- 1Interdepartmental Microbiology Program, Iowa State University, Ames, IA, United States
- 2Department of Food Science and Human Nutrition, Iowa State University, Ames, IA, United States
- 3Eastern Regional Research Center, Agricultural Research Service, United States Department of Agriculture, Wyndmoor, PA, United States
- 4School of Agriculture, University of Wisconsin-Platteville, latteville, WI, United States
The main objective of this study was to evaluate the tolerance of stationary phase (STAT) and long-term survival phase (LTS) Salmonella Enteritidis ATCC 13076 to atmospheric cold plasma (ACP) in phosphate buffered saline (PBS, pH 7.0) and on shell eggs. Salmonella Enteritidis was cultured in tryptic soy broth supplemented with 0.6% (w/v) yeast extract (35°C) for 20 h (STAT) and 21 days (LTS). Cell morphology was determined by light microscopy. The PBS and shell eggs were inoculated with STAT or LTS cells to obtain ∼7.0 log10 CFU/mL or egg. The ACP was applied at 45 kV (PBS) and 60 kV (shell eggs) for 1–4 min and 1–5 min, respectively. Pathogen survivors were enumerated on thin agar layer (TAL) medium and on xylose lysine tergitol-4 (XLT-4) agar after 48 h of incubation (35°C). For survivors on shell eggs, R2 and mean square error values were obtained using Log-linear with Tail and Weibull models. The STAT cells were predominantly rod-shaped whereas LTS cells were coccoid. In PBS, reductions (log10 CFU/mL) of STAT cells were 1.0, 0.95, 1.45, and 1.44 after exposure to ACP for 1, 2, 3, and 4 min, respectively. In contrast, reductions in LTS cells were significantly lower (p< 0.05) at 0.04 (1 min), 0.06 (2 min) 0.01 (3 min), and 0.11 (4 min). A similar pattern was observed for shell eggs whereby LTS cells exhibited much higher tolerance to ACP than STAT cells (p < 0.05). The Log-linear with Tail model produced a better fit of the survival data for STAT cells; times to achieve a 4- and 5- log reduction were 5.29 and 5.78 min, respectively. Sub-lethal injury occurred in both STAT and LTS survivors; however, differences were not significant (P > 0.05). Additionally, there were no observed differences in shell strength and yolk color between ACP-treated and control eggs. Based on these results, LTS cells of S. Enteritidis are more tolerant to ACP than STAT cells and should be considered when developing process validation protocols involving application of ACP to inactivate Salmonella on shell eggs.
1 Introduction
The Centers for Disease Control and Prevention (CDC) estimates that Salmonella is the leading cause of bacterial foodborne illnesses with approximately 1.35 million cases, 26,500 hospitalizations and 420 deaths in the United States annually. In this respect, poultry and poultry products are major vehicles for this pathogen to consumers (CDC, 2021). Salmonella Enteritidis is commonly associated with egg outbreaks and most often contaminates the outer surface of shell eggs via poultry feces. In 2018, Gravil Ridge Farms issued a recall of shell eggs contaminated with S. Enteritidis that resulted in 44 cases and 12 hospitalizations (CDC, 2018). Two S. Enteritidis strains in eggs from Poland were responsible for a large outbreak of salmonellosis that occurred among 18 European countries (Pijnacker et al., 2019).
Globally, eggs are a highly nutritious household staple that provide essential vitamins, amino acids, fatty acids, folate and other micronutrients (Keerthirathne et al., 2017) According to recent estimates, the per capita egg consumption in the United States in 2023 was 281.3 eggs and is projected to reach 284.4 eggs in 2024 (Statistica, 2024). To improve the microbial safety of eggs, several countries including United States, Canada and Japan, employ a chlorine wash (Georgescu et al., 2017; Wan et al., 2017) for reducing the microbial load on the outer surface of shell eggs.
Chlorine is the most widely used sanitizer for shell eggs because it is relatively inexpensive; however, there are several limitations associated with its use. These include its inactivation by organic matter, reaction with organic matter to form harmful chloro-organic by-products (Goslan et al., 2009; Ölmez and Kretzschmar, 2009), pitting of stainless steel equipment at lower pH (<6.5), and decreased antimicrobial effectiveness at higher pH (Nou and Luo, 2010; Tirpanalan et al., 2011). These limitations have fueled the food industry’s interest in seeking alternatives to the use of chlorine as a sanitizing agent (Meireles et al., 2016). Moreover, negative consumer perception of synthetic chemical sanitizers (Buchmüller et al., 2020) has also contributed to the food industry’s interest in alternative antimicrobial treatments. While heat pasteurization may be used as a decontamination method, it can cause quality defects such as egg coagulation and protein denaturation. To destroy Salmonella on shell eggs while mitigating those quality defects, researchers have investigated some non-thermal methods such as acidic electrolyzed water, high pressure carbon dioxide, radio frequency (Keerthirathne et al., 2017), and cold plasma (Gavahian et al., 2019; Illera et al., 2022; Wan et al., 2017).
A novel non-thermal method to kill pathogens on the outer surface of shell eggs involves the use of atmospheric cold plasma (ACP). Cold plasma is a non-equilibrated ionized gas containing an assortment of atoms, molecules, ions, free radicals and other reactive species coexisting in both grounded and excited states (Bourke et al., 2017; Misra et al., 2016). Inactivation of microorganisms by ACP results from additive and/or synergistic effects of various reactive oxygen species (ROS) and reactive nitrogen species (RNS) (Deng et al., 2010; Scholtz et al., 2015). An important advantage of ACP is that microbial inactivation can be achieved with negligible heat damage to food (Niemira, 2012). More recently, no differences in quality characteristics of treated and non-treated whole chicken eggs were reported following ACP inactivation of Salmonella by more than 5.0 log10 CFU/egg (Illera et al., 2023; Abdoli et al., 2024). There are several reports on the inactivation of enteric pathogens on the surface of shell eggs using ACP (Dasan et al., 2018; Gavahian et al., 2019; Georgescu et al., 2017; Lin et al., 2020; Moritz et al., 2021; Wan et al., 2017). However, in all those studies the eggs were artificially inoculated with pathogens in the stationary phase (STAT) of the bacterial life cycle.
The four most recognized phases of the bacterial life cycle are lag, exponential, stationary, and death phase. However, there is a fifth lesser recognized phase called the long-term stationary phase (Finkel, 2006), most recently referred to as the long-term-survival phase (LTS) (Bhullar et al., 2021; Djebbi-Simmons et al., 2019; Wang et al., 2018; Wen et al., 2009). Bacterial transition into this phase is induced by environmental factors such as nutrient limitation, desiccation, waste accumulation, competition, or other stressors (Finkel, 2006). The LTS bacteria are phenotypic variants, that transform from rod-shaped cells to coccobacilli or coccoid cells as they transition through the life cycle (Wang, 2017; Wen et al., 2011). Interestingly, LTS bacteria display an increased tolerance to antibacterial treatments such as high heat and pressure (Wen et al., 2011), UV radiation (Wang et al., 2018), ampicillin (Wang, 2017) and chlorine (Bhullar et al., 2021; Djebbi-Simmons et al., 2019).
In microbial food safety research, a common practice is to use STAT cells of enteric pathogens when evaluating antibacterial treatments and validating the microbial safety of food processes. According to Finkel (2006), bacteria in the natural environment spend most of their life as LTS cells. Therefore, raw agricultural products destined for food processing are more likely to be contaminated with LTS pathogens from environmental sources. Although STAT cells are more robust than exponential phase (EXP) cells; there is increasing evidence suggesting that LTS cells exhibit a higher tolerance to physical or chemical antimicrobial treatments (Bhullar et al., 2021; Djebbi-Simmons et al., 2019; Wang, 2017; Wang et al., 2018; Wen et al., 2009). While there is a growing body of knowledge on inactivation of salmonellae on shell eggs using ACP (Georgescu et al., 2017; Wan et al., 2017), there are no published reports on the inactivation of LTS Salmonella on those eggs by ACP. Accordingly, the main objective of the present study was to investigate the tolerance of STAT and LTS cells of S. Enteritidis to ACP in PBS and on shell eggs. Additional objectives were to determine the extent of sub-lethal injury in pathogen survivors and assess changes in selected quality parameters of the eggs following ACP treatment.
2 Materials and methods
2.1 Bacterial culture and culture conditions
Salmonella enterica serovar Enteritidis (ATCC13076) was acquired from the culture collection of the Microbial Food Safety Laboratory of Iowa State University, Ames Iowa. The stock culture was stored frozen (−80°C) in brain heart infusion (BHI) broth (Difco; Becton Dickinson, Sparks, MD) containing 10% (v/v) glycerol. Frozen cultures in vials were thawed under running cold tap water, then activated by aseptically transferring 0.1 mL of thawed culture to 10 mL of tryptic soy broth with added 0.6% (w/v) yeast extract (TSBYE) followed by incubation (35°C) for 24 h. The activated culture was held at 4°C until used for preparing working cultures. Working cultures were prepared by performing two consecutive 20-h transfers of the activated culture in TSBYE (35°C) prior to the beginning of each experiment.
2.2 Preparation of STAT and LTS cells
A 2.0-mL aliquot of S. Enteritidis working culture was aseptically transferred to each of two screw capped Erlenmeyer flasks containing 200 mL of TSBYE per flask. The flasks of inoculated TSBYE were incubated (35°C) for 21 days to obtain LTS cells (Bhullar et al., 2021). Prior to starting the experiment, two additional flasks each containing 200 mL of TSBYE were inoculated as previously described and incubated (35°C) for 20 h to obtain STAT cells.
2.3 Preparation of inoculum and inoculation of PBS
Forty-mL portions of STAT or LTS cultures in TSBYE were aseptically transferred to respective sterile 50 mL centrifuge tubes. Those cells were harvested by centrifugation (10,000 × g for 10 min, 23°C) using a Sorvall Super T21 centrifuge (American Laboratory Trading, Inc., East Lyme, CT). The pelleted cells were washed by suspending them in PBS (pH 7.0) via vortexing. The washed cells were harvested by centrifugation as previously described. The pelleted STAT and LTS cells were suspended in 40 mL and 4 mL of PBS, respectively, to obtain approximately the same concentration of viable cells for each physiological state of the pathogen. Aliquots (150-µL) of STAT or LTS cell suspension (∼109 CFU/mL) were used to inoculate 15-mL portions of PBS (in petri dishes) to obtain a cell concentration of ∼107 CFU/mL. To prepare the inoculum for shell eggs, 40 mL portions of STAT or LTS working cultures were aseptically transferred to respective 50 mL centrifuge tubes and harvested via centrifugation as previously described. The pelleted cells were suspended in appropriate volumes of spent TSBYE, to obtain a viable cell concentration of ∼109 CFU/mL.
2.4 Preparation and inoculation of shell eggs
Fresh, organic, large brown eggs (66 ± 2 g) were purchased from a local farm in Ames, Iowa and used within 10 days of purchase. Eggs were inspected for shell intactness through illumination, and gently rinsed with distilled water to remove debris from the shells. They were wiped with a paper towel moistened with 70% ethanol followed by a 1-min dip in 70% ethanol (Wan et al., 2017) to reduce microbial contamination. The eggs were air-dried in a laminar flow hood with the blower on for a minimum of 20 min before inoculating them with S. Enteritidis. An aliquot (100 µL) of S. Enteritidis cell suspension was spot inoculated (13–18 spots) on the longitudinal surface of the egg within a 2 × 2 cm area delineated using a magic marker. The inoculated eggs were held at ambient temperature (22 ± 1°C) for 2.5 h in a laminar flow hood (with blower off) to allow attachment of the cells and drying of the inoculum.
2.5 Cold plasma treatment of PBS
The dielectric barrier discharge (DBD) high voltage atmospheric cold plasma (HVACP) set-up depicted in Figure 1 is an atmospheric low temperature plasma generating system used for in-package plasma treatment (Yepez and Keener, 2016). The system is comprised of a transformer (Phenix Technologies, MD, United States) with an input voltage of 120 V (AC) at 60 Hz, a voltage regulator with output voltage controlled within 0–120 kV, two donut shaped aluminum electrodes (each 145 mm diameter) with an inter-electrode discharge distance of 55 mm. The dielectric barriers consist of a plexiglass layer and two 1 mm thick Cuisinart® polypropylene sheets each with dimensions of 355 mm× 272 mm× 2.20 mm (Cuisinart, NJ, United States). A petri-dish (150 mm × 15 mm) without its lid and containing 15 mL of PBS inoculated with S. Enteritidis, was placed inside a polypropylene box (160 × 110 × 24 mm). The lid of the box was closed, and the box was then placed inside a 250 × 250 mm high oxygen barrier plastic bag made of Cryovac® B2360 film (Sealed Air North Carolina, United States). The bag was flushed with atmospheric air then heat-sealed. The packaged box with the sample was positioned between two aluminum electrodes with a plexiglass layer and a polypropylene sheet directly above the package and one polypropylene sheet below the package. An electric field voltage of 45 kV was applied, and the PBS samples were exposed to ACP for 0 (control), 1, 2, 3, and 4 min. After the ACP treatment the samples were held at ambient temperature (22°C ± 1°C) in a laminar flow hood for 24 h before determining the number of pathogen survivors. Each experiment was performed in triplicate.
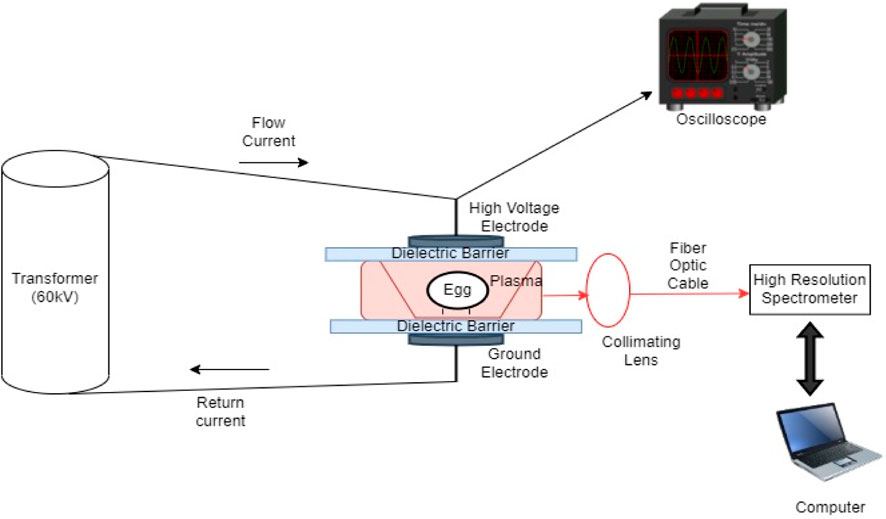
Figure 1. Schematic for experimental setup for high voltage atmospheric cold plasma (HVACP) treatment of shell eggs.
2.6 Cold plasma treatment of shell eggs
The same DBD HVACP system as previously described in Section 2.5, was used for treating the artificially inoculated shell eggs. Each inoculated egg was placed in a sterile Fisher brand standard polystyrene weighing dish (41 mm× 41 mm× 8 mm). Each dish with an egg was placed in a separate sterile Mainstays polypropylene box (161 mm× 85 mm× 57 mm) total volume 942.5 mL (Walmart Inc., Bentonville Arkansas, United States). The box (with lid shut) was placed inside a 310 × 202 mm high oxygen barrier Cryovac® B2360 bag (Sealed Air North Carolina, United States) and the package was flushed with air and heat-sealed. An electric field voltage of 60 kV was applied, and the eggs were exposed to ACP for 0 (control), 1, 2, 3, 4 and 5 min. After ACP treatment, the eggs were held at ambient temperature (22°C ± 1°C) in a laminar flow hood for 24 h before determining the number of pathogen survivors. Each experiment was performed at least three times.
2.7 Microbiological analysis
Aliquots (1.0 mL) of control (0 min; no ACP treatment) and ACP-treated PBS were serially diluted (10-fold) in 0.1% (w/v) peptone and surface-plated on thin agar layer (TAL) medium. The TAL medium consisted of solidified xylose lysine tergitol-4 (XLT-4) agar (selective for Salmonella) with an overlay of TSAYE (∼14 mL) as the non-selective layer to aid resuscitation of sub-lethally injured survivors (Kang and Fung, 2000). Each egg was transferred to a separate appropriately labeled sterile Seward stomacher bag (Seward, Worthington, UK). To each bag, 50 mL of 0.1% (w/v) peptone were added. The eggs were manually rubbed from the outside of the bag, with special attention being placed on the area where the inoculum was deposited. The opening of the bag was twist tied and the bag was manually shaken for 1 min. Ten-fold serial dilutions of the wash solution were prepared in 0.1% (w/v) peptone, and appropriate dilutions were surface-plated on TAL medium and on xylose lysine tergitol-4 (XLT-4) agar. Enumeration of bacterial colonies on agar plates was performed after 48 h of aerobic incubation at 35°C.
2.8 ACP inactivation kinetics for S. Enteritidis
The ACP inactivation kinetics were modelled using two of the nine microbial survival models included in the GInaFit (version 1.8 for Microsoft Office 365) Excel add-in tool (Geeraerd et al., 2005). The Log-linear with Tail model (Geeraerd et al., 2000) was used with the following equation:
where Nt is the microbial population at a given ACP treatment time (min); N0 is the initial microbial population (log CFU/egg); Nres is the residual microbial population characterizing the tailing (log CFU/egg); and kmax (min−1) is the inactivation rate of the log-linear part of the curve.
The Weibull model (Mafart et al., 2002) employed the following equation:
where δ is the time (min) for the first decimal reduction of the microbial population and p accounts for the shape of the survival curve; if p > 1, the curve exhibits a shoulder (convex or concave downward), if p < 1, the curve exhibits tailing (concave), and if p = 1, the survival curve is linear.
The times for obtaining a 4- and 5-log reduction were determined for each model. For the Log-linear with Tail model, D-values were initially calculated as 2.303/kmax, multiplied by x-log reduction (i.e. 4) with the addition of the tailing. For the Weibull model, an x-log reduction was measured using the model parameters (δ and p) in the following equation (Huang et al., 2013):
The models were evaluated using the four statistical measures given by GInaFit: mean sum of squared error (MSE), root mean sum of squared error (RMSE), R2, and R2 adjusted (R2 adj). MSE and RMSE measure statistical deviations between the fitted and observed experimental values, with smaller values indicating a better fit of the data. R2 and R2 adj both measure how well a model fits the data, with values closer to one indicating the better model for the data.
To assess the performance capabilities of both models, bias (Bf) and accuracy (Af) factors were determined with the subsequent equation (Ross, 1996):
where n is the number of experimental observations. Bf indicates whether the observed data lies below or above the model’s predicted values. Therefore, Bf > 1 demonstrates overprediction, Bf < 1 demonstrates underprediction, and Bf = 1 demonstrates an exact prediction. Af indicates how close the predictions are to the observations.
2.9 Determination of sub-lethal injury
The difference in pathogen survivors on TAL medium and the selective (XLT-4) was used to calculate sub-lethal injury. Percent sub-lethal injury was determined by the following equation:
2.10 Morphology of EXP, STAT and LTS cells
The EXP and STAT S. Enteritidis cells were prepared by streak plating a working culture of the pathogen on TSAYE followed by incubation (35°C) for 8 h (EXP) and 24 h (STAT). Cells from colonies of EXP and STAT cells were spread on a cleaned glass slide using an inoculation loop, heat fixed, and stained with crystal violet (for 1.0 min) in preparation for microscopic examination. The LTS cells were cultured in TSBYE (35°C) for 21 days (Bhullar et al., 2021), then harvested by via centrifugation as previously described. The supernatant was discarded, and a portion of the pelleted cells were spread on a cleaned glass slide and prepared for microscopic examination as previously described. All slides were examined at 1,000 × magnification using a Leica DM500 light microscope, and photographs of the bacterial cells were taken using a Leica ICC50W microscope camera (North Central Instruments, Brooklyn Park, MN).
2.11 Optical emission spectroscopy
Optical emission spectra were gathered to analyze the ROS and RNS produced within the package during ACP treatment of shell eggs at 60 kV for 5 min. The emission spectra during treatment of the eggs were captured using a computer-controlled Ocean Optics spectrometer (Ocean Optics, Inc., Florida, United States). Light emitted from the plasma was transmitted to the spectrometer via an Ocean Optics optical fiber with a core diameter of 1,000 µm. A collimating lens (5 mm in diameter) positioned 15 cm away from the edge of the sample container was used to optimize plasma emitted light entering the optical fiber. Integration time was set at 5 s, and six spectra were averaged, resulting in emission spectra being saved every 30 s.
2.12 Quality tests on shell eggs
The effects of ACP on shell strength, and yolk color were evaluated at 24 h after exposure of the eggs to ACP for 1, 3 and 5 min, and compared to those same quality characteristics of control eggs. Shell strength for both treated and control eggs was evaluated as a function of peak positive force required for a 10% compression of the egg using a TA-XTplus texture analyzer (Stable Micro Systems, Godalming, England U.K). A 2-kg load cell was used to calibrate the instrument. A TA-30 probe with a 75 mm diameter was used to compress the egg. Pre-test, test, and post-test speed parameters were set to 1.00 mm/s, 2.00 mm/s, and 2.00 mm/s, respectively, with a distance of 50 mm and a trigger force of 5 g. Egg yolk color was assessed using a Hunter Lab Colorimeter ColorFlex EZ (HunterLab Reston, Virginia) which evaluated L* (lightness) a* (greenness-redness), b* (blueness-yellowness), hue and chroma using the CIE standard illuminant D65 at 10° standard observer. Hue and chroma were calculated using the following equations (Cielab and Space, 2021):
Each analysis was performed in quadruplicate and statistical analysis of the data was performed.
2.13 Statistical analysis
Experiments involving PBS were performed in triplicate whereas shell eggs trials were performed in quadruplicate. The mean number of survivors and standard error of the mean were calculated using Microsoft Excel 2020 (Microsoft Inc., Redmond, WA). Statistical analysis was performed by JMP Pro statistical software version 16 (SAS Institute, Inc., Cary NC) with statistical significance recorded at p < 0.05. Treatment means were evaluated for statistically significant differences (p < 0.05) using a two-way Analysis of Variance (ANOVA) where time was treated as categorical. Tukey’s Honestly Significant Difference (HSD) was used to determine significant differences between treatments. Quality assessments were performed in quadruplicate with the mean and standard deviation reported. Treatment means were assessed for statistical significance using a one-way ANOVA, and Tukey HSD was used to evaluate significant differences among treatments.
3 Results
3.1 Inactivation of S. Enteritidis in PBS
Figure 2 shows the reduction in viable populations of STAT and LTS cells of S. Enteritidis ATCC 13076 in PBS (pH 7.0) after direct treatment with ACP (45 kV). In PBS, the initial viable counts (log10 CFU/mL) of STAT and LTS cells were 6.83 ± 0.06 and 7.69 ± 0.30, respectively, based on bacterial counts on TSAYE. For all ACP treatments tested there was a significantly higher reduction in populations of STAT cells compared to LTS cells (p < 0.05). Average reductions (log10 CFU/mL) of STAT cells were 1.0 ± 0.08, 0.95 ± 0.21, 1.45 ± 0.19, and 1.44 ± 0.16 after exposure to HVACP for 1.0, 2.0, 3.0, and 4.0 min, respectively. In contrast, log10 CFU/mL reductions in LTS cells were only 0.04 ± 0.01 (1 min), 0.06 ± 0.06 (2 min) 0.01 ± 0.04 (3 min), and 0.11 ± 0.07 (4 min) representing <1.0 log reduction for the entire duration of the treatment.
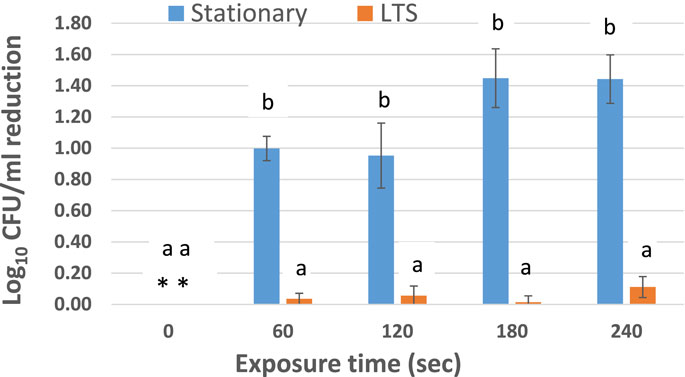
Figure 2. Log reduction of Salmonella Enteritidis ATCC 13076 after exposure to ACP (45 kV) in PBS. The experiment was performed in triplicate. Error bars represent standard error of the mean. For each treatment time, bars that do not share the same letters are significantly different (p < 0.05). Asterisks (**) signify no reduction in control (non-ACP-treated) STAT or LTS cells.
3.2 Inactivation of S. Enteritidis on shell eggs
Figure 3 shows STAT and LTS S. Enteritidis survivors on the outer shell surface of the eggs after direct ACP (60 kV) treatment. The data are based on bacterial colony counts on TAL medium (Figure 3A) and on XLT-4 agar (Figure 3B). The average initial viable counts (log10 CFU/egg) of S. Enteritidis on TAL were 6.85 ± 0.58 and 5.63 ± 0.68 for STAT and LTS cells, respectively, whereas, on XLT-4 viable counts were 6.72 ± 0.60 (STAT) and 5.55 ± 0.70 (LTS). Irrespective of the type of agar medium, there were significantly higher (p < 0.05) numbers of LTS survivors compared to STAT survivors after ACP treatment for 2–5 min (Figures 3A, B). Irrespective of agar medium and exposure (1–5 min) to ACP, numbers of LTS survivors on treated eggs were not significantly different from those on control eggs (p > 0.05). In contrast, all ACP treatments tested significantly decreased numbers of STAT survivors on eggs compared to control (p < 0.05). The STAT survivors (irrespective of agar medium) exhibited an initial steep decline up to 2 min followed by a “tailing” effect from 3 to 5 min that was not observed in LTS survivors, which exhibited a relatively flat profile for the survivor curve (Figures 3A, B).
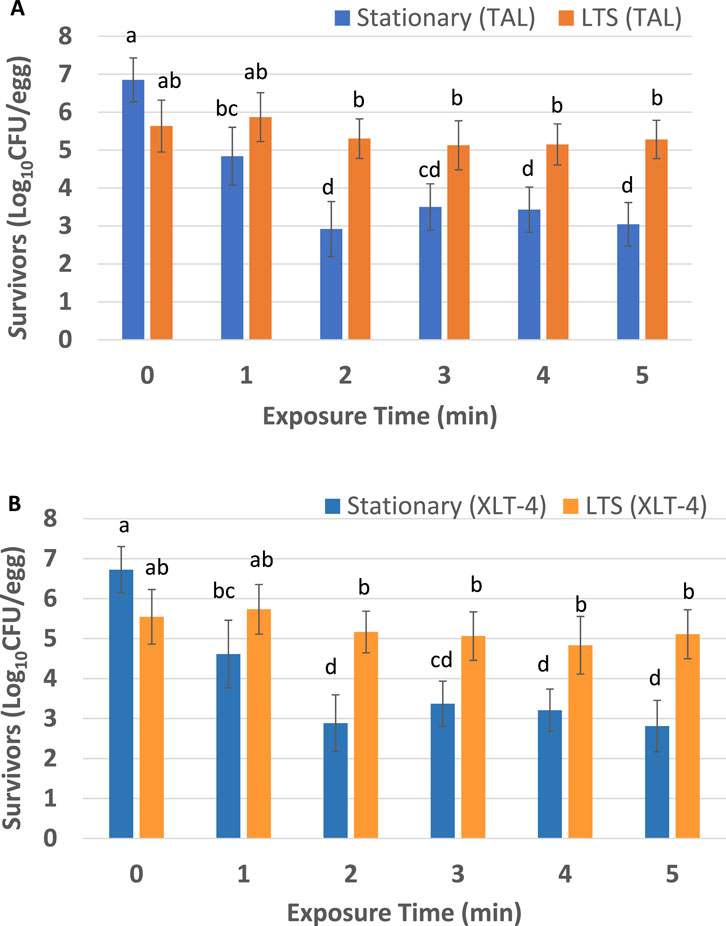
Figure 3. Salmonella Enteritidis ATCC 13076 survivors on shell eggs after exposure to ACP (60 kV). Recovery media used were thin agar layer (TAL) medium (A) and xylose lysine tergitol 4 (XLT-4) agar (B). The experiment was performed in quadruplicate. Error bars represent standard error of the mean. For each exposure time, bars that do not share the same letters are significantly different (p < 0.05).
3.3 ACP inactivation kinetics for S. Enteritidis
Figure 4 shows the logarithmic plots of equations for Log-Linear with Tail Model and Weibull Model fitted to the raw data for STAT S. Enteritidis survivors [log10CFU/egg versus time (min)] on TAL medium (A) and XLT-4 agar (B). The Log-Linear + Tail model exhibited a better fit of the data. The kinetic parameter values (kmax, log10(Nres), δ-, and ρ-values) of two GInaFit survival models for ACP (60 kV) treatment of STAT S. Enteritidis on shell eggs using two recovery media are shown in Table 1. The statistical measures for both the log-linear with tail and the Weibull models are presented in Table 2. Based on the Log-Linear with Tail model, 5.29 and 5.78 min were derived as treatment times to achieve a 4- and 5- log reduction, respectively, in viable STAT cells (Table 2). Kinetic parameter values for ACP treatment of LTS cells could not be determined because the inactivation was less than one (<1) log10 CFU/egg for the total treatment time of 5 min.
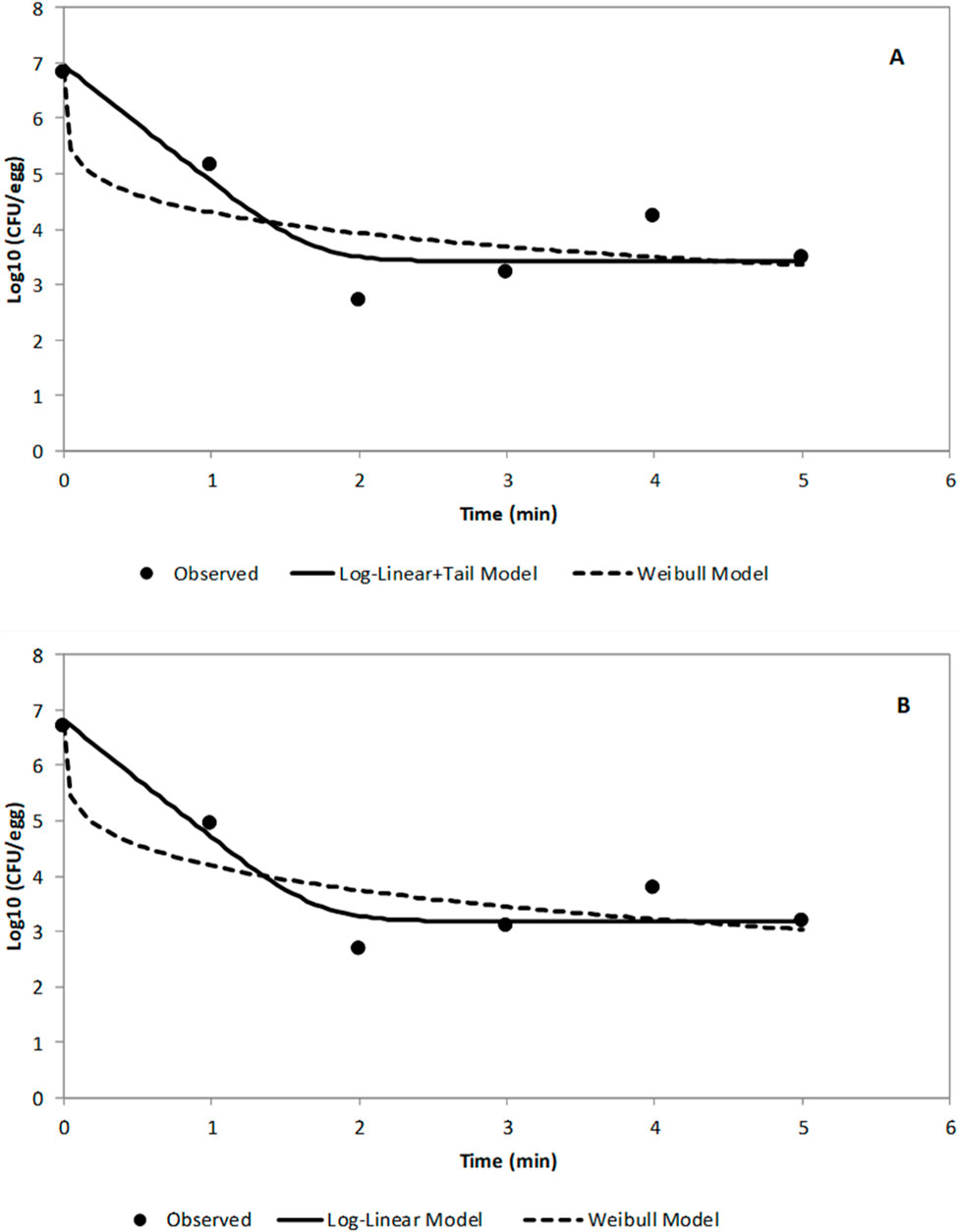
Figure 4. Logarithmic plots of equation for Log-Linear Model and Weibull Model fitted to the raw data for STAT S. Enteritidis survivors on TAL medium (A) and XLT-4 agar (B) as log10CFU/egg versus time (min).
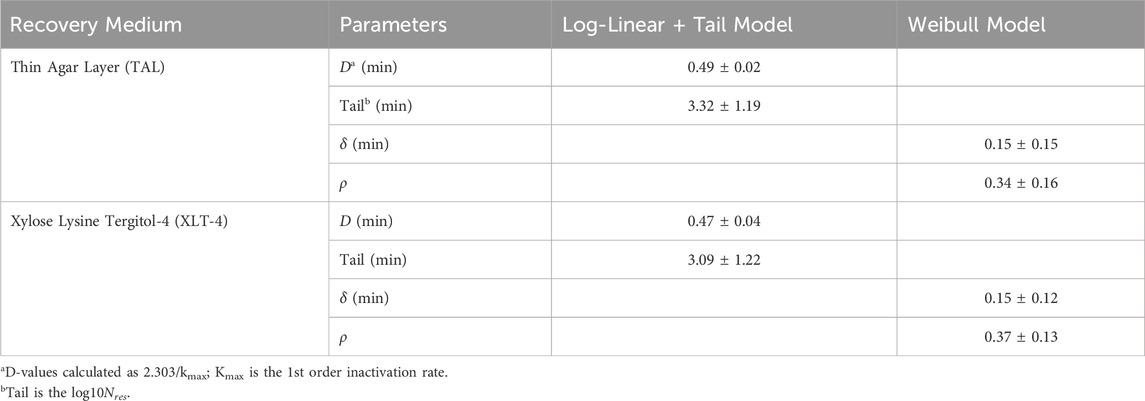
Table 1. Kinetic parameter values of two models for HVACP treatment of stationary phase (STAT) cells of S. Enteritidis ATCC 13076 on shell eggs.
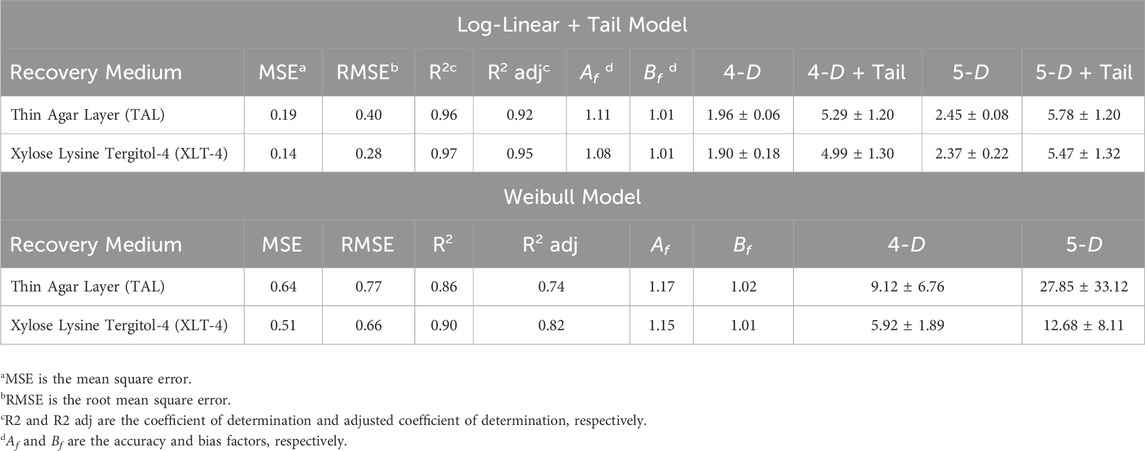
Table 2. Statistical measures (goodness-of-fit), 4- and 5-log reduction time (min) for Log-Linear + Tail and Weibull models for HVACP treatment of stationary phase cells of S. Enteritidis ATCC 13076 on shell eggs.
3.4 Sub-lethal injury in S. Enteriditis survivors on shell eggs
Average values for sub-lethal injury in surviving populations of STAT and LTS cells of S. Enteritidis on shell eggs after 1–5 min of exposure to ACP (60 kV) are shown in Table 3. No sub-lethal injury was observed in S. Enteritidis survivors on control eggs (data not shown). Surviving populations for both life cycle phases (STAT and LTS) of the pathogen endured sub-lethal injury due to ACP treatment. The percentage of sub-lethal injury ranged from 7.22% to 37.11% and 9.40%–30.55% in STAT and LTS survivors, respectively. Irrespective of exposure time to ACP, there were no significant differences in sub-lethal injury in STAT compared to LTS survivors (p > 0.05).

Table 3. Average values of percent sub-lethal injury ±standard error of the mean for survivors of stationary phase (STAT) and long-term survival phase (LTS) Salmonella Enteritidis after 1–5 min of exposure to ACP (60 kV) treatment of artificially inoculated shell eggs.
3.5 Light microscopy images of S. Enteritidis
Figure 5 shows photographs of light microscopy images of EXP (A), STAT (B) and LTS (C) cells of S. Enteritidis ATCC 13076. Both EXP and STAT cell populations (A and B, respectively) consisted of predominantly rod-shaped cells, whereas LTS cells were predominantly coccoid (C). The EXP cells appeared as longer rods with some cells in the process of dividing. The STAT cells were a mixture of shorter rods and coccoid cells. The coccoid LTS cells seemed refractory to the crystal violet stain compared to the EXP and STAT cells, which exhibited a strong blue color.
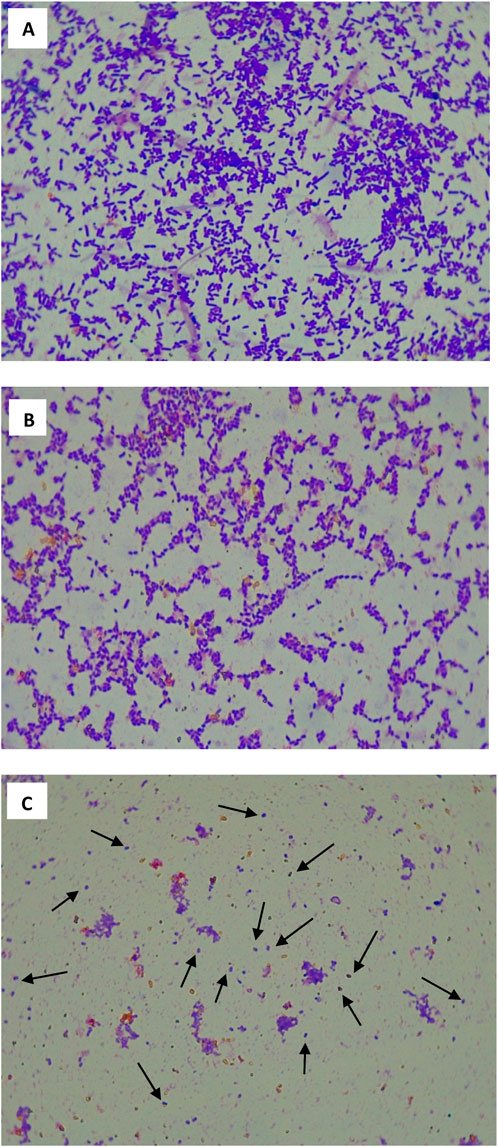
Figure 5. Light microscopy images (1,000 × magnification) of EXP (A), STAT (B) and LTS (C) cells of Salmonella Enteritidis. Arrows point to coccoid LTS cells.
3.6 Optical emission spectroscopy
Figure 6 illustrates the emission spectra resulting from ACP (60 kV) treatment applied to shell eggs in atmospheric air with a relative humidity of 60%. The predominant peaks observed within the air plasma were concentrated in the UV region (300–400 nm), primarily corresponding to excited nitrogen species such as the nitrogen second positive system (SPS) N2(C-B) and the first negative system N2+(B-X) (Misra et al., 2015; Shi et al., 2017). Additionally, the hydroxyl (OH) peak was detected around 300 nm.
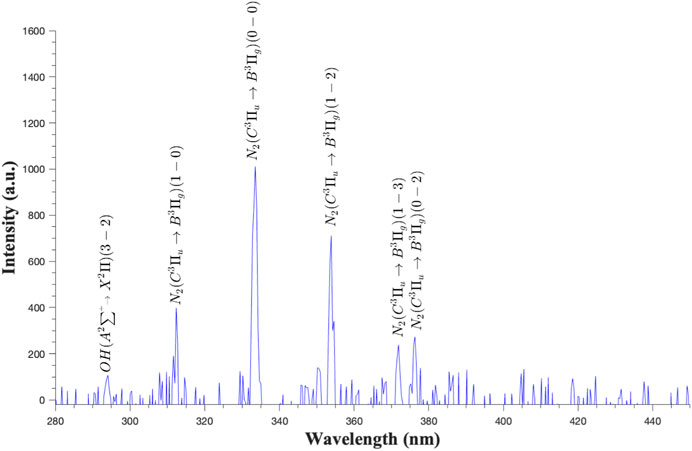
Figure 6. Optical emission spectra of dielectric barrier discharge ACP (60 kV) treatment of shell eggs in air at atmospheric pressure.
3.7 Egg quality analysis
Table 4 shows the effects of ACP on L*, a*, b*, hue (hab), chroma (C*ab) and peak positive force required for a 10% compression of the eggshell for control and treated eggs after 1-, 3-, and 5-min exposure to ACP. No significant difference (p> 0.05) was observed among any of those quality parameters. Eggs treated with ACP seemed to require less force: 1 min (4,625 g), 3 min (4,357 g), 5 min (4,499 g); however, these values remained within the range of those observed for the control eggs (4,300–5,900 g). Similarly, yolk color, determined by L*, a*, b*, hue and chroma of all treated eggs, irrespective of exposure time to ACP, all fell within the observed range of the control eggs.
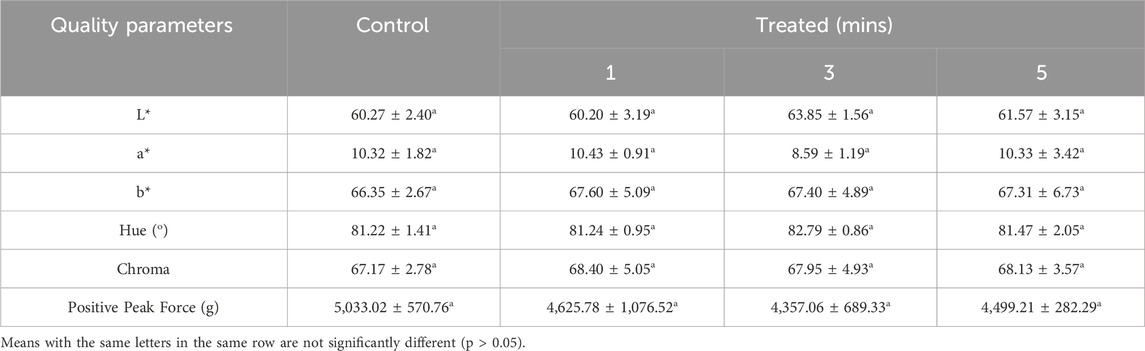
Table 4. Select quality parameters of treated eggs at 1, 3, 5 min with ACP (60 kV), and untreated (control) eggs.
4 Discussion
4.1 Tolerance of S. Enteritidis to HVACP in PBS and on shell eggs
After ACP treatment, PBS and shell eggs were held at ambient temperature (22°C ± 1°C) in their respective packages in a laminar flow hood for 24 h before they were analyzed for survivors. This step was taken to exploit the antimicrobial effect of relatively long-lived reactive oxygen species such as ozone (Yepez et al., 2020). Significantly greater inactivation of STAT cells of S. Enteritidis compared to the LTS cells was observed in vitro and on shell eggs following direct application of ACP at 45 kV and 60 kV, respectively. The ACP treatment at a lower voltage (45 kV) was used for PBS because of the much higher sensitivity of planktonic cells to antimicrobial treatments compared to attached cells (Bhullar et al., 2021; Smet et al., 2019) that precluded adequate recovery of cells for plotting a survivor curve. Our findings on higher tolerance of LTS cells to ACP compared to STAT cells are consistent with those of earlier reports on LTS pathogens (Bhullar et al., 2021; Djebbi-Simmons et al., 2019; Wang, 2017; Wang et al., 2018; Wen et al., 2009). For example, a higher tolerance to heat and high hydrostatic pressure was reported for LTS L. monocytogenes compared to that of the same pathogen in other life cycle phases such as late EXP, STAT, early-death and late-death phases (Wen et al., 2009). For S. Typhimurium, LTS cells exhibited significantly (P < 0.05) higher tolerance than EXP and STAT cells to UV radiation in both 0.85% (w/v) saline and in apple juice (Wang et al., 2018). Significantly higher tolerance to sodium hypochlorite (0.0095%) (p < 0.05) was observed for LTS cells of S. Typhimurium compared to STAT cells on plastic (cutting board, formica laminate) and stainless steel surfaces (Djebbi-Simmons et al., 2019). More recently, higher (p < 0.05) surviving populations of LTS Shiga-toxin producing E. coli compared to those of STAT cells were observed after in vitro exposure to sodium hypochlorite solution and the washing of artificially inoculated lettuce leaves with hypochlorite solutions (Bhullar et al., 2021. These results indicate that LTS cells are more resistant than STAT cells to antimicrobial treatments.
Conditions in highly nutritious laboratory broth with optimum pH, water activity and growth temperature, and no microbial competitors, are atypical of the natural environment of bacteria. On the contrary, nutrient limitation, accumulation of waste, competing microflora, and harsh environmental conditions are more likely the norm than the exception for bacteria in nature (Roszak and Colwell, 1987; Finkel, 2006). Such conditions can cause bacteria to transition to the LTS (Finkel, 2006) thus entering enter a state of dormancy with low metabolic rate (Kolter et al., 1993). For example, nutrient limitation induces cell dormancy in marine bacteria (Novitsky and Morita, 1978). Moreover, Pseudomonas aeruginosa exhibits a dormancy phenotype during its long-term survival in water (Lewenza et al., 2018). Under those previously stated conditions, some Gram-positive bacteria produce endospores; however, most Gram-negative bacteria and non-endospore-forming Gram-positive bacteria form dormant cells. Dormant bacterial cells typically exhibit no growth with little or no metabolic activity, and most antimicrobial agents are ineffective against bacterial cells that are not actively growing and dividing (Reygaert, 2018).
4.2 ACP inactivation kinetics for S. Enteritidis on shell eggs
The Log-linear with Tail and Weibull models were fitted to the survival data derived from bacterial counts on two recovery media: TAL and XLT-4. The survival of S. Enteritidis exhibited deviation from the linear decline in the log number of survivors with time. Tailing of the survivor curves or a sub-population of more resistant bacteria declining at a slower rate was observed (Figure 1; Table 1). The shape parameter of the Weibull model (p < 1) indicates that the S. Enteritidis survival curves fitted with this model were concave. Nevertheless, the Log-linear with Tail model produced a better fit to ACP treatment of STAT cells on shell eggs (based on colony counts on TAL and XLT-4 media) as indicated by higher R2 and R2 adj values and smaller MSE and RMSE values (Table 2). According to Ross et al. (2000), the accuracy factor (Af) values of 0.90–1.15 (an increase or decrease by 0.1–0.15) are considered acceptable. The Af values ranged from 1.08 to 1.11 and 1.15 to 1.17 for the Log-linear with Tail and Weibull models, respectively, further indicating that the Log-linear with Tail model produced better predictions for ACP treatment of STAT cells on the eggs. As compared to the Weibull model, the time needed for a 4- and 5-log inactivation of STAT cells on shell eggs by ACP treatment was lower using Log-linear with Tail model (Table 2).
4.3 ACP-induced sub-lethal injury
Our results indicate that a portion of the S. Enteritidis survivors of ACP treatment of shell eggs was sub-lethally injured irrespective of the life cycle phase of the pathogen (Table 1). Wang et al. (2018) observed that there was less sub-lethal injury in LTS Salmonella Typhimurium compared to STAT cells of that pathogen following treatment to ultraviolet radiation (1,500 µW/cm2) in apple juice (p < 0.05). The LTS survivors of Shiga toxin-producing Escherichia coli after chlorine (40 ppm) treatment of lettuce leaves had significantly less sub-lethal injury compared to STAT survivors (Bhullar et al., 2021). Our results differ from results of those previously mentioned studies in that the extent of ACP-induced sub-lethal injury in STAT and LTS S. Enteritidis survivors was not significantly different (p > 0.05). This difference might be due to the type of sub-lethal injury caused by ACP, which has a very complex chemical composition.
The chemical composition of ACP includes many different reactive species acting independently, additively or in synergy, to inactivate microorganisms (Bourke et al., 2017). Therefore, larger variations in the type and extent of cellular damage are likely to occur following every ACP treatment. For both STAT and LTS cells, we observed consistently large variations in the extent of sub-lethal injury caused by each ACP treatment across replications of the experiment. This in turn reduces statistical significance of the mean values for percent sub-lethal injury across life cycle phases (STAT and LTS). Therefore, from the results of the present study, it is difficult to ascertain whether the extent of ACP-induced sub-lethal injury in S. Enteritidis survivors is significantly affected by the life cycle phase of the pathogen. However, the observed sub-lethal injury in both STAT and LTS survivors has food safety implications. In this context, determination of sub-lethally injured foodborne pathogens is of critical importance for two main reasons. First, failure to account for sub-lethally injured pathogens can overestimate of a treatment’s antimicrobial efficacy, and second, food processes that inflict sub-lethal injury in microorganisms offer opportunities for their application in combination with other food preservation treatments to prevent resuscitation and subsequent proliferation of pathogenic microorganisms (Thomas-Popo et al., 2019). Therefore, we infer that ACP has good potential to be applied as part of a “hurdle technology” system to enhance the microbial safety of shell eggs.
4.4 Morphology of EXP, STAT and LTS cells
As the rod-shaped S. Enteritidis cells transitioned from EXP to STAT to LTS, their morphology changed from elongated or rod-shaped to coccoid or spherical shaped cells (Figures 5A–C). This observation is consistent with earlier reports by Nystrom (2004) and Jaishankar and Srivastava (2017), both of whom reported the shrinking of bacterial cells as they enter the STAT. This shrinkage involved nucleoid condensation, increased periplasmic space, and decreased membrane fluidity that are a result of starvation induction genes, which facilitate increased cell survival under stressful conditions. Similarly, Mañas and Mackey (2004), reported rod-shaped and coccoid shaped E. coli in the EXP and STAT cells, respectively. It is important to note however, that the STAT cells are not exclusively coccoid. As some cells are still replicating, the STAT culture is a heterogenous mixture of rods and cocci. By the time the cells transition into the LTS, the population becomes almost entirely coccoid (Figure 5C). These cells have shrunk even more than STAT cells and appear smaller. Our findings are also consistent with observations of Wen et al. (2009) who reported significantly smaller sized coccoid shaped LTS L. monocytogenes cells, compared to the partially coccoid STAT and rod-shaped EXP cells. Those same authors observed very light staining of the coccoid LTS cells that indicated a change in the cell envelop or a more condensed crystalline (glassy) cytoplasm. Just like the condition of the core of bacterial endospores, cytoplasmic condensation may lower the water activity in the coccoid cells, thus increasing cellular tolerance to many stresses (Wen et al., 2009). During the transition of L. monocytogenes to the LTS in TSBYE, the most significantly upregulated genes were for transport, energy metabolism, and cell envelope structure (Wen et al., 2011). Therefore, our observed increase in tolerance of LTS S. Enteritidis to ACP might be due to alteration in the cell envelope or lowered intracellular water activity, which may reduce intracellular formation of reactive species.
4.5 Optical emission spectroscopy
Optical emission spectroscopy (OES) was conducted to examine the reactive gas species produced within the package during ACP treatment of shell eggs. The emission spectra (Figure 6) show similarity and comparability to emission spectra reported in earlier studies that used atmospheric air to generate plasma (Pankaj et al., 2017; Wan et al., 2017). Our results indicate a low intensity of atomic oxygen peaks, which can be attributed to the self-quenching of O (3P) and O (5P) within the air plasma (Fridman, 2008; Walsh et al., 2010). During ACP treatment, the ionization of gas molecules generates ROS and RNS, which are bactericidal and cause cellular injuries such as DNA damage and membrane lipid peroxidation (Han et al., 2016; Joshi et al., 2011). Our results indicate that the ACP used under the conditions of the present study was a source of both ROS and RNS, which can be lethal to bacteria (Bourke et al., 2017).
4.6 Effect on egg quality
Eggshell strength is a major economic issue for the egg industry. It is of major concern during activities such as egg collection, sorting, and more importantly, during transportation, when a high incidence of damaged shell eggs can occur. Damaged eggshells are estimated to account for 8%–11% of total loss of eggs (Dunn et al., 2009). Therefore, it is important to evaluate the impact of novel antimicrobial interventions on eggshell strength. In the present study, egg quality of ACP-treated and non-treated (control) eggs was assessed through eggshell strength and yolk color. Our results reveal that ACP treatments, irrespective of exposure time, did not significantly affect eggshell strength, although it was observed that eggshell strength decreased slightly after exposure to ACP for 1, 3, and 5 min (P > 0.05). As the shell strength of ACP-treated and control eggs remained within the same range, the results for the treated eggs are likely due to variation among the individual eggs rather than an effect of ACP. Nevertheless, our results are consistent with previous research findings that demonstrated negligible changes of egg shell integrity after ACP treatment of shell eggs (Ragni et al., 2010). These results suggest that ACP, under the conditions tested in the present study, does not compromise shell strength.
Color is a major sensory property that influences consumer perception and choice of certain foods. For example, consumers perceive eggs with intensely colored yolks as a healthy, nutrient-rich food (Williams, 1992; Saleh et al., 2021). Moreover, egg yolk color is one of the main characteristics by which the quality of eggs is judged (Beardsworth and Hernandez, 2004). Therefore, egg yolk color is a major index of consumer perception of the internal quality of shell eggs. We evaluated egg yolk color based on lightness (L*), and color as the green (−), red (+) (a*), and the blue (−), yellow (+) (b*) axes, hue, and color intensity. There were no visual differences observed among yolk samples tested and even after 5 min, the maximum ACP treatment time. These findings are consistent with Dasan et al. (2018), Wan et al. (2017), Georgescu et al. (2017), and Gavahian et al. (2019) who all reported minor changes to yolk color following ACP treatments.
Carotenoids in the egg yolk produce the yellow-orange hue (Anton, 2007). Considering that ACP can exert oxidative effects, the oxidation of carotenoids in egg yolk warranted investigation. Oxidation may can cause color fading, which negatively impacts consumer perception; however, since ACP is a surface treatment, it is possible that potential oxidative effects do not go beyond the shell membrane, thus maintaining the integrity of the yolk color of intact shell eggs.
5 Conclusion
The LTS S. Enteritidis cells on shell eggs exhibit significantly higher tolerance to ACP treatment compared to STAT cells. The LTS cells shrink to predominantly coccoid cells, which are slightly refractile. ACP causes large variations in the extent of sub-lethal injury in S. Enteritidis irrespective of the life cycle phase of the pathogen. Under the test conditions of the present study, ACP has negligible impact on eggshell strength and yolk color. The main results of the present study indicate that LTS cross-protects S. Enteritidis against ACP and should be considered in the design and validation of ACP treatment parameters for ensuring the microbial safety of shell eggs.
Data availability statement
The raw data supporting the conclusions of this article will be made available by the authors, without undue reservation.
Author contributions
KB: Formal Analysis, Investigation, Writing–original draft, Writing–review and editing. AM: Conceptualization, Methodology, Supervision, Writing–review and editing, Project administration, Resources. GP: Conceptualization, Methodology, Supervision, Writing–review and editing, Resources. TB: Resources, Supervision, Writing–review and editing, Methodology. PF-D-S: Resources, Supervision, Writing–review and editing, Methodology. BB-S: Resources, Writing–review and editing, Methodology. VJ: Formal Analysis, Methodology, Writing–review and editing, Software. ZW: Formal Analysis, Methodology, Writing–review and editing, Resources.
Funding
The author(s) declare that financial support was received for the research, authorship, and/or publication of this article. This work was supported by the Iowa Agriculture and Home Economics Experiment Station, Project number IOW04202, sponsored by the Hatch Act and State of Iowa Funds.
Acknowledgments
The authors thank Dr. Amy Andreotti in Biochemistry Biophysics and Molecular Biology, Iowa State University, Ames, IA, for her insights and contribution to this research.
Conflict of interest
The authors declare that the research was conducted in the absence of any commercial or financial relationships that could be construed as a potential conflict of interest.
The author(s) declared that they were an editorial board member of Frontiers, at the time of submission. This had no impact on the peer review process and the final decision.
Publisher’s note
All claims expressed in this article are solely those of the authors and do not necessarily represent those of their affiliated organizations, or those of the publisher, the editors and the reviewers. Any product that may be evaluated in this article, or claim that may be made by its manufacturer, is not guaranteed or endorsed by the publisher.
Abbreviations
ACP, atmospheric cold plasma; BHI, brain heart infusion broth; DBD, dielectric barrier discharge; EXP, exponential phase; HVACP, high voltage atmospheric cold plasma; LTS, long-term survival phase; MSE, mean sum of squared error; RMSE, root mean sum of squared error; STAT, stationary phase; TAL, thin agar layer; TSAYE, tryptic soy agar with yeast extract; TSBYE, tryptic soy broth with yeast extract; XLT-4, xylose lysine tergitol-4.
References
Abdoli, B., Khoshtaghaza, M. H., Ghomi, H., Torshizi, M. A. K., Mehdizadeh, S. A., Pishkar, G., et al. (2024). Cold atmospheric pressure air plasma jet disinfection of table eggs: inactivation of Salmonella enterica, cuticle integrity and egg quality. Int. J. Food Microbiol. 410, 110474. doi:10.1016/j.ijfoodmicro.2023.110474
Anton, M. (2007). Composition and structure of hen egg yolk. Bioact. Egg Compd., 1–6. doi:10.1007/978-3-540-37885-3_1
Beardsworth, P. M., and Hernandez, J. M. (2004). Yolk colour–an important egg quality attribute. Int. Poult. Prod. 12 (5), 17–18.
Bhullar, M. S., Shaw, A., Mendonca, A., Monge, A., Nabwire, L., and Thomas-Popo, E. (2021). Shiga toxin–producing Escherichia coli in the long-term survival phase exhibit higher chlorine tolerance and less sublethal injury following chlorine treatment of romaine lettuce. Foodborne Pathogens Dis. 18, 276–282. doi:10.1089/fpd.2020.2873
Bourke, P., Ziuzina, D., Han, L., Cullen, P. J., and Gilmore, B. F. (2017). Microbiological interactions with cold plasma. J. Appl. Microbiol. 123 (2), 308–324. doi:10.1111/jam.13429
Buchmüller, K., Bearth, A., and Siegrist, M. (2020). Consumers’ perceptions of chemical household products and the associated risks. Food Chem. Toxicol. 143, 111511. doi:10.1016/j.fct.2020.111511
CDC (2018). Outbreak of Salmonella infections linked to gravel Ridge Farms shell eggs - final update. Atlanta, GA, United States: Centers for Disease Control and Prevention. Available at: https://www.cdc.gov/salmonella/enteritidis-09-18/index.html.
Cielab, T., and Space, C. (2021). The CIELAB L * a * b * system – the method to quantify colors of coatings, 1–4.
Dasan, B. G., Yildirim, T., and Boyaci, I. H. (2018). Surface decontamination of eggshells by using non-thermal atmospheric plasma. Int. J. Food Microbiol. 266, 267–273. doi:10.1016/j.ijfoodmicro.2017.12.021
Deng, S., Cheng, C., Ni, G., Meng, Y., and Chen, H. (2010). Bacillus subtilis devitalization mechanism of atmosphere pressure plasma jet. Curr. Appl. Phys. 10 (4), 1164–1168. doi:10.1016/j.cap.2010.02.004
Djebbi-Simmons, D., Xu, W., Janes, M., and King, J. (2019). Survival and inactivation of Salmonella enterica serovar Typhimurium on food contact surfaces during log, stationary and long-term stationary phases. Food Microbiol. 84, 103272. doi:10.1016/j.fm.2019.103272
Dunn, I. C., Joseph, N. T., Bain, M., Edmond, A., Wilson, P. W., Milona, P., et al. (2009). Polymorphisms in eggshell organic matrix genes are associated with eggshell quality measurements in pedigree Rhode Island Red hens. Anim. Genet. 40 (1), 110–114. doi:10.1111/j.1365-2052.2008.01794.x
Finkel, S. E. (2006). Long-term survival during stationary phase: evolution and the GASP phenotype. Nat. Rev. Microbiol. 4 (2), 113–120. doi:10.1038/nrmicro1340
Gavahian, M., Peng, H.-J., and Chu, Y.-H. (2019). Efficacy of cold plasma in producing Salmonella-free duck eggs: effects on physical characteristics, lipid oxidation, and fatty acid profile. J. Food Sci. Technol. 56 (12), 5271–5281. doi:10.1007/S13197-019-03996-Z
Geeraerd, A. H., Herremans, C. H., and Van Impe, J. F. (2000). Structural model requirements to describe microbial inactivation during a mild heat treatment. Int. J. food Microbiol. 59 (3), 185–209. doi:10.1016/s0168-1605(00)00362-7
Geeraerd, A. H., Valdramidis, V. P., and Van Impe, J. F. (2005). GInaFiT, a freeware tool to assess non–log-linear microbial survivor curves. Int. J. Food Microbiol. 102, 95–105. doi:10.1016/j.ijfoodmicro.2004.11.038
Georgescu, N., Apostol, L., and Gherendi, F. (2017). Inactivation of Salmonella enterica serovar Typhimurium on egg surface, by direct and indirect treatments with cold atmospheric plasma. Food control. 76, 52–61. doi:10.1016/j.foodcont.2017.01.005
Goslan, E. H., Krasner, S. W., Bower, M., Rocks, S. A., Holmes, P., Levy, L. S., et al. (2009). A comparison of disinfection by-products found in chlorinated and chloraminated drinking waters in Scotland. Water Res. 43 (18), 4698–4706. doi:10.1016/j.watres.2009.07.029
Han, L., Patil, S., Boehm, D., Milosavljević, V., Cullen, P. J., and Bourke, P. (2016). Mechanisms of inactivation by high-voltage atmospheric cold plasma differ for Escherichia coli and Staphylococcus aureus. Appl. Environ. Microbiol. 82 (2), 450–458. doi:10.1128/AEM.02660-15
Huang, K., Yu, L., Liu, D., Gai, L., and Wang, J. (2013). Modeling of yeast inactivation of PEF-treated Chinese rice wine: effects of electric field intensity, treatment time and initial temperature. Food Res. Int. 54 (1), 456–467. doi:10.1016/j.foodres.2013.07.046
Illera, A. E., Souza, V. R., Nikmaram, N., Tang, L., and Keener, K. M. (2022). High voltage atmospheric cold plasma decontamination of Salmonella enteritidis on chicken eggs. Innovative Food Sci. and Emerg. Technol. 82, 103210. doi:10.1016/j.ifset.2022.103210
Illera, A. E., Souza, V. R., Tang, L., Nikmaram, N., and Keener, K. M. (2023). Effect of high voltage atmospheric cold plasma on chicken eggs quality during refrigerated storage. Food Biosci. 53, 102754. doi:10.1016/j.fbio.2023.102754
Jaishankar, J., and Srivastava, P. (2017). Molecular basis of stationary phase survival and applications. Front. Microbiol. 8, 2000. doi:10.3389/fmicb.2017.02000
Joshi, S. G., Cooper, M., Yost, A., Paff, M., Ercan, U. K., Fridman, G., et al. (2011). Nonthermal dielectric-barrier discharge plasma-induced inactivation involves oxidative DNA damage and membrane lipid peroxidation in Escherichia coli. Antimicrob. agents Chemother. 55 (3), 1053–1062. doi:10.1128/AAC.01002-10
Kang, D. H., and Fung, D. Y. (2000). Application of thin agar layer method for recovery of injured Salmonella typhimurium. Int. J. Food Microbiol. 54 (1-2), 127–132. doi:10.1016/s0168-1605(99)00174-9
Keerthirathne, T. P., Ross, K., Fallowfield, H., and Whiley, H. (2017). Reducing risk of salmonellosis through egg decontamination processes. Int. J. Environ. Res. public health 14 (3), 335. doi:10.3390/ijerph14030335
Kolter, R., Ie, D. A. S., and Torma, A. (1993). The stationary phase of the bacterial life cycle. Annu. Rev. Microbiol. 47, 855–874. doi:10.1146/annurev.mi.47.100193.004231
Lewenza, S., Abboud, J., Poon, K., Kobryn, M., Humplik, I., Bell, J. R., et al. (2018). Pseudomonas aeruginosa displays a dormancy phenotype during long-term survival in water. PLoS ONE 13 (9), 01983844–e198419. doi:10.1371/journal.pone.0198384
Lin, L., Liao, X., Li, C., Abdel-Samie, M. A., and Cui, H. (2020). Inhibitory effect of cold nitrogen plasma on Salmonella Typhimurium biofilm and its application on poultry egg preservation. LWT 126, 109340. doi:10.1016/j.lwt.2020.109340
Mafart, P., Couvert, O., Gaillard, S., and Leguérinel, I. (2002). On calculating sterility in thermal preservation methods: application of the Weibull frequency distribution model. Int. J. Food Microbiol. 72 (1–2), 107–113. doi:10.1016/S0168-1605(01)00624-9
Mañas, P., and Mackey, B. M. (2004). Morphological and physiological changes induced by high hydrostatic pressure in exponential- and stationary-phase cells of Escherichia coli: relationship with cell death. Appl. Environ. Microbiol. 70 (3), 1545–1554. doi:10.1128/AEM.70.3.1545-1554.2004
Meireles, A., Giaouris, E., and Simões, M. (2016). Alternative disinfection methods to chlorine for use in the fresh-cut industry. Food Res. Int. 82, 71–85. doi:10.1016/j.foodres.2016.01.021
Misra, N., Schülter, O. K., and Cullen, P. (2016). “Cold plasma in food and agriculture,” in Cold plasma in food and agriculture. doi:10.1016/c2014-0-00009-3
Misra, N. N., Keener, K. M., Bourke, P., and Cullen, P. J. (2015). Generation of in-package cold plasma and efficacy assessment using methylene blue. Plasma Chem. Plasma Process. 35, 1043–1056. doi:10.1007/s11090-015-9638-5
Moritz, M., Wiacek, C., Weihe, T., Ehlbeck, J., Weltmann, K. D., and Braun, P. G. (2021). Effect of cold atmospheric pressure plasma treatment of eggshells on the total bacterial count inoculated Salmonella Enteritidis and selected quality parameters. Plasma Process. Polym. 18 (1), 2000061. doi:10.1002/ppap.202000061
Niemira, B. A. (2012). Cold plasma decontamination of foods. Annu. Rev. Food Sci. Technol. 3 (1), 125–142. doi:10.1146/annurev-food-022811-101132
Nou, X., and Luo, Y. (2010). Whole-leaf wash improves chlorine efficacy for microbial reduction and prevents pathogen cross-contamination during fresh-cut lettuce processing. J. Food Sci. 75 (5), M283–M290. doi:10.1111/j.1750-3841.2010.01630.x
Novitsky, J. A., and Morita, R. Y. (1978). Possible strategy for the survival of marine bacteria under starvation conditions. Mar. Biol. 48 (3), 289–295. doi:10.1007/BF00397156
Nyström, T. (2004). Stationary-phase physiology. Annu. Rev. Microbiol. 58, 161–181. doi:10.1146/annurev.micro.58.030603.123818
Ölmez, H., and Kretzschmar, U. (2009). Potential alternative disinfection methods for organic fresh-cut industry for minimizing water consumption and environmental impact. LWT - Food Sci. Technol. 42 (3), 686–693. doi:10.1016/J.LWT.2008.08.001
Pankaj, S. K., Wan, Z., Colonna, W., and Keener, K. M. (2017). Degradation kinetics of organic dyes in water by high voltage atmospheric air and modified air cold plasma. Water Sci. Technol. 76 (3), 567–574. doi:10.2166/wst.2017.169
Pijnacker, R., Dallman, T. J., Tijsma, A. S. L., Hawkins, G., Larkin, L., Kotila, S. M., et al. (2019). An international outbreak of Salmonella enterica serotype Enteritidis linked to eggs from Poland: a microbiological and epidemiological study. Lancet Infect. Dis. 19 (7), 778–786. doi:10.1016/S1473-3099(19)30047-7
Ragni, L., Berardinelli, A., Vannini, L., Montanari, C., Sirri, F., Guerzoni, M. E., et al. (2010). Non-thermal atmospheric gas plasma device for surface decontamination of shell eggs. J. Food Eng. 100 (1), 125–132. doi:10.1016/j.jfoodeng.2010.03.036
Reygaert, W. C. (2018). An overview of the antimicrobial resistance mechanisms of bacteria. AIMS Microbiol. 4 (3), 482–501. doi:10.3934/microbiol.2018.3.482
Ross, T. (1996). Indices for performance evaluation of predictive models in food microbiology. J. Appl. Bacteriol. 81 (5), 501–508. doi:10.1111/j.1365-2672.1996.tb03539.x
Ross, T., Dalgaard, P., and Tienungoon, S. (2000). Predictive modelling of the growth and survival of Listeria in fishery products. Int. J. Food Microbiol. 62 (3), 231–245. doi:10.1016/S0168-1605(00)00340-8
Roszak, D. B., and Colwell, R. (1987). Survival strategies of bacteria in the natural environment. Microbiol. Rev. 51 (3), 365–379. doi:10.1128/mr.51.3.365-379.1987
Saleh, A. A., Gawish, E., Mahmoud, S. F., Amber, K., Awad, W., Alzawqari, M. H., et al. (2021). Effect of natural and chemical colorant supplementation on performance, egg-quality characteristics, yolk fatty-acid profile, and blood constituents in laying hens. Sustainability 13 (8), 4503. doi:10.3390/su13084503
Scholtz, V., Pazlarova, J., Souskova, H., Khun, J., and Julak, J. (2015). Nonthermal plasma - a tool for decontamination and disinfection. Biotechnol. Adv. 33 (6), 1108–1119. doi:10.1016/j.biotechadv.2015.01.002
Shi, H., Ileleji, K., Stroshine, R. L., Keener, K., and Jensen, J. L. (2017). Reduction of aflatoxin in corn by high voltage atmospheric cold plasma. Food Bioprocess Technol. 10, 1042–1052. doi:10.1007/s11947-017-1873-8
Smet, C., Govaert, M., Kyrylenko, A., Easdani, M., Walsh, J. L., and Van Impe, J. F. (2019). Inactivation of single strains of Listeria monocytogenes and Salmonella typhimurium planktonic cells biofilms with plasma activated liquids. Front. Microbiol. 10 (JULY), 1539–1615. doi:10.3389/fmicb.2019.01539
Statistica (2024). Per capita consumption of eggs in the U.S. 2000-2024. Available at: https://www.statista.com/statistics/183678/per-capita-consumption-of-eggs-in-the-us-since-2000/.
Thomas-Popo, E., Mendonça, A., Misra, N. N., Little, A., Wan, Z., Moutiq, R., et al. (2019). Inactivation of Shiga-toxin-producing Escherichia coli, Salmonella enterica and natural microflora on tempered wheat grains by atmospheric cold plasma. Food control. 104, 231–239. doi:10.1016/j.foodcont.2019.04.025
Tirpanalan, O., Zunabovic, M., Domig, K. J., and Kneifel, W. (2011). Mini review: antimicrobial strategies in the production of fresh-cut lettuce products. Sci. against Microb. Pathogens Commun. Curr. Res. Technol. Adv. 1, 176–188.
Wan, Z., Chen, Y., Pankaj, S. K., and Keener, K. M. (2017). High voltage atmospheric cold plasma treatment of refrigerated chicken eggs for control of Salmonella Enteritidis contamination on egg shell. LWT - Food Sci. Technol. 76, 124–130. doi:10.1016/j.lwt.2016.10.051
Wang, F. (2017). Influence of long-term survival on resistance of Salmonella enterica serovar Typhimurium and Escherichia coli O157:H7 to physical or chemical food processes. ProQuest dissertations and theses. Available at: https://dr.lib.iastate.edu/entities/publication/edf63ccd-0673-4d8b-a762-70da5ffe24c5.
Wang, F., Mendonca, A., Brehm-Stecher, B. F., Dickson, J., Dispirito, A., Shaw, A., et al. (2018). Long-term survival phase cells of Salmonella Typhimurium ATCC 14028 have significantly greater resistance to ultraviolet radiation in 0.85% saline and apple juice. Foodborne Pathogens Dis. 15 (9), 538–543. doi:10.1089/fpd.2018.2423
Walsh, J. L., Iza, F., Janson, N. B., Law, V. J., and Kong, M. G. (2010). Three distinct modes in a cold atmospheric pressure plasma jet. J. Phys. D: Appl. Phys. 43 (7), 075201. doi:10.1088/0022-3727/43/7/075201
Wen, J., Anantheswaran, R. C., and Knabel, S. J. (2009). Changes in barotolerance, thermotolerance, and cellular morphology throughout the life cycle of Listeria monocytogenes. Appl. Environ. Microbiol. 75 (6), 1581–1588. doi:10.1128/AEM.01942-08
Wen, J., Deng, X., Li, Z., Dudley, E. G., Anantheswaran, R. C., Knabel, S. J., et al. (2011). Transcriptomic response of Listeria monocytogenes during the transition to the long-term-survival phase. Appl. Environ. Microbiol. 77 (17), 5966–5972. doi:10.1128/AEM.00596-11
Williams, W. D. (1992). Origin and impact of color on consumer preference for food. Poult. Sci. 71 (4), 744–746. doi:10.3382/ps.0710744
Yepez, X. V., and Keener, K. M. (2016). High-voltage atmospheric cold plasma (HVACP) hydrogenation of soybean oil without trans-fatty acids. Innovative Food Sci. Emerging Technol. 38, 169–174. doi:10.1016/j.ifset.2016.09.001
Keywords: Salmonella, eggs, long-term survival, sub-lethal injury, non-thermal technology
Citation: Barry K, Mendonça A, Phillips GJ, Boylston T, Fortes-Da-Silva P, Brehm-Stecher B, Juneja V and Wan Z (2024) Long-term-survival phase cells of Salmonella enteritidis ATCC 13076 exhibit significantly greater tolerance to atmospheric cold plasma treatment of shell eggs. Front. Food. Sci. Technol. 4:1442761. doi: 10.3389/frfst.2024.1442761
Received: 02 June 2024; Accepted: 30 September 2024;
Published: 18 October 2024.
Edited by:
Fernando Perez Rodriguez, University of Cordoba, SpainReviewed by:
Leonardo do Prado-Silva, University of São Paulo, BrazilPantu Kumar Roy, Gyeongsang National University, Republic of Korea
Copyright © 2024 Barry, Mendonça, Phillips, Boylston, Fortes-Da-Silva, Brehm-Stecher, Juneja and Wan. This is an open-access article distributed under the terms of the Creative Commons Attribution License (CC BY). The use, distribution or reproduction in other forums is permitted, provided the original author(s) and the copyright owner(s) are credited and that the original publication in this journal is cited, in accordance with accepted academic practice. No use, distribution or reproduction is permitted which does not comply with these terms.
*Correspondence: Aubrey Mendonça, YW1lbmRvbkBpYXN0YXRlLmVkdQ==
†Present address: Gregory J. Phillips, Department of Infectious Diseases, College of Veterinary Medicine, University of Georgia, Athens, United States