- 1Department of Sports Research, Japan Institute of Sports Sciences, Tokyo, Japan
- 2Faculty of Sport Sciences, Waseda University, Saitama, Japan
- 3Department of Sports Sciences, Japan Institute of Sports Sciences, Tokyo, Japan
- 4Department of Fundamental Education, Dokkyo Medical University, Tochigi, Japan
- 5Department of Physical Activity Research, National Institutes of Biomedical Innovation, Health and Nutrition, Tokyo, Japan
- 6Laboratory of Gut Microbiome for Health, Microbial Research Center for Health and Medicine, National Institutes of Biomedical Innovation, Health and Nutrition, Osaka, Japan
- 7Artificial Intelligence Center for Health and Biomedical Research, National Institutes of Biomedical Innovation, Health and Nutrition, Osaka, Japan
- 8Laboratory of Vaccine Materials and Laboratory of Gut Environmental System, Microbial Research Center for Health and Medicine, National Institutes of Biomedical Innovation, Health and Nutrition, Osaka, Japan
- 9Institute for Protein Research, Osaka University, Osaka, Japan
Introduction: The gut microbiome plays a fundamental role in host homeostasis through regulating immune functions, enzyme activity, and hormone secretion. Exercise is associated with changes in gut microbiome composition and function. However, few studies have investigated the gut microbiome during training periodization. The present study aimed to investigate the relationship between training periodization and the gut microbiome in elite athletes.
Methods: In total, 84 elite athletes participated in the cross-sectional study; and gut microbiome was determined during their transition or preparation season period. Further, 10 short-track speed skate athletes participated in the longitudinal study, which assessed the gut microbiome and physical fitness such as aerobic capacity and anaerobic power in the general and specific preparation phase of training periodization. The gut microbiome was analyzed using 16S rRNA sequencing.
Results: The cross-sectional study revealed significant differences in Prevotella, Bifidobacterium, Parabacteroides, and Alistipes genera and in enterotype distribution between transition and preparation season phase periodization. In the longitudinal study, training phase periodization altered the level of Bacteroides, Blautia, and Bifidobacterium in the microbiome. Such changes in the microbiome were significantly correlated with alternations in aerobic capacity and tended to correlate with the anaerobic power.
Discussion: These findings suggest that periodization alters the gut microbiome abundance related to energy metabolism and trainability of physical fitness. Athlete's condition may thus be mediated to some extent by the microbiota in the intestinal environment.
1. Introduction
The gut microbiota lives in the human gastrointestinal tract; they decompose dietary fiber that the host cannot digest. Further, they are involved in the production of short-chain fatty acids and vitamins that are beneficial to the host. Intestinal bacteria are increasingly reported to be responsible for regulating other biological functions such as immune functions, enzyme activity, and hormone secretion (1). The gut microbiome forms a complex ecosystem and the composition, profile, and diversity of gut bacteria as an aggregate reflects the host health status (2), i.e., loss of diversity in the gut microbiome and subsequent imbalance can give rise to immune allergies and metabolic disorders leading to obesity, metabolic syndrome, and lifestyle-related diseases (3–5). Contrarily, the gut microbiome also plays adaptation in the compositional profile owing to aging, diet habit, or drug metabolism (6, 7). Exercise has been suggested to alter in the composition and function of the gut microbiome, along with promoting energy metabolism and improving physical function (8). A report by Estaki et al. indicates that the gut microbiome profile in healthy individuals is associated with maximal oxygen uptake (VO2max), which is an index of aerobic fitness (9). However, there are few reports regarding the gut microbiome in elite athletes; therefore, elucidation of the gut microbiome composition in athletes remains a topic of interest (8).
Athletes require high-intensity training to improve their physical performance. However, the physical burden of high-intensity training can worsen the physical conditions of athletes (10). Condition deterioration is caused by systemic dysfunction, including the cardiovascular and digestive systems, endocrine responses, and autonomic nervous activity (11), which may manifest as decreased fitness, diminished immune function, increased inflammatory responses, and gastrointestinal disorders, among others (12). In other words, athletes who are in poor condition often complain of decreased heart rate (HR) during exercise; they may also present gastrointestinal symptoms such as diarrhea or stomach aches (10, 13). Further, 30%–50% of athletes are reported to suffer from gastrointestinal symptoms during their training periods (14). Taken together, it seems important to examine the possible relationships between physical fitness and the intestinal environment. The gut microbiome in elite rugby athletes is reported to demonstrate high diversity and is associated with upregulation of pathways responsible for amino acid biosynthesis, carbohydrate metabolism, and short-chain fatty acid synthesis (15). Another study has reported that the extent of training by college swimmer athletes exhibited a positive correlation with the gut microbiome involved in short-chain fatty acid synthesis (16). Training volume and intensity vary from extensive to intensive workload or general to special tasks corresponding to their sports' seasonal periodization structured into macro- (a few months) and meso- (a few weeks) cycles for best performance and attenuating overtraining (17). Taken together, the gut microbiome of athletes may be related to changes in physical fitness and/or condition. However, the changes occurring in the gut microbiome of athletes with changes in fitness during training periodization remain to be studied.
Therefore, the purpose of this study was to investigate the effect of training periodization in athletes on the gut microbiome along with physical fitness. Fecal collection and physical fitness measurements were conducted during separate periodization in elite international level athletes using cross-sectional and longitudinal study designs. We especially focused on the phases of training for elite short-track ice skaters, who require both aerobic and anaerobic capacity because they perform in races involving short, middle, and long-distance skating. We then examined the interrelationship among gut microbiome compositional profiles, stool conditions, and aerobic and anaerobic fitness.
2. Materials and methods
2.1. Participants
The present study used a cross-sectional and a longitudinal design. We recruited Japanese elite athletes who were at the international level and belonged to the national team in their respective sport. In total, 84 athletes (aged 24 ± 5 years, 49 men and 35 women) across different sports participated in the cross-sectional study and 10 elite short-track speed skaters (aged 22 ± 4 years, 4 men and 6 women) participated in the longitudinal study. This study was performed in accordance with the Declaration of Helsinki and was approved by the ethic committee of the Japan Institute of Sports Sciences (049-01 and 049-02) and National Institute of Biomedical Innovation, Health and Nutrition (KENEI 91). All athletes provided written informed consent prior to participating in the study.
2.2. Procedures
2.2.1. Cross-sectional study
Eighty-four athletes were separated into a macro-cycle of training periodization including a transition period (n = 22) (10 men and 12 women) and preparation period (n = 62) (25 men and 37 women). The participants visited the laboratory center once during their training periodization, and fecal samples were collected. The participants belonged to the national team as Olympic reinforcement-designed athletes in one of following sports; Alpine snowboarding, fencing, jumping events, racewalking, rhythmic gymnastics, short distance running events, sailing, short-track speed skating, and soccer.
2.2.2. Longitudinal study
Ten short-track speed skaters (4 men and 6 women) in the preparation period of training periodization visited the laboratory center on two separate meso-cycles at a 3-months interval for general preparation (the beginning of the phase at high volume and low technique training after the transition period) and specific preparation (the end of the phase at low volume and high technique training before the competition period) phase. Before and after the longitudinal periodization, the participants were assessed for physical fitness over two days, an aerobic maximal exercise test on the first day and anaerobic supramaximal pedaling for 90 s on the second day. Further, the fecal samples were collected within 5 days of physical fitness evaluation in each meso-cycle phase of periodization.
2.3. Measurement
2.3.1. Gut microbiome
Fecal samples were collected in commercial vials containing 3 ml guanidine thiocyanate (GuSCN) solution (TechnoSuruga Laboratory Co., Ltd., Shizuoka, Japan), mixed by vortexing, and stored at room temperature (25 °C). The gut microbiome was analyzed as described previously (18). Briefly, the mixture was homogenized using the bead beating method on a Cell Destroyer PS1000 (Bio Medical Sciences, Tokyo, Japan) and DNA was extracted using a Gene Prep Star PI-80X device (Kurabo Industries LTD.). Samples were stored at −30 °C until use. The V3-V4 region of the 16S rRNA gene was amplified from fecal DNA samples using the following primers: forward: 5′-TCGTCGGCAGCGTCAGATGTGTATAAGCGACAGCCTACGGGNGGCWGCAG-3′, and reverse: 5′-GTCTCGTGGGCTCGGAGATGTGTATAAGAGACAGGACTACHVGGGTATC TAATCC-3′. The cycling parameters were as follows: initial denaturation at 95 °C for 3 min, followed by 25 cycles of denaturation (95 °C for 30 s), annealing (55 °C for 30 s), and extension (68 °C for 1 min), and a final extension step at 68 °C for 5 min. After adding the sequencing adapters, the amplicons were sequenced using the Illumina MiSeq platform (Illumina Inc., San Diego, USA) according to the manufacturer's instruction. Sequence reads from Illumina MiSeq were analyzed using Quantitative Insights Into Microbial Ecology (QIIME) and QIIME Analysis Automating Script (Auto-q) as previously described (19). The obtained paired-end reads were selected, and chimeric sequences were removed using USEARCH v6.1. Open-reference operational taxonomic unit (OTU) picking, and taxonomy classification were performed based on sequence similarity (>97%) by using UCLUST software with the SILVA v128 reference sequence.
2.3.2. Stool condition and diet questionnaire
We evaluated the stool frequency, volume, color, and form using a previously developed assessment tool and validated against objective measurements of stool characteristics including stool weight, moisture, hardness, and color in adults (20). The frequency of excretion was evaluated as number of excretions per week using six responses ranging from less than 2 times to more than 7 times. Stool volume was estimated by number of fecal units (response number 1–8, from 1, 0.5 units to 8, >4 units) based on the model stool unit (2 cm diameter × 10 cm length, cylindrical in shape). Stool form was measured based on the modified Bristol stool form scale (21). The seven types range from very hard (type 1) to very loose (type 7). For stool color assessment, the closest of the six colors indicated on the stool assessment tool compared to the actual color was selected according to the color standard Z8721: 1, 5Y8/12 (yellow); 2, 2.5Y7/12 (light yellowish-brown); 3, 10YR5/8 (yellowish-brown); 4, 7.5YR7/12 (brown); 5, 5Y4/4 (greenish-dark brown); 6, 2.5GY4/3 (dark brown). Furthermore, stool odor was evaluated on the scale from 1 to 3 (1, odorless; 2, normal; 3, stronger than usual). Habitual diet during the preceding month were assessed by the brief self-administered diet history questionnaire (BDHQ) (22). The daily intake of protein, fat, and carbohydrate were calculated from the record.
2.3.3. Physical fitness
In the longitudinal study, before and after training periodization, the participants were measured for body composition and for aerobic and anaerobic fitness. Body composition was assessed using air displacement plethysmography (BODPOD; Cosmed, Concord, USA), and percentage body fat and fat-free mass was calculated. Aerobic fitness was measured by a maximal graded exercise test using a cycle ergometer to determine the VO2max. After sufficient warm-up, the exercise started at 120 W for male and 80 W for female athletes, and the work load was increased by 40 W every 3 min until stage 7 and by 20 W every 3 min above stage 8 until they reached an exhaustive state; if one of following criteria: unable to maintain cadence (<80 rpm) for several seconds, attainment of age-predicted maximal HR (220 - age), respiratory exchange ratio >1.1, rating of perceived exertion >19, or clinical complaints of exhaustion by the participants. HR was monitored continuously with wireless Polar sensor (RS800CX; Polar Electro, Finland). The participants were instructed to maintain a cadence of 80 rpm during the test. We measured the average oxygen uptake every 30 s during the exercise test using an online computer-assisted circuit spirometer (AE300S; Minato Medical Science, Osaka, Japan) and evaluated the highest value as VO2max. Ventilatory threshold (VT) was calculated using a regression analysis of the slopes of carbon dioxide production, oxygen ultake, and the minute ventilation plot. In addition, we measured blood lactate concentration at the end of each stage and calculated HR at onset blood lactate accumulation (OBLA: defined as 4 mmol/L) as an index of submaximal aerobic fitness. Anaerobic fitness was measured by a 90 s supramaximal pedaling test on a cycle ergometer (Power Max III; Konami LTD., Tokyo, Japan). The load was set at 7.5% kp of their body weight. The participants were strongly encouraged to maintain pedaling at a maximal effort throughout the entire exercise from the beginning to end. We evaluated the average power output for the initial 30 s.
2.4. Statistical analyses
All statistical analyses were performed using SPSS version 24 (IBM Inc., Chicago, USA) and R version 3.4.2. In the cross-sectional study, continuous, ordinal, and categorical variables were analyzed using an unpaired Student t-test, Mann–Whitney test, and χ2 test. In the longitudinal study, we used a paired Student's t-test or Wilcoxson rank test and r or Cramer's effect size (ES) was calculated to identify the changes in variables before and after training periodization. Univariable Spearman correlation analyses were used to determine the relation between variables of interest. For analyzing the changes in stool form (Bristol scale), direction to the ideal normal form (Type 4) was regarded as the degree of improvement. In the longitudinal study, one athlete dropped out because of lower limb injury (n = 9), one athlete canceled the measurement of anaerobic power pedaling owing to lower limb pain, and another athlete lost the response sheet of the stool condition questionnaire report; the data of anaerobic power and stool condition were analyzed for 8 participants (n = 8). Statistical analyses of the gut microbiome were performed using R software. Principal coordinate analysis (PCoA) based on the Bray-Curtis distance metric was performed using multivariate techniques of global view using the R packages vegan and ade4. Jensen–Shannon divergence was used for enterotype analysis as previously described (23). The top 20 most abundant genera in the microbiome were presented in boxplot analyses. Data are expressed as the means ± SD and number of counts (distribution %) as appropriate. Statistical significance was set a priori at P < 0.05 for all comparisons.
3. Results
Table 1 shows the participant characteristics between the transition and preparation period in the cross-sectional study. The percentage of female, daily intake of diet and macronutrients, and stool conditions were not different between the two groups. There were no differences in the gut microbiome profile between sexes. Figure 1 depicts the gut microbiome community structure by genus level PCoA and enterotype analysis clustering using Jensen–Shannon divergence. The enterotype was characterized by the predominance of three genera, Bacteroides (B), Prevotella (P), and Ruminococcus (R). Athletes in their preparation period exhibited a higher frequency of type B and a lower frequency of type P (X2 = 6.033, P < 0.05) (Figure 1B). Figure 2 shows the comparison of the most abundant gut microbiome at the genus level in the cross-sectional macro-cycle periodization in the transition and preparation period. The distribution of Prevotella was significantly lower and that of Bifidobacterium, Parabacteroides, Alistipes was significantly higher in the preparation period than in the transition period (Figure 2).
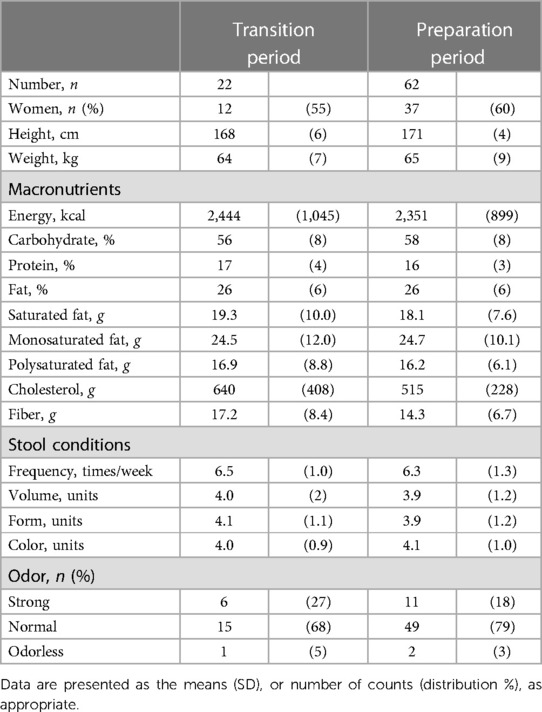
Table 1. The participants’ characteristics and stool condition between transition and preparation period of training periodization.
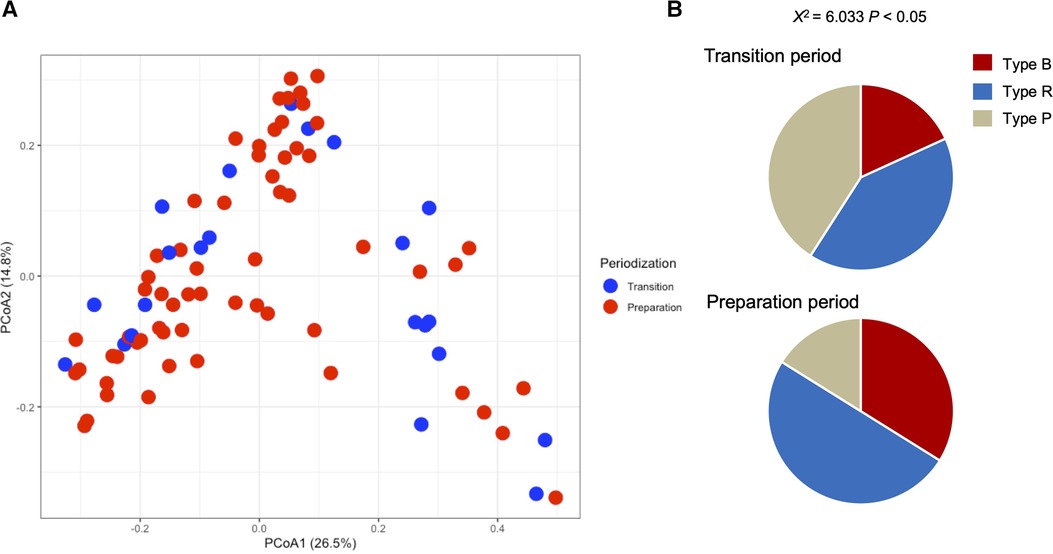
Figure 1. Gut microbiota profile presented by principal coordinate analysis (PCoA, genus level) (A) and the frequency of each enterotype, Bacteroides (Type B), Ruminococcus (Type R), and Prevotella (Type P), in the transition and preparation period (B).
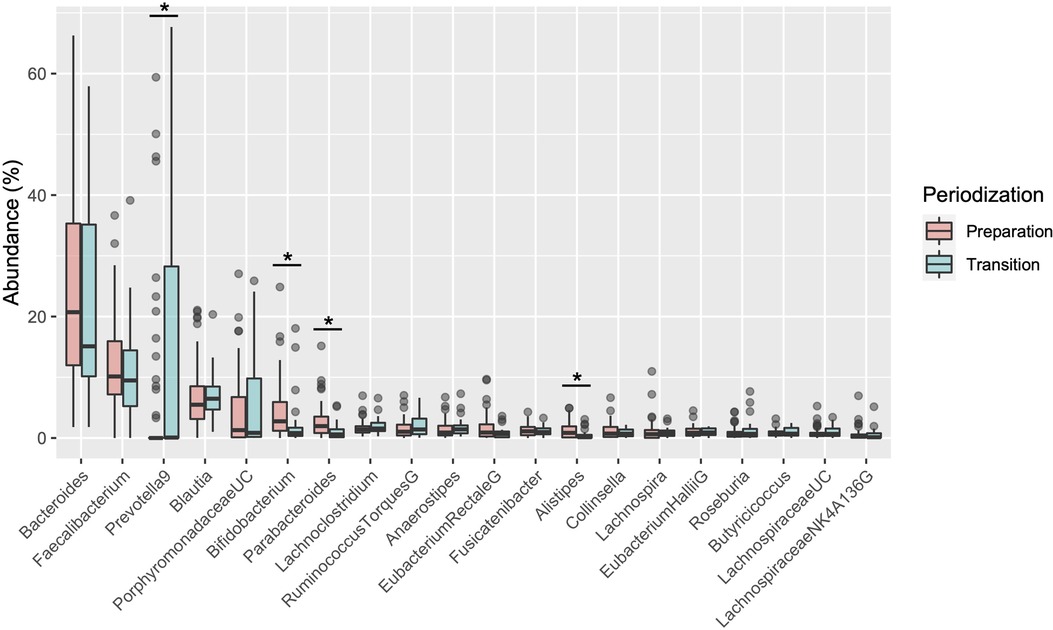
Figure 2. Boxplot of differences in the gut microbiota relative abundance at the genus level. *indicates a significant difference (P < 0.05) between transition and preparation periodization.
Table 2 shows the changes in the participant characteristics in the longitudinal study. The percentage of body fat was significantly lower (P = 0.012, ES = 0.70), and fat-free mass was significantly higher (P = 0.006, ES = 0.75) in specific preparation phase than that of general preparation phase, however, body weight was not changed (P = 0.162, ES = 0.35). In the transition training phase, submaximal aerobic capacity of HR at 4 mmol/L of lactate and anaerobic power output was significantly increased (P = 0.006, ES = 0.77 and P = 0.011, ES = 0.74, respectively), VT tended to increase (P = 0.055, ES = 0.54), but VO2max did not change significantly (P = 0.425, ES = 0.07). There were no significant changes in diet macronutrients and stool conditions such as the frequency (P = 0.257, ES = 0.38), volume (P = 0.395, ES = 0.28), color (P = 0.785, ES = 0.09), form (P = 0.317, ES = 0.33), and odor (P = 0.624, ES = 0.32) (Table 2). Figure 3 depicts the gut microbiome composition profile in each athlete. PCoA of microbial genus abundance indicates that the microbiota profile composition was changed in athlete in the longitudinal study besides over the athletes in the cross-sectional study (Figure 3A). All athletes in the longitudinal study exhibited that the changes were within same enterotype cluster. Moreover, athletes with increased VO2max showed changes in the adverse direction for the genus Bacteroides (negatively both PCoA1 and PCoA2 component). Figure 3B shows the longitudinal changes in the gut microbiome at the phylum level during the general and specific preparation phase. Bacteroidetes phylum distributions were significantly decreased (P = 0.028, ES = 0.73), whereas Firmicutes and Actinobacteria phylum distributions were significantly increased (P = 0.028, ES = 0.73 and P = 0.021, ES = 0.77, respectively) in the specific phase compared to those in the general phase. Moreover, at the genus level, the distribution of Bacteroides was significantly decreased (P = 0.028, ES = 0.69), whereas that of Blautia and Bifidobacterium was significantly increased (P = 0.015, ES = 0.81 and P = 0.021, ES = 0.80, respectively) after training periodization (Figure 4). A tendency of increase in Fusicatenibater and Anaerostipes distribution was observed (P = 0.066, ES = 0.61 and P = 0.066, ES = 0.61, respectively). The relationships between the gut microbiome and physical fitness, in which changes were examined before and after preparation periodization, are shown in Figure 5. Significant correlations were evidenced between the changes in VO2max and Bacteroides (r = −0.667, P < 0.05) (Figure 5A), and a tendency of correlation was observed in the changes in anaerobic power output and Fusicatenibacter (r = 0.677, P = 0.071) (Figure 5B). Improvement in the Bristol scale was significantly correlated with changes in Fusicatenibacter abundance (r = 0.671, P < 0.05) (Figure 5C), and anaerobic power output (r = 0.730, P < 0.05) (Figure 5D).
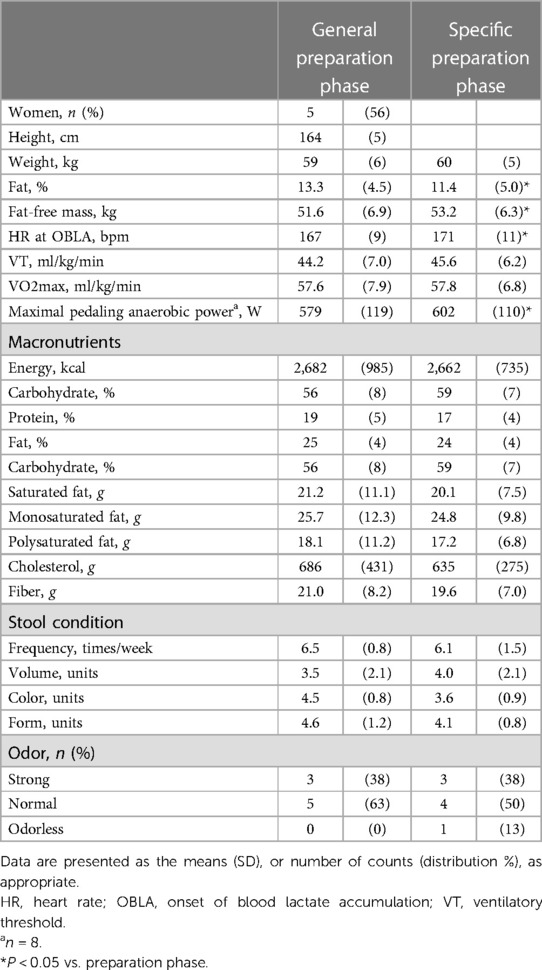
Table 2. The participants’ characteristics and stool conditions in general and specific preparation phase of training periodization.
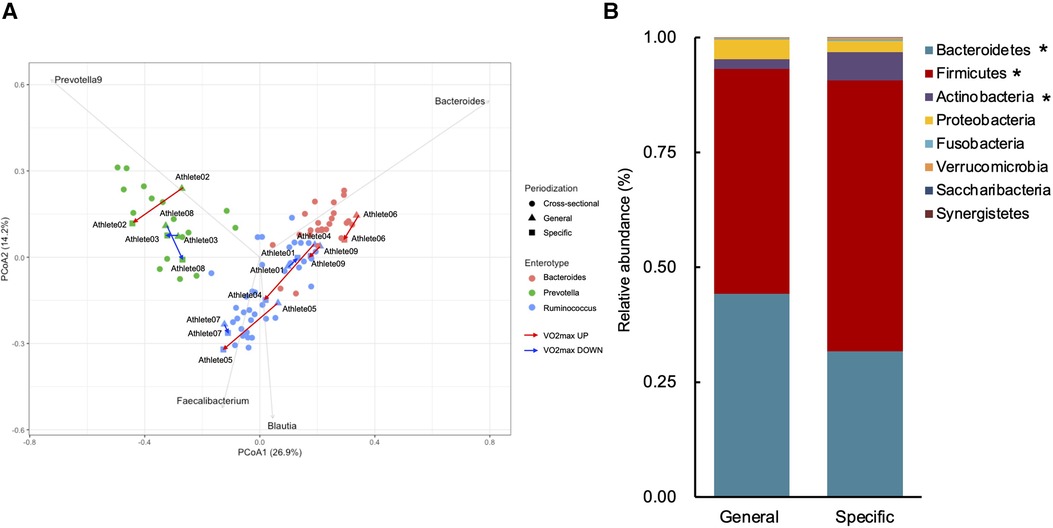
Figure 3. Gut microbiota profile presented by principal coordinate analysis (PCoA, genus level) (A). Triangles indicate the preparation training phase, squares indicate the transition training phase, and circles indicate the cross-sectional study. Gray arrows indicate enterotype drivers; Bacteroides (pink), Prevotella (green), and Rumicnococcus (Blutia & Faecalibacterium) (blue). Red arrows indicate increased VO2max athletes and blue arrows indicate decreased VO2max athletes. Comparison of gut microbiota relative abundance at the phylum level (B). *indicates a significant change (P < 0.05) between the general and specific preparation phase periodization.
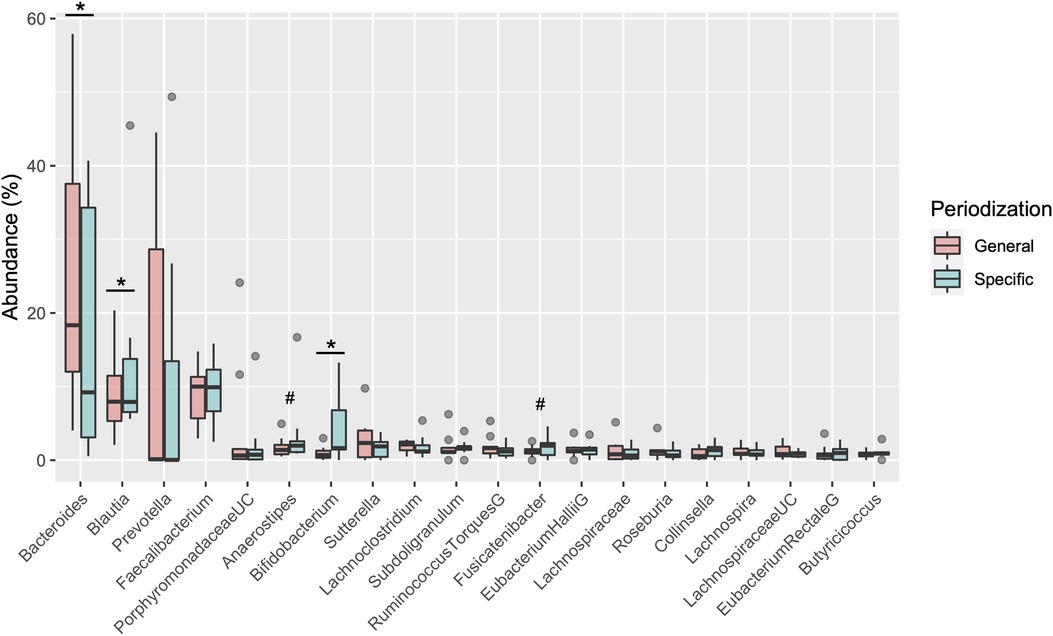
Figure 4. Boxplot of changes in gut microbiota relative abundance at the genus level. *indicates a significant difference (P < 0.05) and # indicates tended to a difference (P = 0.066) before and after the periodization.
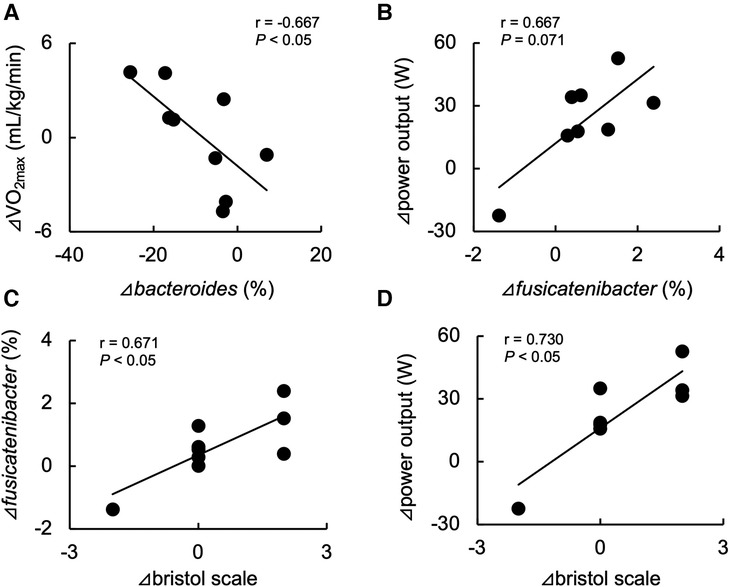
Figure 5. Relationship between changes in maximal oxygen uptake (VO2max) and Bacteroides abundance (A), anaerobic power output and Fusicatenibacter abundance (B), and improvements in the Bristol scale and Fusicatenibacter abundance (C) and anaerobic power output (D) before and after the preparation phase periodization.
4. Discussion
The present study investigated the impact of training periodization on the gut microbiome, stool condition, and physical fitness in elite Japanese athletes. The salient findings of the study were as follows: we observed significant differences in enterotype distribution and the abundance of genera such as Prevotella, Bifidobacterium, Alistipes, and Parabacteroides in the microbiome during the macro cycle of periodization in the cross-sectional design. We also observed changes in Bacteroides, Blautia, and Bifidobacterium in the microbiome along with a tendency for increase in the genera Fusicatenibacter and Anaerostipes in the longitudinal phase of the preparation period. In addition, a decrease in the genus Bacteroides was correlated with a change in VO2max, and an increase in Fusicatenibacter tended to be associated with enhancement of anaerobic power. These results suggest that changes in the gut microbiome of athletes by season and/or training may be associated with their physical fitness and condition.
Emerging evidence has demonstrated the relation among the gut microbiome, lifestyle, and several diseases; researchers have also focused on physical fitness and exercise training (8). In the present study, a difference was observed in the prevalence of gut microbial clusters categorized as Bacteroides, Prevotella, Ruminococcus enterotypes (4). We observed a higher percentage of the Bacteroides enterotype and a lower percentage of the Prevotella enterotype in the preparation period than that in the transition period of the cross-sectional design. The Bacteroides enterotype is associated with long-term consumption of a protein- and animal fat-rich diet, whereas the Prevotella enterotype is associated with a carbohydrate- and fiber-rich diet (24). Prevotella species are reported to exert a beneficial effect in protecting against Bacteroides-induced glucose intolerance and improving glucose metabolism (25). Based on the genus composition of the gut microbiome, abundance of Prevotella was significantly higher in the transition period than in the preparation period (Figure 2). A previous study showed that diet intervention contained high-fat/low-fiber or low-fat/high-fiber for 10 days affected gut microbiome composition but could not change the enterotype in healthy adults (24). In the current study, we first performed the cross-sectional and longitudinal changes in the athlete's microbiome without dietary intake variance. Interestingly, the results revealed that the changes in the microbiome components depend on individual variability and that some skate athletes showed large changes inter-individually, but no changes were found in the enterotype. Taken together, these results indicate that the gut microbiome profile show changes as large as inter-individual differences, but these changes remain within the same cluster.
The abundances of Bifidobacterium, Prevotella, Parabacteroides, and Alistipes genera were significantly different in the cross-sectional study between the transition and preparation period; in the longitudinal design, the abundance of Bifidobacterium and Blautia increased and that of the Bacteroides genus decreased after the specific preparation phase. Bifidobacterium was detected as a significant genus in both the cross-sectional and longitudinal studies. Bifidobacterium, Prevotella or Blautia are the predominant bacteria often found in the intestine of Japanese individuals, and are considered to have beneficial effects on intestinal homeostasis through enhanced energy metabolism, anti-inflammatory action, and maintenance of intestinal pH, by fermenting polysaccharides into organic acids such as lactic acid, acetic acid, and butyric acid (11, 26). The genus Bifidobacterium has been previously used as a probiotic, and dietary supplements combined with the genera Lactobacillus and Streptococcus have been reported to extend athlete exhaustion time under heat stress in long-distance running (27). These findings suggest that training for preparation periodization induces changes in the specific microbiome composition including Bifidobacterium, rather than in a single genus, which may have an ergogenic effect on physical function.
Cronin et al. reported that no significant changes in gut microbiome were observed in healthy individuals even after combined aerobic and resistance exercise at mild intensity 3 day/week for eight weeks (28). Meanwhile, Allen et al. examined the compositional profile of the gut microbiome in lean and obese people and reported that the gut microbiome composition was significantly different before the beginning of exercise training; however, there was no difference after six weeks of moderate-intensity aerobic exercise training, and the abundance of Bacteroides and Faecalibacterium was changed (29). Karl et al. showed that high-intensity interval training in military personnel altered the abundance of Bacteroidetes and Firmicutes phyla over a short period of four days, significantly changing the compositional profile of the gut microbiome (30). In a study on rower athletes, the gut microbiome exhibited a decreasing tendency in the abundance of Bacteroides, and an increasing tendency in abundance of Rosebuila and Subdoligranulim in all athletes during the competition season with improved physical condition (31). In the present longitudinal study, we demonstrated decreased an abundance of the phylum Bacteroidetes and genus Bacteroides, and an increased abundance of the phylum Firmicutes in the specific preparation phase, which was consistent with previous studies. Similarly, the increased Firmicutes and Bacteroidetes ratio (F/B) has been associated with higher VO2max in general heathy young adults (32). On the contrary, the compositional ratio of F/B is elevated in obese populations with a body mass index (BMI) >30 (33, 34). However, in Japanese cohorts, as obese individuals forma minority, no relationship between F/B and BMI has been demonstrated in the normal range of BMI (35). As the consensus of this association could not be confirmed, the increased F/B in the longitudinal study could be interpreted as a gut microbiome adaptation contributing to the efficient absorption of ingested micronutrients with much energy expenditure during preparation periodization.
A previous study has reported that the aerobic fitness of VO2max is positively correlated with the microbiome, and is related to the production of short-chain fatty acids, which serve as energy sources through the genera Lacnospiraceae, Roseburia, and Clostridiales (9). In the current study, although VO2max was not significantly altered by training periodization, the changes in individual VO2max were negatively correlated with the abundance of Bacteroides. Furthermore, our PCoA supported this relationship, our findings indicated that increased VO2max showed the same change direction of decreased in both PCoA1 and PCoA2 components which were mainly against the predominance of Bacteroides (Figure 3). Bacteroides is known to play a role in the metabolism of organic acids, bile acids, and proteins, leading to regulation of energy homeostasis in the host (36). An overabundance of protein promotes fermentation of amino acids by the genus Bacteroides in the gastrointestinal tract, which then accumulates toxic by-products such as amines, phenols, hydrogen sulfide, indoles, and ammonia (37). An excessive increase in circulating ammonia during intensive exercise has been associated with central fatigue and with reduced exhaustion performance (38). Taken together, a decrease in the genus Bacteroides seems to attenuate ammonia elevation during exercise and changes the maximal graded exercise test performance. On the other hand, there is no correlations between submaximal aerobic capacity such as VT or OBLA and microbial changes. These results suggest that changes in the abundance of Bacteroides among other gut microbiome components are partly related to the trainability of maximal rather than submaximal aerobic fitness in athletes.
Anaerobic power performance as determined by supramaximal pedaling was significantly increased after the specific preparation phase, and the degree of change tended to be related to elevated levels of the genus Fusicatenibacter, which showed an increasing tendency among gut microbiomes. Bacteria of the genus Fusicatenibacter promote lactose decomposition and produce organic acids in the intestine, including lactic acid and short-chain fatty acids (39). These organic acids, when absorbed by the large intestine, pass through the hepatic portal vein, and are metabolized to glycogen and fatty acids in the liver and muscles to provide energy (40). Gut bacteria may thus be associated with glycolytic energy metabolism mechanisms such as glycogen storage and lactic acid metabolism in muscles.
In this study, we used the Bristol scale (41) to evaluate the stool form condition status. The Bristol scale assesses the form of fecal matter across seven types, ranging from hard stools with long intestinal transit time and high water absorption to watery stools with short intestinal transit time and low water absorption (42). A study in Japanese adults showed that stool form is related to the intestinal environment and that quality-of-life indices are rated lower for individuals with constipation (43). In this study, no changes were observed in the mean values of the Bristol scale scores during the periodization. However, analysis of the degree of improvement in the Bristol scale with athlete periodization revealed a significant correlation with changes in the genus Fusicatenibacter and anaerobic power performance when change from hard or watery stools to normal fecal form in the ideal direction was determined as the degree of improvement. However, this relation was not observed in aerobic fitness. The genus Fusicatenibacter may mediate the link between improvement of stool condition and enhancement of anaerobic power performance. Taken together, stool form may be a surrogate marker for evaluating gastrointestinal and physical conditions.
The present study had some limitations. First, this study was conducted small sample size. It is reported that sex, sports characteristics, or sports specific diet would affect gut microbial structure (35, 44). Because we recruited only elite athletes who are international level in various sports, the number in each sport was very small, even in longitudinal study. For that reason, we might not have found differences in gut microbiome or macronutrient among sexes and sport events in the present study. Therefore, the results of present study may limit to generalize our findings to specific athlete. Next, this study was observational cross-sectional and longitudinal design and we found aerobic and anaerobic capacity correlated with some gut microbiome or stool condition. Therefore, we cannot establish a causal relationship between physical fitness and stool condition. Furthermore, we did not evaluate mechanistic insight. Barton et al. have reported that gut microbiome of athletes is associated with metabolic pathway including synthesis of organic enzyme, carbohydrate degradation, and short-chain fatty acid compared to sedentary subject (15). Therefore, further studies are warranted to investigate the relationship between physical fitness and gastrointestinal environment with metabolic function in the interventional design.
In conclusion, the gut microbiome of athletes was cross-sectionally and longitudinally examined during training periodization in this study. Certain changes were observed in the gut microbiome including a decrease in abundance of the genus Bacteroides, an increase in genus Blautia and Bifidobacterium, and an increasing trend for the genus Fusicatenibacter. Furthermore, the degree of improvement in the altered gut microbiome and defecation status were related to changes in VO2max and anaerobic power. These findings indicate that stool condition can be employed to assess the condition of athletes, and suggest the possibility of intervention targeting specific gut microbiome flora for improved conditioning.
Data availability statement
The original contributions presented in the study are included in the article, further inquiries can be directed to the corresponding authors on reasonable request.
Ethics statement
The studies involving human participants were reviewed and approved by the Ethic Committee of the Japan Institute of Sports Sciences and National Institute of Biomedical Innovation, Health and Nutrition. Written informed consent to participate in this study was provided by the participants’ legal guardian/next of kin.
Author contributions
NA and MM: contributed to conception and design of the study. NA, MN, NE, MH, TN, and HN: contributed to the subject recruitment and data collection. JP, KH, KM, and JK: were involved in processing and analyzing the sequence data. NA: wrote the draft of the manuscript. MM: edited and revised manuscript. All authors contributed to the article and approved the submitted version.
Funding
This work was supported by MIZUNO SPORTS PROMOTION FOUNDATION to NA, Health and Labour Sciences Research Grant (grant no. 201709002B) and JSPS KAKENHI (grant no. 16K00944 and 20H04117) to MM, and the Ministry of Health and Welfare of Japan and Public/Private R&D Investment Strategic Expansion PrograM: PRISM (grant no. 20AC5004) and the Japan Agency for Medical Research and Development (AMED) (grant no. JP22gm1010006h0004 and JP22ae0121042h0002) to JK.
Acknowledgments
This study was conducted as a part of the Sports Medicine/Science Research Program of Japan Institute of Sports Sciences (JISS). The authors wish to thank the participants for their invaluable contribution to the study and thank the staff and coaches in the Japan Skating Federation.
Conflict of interest
The authors declare that the research was conducted in the absence of any commercial or financial relationships that could be construed as a potential conflict of interest.
Publisher's note
All claims expressed in this article are solely those of the authors and do not necessarily represent those of their affiliated organizations, or those of the publisher, the editors and the reviewers. Any product that may be evaluated in this article, or claim that may be made by its manufacturer, is not guaranteed or endorsed by the publisher.
References
1. Flint HJ, Duncan SH, Scott KP, Louis P. Links between diet, gut microbiota composition and gut metabolism. Proc Nutr Soc. (2015) 74:13–22. doi: 10.1017/S0029665114001463
2. Clark A, Mach N. The crosstalk between the gut microbiota and mitochondria during exercise. Front Physiol. (2017) 8:319. doi: 10.3389/fphys.2017.00319
3. Qin J, Li Y, Cai Z, Li S, Zhu J, Zhang F, et al. A metagenome-wide association study of gut microbiota in type 2 diabetes. Nature. (2012) 490:55–60. doi: 10.1038/nature11450
4. Arumugam M, Raes J, Pelletier E, Le Paslier D, Yamada T, Mende DR, et al. Enterotypes of the human gut microbiome. Nature. (2011) 473:174–80. doi: 10.1038/nature09944
5. Sheng S, Yan S, Chen J, Zhang Y, Wang Y, Qin Q, et al. Gut microbiome is associated with metabolic syndrome accompanied by elevated gamma-glutamyl transpeptidase in men. Front Cell Infect Microbiol. (2022) 12:946757. doi: 10.3389/fcimb.2022.946757
6. O’Toole PW, Jeffery IB. Microbiome-health interactions in older people. Cell Mol Life Sci. (2018) 75:119–28. doi: 10.1007/s00018-017-2673-z
7. Jandhyala SM, Talukdar R, Subramanyam C, Vuyyuru H, Sasikala M, Nageshwar Reddy D. Role of the normal gut microbiota. World J Gastroenterol. (2015) 21(29):8787–803. doi: 10.3748/wjg.v21.i29.8787
8. Dalton A, Mermier C, Zuhl M. Exercise influence on the microbiome-gut-brain axis. Gut Microbes. (2019) 10:555–68. doi: 10.1080/19490976.2018.1562268
9. Estaki M, Pither J, Baumeister P, Little JP, Gill SK, Ghosh S, et al. Cardiorespiratory fitness as a predictor of intestinal microbial diversity and distinct metagenomic functions. Microbiome. (2016) 4:42. doi: 10.1186/s40168-016-0189-7
10. MacKinnon LT. Special feature for the olympics: effects of exercise on the immune system: overtraining effects on immunity and performance in athletes. Immunol Cell Biol. (2000) 78:502–9. doi: 10.1111/j.1440-1711.2000.t01-7-.x
11. Clark A, Mach N. Exercise-induced stress behavior, gut-microbiota-brain axis and diet: a systematic review for athletes. J Int Soc Sports Nutr. (2016) 13:43. doi: 10.1186/s12970-016-0155-6
12. Purvis D, Gonsalves S, Deuster PA. Physiological and psychological fatigue in extreme conditions: overtraining and elite athletes. PMR. (2010) 2:442–50. doi: 10.1016/j.pmrj.2010.03.025
13. Waterman JJ, Kapur R. Upper gastrointestinal issues in athletes. Curr Sports Med Rep. (2012) 11:99–104. doi: 10.1249/JSR.0b013e318249c311
14. Diduch BK. Gastrointestinal conditions in the female athlete. Clin Sports Med. (2017) 36:655–69. doi: 10.1016/j.csm.2017.06.001
15. Barton W, Penney NC, Cronin O, Garcia-Perez I, Molloy MG, Holmes E, et al. The microbiome of professional athletes differs from that of more sedentary subjects in composition and particularly at the functional metabolic level. Gut. (2018) 67:625–33. doi: 10.1136/gutjnl-2016-313627
16. Hampton-Marcell JT, Eshoo TW, Cook MD, Gilbert JA, Horswill CA, Poretsky R. Comparative analysis of gut microbiota following changes in training volume among swimmers. Int J Sports Med. (2020) 41:292–9. doi: 10.1055/a-1079-5450
17. Plisk SS, Stone MH. Periodization strategies. Strength Cond. (2003) 25:19–37. doi: 10.1519/1533-4295(2003)025%3C0019:PS%3E2.0.CO;2
18. Hosomi K, Ohno H, Murakami H, Natsume-Kitatani Y, Tanisawa K, Hirata S, et al. Method for preparing DNA from feces in guanidine thiocyanate solution affects 16S rRNA-based profiling of human microbiota diversity. Sci Rep. (2017) 7:4339. doi: 10.1038/s41598-017-04511-0
19. Mohsen A, Park J, Chen YA, Kawashima H, Mizuguchi K. Impact of quality trimming on the efficiency of reads joining and diversity analysis of illumina paired-end reads in the context of QIIME1 and QIIME2 microbiome analysis frameworks. BMC Bioinformatics. (2019) 20:581. doi: 10.5281/zenodo.1439555
20. Ohno H, Murakami H, Tanisawa K, Konishi K, Miyachi M. Validity of an observational assessment tool for multifaceted evaluation of faecal condition. Sci Rep. (2019) 9:3760. doi: 10.1038/s41598-019-40178-5
21. Heaton KW, Radvan J, Cripps H, Mountford RA, Braddon FE, Hughes AO. Defecation frequency and timing, and stool form in the general population: a prospective study. Gut. (1992) 33:818–24. doi: 10.1136/gut.33.6.818
22. Kobayashi S, Murakami K, Sasaki S, Okubo H, Hirota N, Notsu A, et al. Comparison of relative validity of food group intakes estimated by comprehensive and brief-type self-administered diet history questionnaires against 16 d dietary records in Japanese adults. Public Health Nutr. (2011) 14(7):1200–11. doi: 10.1017/S1368980011000504
23. Park J, Kato K, Murakami H, Hosomi K, Tanisawa K, Nakagata T, et al. Comprehensive analysis of gut microbiota of a healthy population and covariates affecting microbial variation in two large Japanese cohorts. BMC Microbiol. (2021) 21(1):151. doi: 10.1186/s12866-021-02215-0
24. Wu GD, Chen J, Hoffmann C, Bittinger K, Chen YY, Keilbaugh SA, et al. Linking long-term dietary patterns with gut microbial enterotypes. Science. (2011) 334:105–8. doi: 10.1126/science.1208344
25. Kovatcheva-Datchary P, Nilsson A, Akrami R, Lee YS, De Vadder F, Arora T, et al. Dietary fiber-induced improvement in glucose metabolism is associated with increased abundance of prevotella. Cell Metab. (2015) 22:971–82. doi: 10.1016/j.cmet.2015.10.001
26. Mattarelli P, Holzapfel W, Franz CMAP, Endo A, Felis GE, Hammes W, et al. Recommended minimal standards for description of new taxa of the genera Bifidobacterium, Lactobacillus and related genera. Int J Syst Evol Microbiol. (2014) 64:1434–51. doi: 10.1099/ijs.0.060046-0
27. Shing CM, Peake JM, Lim CL, Briskey D, Walsh NP, Fortes MB, et al. Effects of probiotics supplementation on gastrointestinal permeability, inflammation and exercise performance in the heat. Eur J Appl Physiol. (2014) 114:93–103. doi: 10.1007/s00421-013-2748-y
28. Cronin O, Barton W, Skuse P, Penney NC, Garcia-Perez I, Murphy EF, et al. A prospective metagenomic and metabolomic analysis of the impact of exercise and/or whey protein supplementation on the gut microbiome of sedentary adults. MSystems. (2018) 3:e00044–18. doi: 10.1128/mSystems.00044-18
29. Allen JM, Mailing LJ, Niemiro GM, Moore R, Cook MD, White BA, et al. Exercise alters gut microbiota composition and function in lean and obese humans. Med Sci Sports Exerc. (2018) 50:747–57. doi: 10.1249/MSS.0000000000001495
30. Karl JP, Margolis LM, Madslien EH, Murphy NE, Castellani JW, Gundersen Y, et al. Changes in intestinal microbiota composition and metabolism coincide with increased intestinal permeability in young adults under prolonged physiological stress. Am J Physiol Gastrointest Liver Physiol. (2017) 312:G559–71. doi: 10.1152/ajpgi.00066.2017
31. Keohane DM, Woods T, O’Connor P, Underwood S, Cronin O, Whiston R, et al. Four men in a boat: ultra-endurance exercise alters the gut microbiome. J Sci Med Sport. (2019) 22:1059–64. doi: 10.1016/j.jsams.2019.04.004
32. Durk RP, Castillo E, Márquez-Magaña L, Grosicki GJ, Bolter ND, Lee CM, et al. Gut microbiota composition is related to cardiorespiratory fitness in healthy young adults. Int J Sport Nutr Exerc Metab. (2019) 29:249–53. doi: 10.1123/ijsnem.2018-0024
33. Ley RE, Turnbaugh PJ, Klein S, Gordon JI. Microbial ecology: human gut microbes associated with obesity. Nature. (2006) 444:1022–3. doi: 10.1038/4441022a
34. Koliada A, Syzenko G, Moseiko V, Budovska L, Puchkov K, Perederiy V, et al. Association between body mass index and firmicutes/bacteroidetes ratio in an adult Ukrainian population. BMC Microbiol. (2017) 17:120. doi: 10.1186/s12866-017-1027-1
35. Takagi T, Naito Y, Inoue R, Kashiwagi S, Uchiyama K, Mizushima K, et al. Differences in gut microbiota associated with age, sex, and stool consistency in healthy Japanese subjects. J Gastroenterol. (2019) 54:53–63. doi: 10.1007/s00535-018-1488-5
36. Nicholson JK, Holmes E, Kinross J, Burcelin R, Gibson G, Jia W, et al. Host-gut microbiota metabolic interactions. Science. (2012) 336:1262–7. doi: 10.1126/science.1223813
37. den Besten G, van Eunen K, Groen AK, Venema K, Reijngoud DJ, Bakker BM. The role of short-chain fatty acids in the interplay between diet, gut microbiota, and host energy metabolism. J Lipid Res. (2013) 54:2325–40. doi: 10.1194/jlr.R036012
38. Guezennec CY, Abdelmalki A, Serrurier B, Merino D, Bigard X, Berthelot M, et al. Effects of prolonged exercise on brain ammonia and amino acids. Int J Sports Med. (1998) 19:323–7. doi: 10.1055/s-2007-971925
39. Takada T, Kurakawa T, Tsuji H, Nomoto K. Fusicatenibacter saccharivorans gen. Nov., sp. Nov., isolated from human faeces. Int J Syst Evol Microbiol. (2013) 63:3691–6. doi: 10.1099/ijs.0.045823-0
40. Mascolo N, Rajendran VM, Binder HJ. Mechanism of short-chain fatty acid uptake by apical membrane vesicles of rat distal colon. Gastroenterology. (1991) 101:331–8. doi: 10.1016/0016-5085(91)90008-9
41. Lewis SJ, Heaton KW. Stool form scale as a useful guide to intestinal transit time. Scand J Gastroenterol. (1997) 32:920–4. doi: 10.3109/00365529709011203
42. Degan LP, Phillips SF. How well does stool form reflect colonic transit? Gut. (1996) 33:818–24. doi: 10.1136/gut.39.1.109
43. Tanabe A, Adachi K, Yamaguchi Y, Izawa S, Yamamoto S, Hijikata Y, et al. Gut environment and dietary habits in healthy Japanese adults and their association with bowel movement. Digestion. (2020) 101:706–16. doi: 10.1159/000501961
Keywords: gut microbiota, stool condition, fitness, training season, athlete
Citation: Akazawa N, Nakamura M, Eda N, Murakami H, Nakagata T, Nanri H, Park J, Hosomi K, Mizuguchi K, Kunisawa J, Miyachi M and Hoshikawa M (2023) Gut microbiota alternation with training periodization and physical fitness in Japanese elite athletes. Front. Sports Act. Living 5:1219345. doi: 10.3389/fspor.2023.1219345
Received: 9 May 2023; Accepted: 4 July 2023;
Published: 14 July 2023.
Edited by:
Jan Jacek Kaczor, University of Gdansk, PolandReviewed by:
Bruce Rogers, University of Central Florida, United StatesArianna Mazzoli, University of Naples Federico II, Italy
© 2023 Akazawa, Nakamura, Eda, Murakami, Nakagata, Nanri, Park, Hosomi, Mizuguchi, Kunisawa, Miyachi and Hoshikawa. This is an open-access article distributed under the terms of the Creative Commons Attribution License (CC BY). The use, distribution or reproduction in other forums is permitted, provided the original author(s) and the copyright owner(s) are credited and that the original publication in this journal is cited, in accordance with accepted academic practice. No use, distribution or reproduction is permitted which does not comply with these terms.
*Correspondence: Nobuhiko Akazawa bm9idWhpa28uYWthemF3YUBhb25pLndhc2VkYS5qcA== Motohiko Miyachi bWl5YWNoaW1Ad2FzZWRhLmpw