). Inactivation of wine-associated microbiota by continuous pulsed electric field treatments. Innovative Food Sci. Emerg. Technol. 29, 187–192. doi:10.1016/j.ifset.2015.03.009
Heinz, V., Alvarez, I., Angersbach, A., and Knorr, D. (2001). Preservation of liquid foods by high intensity pulsed electric fields—Basic concepts for process design. Trends Food Sci. Technol. 12 (3–4), 103–111. doi:10.1016/S0924-2244(01)00064-4
Henderson, C. M., and Block, D. E. (2014). Examining the role of membrane lipid composition in determining the ethanol tolerance of Saccharomyces cerevisiae. Appl. Environ. Microbiol. 80 (10), 2966–2972. doi:10.1128/AEM.04151-13
Kotnik, T., Rems, L., Tarek, M., and Miklavčič, D. (2019). Membrane electroporation and electropermeabilization: mechanisms and models. Annu. Rev. Biophysics 48 (1), 63–91. doi:10.1146/annurev-biophys-052118-115451
Malfeito-Ferreira, M. (2011). Yeasts and wine off-flavours: A technological perspective. Ann. Microbiol. 61 (1), 95–102. doi:10.1007/s13213-010-0098-0
Mesías, F. J., Martín, A., and Hernández, A. (2021). Consumers’ growing appetite for natural foods: perceptions towards the use of natural preservatives in fresh fruit. Food Res. Int. 150, 110749. doi:10.1016/J.FOODRES.2021.110749
Mosqueda-Melgar, J., Raybaudi-Massilia, R. M., and Martín-Belloso, O. (2008). Non-thermal pasteurization of fruit juices by combining high-intensity pulsed electric fields with natural antimicrobials. Innovative Food Sci. Emerg. Technol. 9 (3), 328–340. doi:10.1016/J.IFSET.2007.09.003
Puértolas, E., López, N., Condón, S., Raso, J., and Alvarez, I. (2009). Pulsed electric fields inactivation of wine spoilage yeast and bacteria. Int. J. Food Microbiol. 130 (1), 49–55. doi:10.1016/j.ijfoodmicro.2008.12.035
Saldaña, G., Monfort, S., Condón, S., Raso, J., and Álvarez, I. (2012). Effect of temperature, pH and presence of nisin on inactivation of Salmonella Typhimurium and Escherichia coli O157:H7 by pulsed electric fields. Food Res. Int. 45 (2), 1080–1086. doi:10.1016/j.foodres.2011.03.059
Saulis, G. (2010). Electroporation of cell membranes: the fundamental effects of pulsed electric fields in food processing. Food Eng. Rev. 2 (2), 52–73. doi:10.1007/s12393-010-9023-3
Smith, K., Mittal, G. S., and Griffiths, M. W. (2002). Pasteurization of milk using pulsed electrical field and antimicrobials. J. Food Sci. 67 (6), 2304–2308. doi:10.1111/j.1365-2621.2002.tb09545.x
Somolinos, M., García, D., Condón, S., Mañas, P., and Pagán, R. (2007). Relationship between sublethal injury and inactivation of yeast cells by the combination of sorbic acid and pulsed electric fields. Appl. Environ. Microbiol. 73 (12), 3814–3821. doi:10.1128/AEM.00517-07
Somolinos, M., Mañas, P., Condón, S., Pagán, R., and García, D. (2008). Recovery of Saccharomyces cerevisiae sublethally injured cells after pulsed electric fields. Int. J. Food Microbiol. 125 (3), 352–356. doi:10.1016/j.ijfoodmicro.2008.04.023
Tedesco, F., Siesto, G., Pietrafesa, R., Romano, P., Salvia, R., Scieuzo, C., et al. (2022). Chemical methods for microbiological control of winemaking: an overview of current and future applications. Beverages 8 (3), 58. doi:10.3390/beverages8030058
Teissie, J., Golzio, M., and Rols, M. P. (2005). Mechanisms of cell membrane electropermeabilization: A minireview of our present (lack of ?) knowledge. Biochimica Biophysica Acta (BBA) - General Subj. 1724 (3), 270–280. doi:10.1016/j.bbagen.2005.05.006
Timmermans, R. A. H., Mastwijk, H. C., Berendsen, L. B. J. M., Nederhoff, A. L., Matser, A. M., Van Boekel, M. A. J. S., et al. (2019). Moderate intensity Pulsed Electric Fields (PEF) as alternative mild preservation technology for fruit juice. Int. J. Food Microbiol. 298, 63–73. doi:10.1016/j.ijfoodmicro.2019.02.015
Timmermans, R., Nierop Groot, M., and Matser, A. (2022). “Liquid food pasteurization by pulsed electric fields,” in Pulsed electric fields technology for the food industry. Editor J. Raso (Cham: Springer International Publishing), 299–323. doi:10.1007/978-3-030-70586-2_8
Valdetara, F., Škalič, M., Fracassetti, D., Louw, M., Compagno, C., du Toit, M., et al. (2020). Transcriptomics unravels the adaptive molecular mechanisms of Brettanomyces bruxellensis under SO2 stress in wine condition. Food Microbiol. 90, 103483. doi:10.1016/j.fm.2020.103483
van Wyk, S., Farid, M. M., and Silva, F. V. M. (2018). SO2, high pressure processing and pulsed electric field treatments of red wine: effect on sensory, Brettanomyces inactivation and other quality parameters during one year storage. Innovative Food Sci. Emerg. Technol. 48, 204–211. doi:10.1016/J.IFSET.2018.06.016
Wedral, D., Shewfelt, R., and Frank, J. (2010)., 43. Academic Press, 1474–1479. doi:10.1016/J.LWT.2010.06.010The challenge of Brettanomyces in wineLWT - Food Sci. Technol.10
WHO (2009). Evaluation of certain food additives. WHO technical report 952. Sixty-Ninth report of the joint FAO/WHO expert committee on food additives. 17-26 june 2008’, World health organization technical report series, (974). Available at: http://whqlibdoc.who.int/trs/WHO_TRS_952_eng.pdf.
Keywords: pulsed electric fields, incubation effect, synergetic effect, yeast inactivation, red wine decontamination, sulfite reduction
Citation: Delso C, Berzosa A, Sanz J, Álvarez I and Raso J (2023) Synergetic effect of combining PEF treatments with sublethal doses of SO2 on the inactivation of Saccharomyces bayanus and Brettanomyces bruxellensis in red wine. Front. Food. Sci. Technol. 3:1209452. doi: 10.3389/frfst.2023.1209452
Received: 20 April 2023; Accepted: 21 August 2023;
Published: 04 September 2023.
Edited by:
Stanley Brul, University of Amsterdam, NetherlandsReviewed by:
Huub Lelieveld, Global Harmonization Initiative (GHI), AustriaGianpiero Pataro, University of Salerno, Italy
Copyright © 2023 Delso, Berzosa, Sanz, Álvarez and Raso. This is an open-access article distributed under the terms of the Creative Commons Attribution License (CC BY). The use, distribution or reproduction in other forums is permitted, provided the original author(s) and the copyright owner(s) are credited and that the original publication in this journal is cited, in accordance with accepted academic practice. No use, distribution or reproduction is permitted which does not comply with these terms.
*Correspondence: Javier Raso, jraso@unizar.es