- 1Peanut Research Institute, College of Agronomy, Shenyang Agricultural University, Shenyang, China
- 2College of Agronomy, Qingdao Agricultural University, Qingdao, China
Early sowing has been extensively used in high-latitude areas to avoid drought stress during sowing; however, cold damage has become the key limiting factor of early sowing. To relieve cold stress, plants develop a series of physiological and biochemical changes and sophisticated molecular regulatory mechanisms. The biomembrane is the barrier that protects cells from injury as well as the primary place for sensing cold signals. Chilling tolerance is closely related to the composition, structure, and metabolic process of membrane lipids. This review focuses on membrane lipid metabolism and its molecular mechanism, as well as lipid signal transduction in peanut (Arachis hypogaea L.) under cold stress to build a foundation for explicating lipid metabolism regulation patterns and physiological and molecular response mechanisms during cold stress and to promote the genetic improvement of peanut cold tolerance.
Introduction
Peanut (Arachis hypogaea L.), one of the most important grain legumes as the source of edible oils and proteins, is cultivated in the semi-arid tropical and subtropical regions of the world (Katam et al., 2016). Recent statistics have shown that extreme weather events, particularly drought conditions caused by the changes in the global climate and water cycle, have occurred at an increasing frequency and intensity in peanut-producing countries, such as China and India (Yu et al., 2014; Lesk et al., 2016). In recent years, peanut planting areas have rapidly developed in high-latitude areas such as Northeast China. However, these regions are subjected to severe water-deficient conditions and seasonal drought, particularly from early May to the mid-May, the area covered by drought has been above 30% of the nation’s crop (Yang et al., 2018; Zhang et al., 2018; Figure 1A). According to the statistics of the Ministry of Water Resources of the People’s Republic of China (2018), the annual loss of industrial crops caused by drought in China accounts to 28.22 billion yuan, and peanuts account for about 20% (Li et al., 2014; Aninbon et al., 2016; Qin et al., 2017). Planting spring cultivars earlier is a feasible measure to circumvent spring sowing drought in peanut production, as well as in prolonging the vegetative growth period and increase nutrient accumulation for crop propagation (Rana et al., 2017). However, as a thermophilic crop, peanut needs relatively higher temperature throughout the whole development process (Wang et al., 2003). The lowest temperature of peanut germination is 12-15°C, and the peanut plant shows maximum growth at 28°C but experiences severe metabolic perturbations below 12°C (Bell et al., 1994). Sowing spring peanut earlier in Northeast China can impart deleterious effects on seed germination. In addition, chilling injury events have frequently occurred in Northeast China in the past few years (Ma et al., 2017; Shen et al., 2019; Figures 1B,C), severely influencing the peanut growth, development, bloom, and yield (Kakani et al., 2002; Wang et al., 2003; Chen et al., 2014; Table 1). Therefore, it is essential to optimize the comprehensive evaluation system of peanut cold tolerance and breed peanut germplasm with cold tolerance in Northeast China.
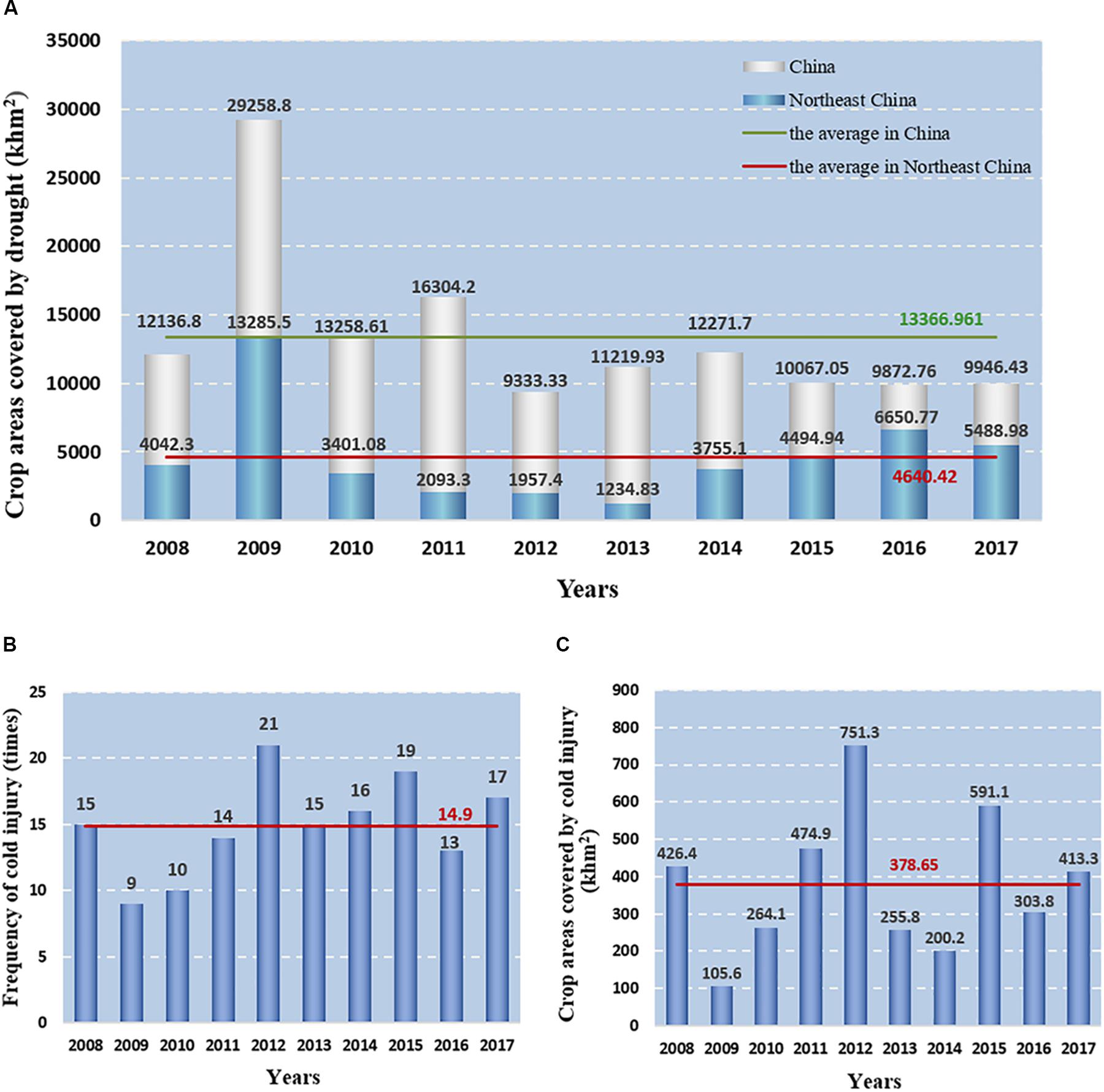
Figure 1. The occurrence of drought and low temperature disasters in China and Northeast China during 2008–2017. (A) The crop areas covered by drought in China and Northeast China. (B) Frequency of cold injury in Northeast China, and red line indicates the mean value. (C) The crop areas covered by cold injury in Northeast China, and red line indicates the mean value.
Research studies on cold stress in plants have been conducted in the early 1830s, with a history of more than 180 years. Breeders have been trying to develop new varieties to resolve the problem of peanut chilling damage and have made certain progress, and a few cold-tolerance early-maturing cultivars with ability to germinate in cooler soils have been released (Ntare et al., 2001; Gorbet and Shokes, 2002; Upadhyaya et al., 2003, 2006). However, cold tolerance in plants is an intricate quantitative trait that always occurs in combination or in succession and it is not controlled by a single regulatory pathway or gene, making conventional breeding approaches for cold tolerance challenging (Kumar et al., 2015; Wang et al., 2017). With the development of biotechnology in agriculture, extensive and in-depth studies on the mechanism of cold tolerance in plants in terms of morphologicalanatomical, physiological, biochemical, and molecular biology have been conducted. Lyons (1973) proposed that chilling damage initially occurs at the cellular and organ levels. The biomembrane system, including cell membrane, nuclear membrane and organelle membrane, is the initial site of injury, particularly in terms of its structure, function, stability, and enzyme activity, thereby resulting in substantial metabolic imbalance, especially involving respiration and photosynthesis. These changes in turn affect the plant growth and development and eventually incur damages at the whole-plant level, leading to the occurrence of chilling damage. Biomembrane is also the main repository of lipid for peanut plants (Yu, 2008), and fatty acid is the main component of biomembrane, which has been used as the primary index to evaluate peanut quality. Recent studies have further shown that chilling tolerance in peanut is closely correlated with the composition and structure of the membrane lipids, particularly the saturation of membrane fatty acids (Tang, 2011). The complex physiological, biochemical, and molecular mechanisms between membrane lipid metabolism and cold tolerance is being continuously explored to improve cold tolerance by means of high-throughput gene identification, gene editing, and transgenic technology.
In this review, we summarize the effects of cold stress on membrane lipid metabolism, including permeability, peroxidation, component change, and unsaturation, as well as its molecular mechanism and lipid signal transduction in peanut under cold stress, to lay a foundation for the elucidation of lipid metabolism regulatory patterns and physiological and molecular response mechanisms in cold stress, as well as to promote the genetic improvement of peanut cold tolerance.
Effects of Cold Stress on Membrane Permeability
The regulatory mechanism of biomembrane fluidity is one of the principal mechanisms that plants accommodate to changes in temperature conditions (Figure 2) and it is affected by the distribution ratio of various lipids on the membrane and the unsaturation of the glycerol lipid group (Li et al., 2016; Barrero-Sicilia et al., 2017). When peanut plants are subjected to cold stress, membrane lipids change from liquid crystal state to the gel state (Murata et al., 1982), which can cause the cessation of protoplast flow and an increase in membrane permeability, resulting in electrolyte leakage and loss of balance of intracellular ions (Huang et al., 2015). Chilling injury symptoms include dehydration, wilt, chlorosis, and accelerated senescence consequently happen (Upadhyaya et al., 2009). To maintain turgidity and original metabolic process, various organic and inorganic substances, such as inorganic salt, proline, betaine, soluble sugars, and soluble proteins, accumulate in plant cells via osmotic regulation, which lead to an increase in the concentration of cell fluid and a decrease in osmotic potential (Kishor and Sreenivasulu, 2014). Generally, under cold stress, proline, soluble sugars, and soluble proteins accumulate in the cytosol of sensitive and tolerant cultivars. Furthermore, the increasing amplitude of these osmotic regulation substances in varieties with stronger cold tolerance is larger than cold-sensitive varieties. However, the content of these significantly decreases in the cytosol when peanut plants are subjected to unbearable chilling (Bai D.M. et al., 2018; Kazemi-Shahandashti and Maali-Amiri, 2018).
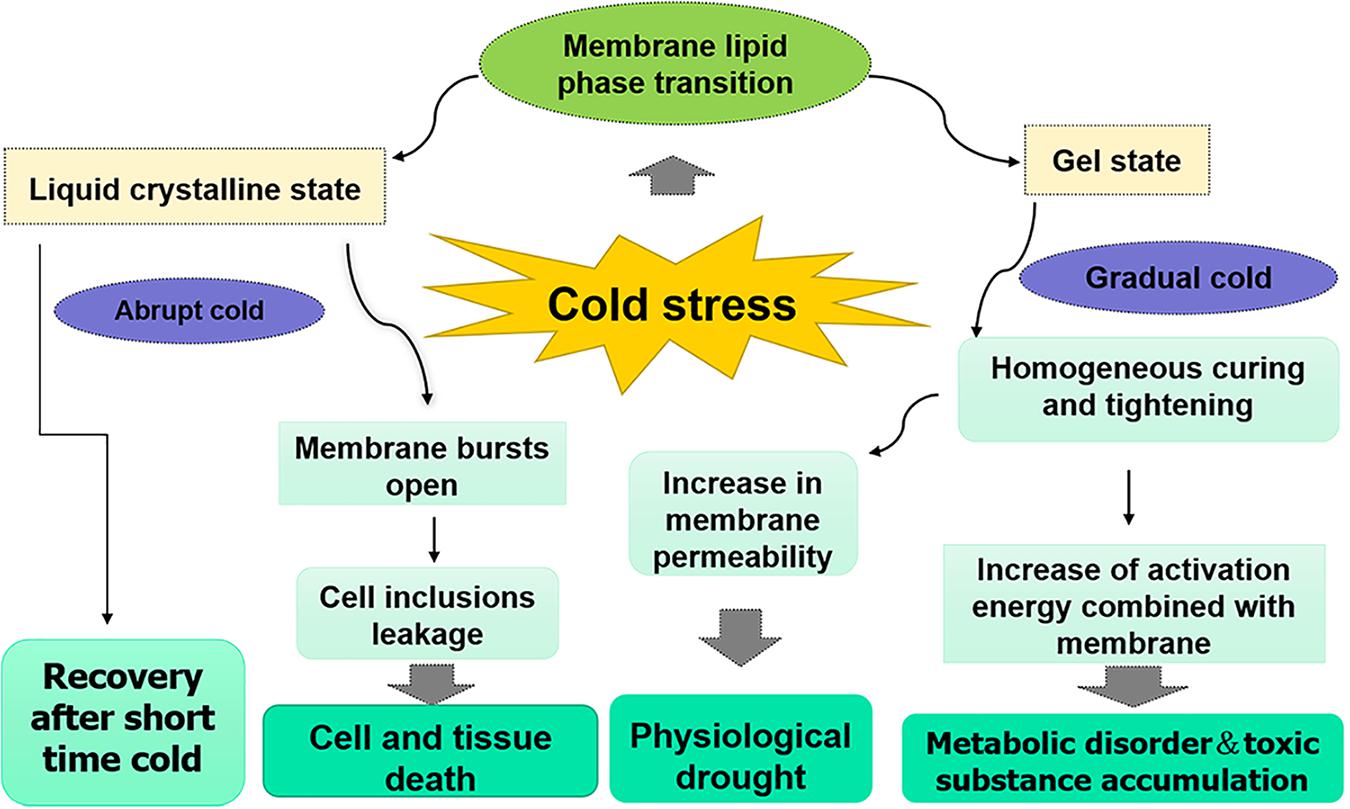
Figure 2. Effects of cold stress on membrane permeability. The main reason for the increase of membrane permeability in plants under cold stress is the phase transition of membrane lipids. When plants encounter an abrupt cold, membrane lipid is in liquid crystalline state, cold tolerant plants can recover in a short time, but the electrolyte leakage caused by membrane bursts open will happen in cold sensitive plants and ultimately lead to cell and tissue death. When plants encounter a gradual cold, membrane lipid is in gel state, the permeability of membrane increases with the prolongation of cold time, resulting in the loss of intracellular water and physiological drought. At the meantime, the increased activation energy of enzymes bound to membrane leads to metabolic disorder and toxic substance accumulation in plants.
Free proline accumulation is a heritable trait (Hanson et al., 1979) and can be used in screening genotypes for cold tolerance (Kim and Tai, 2011). Δ1-Pyrroline-5-carboxylate synthetase (P5CS) is a key enzyme in the glutamate pathway of proline biosynthesis. The overexpression of the P5CS gene in transgenic (introgressed with cDNA of the P5CS gene) plants results in increased cytoprotection and tolerance. The cDNA of the P5CS gene results in high levels of P5CS enzyme and a 10- to 18-fold increase in proline content, which contributes to both cold and drought tolerance through enhanced biomass production (Banavath et al., 2018). With the discovery of cDNA for P5CS and P5CR genes, future research could be directed at introgression of the gene, and the effect thereof, on yield and quality attributes of peanut under cold stress conditions in Northeast China.
Effects of Cold Stress on Membrane Lipid Peroxidation
The damage of the plant biomembrane system at low temperature is also related to membrane lipid peroxidation and protein destruction induced by reactive oxygen species (ROS) (Grant et al., 2014). Membrane lipid peroxidation refers to a series of free radical reactions on double bonds of unsaturated fatty acid on the membrane, which is initiated by oxygen free radicals (O2−., H2O2, ⋅OH) on unsaturated fatty acids in lipids (Thomas et al., 2016). ROS in vivo would produce abundantly and accumulate rapidly under cold stress that is far beyond the scavenging ability of antioxidant system, which breaks down the original equilibrium state of ROS. At this point, ROS begin to attack biological macromolecules, such as membrane lipids, nucleic acids, and proteins. The structure of the membrane system is also destroyed, resulting in a decrease in the photosynthetic rate, development of metabolic disorders, and massive accumulation of toxic substances in plants (Liu et al., 2013; Choudhury et al., 2016; Huang et al., 2016). Malondialdehyde (MDA) is the product of membrane lipid peroxidation that accumulates to higher concentrations in sensitive than tolerant genotypes (Iqbal et al., 2018a,b; Zhong et al., 2018). Cold-tolerant cultivars can resist external environmental stress by relying on the antioxidant enzyme system, which scavenges ROS and superoxide anion free radicals produced in plant cells, which mainly include superoxide dismutase (SOD), ascorbate peroxidase (APX), catalase (CAT), glutathione peroxidase (GPX), monodehydroascorbate reductase (MDHAR), dehydroascorbate reductase (DHAR), glutathione reductase (GR), and glutathione S-transferase (GST) (Tian et al., 2015).
Antioxidant enzymes are located among different sites of plant cells and work together with ROS-generating pathways to maintain ROS homeostasis (Zhang et al., 2015; Nejat and Mantri, 2017; Iqbal et al., 2019). The transcription factor APETALA2/ethylene response factor (AP2/ERF) plays an important regulatory role in signal transduction of the plant responses to various stresses including low temperature (Sakuma et al., 2002), which confers cold tolerance by promoting polyamine turnover, antioxidant protection, and proline accumulation. ERF1-Overexpressing plants have higher antioxidant activities, which are attributable to higher expression of genes, such as Cu, Zn-SOD, CAT1, CAT2, CAT3, and cpAPX, and accumulate more proline that is associated with induced P5CS and reduced PROX2 transcription compared to the wild-type. These transgenic plants show reduced MDA contents, H2O2, and ROS accumulation under cold stress, which contribute to alleviating oxidative damage to biomembrane after cold stress treatment (Zhuo et al., 2018). To date, a variety of AP2/ERF transcription factors have been successfully identified and investigated in many plants, including Arabidopsis, rice (Nakano et al., 2006), wheat (Zhuang et al., 2011), soybean (Zhang et al., 2008) and rapeseed (Du et al., 2016). The peanut genome has eight ERFs, including AhERF1–6, AhERF008, and AhERF019. However, different expression patterns in relation to responses to abiotic stress have been described. For example, the expression of AhERF4 and AhERF6 is rapid and is substantially enhanced by abiotic stress, whereas the expression of AhERF1 and AhEERF5 are slightly enhanced under certain stress conditions (Chen et al., 2012; Wan et al., 2014). Interestingly, AhDREB1 can improve tolerance to cold stress via the ABA-dependent pathway in Arabidopsis, and histone acetylation can affect the expression of AhDREB1 under osmotic stress conditions, thereby improving plant cold tolerance (Bai H. et al., 2018).
Effects of Cold Stress on Membrane Lipid Component
The membrane lipids of peanut plants are mainly composed of phospholipids (PL), which include phosphatidyl choline (PC), phosphatidyl ethanolamine (PE), phosphatidyl inositol (PI), phosphatidyl glycerol (PG), phosphatidic acid (PA), glycolipids (GLs) that consist of mono-galactose diglyceride (MGDG) and di-galactose diglyceride (DGDG), and a small amount of sulfolipids (SLs) and neutral lipids (NLs), such as cholesterol (Jouhet et al., 2004). Biomembrane is a dynamic equilibrium system that adaptively adjusts the internal composition based on changes in external temperature. Changes in lipid components are closely related to peanut abiotic stress, and the distribution ratio of lipids on the biomembrane of different tolerant cultivars will change with different degrees under various stresses (Lauriano et al., 2000; Sui et al., 2018). Phospholipids content is positively correlated to cold tolerance in plants, and cold tolerance is weakened when PL synthesis is blocked (Saita et al., 2016). Phosphatidyl glycerol is the main factor determining the membrane lipid phase transition for containing much saturated fatty acids, although it only accounts for 3–5% of thylakoid membrane lipids. The percentage of high-melting point molecules (C16:0/16:0 + C16:0/16:1t + C18:0/16:0 + C18:0/16:1t) in total molecular species or saturated fatty acids (C16:0 + C16:1t + C18:0) in total fatty acids in PG is significantly related to plant cold sensitivity, which is higher in cold-sensitive cultivars (Eriksson et al., 2011). The MGDG and DGDG are important components of thylakoid membrane lipids, which are closely related to photosynthesis, and their contents also change dynamically at low temperature (Kobayashi, 2016). Lipidomic analysis of maize leaves after cold treatment shows an increase in the PA and DGDG, but a decrease in PC and MGDG, resulting in enhanced turnover of PC to PA, which serves as precursors for galactolipid synthesis under low temperature conditions (Gu et al., 2017).
Lipid transfer protein (LTP) acts as a carrier for lipid transfer among different cell membranes. Changes in LTP activity can lead to alterations in membrane lipid composition and affect cold tolerance (Sun et al., 2015). Choi and Hwang (2015) reported that the BLT101-overexpressing transgenic wheat lines (BLT101ox) under cold stress loose less water and showed decreased expression of the genes induced by hormones (such as auxin and cytokinin) compared to non-transgenic (NT) plants. After prolonged cold treatment, BLT101ox leaves show normal phenotypes, whereas the NT plants displaydehydrated and withered leaves. Non-specific LTPs (nsLTPs), small molecular basic protein with abundant content, are responsible for the intermembrane transport of phospholipids by changing the composition of membrane lipids, participating in the biosynthesis of membranes, and transporting lipids among different organelles (Liu F. et al., 2015). There is also evidence that nsLTP is closely related to stress tolerance (Gangadhar et al., 2016). LTP3 is positively regulated by the transcription factor MYB96, which mediates freezing and drought stress (Guo et al., 2013).
Effects of Cold Stress on Membrane Lipid Unsaturation
Plants can modulate the stability and fluidity of membrane by changing the unsaturation of fatty acids in membrane lipids, which is of great significance for organisms to maintain normal photosynthesis and respiratory metabolism and resist cold stress (Mironov et al., 2012; Karabudak et al., 2014). In general, the content of unsaturated fatty acids in lipid membranes increases with decreasing temperature. In addition, compared to cold-sensitive cultivars, the content and the degree (number of double bonds) of unsaturated fatty acids in lipids are higher in cold-tolerant cultivars (Nejadsadeghi et al., 2015). The biomembrane of chilling sensitive genotypes undergoes a phase transition from liquid crystal to gel even at room temperature due to high saturation of fatty acids, whereas cold-tolerant genotypes can keep the phase transition temperature lower than the cold treatment temperature, thus avoiding phase transition (Ianutsevich et al., 2016). The main fatty acids in various peanut cultivars are similar, including palmitic acid (16:0), stearic acid (18:0), oleic acid (18:1), linoleic acid (18:2), linolenic acid (18:3), and arachidic acid (20:0). However, their contents vary among cultivars after low temperature treatment, i.e., contents of 18:1, 18:2, and 18:3 rapidly increase, whereas those of 16:0 and 18:0 decrease (Tang, 2011).
In plant cells, saturated fatty acids are synthesized by the type II fatty acid synthase system with the aid of an acyl carrier protein (ACP). The biosynthesis of unsaturated fatty acid is conducted by the desaturation of saturated fatty acids depending on two kinds of acyl lipases, which include glycerol-3-phosphateacyl transferase (GPAT) that is responsible for the lipidization on the C-1 position of the glycerol skeleton, and monoacyl-glycerol-3-phosphateacyl transferase (MGAT) that is responsible for the lipidization at the C-2 position, as well as various fatty acid desaturases (FAD) (Klempova et al., 2013). Acyl carrier protein is a small, acidic protein that plays an essential role in fatty acid synthesis by elongating fatty acid chains. In peanut, AhACP1, AhmtACP3, AhACP4, and AhACP5 have been identified and have been proven to be closely linked with plant cold tolerance (Wei, 2012; Lei et al., 2014; Chi et al., 2017). The overexpression (OE) and antisense-inhibition (AT) of AhACP1 in transgenic tobacco could alter the content of total lipids and composition of fatty acid in leaves, leading to a significant increase or decrease in the content of C18:2 and C18:3, thereby becoming more tolerant or sensitive to cold stress, respectively. It has been suggested that AhACP1 bound to C18:1 might be the specific substrate of oleoyl-ACP thioesterase or GPAT and participate in membrane lipid synthesis (Yurchenko et al., 2014). The GPAT is the first acyl-lipidase in PG biosynthesis that can transfer the aliphatic acyl to C-1 position of glycerol 3-phosphate (G-3-P) to synthesize 1-acyl-glycerol-3-phosphoric acid (GPA). Cui et al. (2017) indicated that GPATs from different chilling-tolerant varieties have different selectivities to acyl group substrates, i.e., cold-sensitive genotypes prefer C16: 0, whereas chilling-tolerant genotypes have the same selectivity for C16:0 and C18:1. The expression of GPAT under cold stress is closely correlated to cold tolerance (Li et al., 2018). AhGPAT3 and AhGPAT5 are two genes that encode the GPAT protein, which plays a prominent role in the synthesis of peanut fatty acids, while its function in cold remains unclear (Hao et al., 2018).
Fatty Acids of Membrane Lipids and Genetic Engineering of Cold Tolerance
With the development of biotechnology, progress has been made in the genetic engineering of peanut cold tolerance. Several genes related to cold tolerance have been cloned and transferred to plants for functional studies (Cheng et al., 2013; Chen N. et al., 2016; Liu et al., 2016). The molecular regulatory mechanism under cold stress of fatty acid desaturation in membrane lipids includes regulating the expression of FAD to change the number of enzyme proteins, regulating the activity of FAD at post-translation level, and changing the available substrates to regulate the activity of FAD (Tovuu et al., 2016; Zhao et al., 2018). The fatty acid unsaturation of membrane lipid was mainly determined by the type and quantity of FAD, but few FADs in peanut have been functionally validated (Chi et al., 2011; Figure 3).
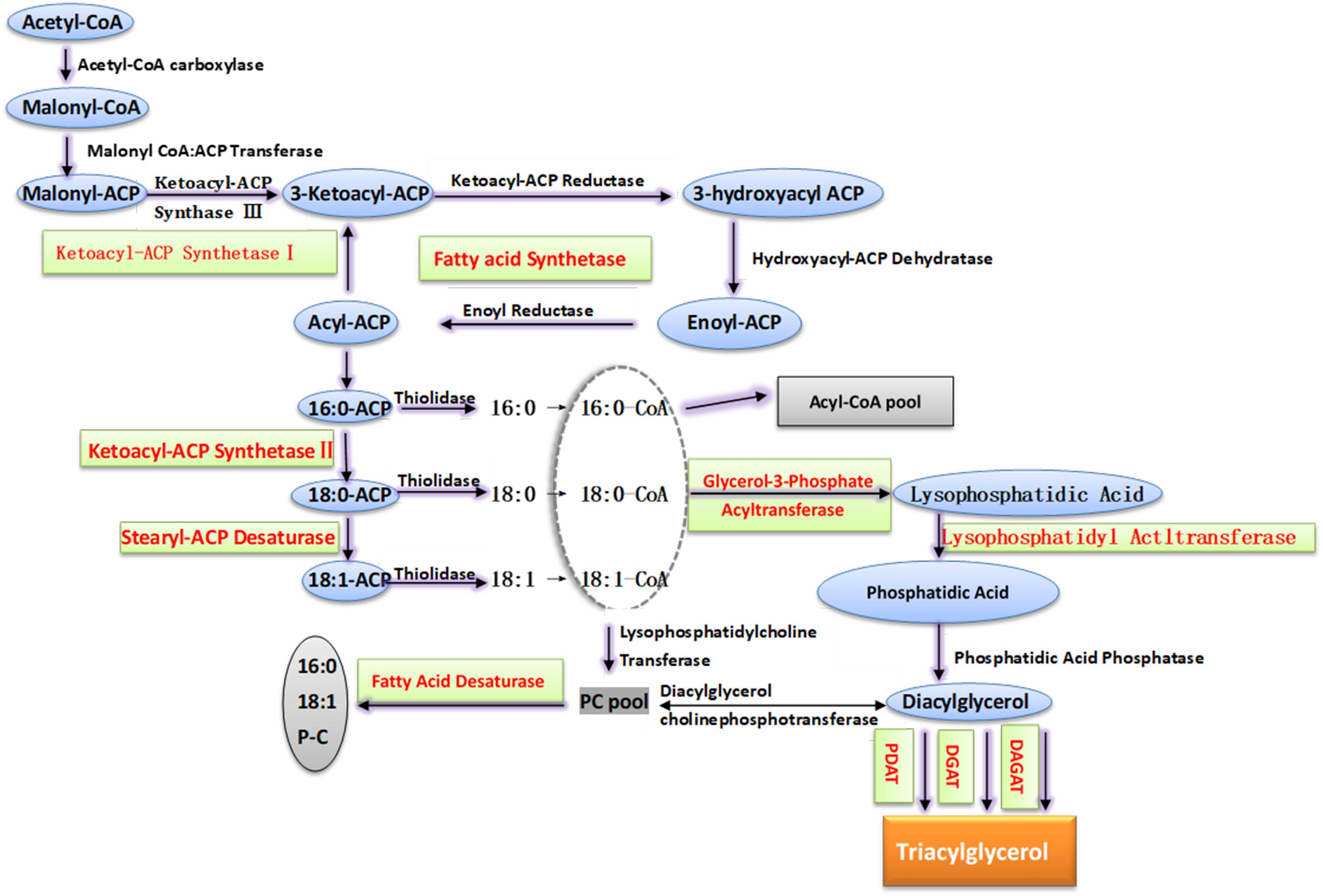
Figure 3. The pathway of membrane lipid biosynthesis in peanut. According to the substrate, the synthesis of triacylglycerol is divided into non-acyl-CoA dependent pathway and acyl-CoA dependent pathway. In the former, acyl groups transfer from phospholipid to diacylglycerol (DAG) to form triacylglycerol, and this step is catalyzed by phospholipid:diacylglycerol acyltransferase (PDAT). In the latter, glycerol-3-phosphate (G3P) is catalyzed by glycerol-3-phosphate acyltransferase (GPAT), lysophosphatidic acid acyltransferase (LPAAT) and diacylglycerol acyltransferase (DGAT) in turn and added the aliphatic group. The red characters in figure indicate the key genes in the lipid synthesis that have been identified and proved to play a vital role in peanut abiotic stress.
ω-3 FAD is considered the rate-limiting enzyme for the biosynthesis from diene fatty acids to triene fatty acids, and mainly responsible for catalyzing the introduction of the third double bond at ω-3 position. According to differences in subcellular localization, ω -3 FAD in higher plants can be divided into three types: FAD3 in the endoplasmic reticulum and FAD7 and FAD8 in plastids (Zhang et al., 2014). In Arabidopsis, FAD3, FAD7, and FAD8 have been proven to mediate the synthesis of trienoic fatty acids from C18:2 and C16:2, and their expression enhance chilling tolerance (Chen et al., 2015; Roman et al., 2015). Interestingly, the structures of FAD7 and FAD8 are highly similar and thus have the same functions, while their enzyme activities vary in terms of responses to low temperature. No significant changes in triene fatty acid content were observed in the leaves of fad7 mutant after cold treatment, and the expression of most FAD7 genes is not affected by low temperature. Inversely, the FAD8 gene is hardly expressed at normal temperature but induced by low temperature. It follows that FAD7 is involved in plant growth and development under normal temperature, whereas FAD8 participates in plant response to low temperature (Tang, 2007; Liu et al., 2014). The overexpression of OsFAD8 substantially increases C16:3 and C18:3 content in leaves of transgenic rice lines, resulting in the damage of plant survival at 2°C for 7 days alleviated. The content of triene fatty acids in rice lines of OsFAD8 silencing is reduced by 40.2% compared to that in the wild-type, although chilling tolerance decreased, allowing the plants to further adapt to a high-temperature environment (Nair et al., 2009). Wu et al. (2015) cloned a ω-3 Δ15- fatty acid dehydrogenase gene, AhFAD3A, which participates in the synthesis of α-C18:3 from cotyledons of germinated peanuts, and the expression of AhFAD3A is positively correlated with the formation of α -linolenic acid in peanut kernels and may be related to peanut tolerance.
The ω-6 FAD catalyzes monoenoic fatty acids to introduce the second double bond at ω-6 and form diene fatty acids, including FAD2 in endoplasmic reticulum and FAD4 and FAD6 in plastids (Park et al., 2016). Most of the known ω-6 FAD genes have multiple copies, and various copies of the same gene in the same plant vary in terms of the coding region, intron, and the length of the 5′UTR and 3′UTR (Cheng et al., 2013). FAD2 gene family is functionally responsible for the conversion of C18:1 to C18:2 in peanut, and six novel full-length cDNA sequences (AhFAD2-1, -2, -3, -4, -5, and -6) have been identified. In addition, the AhFAD2-1 gene is upregulated in developing seeds of peanut plants compared to the AhFAD2-2 gene, while the AhFAD2-2 gene is expressed most abundantly in the flowers, and they all play a major role in the conversion of oleic to linoleic acid (Wang et al., 2015; Wen et al., 2018). Accumulation of C16:1 and C18:1 in the fad6 mutant of Arabidopsis thaliana results in a decrease in the level of polyunsaturated fatty acids in chloroplast membrane lipids and the number of thylakoids under cold stress.
Acyl-ACP desaturase is the only soluble desaturase family in peanut, including Δ9 stearyl ACP desaturase (SAD) and Δ 6 palmityl ACP desaturase (PAD). The SAD catalyzes the conversion of stearoyl-ACP to oleoyl-ACP and determines the properties of most cellular glycerol-lipids (Liu H.L. et al., 2015). Moreover, the SAD gene is induced by cold stress and enhances cold tolerance by increasing the enzyme activity and the unsaturated fatty acid content (Luo et al., 2014). AhSAD3, AhSAD3A, and AhSAD3B have been identified as possible target genes for manipulation of fatty acid saturation in peanut (Florin et al., 2011). Transgenic plants that overexpress the SsSAD gene exhibit significantly higher linoleic (18:2) and linolenic acid (18:3) content and advanced freezing tolerance (Peng et al., 2018). The expressions of GhSAD2 gene in cotton plants after cold treatment at several levels were all upregulated; the level is highest after 6 h and then gradually decreases, thereby proving that the GhSAD2 gene may play a vital role in the synthesis of unsaturated fatty acids in cottonseed oil. At the same time, it also plays a certain physiological role in cold tolerance (Cai et al., 2017).
The Signal Transduction of Membrane Lipids Under Cold Stress
In the currently accepted model for temperature sensing, cold stress causes a change in membrane fluidity, and rearrangement of the cytoskeleton, followed by an influx of calcium that triggers downstream responses to confer cold tolerance(Guo and Liu, 2018). When cold signals are sensed by the plasma membrane, a series of signal transduction processes of membrane lipids are activated, leading to downstream actions to deliver the cold signal (Figure 4).
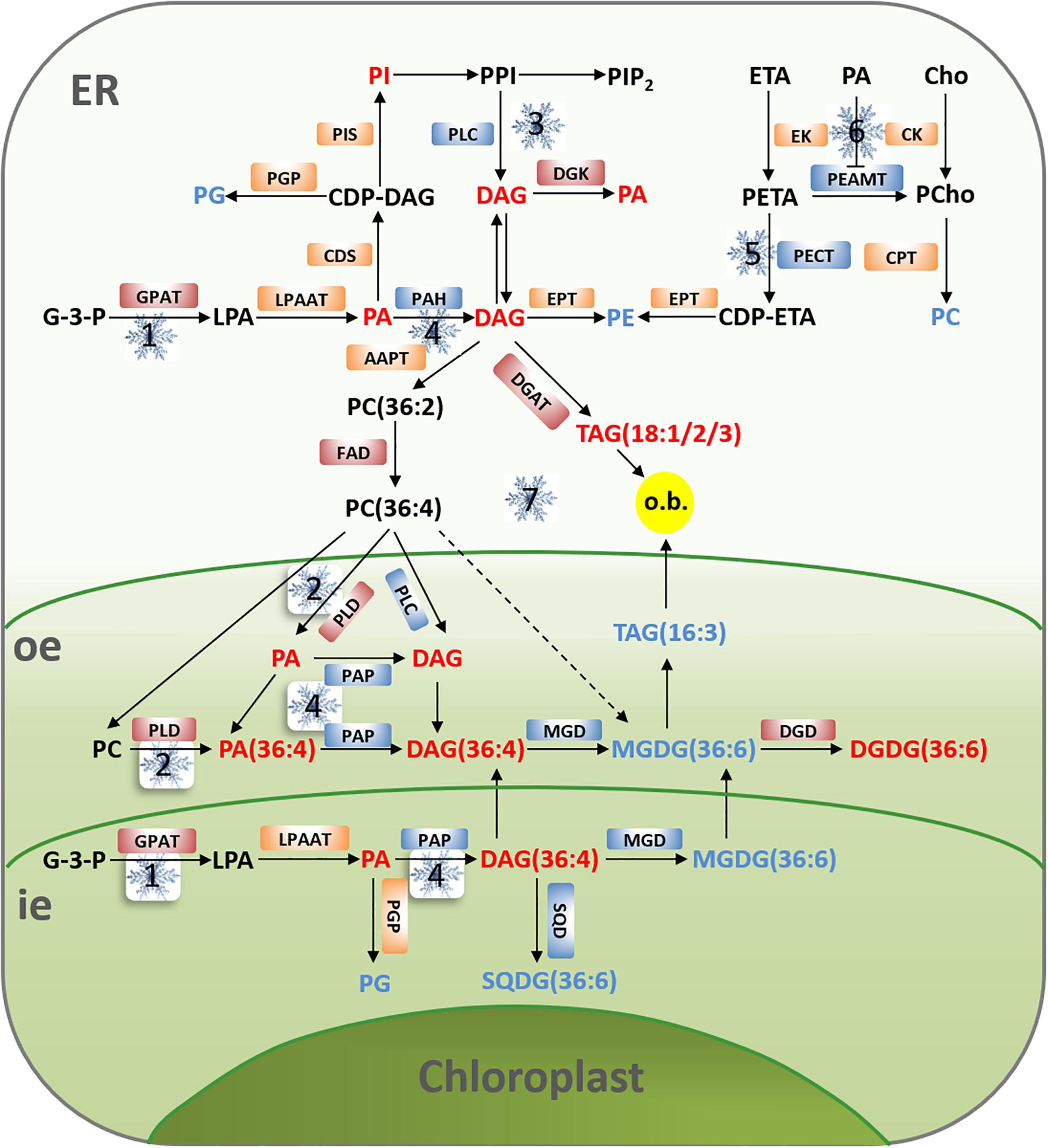
Figure 4. Model illustrating potential effects of cold stress on membrane lipid pathway in peanut. Under cold stress, there is an increase in the content of PA, PI, DAG, and DGDG (the red words), but a decrease in the content of PC, PE, PG, MGDG, and SQDG (the blue words). The main pathways to rapid cold-induced PA formation include the activity of GPAT is up regulated in the de novo biosynthesis of phospholipids (1), enhanced hydrolysis of PC by PLD resulted from the increase of PLD activity (2), the phosphorylation of PLC-generated DAG from PPI leads to DAG accumulation, which might cause an increased content of PA as a result from phosphorylation of DAG by DGK (3), or the inhibition of PAH/PAP activity by DAG (4). The activity of PECT is proposed to be down regulated by cold stress, and this would lead to reduced PE formation (5). As a second messenger, PA can inhibit the activity of PEAMT, thereby blocking the synthesis pathway of PC (6). During cold stress, the requirement for eukaryotic galactolipid biosynthesis is reduced and the activity of DGAT is upregulated, excess PC is converted to DAG and subsequently acylated to 18:1-, 18:2-, and 18:3-rich molecular species of TAG, which are contained in cytoplasmic oil bodies (o.b.). Simultaneously, turnover of MGDG in the chloroplast results in accumulation of low amounts of 16:3-containing, chloroplastic TAG (7). The red and blue boxes, respectively, represent the up-regulation and inhibition of the enzyme activities, the enzyme activities in orange boxes have no significant change or are not very clear yet before and after cold stress. AAPT, aminoalcohol phosphotransferase; CDP-ETA, cytidine diphosphate-ethanolamine; CDP-DAG, cytidine diphosphate-diacylglycerol; CDS, CDP-DAG synthase; Cho, choline; CK, choline kinase; CPT, phosphocholone cytidylyl transferase; EK, ethanolamine kinase; EPT, CDP-ethanolamine phosphotransferase; LPA, lysophosphatidic acid; LPAAT, lysophosphatidic acid acyltransferase; PAH, phosphatidate phosphohydrolase; PAP, phosphatidic acid phosphatase; PCho, phosphocholine; PEAMT, phosphoethanolaminemethyltranferase; PECT, phosphoethanolamine cytidylyl transferase; PETA, phosphoethanolamine; PGP, phosphatidic glycerol phosphatase; PIP2, phosphatidylinositol-4,5-bisphosphate; PIS, phosphatidylinositol synthase; SQD, sulfoquinovosyl diacylglycerol synthase; SQDG, sulfoquinovosyl diacylglycerol.
Phosphatidic acid is the precursor of PL biosynthesis, acts as the main lipid signal in eukaryotes, binds to specific protein, and activates the MAPK signal pathway, Ca2+-dependent protein kinase, NADPH oxidase, and ion channel (Testerink and Munnik, 2011). The biosynthesis of PA involves two different pathways: one is the direct hydrolysis of PL by phospholipase D (PLD), and the other way is phospholipase C (PLC) that catalyzes the hydrolysis of poly-phosphatidylethanolamine (PPI) together with diacylglycerol kinase (DGK) and synthesis PA from diacylglycerol (DAG) (Arisz et al., 2009). Extensive research suggests that PA is involved in the processes of plant growth, differentiation, reproduction, hormone response, and signal transduction under various biological and abiotic stresses (Hou et al., 2016; Meringer et al., 2016). Under cold stress, the two pathways of PLD/DGK and PLD are both responsive (Chen et al., 2015). Phospholipase D can catalyze the hydrolysis of phosphodiester bond and produce inositol triphosphate (IP3), diester glycerol (DAG), acetylcholine (Ach), and PA. As the second messengers in cells, IP3, DAG, PA, and Ach can cause a series of secondary reactions by changing intracellular Ca2+ and protein kinase K (PRK) levels, thus completing the process of cell response to cold signals (Hong et al., 2016). Moreover, the activity of PLD is closely related to the response of plant to low temperature (Muzi et al., 2016), short-term chilling stress (0–180 min) causes rapid and transient increases in PLD activity of young leaves, while long-term chilling stress (24–36 h) causes significant decreases in PLD activity in young leaves and roots (Peppino et al., 2017). Furthermore, PLD is also involved in ABA signal transduction under cold stress. ABA influences the activity of mitochondrial membrane – binding PLD in alpine ion mustard leaves through the mediation of Ca2+ under cold stress (He et al., 2017). As the initial enzyme of PL degradation, PLD can accelerate the degradation of PL, resulting in PA accumulation in the membrane. Phosphatidic acid can regulate the negative regulatory factor ABI1 of the ABA signaling pathway, as well as PLDal and PA that mediate ABA to induce upstream ROS accumulation and stomatal closure, thus contributing to chill tolerance in plants (Guo et al., 2012).
The MGDG and DGDG are vital components of chloroplast and thylakoid membrane lipids and are closely related to plant photosynthesis. They are synthesized by the catalysis of galactosylglycerol synthetase (MGD) and digalactoglycerol synthase (DGD), respectively (Rocha et al., 2018). Sensitive to feezing 2 (SFR2) is classified as a family I glycosyl hydrolase but has recently been shown to have galactosyltransferase (GAT) activity (Barnes et al., 2016). During freezing conditions, SFR2 transfers galactosyl from MGDG to another MGDG and produces oligogalactosols, including galactosyl diacylglycerol (GDG) and trigalactosyl diacylglycerol (TGD), leaving diacylglycerol (DAG) as a by-product (Roston et al., 2014). The DAG is converted into triacylglycerol (TAG), then TAG and oligogalactolipids derived from MGDG specifically increase in response to freezing (Vu et al., 2014). Therefore, the metabolic pathway of TAG is closely related to plant cold tolerance (Figures 3, 4). Diacylglycerol acyltransferase (DGAT) is a rate-limiting enzyme in the Kennedy pathway, one of the biosynthesis pathways of triacylglycerol (TAG) (Kennedy, 1963). In peanut, AhDGAT1-1 and AhDGAT1-2 heterologous expression in a Saccharomyces cerevisiae TAG-deficient quadruple mutant could restore lipid body formation, synthesis TAG and markedly accumulate higher levels of fatty acids (Peng et al., 2013; Tang et al., 2013; Chi et al., 2014). Recent studies have shown that DAGT1 plays a role in adaptive responses to chilling injury in plants, which can modulate the production of TAG and PA that cooperate with DGK (Chi et al., 2014; Chen B.B. et al., 2016; Yan et al., 2018). The expression of DGAT1, DGK2, DGK3, and DGK5 in A. thaliana during cold stress can regulate the dynamic balance of DAG, TAG, and PA, thereby maintaining the integrity of membrane system and intracellular redox state (Tan et al., 2018). Furthermore, DGAT1 and SFR2 coexist in chloroplasts and the activity of DGAT1 may be necessary for the SFR2 pathway, and DGAT1 may improve cold tolerance by SFR2-mediated cold tolerance (Arisz et al., 2018).
Conclusion and Prospective
Cold damage has become the key limiting factor of early sowing conducted to alleviate the spring sowing drought in peanut production in Northeast China. To cope with cold stress, plants have developed a series of physiological and biochemical changes and sophisticated molecular regulatory mechanisms, which display similarities and differences in various plant species. However, knowledge about physiological and molecular regulation mechanisms of peanut under cold stress in recent years has not been systematically documented. The plasma membrane is the barrier that protects the cell from injury and is also the primary place that senses cold signal. In the present review, we summarized the information on membrane lipid metabolism and its molecular response mechanisms, as well as lipid signal transduction in peanut under cold stress. Despite progress in elucidating the mechanism of cold tolerance in peanut, further investigations are warranted.
The cold signal is transduced from the extracellular to intracellular regions after being sensed by the plasma membrane and causes a series of physiological and biochemical changes. The ability to tolerate cold in peanut is based on the signal transduction by various factors in plant cells. However, how cold signals are perceived by plasma membranes and how cold signals transduce into intracellular through membranes are poorly understood. The diversity in plasma membrane composition, structure, and function is determined by the membrane lipids and membrane proteins. The interaction between membrane lipids and membrane proteins with different structures leads to differences in plasma membrane function. It is the key for analysis of cold signal transduction and elucidation of cold tolerance mechanism in peanut to understand the dynamic changes of plasma membrane structure and identify the function of the key protein.
The unsaturation of membrane lipids is closely related to cold tolerance in peanut. The proportion of unsaturated fatty acids has been regarded as an important index to measure the cold tolerance. Changing the ratio of saturated fatty acid to unsaturated fatty acid to improve cold tolerance peanut has become the research direction in recent years. However, the fatty acid composition of various lipids is variable, and it is not enough to analyze the fatty acid composition of membrane lipids in isolation to understand the physiological mechanism of membrane lipids. The main phospholipid molecules that make up the cell membrane and the main glycolipid molecules forming the chloroplast thylakoid membrane are also important in studying the physical phase transition of the membrane system at low temperature.
The development of emerging biotechnological methods in recent years, including CRISPR/Cas9, as well as the integration of omics and multi-omics, has impacted the agricultural sector by allowing the analysis of changes in lipid metabolism intermediates in the plasma membrane, the identification of differentially expressed genes related to lipids, and establishing a regulatory network for lipid metabolism under cold stress. This will be of great significance for how to satisfy plant growth requirements under deteriorating living conditions to sustain or even improve crop production.
Author Contributions
HZ wrote the manuscript. JD, XZ, JR, LX, CJ, XW, JW, and SZ conceived the study. YZ provided valuable references and made great contributions to the later revision. HY revised the manuscript and gave final approval of the version to be published.
Funding
Dr. Xu Quan provided the patient revision for this manuscript. This study was supported by the National Agricultural Research System of China (CARS-13).
Conflict of Interest Statement
The authors declare that the research was conducted in the absence of any commercial or financial relationships that could be construed as a potential conflict of interest.
References
Aninbon, C., Jogloy, S., Vorasoot, N., Patanothai, A., Nuchadomrong, S., and Senawong, T. (2016). Effect of end of season water deficit on phenolic compounds in peanut genotypes with different levels of resistance to drought. Food Chem. 196, 123–129. doi: 10.1016/j.foodchem.2015.09.022
Arisz, S. A., Christa, T., and Teun, M. (2009). Plant PA signaling via diacylglycerol kinase. Biochim. Biophys. Acta 1791, 869–875. doi: 10.1016/j.bbalip.2009.04.006
Arisz, S. A., Heo, J. Y., Koevoets, I. T., Zhao, T., Van, E. P., Meyer, J., et al. (2018). Diacylglycerol acyltransferase 1 contributes to freezing tolerance. Plant Physiol. 177, 1410–1424. doi: 10.1104/pp.18.00503
Awal, M. A., and Ikeda, T. (2002). Effects of changes in soil temperature on seedling emergence and phenological development in field-grown stands of peanut (Arachis hypogaea). Environ. Exp. Bot. 47, 101–113. doi: 10.1016/s0098-8472(01)00113-7
Bai, D. M., Xue, Y. Y., Zhao, J. J., Huang, L., Tian, Y. X., Quan, B. Q., et al. (2018). Identification of cold - tolerance during germination stage and genetic diversity of SSR markers in peanut landraces of Shanxi province. Acta Agronomica Sin. 44, 1459–1467. doi: 10.3724/SP.J.1006.2018.01459
Bai, H., Su, L. C., Hu, B., and Ling, L. (2018). Expression of AhDREB1, an AP2/ERF transcription factor gene from peanut, is affected by histone acetylation and increases abscisic acid sensitivity and tolerance to osmotic stress in Arabidopsis. Int. J. Mol. Sci. 19:e1441. doi: 10.3390/ijms19051441
Banavath, J. N., Chakradhar, T., Pandit, V., Konduru, S., Guduru, K. K., Akila, C. S., et al. (2018). Stress inducible overexpression of AtHDG11 leads to improved drought and salt stress tolerance in peanut (Arachis hypogaea L.). Front. Chem. 6:34. doi: 10.3389/fchem.2018.00034
Barnes, A. C., Benning, C., and Roston, R. L. (2016). Chloroplast membrane remodeling during freezing stress is accompanied by cytoplasmic acidification activating sensitive to freezing2. Plant Physiol. 171, 2140–2149. doi: 10.1104/pp.16.00286
Barrero-Sicilia, C., Silvestre, S., Haslam, R. P., and Michaelson, L. V. (2017). lipid remodelling: unravelling the response to cold stress in Arabidopsis and its extremophile relative eutrema salsugineum. Plant Sci. 263, 194–200. doi: 10.1016/j.plantsci.2017.07.017
Bell, M. J., Gillespie, T. J., Roy, R. C., Michaels, T. E., and Tollenaar, M. (1994). Peanut leaf photosynthetic activity in cool field environments. Crop Sci. 34, 1023–1029. doi: 10.2135/cropsci1994.0011183X003400040035x
Cai, M., Li, W., Liu, Y., Yu, Y., Kong, X., Wang, J., et al. (2017). Expression vector construction, transformation and cold resistance of GhSAD2 Gene from upland cotton. Acta Agric. Boreali Sin. 32, 60–66. doi: 10.7668/hbnxb.2017.06.009
Chang, B., Zhong, P., Liu, J., Tang, Z., Gao, Y., Yu, H., et al. (2019). Effect of low temperature stress and gibberellin on seed germination and seedling physiological responses in peanut. Acta Agronomica Sin. 45, 118–130. doi: 10.3724/SP.J.1006.2019.84043
Chen, B. B., Wang, J., Zhang, G., Liu, J., Manan, S., Hu, H., et al. (2016). Two types of soybean diacylglycerol acyltransferases are differentially involved in triacylglycerol biosynthesis and response to environmental stresses and hormones. Sci. Rep. 6:28541. doi: 10.1038/srep28541
Chen, N., Chi, X., Cheng, G., Pan, L., Chen, M., Wang, T., et al. (2016). Profiling of genes encoding cold stress-related transcription factors in peanut. J. Nuclear Agric. Sci. 30, 19–27. doi: 10.11869/j.issn.100-8551.2016.01.0019
Chen, N., Yang, Q., Su, M., Pan, L., Chi, X., Chen, M., et al. (2012). Cloning of six ERF family transcription factor genes from peanut and analysis of their expression during abiotic stress. Plant Mol. Biol. Rep. 30, 1415–1425. doi: 10.1007/s11105-012-0456-0
Chen, N., Yang, Q. L., Hu, D. Q., Pan, L. J., Chi, X. Y., Chen, M. N., et al. (2014). Gene expression profiling and identification of resistance genes to low temperature in leaves of peanut (Arachis hypogaea L.). Sci. Hortic. 169, 214–225. doi: 10.1016/j.scienta.2014.01.043
Chen, Y., Cui, Q., Xu, Y., Yang, S., Ming, G., and Wang, Y. (2015). Effects of tung oilseed FAD2 and DGAT2 genes on unsaturated fatty acid accumulation in Rhodotorula glutinis and Arabidopsis thaliana. Mol. Genet. Genomics 290, 1605–1613. doi: 10.1007/s00438-015-1011-0
Cheng, J., Emj, S., Huang, B., Krens, F. A., Dechesne, A. C., Visser, R. G. F., et al. (2013). Isolation and characterization of the omega-6 fatty acid desaturase (FAD2) gene family in the allohexaploid oil seed crop Crambe abyssinica Hochst. Mol. Breed. 32, 517–531. doi: 10.1007/s11032-013-9886-0
Chi, X., Chen, N., Wang, T., Wang, M., Chen, M., Pan, L., et al. (2017). Cloning and expression analysis of mtACP3 genes in peanut. Chin. Agric. Sci. Bull. 33, 35–42.
Chi, X., Hu, R., Zhang, X., Chen, M., Chen, N., Pan, L., et al. (2014). Cloning and functional analysis of three diacylglycerol acyltransferase genes from peanut (Arachis hypogaea L.). PLoS One 9:e105834. doi: 10.1371/journal.pone.0105834
Chi, X., Yang, Q., Pan, L., Chen, M., He, Y., Yang, Z., et al. (2011). Isolation and characterization of fatty acid desaturase genes from peanut (Arachis hypogaea L.). Plant Cell Rep. 30, 1393–1404. doi: 10.1007/s00299-011-1048-4
Choi, C., and Hwang, C. H. (2015). The barley lipid transfer protein, BLT101, enhances cold tolerance in wheat under cold stress. Plant Biotechnol. Rep. 9, 197–207. doi: 10.1007/s11816-015-0357-4
Choudhury, F. K., Rivero, R. M., Blumwald, E., and Mittler, R. (2016). Reactive oxygen species, abiotic stress and stress combination. Plant J. 90, 856–867. doi: 10.1111/tpj.13299
Cui, L. B., Zhu, L., and Jiang, L. X. (2017). The effect of ABA on biosynthesis of fatty acids and storage proteins and the relevant mechanism in cruciferae oilseed. J. Agric. Biotechnol. 25, 1059–1071. doi: 10.3969/j.issn.1674-7968.2017.07.003
Du, C., Hu, K., Xian, S., Liu, C., Fan, J., Tu, J., et al. (2016). Dynamic transcriptome analysis reveals AP2/ERF transcription factors responsible for cold stress in rapeseed (Brassica napus L.). Mol. Genet. Genom. 291, 1053–1067. doi: 10.1007/s00438-015-1161-0
Eriksson, S. K., Michael, K., Jan, P., Gerhard, G., and Pia, H. (2011). Tunable membrane binding of the intrinsically disordered dehydrin Lti30, a cold-induced plant stress protein. Plant Cell 23, 2391–2404. doi: 10.1105/tpc.111.085183
Florin, S., Brand, Y., Brand, A., Hedvat, I., and Ran, H. (2011). Identification and molecular characterization of homeologous Δ9-Stearoyl acyl carrier protein desaturase 3, genes from the allotetraploid peanut (Arachis hypogaea). Plant Mol. Biol. Rep. 29, 232–241. doi: 10.1007/s11105-010-0226-9
Gangadhar, B. H., Sajeesh, K., Venkatesh, J., Baskar, V., Kumar, A., Yu, J. W., et al. (2016). Enhanced tolerance of transgenic potato plants over-expressing non-specific lipid transfer protein-1 (StnsLTP1) against multiple abiotic stresses. Front. Plant Sci. 7:1228. doi: 10.3389/fpls.2016.01228
Gorbet, D. W., and Shokes, F. M. (2002). Registration of ‘C-99R’ peanut. Crop Sci. 42, 2207–2207. doi: 10.2135/cropsci2002.2207
Grant, O. M., Brennan, D., Mellisho, P., Salas, C. D., and Dix, C. J. (2014). Impact of enhanced capacity to scavenge reactive oxygen species on cold tolerance of tobacco. Int. J. Plant Sci. 175, 544–554. doi: 10.1086/675976
Gu, Y., He, L., Zhao, C., Wang, F., Yan, B., Gao, Y., et al. (2017). Biochemical and transcriptional regulation of membrane lipid metabolism in maize leaves under low temperature. Front. Plant Sci. 8:2053. doi: 10.3389/fpls.2017.02053
Guo, L., Mishra, G., Markham, J. E., Li, M., Tawfall, A., Welti, R., et al. (2012). Inter-relationship between sphingosine kinase and phospholipase D in signaling Arabidopsis response to abscisic acid. J. Biol. Chem. 287, 8286–8296. doi: 10.1074/jbc.M111.274274
Guo, L., Yang, H., Zhang, X., and Yang, S. (2013). Lipid transfer protein 3 as a target of MYB96 mediates freezing and drought stress in Arabidopsis. J. Exp. Bot. 64, 1755–1767. doi: 10.1093/jxb/ert040
Guo, X., and Liu, D. (2018). Cold signaling in plants: Insights into mechanisms and regulation. J. Integr. Plant Biol. 60, 745–756. doi: 10.1111/jipb.12706
Hanson, A. D., Nelsen, C. E., Pedersen, A. R., and Everson, E. H. (1979). Capacity for proline accumulation during water stress in barley and its implications for breeding for drought resistance. Crop Sci. 19, 489–493. doi: 10.2135/cropsci1979.0011183X001900040015x
Hao, C. C., Liang, C. W., Shi, L., Li, H. Y., Chen, M. N., Pan, L. J., et al. (2018). Cloning and expression analysis of glycerol-3-phosphate acyltransferase (GPAT) genes in peanut. J. Peanut Sci. 47, 10–18. doi: 10.14001/j.issn.1002-4093.2018.01.001
He, W., Zhang, X., Yang, P., Qiu, Y., Wang, X., and Yang, N. (2017). Effects of abscisic acid on mitochondrial membrane phospholipase D activity under low temperature stress. Guangxi plants 37, 742–748. doi: 10.11931/guihaia.gxzw201604018
Hong, Y., Zhao, J., Guo, L., Kim, S. C., Deng, X., Wang, G., et al. (2016). Plant phospholipases D and C and their diverse functions in stress responses. Prog. Lipid Res. 62, 55–74. doi: 10.1016/j.plipres.2016.01.002
Hou, Q., Ufer, G., and Bartels, D. (2016). Lipid signalling in plant responses to abiotic stress. Plant Cell Environ. 39, 1029–1048. doi: 10.1111/pce.12666
Huang, S., Van, A. O., Schwarzl, A. M., Belt, K., and Millar, A. H. (2016). Roles of mitochondrial reactive oxygen species in cellular signaling and stress response in plants. Plant Physiol. 171, 1551–1559. doi: 10.1104/pp.16.00166
Huang, X., Chen, M. H., Yang, L. T., Li, Y. R., and Wu, J. M. (2015). Effects of exogenous abscisic acid on cell membrane and endogenous hormone contents in leaves of sugarcane seedlings under cold stress. Sugar Tech. 17, 59–64. doi: 10.1007/s12355-014-0343-0
Ianutsevich, E. A., Danilova, O. A., Groza, N. V., and Tereshina, V. M. (2016). Membrane lipids and cytosol carbohydrates in Aspergillus niger, under osmotic, oxidative, and cold impact. Microbiology 85, 302–310. doi: 10.1134/S0026261716030152
Iqbal, H., Yaning, C., Waqas, M., Rehman, H., Shareef, M., and Iqbal, S. (2018a). Hydrogen peroxide application improves quinoa performance by affecting physiological and biochemical mechanisms under water-deficit conditions. J. Agron. Crop Sci. 204, 541–553. doi: 10.1111/jac.12284
Iqbal, H., Yaning, C., Waqas, M., Shareef, M., and Raza, S. T. (2018b). Differential response of quinoa genotypes to drought and foliage- applied H2O2 in relation to oxidative damage, osmotic adjustment and antioxidant capacity. Ecotox. Environ. Safe 164, 344–354. doi: 10.1016/j.ecoenv.2018.08.004
Iqbal, M., Raja, N. I, Mashwani, Z. U., Wattoo, F. H., Hussain, M., Ejaz, M., et al. (2019). Assessment of AgNPs exposure on physiological and biochemical changes and antioxidative defence system in wheat (Triticum aestivum L) under heat stress. IET Nanobiotechnol. 13, 230–236. doi: 10.1049/iet-nbt.2018.5041
Jouhet, J., Maréchal, E., Baldan, B., Bligny, R., Joyard, J., and Block, M. A. (2004). Phosphate deprivation induces transfer of DGDG galactolipid from chloroplast to mitochondria. J. Cell Biol. 167, 863–874. doi: 10.1083/jcb.200407022
Kakani,P. V. V., Craufurd, P. Q., and Wheeler, T. R. (2002). Response of in vitro pollen germination and pollen tube growth of groundnut (Arachis hypogaea L.) genotypes to temperature. Plant Cell Environ. 25, 1651–1661. doi: 10.1046/j.1365-3040.2002.00943.x
Karabudak, T., Bor, M., Ozdemir, F., and Turkan, I. (2014). Glycine betaine protects tomato (Solanum lycopersicum) plants at low temperature by inducing fatty acid desaturase7 and lipoxygenase gene expression. Mol. Biol. Rep. 41, 1401–1410. doi: 10.1007/s11033-013-2984-6
Katam, R., Sakata, K., Suravajhala, P., Pechan, T., Kambiranda, D. M., Naik, K. S., et al. (2016). Comparative leaf proteomics of drought-tolerant and -susceptible peanut in response to water stress. J. Proteomics 143, 209–226. doi: 10.1016/j.jprot.2016.05.031
Kazemi-Shahandashti, S. S., and Maali-Amiri, R. (2018). Global insights of protein responses to cold stress in plants: Signaling, defence, and degradation. J. Plant Physiol. 226, 123–135. doi: 10.1016/j.jplph.2018.03.022
Kennedy, J. F. (1963). The mechanics of dunes and antidunes in erodible-bed channels. J. Fluid Mech. 16, 521–544. doi: 10.1017/S0022112063000975
Ketring, D. L. (1984). Temperature effects on vegetative and reproductive development of peanut1,2. Crop Sci. 24, 877–882. doi: 10.2135/cropsci1984.0011183X002400050012x
Kim, S. I., and Tai, T. H. (2011). Evaluation of seedling cold tolerance in rice cultivars: a comparison of visual ratings and quantitative indicators of physiological changes. Euphytica 178, 437–447. doi: 10.1007/s10681-010-0343-4
Kishor, P. B. K., and Sreenivasulu, N. (2014). Is proline accumulation per se, correlated with stress tolerance or is proline homeostasis a more critical issue? Plant Cell Environ. 37, 300–311. doi: 10.1111/pce.12157
Klempova, T., Mihalik, D., and Certik, M. (2013). Characterization of membrane-bound fatty acid desaturases. Gen. Physiol. Biophys. 32, 639–645. doi: 10.4149/gpb_2013051
Kobayashi, K. (2016). Role of membrane glycerolipids in photosynthesis, thylakoid biogenesis and chloroplast development. J. Plant Res. 129, 565–580. doi: 10.1007/s10265-016-0827-y
Kumar, D., Datta, R., Hazra, S., Sultana, A., Mukhopadhyay, R., and Chattopadhyay, S. (2015). Transcriptomic profiling of Arabidopsis thaliana mutant pad2.1 in response to combined cold and osmotic stress. PLoS One 10:e0122690. doi: 10.1371/journal.pone.0122690
Lauriano, J. A., Lidon, F. C., Carvalho, C. A., Campos, P. S., and do Céu Matos, M. (2000). Drought effects on membrane lipids and photosynthetic activity in different peanut cultivars. Photosynthetica 38, 7–12. doi: 10.1023/a:1026775319916
Lei, S., Tang, G. Y., Xu, P. L., Zhao, X. B., and Liu, Z. J. (2014). Cloning and analysis of 5′ flanking regions of Arachisis hypogaea L. genes encoding plastidial acyl carrier protein. Acta Agronomica Sin. 40, 381–389. doi: 10.3724/SP.J.1006.2014.00381
Lesk, C., Rowhani, P., and Ramankutty, N. (2016). Influence of extreme weather disasters on global crop production. Nature 529, 84–87. doi: 10.1038/nature16467
Li, Q., Shen, W., Zheng, Q., Fowler, D. B., and Zou, J. (2016). Adjustments of lipid pathways in plant adaptation to temperature stress. Plant Signal. Behav. 11:e1058461. doi: 10.1080/15592324.2015.1058461
Li, X., Liu, P., Yang, P., Fan, C., Sun, X., and Zhang, J. (2018). Characterization of the glycerol-3-phosphate acyltransferase gene and its real-time expression under cold stress in Paeonia lactiflora pall. PLoS One 13:e0202168. doi: 10.1371/journal.pone.0202168
Li, X., Lu, J., Liu, S., Liu, X., Lin, Y., Li, L., et al. (2014). Identification of rapidly induced genes in the response of peanut (Arachis hypogaea) to water deficit and abscisic acid. BMC Biotechnol. 14:58. doi: 10.1186/1472-6750-14-58
Liu, C., Pan, S., Xue, H., and Liu, F. (2014). Analysis on FAD8 gene expression regulations in transcriptional level on Chinese cabbage. Exp. Technol. Manage. 45, S26–S31. doi: 10.1016/S0020-1383(14)70017-8
Liu, F., Zhang, X., Lu, C., Zeng, X., Li, Y., Fu, D., et al. (2015). Non-specific lipid transfer proteins in plants: presenting new advances and an integrated functional analysis. J. Exp. Bot. 66, 5663–5681. doi: 10.1093/jxb/erv313
Liu, H. L., Chen, X. S., Yang, F. J., Bai, D. M., Sun, X. P., Lu, Y., et al. (2017). Research of identification method on low temperature resistance of peanut germplasm resources phenotype. J. Peanut Sci. 46, 20–25. doi: 10.14001/j.issn.1002-4093.2017.03.004
Liu, H. L., Shen, H. T., Chen, C., Zhou, X. R., Liu, H., and Zhu, J. B. (2015). Identification of a putative stearoyl acyl-carrier-protein desaturase gene from Saussurea involucrate. Biol. Plant. 59, 316–324. doi: 10.1007/s10535-015-04870
Liu, Y., Chen, S., Cheng, Z., Wang, J., Song, Y., Hao, J., et al. (2016). Vector construction to overexpress AhPLDα genes from peanut and genetic transformation into Arabidopsis thaliana. Acta Agric. Boreali Sin. 31, 31–38. doi: 10.7668/hbnxb.2016.06.006
Liu, Y. F., Han, X. R., Zhan, X. M., Yang, J. F., Wang, Y. Z., Song, Q. B., et al. (2013). Regulation of calcium on peanut photosynthesis under low night temperature stress. JIA 12, 2172–2178. doi: 10.1016/S2095-3119(13)60411-6
Luo, X. Q., Ou, W. J., Li, K. M., and Chen, S. B. (2014). The structure and function prediction of the cold resistance enzyme stearoyl-ACP desaturation. J. Fujian Agric. For. Univ. 43, 484–489.
Lyons, J. M. (1973). Chilling injury in plants. Annu. Rev. Plant Physiol. 24, 445–466. doi: 10.1146/annurev.pp.24.060173.002305
Ma, Q. Y., Zhang, J. Q., Lai, Q., Zhang, F., Dong, Z. H., and Ls, A. (2017). Temporal and spatial variations of extreme climatic events in songnen grassland, northeast china during 1960-2014. Chin. J. Appl. Ecol. 28, 1769–1778. doi: 10.13287/j.1001-9332.201706.002
Meringer, M. V., Villasuso, A. L., Margutti, M. P., Usorach, J., Pasquare, S. J., Giusto, N. M., et al. (2016). Saline and osmotic stresses stimulate PLD/diacylglycerol kinase activities and increase the level of phosphatidic acid and proline in barley roots. Environ. Exp. Bot. 128, 69–78. doi: 10.1016/j.envexpbot.2016.03.011
Ministry of Water Resources of the People’s Republic of China (2018). Bulletin of Flood and Drought Disasters in China. Beijing: Sinomap Press.
Mironov, K. S., Sidorov, R. A., Trofimova, M. S., Bedbenov, V. S., Tsydendambaev, V. D., Allakhverdiev, S. I., et al. (2012). Los Light-dependent cold-induced fatty acid unsaturation, changes in membrane fluidity, and alterations in gene expression in Synechocystis. Biochim. Biophys. Acta Bioenerg. 1817, 1352–1359. doi: 10.1016/j.bbabio.2011.12.011
Murata, N., Sato, N., Takahashi, N., and Hamazaki, Y. (1982). Compositions and positional distribution of fatty acids in phospholipids from leaves of chilling-sensitive and chilling-resis-tant plants. Plant Cell Physiol. 23, 1071–1079. doi: 10.1093/oxfordjournals.pcp.a076437
Muzi, C., Camoni, L., Visconti, S., and Aducci, P. (2016). Cold stress affects H+-ATPase and phospholipase D activity in Arabidopsis. Plant Physiol. Biochem. 108, 328–336. doi: 10.1016/j.plaphy.2016.07.027
Nair, P. M. G., Kang, I. S., Moon, B. Y., and Lee, C. H. (2009). Effects of low temperature stress on rice (Oryza sativa L.) plastid s-3 desaturase gene, OsFAD8 and its functional analysis using T-DNA mutants. Plant Cell Tissue Organ 98, 87–96. doi: 10.1007/s11240-009-9541-y
Nakano, T., Suzuki, K., Fujimura, T., and Shinshi, H. (2006). Genome-wide analysis of the ERF gene family in Arabidopsis and rice. Plant Physiol. 140, 411–432. doi: 10.1104/pp.105.073783
Navarro, S., Donahaye, E., Kleinerman, R., and Haham, H. (2010). The influence of temperature and moisture content on the germination of peanut seeds. Peanut Sci. 16, 6–9. doi: 10.3146/i0095-3679-16-1-2
Nejadsadeghi, L., Reza, M. A., Hassan, Z., Sanaz, R., and Behzad, S. (2015). Membrane fatty acid compositions and cold-induced responses in tetraploid and hexaploid wheats. Mol. Biol. Rep. 42, 363–372. doi: 10.1007/s11033-014-3776-3
Nejat, N., and Mantri, N. (2017). Plant immune system: crosstalk between responses to biotic and abiotic stresses the missing link in understanding plant defence. Curr. Issues Mol. Biol. 23, 1–16. doi: 10.21775/cimb.023.001
Nigam, S. N., Rao, R. C. N., and Wynne, J. C. (2010). Effects of temperature and photoperiod on vegetative and reproductive growth of groundnut (Arachis hypogaea L.). J. Agron. Crop Sci. 181, 117–124. doi: 10.1111/j.1439-037X.1998.tb00406.x
Ntare, B. R., Williams, J. H., and Dougbedji, F. (2001). Evaluation of groundnut genotypes for tolerance under field conditions in a sahelian environment using a simple physiological model for yield. J. Agr. Sci. 136, 81–88. doi: 10.1017/S0021859600008583
Park, H. G., Kothapalli, K. S. D., Park, W. J., DeAllie, C., Liu, L., Liang, A., et al. (2016). Palmitic acid (16:0) competes with omega-6 linoleic and omega-3 α-linolenic acids for FADS2 mediated 6-desaturation. Biochim. Biophys. Acta 1861, 91–97. doi: 10.1016/j.bbalip.2015.11.007
Peng, D., Zhou, B., Jiang, Y., Tan, X. F., Yuan, D. Y., and Zhang, L. (2018). Enhancing freezing tolerance of Brassica napus, L. by overexpression of a stearoyl-acyl carrier protein desaturase gene (SAD) from Sapium sebiferum, (L.) roxb. Plant Sci. 272, 32–41. doi: 10.1016/j.plantsci.2018.03.028
Peng, Z., Li, L., Yang, L., Zhang, B., Chen, G., and Bi, Y. (2013). Overexpression of peanut diacylglycerol acyltransferase 2 in Escherichia coli. PLoS One 8:e61363. doi: 10.1371/journal.pone.0061363
Peppino, M. M., Reyna, M., Meringer, M. V., Racagni, G. E., and Villasuso, A. L. (2017). Lipid signalling mediated by PLD/PA modulates proline and H2O2 levels in barley seedlings exposed to short- and long-term chilling stress. Plant Physiol. Biochem. 113, 149–160. doi: 10.1016/j.plaphy.2017.02.008
Prasad, P. V., Boote, K. J., Thomas, J. M., Allen, L. H., and Gorbet, D. W. (2006). Influence of soil temperature on seedling emergence and early growth of peanut cultivars in field conditions. J. Agron. Crop Sci. 192, 168–177. doi: 10.1111/j.1439-037X.2006.00198.x
Qin, F., Xu, H. L., and Ci, D. (2017). Drought stimulation by hypocotyl exposure altered physiological responses to subsequent drought stress in peanut seedlings. Acta Physiol. Plant 39:152. doi: 10.1007/s11738-017-2447-0
Rana, V. K., Mahmoud, T., Mohammad, M., Hamid, M., and Mohammad, P. (2017). Effects of freeze and cold stress on certain physiological and biochemical traits in sensitive and tolerant barley (Hordeum vulgare) genotypes. J. Plant Nutr. 41, 102–111. doi: 10.1080/01904167.2017.1381730
Rocha, J., Nitenberg, M., Agnes, G. E., Juliette, J., Eric, M., Block, M. A., et al. (2018). Do galactolipid synthases play a key role in the biogenesis of chloroplast membranes of higher plants? Front. Plant Sci. 9:126. doi: 10.3389/fpls.2018.00126
Roman, A., Hernandez, M. L., Soria-Garcia, A., Lopez-Gomollon, S., Lagunas, B., Picorel, R., et al. (2015). Non-redundant contribution of the plastidial FAD8 ω-3 desaturase to glycerolipid unsaturation at different temperatures in Arabidopsis. Mol. Plant 8, 1599–1611. doi: 10.1016/j.molp.2015.06.004
Roston, R. L., Wang, K., Kuhn, L. A., and Christoph, B. (2014). Structural determinants allowing transferase activity in sensitive to freezing 2, classified as a family I glycosyl hydrolase. J. Biol. Chem. 289, 26089–26106. doi: 10.1074/jbc.M114.576694
Saita, E., Albanesi, D., and Mendoza, D. (2016). Sensing membrane thickness: lessons learned from cold stress. BBA 1861, 837–846. doi: 10.1016/j.bbalip.2016.01.003
Sakuma, Y., Liu, Q., Dubouzet, J. G., Abe, H., Shinozaki, K., and Yamaguchi-Shinozaki, K. (2002). DNA-binding specificity of the ERF/AP2 domain of Arabidopsis DREBs, transcription factors involved in dehydration- and cold-inducible gene expression. Biochem. Biophys. Res. Commun. 290, 998–1009. doi: 10.1006/bbrc.2001.6299
Shen, X., Liu, B., Xue, Z., Jiang, M., Lu, X., and Zhang, Q. (2019). Spatiotemporal variation in vegetation spring phenology and its response to climate change in freshwater marshes of Northeast China. Sci. Total Environ. 666, 1169–1177. doi: 10.1016/j.scitotenv.2019.02.265
Shi, P. X., Wang, M. L., Yu, H. B., Pan, D. C., Wu, Z. P., Wang, H. X., et al. (2009). Effect of low temperature at germination stage on seedlings of peanuts with different maturation degree. Crops 01, 78–81. doi: 10.16035/j.issn.1001-7283.2009.01.022
Song, Y. K., and Wang, D. P. (1979). A preliminary study on the effect of temperature on flowering and pod formation of peanut (Arachis hypogaea L.). Chin. J. Oil Crop Sci. 01, 32–37.
Sorensen, R. B., Nuti, R. C., and Butts, C. L. (2009). Yield and plant growth response of peanut to midseason forage harvest. Agron. J. 101:5.
Sui, N., Wang, Y., Liu, S., Yang, Z., Wang, F., and Wan, S. (2018). Transcriptomic and physiological evidence for the relationship between unsaturated fatty acid and salt stress in peanut. Front. Plant Sci. 9:7. doi: 10.3389/fpls.2018.00007
Sun, W., Li, Y., Zhao, Y., and Zhang, H. (2015). The TsnsLTP4, a nonspecific lipid transfer protein involved in wax deposition and stress tolerance. Plant Mol. Biol. Rep. 33, 962–974. doi: 10.1007/s11105-014-0798-x
Tan, W. J., Yang, Y. C., Zhou, Y., Huang, L. P., Xu, L., Chen, Q. F., et al. (2018). Diacylglycerol acyltransferase and diacylglycerol kinase modulate triacylglycerol and phosphatidic acid production in the plant response to freezing stress. Plant Physiol. 177, 1303–1318. doi: 10.1104/pp.18.00402
Tang, G., Liu, Z., Xu, P., Zhao, X., and Shan, L. (2013). Cloning and functional analysis of diacylglycerol acyltransferase gene in Arachis hypogaea. Acta Bot. Boreali Occidentalia Sin. 33, 857–863. doi: 10.1371/journal.pone.0105834
Tang, S. (2007). Cloning and expression analysis of three cDNAs encoding omega -3 fatty acid desaturases from Descurainia sophia. Biotechnol. Lett. 29, 1417–1424. doi: 10.1007/s10529-007-9391-9
Tang, Y. Y. (2011). Screening of Peanut Genotypes for Low Temperature Tolerance and Identification of Low Temperature Responsive Genes. Qingdao: Ocean University of China.
Testerink, C., and Munnik, T. (2011). Molecular, cellular, and physiological responses to phosphatidic acid formation in plants. J. Exp. Bot. 62, 2349–2361. doi: 10.1093/jxb/err079
Thomas, A. H., Catala, A., and Vignoni, M. (2016). Soybean phosphatidylcholine liposomes as model membranes to study lipid peroxidation photoinduced by pterin. Biochim. Biophys. Acta Biomembr. 1858, 139–145. doi: 10.1016/j.bbamem.2015.11.002
Tian, X., Liu, Y., Huang, Z., Duan, H., Tong, J., He, X., et al. (2015). Comparative proteomic analysis of seedling leaves of cold-tolerant and -sensitive spring soybean cultivars. Mol. Biol. Rep. 42, 581–601. doi: 10.1007/s11033-014-3803-4
Tovuu, A., Zulfugarov, I. S., Wu, G., Kang, I. S., Kim, C., Moon, B. Y., et al. (2016). Rice mutants deficient in ω-3 fatty acid desaturase (FAD8) fail to acclimate to cold temperatures. Plant Physiol. Biochem. 109, 525–535. doi: 10.1016/j.plaphy.2016.11.001
Upadhyaya, H. D., Ortiz, R., Bramel, P. J., and Singh, S. (2003). Development of a core collection using taxonomical, geographical and morphological descriptors. Genet. Res. Crop Evol. 50, 139–148. doi: 10.1023/A:1022945715628
Upadhyaya, H. D., Reddy, L. J., Dwivedi, S. L., Gowda, C. L. L., and Singh, S. (2009). Phenotypic diversity in cold-tolerant peanut (Arachis hypogaea L.) germplasm. Euphytica 165, 279–291. doi: 10.1007/s10681-008-9786-2
Upadhyaya, H. D., Reddy, L. J., Gowda, C. L. L., and Singh, S. (2006). Identification of diverse groundnut germplasm: sources of early maturity in a core collection. Field Crop Res. 97, 261–271. doi: 10.1016/j.fcr.2005.10.010
Vu, H. S., Shiva, S., Hall, A. S., and Welti, R. (2014). A lipidomic approach to identify cold-induced changes in Arabidopsis membrane lipid composition. Methods Mol Biol. 1166, 199–215. doi: 10.1007/978-1-4939-0844-8_15
Wan, L., Wu, Y., Huang, J., Dai, X., Yong, L., Yan, L., et al. (2014). Identification of ERF genes in peanuts and functional analysis of AhERF008, and AhERF019, in abiotic stress response. Funct. Integr. Genomic. 14, 467–477. doi: 10.1007/s10142-014-0381-4
Wang, C., Cheng, B., Zheng, Y., Sha, J., Li, A., and Sun, X. (2003). Effects of temperature to seed emergence, seedling growth and anthesis of peanut. J. Peanut Sci. 32, 7–11. doi: 10.14001/j.issn.1002-4093.2003.04.002
Wang, D. Z., Jin, Y. N., Ding, X. H., Wang, W. J., Zhai, S. S., Bai, L. P., et al. (2017). Gene regulation and signal transduction in the ICE-CBF-COR signaling pathway during cold stress in plants. Biochemistry 82, 1444–1462. doi: 10.1134/s0006297917100030
Wang, Y., Zhang, X., Zhao, Y., Prakash, C. S., He, G., and Yin, D. (2015). Insights into the novel members of the FAD2 gene family involved in high-oleate fluxes in peanut. Genome 58, 375–383. doi: 10.1139/gen-2015-0008
Wei, L. (2012). Cloning and Functional Analysis of ARACHIDONYL Carrier Protein Gene in Peanut. Jinan: Shandong Normal University.
Wen, S., Liu, H., Li, X., Chen, X., Hong, Y., Li, H., et al. (2018). TALEN-mediated targeted mutagenesis of fatty acid desaturase 2 (FAD2) in peanut (Arachis hypogaea L.) promotes the accumulation of oleic acid. Plant Mol. Biol. 97, 177–185. doi: 10.1007/s11103-018-0731-z
Wu, L., Li, F., Wu, X., Lin, S., and Wang, M. (2015). Cloning and expression characteristics of one -3 cis15fatty acid dehydrogenase gene AhFAD3A in Arachis hypogaea L. Chin. J. Oil Crop Sci. 37, 41–47. doi: 10.7505/j.issn.1007-9084.2015.01.007
Yan, B., Xu, X., Gu, Y., Zhao, Y., Zhao, X., He, L., et al. (2018). Genome-wide characterization and expression profiling of diacylglycerol acyltransferase genes from maize. Genome 61, 735–743. doi: 10.1139/gen-2018-0029
Yang, X., Xu, Z., Zuo, D., and Cai, S. (2018). Assessment on the risk of agricultural drought disaster in the three provinces of Northeast China. Acta Geogr. Sin. 73, 1324–1337. doi: 10.11821/dlxb201807011
Yu, M., Li, Q., Hayes, M. J., Svoboda, M. D., and Heim, R. R. (2014). Are droughts becoming more frequent or severe in China based on the standardized precipitation evapotranspiration Index: 1951–2010. Int. J. Climatol. 34, 545–558. doi: 10.1002/joc.3701
Yu, S. (2008). Cloning and Expression Analysis of the Key Enzymes in Fatty Acid Metabolism of Peanut. Nanjing: Nanjing Agricultural University.
Yurchenko, O. P., Park, S., Ilut, D. C., Inmon, J. J., Millhollon, J. C., Liechty, Z., et al. (2014). Genome-wide analysis of the omega-3 fatty acid desaturase gene family in Gossypium. BMC Plant Biol. 14:312. doi: 10.1186/s12870-014-0312-5
Zhang, F., Zhu, X. Q., Guo, Y. L., Wan, X. Q., Lin, T. T., Chen, Q. B., et al. (2014). Ultrastructural changes and dynamic expressions of FAD7, Cu/Zn-SOD, and Mn-SOD, in Neosinocalamus affinis, under cold stress. Russ. J. Plant Physiol. 61, 760–767. doi: 10.1134/S1021443714050173
Zhang, G., Chen, M., Chen, X., Xu, Z., Guan, S., Li, L. C., et al. (2008). Phylogeny, gene structures, and expression patterns of the ERF gene family in soybean (Glycine max L.). J. Exp. Bot. 59, 4095–4107. doi: 10.1093/jxb/ern248
Zhang, X., Wan, Q., Liu, F., Zhang, K., Sun, A., Luo, B., et al. (2015). Molecular analysis of the chloroplast Cu/Zn-SOD gene (AhCSD2) in peanut. Crop J. 3, 246–257. doi: 10.1016/j.cj.2015.03.006
Zhang, Y., Wang, S., and Feng, J. (2018). Drought events and its causes in 2017 in China. J. Arid Meteorol. 36, 331–338.
Zhao, X., Li, C., Wan, S., Zhang, T., Yan, C., and Shan, S. (2018). Transcriptomic analysis and discovery of genes in the response of Arachis hypogaea to drought stress. Mol. Bio. Rep. 45, 119–131. doi: 10.1007/s11033-018-4145-4
Zhong, P., Liu, J., Wang, J., and Chang, B. (2018). Physiological responses and cold resistance evaluation of peanut under low-temperature stress. J. Nuclear Agric. Sci. 32, 1195–1202. doi: 10.11869/j.issn.100-8551.2018.06.1195
Zhuang, J., Chen, J. M., Yao, Q. H., Xiong, F., Sun, C. C., Zhou, X. R., et al. (2011). Discovery and expression profile analysis of AP2/ERF family genes from Triticum aestivum. Mol. Biol. Rep. 38, 745–753. doi: 10.1007/s11033-010-0162-7
Keywords: peanut, cold stress, membrane lipid metabolism, molecular mechanism, lipid signal transduction
Citation: Zhang H, Dong J, Zhao X, Zhang Y, Ren J, Xing L, Jiang C, Wang X, Wang J, Zhao S and Yu H (2019) Research Progress in Membrane Lipid Metabolism and Molecular Mechanism in Peanut Cold Tolerance. Front. Plant Sci. 10:838. doi: 10.3389/fpls.2019.00838
Received: 11 April 2019; Accepted: 12 June 2019;
Published: 27 June 2019.
Edited by:
Jose C. Jimenez-Lopez, Consejo Superior de Investigaciones Científicas (CSIC) Granada, SpainReviewed by:
Hassan Iqbal, Xinjiang Institute of Ecology and Geography (CAS), ChinaRenu Deswal, University of Delhi, India
Copyright © 2019 Zhang, Dong, Zhao, Zhang, Ren, Xing, Jiang, Wang, Wang, Zhao and Yu. This is an open-access article distributed under the terms of the Creative Commons Attribution License (CC BY). The use, distribution or reproduction in other forums is permitted, provided the original author(s) and the copyright owner(s) are credited and that the original publication in this journal is cited, in accordance with accepted academic practice. No use, distribution or reproduction is permitted which does not comply with these terms.
*Correspondence: Haiqiu Yu, yuhaiqiu@syau.edu.cn