- 1Institute of Soil, Water and Environmental Sciences, Agricultural Research Organization, Volcani Center, Rishon LeZiyyon, Israel
- 2Department of Food Quality and Safety, Agricultural Research Organization, Volcani Center, Rishon LeZiyyon, Israel
- 3Unit of Medicinal and Aromatic Plants, Newe Ya’ar Research Center, Agriculture Research Organization, Ramat Yishay, Israel
Crop produce can be contaminated in the field during cultivation by bacterial human pathogens originating from contaminated soil or irrigation water. The bacterial pathogens interact with the plant, can penetrate the plant via the root system and translocate and survive in above-ground tissues. The present study is first to investigate effects of an abiotic stress, salinity, on the interaction of plants with a bacterial human pathogen. The main sources of human bacterial contamination of plants are manures and marginal irrigation waters such as treated or un-treated wastewater. These are often saline and induce morphological, chemical and physiological changes in plants that might affect the interaction between the pathogens and the plant and thereby the potential for plant contamination. This research studied effects of salinity on the internalization of the bacterial human pathogen Salmonella enterica serovar Newport via the root system of sweet-basil plants, dissemination of the bacteria in the plant, and kinetics of survival in planta. Irrigation with 30 mM NaCl-salinity induced typical salt-stress effects on the plant: growth was reduced, Na and Cl concentrations increased, K and Ca concentrations reduced, osmotic potential and anti-oxidative activity were increased by 30%, stomatal conductance was reduced, and concentrations of essential-oils in the plants increased by 26%. Despite these physical, chemical and morphological changes in the plants, root internalization of the bacteria and its translocation to the shoot were not affected, and neither was the die-off rate of Salmonella in planta. The results demonstrate that the salinity-induced changes in the sweet-basil plants did not affect the interaction between Salmonella and the plant and thereby the potential for crop contamination.
Introduction
It is now recognized that human pathogens from soil or irrigation water may become associated with the plant following external contamination (Reviewed by Erickson, 2012), penetrate internal plant tissues via the shoot as well as the root, and translocate and survive inside the plant (Guo et al., 2002; Solomon et al., 2002; Jablasone et al., 2005; Bernstein et al., 2007b; Klerks et al., 2007a; Mootian et al., 2009; reviewed by Erickson, 2012; Hirneisen et al., 2012; Kisluk and Yaron, 2012). The interaction between the plants and human pathogens is not well understood and due to potential health risks involved, is currently the focus of numerous investigations (Barak and Schroeder, 2012; Wiedemann et al., 2015). The physiological and molecular responses of the plant to colonization with bacterial human pathogens and the factors which affect it are the focus of numerous studies (Brandl, 2006; Klerks et al., 2007b; Schikora et al., 2008; Teplitski et al., 2009; Gu et al., 2011; Gutierrez-Rodriguez et al., 2012; Hirneisen et al., 2012; Markland and Kniel, 2015).
A common source for contamination of agricultural fields by human pathogenic bacteria are soil amendments such as organic manures and marginal irrigation waters such as treated or un-treated effluents. These are often saline and contain higher concentrations of Na and Cl than tap water, as well as higher levels of microorganisms including human pathogenic bacteria (Gerba and Smith, 2005; World Health Organization [WHO], 2006; Scheierling et al., 2011). Their application to the field may therefore impose salt-stress on the plants and increase health risks involved in crop contamination with human pathogenic microorganisms.
Salt-stress induces a range of molecular, physiological and morphological changes in plant tissues, which in addition to their effect on the performance of the plant may affect its response to contaminating human pathogens. Specifically, salinity increases root branching (Bernstein and Kafkafi, 2002) and hence the openings potentially available for bacterial internalization through the root; it stimulates early root vasculature development and hence the potential for root-shoot transport of root internalized pathogens; salinity-induced changes in quantity of root exudates and concentration of specific exudate constituents such as free amino acids (Marin et al., 2010) may affect pathogen chemotaxis toward roots (Klerks et al., 2007b); and inhibited root elongation reduces the volume of soil explored by roots and hence its contact with pathogens (Reviewed by Bernstein and Kafkafi, 2002; Bernstein, 2013). Additionally, increased osmotic potential and salts concentrations in the plant cells under salinity, and intensified oxidative or anti-oxidative responses (Hasegawa et al., 2000; Munns and Tester, 2008; Bernstein et al., 2010) may affect in planta survival of internalized bacteria. Furthermore, exposure to abiotic stresses such as salinity, can prime various antibacterial induced resistance phenomena in plants (Beckers and Conrath, 2007; Foyer et al., 2016) which may affect human pathogens. Plants are known to accumulate antimicrobial compounds, i.e., phytoalexins, as a result of infection or stress. These elicitators include a wide range of chemical compounds of microbial and plant origin, as well as inorganic salts that typically accumulate in the plant under salinity (reviewed in Kuc, 1995; Boller and Felix, 2009). In basil, salinity increases the accumulation of salts in the plant and augment the concentration of essential oils, which are known to have antibacterial properties (Bernstein et al., 2009b). Therefore, among other effects, salinity may prime systemic resistance in plants, which might influence the plant’s response to microorganisms.
Although Salmonella is known to be tolerant to high NaCl levels (Kang et al., 2007), no information is available concerning effects of salinity on the association of human pathogenic bacteria with plants. Such data is important for understanding the potential for contamination of produce by pathogens from contaminated water or manure under saline conditions.
Salmonella is a common etiological agent of food-borne infections (Sivapalasingam et al., 2004), and Salmonellosis outbreaks have been reported to be associated with the consumption of numerous plant products (Campbell et al., 2001; Horby et al., 2003; Fatica and Schneider, 2011) and recently also with sweet basil (Pakalniskiene et al., 2006; Pezzoli et al., 2007). Salmonella from contaminated soil can internalize sweet-basil through the root, and transport in planta to vegetative and reproductive organs of the plant (Gorbatsevich et al., 2013). The survival duration of the pathogen in planta is very short (<30 h), possibly due to the presence of antibacterial substances of the essential oils in this aromatic herb. Salinity was previously demonstrated to affect the content of essential oils in sweet-basil (Bernstein et al., 2009b) and in other aromatic plants (El-Fadl et al., 1990) as well as oil composition (Tarchoune et al., 2013). Although S. enterica strains can tolerate a wide range of salinities (Kang et al., 2007), the induced osmotic pressure might also affect the physiology of the pathogen. It is therefore feasible that persistence of the bacteria in the plant tissues will be affected by exposure of the host plant to saline conditions during cultivation.
Sweet basil (Ocimum basilicum L.) is a popular medicinal, culinary and ornamental herb (Roberto and Simon, 2000; Simon, 2000). It is marketed as a dry and fresh herb and is also cultivated for industrial production of essential oils (Dudai et al., 2002). The essential oil, of sweet basil is rich in phenolic compounds, and is used in the pharmaceutical and perfume industries throughout the world
This study was undertaken to evaluate the following hypotheses: (i) Salinity stress affects root internalization of S. enterica serovar Newport into sweet-basil. (ii) In planta transport of the human pathogen is reduced under salinity. (iii) Salinity affects survival kinetics of the human pathogen in the leaf tissues of sweet-basil. To test these hypotheses we studied: (1) Effects of plant exposure to salinity on the internalization of Salmonella into the plants via the root system, (2) Effect of salinity on the survival of Salmonella within leaf tissues. (3) Growth kinetics of Salmonella in plant sap from plants cultivated with non-saline or saline stressed plants. (4) Effect of salinity on survival of Salmonella in the root growing medium (5) Plant physiological parameters associated with plant response to salinity (salts and mineral accumulation, osmotic potential, relative water content).
Materials and Methods
Plant Material and Growing Conditions
The effect of soil salinity on the ability of Salmonella cells to enter the roots and translocate to the aerial parts of the plant and survive in planta was studied in pot-grown basil plants. Twenty-day-old basil seedling (O. basilicum, cv. Perrie) (Dudai et al., 2002) were transferred to 1500 ml black plastic pots, one seedling per pot. Each pot contained 1000 g of water-saturated potting mixture consisting of 40% peat, 40% Coir (coconut fibers), 20% tuff (granulated volcanic ash), and added fertilizers. The pre-mixed fertilizers consisted of a 4-months slow-release Multicot (20:10:20 NPK) 2 kg m-3 potting mixture, and 0.5 kg 20:10:15 NPK per m-3 of potting mixture as a soluble starter with added microelements. The pots were kept in a naturally ventilated greenhouse, (20°C and 42°C minimum and maximum temperatures, respectively) and were watered daily. The plants were cultivated for 10 weeks, from the seedling stage until flowering.
The salinization treatment was initiated when the plants were 30 days post-germination. The plants were drip-irrigated daily, from day 30 to 60, with non-saline water (control) or water containing 30 mM NaCl (salt-treatment). To prevent over salinization of the growing media the irrigation volume was regulated to allow generation of 25–50% of leachate. Throughout the salinization period the EC (electric conductivity) of the leachate was monitored regularly and did not increase above 3.5 dSm-1, i.e., 0.5 EC unit above the EC value of the irrigation solution in the salt treatment.
Physiological Characteristics of the Plant Tissue
A flow-chart describing the experimental set-up is presented in Supplementary Figure 1. Leaves of 60-day-old plants, were sampled for the analysis of Na, Cl, K, Mg, Ca, osmotic potential, relative water content, antioxidative activity, content of essential oil in the leaves, and root and shoot fresh-weight (FW). Parallel leaves were used for the bacteriological studies in the project. All measurements were conducted with five biological repeats, for plants irrigated with non-saline and saline water. Na and Cl were analyzed as described in Bernstein et al. (2009b). Potassium, Mg, and Ca were analyzed as described in Neves-Piestun and Bernstein (2005). Root and shoot FW, area of individual leaf, and contents of essential oils were determined as described by Bernstein et al. (2009b). Osmotic potential measurements were conducted following Shoresh et al. (2011). Antioxidant activity was measured by a radical scavenging assay using 1, 1-diphenyl-2-picryldrazyl (DPPH) according to Bernstein et al. (2009a) and the antioxidant activity is expressed as chlorogenic acid equivalent. Photosynthesis and stomatal conductance measurements were conducted with a Li-Cor 6400 gas exchange photosynthesis system (Li-Cor, Lincoln, Nebraska, USA) at 400 μmol mol-1 CO2, 21% (v/v) O2, air RH range of 30 ± 1.5%, the temperature was 32 ±0.5°C and photosynthetic active radiation (PAR) of 200 μ m-2s-1. Measurements were performed after 3 h of light. Relative water content in the plant tissue, in percentage, was calculated as of the equation RWC (%) = 100 × (Wf-Wd)/(Wt-Wd). Wf is fresh weight of the leaf tissue; Wt, is weight of the turgid leaf tissue following hydration in double distilled water for 24 h; and Wd is dry weight of the leaf tissue. Root branching was measured as the averaged number of 2nd order laterals per cm root length in 10 cm long white roots. Data for each replicated plant was averaged from measurements of three roots. The experiment was repeated three times.
Bacterial Strain and Inoculum Preparation
Salmonella enterica serovar Newport (strain 96E01153C-TX) was used throughout the study. This strain was originally isolated from alfalfa seeds associated with an outbreak of salmonellosis (Inami and Moler, 1999). It was used in previous studies (Barak et al., 2002; Charkowski et al., 2002) and was deposited in the ‘California Department of Health Services Microbial Diseases Laboratory Branch’ collection (Friedman et al., 2002). Bacterial cultures were kept as glycerol stocks at -20°C. Before each experiment fresh cultures were grown overnight in Lysogeny broth (LB) broth (Difco, Lawrence, KS, USA) supplemented with nalidixic acid (30 μg ml-1) and streptomycin (50 μg ml-1) under shaking (150 rpm) at 37°C. Bacteria were washed once by centrifugation (6000 rpm for 5 min at 20°C) and suspended in sterilized distilled water (SDW) to a final concentration of 1 × 109 cfu ml-1. Bacterial concentration was determined essentially following Golberg et al. (2011) and Gorbatsevich et al. (2013), except that 10-fold dilutions were plated on xylose lysine deoxycholate (XLD) agar (Difco, Lawrence, KS, USA) plates supplemented with nalidixic acid streptomycin in order to confirm the presence of the inoculated Salmonella strain.
Bacterial Internalization through the Root
Sixty-day-old control and salinized plants, 20 plants per treatment were used for quantification of bacterial internalization through the root. The experiment was repeated three times. In each replicated experiment, the soil in half of the pots was inoculated with Salmonella, and bacterial concentration in the shoot was determined 24 h later to allow time for root uptake and in planta translocation to the shoot. The concentration of Salmonella in the shoot in each experiment was quantifies in 10 independent replicates, i.e., 10 inoculated plants, and 10 non-inoculated plants in each of the non-salinized (control) and the salinity treatments. To minimize contamination of the aerial parts of the plant during inoculation and by direct contact with the contaminated potting mixture, the experimental pots were individually covered with a plastic sheet as is described by Bernstein et al. (2007a). In short, the experimental pots were individually covered above the growing medium with a white, opaque, perforated polypropylene sheet (50 μm thickness; Salina, Nazareth Illit, Israel). The seedlings emerged through a 30-mm-long slit in the polypropylene cover. Ten pinpuncture holes in each cover allowed additional aeration.
Average night and day temperatures in the greenhouse throughout the duration of the experiments were 25.0 ± 0.4 and 27.0 ± 0.6°C, respectively. Pot inoculation was performed by carefully pipetting under the plastic cover 10 ml of bacterial suspension containing 109 CFU ml-1 (inoculation treatment) or 10 ml of water (control). Final concentration of bacteria in the soil was 106 CFU g soil-1. Twenty-four hours after inoculation of the potting medium ten plants per treatment were aseptically excised, 5 cm above the pot cover. Ten grams of plant tissue was sampled for bacteriological analysis from each replicated pot. For quantification of Salmonella in the experimental pots, 1 g of wet potting medium, at 75–80% of field capacity, was sampled from the root growing zone area 24 h after inoculation, in parallel to the collection of plant material for the bacteriological analysis. Bacterial quantification in plant- and soil samples was performed as described below.
Survival of Salmonella in the Leaf
Survival of Salmonella in the leaf was evaluated with an experimental system developed to generate consistent loading of bacteria into the plant tissue. The cut edge of the petiole of excised leaves from 60-day-old plants cultivated as described in section 2.4 above, was immersed in 1.5 ml of Salmonella suspension in an eppendorf tube containing 1 × 109 CFU ml-1. Each tube was sealed with parafilm to prevent contamination of the leaf surface, and to ensure that all water loss is due to uptake by the plant. The leaves were allowed to take up the bacterial suspension or clear water for 18 h at 25°C. Five replicated leaves from each treatment were taken for enumeration of Salmonella. The petioles of the remaining leaves were gently washed in water to remove unattached bacteria and the leaves were transferred to new tubes filled with sterilized fresh water. Salmonella enumeration was performed at 6, 22, and 28 h after the transfer. The tissue analyzed included the excised leaf tissue, excluding the petiole section which came in contact with the loading suspension. Each experiment was performed with five replicated leaves and the experiment was repeated three times on different days. Leaves selected for the analysis were the largest mature leaves on the plant, from the 8th to 10th node down from the top of the main branch. To confirm the internal localization of the loaded Salmonella within the leaf tissue, leaves were disinfected with 1% AgNO3 following Franz et al. (2007) and Gorbatsevich et al. (2013). Preliminary testing confirmed that the disinfectant kills the Salmonella on the leaf surface. Specifically, no bacteria were detected in leaf prints on LB agar after surface sterilization, demonstrating the efficiency of the surface-disinfection; and no Salmonella colonies were detected in leaf prints prior to the surface disinfection, negating the possibility that Salmonella reached the aerial parts by external migration. No Salmonella were identified also in the clean water after immersion of the loaded leaves, demonstrating that the reduction in bacterial number in the phyllosphere was not a result of their migration to the water (Gorbatsevich et al., 2013). Enumeration of Salmonella was performed as described previously (Golberg et al., 2011; Gorbatsevich et al., 2013). Furthermore, no difference in the number of Salmonella CFU was detected among leaves prior to and after surface sterilization (data not shown), supporting that the identified cells resided internally.
Survival of Salmonella in Plant Sap In Vitro
The effect of salinity on the kinetics of Salmonella survival was investigated also in vitro in plant sap. The sap was expressed from fresh vegetative tissue of sweet-basil plants irrigated with non-saline vs. saline irrigation water. The experiment was conducted with 10 biological repeats and was repeated three times. The plants were sampled for the analysis 60 days post-germination. For the expression of the sap, fresh basil leaves were ground with a coffee mill pre-sterilized with 70% ethanol. The ground plant material was centrifuged at 10000 rpm for 15 min at room temperature and the obtained sap was filtered through a 0.22 μm membrane for removal of bacteria. One hundred microlitre of the filtered sap was pipeted into a 1.5 ml eppendorf tube and was spiked with 10 μl of the bacterial suspension to give a final concentration of 103 cfu ml-1 Salmonella. The tubes were incubated at 30°C and the concentration of the bacteria in the sap was followed over 24 h. Bacteriological analysis was conducted as described above.
Effect of Salinity on the Fate of Salmonella in the Potting Medium
The effect of saline irrigation on microbial survival in potting medium was tested in the experimental pots without plants. This allowed to evaluate the sensitivity of Salmonella to salinity under the experimental conditions. Fifty 1500 ml black plastic pots, same as those used for plant cultivation, were each filled with 1000 g of the potting medium (Tuff Merom Golan, Merom Golan, Israel). The potting medium was saturated as was previously described (Bernstein et al., 2007a) with water containing a range of NaCl salinities (0, 20, 50, 150, and 500 mM NaCl), 10 pots per salinity level. The experimental pots were individually covered above the soil level as previously described (Bernstein et al., 2007a). Sixteen hours thereafter, five pots per salinity level were each inoculated with 40 ml of suspension containing 109 CFU ml-1 Salmonella Newport (107 CFU g-1 soil). The pots were kept in a greenhouse under the same conditions practiced for plant cultivation. Potting medium from five inoculated and five uninoculated pots for each salinity level was sampled for bacteriological analysis of Salmonella and for total aerobic bacterial count, 24 h and 5 days following inoculation. The experiment was repeated three times.
Bacteriological Analysis of Plant and Soil Samples
Plant tissue sampled for bacteriological analysis from all experiments was first surface sterilized by dipping in 1% AgNO3 for 10 s, followed by two washing steps 10 s each in SDW as is described above for the ‘in leaf’ survival experiments. The effectiveness of this method for basil was demonstrated before (Gorbatsevich et al., 2013). The plant tissue was then cut into several pieces (about 1 cm2 each), and placed in a pre-weighed plastic stomacher bag (Plastiques gosselin, Borre, France) to determine its fresh weight. Buffered peptone water (BPW; 50 ml) was added to each bag, and the contents were crushed for 1 min by a stomacher (Interscience BagMixer 400, Saint Nom La Bretèche, France). Enumeration of Salmonella CFU in the pummeled suspensions was conducted following Gorbatsevich et al. (2013). Since preliminary studies demonstrated that Salmonella concentration in the suspension was sometimes below the detection limit (<100 CFU g plant-1), the BPW suspension was also further incubated for 24 h at 37°C for pre-enrichment and then used for a qualitative assessment of the presence of Salmonella by plating on XLD plates. If no suspected Salmonella colonies were observed on the XLD agar after the 24 h pre enrichment, 1 ml of BPW pre-enriched culture was enriched in 9 ml of tetrathionate brilliant green (TBG) and in 9 ml of Rappaport-Vassiliadis soy peptone (RVS) broths (Hy-Labs, Rehovot, Israel). After incubation overnight of TBG (at 37°C) and RVS (at 42°C) the presence of Salmonella was determined following Golberg et al. (2011) and Gorbatsevich et al. (2013).
For quantification of Salmonella in the potting medium, 5 g of potting medium was taken from each pot and placed in 50-ml tubes, containing 45 ml of BPW. The samples were mixed for 1 min each at maximum speed with a Genie 2 vortex and then incubated on a shaking platform for 1 h at 25°C, to facilitate release of medium-associated bacteria. Determination of Salmonella concentration in the potting medium extracts was performed by plating 100 μl of 10-fold serial dilutions on XLD agar following incubation overnight at 37°C. Duplicate plating was performed for each determination. The plates were examined after incubation and typical black colonies were presumptively identified as Salmonella. No such colonies were found in any of the control treatments (uninoculated potting medium). To confirm black colonies observed after the plating in either plant or soil samples as Salmonella, additional biochemical and serological tests were performed following the U.S. Food and Drug Administration Bacteriological Analytical Manual methods1. For biochemical evaluation suspected Salmonella cells were grown on TSI (triple sugar iron) and LI (lysine iron) agar (Hy-Labs, Rehovot, Israel). For serological evaluation, somatic (O) antiserum was used (central laboratories of Ministry of health, Jerusalem, Israel). Potting medium samples were also tested for total culturable aerobic bacteria by plating decimal dilutions of the BPW suspension on LB plates and incubating the plates overnight at 37°C. Following the experiments, all the contaminated materials, including plants and potting medium were decontaminated by autoclave before disposal.
Statistical Analysis
Statistical analysis was carried out with the JMP software package, version 5 (2002, SAS, Cary, NC, USA). Means and standard errors of Salmonella CFU in the potting medium and plant tissues, and the total bacterial count in the potting medium were determined. Data were subjected to one-way ANOVA analysis and Tukey honestly significant difference for comparison of means. Most probable number, MPN, was calculated as described by Blodgett (2010), for a ‘single dilution with positive tubes’.
Results
Plants Response to Salinity
The basil plants demonstrated a characteristic response of plants to salinity. Growth of the root and the shoot and the area of individual leaves were reduced under salinity (Table 1). The decrease in shoot/root biomass ratio represents higher growth sensitivity of the shoot compared to the root to salinity. Salinity induced a change in the nutritional status of the plant tissues: the salinity source ions, Na and Cl, increased about 4- and 9-fold, respectively, under salinization in the leaf tissues (Figure 1A) and the concentration of the macronutrients Ca and K were significantly reduced (Figure 1B). The osmotic potential of the tissue sap increased by 30%, and the relative water content of the tissue reduced by 6% (Figure 2) representing a change in the water status of the plant. Furthermore, CO2 fixation and stomatal conductance were reduced by 29 and 26%, respectively; and the % of essential oil content in leaves was increased by 26% (Table 1). The increase in antioxidant activity (by 30%) demonstrates a stress-induced change in the oxidative state of the plant tissue (Table 1).
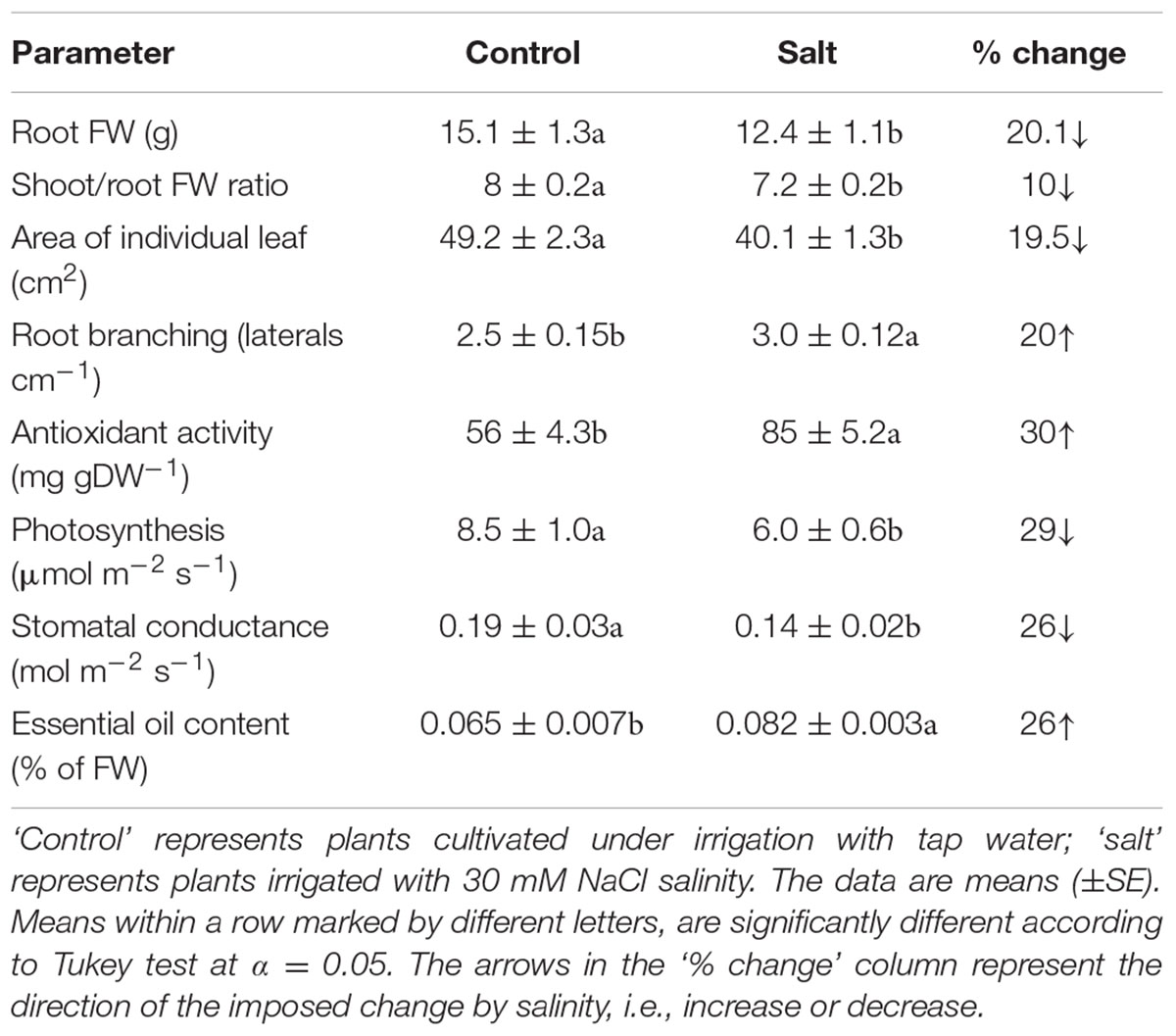
TABLE 1. Effect of irrigation with saline water on morphological and physiological characteristics of the plants.
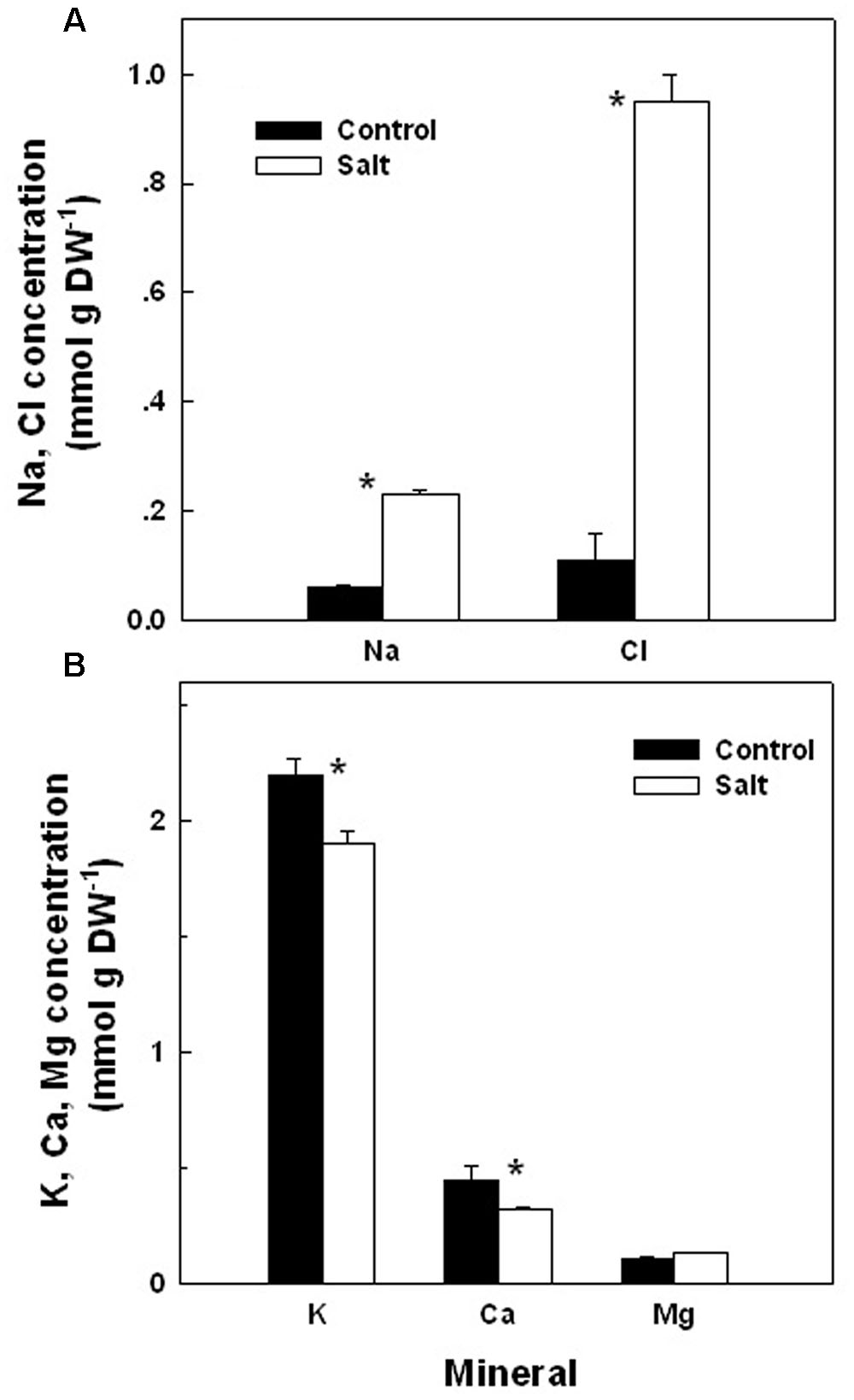
FIGURE 1. Effect of irrigation with saline water on the concentrations of the salts Na and Cl (A), and the macronutrients K, Ca, and Mg (B), in leaves of plants cultivated under non-saline (Control) and saline irrigation (Salt). The data are means ± SE. Asterisks represent a significant difference between the concentration of a mineral element in control vs. salt plants (P < 0.05).
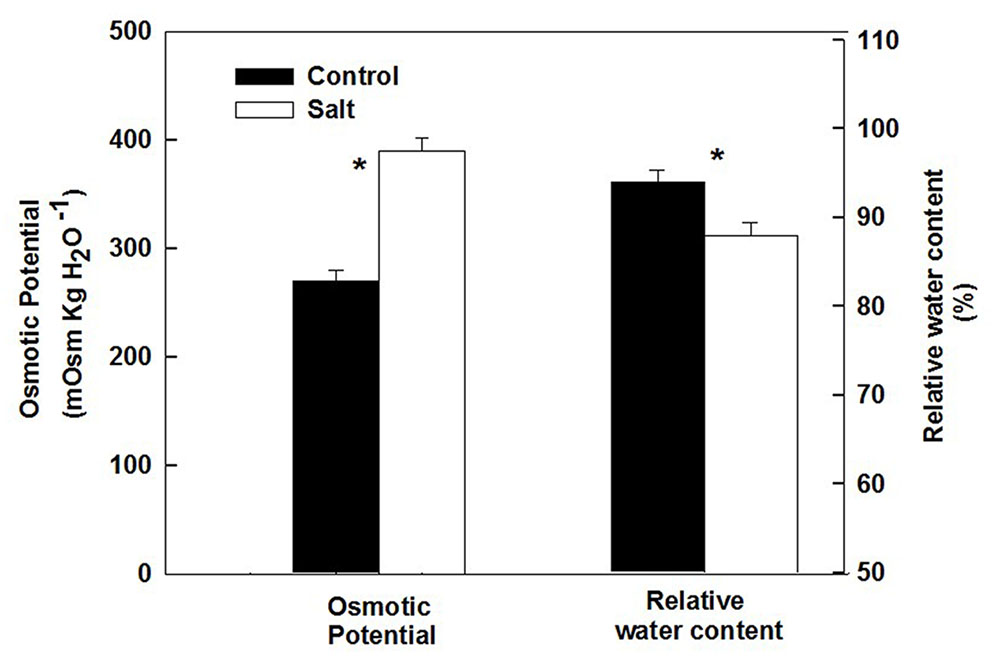
FIGURE 2. Effect of irrigation with saline water on osmotic potential and relative water content of leaves from plants cultivated under non-saline (control) and saline irrigation (salt). The data are means ± SE. Asterisks represent a significant difference between the control and salt plants (P < 0.05).
Effect of Salinity on the Ability of Salmonella to Contaminate Plants via the Roots
The susceptibility of the plants to Salmonella penetration via the roots did not vary with exposure of the roots to salinity (Table 2). In both salinized and non-salinized plants, no Salmonella cells were identified in the above ground plant tissue by direct plating, and the same number of replicates (5 out of 10) were tested positive for Salmonella after an enrichment step (Table 2). MPN for positive identification in the enrichment tests was 70.7g-1 with a 28.9 (low) and 173.7 (high) 95% confidence limit. The concentration of the pathogen in the potting medium, and the total bacterial count, were not affected as well by the saline irrigation (Table 2). No Salmonella were observed in uninoculated plants and on the surface of disinfected leaves (data not shown) supporting that the identified Salmonella was localized to inner tissues of the plants.

TABLE 2. Effect of irrigation with saline water on the internalization of Salmonella into plants via the root system.
Effect of Salinity on the Survival of Salmonella in Leaves
The survival duration of Salmonella in the basil phyllosphere was investigated in leaves from plants cultivated under non-stressed and salt-stressed conditions. A considerable reduction in Salmonella counts in the leaf tissue was apparent already 6 h after the end of the inoculation period, and no Salmonella was detected 16 and 24 h thereafter (Figure 3). The rate of decrease in Salmonella CFU was not significantly different in leaves from control and salt-stressed plants, ranging 2.9 × 103 - 2.1 × 103 CFU g-1 day-1 at the first 6 h following inoculation (p = 0.554). The lack of effect of the salinity exposure on Salmonella occurred despite the changes in chemical and physical parameters in the plant.
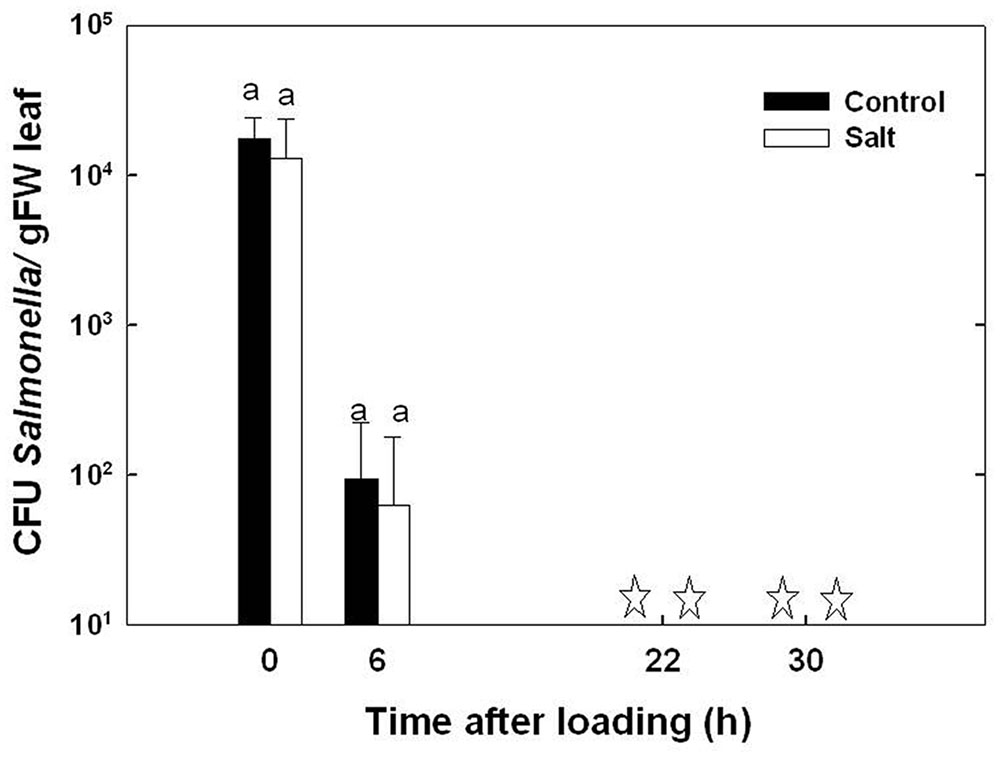
FIGURE 3. Effect of irrigation with saline water on the survival of Salmonella within the leaf. The presence of Salmonella in the leaves was determined at the end of the pathogen loading period (time 0), and 6, 22, and 30 h thereafter. ‘Control’ represents leaves from plants cultivated under irrigation with tap water; ‘salt’ represents leaves from plants irrigated with 30 mM NaCl salinity. The data are means (±SE). Means in a single sampling day, marked by same letters, are not significantly different according to Tukey test at α = 0.05. Asterisks represent that no bacteria (< 1 CFU/g-1) were detected in the leaves even after an enrichment test.
Kinetics of Salmonella Growth in Sap Expressed from Control and Salinized Plants
Sap extracted from leaves of control as well as salt-stressed plants supported growth of the Salmonella population in vitro (Figure 4). The kinetics of bacterial population increase was similar in sap from plants cultivated under control and salt conditions, demonstrating a sigmoidal curve with leveling off at 20 h after inoculation (Figure 4). The rate of growth was similar under control and salt conditions (Figure 4 insert). No statistical difference was found between the control and salt treatment in the concentration (Figure 4) or the concentration increase rate (Figure 4 insert) at any of the time points analyzed (p-values ranged: 0.857–0.098). The lack of changes in the kinetics of bacterial growth in vitro occurred despite the physical differences between the sap of the two treatments. Both electrical conductivity (EC) level and the osmotic potential of the sap significantly increased under salinization, while no changes in pH level were apparent (Figure 5).
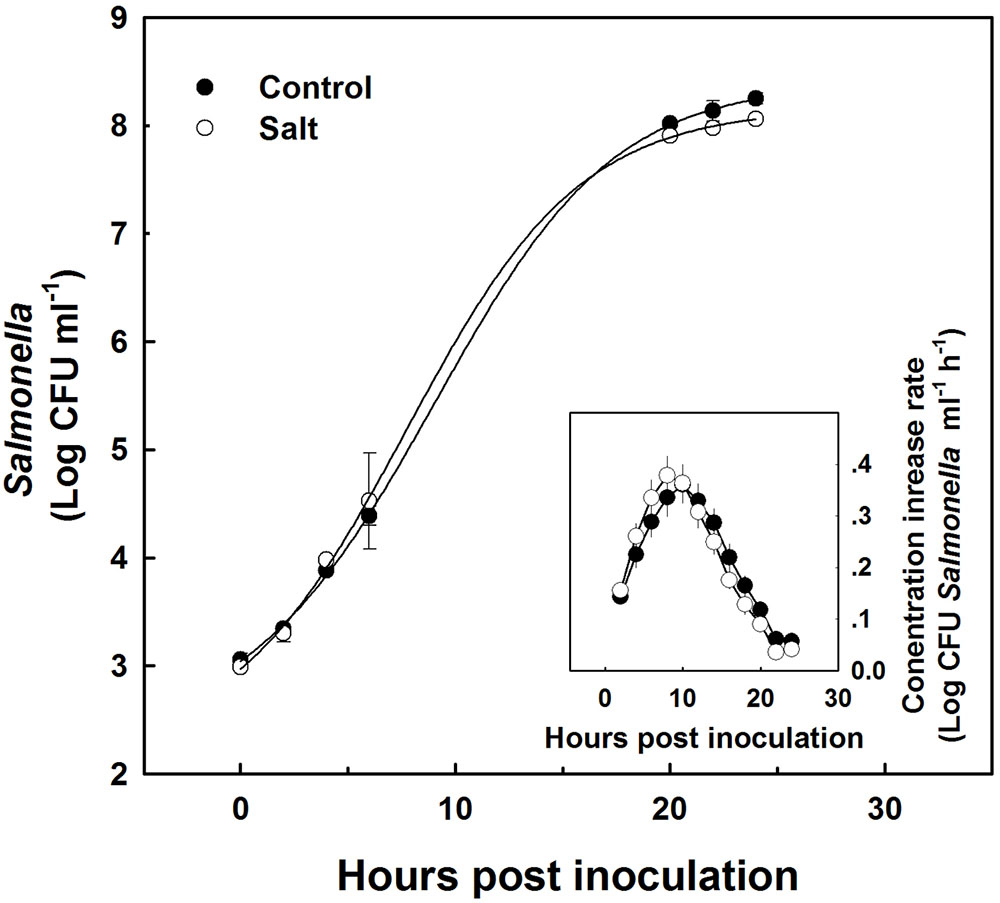
FIGURE 4. Growth kinetics of Salmonella in plant sap expressed from shoots of sweet basil plants cultivated with non-saline (control) or saline irrigation (salt). Changes in Salmonella concentration (main figure), and rate of concentration change (insert) over time following spiking of the sap with 103 CFU ml-1 Salmonella. In the main figure, time 0 present results immediately following sap inoculation; and the lines represent a sigmoidal curve-fit to the data. The data are means ± SE. In the insert, the averaged rates and SE were calculated from the sigmoidal curve-fit data for the individual biological repeats. The difference in concentration and rate between the control and the salt treatment was not statistically different (P < 0.05) for any of the sampling times.
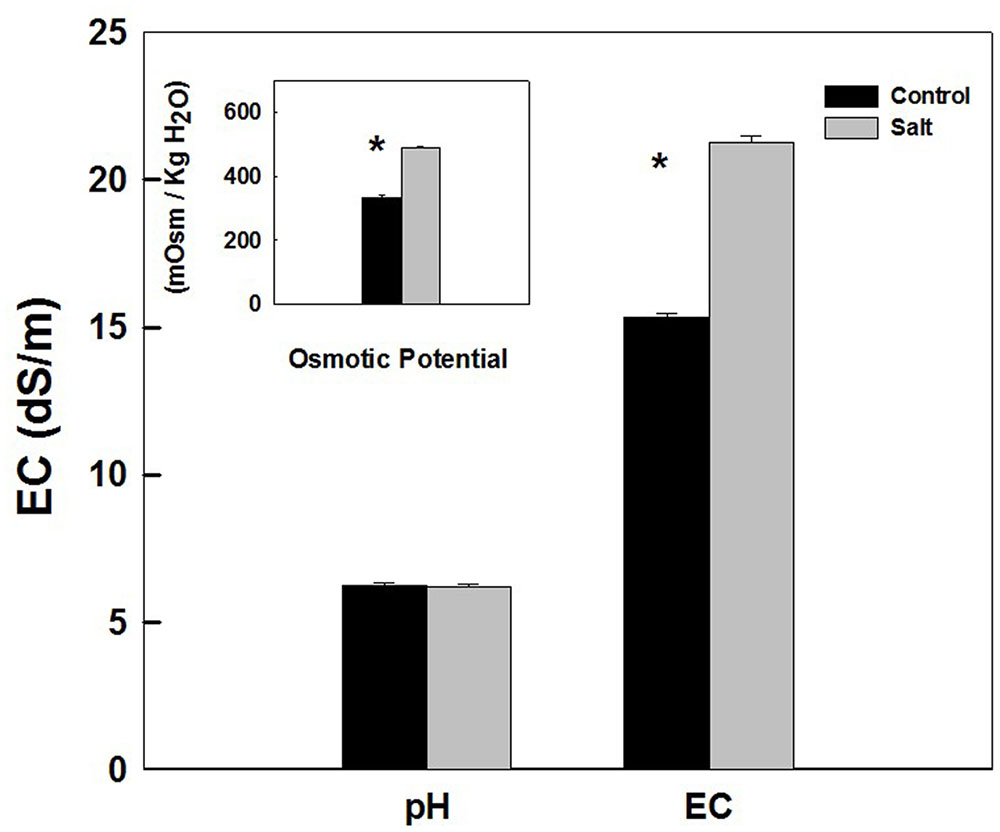
FIGURE 5. pH, electrical conductivity (EC), and osmotic potential of the plant sap used for the in vitro experiments described in Figure 4. The sap was expressed from shoots of sweet basil cultivated under non-saline (Control) and saline irrigation (Salt). The data are means ± SE. Asterisks represent a significant difference between the control and salt plants (P < 0.05).
Effect of Saline Irrigation Water on Microbial Survival in the Potting Medium
The concentrations of Salmonella and total aerobic bacteria in the potting medium were not affected by the salinity level applied (0–500 mM NaCl) (Figures 6A,B). In both measurements conducted, 24 h and 5 days after inoculation, no statistical differences were recorded in Salmonella concentrations or total aerobic bacterial counts throughout the salinity range tested (P = 0.2132 and 0.1875 for 24 h and 5 days after inoculation, respectively). However, the concentrations of salmonella as well as the total bacterial count in the potting medium were reduced over time, i.e., between the first and second measurement. A 1–1.5 log CFUg-1 reduction was recorded between the first and second measurement (Figures 6A,B). This similarity in the extent of concentration decline throughout the salinity concentration range, suggests that the decrease observed over time in the total bacterial count, reflects die-off of the Salmonella population. It also suggests that the total heterotrophic populations were not very high in the potting soil.
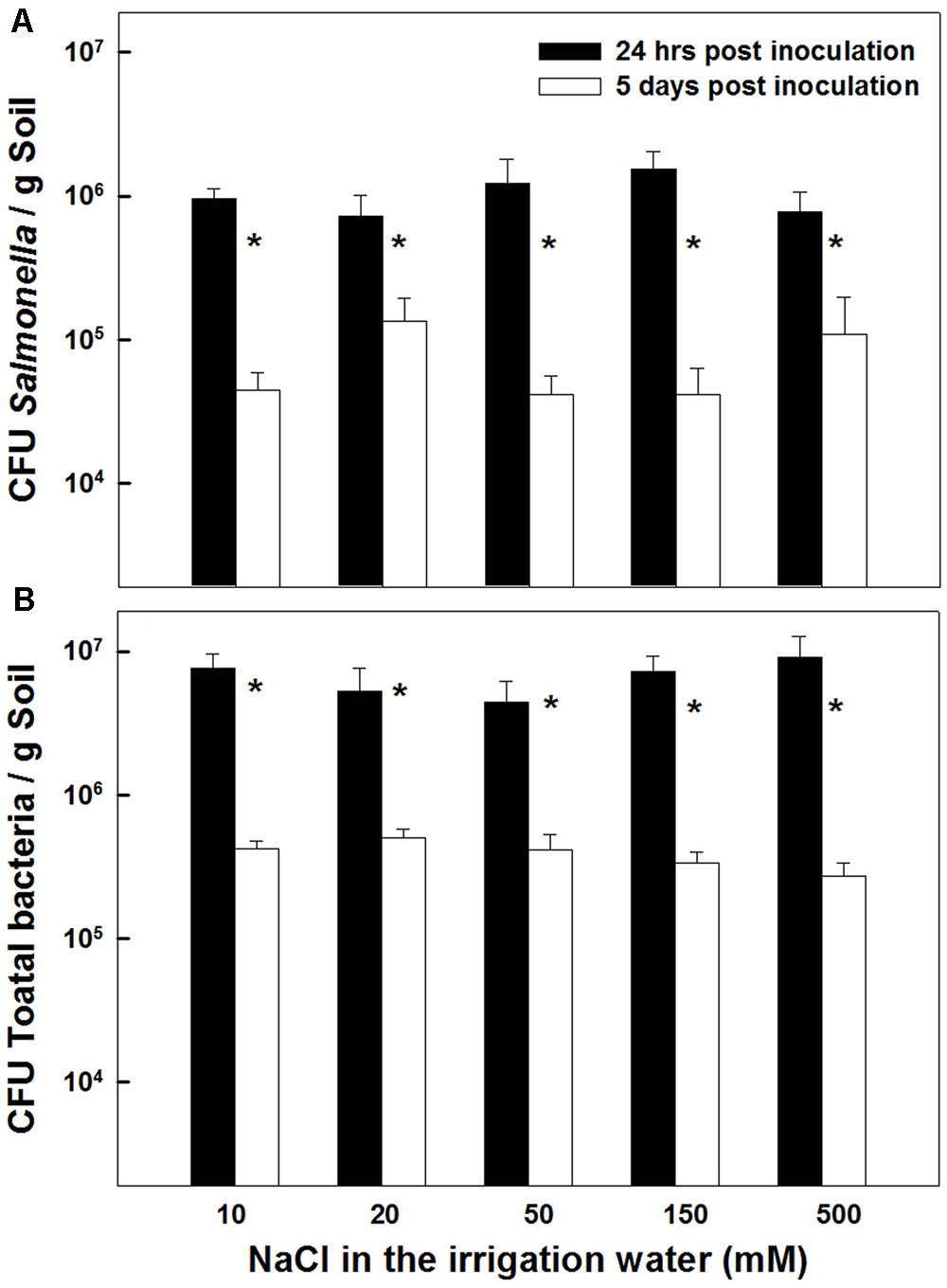
FIGURE 6. Effect of irrigation with saline water on survival of Salmonella (A), and total aerobic bacteria (B), in the potting medium 24 h, and 5 days following inoculation. Data are averages ± SE. The soil was inoculated with 107 CFU Salmonella g soil-1. Asterisks represent significant difference of the measurement on the 1st vs. 5th day after inoculation (P < 0.05) at any salinity level.
Discussion
Effect of Salinity on Shoot Contamination by Root-Internalized Salmonella
The presence of root-internalized Salmonella in the shoot is an integrated result of root internalization, transport to the shoot and in planta persistence. Salinity induces a range of changes in the plant, which may singularly or collectively affect each of these processes and hence the subsequent internal contamination of the shoot by human pathogens present in the soil. The results of this study demonstrate that internal shoot contamination was not affected by saline irrigation (Table 2), suggesting that plant’s susceptibility to root internalization, transport to the shoot and persistence were not compromised by the salinity-induced physiological or morphological changes.
The natural ruptures created by the emergence of lateral roots from the pericycle through the cortex and epidermis are an accepted possibility for entrance ports of pathogens into roots (Lugtenberg et al., 2001; Jablasone et al., 2005; Hirneisen et al., 2012). The increase in root branching observed under salinity (Table 1) increased the number of entry points for the bacteria and hence the potential for root internalization. Additionally, the reduction in shoot/root ratio under salinity (Table 1) which indicates that compared to the control plants, under salinity a larger root mass supported internalization into a unit mass of leaves, represents as well increased potential for internalization into a unit leaf mass. On the other hand, the smaller root system that developed under salinity (Table 1; Bernstein et al., 2009b) restricted the cumulative number of laterals in the root, and thereby the opportunity for bacterial root internalization. The reduced stomatal conductance and hence the potential for transpiration rate under salinity (Table 2) lowers the volume of ascending sap that reaches the leaf and is therefore expected as well to have a restrictive effect on the transport of soil-contaminating bacteria to the shoot. The lack of salinity effects on the incidence of root-internalized bacteria in the shoot (Table 2) could result from integration and balancing out of these opposing affects (Table 1). Zhang et al. (2009) reported that heat and drought stress did not affect the susceptibility of plants (romaine and iceberg lettuce) to contamination by root uptake of E. coli O157:H7, and DiCaprio et al. (2015) reported that drought stress but not flooding stress affected the rates of internalization and dissemination of Human norovirus into lettuce.
Effect of Salinity on the Survival of Salmonella In Planta
Following root internalization the enteric pathogens are exposed to the internal environment of the plant. Numerous plant characteristics, including morphology, water content, phosphate concentration, amounts of bacterial inhibitory phenolics, leaf thickness and endophytic bacteria were shown to affect bacterial populations in the phyllosphere (Thompson et al., 1993; Yadav et al., 2005; Gu et al., 2013; Wiedemann et al., 2015). The prevalence of enteropathogens beneath the epidermis of leaves was found to vary between plant species (Salmonella Typhimurium: Golberg et al., 2011), and between abaxial and adaxial internalization into leaves (E. coli O157:H7: Erickson et al., 2010). Despite the differences in chemical and physical parameters in leaves of the salt-stressed and non-stressed plants (Table 1) the rate of decrease in Salmonella counts was similar under control and saline conditions (2905±350 and 2130±350 CFU h-1 for the control and salinity, respectively, at the first 6 h after loading, Figure 3). The lack of effect of saline-irrigation on the survival of Salmonella in the tissue suggests that the levels reached in the leaves (0.23 and 0.95 μ g DW-1 of Na and Cl, respectively) were not toxic for the bacteria, as could be judged also by the salt-challenge experiments (Figure 6). Tolerance of Salmonella to higher salinities, was also documented (1M NaCl, Kang et al., 2007). Similarly, the reduction in concentration of the macronutrients K and Ca under saline irrigation, did not affect the persistence of Salmonella in the plant, suggesting that the reduced levels were within the range sufficient for survival or adaptation. Intracellular K is known to involve in the osmo-protection response and regulation of turgor in bacteria including enteric bacteria (Booth and Higgins, 1990; Prince and Villarejo, 1990; Meury and Kohiyama, 1991). The salinity-induced changes in two parameters, (e.g., 30% increase of osmotic potential of the leaf sap, and 6% reduction of the relative water content of the leaf tissue) demonstrate altered water relations in the plant (Figure 2). The level of osmotic potential reached under saline irrigation, about 380 mOsm H2O-1, is well within the tolerance level of Salmonella justifying the lack of effects on bacterial survival.
Salmonella was able to survive only very short durations within the leaf (<22 h). Sweet-basil is an aromatic plant which produces essential oils that exhibit antibacterial activity (Bagamboula et al., 2004). Essential oils are known to be active against a wide range of microorganisms including food-borne pathogens (Oussalah et al., 2006). The antimicrobial activity of the essential-oils is assigned only to a small number of the oil constituents (Bakkali et al., 2008). Since environmental conditions such as salinity affect concentrations of only part of the oil components (Dudai, 2005), the increase in essential-oil content under salinity (Table 1) does not necessarily imply higher concentrations of active antibacterial components and a more pronounced antibacterial activity. The lack of salinity effects on bacterial fitness (Figure 3), despite the increased oil content (Table 1), may result from stress effects on the composition of the essential oil (El-Fadl et al., 1990). The essential oils are formed in specialized glands on the plant surfaces and are not found in large quantities within the plant tissues. Whether residual essential oils dissipating to inner plant tissues or to the leaf surface between the oil glands can limit Salmonella survival in the phyllosphere is still to be determined.
Exposure to abiotic stresses such as salinity is known to influence the plants defense system to microorganisms by priming an induced resistance system (Beckers and Conrath, 2007; Balmer et al., 2015; Ramegowda and Senthil-Kumar, 2015). It is accepted today that there is a connection between biotic and abiotic stresses in plants at the molecular level (Conrath et al., 2002; Borges et al., 2014; Foyer et al., 2016), and that priming enable cross-talk between defense mechanisms against pathogens and the response to abiotic stresses (Maleck and Dietrich, 1999; Waller et al., 2005; Ton et al., 2009; Foyer et al., 2016). Inorganic salts such as Na and Cl, which accumulated under salinity in the basil plants (Figure 1), are among the elicitors of phytoalexins (e.g., plant antimicrobial compounds) accumulation (reviewed in Kuc, 1995). The sustained transport and viability of Salmonella under saline irrigation suggest that priming or systemic resistance were not induced by salinity or by the presence of Salmonella, or that it did not affect the pathogen.
Kinetics of Salmonella Culture Growth in Plant Sap In Vitro
In vitro experiments with sweet-basil leaf sap, spiked with S. enterica serovar Newport, demonstrated similar growth rates of the bacteria in sap expressed from plants irrigated with non-saline, or saline irrigation (Figure 4). This is in spite of the higher levels of osmotic potential and EC imposed by the salt-stress. Unlike the leaf phyllosphere (Figure 2) the sap expressed from the leaf was able to support growth of the pathogen in vitro (Figure 4). The micro-environments to which the pathogen is exposed to inside the leaf tissue, vary from the conditions present in the sap expressed from the leaf. For example, in the sap, the pathogen may be exposed to a wider selection of carbon and nitrogen sources otherwise compartmentalized in specific organelles or tissues. While restriction of available nutrients within the leaf might inhibit bacterial growth, the rapid clearance of Salmonella in the leaf may perhaps be attributed to the presence of an active innate immunity in the intact tissue compared to the sap. Moreover, essential oils normally found in specialized glands on the leaf surface when incorporated to the sap are much diluted and serve as a food source for the bacteria rather than diminish it. Furthermore, competition with plant natural endophytic microflora, either bacteria or fungi may affect fitness of Salmonella inside the plant (Klerks et al., 2007a) but not in the plant sap that was filtered for removal of bacteria. The results for culture-growth kinetics of the in vitro experiments complement the results for in planta die-off rates that both demonstrate a lack of salinity effects.
Effect of Salinity on Fate of Salmonella in the Potting Medium
It is well documented that a range of human pathogens, including Salmonella, can survive extended periods of time in soils (Santamaria and Toranzos, 2003; Gerba and Smith, 2005; Bernstein et al., 2007a; Liu et al., 2010; Jacobsen and Bech, 2012). Two to four months survival periods for enteric bacteria in soil were reported in a review by Gerba et al. (1975), and survival duration up to 29 weeks (Islam et al., 2004) was reported for Salmonella. It is therefore not surprising that 5 days following inoculation, a considerable number of viable Salmonella cells were detected in the potting medium (Figure 1A).
Salmonella species are well adapt for survival in diverse environments. However, exposure to a range of environmental stresses, such as nutrient deprivation, pH extremes, oxidative stress, salinity and heat shock, can affect their persistence and virulence (Archer, 1996; Gerba and Smith, 2005). Entero-pathogens encounter osmotic-stress frequently in the host gut, and consequently have evolved an array of stress-adaption mechanisms, that allow them to grow at widely different external osmotic pressures (Brandl, 2006). Result of the present study, which display a tolerance of Salmonella to a wide range of irrigation-water salinities (Figure 6), demonstrate that under conventional saline irrigation setups utilized for crop production (<500 mM NaCl) as well as conditions applied in the present project, the bacteria is able to persist in the soil.
For practical implications, four issues should be considered. First, the presence of plants in the soil and the soil type may affect bacterial persistence (Islam et al., 2004; Bernstein et al., 2008). Second, the concentration of the pathogen applied to the potting medium in the present study was higher than is expected for sporadically contaminated irrigation water. Pathogen survival in soils may be affected by the initial population size as well as by competition with indigenous populations in the soil, which seems lower in the potting mixture compared to soil-based systems. Third, bacteria response to salinity may be affected by the duration of exposure to the stress. Fourth, the behavior of Salmonella may vary in the potting medium used in this study and in various soils, as discussed above. Therefore, more studies are needed before extrapolating bacterial response to salinity in soils and for longer durations then evaluated here. Additionally, bacterial internalization through the root is known to vary with growing conditions, and in most studies involving soil-grown crops, internalization was sporadic and at low levels. It is therefore considered that in undamaged plants, the risk of produce contamination by root internalized bacterial pathogens from contaminated soil may be low (Warriner and Namvar, 2010; Hirneisen et al., 2012; Orlofsky et al., 2016).
Conclusion
The lack of effect of irrigation with saline water on contamination of the shoot with Salmonella suggests that the susceptibility to root internalization, transport to the shoot, and in planta persistence were not compromised. Our results, favor the notion that exposure to salinity does not compromise root penetration and/or in planta transport and survival of enteric pathogenic within the plant.
Author Contributions
NB developed the project, analyzed the results, and wrote the manuscript. EG conducted the experiments, SS regulated the microbiological analyses, ND regulated the essential oils analyses.
Conflict of Interest Statement
The authors declare that the research was conducted in the absence of any commercial or financial relationships that could be construed as a potential conflict of interest.
Acknowledgment
This study was funded by the Israeli ‘Vegetable Growers Association’ Fund, grant No. 02-0005, and by the Chief Scientist Fund of the Ministry of Agriculture, Israel, grant No. 421-0181-09, 421-0177-09.
Supplementary Material
The Supplementary Material for this article can be found online at: http://journal.frontiersin.org/article/10.3389/fpls.2017.00675/full#supplementary-material
Footnotes
References
Archer, D. L. (1996). Preservation microbiology and safety: evidence that stress enhances virulence, and triggers adaptive mutations. Trends Food Sci. Technol. 7, 91–95. doi: 10.1016/0924-2244(96)81303-3
Bagamboula, C. F., Uyttendaeleand, M., and Debevere, J. (2004), Inhibitory effect of thyme and basil essential oils, carvacrol, thymol, estragol, linalool and p-cymene towards Shigella sonnei and S. flexneri. Food Microbiol. 21, 33–42. doi: 10.1016/S0740-0020(03)00046-7
Bakkali, F., Averbeck, S., Averbeck, D., and Idaomar, M. (2008). Biological effects of essential oils–A review. Food Chem. Toxicol. 46, 446–475. doi: 10.1016/j.fct.2007.09.106
Balmer, A., Pastor, V., Gamir, J., Flors, V., and Mauch-Mani, B. (2015). The ‘prime-ome’: towards a holistic approach to priming. Trends Plant Sci. 20, 443–452. doi: 10.1016/j.tplants.2015.04.002
Barak, J. D., and Schroeder, B. K. (2012). Interrelationships of food safety and plant pathology: the life cycle of human pathogens on plants. Annu. Rev. Phytopathol. 50, 241–266. doi: 10.1146/annurev-phyto-081211-172936
Barak, J. D., Whitehand, L. C., and Charkowski, A. O. (2002). Differences in attachment of Salmonella enterica serovars and Escherichia coli O157: H7 to alfalfa sprouts. Appl. Environ. Microbiol. 68, 4758–4763. doi: 10.1128/AEM.68.10.4758-4763.2002
Beckers, G. J., and Conrath, U. (2007). Priming for stress resistance: from the lab to the field. Curr. Opin. Plant Biol. 10, 425–431. doi: 10.1016/j.pbi.2007.06.002
Bernstein, N. (2013). “Effects of salinity on root growth,” In Plant Roots: The Hidden Half, 4th Edn, eds A. Eshel, and T. Beeckman (Boca Raton, FL: CRC Press), 848. doi: 10.1201/b14550-42
Bernstein, N., Chaimovitch, D., and Dudai, N. (2009a). Effect of irrigation with secondary treated effluent on essential oil, antioxidant activity, and phenolic compounds in oregano and rosemary. Agron. J. 101, 1–10. doi: 10.2134/agronj2007.0144
Bernstein, N., Kravchik, M., and Dudai, N. (2009b). Salinity-induced changes in essential oil, pigments and salts accumulation in sweet basil (Ocimum basilicum), in relation to alterations of morphological development. Ann. Appl. Biol. 156, 167–177. doi: 10.1111/j.1744-7348.2009.00376.x
Bernstein, N., Guetsky, R., Friedman, H., Bar-Tal, A., and Rot, I. (2008). Monitoring bacterial populations in agricultural greenhouse production system irrigated with reclaimed wastewater. J. Hortic. Sci. Biotechnol. 83, 821–827. doi: 10.1080/14620316.2008.11512467
Bernstein, N., and Kafkafi, U. (2002). “Root growth under salinity stress,” in Plant Roots: The Hidden Half 3rd Edn, eds Y. Waisel, A. Eshel, and U. Kafkafi (Boca Raton, FL: CRC Press), 787–805.
Bernstein, N., Sela, S., and Neder-Lavon, S. (2007a). Effect of irrigation regimes on persistence of Salmonella enterica serovar Newport in small experimental pots designed for plant cultivation. Irrig. Sci. 26, 1–8. doi: 10.1007/s00271-006-0059-3
Bernstein, N., Sela, S., and Neder-Lavon, S. (2007b). Assessment of contamination potential of lettuce by Salmonella enterica serovar Newport added to the plant growing medium. J. Food Prot. 70, 1717–1722.
Bernstein, N., Shoresh, M., Xu Y., and Huang B. (2010). Involvement of the plant antioxidative response in the differential growth sensitivity to salinity of leaves vs. roots during cell development. Free Radic. Biol. Med. 49, 1161–1171. doi: 10.1016/j.freeradbiomed.2010.06.032
Blodgett, R. (2010). Most Probable Number From Serial Dilutions. Bacteriological Analytical Manual, Appendix 2. Available at: http://www.fda.gov/Food/ScienceResearch/LaboratoryMethods/BacteriologicalAnalyticalManualBAM/ucm109656.htm#tab5
Boller, T., and Felix, G. (2009). A renaissance of elicitors: perception of microbe-associated molecular patterns and danger signals by pattern-recognition receptors. Annu. Rev. Plant Biol. 60, 379–406. doi: 10.1146/annurev.arplant.57.032905.105346
Booth, I. R., and Higgins, C. F. (1990). Enteric bacteria and osmotic stress: intracellular potassium glutamate as a secondary signal of osmotic stress? FEMS Microbiol. Lett. 6, 239–246. doi: 10.1111/j.1574-6968.1990.tb04097.x
Borges, A. A., Jiménez-Arias, D., Expósito-Rodríguez, M., Sandalio, L. M., and Pérez, J. A. (2014). Priming crops against biotic and abiotic stresses: MSB as a tool for studying mechanisms. Front. Plant Sci. 5:642. doi: 10.3389/fpls.2014.00642
Brandl, M. T. (2006). Fitness of human enteric pathogens on plants and implications for food safety. Annu. Rev. Phytopathol. 44, 367–392. doi: 10.1146/annurev.phyto.44.070505.143359
Campbell, V. J., Mohle-Boetani, J., Reporter, R., Abbott, S., Farrar, J., Brandl, M. T., et al. (2001), An outbreak of Salmonella serotype Thompson associated with fresh cilantro. J. Infect. Dis. 183, 984–987.
Charkowski, A. O., Barak, J. D., Sarreal, C. Z., and Mandrell, R. E. (2002). Differences in growth of Salmonella enterica and Escherichia coli O157: H7 on alfalfa sprouts. Appl. Environ. Microbiol. 68, 3114–3120. doi: 10.1128/AEM.68.6.3114-3120.2002
Conrath, U., Pieterse, C. M. J., and Mauch-Mani, B. (2002). Priming in plant-pathogen interactions. Trends Plant Sci. 7, 210–216. doi: 10.1016/S1360-1385(02)02244-6
DiCaprio, E., Purgianto, A., and Lia, J. R. (2015). Effects of abiotic and biotic stresses on the internalization and dissemination of human norovirus surrogates in growing romaine lettuce. Appl. Environ. Microbiol. 81, 4791–4800. doi: 10.1128/AEM.00650-15
Dudai, N. (2005), “Factors affecting content and composition of essential oils in aromatic plants,” in Crops Growth, Quality and Biotechnology. Part III: Quality Management of Food Crops for Processing Technology, ed. R. Dris (Helsinki: WFL Publisher).
Dudai, N., Chaimovitsh, D., Reuveni, R., Ravid, R., Larkov, O., and Putievsky, E. (2002). “Breeding of sweet basil (Ocimum basilicum) resistant to Fusarium wilt caused by Fusarium oxysporum f. sp. Basilicum,” in Breeding Research of Aromatic and Medicinal Plants. eds C. B. Johnson, and C. Franz (New York, NY: The Haworth Press, Inc.), 45–51.
El-Fadl, I. A., Abd-Ella, M. K., and Hussein, E. H. (1990). Effect of irrigation by saline water on the growth and some principal compounds of peppermint and spearmint in two types of soil. J. Agric. Res. Tanta Univ. 16, 276–295.
Erickson, M. C. (2012). Internalization of fresh produce by foodborne pathogens. Annu. Rev. Food Sci. Technol. 3, 283–310. doi: 10.1146/annurev-food-022811-101211
Erickson, M. C., Webb, C. C., Diaz-Perez, J. C., Phatak, S. C., Silvoy, J. J., Davey, L., et al. (2010). Surface and internalized Escherichia coli O157:H7 on field-grown spinach and lettuce treated with spray-contaminated irrigation water. J. Food Prot. 73, 1023–1029. doi: 10.4315/0362-028X-73.6.1023
Fatica, M. K., and Schneider, K. R. (2011). Salmonella and produce: survival in the plant environment and implications in food safety. Virulence 2, 573–579. doi: 10.4161/viru.2.6.17880
Foyer, C. H., Rasool, B., Davey, J., and Hancock, R. D. (2016). Cross tolerance to biotic and abiotic stresses in plants: a focus on resistance to aphid infestation. J. Exp. Bot. 67, 2025–2037 doi: 10.1093/jxb/erw079
Franz, E., Visser, A. A., Van Diepeningen, A. D., Klerks, M. M., Termorshuizen, A. J., and van Bruggen, A. H. C. (2007). Quantification of contamination of lettuce by GFP-expressing Escherichia coli O157:H7 and Salmonella enterica serovar Typhimurium. Food Microbiol. 24, 106–112. doi: 10.1016/j.fm.2006.03.002
Friedman, M., Henika, P. R., and Mandrell, R. E. (2002). Bactericidal activities of plant essential oils and some of their isolated constituents against Campylobacter jejuni, Escherichia coli, Listeria monocytogenes, and Salmonella enterica. J. Food prot. 65, 1545–1560. doi: 10.4315/0362-028X-65.10.1545
Gerba, C. P., and Smith, J. E. (2005). Source of pathogenic microorganisms and their fate during land application of wastes. J. Environ. Qual. 34, 42–48.
Gerba, C. P., Wallis, C., and Melnick, J. L. (1975). Fate of wastewater bacteria and viruses in soil. J. Irrig. Drain. Eng. 101, 157–174.
Golberg, D., Kroupitski, Y., Belausov, E., Pinto, R., and Sela, S. (2011). Salmonella Typhimurium internalization is variable in leafy vegetables and fresh herbs. Int. J. Food Microbiol. 145, 250–257. doi: 10.1016/j.ijfoodmicro.2010.12.031
Gorbatsevich, E., Sela, S., Pinto, R., and Bernstein, N. (2013). Root internalization, transport and in-planta survival of Salmonella enterica serovar Newport in sweet-basil. Environ. Microbiol. Rep. 5, 151–159. doi: 10.1111/1758-2229.12008
Gu, G., Cevallos-Cevallos, J. M., Vallad, G. E., and van Bruggen, A. H. (2013). Organically managed soils reduce internal colonization of tomato plants by Salmonella enterica serovar Typhimurium. Phytopathology 103, 381–388. doi: 10.1094/PHYTO-04-12-0072-FI
Gu, G., Hu, J., Cevallos-Cevallos, J. M., Richardson, S. M., Bartz, J. A., and van Bruggen, A. H. (2011). Internal colonization of Salmonella enterica serovar Typhimurium in tomato plants. PLoS ONE 6:e27340. doi: 10.1371/journal.pone.0027340
Guo, X., van Iersel, M. W., Brackett, R., and Beuchat, L. R. (2002). Evidence of association of Salmonella with tomato plants grown hydroponically in inoculated nutrient solution. Appl. Environ. Microbiol. 68, 3639–3643. doi: 10.1128/AEM.68.7.3639-3643.2002
Gutierrez-Rodriguez, E., Gundersen, A. Sbodio, A. O., and Suslow, T. V. (2012). Variable agronomic practices, cultivar, strain source and initial contamination dose differentially affect survival of Escherichia coli on spinach. J. Appl. Microbiol. 112, 109–118. doi: 10.1111/j.1365-2672.2011.05184.x
Hasegawa, P. M., Bressan, R. A., Zhu, J. K., and Bohnert, H. J. (2000). Plant cellular and molecular responses to high salinity. Annu. Rev. Plant Phys. 51, 463–499. doi: 10.1146/annurev.arplant.51.1.463
Hirneisen, K. A., Sharma, N., and Kniel, K. E. (2012). Human enteric pathogen internalization by root uptake into food crops. Foodborne Pathog. Dis. 9, 396–405. doi: 10.1089/fpd.2011.1044
Horby, P. W., O’Brien, S. J., Adak, G. K., Graham, C., Hawker, J. I., Hunter, P., et al. (2003). A national outbreak of multi-resistant Salmonella enterica serovar Typhimurium definitive phage type (DT) 104 associated with consumption of lettuce. Epidemiol. Infect. 130, 169–178. doi: 10.1017/S0950268802008063
Inami, G. B., and Moler, S. E. (1999). Detection and isolation of Salmonella from naturally contaminated alfalfa seeds following an outbreak investigation. J. Food Prot. 62, 662–664. doi: 10.4315/0362-028X-62.6.662
Islam, M., Doyle, M. P., Phatak, S. C., Millner, P., and Jiang, X. (2004). Persistence of enterohemorrhagic Escherichia coli O157:H7 in soil and on leaf lettuce and parsley grown in fields treated with contaminated manure composts or irrigation water. J. Food Prot. 67, 1365–1370. doi: 10.4315/0362-028X-67.7.1365
Jablasone J., Warriner, K., and Griffiths, M. (2005). Interactions of Escherichia coli O157:H7, Salmonella typhimurium and Listeria monocytogenes plants cultivated in a gnotobiotic system. Int. J. Food Microbiol. 99, 7–18. doi: 10.1016/j.ijfoodmicro.2004.06.011
Jacobsen, C. S., and Bech, T. B. (2012). Soil survival of Salmonella and transfer to freshwater and fresh produce, Food Res. Int. 45, 557–566
Kang, M. S., Besser, T. E., Hancock, D. D., and Call, D. R. (2007). Multiple environmental stress tests show no common phenotypes shared among contemporary epidemic strains of Salmonella enterica. Appl. Environ. Microbiol. 73, 3101–3104. doi: 10.1128/AEM.02607-06
Kisluk, G., and Yaron, S. (2012). Presence and persistence of Salmonella enterica serotype Typhimurium in the phyllosphere and rhizosphere of spray-irrigated parsley. Appl. Environ. Microbiol. 78, 4030–4036. doi: 10.1128/AEM.00087-12
Klerks, M. M., Franz, E., Zijlstra, C., Van Gent-Pelzer, M., and van Bruggen, A. H. C. (2007a). Differential interaction of Salmonella enterica serovars with lettuce cultivars and plant–microbe factors influencing the colonization efficiency. ISME J. 1, 620–631.
Klerks, M. M., Van Gent-Pelzer, M., Franz, E., Zijlstra, C., and van Bruggen, A. H. C. (2007b). Physiological and molecular response of Lactuca sativa to colonization by Salmonella enterica serovar Dublin. Appl. Environ. Microbiol. 73, 4905–4914.
Kuc, J. (1995). Phytoalexins, stress metabolism, and disease resistance in plants. Annu. Rev. Phytopathol. 33, 275–297. doi: 10.1146/annurev.py.33.090195.001423
Liu, Y., Wang, C., Tyrell, G., and Li, X. F. (2010). Production of Shiga-like toxins in viable but nonculturable Escherichia coli O157:H7. Water Res. 44, 711–718 doi: 10.1016/j.watres.2009.10.005
Lugtenberg, B. J., Dekkers, L. C., and Bloemberg, G. V. (2001). Molecular determinants of rhizosphere colonization by Pseudomonas. Annu. Rev. Phytopathol. 39, 461–490. doi: 10.1146/annurev.phyto.39.1.461
Maleck, K., and Dietrich, R. A. (1999). Defense on multiple fronts: how do plants cope with diverse enemies? Trends Plant Sci. 4, 215–219.
Marin, J. A., Andreu P., Carrasco A., and Arbeloa A. (2010). “Determination of proline concentration, an abiotic stress marker, in root exudates of excised root cultures of fruit tree rootstocks under salt stress. Revue des Régions Arides –24 (2/2010),” in Proceedings of the 3rd Meeting International Aridoculture et Cultures Oasisennes, Zaragoza.
Markland, S. M., and Kniel, K. E. (2015). “Human pathogen-plant interactions: concerns for food safety,” in Advances in Botanical Research: Plant Microbe Interactions, Vol. 75, eds H. Bais, and J. Sherrier (Amsterdam: Elsevier), 75, 115–135 doi: 10.1016/bs.abr.2015.08.002
Meury, J., and Kohiyama, M. (1991). Role of heat shock protein DnaK in osmotic adaptation of Escherichia coli. J. Bacteriol. 173, 4404–4410. doi: 10.1128/jb.173.14.4404-4410.1991
Mootian, G., Wu, W. H., and Matthews, K. R. (2009). Transfer of Escherichia coli O157:H7 from soil, water, and manure contaminated with low numbers of the pathogen to lettuce plants. J. Food Prot. 72, 2308–2312. doi: 10.4315/0362-028X-72.11.2308
Munns, R., and Tester, M. (2008). Mechanisms of salinity tolerance. Annu. Rev. Plant Biol. 59, 651–681. doi: 10.1146/annurev.arplant.59.032607.092911
Neves-Piestun, B. G., and Bernstein, N. (2005). Salinity induced changes in the nutritional status of expanding cells may impact leaf growth inhibition in Maize. Funct. Plant Biol. 32, 141–152. doi: 10.1071/FP04113
Orlofsky, E., Bernstein, N., Sacks, M., Vonshak, A., Benami, M., and Kundu, A. (2016). Comparable levels of microbial contamination in soil and on tomato crops after drip irrigation with treated wastewater or potable water. Agric. Ecosyst. Environ. 215, 140–150. doi: 10.1016/j.agee.2015.08.008
Oussalah, M. S., Saucier, L. C., and Lacroix, M. (2006). Inhibitory effects of selected plant essential oils on the growth of four pathogenic bacteria: E. coli O157:H7, Salmonella Typhimurium, Staphylococcus aureus and Listeria monocytogenes. Food Control 18, 414–420. doi: 10.1016/j.foodcont.2005.11.009
Pakalniskiene, J., Falkenhorst, G., Lisby, M., Madsen, S. B., Olsen, K. E., Nielsen, E. M., et al. (2006). A foodborne outbreak of enterotoxigenic E.coli and Salmonella Anatum infection after a high-school dinner in Denmark, November 2004. Epidemiol. Infect. 6, 1–6. doi: 10.1017/S0950268808000484
Pezzoli, L., Elson, R., Little, C., Fisher, I., Yip, H., Peters T., et al. (2007). International outbreak of Salmonella Senftenberg. Euro Surveill. 12:E070614.3.
Prince, W. S., and Villarejo, M. R. (1990). Osmotic control of proU transcription is mediated through direct action of potassium glutamate on transcription complex. J. Biol. Chem. 265, 17673–17679.
Ramegowda, V., and Senthil-Kumar, M. (2015). The interactive effects of simultaneous biotic and abiotic stresses on plants: mechanistic understanding from drought and pathogen combination. J. Plant Physiol. 176, 47–54. doi: 10.1016/j.jplph.2014.11.008
Roberto, F. V., and Simon, J. E. (2000). Chemical characterization of basil (Ocimum spp.) found in the markets and used in traditional medicine in Brazil. Econ. Bot. 54, 207–216. doi: 10.1007/BF02907824
Santamaria, J., and Toranzos, G. A. (2003). Enteric pathogens and soil: a short review. Int. Microbiol. 6, 5–9.
Scheierling, S. A., Bartone, C. R., Mara, D. D., and Drechsel, P. (2011). Towards an agenda for improving wastewater use in agriculture. Water Int. 36, 420–440 doi: 10.1080/02508060.2011.594527
Schikora, A., Carreri, A., Charpentier, E., and Hirt, H. (2008). The dark side of the salad: Salmonella typhimurium overcomes the innate immune response of Arabidopsis thaliana and shows an endopathogenic lifestyle. PLoS ONE 3:e2279. doi: 10.1371/journal.pone.0002279
Shoresh, M., Spivak, M., and Bernstein, N. (2011). Involvement of calcium-mediated effects on ROS metabolism in the regulation of growth improvement under salinity. Free Radic. Biol. Med. 51, 1221–1234. doi: 10.1016/j.freeradbiomed.2011.03.036
Simon, J. E. (2000). Sweet Basil: A Production Guide. Cooperative Extension Service. West Lafayette, IN: Purdue University, 83, 554–560. doi: 10.1094/PHYTO-09-15-0204-RVW
Sivapalasingam, S., Friedman, C. R., Cohen, L., and Tauxe, R. V. (2004). Fresh produce: a growing cause of outbreaks of foodborne illness in the United States, 1973 through 1997. J. Food Prot. 67, 2342–2353. doi: 10.4315/0362-028X-67.10.2342
Solomon, E. B., Yaron, S., and Matthews, K. R. (2002). Transmission of Escherichia coli O157:H7 from contaminated manure and irrigation water to lettuce plant tissue and its subsequent internalization. Appl. Environ. Microbiol. 68, 397–400. doi: 10.1128/AEM.68.1.397-400.2002
Tarchoune, I., Baâtour, O., Harrathi, J., Hamdaoui, G., Lachaâl, M., Ouerghi, Z., et al. (2013). Effects of two sodium salts on fatty acid and essential oil composition of basil (Ocimum basilicum L.) leaves. Acta Physiol. Plant. 35, 2365. doi: 10.1007/s11738-013-1271-4
Teplitski, M., Barak, J. D., and Schneider, K. R. (2009). Human enteric pathogens in produce: un-answered ecological questions with direct implications for food safety. Curr. Opin. Biotechnol. 20, 166–171. doi: 10.1016/j.copbio.2009.03.002
Thompson, I. P., Bailey, M. J., Fenlon, J. S., Fermor, T. R., Lilley, A. K., Lynch, J. M., et al. (1993). Quantitative and qualitative seasonal changes in the microbial community from the phyllosphere of sugar beet (Beta vulgaris). Plant Soil 150, 177–191. doi: 10.1007/BF00013015
Ton, J., van der Ent, S., van Hulten, M., Pozo, M., van Oosten, V., van Loon, L. C., et al. (2009). Priming as a mechanism behind induced resistance against pathogens, insects and abiotic stress. IOBC/wprs Bull. 44, 3–13.
Waller, F., Achatz, B., Baltruschat, H., Fodor, J., Becker, K., Fischer, M., et al. (2005). The endophytic fungus Piriformospora indica reprograms barley to salt-stress tolerance, disease resistance, and higher yield. Proc. Natl. Acad. Sci. U.S.A. 102, 13386–13391. doi: 10.1073/pnas.0504423102
Warriner, K., and Namvar, A. (2010). The tricks learnt by human enteric pathogens from phytopathogens to persist within the plant environment. Curr. Opin. Biotechnol. 21, 131–136. doi: 10.1016/j.copbio.2010.01.004
Wiedemann, A., Virlogeux-Payant, I., Chaussé, A. M., Schikora, A., and Velge, P. (2015). Interactions of Salmonella with animals and plants. Front. Microbiol. 5:791. doi: 10.3389/fmicb.2014.00791
World Health Organization [WHO] (2006). WHO Guidelines for The Safe Use of Wastewater, Excreta, and Greywater. Wastewater. (use)in Agriculture. Vol. 2 Geneva: WHO.
Yadav, R. K., Karamanoli, K., and Vokou, D. (2005). Bacterial colonization of the phyllosphere of Mediterranean perennial species as influenced by leaf structural and chemical features. Microb. Ecol. 50, 185–196. doi: 10.4315/0362-028X-72.12.2471
Keywords: contamination, basil, internalization, human pathogens, persistence, root, salt stress, Salmonella
Citation: Bernstein N, Sela (Saldinger) S, Dudai N and Gorbatsevich E (2017) Salinity Stress Does Not Affect Root Uptake, Dissemination and Persistence of Salmonella in Sweet-basil (Ocimum basilicum). Front. Plant Sci. 8:675. doi: 10.3389/fpls.2017.00675
Received: 29 December 2016; Accepted: 12 April 2017;
Published: 02 May 2017.
Edited by:
Puneet Singh Chauhan, National Botanical Research Institute (Council of Scientific and Industrial Research), IndiaReviewed by:
Anandham Rangasamy, Tamil Nadu Agricultural University, IndiaPraveen Rahi, National Centre for Cell Science, India
Copyright © 2017 Bernstein, Sela (Saldinger), Dudai and Gorbatsevich. This is an open-access article distributed under the terms of the Creative Commons Attribution License (CC BY). The use, distribution or reproduction in other forums is permitted, provided the original author(s) or licensor are credited and that the original publication in this journal is cited, in accordance with accepted academic practice. No use, distribution or reproduction is permitted which does not comply with these terms.
*Correspondence: Nirit Bernstein, bmlyaXRAdm9sY2FuaS5hZ3JpLmdvdi5pbA==