- Ranjan Plant Physiology and Biochemistry Laboratory, Department of Botany, University of Allahabad, Allahabad, India
In the present study, the role of sulfur (K2SO4: S; 60 mg S kg-1 sand) and/or calcium (CaCl2: Ca; 250 mg Ca kg-1 sand) applied alone as well as in combination on growth, photosynthetic performance, indices of chlorophyll a fluorescence, nitrogen metabolism, and protein and carbohydrate contents of Indian mustard (Brassica juncea L.) seedlings in the absence and presence of arsenic (Na2HAsO4.7H2O: As1; 15 mg As kg-1 sand and As2; 30 mg As kg-1 sand) stress was analyzed. Arsenic with its rising concentration negatively affected the fresh weight, root/shoot ratio, leaf area, photosynthetic pigments content, photosynthetic oxygen yield, and chlorophyll a fluorescence parameters: the O–J, J–I and I–P rise, QA- kinetic parameters, i.e., ΦP0, Ψ0, ΦE0, and PIABS, along with Fv/F0 and Area while increased the energy flux parameters, i.e., ABS/RC, TR0/RC, ET0/RC, and DI0/RC along with F0/Fv and Sm due to higher As/S and As/Ca ratio in test seedlings; however, exogenous application of S and Ca and their combined effect notably counteracted on As induced toxicity on growth and other important growth regulating processes. Moreover, inorganic nitrogen contents, i.e., nitrate (NO3-) and nitrite (NO2-) and the activities of nitrate assimilating enzymes, viz., nitrate reductase (NR) and nitrite reductase (NiR) and ammonia assimilating enzymes, viz., glutamine synthetase (GS) and glutamate synthase (GOGAT) along with protein and carbohydrate contents were severely affected with As toxicity; while under similar condition, ammonium (NH4+) content and glutamate dehydrogenase (GDH) activity in both root and leaves showed reverse trend. Furthermore, S and Ca supplementation alone and also in combination to As stressed seedlings ameliorated these parameters except NH4+ content and GDH activity, which showed an obvious reduction under similar conditions. These findings point out that exogenous application of S and/or Ca particularly S+Ca more favorably regulated the photosynthesis, contents of protein, carbohydrate and inorganic nitrogen, and the activities of nitrate and ammonia assimilating enzymes, which might be linked with the mitigation of As stress. Our results suggest that exogenous application of S+Ca more efficiently defends Brassica seedlings by declining As accumulation in root and shoot tissues and by maintaining the photosynthesis and nitrogen metabolism as well.
Introduction
By 2050, the target of raising 70% agricultural productivity to feed about 2.3 billion people is facing complex challenges due to biotic and abiotic stresses (Food and Agricultural Organization [FAO], 2009), which negatively affect crop productivity. In recent decades, arsenic (As) contamination is receiving attention especially in South-East Asia including India and Bangladesh (Singh et al., 2015), where the situation has become fatal as its lower doses causes skin cancer and at higher doses it may lead to the death (Kumar et al., 2015). Through its different sources (pyrite ores, pesticides, phosphate fertilizers, mining and smelting, semi-conductors, coal combustion, etc.), arsenic is frequently released into water bodies, which is used for the irrigation of crops and fodder and thereby it enters into food chain (Gupta and Ahmad, 2014). In Indian continent, the soil concentration of As ranges from 3.34 to 105 mg kg-1 on contaminated sites (Patel et al., 2005). It principally exists in two inorganic forms, i.e., Arsenate (AsV) and arsenite (AsIII); AsV enters in root cells through phosphate transporters and replaces phosphate in ATP metabolism, resulting into the interruption of energy flow in cells, while AsIII enters via aquaporin channels and hampers cellular functioning by interfering with sulfhydryl (–SH) group of enzymes, their cofactors, and proteins and causes cell death (Singh et al., 2016). Once after entrance in plant, As negatively affects plant growth, water and nutrients uptake, leaf gas exchange, chlorophyll biosynthesis, photosynthetic efficiency, chlorophyll a fluorescence, and ribulose 1,5-bisphosphate carboxylase/oxygenase (RuBisCo) activity, which in turn reduces the growth of plants (Stoeva and Bineva, 2003; Liu et al., 2007; Singh et al., 2013; Gupta and Ahmad, 2014). In addition, enhanced level of As also negatively affects nitrogen metabolism thereby affecting inorganic nitrogen contents and activities of nitrate and ammonia assimilating enzymes (Singh et al., 2009). The AsV most commonly induces reactive oxygen species (ROS) generation via conversion of AsV into AsIII that leads to electrons leakage from electron transport chain to O2, thereby causing a great loss to the integrity of cell membranes, DNA, lipids, and proteins, which are the backbone of plant cell functioning (Singh et al., 2013). To relieve As toxicity, plants have evolved antioxidant defense system (Liu et al., 2007; Singh et al., 2013; Mishra et al., 2016).
So, in order to cope up with the problems derived from As toxicity, different mineral nutrients have been frequently introduced by workers. In this chain, sulfur (S) and calcium (Ca) being an important signaling agent have been studied so far for their renowned role in plants. Sulfur is the major component of iron–sulfur (Fe–S) clusters, sulfo-lipids, vitamins (biotin and thiamine), glucosinolates, proteins, cysteine (Cys), glutathione (GSH), methionine, and thioredoxin system that regulate the physiological processes of plants (Khan and Khan, 2014). Besides, it also shares major role in build-up of photosynthetic apparatus and electron transport system and in coenzymes and prosthetic groups like ferredoxin that are vital for nitrogen assimilation (Marschner, 1995) and its deficiency directly interferes with the photosynthetic efficiency thereby influencing the activity of RuBisCo (Lunde et al., 2008). However, due to drastic reduction in the rate of S emission into atmosphere and excessive use of NPK fertilizers, only a trace amount of S is being added in the soil thereby leading to deficiency of S in soils. Therefore, sufficient amount of S is not only needed to maintain the status of plant metabolism but also in developing tolerance in plants against heavy metals in order to detoxify them (Liang et al., 2016).
Besides S, calcium (Ca) enriched growth condition could be another approach in reducing As toxicity of plants. Special attention has been paid to Ca, as it regulates cytoplasmic streaming, cell division and elongation, photomorphogenesis, and its protective role against environmental stresses (Kader and Lindberg, 2010). Additionally, Ca is a renowned secondary messenger and plays important role in cell metabolism and in the nutrients’ absorption across the cell membranes (Ahmad et al., 2015; Singh et al., 2018). The Ca has also proven to improve the photosynthetic electron transport, activities of key enzymes of Calvin cycle, and antioxidant capacity of stressed plants (You et al., 2002; Ahmad et al., 2015, 2016; Hochmal et al., 2015; Singh et al., 2018).
Indian mustard (Brassica juncea L.) of Brassicaceae family is ranked third among oil seed crop of world after soybean and palm. It is receiving attention due to its phytochemical content and medicinal properties. The residues of the plant are often used as cattle feed, biofuels, and fertilizer for the soil. The plant of B. juncea is an ideal system for evaluating metal toxicity as it is a well-known metal hyper-accumulator. Most of the studies regarding involvement of mineral nutrients in stress tolerance in plants were either dealing with S or Ca; however, no such study regarding their simultaneous effect (a prevailing condition of fields) on photosynthetic performance and status of nitrogen metabolism was reported. Therefore, present study was undertaken to evaluate the regulatory action of S and Ca individually and/or in combination in As-challenged B. juncea L. seedlings. Moreover, to get deeper insight of the present study, growth, As accumulation, photosynthetic pigments content, photosynthetic performance, and status of inorganic nitrogen contents and nitrogen assimilating enzymes were examined.
Materials and Methods
Plant Material and Growth Conditions
To carry out the present study, seeds of B. juncea L. were procured from local market branded as Green India Hybrid seeds Pvt. Ltd. Faizabad, Uttar Pradesh, India, and sterilized with sodium hypochlorite solution (2%, v/v) for 10 min. After that, seeds were repeatedly washed with distilled water following their wrapping in muslin cloth and kept in darkness for germination at 25 ± 2°C. After 48 h, germinated seeds were sown in plastic cups having 150 g acid washed sand and kept in darkness. After 48 h, seedlings were shifted and allowed to grow in plant growth chamber (CDR model GRW-300 DGe, Athens) having a daily cycle of 16:8 h light:dark photoperiod with photosynthetically active radiation (PAR): 150 μmol photons m-2 s-1 and relative humidity: 65–70% at 22 ± 2°C. On alternate days, seedlings were irrigated with 50% Hoagland solution (Hoagland and Arnon, 1950). Thereafter, 30-days-old seedlings were treated with As (Na2HAsO4.7H2O), S (K2SO4), and Ca (CaCl2) by dissolving the respected compounds in Hoagland nutrient solution. The experimental design consisted of: control (untreated seedlings), As1 (15 mg As kg-1 sand), As2 (30 mg As kg-1 sand), S (60 mg S kg-1 sand), As1+S, As2+S, Ca (250 mg Ca kg-1 sand), As1+Ca, As2+Ca, S+Ca, As1+S+Ca, and As2+S+Ca. It should be noted that the doses of As, S, and Ca used to carry out the experimental work in the present study were selected on the basis of screening experiments. Different parameters were analyzed after 7 days of treatment, on the root and shoot (stem+leaves)/leaves of test seedlings.
Growth Attributes
Growth was measured in terms of fresh weight (FW), root/shoot ratio, and leaf area of the seedlings. For the measurement of FW, untreated and treated seedlings were carefully uprooted, washed with distilled water (to remove the sand particles), dried with tissue paper, and then the weight was taken using single pan digital electronic balance (Model CA 223, Contech, India). The root/shoot ratio was calculated as: root FW/ shoot FW. Leaf area was measured using leaf area meter (Systronics, India).
Metal Accumulation
As, S, and Ca contents in root and shoot of test seedlings was assayed following the method of Allen et al. (1986).
Photosynthetic Pigments Content
The photosynthetic pigments content were assayed by the method of Lichtenthaler (1987) by recording the absorbance at 663, 646, and 470 nm in spectrophotometer (Shimadzu double beam UV–Visible spectrophotometer-1700, Japan).
Estimation of Photosynthesis and Respiration
Photosynthetic oxygen yield and respiratory oxygen uptake rates were performed in terms of oxygen evolution/consumption in leaf discs from untreated and treated samples in the presence and absence of light, respectively, by the method of Kurra-Hotta et al. (1987) using Clark type oxygen electrode (Digital Oxygen System, Model-10, Rank Brothers, United Kingdom).
Measurement of Chlorophyll a Fluorescence Transient
Polyphasic Chl a fluorescence transients in 30 min dark adapted leaves (for the complete oxidation of reaction centers) was carried out using leaf fluorometer (FluorPen FP 100, Photon System Instrument, Czechia) following the method of Strasser et al. (2000). The shape of J–I–P rise is associated with the complexity of reduction kinetic of PS II acceptor side. The O–J agrees with the photochemical reduction of primary electron acceptor of PS II (QA), J–I corresponds to complete closure of PS II reaction center or QB quenching mechanism, and I–P is related to reduction of PQ pool and pool size of final PS I electron acceptor. The quantum yield of primary photochemistry (ΦP0 or Phi_P0), yield of electron transport per trapped exciton (Ψ0 or Psi_0), quantum yield of electron transport (ΦE0 or Phi_E0), performance index (PIABS) of PS II, the energy fluxes for absorption of photon per active reaction center (ABS/RC), trapped energy flux per active reaction center (TR0/RC), electron transport flux per active reaction center (ET0/RC), energy dissipation flux per active reaction center (DI0/RC), size and number of active reaction centers (Fv/F0), efficiency of water splitting complex (F0/Fv), energy necessary for the closure of all reaction centers (Sm), and the area above the fluorescence induction curve between F0 and Fm (Area) were analyzed.
Estimation of Inorganic Nitrogen Contents
Nitrate (NO3-), Nitrite (NO2-), and Ammonium (NH4+) Contents
The assay of nitrate content is based on nitration of salicylic acid in acidic conditions, following the method proposed by Cataldo et al. (1975). Absorbance of the solution was recorded at 410 nm and nitrate content in the solution was calculated with the help of calibration curve prepared by KNO3 and expressed in terms of μmol NO3- g-1 FW.
Nitrite content was assayed following the method of Snell and Snell (1949). The absorbance of the reaction mixture was recorded at 540 nm and the nitrite content in the solution was calculated with the help of standard curve prepared by KNO2 and expressed in terms of μmol NO2- g-1 FW.
Ammonium content was assayed following the method of Molins-Legua et al. (2006) using the Nessler reagent. The absorbance of the reaction mixture was recorded at 425 nm and ammonium content was calculated with the help of calibration curve prepared by NH4Cl and expressed as μmol NH4+ g-1 FW.
Estimation of Nitrogen Assimilating Enzymes
Nitrate Reductase (NR) and Nitrite Reductase (NiR) Activities
The assay of nitrate reductase (NR; EC 1.6.6.1) activity was based on the total nitrite formed by the method of Robin (1979). The absorbance of reaction mixture was recorded at 540 nm, and the content of NO2- formed was calculated using a standard calibration curve prepared by NaNO2 and was expressed in terms of μmol NO2- formed g-1 FW h-1.
The assay of nitrite reductase (NiR; EC 1.7.7.1) activity is based on the reduction in the content of nitrite in the reaction mixture as described by Debouba et al. (2006). The absorbance of reaction mixture was recorded at 540 nm and NiR activity was expressed in terms of μmol NO2- reduced g-1 FW h-1.
Estimation of Ammonium Assimilating Enzymes
GS-GOGAT Pathway
Glutamine synthetase (GS) and glutamate synthase (GOGAT) activities
Glutamine synthetase (GS; EC 6.3.1.2) activity was assayed by the method of Lillo (1984). The absorbance of the reaction mixture was monitored spectrophotometrically at 540 nm and the enzyme activity was expressed in terms of A540 nm g-1 FW h-1.
Glutamine-2-oxoglutarate aminotransferase or glutamate synthase (NADH-GOGAT; EC 1.4.1.14) activity was assayed following the method of Singh and Srivastava (1986). The decrease in absorbance of the reaction mixture was recorded spectrophotometrically at 340 nm for 5 min and the activity of GOGAT was calculated by standard curve prepared with NADH and expressed in terms of nmol NADH oxidized g-1 FW h-1.
Alternate (NADH-GDH) Pathway
Glutamate dehydrogenase (GDH) activity
The aminating activity of glutamate dehydrogenase (GDH; EC 1.4.1.2) was assayed following the method of Singh and Srivastava (1983). The reaction was initiated by adding the substrate ammonium sulfate in the reaction mixture and decrease in absorbance was read at 340 nm for 5 min. The activity of GDH enzyme was calculated by the standard curve prepared with NADH and expressed in terms of nmol NADH oxidized g-1 FW h-1.
Estimation of Contents of Protein and Carbohydrate
The contents of protein and carbohydrate in root and leaf tissues were measured spectrophotometrically at 595 and 490 nm, following the procedure of Bradford (1976) and Dubois et al. (1956), respectively.
Statistical Analysis
Results were statistically analyzed by analysis of variance (ANOVA). Tukey test was applied for the mean separation for significant differences among treatments at P < 0.05 levels. The results presented are the means of three replicates (n = 3) to check the reproducibility of the results.
Results
Growth Attributes
The impact of S, Ca, and S+Ca on As stressed Brassica seedlings was examined by analyzing the FW of the plant, root/shoot ratio, and leaf area, which have been portrayed in Figure 1. Both the doses of As, i.e., As1 and As2, significantly (P < 0.05) declined the FW by 16 and 37%, root/shoot ratio by 9 and 24%, and leaf area by 15 and 34%, respectively, over the values of control. Contrastingly, exogenous application of S and Ca alone and in combination (S+Ca) markedly ameliorated the negative effect of As on above parameters. The S+Ca application completely recovered the negative effect of As under As1 stress on FW and root/shoot ratio; while only 9 and 4% inhibition, respectively, under As2 stress in comparison to As2 stress alone was observed except for leaf area where the negative effect of both the doses i.e., As1 and As2 was totally recovered.
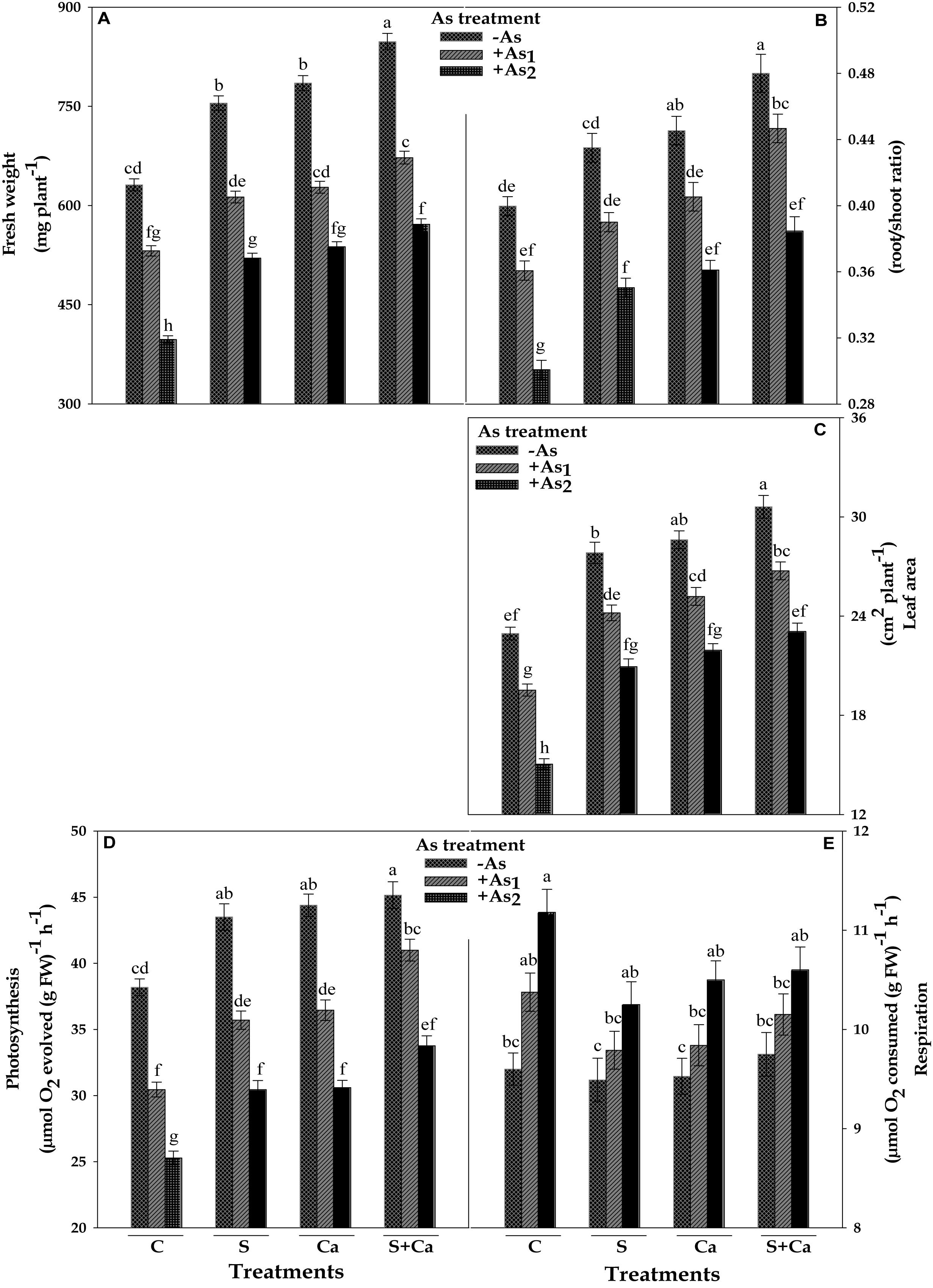
FIGURE 1. Impact of exogenous application of S and Ca individually and in combination on fresh weight (A), root/shoot ratio (B), leaf area (C), photosynthetic O2 evolution (D), and respiratory rate (E) of Brassica juncea L. seedlings exposed to As1 (15 mg arsenic kg-1 sand) and As2 (30 mg arsenic kg-1 sand) stress. Data represent the mean value ± standard error of three replicates (n = 3). Bars followed by different letters show significant difference according to Tukey test at P < 0.05 significance level.
Metal Accumulation
The results pertaining to As, S, and Ca accumulation were measured in terms of As/S and As/Ca ratio in root and shoot (stem+leaves) and have been portrayed in Table 1. Arsenic at both the doses, i.e., As1 and As2, sharply enhanced the ratio of As/S from 0.080 to 0.146 and As/Ca from 0.103 to 0.195 in roots, respectively, over the values of control. Corresponding increase in the ratio of As/S in shoots was from 0.053 to 0.081 and in case of As/Ca, it was enhanced from 0.048 to 0.076. However, upon addition of S and Ca either alone or in combination to As stressed Brassica seedlings, a significant reduction in As/S and As/Ca ratio was noticed and the maximum reduction was found under S+Ca treatment.
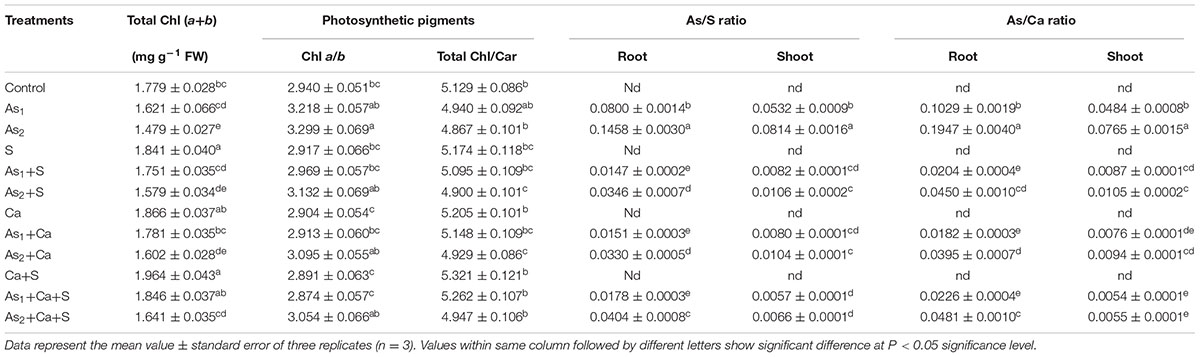
TABLE 1. Impact of exogenous application of S and Ca individually and in combination on photosynthetic pigment contents in leaves and As/S and As/Ca ratio in root and shoot of Brassica juncea L. seedlings exposed to As1 (15 mg arsenic kg-1 sand) and As2 (30 mg arsenic kg-1 sand) stress.
Photosynthetic Pigments
The results pertaining to photosynthetic pigments content were measured in terms of total chlorophyll (Chl a+b) content and the ratios of Chl a/b and total Chl/Car which have been depicted in Table 1. Results revealed that both the doses of As, i.e., As1 and As2 caused significant (P < 0.05) damage showing a decrease of 9 and 17% in total Chl content and 4 and 5% in Chl/Car ratio; while a significant increase of 9 and 12% in Chl a/b ratio, respectively, over the value of respective control was noticed. Exogenous supplementation of Ca and S+Ca completely recovered the loss to Chl content and also Chl/Car ratio in As1 stressed seedlings while with S supplementation still a marginal decrease was noticed. Under As2 stress though the supplementation of S, Ca, and S+Ca resulted into significant improvement in the pigments content, the seedlings still showed a decrease of 11, 10, and 8% in total Chl content and 4, 4, and 3% in Chl/Car ratio, respectively. Furthermore, the increased value of Chl a/b due to As1 and As2 treatment decreased significantly with the supplementation of S, Ca, and S+Ca and this effect was more prominent with combined treatment of S and Ca.
Photosynthesis and Respiration
The results pertaining to photosynthetic oxygen evolution and respiratory oxygen uptake have been framed in Figure 1. The results showed that As1 and As2 doses declined the photosynthetic oxygen evolution rate by 20 and 34%; contrastingly, the respiratory oxygen uptake rate was enhanced by 8 and 16%, respectively, over the values of respective control. However, exogenous application of S, Ca, and S+Ca significantly ameliorated the damaging effect on photosynthetic oxygen evolution rate with more pronounced effect of S+Ca treatment under As1 stress which showed a complete amelioration, while only 12% inhibition was noticed under As2 treatment. Contrastingly, under As stress, S, Ca, and S+Ca supplementation resulted into significant decrease in respiratory rate; however, the values were still higher than that of untreated control.
Chlorophyll a Fluorescence Transient
The effects of tested doses of As on Chl a fluorescence characteristics in Brassica seedlings exposed to S, Ca, and S+Ca were measured by OJIP test and the results have been portrayed in Figures 2, 3. A characteristic OJIP transient rise was noticed. While a sharp decrease in O–J, J–I, and I–P rise was recorded under As1 and As2 treatments (Figure 2). Upon exogenous application of S, Ca, and S+Ca either alone or to As treated Brassica seedlings, a significant amelioration in decrease of O–J, J–I, and I–P rise was observed.
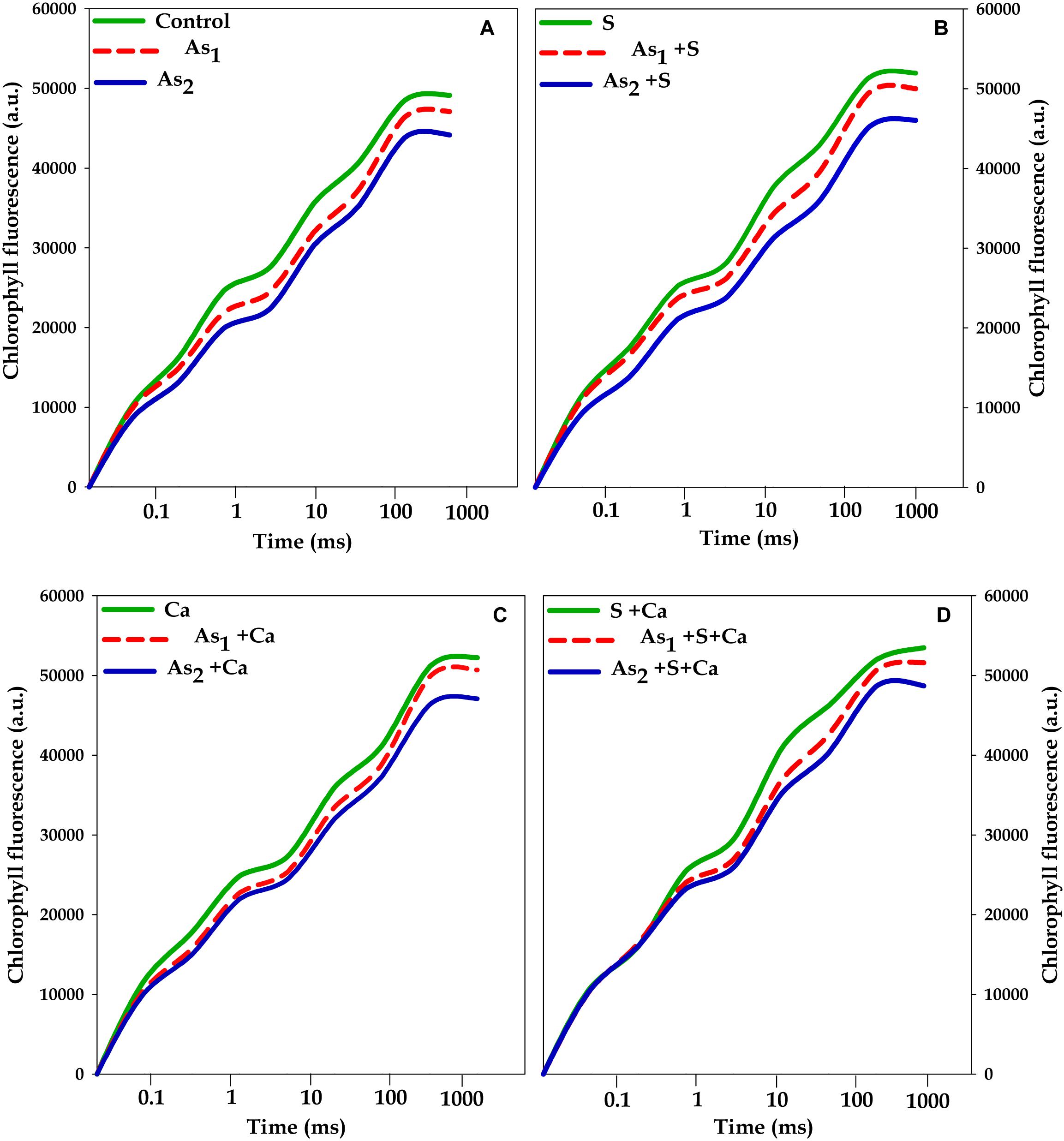
FIGURE 2. Impact of exogenous application of S (B) and Ca (C) individually and in combination (D) on OJIP transient of Brassica juncea L. seedlings exposed to As1 (15 mg arsenic kg-1 sand) and As2 (30 mg arsenic kg-1 sand) stress (A).
The ΦP0, Ψ0, ΦE0, PIABS, and Area of PS II sharply declined under As stress in dose dependent manner over the control values (Figure 3). On the other hand, exogenous application of S, Ca, and S+Ca to As treated test seedlings significantly alleviated the damaging effect and a marginal increase in ΦP0, Ψ0, ΦE0, PIABS, and Area was recorded. Arsenic treatment also decreased the size and number of active reaction centers (Fv/F0) in the photosynthetic apparatus that was symbolized by increased energy fluxes parameters, i.e., ABS/RC, TR0/RC, DI0/RC, and ET0/RC along with the increase in F0/Fv and Sm values. However, S, Ca, and S+Ca significantly improved the proportion of size and number of active reaction centers thereby declining the values for energy flux parameters and F0/Fv and Sm when subjected to As stressed seedlings.
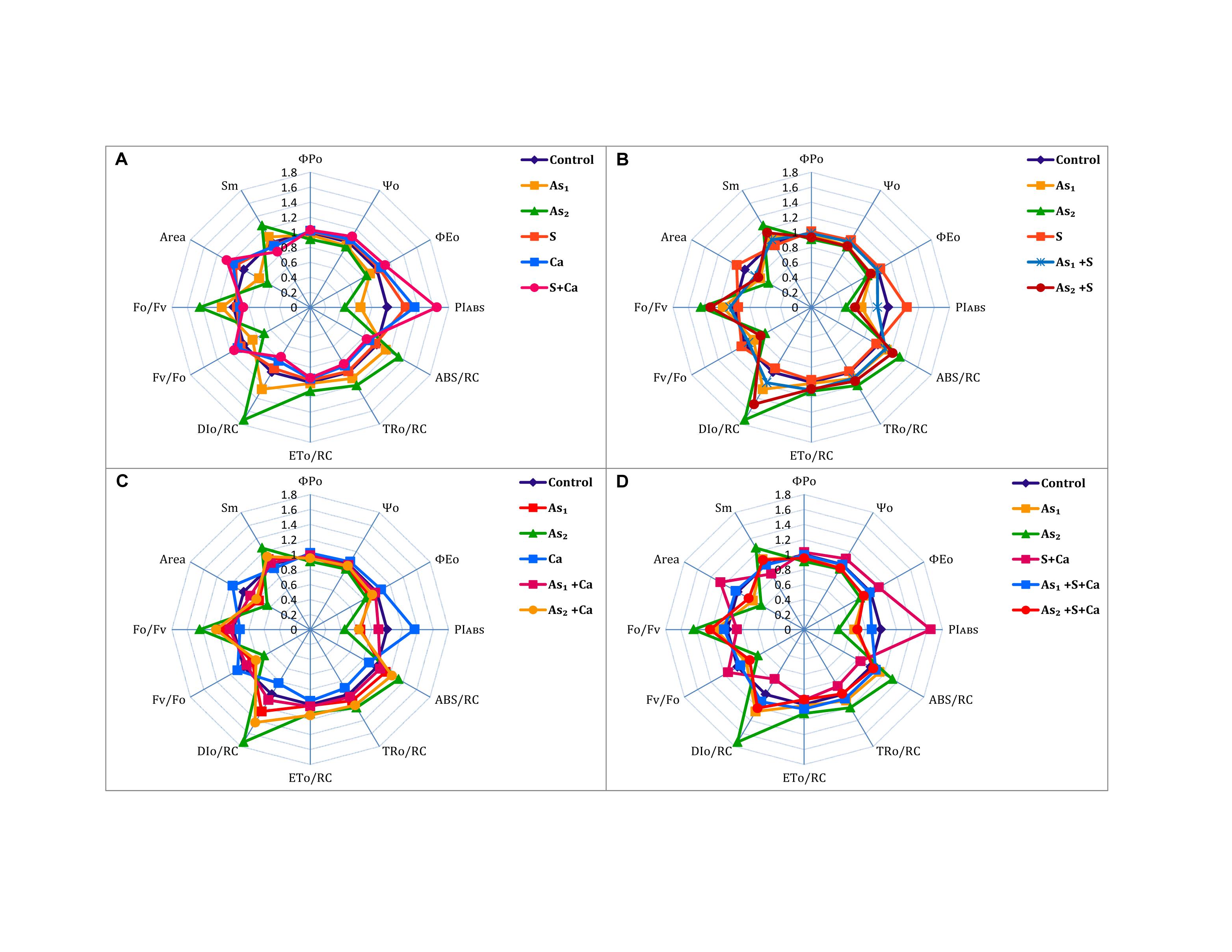
FIGURE 3. Impact of exogenous application of S (B) and Ca (C) individually and in combination (D) on chlorophyll fluorescence indices of Brassica juncea L. seedlings exposed to As1 (15 mg arsenic kg-1 sand) and As2 (30 mg arsenic kg-1 sand) stress (A).
Inorganic Nitrogen Contents
Results pertaining to inorganic nitrogen contents, i.e., NO3-, NO2-, and NH4+ and NH4+/NO3- ratio in test seedlings have been portrayed in Figure 4. The results suggested that As1 and As2 doses markedly reduced the contents of NO3- by 22 and 39%, NO2- by 8 and 20% in roots and corresponding decrease in the contents of NO3- it was 12 and 28% and of NO2- it was 6 and 16% of NO2- it was in leaves, respectively, with respect to control. Contrastingly, NH4+ content was increased by 12 and 28% in root and 7 and 18% in leaves, respectively, under As1 and As2 stress; therefore, a sharp increase in NH4+/NO3- ratio in both root and leaves was noticed. When As1 and As2 stressed seedlings were subjected to S, Ca, and S+Ca treatment, the levels of NO3- and NO2- were found to improve in both root and leaves and under S+Ca treatment the levels of NO3- and NO2- in As1 treated seedlings were found to be even more than the level of control sample (Figure 4). Reverse to this, As induced increment in NH4+ accumulation in root and leaves exhibited a declining trend following supplementation with S, Ca, and S+Ca; hence, a substantial decrease in NH4+/NO3- ratio was noticed under individual as well as combined supplementation with S and Ca even in As stressed seedlings.
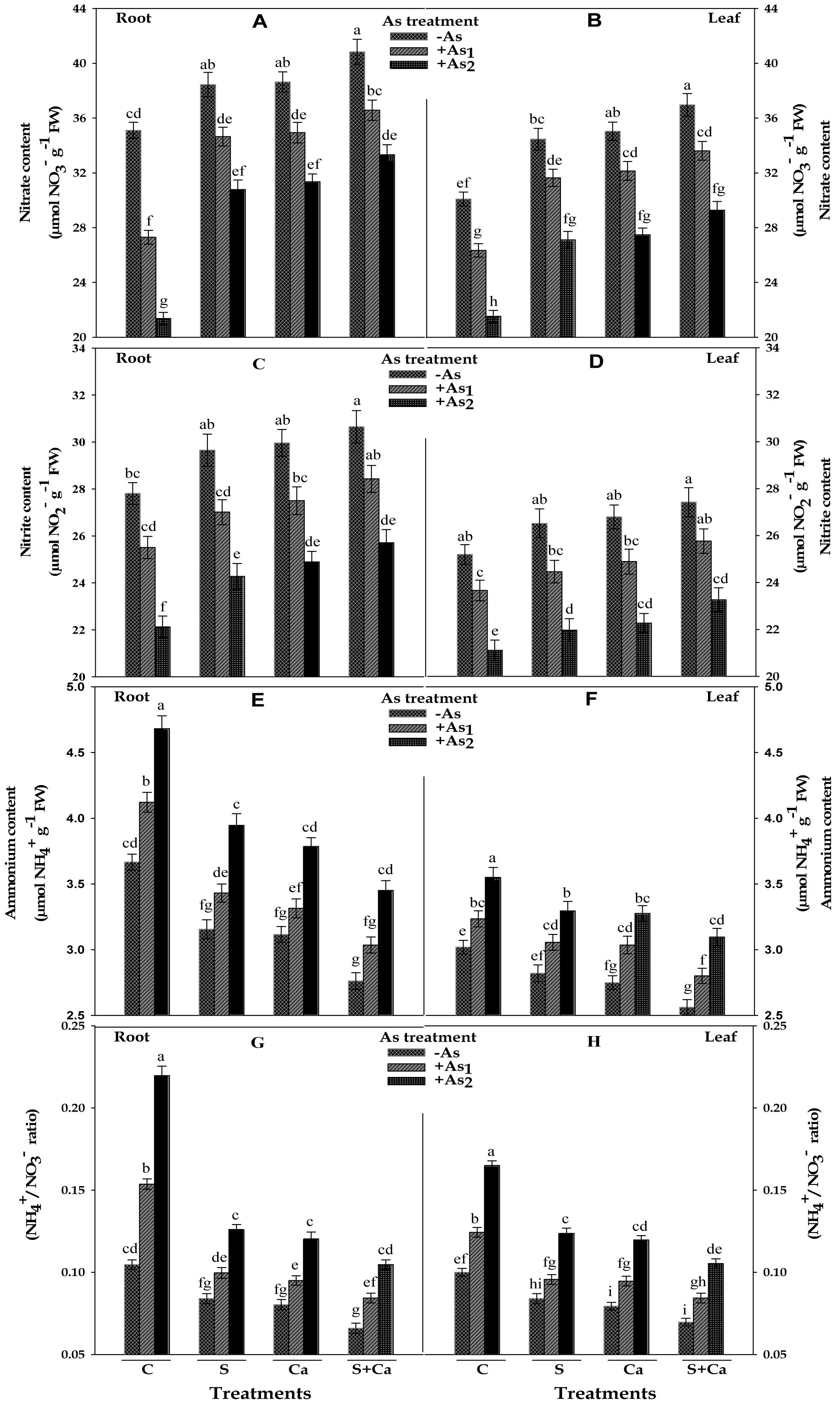
FIGURE 4. Impact of exogenous application of S and Ca individually and in combination on inorganic nitrogen contents: nitrate (A,B), nitrite (C,D), and ammonium (E,F) and NH4+/NO3- ratio (G,H) in root and leaves of Brassica juncea L. seedlings exposed to As1 (15 mg arsenic kg-1 sand) and As2 (30 mg arsenic kg-1 sand) stress. Data represent the mean value ± standard error of three replicates (n = 3). Bars followed by different letters show significant difference according to Tukey test at P < 0.05 significance level.
Activities of Nitrate Assimilating Enzymes
The results related with activities of NR (NRA) and NiR (NiRA) and the ratio of NiRA/NRA in test seedlings have been portrayed in Figure 5. Under As1 and As2 stress, the activity of NR was decreased by 25 and 50% and NiR by 19 and 44% in root and corresponding decrease in the activities of NR was 20 and 38% and in NiR it was 15 and 27% in leaves, respectively, over their control values. Furthermore, NiRA/NRA ratio to root as well as in leaves was found to increase with As treatment which was maximum under As2 stress. Upon S, Ca, and S+Ca supplementation, the negative effect on NR and NiR activities caused by As1 and As2 stress was alleviated; thus, a significant decline in NiRA/NRA ratio in both root and leaves was observed (Figures 5A–F). Moreover, S+Ca treatment to As stressed seedlings was found to be more effective in alleviating the negative effect on NR and NiR activities.
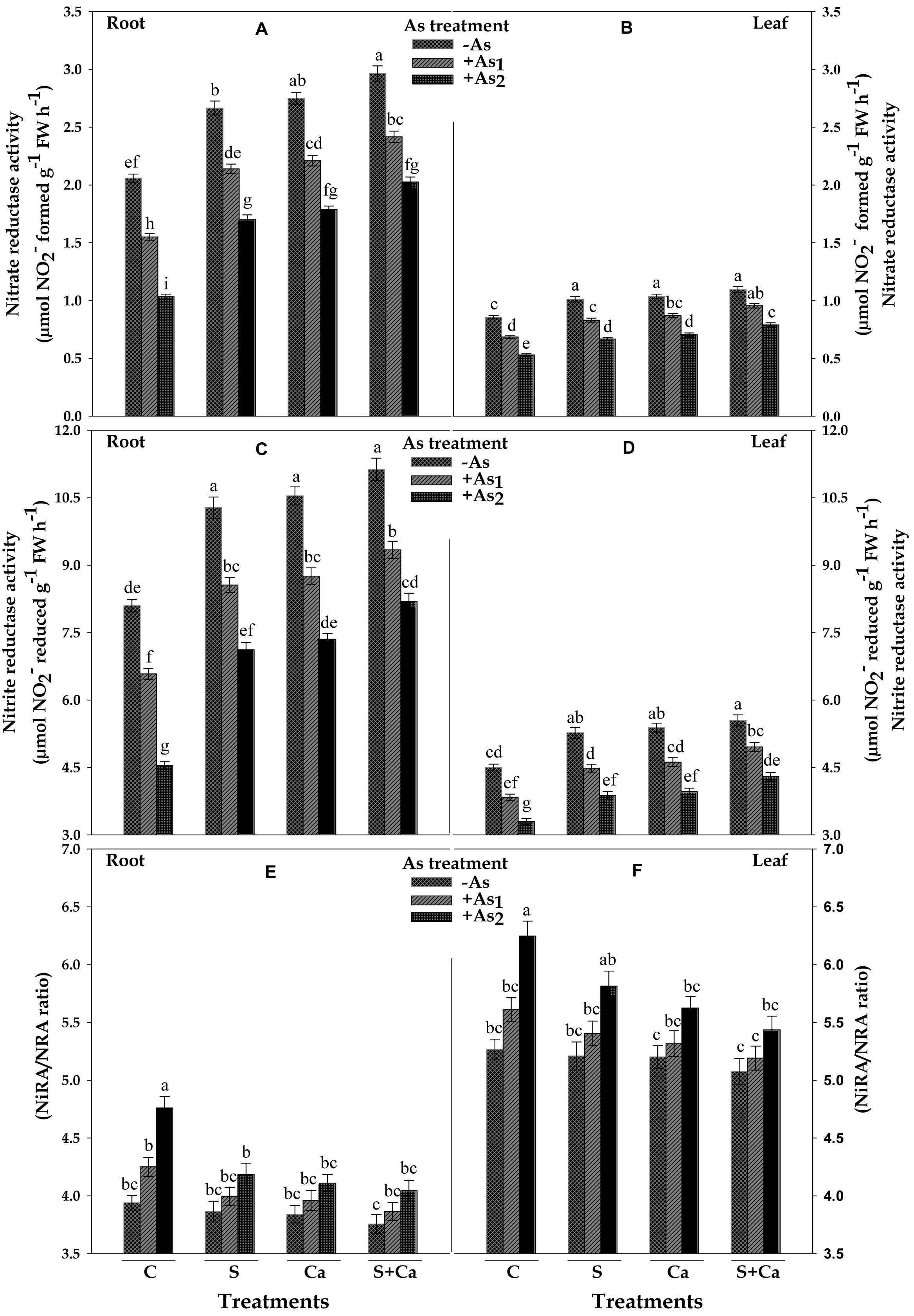
FIGURE 5. Impact of exogenous application of S and Ca individually and in combination on activities of nitrate assimilating enzymes: nitrate reductase (NRA) (A,B) and nitrite reductase (NiRA) (C,D) and NiRA/NRA ratio (E,F) in root and leaves of Brassica juncea L. seedlings exposed to As1 (15 mg arsenic kg-1 sand) and As2 (30 mg arsenic kg-1 sand) stress. Data represent the mean value ± standard error of three replicates (n = 3). Bars followed by different letters show significant difference according to Tukey test at P < 0.05 significance level.
Activities of Ammonium Assimilating Enzymes
The results showing the effect of As on activities of ammonium assimilating enzymes, i.e., GS, GOGAT, and GDH of test plant have been depicted in Figure 6. The results revealed that As1 and As2 treatment decreased the GS activity by 38 and 52% and GOGAT by 21 and 40% in root and corresponding decrease in the GS activity was 19 and 39% and in GOGAT it was 16 and 34% in leaves, respectively, as compared to control. When As1 and As2 treated seedlings were supplied with S, Ca, and S+Ca, significant improvement in the GS and GOGAT activities of root and leaves of test plant was noticed and was maximum under S+Ca supplementation. In contrast to this, GDH activity under As stress exhibited appreciable enhancement showing a rise of 110 and 142% in root and 106 and 131% in leaves and the activity was further accelerated upon S+Ca application as it was raised by 217 and 235% in root and 209 and 224% in leaves in As1 and As2 stressed test seedlings, respectively, over the values of control.
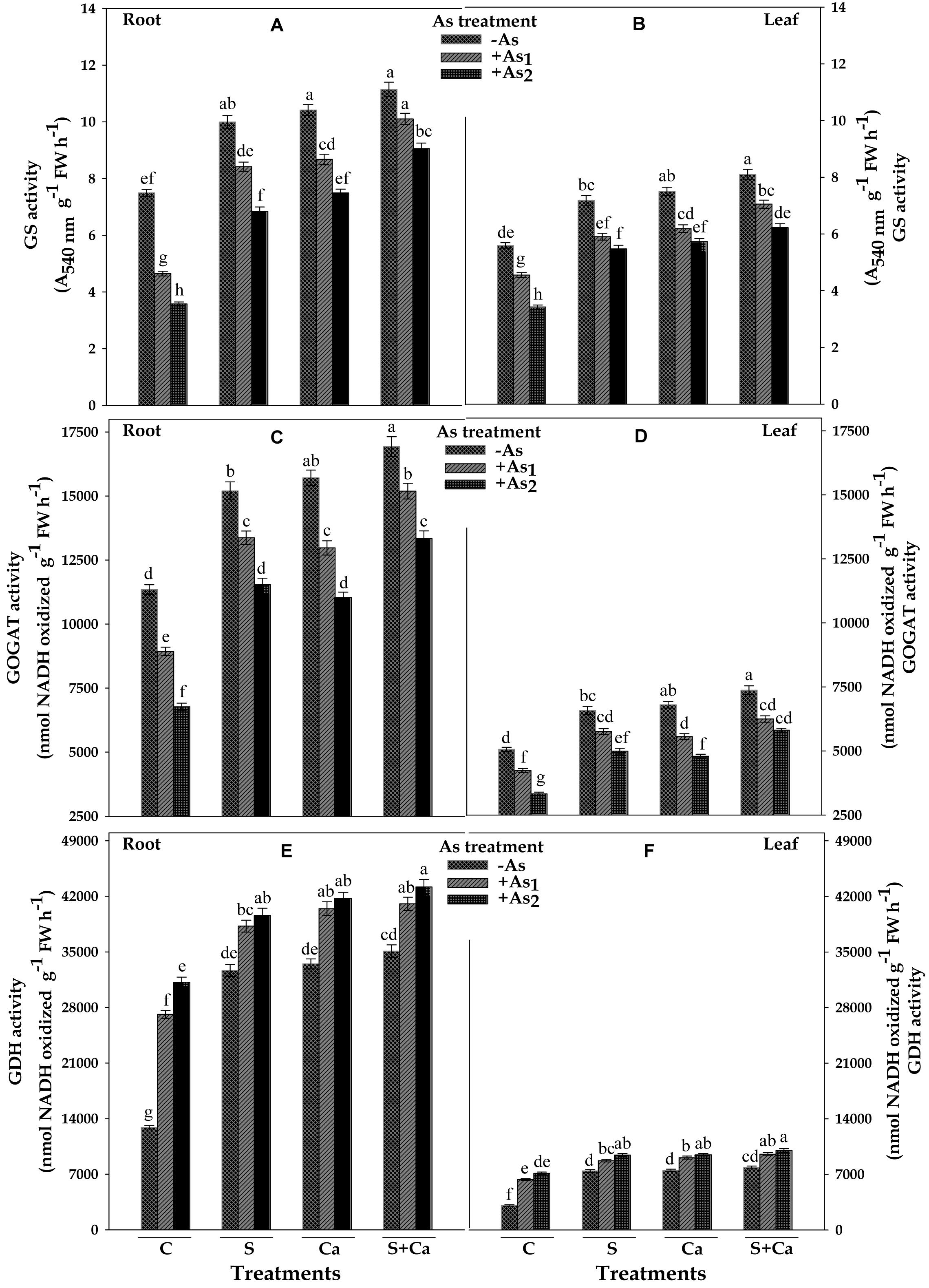
FIGURE 6. Impact of exogenous application of S and Ca individually and in combination on activities of ammonium assimilating enzymes: glutamine synthetase (GS) (A,B), glutamate synthase (GOGAT) (C,D), and glutamate dehydrogenase (GDH) (E,F) in root and leaves of Brassica juncea L. seedlings exposed to As1 (15 mg arsenic kg-1 sand) and As2 (30 mg arsenic kg-1 sand) stress. Data represent the mean value ± standard error of three replicates (n = 3). Bars followed by different letters show significant difference according to Tukey test at P < 0.05 significance level.
Protein and Carbohydrate Contents
Results pertaining to protein and carbohydrate contents in root and leaves of test seedlings have been portrayed in Figure 7. The results revealed that As1 and As2 doses of As, noticeably declined the content of protein by 16 and 33%, carbohydrate by 10 and 27% in root, and the corresponding decline in the content of protein was 15 and 27% and of carbohydrate it was 14 and 35% in leaves, respectively, in respect of control. However, exogenous application of S, Ca, and S+Ca to As1 and As2 stressed seedlings significantly ameliorated the negative effect of As on protein and carbohydrate contents and the effect was more pronounced in case of root as well as leaves under As1 stress (Figure 7).
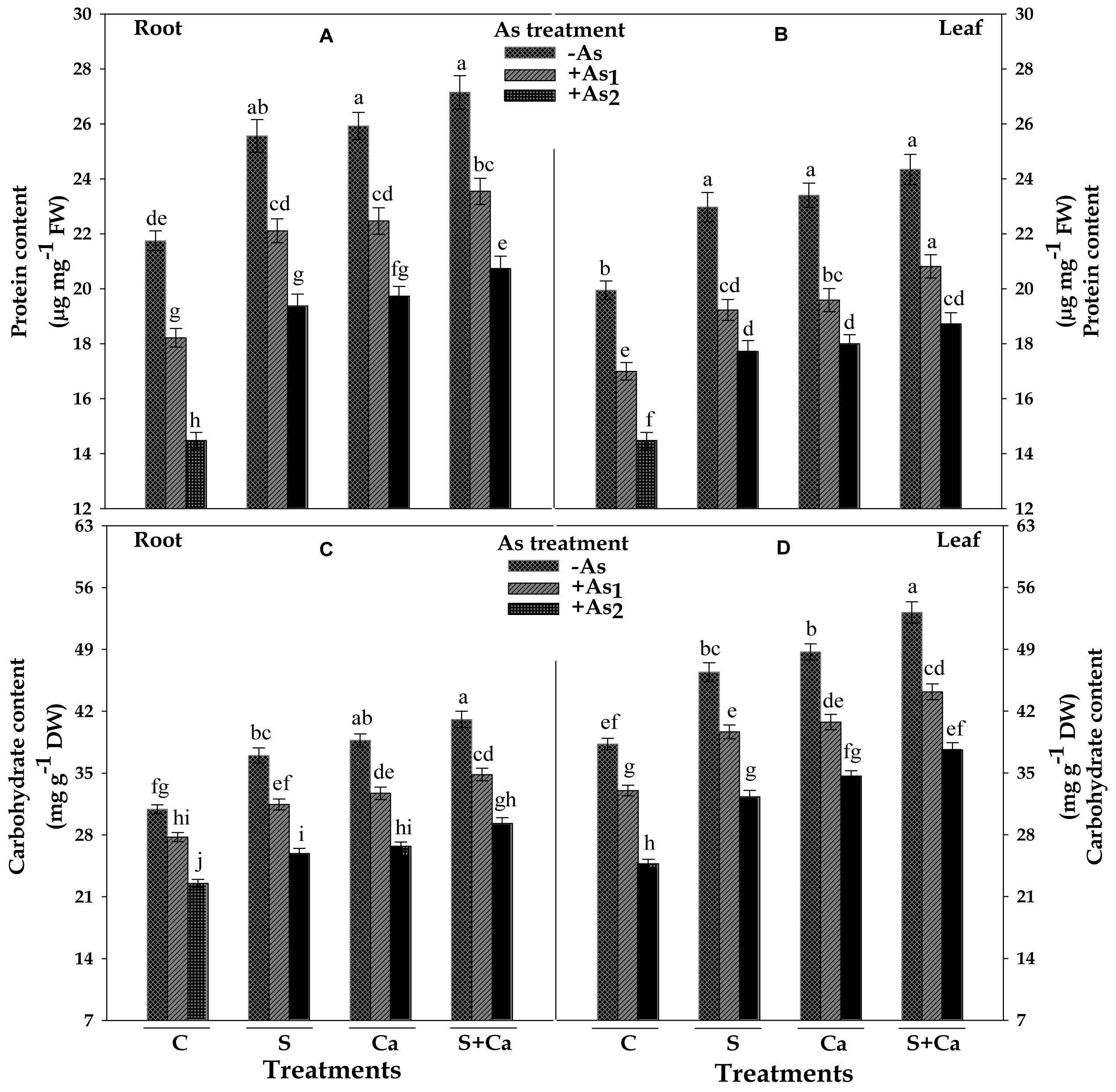
FIGURE 7. Impact of exogenous application of S and Ca individually and in combination on protein (A,B) and carbohydrate (C,D) contents in root and leaves of Brassica juncea L. seedlings exposed to As1 (15 mg arsenic kg-1 sand) and As2 (30 mg arsenic kg-1 sand) stress. Data represent the mean value ± standard error of three replicates (n = 3). Bars followed by different letters show significant difference according to Tukey test at P < 0.05 significance level.
Table 2 shows results for two-way ANOVA test for arsenic and mineral nutrients (S and Ca) on the studied parameters. Both the variables when subjected individually to the seedlings significantly affected all the studied parameters (except for the ratio for root to shoot). However, the interactive effect of both the variables was significant on FW, root/shoot, nitrate content, NH4+/NO3-, NiRA/NRA, and GS and GDH activities and due to their significant impact on nitrate content and important enzymes of ammonium assimilation, better growth was noticed in comparison to S and Ca alone.
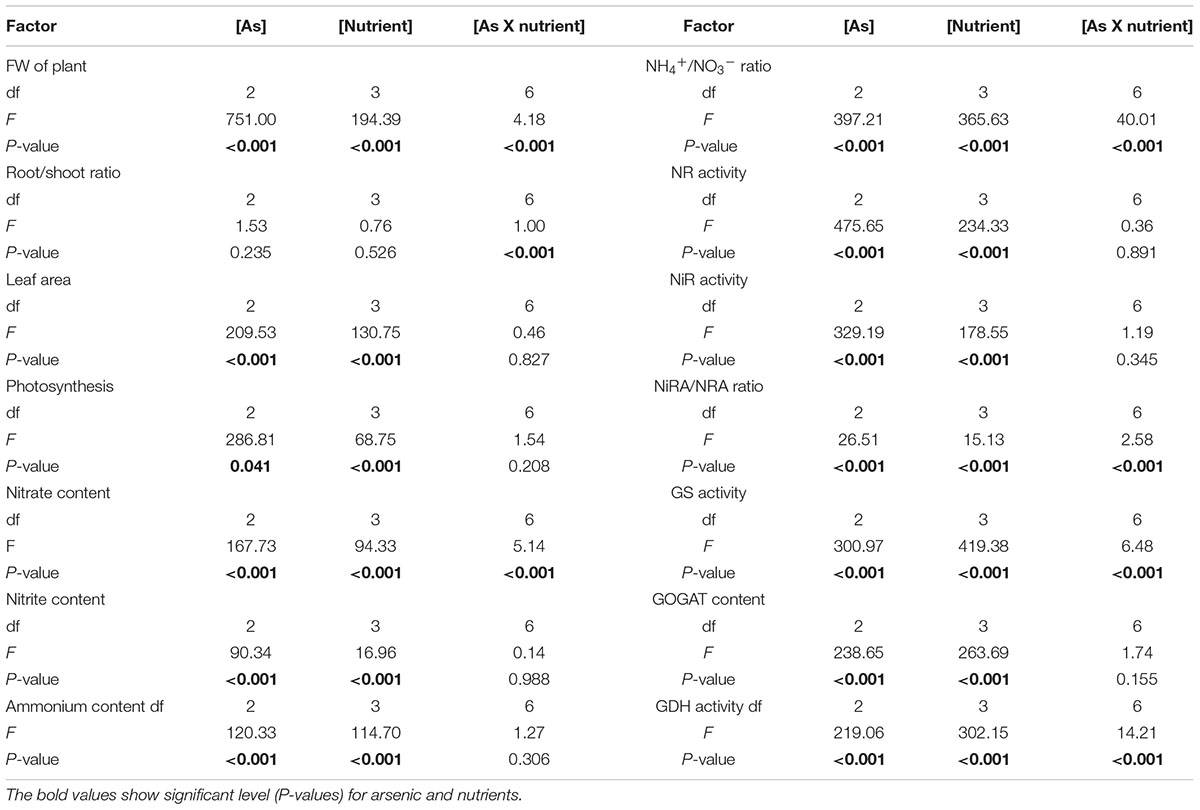
TABLE 2. Two-way ANOVA results for individual and interactive effect of arsenic and nutrients on the studied parameters in Brassica L. seedlings.
Discussion
The present study was designed to explicate the S and Ca induced recovery of C and N skeleton in terms of photosynthesis and nitrogen metabolism in As-challenged Brassica seedlings. From the results, it is clear that As exhibited toxicity to Brassica at both the doses by posing negative effect on growth attributes measured in terms of FW, root/shoot ratio, and leaf area (Figures 1A–C). The As induced reduction in root/shoot ratio could be a resultant of higher As accumulation in root as it is in direct contact of sand that severely damaged the roots thereby resulting into lower root/shoot ratio (Table 1). Further, the reduction in leaf area could be attributed to: (i) decrease in light harvesting components (Table 1) and consequently the photosynthetic rate (Figure 1), (ii) As induced overproduction of ROS (Singh et al., 2018), and (iii) decreased activities of nitrogen assimilating enzymes (Figures 5, 6). Arsenic accumulation in Brassica seedlings showed higher As/S and As/Ca ratio in root than shoots, indicating that greater amount of As was restricted in roots and less was translocated to shoot (Table 1). The higher As accumulation in roots could also be due to the damage and alteration in plasma membrane and cell wall structures as was suggested by Singh et al. (2013). On the other hand, application of S and Ca either alone or in combination regulated the As toxicity thereby improving the root/shoot ratio and leaf area in test plant, which probably could be due to: (i) upregulation in the uptake and translocation of water and mineral nutrients [Mn, K, Mg, Fe, Cu, Zn, and P including S and Ca (data not shown)], which are necessary as cofactors in different enzymes, chlorophyll biosynthesis, photosynthetic activity (Sebastian and Prasad, 2014), etc., (ii) by recovering membrane integrity that was lost due to As accumulation (Ahmad et al., 2015) due to which higher As/S and As/Ca ratio in roots was observed (Table 1), (iii) by favoring the Ca mediated cell elongation and expansion (Ahmad et al., 2016), and (iv) by improving the activities of nitrogen and ammonium assimilating enzymes (Figures 5, 6). When As stressed seedlings were treated with exogenous S, Ca, and S+Ca, a decrease in As/S and As/Ca ratio was observed in both root and shoot, which was more pronounced in roots than shoot thereby signifying that more S and Ca were maintained in roots that might have played an important role in the formation of thiol containing compounds and in free radical scavenging (Price et al., 1994; Dixit et al., 2015) to detoxify greater amount of As and ROS induced by As in roots.
Being essential components of photosynthesis, photosynthetic pigments (total chlorophyll, i.e., Chl a+b; Chl a/b and Chl/Car) were also analyzed and found to be severely damaged when exposed to As stress in dose-dependent manner (Table 1 and Figure 8). This As induced damaging effect on photosynthetic pigments could be due to: (i) the competition as well as replacement of inorganic phosphate (iP), needed for Chl biosynthesis (Mishra et al., 2016), (ii) repression of the activities of enzymes (require –SH) needed for pigment biosynthesis (Singh et al., 2013), (iii) degradation of chlorophyll biosynthesis precursors (Mishra et al., 2016), and (iv) oxidation of photochemical apparatus that damages photosynthetic pigments (Stoeva et al., 2005). Our findings show conformity with the studies of Singh et al. (2016) and Mishra et al. (2016) in As-challenged Oryza sativa and Ceratophyllum demersum, respectively. Arsenic also leads to chlorosis by upregulating the conversion of Chl b into Chl a; hence, relatively higher loss in Chl b content causes higher Chl a/b ratio, which is the symptom of inadequacy of peripheral antenna system. Contrastingly, Chl/Car ratio was decreased with increasing concentration of As (Table 1) thereby implying a great loss in Car content that might have occurred due to the damage to thylakoid membrane, which is in consonance with the findings of Stoeva et al. (2005). However, exogenous S and Ca improved the Chl biosynthesis by improving the uptake of Mn2+, Zn2+, Fe2+, and Mg2+ (data not shown) and also improving the Car content. Calcium induced improvement in Chl synthesis could be due to its role as secondary messenger in cytokinin action as cytokinin is involved in distribution of mineral nutrients within plants (Lechowski and Białczyk, 1993).
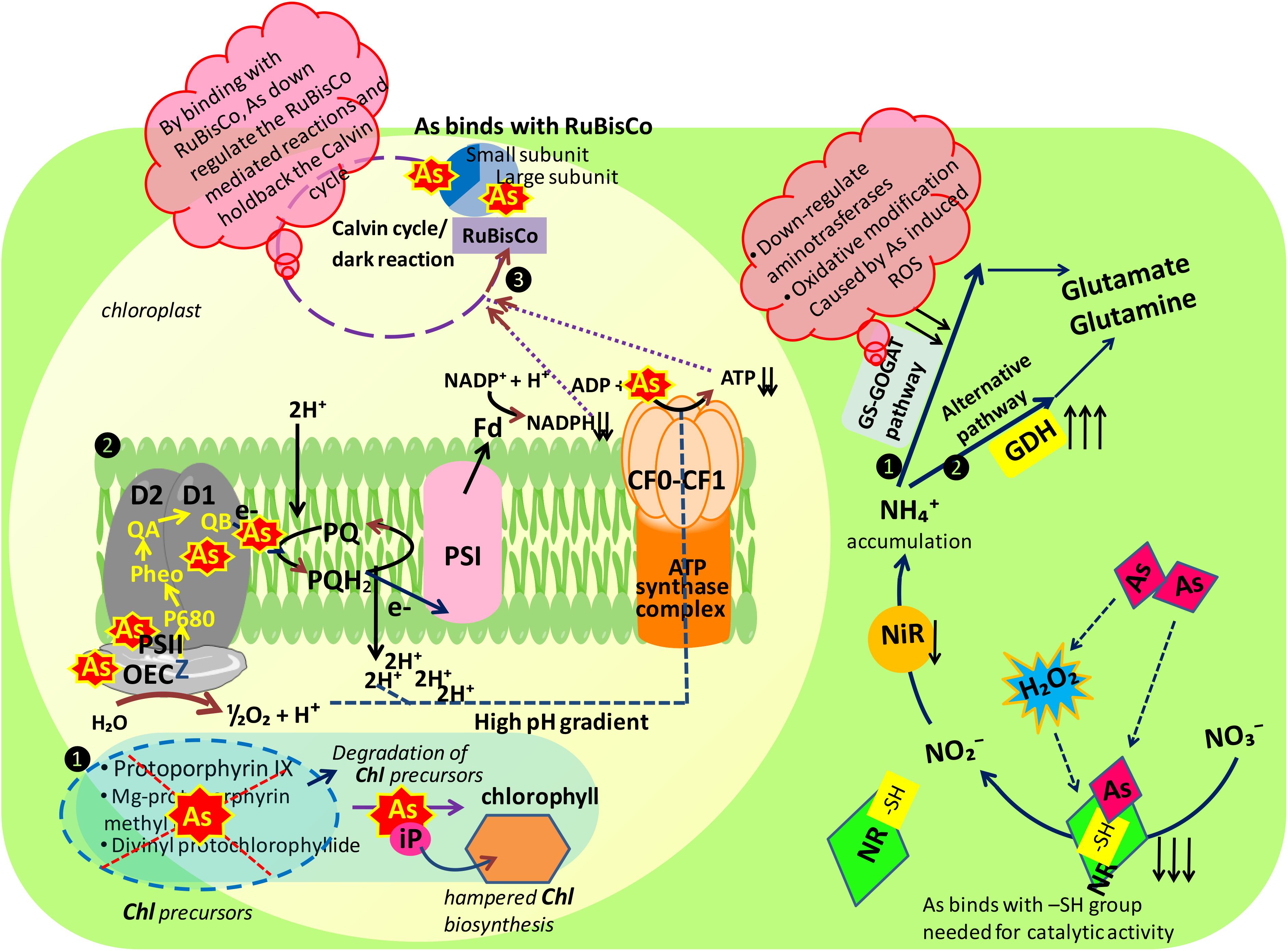
FIGURE 8. Schematic representation of major mechanisms underlying arsenic toxicity in Brassica juncea L. seedlings. Figure illustrating the mode of action of arsenic at probable sites of two major mechanisms playing major role in plant metabolism: photosynthesis and nitrogen metabolism, As, arsenic; inorganic nitrogen contents: NO3-, nitrate; NO2-, nitrite, and NH4+, ammonium; nitrate assimilating enzymes: NR, nitrate reductase and NiR, nitrite reductase; ammonia assimilating enzymes: 1. GS-GOGAT pathway: GS, glutamine synthetase and GOGAT, glutamine 2-oxoglutarate aminotransferase; 2. alternative pathway: GDH, glutamate dehydrogenase; photosynthetic apparatus: Chl, chlorophyll; OEC, oxygen evolving complex; PS I, photosystem I; PS II, photosystem II; Fd, ferredoxin; PQ, plastoquinone; -SH, sulfhydryl group.
Any alteration in chlorophyll biosynthesis directly hinders the process of photosynthesis, and therefore affects plant growth. In our study, As adversely affects photosynthetic O2 yield and the extent of inhibition was concentration dependent (Figure 1). The possible reason behind the loss of photosynthetic efficiency could be: (i) damage to the photosynthetic pigments (Table 1) and membranes harboring the photosystems (Rahman et al., 2007), (ii) Chl is used as a source of metabolic carbon when carbohydrate availability is low (Araújo et al., 2011), (iii) damage to PS II reaction centers (Figure 3) along with the reduction in photosynthetic linear electron flow (LEF; Figure 2) thereby decreasing the potential to produce ATP and NADPH, both of which are needed to fuel the carbon fixation reactions (Figure 8), and (iv) the significant decline in 29 kDa ribonucleoproteins thereby holding back the dark reactions/Calvin cycle of photosynthesis. Moreover, As (AsV) most possibly has replaced iP in photophosphorylation reactions thereby uncoupling electron transport for ATP synthesis in thylakoid (Watling-Payne and Selwyn, 1974). Interestingly, both S and Ca supplementation either alone or in combination to As stressed Brassica plant noticeably improved the photosynthetic O2 evolution rate (Figure 1). The increase in photosynthetic rate could be explained on the basis that As stressed plant requires more energy to maintain the cellular homeostasis, so, in order to fulfill the energy demand, exogenous S might have up regulated the ferredoxin-NADP reductase (FNR) level, that catalyzes the production of NADPH+H+ required for CO2 assimilation and energy production (Dixit et al., 2015). High S concentration may also up regulate the Fe–S clusters, which is essential and versatile cofactors of proteins involved in catalysis and electron transport (Lill, 2009). The increased photosynthetic efficiency could also be drawn on the basis that S and Ca might have partially replaced the LEF thereby switching on the cyclic electron flow (CEF) around PSI, where CEF can oxidize P700 into P700+ and consumes excess reducing power of NADPH through the NADPH dehydrogenase-dependent pathway, which provides energy to Calvin cycle (Hochmal et al., 2015) thereby meeting energy demand. Most possibly exogenous Ca stores by binding to the proteins or the thylakoid membrane and regulates CEF via calcium sensor proteins (CAS; Hochmal et al., 2015).
To pinpoint the targets/sites of As on photosynthesis, polyphasic Chl a fluorescence transient was analyzed (Figure 2). The OJIP transient signifies sequential reduction of electron transport pool of PS II (Govindjee, 1995; Misra et al., 2012; Kalaji et al., 2014, 2017). In the present study, the intensity of fluorescence in the OJIP transient decreased with increasing concentration of As (Figure 2), which may be due to: (i) inhibition of electron transport at PS II donor side, thereby resulting into P680+ accumulation (Govindjee, 1995) and (ii) due to decreased pool size of QA- (Kalaji et al., 2014). The kinetic parameters for Chl a fluorescence, i.e., Ψ0, ΦP0, ΦE0, as well as PIABS, were found to diminish after As treatment (Figure 3) that suggests toward the sluggish electron flow from PS II to PS I and limitation of QA- reoxidation which could be associated with poor diffusion of PQ across the thylakoid membranes (Magyar et al., 2018). The increase in Sm value under As stress implied that the heterogeneity of PQ increased the electron donation capacity and QA reduction on acceptor side of PS II, which indicates that As decreased the total electron accepting capacity. The adverse effect of As on performance of PS II photochemistry might have occurred due to: (i) As accumulation inside the chloroplast that might have interrupted the functioning of thylakoid membranes, (ii) significant decrease in number of active RCs as clear from Fv/F0 values, thereby increasing the energy flux parameters, i.e., ABS/RC, TR0/RC, ET0/RC, and DI0/RC of PS II, (iii) downstream of QA- electron transfer thereby changing the rate of electron flow from QA- to QB, (iv) by increasing the degradation rate of D1 protein of PS II that shifted the equilibrium between synthesis and degradation thereby leading into inactivation of RCs, and (v) impairment of PS II on donor side (Tóth et al., 2012). The decline in the size and number of active photosynthetic reaction centers (Fv/F0) indicates that As either decreased the rate of photochemistry or pool size of QA (Krause and Weiss, 1991). The lowered PIABS of As stressed test plant was due to damaging effects on traits of PSII photochemistry: ΦP0, Ψ0, and ΦE0. Furthermore, increased F0/Fv value under As treatments indicated toward As-induced damaging effects on OEC, which could be due to decline in uptake of nutrients like Mn, which is an important component of OEC (data not shown). The As induced reduction in Area (symbolizes pool size of QA on the PS II reduction side) under As stress indicates that As inhibited electron transport rate at PS II donor side. Results clearly revealed that As affected both the acceptor as well as the donor side of PS II, thereby impairing the photosynthetic performance (Figure 1). On the other hand, S and Ca alone and in combination restored the functional and structural attributes of PS II such as quantum yield, size and number of RCs, and OEC as evidence by increased ΦP0 and Fv/F0 values and decreased F0/Fv values, respectively. On S and Ca application to As stressed Brassica seedlings, a steep decline in ABS/RC, TR0/RC, ET0/RC, and DI0/RC (Figure 3) was obtained indicating that PS II apparatus maintained the equilibrium of energy fluxes for absorption, trapping, and electron transport through regulation of active RCs of PS II in response to As stress (Misra et al., 2001). This might have occurred due to higher plastoquinone levels, P700-content, and Hill activity as reported by Buschmann and Lichtenthaler (1977) following S and Ca treatments. Indeed, in the present study, the improved Area value (proportional to the pool size of the electron acceptors QA on the reduction side of PS II) under S and Ca application particularly S+Ca indicates that it might be an outcome of increase in the electron transfer from the reaction centers to the quinone pool. In addition to this, S and Ca might have recovered D1 protein either by increasing its synthesis or by reducing the degradation rate that finally improved the efficiency of PS II.
The dark respiratory O2 uptake rate was increased with increasing doses of As (Figure 1), which could be the result of partial uncoupling of ETC due to thylakoid membrane disruption; therefore, increase in O2 uptake rate was recorded to meet the situation of both, i.e., disrupted respiratory apparatus and the demand of ATP for carrying out the basic life processes by increasing the respiratory electron transport rate (Prasad and Zeeshan, 2005). Another possibility could be excessive ROS production that possibly consumes more O2 (actively accepts electron at intermediate stage of respiratory and photosynthetic ETC); therefore, an obvious demand of O2 was observed. The marginal decrease in the rate of dark respiration under S and Ca treatment could be due to the proper functioning of basal metabolism as S involves in various cellular redox processes and also increased the S assimilation by enhancing ATPS activity (Liang et al., 2016).
Nitrogen metabolism is an important biochemical process which maintains the N-status of plants; therefore, different indices were studied to get deeper insight regarding the degree of damage posed by As stress, while protein synthesis is closely linked with the formation of new tissues, which are major sink for N compounds. In plants, NO3- the most preferential source of nitrogen once absorbed by roots is assimilated to NH4+ through sequential reduction by NR and NiR. In the present study, NO3- content was severely affected by As in dose-dependent manner (Figure 4) which could be due to: (i) overproduction of ROS that causes membrane damage and electrolyte leakage, which might decreased NO3- absorption by root cells, (ii) uptake competition between NO3- and other existing anions like Cl-, SO42-, and CO32-, which may have unfavorable influences on plasma membrane permeability, and (iii) alteration in transpiration rate, which may result into limited NO3- flux from root to leaves. Being a committed enzyme in NO3- assimilation, NR is very sensitive to As stress; therefore, a marked inhibition in NR activity under As (Figure 5) stress was recorded that may restrict NO2- formation in comparison to control. It is known that –SH groups are required for catalytic activity of NR (Figure 8; Solomonson and Barber, 1990), so it is possible that As might have interacted with this group present in the active sites of NR enzyme (Hartley-Whitaker et al., 2002) thereby hampering it. Another possibility could be that As induces accumulation of endogenous H2O2, and NR is very sensitive to H2O2 as reported by Sharma and Dubey (2005), so H2O2 might have reduced the activity of this enzyme. Both NR and NiR enzymes are co-regulated on the induction side (Migge et al., 1997). Similar to NR, NiR activity was also inhibited by As (Figure 5) but less affected than NR as have been reported in Pteris vittata and Pteris ensiformis (Singh et al., 2009). The possible explanation could be, as the main regulator of NiR activity is the activity of NR, which is induced by NO3- (substrate; Dguimi et al., 2009), so the decline in NiR activity may be associated with reduced availability of NO2- ions, which is considered to be originated primarily from NR-catalyzed NO3- reduction. Another possibility may be heavy damage to photosynthetic ETC, which donates electron to reduce NO2- via ferredoxin. Further, As may decrease NR and NiR activities by affecting the enzyme synthesis or by bringing conformational changes in the enzymes’ structure. On the other hand, S and Ca supplementation either alone or in combination to As stressed seedlings enhanced NO3- content in tissues (Figure 4), which might be due to increased rate of transpiration (Wu et al., 2011) that facilitated the absorption and translocation of NO3- to leaves; as a consequence, NR and NiR activities were increased. Furthermore, comparatively less effect on NR activity under S and Ca treatment could be correlated with the betterment in NO3- content under S and Ca, and NR is a substrate-inducible enzyme (as discussed above); so, improved NO3- content improves NR activity.
The NH4+ formed by sequential reduction of NO3- is rapidly assimilated into organic compounds (amino acids) by ammonia assimilating enzymes, i.e., GS and GOGAT. The substantial decrease in GS and GOGAT activity caused by As might disturbed the C/N equilibrium, which negatively regulated the growth of test plant (Figures 1A,B). This decrease in GS activity could be due to the downregulation of aminotrasferases enzymes (involved in glycolysis, amino acid metabolism, photorespiration, and N used efficiency; Good et al., 2007), which would have resulted into lesser N content in Brassica seedlings as reported by Dixit et al. (2015). The decrease in GOGAT activity might be due to oxidative modifications credited by excessive ROS generation, under As treatment (Figure 6). Hence, as an outcome of decreased GS and GOGAT activities, a sharp increase in NH4+ content in root and leaf tissues of test seedlings under As stress was observed, which suggests impairment in NH4+ assimilation process. The decreased NO3- and increased NH4+ content justifies the reason for increased NH4+/NO3- ratio (Figure 4). The higher ratio of NH4+ to NO3- in B. juncea was caused mainly by reduction in NO3- uptake and NH4+ assimilation rate rather than NH4+ uptake, which could be justified by higher NH4+ accumulation and decline in GS activity. However, S and Ca application either alone or in combination to As stressed Brassica seedlings caused less inhibitory effect on GS and GOGAT activities (Figure 6), which could be explained as: (i) proper incorporation of NH4+ into glutamate as less NH4+ accumulation was found in root and leaves in comparison to As treatment alone (Figure 4) and (ii) less ROS accumulation that thereby causes minor alteration in active sites of these enzymes.
Moreover, when NH4+ gets hyper accumulated in plants, it directly incorporated into glutamate by triggering the GDH (aminating) enzyme and its activity was sharply increased at tested doses of As (Figures 6E,F). This increase in GDH activity (with high Km) might be the plants’ compensation for the inhibited GS and GOGAT activities. Thus, increased GDH activity might have helped in relieving the pressure caused by accumulation of NH4+ (Skopelitis et al., 2006); so, increased GDH activity could be a selective advantage to carry on NH4+ assimilation under As stress in test seedlings.
In the present study, the decrease in protein content subjected to As stress (Figure 7) might result from: (i) enhanced protein degradation rate as a result of increased protease activity (Stoeva and Bineva, 2003), (ii) As may reacts with –SH group of enzymes and proteins (Ullrich-Eberius et al., 1989) and (iii) decline in amino acid content as an outcome of considerable inhibition in GS and GOGAT activities in Brassica seedlings (Figure 6). Carbohydrate content might be decreased as: (i) Chl a contents are directly associated with carbohydrate production, so decreased Chl a contents might have decreased the carbohydrate content (Figure 7; Choudhury et al., 2011) and (ii) decrease in photosynthetic rate (Figure 1). Contrary to this, S and Ca improved the protein and carbohydrate contents in As stressed plant. The role of S and Ca in enhancing protein content may be attributed to: (i) enhanced protein synthesis as S is involved in di-sulfide (S–S) linkage of protein, (ii) decrease in proteolysis, (iii) lowering of enzyme denaturation processes, that occurs during abiotic stress (Levitt, 1980), and (iv) improvement in amino acid influx due to improvement in GS-GOGAT pathway (Figure 6). On the other hand, the increase in carbohydrate content induced by S and Ca have also been reported in chickpea plant grown under Cd stress (Ahmad et al., 2016) and flax plant grown under newly reclaimed sandy soils (Bakry et al., 2015). The explanation for this increment in carbohydrate content could be the improved photosynthetic efficiency (Figure 1) that might enhance the biosynthesis of carbohydrates.
Conclusion
From the above study, it is concluded that B. juncea L. seedlings, the third highest oil seed crop of the world, is suffering from As toxicity especially in South-East Asia, where As contamination is at alarming stage. Results clearly revealed that As negatively regulates the growth by interfering with the two key metabolic processes, i.e., photosynthesis and nitrogen metabolism (by inhibiting enzyme activities) as evident from higher As/S and As/Ca ratio in root followed by shoots/leaves. Contrastingly, S and/or Ca application following As treatment provides significant protection to Brassica seedlings by improving the photosynthetic performance and activities of enzymes of nitrogen metabolism. Furthermore, in the present study, the more pronounced role of S+Ca was observed in As-challenged Brassica seedlings.
Author Contributions
SP designed the experiment. RS and PP performed the experiment. SP, RS, and PP wrote and finalized the manuscript.
Conflict of Interest Statement
The authors declare that the research was conducted in the absence of any commercial or financial relationships that could be construed as a potential conflict of interest.
Acknowledgments
We are very grateful to the Department of Botany, University of Allahabad, Allahabad, for providing necessary lab facilities. The University Grants Commission, New Delhi, is also acknowledged for providing financial support to RS and PP (as UGC-AU research scholar) to carry out the present work.
References
Ahmad, P., Abdel Latef, A. A., Abd_Allah, E. F., Hashem, A., Sarwat, M., Anjum, N. A., et al. (2016). Calcium and potassium supplementation enhanced growth, osmolyte secondary metabolite production, and enzymatic antioxidant machinery in cadmium–exposed Chickpea (Cicer arietinum L.). Front. Plant Sci. 7:513. doi: 10.3389/fpls.2016.00513
Ahmad, P., Sarwat, M., Bhat, N. A., Wani, M. R., Kazi, A. G., and Tan, L. P. (2015). Alleviation of cadmium toxicity in Brassica juncea L. (Czern. & Coss.) by calcium application involves various physiological and biochemical strategies. PLoS One 10:e0114571. doi: 10.1371/journal.pone.0114571
Allen, S. E., Grimshaw, H. M., and Rowland, A. P. (1986). “Chemical analysis,” in Methods in Plant Ecology, eds P. D. Moore and S. B. Chapman (Oxford: Blackwell), 285–344.
Araújo, W. L., Tohge, T., Ishizaki, K., Leaver, C. J., and Fernie, A. R. (2011). Protein degradation– an alternative respiratory substrate for stressed plants. Trends Plant Sci. 16, 489–498. doi: 10.1016/j.tplants.2011.05.008
Bakry, A. B., Sadak, M. S., and El-karamany, M. F. (2015). Effect of humic acid and sulfur on growth, some biochemical constituents, yield and yield attributes of flax grown under newly reclaimed sandy soils. ARPN J. Agri. Biol. Sci. 10, 247–259.
Bradford, M. M. (1976). A rapid and sensitive method for the quantitation of microgram quantities of protein utilizing the principle of protein–dye binding. Anal. Biochem. 72, 248–254. doi: 10.1016/0003-2697(76)90527-3
Buschmann, C., and Lichtenthaler, H. K. (1977). Hill–activity and P700 concentration of chloroplasts isolated from radish seedlings treated with–indole acetic acid, kinetin of gibberellic acid. Z. Naturforsch. C 32, 798–802.
Cataldo, D. A., Haroon, M., Schrader, L. E., and Youngs, V. L. (1975). Rapid colorimetric determination of nitrate in plant tissue by nitration of salicylic acid. Commun. Soil Sci. Anal. 6, 71–80. doi: 10.1080/00103627509366547
Choudhury, B., Chowdhury, S., and Biswas, A. K. (2011). Regulation of growth and metabolism in rice (Oryza sativa L.) by arsenic and its possible reversal by phosphate. J. Plant Int. 6, 15–24.
Debouba, M., Gouia, H., Suzuki, A., and Ghorbel, M. H. (2006). NaCl stress effects on enzymes involved in nitrogen assimilation pathway in tomato seedlings. J. Plant Physiol. 163, 1247–1258. doi: 10.1016/j.jplph.2005.09.012
Dguimi, H. M., Debouba, M., Ghorbel, M. H., and Gouia, H. (2009). Tissue-specific cadmium accumulation and its effects on nitrogen metabolism in tobacco (Nicotiana tabaccum, Bureley v.Fb9). Ecology 332, 58–68. doi: 10.1016/j.crvi.2008.08.021
Dixit, G., Singh, A. P., Kumar, A., Dwivedi, S., Deeba, F., and Tripathi, R. D. (2015). Sulfur alleviates arsenic toxicity by reducing its accumulation and modulating proteome, amino acids and thiol metabolism in rice leaves. Sci. Rep. 5:16205. doi: 10.1038/srep16205
Dubois, M., Gilles, K. A., Hamilton, J. K., Rebers, P. A., and Smith, F. (1956). Colorimetric method for determination of sugars and related substances. Anal. Chem. 28, 350–356. doi: 10.1021/ac60111a017
Food and Agricultural Organization [FAO] (2009). How to Feed the World in 2050. Rome: Food and Agriculture Organization.
Good, A. G., Johnson, S. J., Pauw, M. D., Carroll, R. T., Savidov, N., Vidmar, J., et al. (2007). Engineering nitrogen use efficiency with alanine aminotransferase. Botany 85, 252–262. doi: 10.1139/B07-019
Govindjee, E. (1995). Sixty-three years since Kautsky: chlorophyll a fluorescence. Aust. J. Plant Physiol. 22, 131–160. doi: 10.1071/PP9950131
Gupta, M., and Ahmad, M. A. (2014). Arsenate induced differential response in rice genotypes. Ecotoxicol. Environ. Saf. 107, 46–54. doi: 10.1016/j.ecoenv.2014.04.030
Hartley-Whitaker, J., Woods, C., and Meharg, A. A. (2002). Is differential phytochelatin production related to decreased arsenate influx in arsenate tolerant Holcus lanatus? New Phytol. 155, 219–225. doi: 10.1046/j.1469-8137.2002.00455.x
Hoagland, D. R., and Arnon, D. I. (1950). The water culture method for growing plants without soil. Cal. Agric. Exp. Station Circ. 347, 1–39.
Hochmal, A. K., Schulze, S., Trompelt, K., and Hippler, M. (2015). Calcium–dependent regulation of photosynthesis. BBA Bioenergetics 1847, 993–1003. doi: 10.1016/j.bbabio.2015.02.010
Kader, M. A., and Lindberg, S. (2010). Cytosolic calcium and pH signaling in plants under salinity stress. Plant Signal. Behav. 3, 1–7. doi: 10.4161/psb.5.3.10740
Kalaji, H. M., Schansker, G., Brestic, M., Bussotti, F., Calatayud, A., Ferroni, L., et al. (2017). Frequently asked questions about chlorophyll fluorescence, the sequel. Photosynth. Res. 132, 13–66. doi: 10.1007/s11120-016-0318-y
Kalaji, H. M., Schansker, G., Ladle, R. J., Goltsev, V., Bosa, K., and Allakhverdiev, S. I. (2014). Frequently asked questions about in vivo chlorophyll fluorescence: practical issues. Photosynth. Res. 122, 121–158. doi: 10.1007/s11120-014-0024-6
Khan, M. I. R., and Khan, N. A. (2014). Ethylene reverses photosynthetic inhibition by nickel and zinc in mustard through changes in PS II activity, photosynthetic nitrogen use efficiency, and antioxidant metabolism. Protoplasma 251, 1007–1019. doi: 10.1007/s00709-014-0610-7
Krause, G. H., and Weiss, E. (1991). Chlorophyll fluorescence and photosynthesis: the basic. Ann. Rev. Plant Physiol. 42, 313–349. doi: 10.1146/annurev.pp.42.060191.001525
Kumar, S., Dubey, R. S., Tripathi, R. D., Chakrabarty, D., and Trivedi, P. K. (2015). Omics and biotechnology of arsenic stress and detoxification in plants: current updates and prospective. Environ. Int. 74, 221–230. doi: 10.1016/j.envint.2014.10.019
Kurra-Hotta, M., Satoh, K., and Katoh, S. (1987). Relationship between photosynthesis and chlorophyll content during leaf senescence of rice seedlings. Plant Cell Physiol. 28, 1321–1329.
Lechowski, Z., and Białczyk, J. (1993). Calcium mediated action on chlorophyll synthesis in isolated embryo of Scots pine. Biol. Plant. 35:53. doi: 10.1007/BF02921119
Liang, T., Ding, H., Wang, G., Kang, J., Pang, H., and Lv, J. (2016). Sulfur decreases cadmium translocation and enhances cadmium tolerance by promoting sulfur assimilation and glutathione metabolism in Brassica chinensis L. Ecotoxicol. Environ. Saf. 124, 129–137. doi: 10.1016/j.ecoenv.2015.10.011
Lichtenthaler, H. K. (1987). Chlorophylls and carotenoids pigments of photosynthetic membranes. Methods Enzymol. 148, 350–382. doi: 10.1016/0076-6879(87)48036-1
Lill, R. (2009). Function and biogenesis of iron–sulphur proteins. Nature 460, 831–838. doi: 10.1038/nature08301
Lillo, C. (1984). Diurnal variations of nitrite reductase, glutamine synthetase, glutamate synthase, alanine amino transferase and aspartate amino transferase in barley leaves. Physiol. Plant. 61, 214–218. doi: 10.1111/j.1399-3054.1984.tb05899.x
Liu, X., Zhang, S., Shan, X. Q., and Christie, P. (2007). Combined toxicity of cadmium and arsenate to wheat seedlings and plant uptake and antioxidative enzyme responses to cadmium and arsenate co-contamination. Ecotoxicol. Environ. Saf. 68, 305–313. doi: 10.1016/j.ecoenv.2006.11.001
Lunde, C., Zygadlo, A., Simonsen, H. T., Nielsen, P. L., Blennow, A., and Haldrup, A. (2008). Sulfur starvation in rice: the effect on photosynthesis, carbohydrate metabolism, and oxidative stress protective pathways. Physiol. Plant. 134, 508–521. doi: 10.1111/j.1399-3054.2008.01159.x
Magyar, M., Sipka, G., Kovács, L., Ughy, B., Zhu, Q., Han, G., et al. (2018). Rate–limiting steps in the dark to light transition of Photosystem II - revealed by chlorophyll–a fluorescence induction. Sci. Rep. 8:2755. doi: 10.1038/s41598-018-21195-2
Migge, A., Meya, G., Carryol, E., Hirel, B., and Becker, T. W. (1997). Coaction of light and the nitrogen substrate in controlling the expression of the tomato genes encoding nitrite reductase and nitrate reductase. J. Plant Physiol. 151, 151–158. doi: 10.1016/S0176-1617(97)80147-2
Mishra, S., Alfeld, M., Sobotka, R., Andresen, E., Falkenberg, G., and Küpper, H. (2016). Analysis of sublethal arsenic toxicity to Ceratophyllum demersum: subcellular distribution of arsenic and inhibition of chlorophyll biosynthesis. J. Exp. Bot. 67, 4639–4646. doi: 10.1093/jxb/erw238
Misra, A. N., Misra, M., and Singh, R. (2012). “Chlorophyll fluorescence in plant biology,” in Biophysics, ed. A. N. Misra (Rijeka: Intech Open), 171–192.
Misra, A. N., Srivastava, A., and Strasser, R. J. (2001). Utilization of fast chlorophyll a fluorescence technique in assessing the salt/ion sensitivity of mung bean and Brassica seedlings. J. Plant Physiol. 158, 1173–1181. doi: 10.1078/S0176-1617(04)70144-3
Molins-Legua, C., Meseguer-Lloret, S., Moliner-Martinez, Y., and Campıns-Falco, P. (2006). Aguide for selecting the most appropriate method for ammonium determination in water analysis. Trends Anal. Chem. 20, 282–290. doi: 10.1016/j.trac.2005.12.002
Patel, K. S., Shrivas, K., Brandt, R., Jakubowski, N., Corns, W., and Hoffmann, P. (2005). Arsenic contamination in water, soil, sediment and rice of central India. Environ. Geochem. Health 27, 131–145. doi: 10.1007/s10653-005-0120-9
Prasad, S. M., and Zeeshan, M. (2005). UV–B radiation and cadmium induced changes in growth, photosynthesis, and antioxidant enzymes of cyanobacterium Plectonema boryanum. Biol. Plant. 49, 229–236. doi: 10.1007/s10535-005-0236-x
Price, A. H., Taylor, A., Ripley, S. J., Griffiths, A., Trewavas, A. J., and Knight, M. R. (1994). Oxidative signals in tobacco increase cytosolic calcium. Plant Cell 12, 1387–1398.
Rahman, M. A., Hasegawa, H., Rahman, M. M., Islam, M. N., Miah, M. A. M., and Tasmin, A. (2007). Effect of arsenic on photosynthesis, growth and yield of five widely cultivated rice (Oryza sativa L.) varieties in Bangladesh. Chemosphere 67, 1072–1079. doi: 10.1016/j.chemosphere.2006.11.061
Robin, P. (1979). Etude de quelques conditions d’extraction de la nitrate reductase des racines et des feuilles de plantules de maïs. Physiol. Veg. 17, 45–54.
Sebastian, A., and Prasad, M. N. V. (2014). Photosynthesis mediated decrease in cadmium translocation protect shoot growth of Oryza sativa seedlings up on ammonium phosphate–sulfur fertilization. Environ. Sci. Pollut. Res. 21, 986–997. doi: 10.1007/s11356-013-1948-7
Sharma, P., and Dubey, R. S. (2005). Drought induces oxidative stress and enhances the activities of antioxidant enzymes in growing rice seedlings. Plant Growth Regul. 46, 209–221. doi: 10.1007/s10725-005-0002-2
Singh, A. P., Dixit, G., Kumar, A., Mishra, S., Singh, P. K., Dwivedi, S., et al. (2016). Nitric oxide alleviated arsenic toxicity by modulation of antioxidants and thiol metabolism in rice (Oryza sativa L.). Front. Plant Sci. 6:1272. doi: 10.3389/fpls.2015.01272
Singh, N., Ma, L. Q., Vu, J. C., and Raj, A. (2009). Effects of arsenic on nitrate metabolism in arsenic hyperaccumulating and non–hyperaccumulating ferns. Environ. Pollut. 157, 2300–2305. doi: 10.1016/j.envpol.2009.03.036
Singh, R., Parihar, P., and Prasad, S. M. (2018). Simultaneous exposure of sulphur and calcium hinder As toxicity: up-regulation of growth, mineral nutrients uptake and antioxidants system. (in press). doi: 10.1016/j.ecoenv.2018.05.060
Singh, R., Singh, S., Parihar, P., Singh, V. P., and Prasad, S. M. (2015). Arsenic contamination, consequences and remediation techniques: a review. Ecotoxicol. Environ. Saf. 112, 247–270. doi: 10.1016/j.ecoenv.2014.10.009
Singh, R. P., and Srivastava, H. S. (1983). Regulation of glutamate dehydrogenase activity by amino acids in maize seedlings. Physiol. Plant. 57, 549–554. doi: 10.1016/j.jplph.2009.04.006
Singh, R. P., and Srivastava, H. S. (1986). Increase in glutamate synthase (n.d.) activity in maize seedlings in response to nitrate and ammonium nitrogen. Physiol. Plant. 66, 413–416. doi: 10.1111/j.1399-3054.1986.tb05944.x
Singh, V. P., Srivastava, P. K., and Prasad, S. M. (2013). Nitric oxide alleviates arsenic–induced toxic effects in ridged Luffa seedlings. Plant Physiol. Biochem. 71, 155–163. doi: 10.1016/j.plaphy.2013.07.003
Skopelitis, D. S., Paranychianakis, N. V., and Paschalidis, K. A. (2006). Abiotic stress generates ROS that signal expression of anionic glutamate dehydrogenases to form glutamate for proline synthesis in tobacco and grapevine. Plant Cell 18, 2767–2781. doi: 10.1105/tpc.105.038323
Snell, F. D., and Snell, C. T. (1949). Colorimetric Methods of Analysis, 3rd Edn. New York, NY: D. Van Nostrand Co.
Solomonson, L. P., and Barber, M. J. (1990). Assimilatory nitrate reductase: functional properties and regulation. Annu. Rev. Plant Physiol. Plant Mol. Biol. 41, 225–253. doi: 10.1146/annurev.pp.41.060190.001301
Stoeva, N., Berova, M., Vassilev, A., and Zlatev, Z. (2005). Effect of arsenic on some physiological parameters in bean plants. Biol. Planta. 49, 293–296. doi: 10.1007/s10535-005-3296-z
Stoeva, N., and Bineva, T. (2003). Oxidative changes and photosynthesis in oat plants grown in As–contaminated soil. Bulg. J. Plant Phsiol. 29, 87–95.
Strasser, R. J., Srivastava, A., and Tsimilli-Michael, M. (2000). “The fluorescence transient as a tool to characterize and screen photosynthetic samples,” in Probing Photosynthesis: Mechanisms, Regulation and Adaptation, eds M. Yunus, U. Pathre, and P. Mohanty (New York, NY: Taylor & Francis), 445–483.
Tóth, T., Zsiros, O., Kis, M., Garab, G., and Kovács, L. (2012). Cadmium exerts its toxic effects on photosynthesis via a cascade mechanism in the cyanobacterium, Synechocystis PCC 6803. Plant Cell Environ. 35, 2075–2086. doi: 10.1111/j.1365-3040.2012.02537.x
Ullrich-Eberius, C., Sanz, A., and Novacky, A. J. (1989). Evaluation of arsenate–and vanadate–associated changes of electrical membrane potential and phosphate transport in Lemna gibba–G1. J. Exp. Bot. 40, 119–128. doi: 10.1093/jxb/40.1.119
Watling-Payne, A. S., and Selwyn, M. J. (1974). Inhibition and uncoupling of photophosphorylation in isolated chloroplasts by organotin, organomercury and diphenyleneiodonium compounds. Biochem. J. 142, 65–74. doi: 10.1042/bj1420065
Wu, Z., Ren, H., McGrath, S. P., Wu, P., and Zhao, F. J. (2011). Investigating the contribution of the phosphate transport pathway to arsenic accumulation in rice. Plant Physiol. 157, 498–508. doi: 10.1104/pp.111.178921
Keywords: chlorophyll a fluorescence, glutamate dehydrogenase, glutamate synthase, glutamine synthetase, nitrate reductase, nitrite reductase, OJIP transient
Citation: Singh R, Parihar P and Prasad SM (2018) Sulfur and Calcium Simultaneously Regulate Photosynthetic Performance and Nitrogen Metabolism Status in As-Challenged Brassica juncea L. Seedlings. Front. Plant Sci. 9:772. doi: 10.3389/fpls.2018.00772
Received: 15 March 2018; Accepted: 18 May 2018;
Published: 19 June 2018.
Edited by:
Veronica Graciela Maurino, Heinrich Heine Universität Düsseldorf, GermanyReviewed by:
Amarendra Narayan Misra, Khallikote University, IndiaWei Huang, Kunming Institute of Botany (CAS), China
Copyright © 2018 Singh, Parihar and Prasad. This is an open-access article distributed under the terms of the Creative Commons Attribution License (CC BY). The use, distribution or reproduction in other forums is permitted, provided the original author(s) and the copyright owner are credited and that the original publication in this journal is cited, in accordance with accepted academic practice. No use, distribution or reproduction is permitted which does not comply with these terms.
*Correspondence: Sheo M. Prasad, cHJvZnNtcHJhc2FkQGdtYWlsLmNvbQ==
†These authors have contributed equally to this work.