Corrigendum: Accumulation of Anthocyanins: An Adaptation Strategy of Mikania micrantha to Low Temperature in Winter
- 1Guangzhou Key Laboratory of Subtropical Biodiversity and Biomonitoring, Guangdong Provincial Key Laboratory of Biotechnology for Plant Development, School of Life Sciences, South China Normal University, Guangzhou, China
- 2College of Life Science, Zhao Qing University, Zhaoqing, China
The accumulation of anthocyanins in leaves and stems of Mikania micrantha improves its adaptability to low-temperature environments during winter in areas where this species is invasive. The accumulation of anthocyanins in M. micrantha causes the plants to exhibit red coloration when encountering low-temperature environments during winter. Many studies have reported that the accumulation of anthocyanins near the plant surface filters light and improves photoprotection. However, the results of this study showed that the main role of anthocyanins accumulation in M. micrantha during winter was to increase both antioxidant capability and tolerance to low temperature. The results showed that the anthocyanin contents were significantly higher in red leaves and stems than in green leaves and stems, with more than 60-fold greater content in red leaves than in green leaves. In addition, the total antioxidant capability was significantly greater in red leaves and stems than in green leaves and stems. After 4°C treatment for 12 h, a large amount of reactive oxygen species accumulated in green leaves and stems, and the maximum photochemical efficiency decreased significantly. Compared with that of the green leaves, the net photosynthetic rate of red leaves was significantly higher. The biomass statistics revealed that the dry matter accumulation of M. micrantha plants with relatively large amounts of anthocyanins was significantly greater than that of plants with relatively low anthocyanin levels during the same period. Our results suggest that the accumulation of anthocyanins during winter is an adaptation strategy of M. micrantha to low winter temperatures.
Introduction
Low-temperature stress is considered the main cause of plant growth constraints and crop yield reductions (Jin et al., 2017; Sun et al., 2018). Low-temperature stress can damage the photosynthetic apparatus and reduce photosynthetic efficiency (Yang et al., 2016), induce oxidative stress, and lead to the generation of reactive oxygen species (ROS) in tissues, reduce the fluidity of membranes (Gusta and Wisniewski, 2013), induce the degradation of proteins (Ruelland et al., 2009), and cause the inhibition of the activity of antioxidant enzymes (Baek and Skinner, 2003). Higher plants have developed many strategies to reduce the damage caused by low-temperature stress (Chinnusamy et al., 2007). Under low-temperature conditions, some plant species present tolerance mechanisms in a genotype-, organ-, or environment-specific manner via various complex networks of metabolic pathways (Zhang et al., 2014). Plants can improve their membrane fluidity by increasing the amount of unsaturated fatty acids. The accumulation of nonenzymatic substances (such as anthocyanins, phenylpropanoids, and terpenoids) increases antioxidant capabilities, thereby reducing oxidative stress caused by low temperature (Arnholdt-Schmitt et al., 2006; Sivankalyani et al., 2016). Moreover, anthocyanin accumulate increased significantly in flowers, seeds, fruits, and vegetative tissues in response to low-temperature stress (Allan et al., 2008; Liu et al., 2018).
Anthocyanins are natural, water-soluble pigments that belong to the flavonoid family of plant secondary metabolites. Anthocyanins are ubiquitous in flowers, fruits, stems, and leaves of plants (Tanaka and Ohmiya, 2008). The function of anthocyanin in plants involves protection against various biotic and abiotic stresses (Ahmed et al., 2014). Various environmental factors such as nutrition, drought, light, and temperature affect the accumulation of anthocyanins in many plant species (Allan et al., 2008). In the anthocyanin biosynthetic pathway, chalcone synthase (CHS), chalcone isomerase (CHI), flavanone 3-hydroxylase (F3H), dihydroflavonol 4-reductase (DFR), and anthocyanidin synthase (ANS) are the main enzymes controlling the synthesis of anthocyanins (Tanaka et al., 2008; Liu et al., 2018). Anthocyanins can protect plants against UV light, pathogens, and low temperature (Field et al., 2001; Sivankalyani et al., 2016). Low-temperature conditions can cause oxidative stress in plants. Anthocyanins exhibit antioxidant activity and can improve the antioxidant capability of plants (Pojer et al., 2013). Under low-temperature stress, the expression of genes related to anthocyanin synthesis in plants significantly increases, and anthocyanin accumulation increases, which improves plant tolerance to low temperature (Lo Piero et al., 2005; Naing et al., 2018). In woody plants, the accumulation of anthocyanin in young leaves during winter can reduce oxidative damage and increase photosynthetic rates (Zhu et al., 2018).
Mikania micrantha HBK belongs to the Asteraceae family and is a fast-growing and rapidly reproducing perennial creeping vine indigenous to Central and South America. M. micrantha is among the top 100 invasive plants worldwide (Holm et al., 1977; Yang et al., 2005). It is currently considered an invasive species in Southeast Asia and the Pacific region, including in South China (Wang et al., 2016). Substantial damage to agriculture, forestry, and ecological balance has occurred because of the rapid spread of M. micrantha in South China (Zhang et al., 2004). As a creeping vine, M. micrantha covers trees and grasses and ultimately kills them by depriving them of their light source (Siwakoti, 2007). M. micrantha is a thermophilic and sun-loving plant species. However, in South China, where M. micrantha is invasive, it also encounters a low-temperature environment (below 15°C) during winter (Yan et al., 2013); in the field, most of the M. micrantha plants had accumulated anthocyanins and turned red during the winter. This study aimed to elucidate the role of anthocyanins in the invasive capability of M. micrantha during winter.
Materials and Methods
Plant Materials
In September, M. micrantha was planted via vegetative reproduction in the biological garden of South China Normal University, Guangzhou, China. Branches of M. micrantha without leaves were cut into segments that had two stem nodes; the segments were subsequently planted into soft soil that was tilled in accordance with the cutting method. The planting area was maintained with routine weeding and watering. In December, the same batch of M. micrantha planted in September exhibited different colors, with some plants having turned red and some remaining green. The stems and leaves of different-colored plants were selected as research materials. Three to 15 biological replicates were incorporated in this study.
Pigment Estimation
Anthocyanins were extracted from 0.05 g of fresh leaves or stems in 2 ml of a methanol:HCl (99:1, v/v) solution at 4°C in the dark for 24 h (Manetas et al., 2003; Hughes et al., 2007). Chlorophyll (Chl) was removed from the extracts by the addition of 2 ml of chloroform and 1 ml of purified water. After the solution was mixed, the anthocyanins dissolved in the upper water-methanol phase, and the Chl dissolved in the lower chloroform phase. The absorption spectra of the anthocyanin extracts at 420–700 nm were recorded against that of methanol:HCl (99:1, v/v) as a blank using a UV-Vis 2450 spectrophotometer (Shimadzu, Tokyo, Japan). The absorbance at 530 nm was used to calculate the anthocyanin content, and different concentrations of cyanidin-3-glucoside were used as standards.
The Chl was extracted from 0.05 g of fresh leaves in 4 ml of an 80% acetone solution at 4°C in the dark for 24 h. The absorbance of the Chl extracts was recorded using a UV-Vis 2450 spectrophotometer (Shimadzu, Tokyo, Japan), and the Chl content was calculated according to the methods of Wellburn (1994).
Total phenols were extracted from 0.05 g of fresh leaves or stems in 2 ml of a 95% methanol solution at 4°C in the dark for 24 h. The total phenol content was measured according to the Folin–Ciocalteu method as described by Ainsworth and Gillespie (2007). First, 1 ml of 10% Folin–Ciocalteu reagent and 2 ml of 0.7-M Na2CO3 were added to a 0.5-ml sample. The absorbance of the mixture was subsequently measured at 765 nm (UV-2450, Shimadzu, Kyoto, Japan) after it was mixed for 5 min. Different concentrations of gallic acid were used as standards.
Flavonoids were extracted from 0.05 g of fresh leaves or stems in the same way as the total phenols were and measured according to the method described by Heimler et al. (2005), with slight modifications. One hundred fifty microliters of the sample, 1.85 ml of deionized water, 0.2 ml of 5% NaNO2, 0.3 ml of 10% AlCl3 (freshly prepared), and 1 ml of 1-M NaOH solution were added to a 5-ml centrifuge tube. The absorbance of the mixture was measured at 510 nm (UV-2450, Shimadzu, Kyoto, Japan) after it was mixed for 5 min. Different concentrations of catechin were used standards.
Total Antioxidant Capability Determination
The total antioxidant capability was evaluated by a 1,1-diphenyl-2-picrylhydrazyl (DPPH) test according to the method of Saha et al. (2008), with slight modifications. At 4°C in the dark for 24 h, 0.05 g of fresh leaves or stems were placed in 2 ml of a 95% methanol solution. For 5 min in the dark, 0.1 ml of the sample was then added to 2.9 ml of 120-μM DPPH (dissolved in 95% methanol). The mixture was subsequently measured at 517 nm (UV-2450, Shimadzu, Kyoto, Japan). Different concentrations of DPPH were used as standards, and the free radical-scavenging capability of the mixture was calculated according to the absorbance of the mixture at 517 nm.
Gene Expression Analysis
Total RNA from the leaf samples was extracted using TRIzol reagent (Invitrogen, MA, USA) according to the manufacturer’s instructions. Complementary DNA was synthesized using TopScript™ RT DryMIX (dT18) (Enzynomic, Daejeon, Korea) according to the manufacturer’s instructions. Quantitative reverse transcription polymerase chain reaction analysis was performed using a SYBR Premix Ex Taq™ II Kit (Takara) in conjunction with a Bio-Rad CFX96 Real-Time PCR System (CFX96, Bio-Rad, USA). Actin was used as a reference gene; the primer pairs for which were 5′-TGAAATACCCCATTGAGCATGG-3′ (forward) and 5′-GAATCCAGTACAATACCTGTGGTAG-3′ (reverse). The relative expression of the anthocyanin biosynthetic pathway genes chalcone synthase (CHS), chalcone isomerase (CHI), flavanone 3-hydroxylase (F3H), dihydroflavonol 4-reductase (DFR), and anthocyanidin synthase (ANS) was measured. The primers for real-time PCR were as follows: 5′-ACATGCCTGGTGCAGATTACCA-3′ (forward) and 5′-AAGTGGGAATCGGAAGGTCCAC-3′ (reverse) for CHS; 5′-GGAGGCGGTTCTGGAATCTATC-3′ (forward) and 5′-TCGTCCTTGTTCTTCATCATTAGC-3′ (reverse) for CHI; 5′-TTGCAGGCCAGGCCCATT-3′ (forward) and 5′-TGCAAGATTGGAGGGAGATTGT-3′ (reverse) for F3H; 5′-AGCTTTGATGAAGCCATTSAAGGTTGC-3′ (forward) and 5′-TTCTTCACTGTCTTGGCTTT-3′ (reverse) for DRF; and 5′-TCAGCCGGTTGAAGAGAAGGAG-3′ (forward) and 5′-GAGGGCCAAATGGTCAAATCACGT-3′ (reverse) for ANS. The 10-μl reaction mixture consisted of 5 μl of 2× Premix Ex Taq II, 0.4 μl of the forward primer (10 μM), 0.4 μl of the reverse primer (10 μM), 0.5 μl of complementary DNA template (< 100 ng), and 3.7 μl of ddH2O. The cycle conditions were as follows: initial denaturation at 95°C for 30 s; 40 cycles at 95°C for 5 s and 64°C for 34 s; 1 cycle at 95°C for 15 s (for recording a melting curve); and one cycle at 64°C for 1 min. The relative gene transcript levels were calculated using the 2-ΔΔCT method (Livak and Schmittgen, 2001).
Rubisco Protein
Fresh leaves (0.1 g) were weighed, placed in a precooled mortar (with a small amount of inert quartz) on ice, and homogenized in 1.5 ml of 60-mM Tris-HCl (pH 7.8) buffer containing 5% (w/v) polyvinylpyrrolidone, 0.1% (w/v) NaCl, and 2% (v/v) glycerol. The grinding fluid was poured into a 2-ml centrifuge tube, which was subsequently centrifuged at 13,000×g for 10 min at 4°C; the total protein was contained within the supernatant. To analyze the Rubisco content, 0.1 ml of the supernatant was added to an equal volume of protein loading buffer, which was then incubated at 100°C for 5 min after it was mixed. The 12.5% sodium dodecyl sulfate polyacrylamide gel electrophoresis (SDS-PAGE) gel was made according to the methods of Zhang et al. (2016). Each 20-μl sample was loaded in the wells of the gel, and the Rubisco protein was separated by SDS-PAGE with a Mini-PROTEAN 3 system (Bio-Rad, USA). The large and small subunits of Rubisco were recognized according to their molecular mass and the included marker stained by Coomassie Brilliant Blue R-250. The Rubisco large subunit (LS) was identified using rabbit antibodies against the Rubisco protein. After being separated on a 12.5% SDS-PAGE gel, the total proteins were blotted onto a polyvinylidene fluoride (PVDF) membrane, and then, the PVDF membrane was stained by Ponceau S for 10 min. The results stained by Ponceau S were recorded with digital camera, and then, the PVDF membrane was washed three times (5 min each) in Tris-buffered saline with Tween 20 (TBST) buffer [8.8 g of NaCl, 20 ml of 1 M Tris-HCl (pH = 8.0), and 0.5 ml of Tween 20 added to 1 l of water]. The PVDF membrane containing the blots was subsequently preincubated in TBST buffer that contained 5% nonfat powdered milk for 1.5 h at room temperature. The preincubated PVDF membrane was washed three times (3 min each) in TBST buffer and then incubated with anti-Rubisco antibody (1:1,000 dilution) (Bioss, Beijing, China) for 1 h at room temperature. The incubated PVDF membrane was washed three times (5 min each) in TBST buffer and then incubated for 50 min with goat anti-rabbit HRP-conjugated secondary antibody (1:3,000 dilution) at room temperature, after which the membrane was washed another three times (5 min each) in TBST buffer. The anti-Rubisco labeling of the blots was visualized via chemiluminescent HRP substrate (Millipore, Billerica, USA) and imaged with a Tanon 5200 system (Tanon, Shanghai, China).
Chlorophyll Fluorescence Determination
A Chl fluorescence imaging system (Technologica, UK) was used to measure Chl fluorescence. Leaves and stems were removed from M. micrantha plants growing at 4°C for 12 h, and a room temperature treatment was used as a control (CK). The leaves and stems were then placed in the dark for 30 min. The minimum fluorescence (F0) and the maximum fluorescence (Fm) of the leaves and stems were measured using a 6,000 μmol m–2 s–1 saturating pulse. The maximum photochemical efficiency (Fv/Fm) of photosystem II (PSII) was calculated as Fv/Fm = (Fm – F0)/Fm (Oxborough and Baker, 1997).
Gas Exchange
Gas exchange was measured using an LI-6800 Portable Photosynthesis System (LI-COR, Inc., USA) in the morning (9:00–11:00) on sunny days in accordance with the method described by Mega et al. (2019), with moderate adjustments. The gas exchange of fully expanded mature M. micrantha leaves was measured at an irradiance of 800 μmol m–2 s–1. The ratio of the red and blue light of the irradiance in the leaf measurement chamber was set to 9:1, the mean relative air humidity was 45%, and the corresponding mean temperature was 20°C. The net photosynthetic rate (Pn), intercellular CO2 concentration (Ci), stomatal conductance (Gs), and transpiration rate (Tr) were recorded when they were relatively stable.
Stomatal Observations
The stomata were measured in accordance with the method described by Zhang et al. (2016), with slight modifications. The leaves were cut into 2 × 2-mm fragments and put into a fixing solution that consisted of 2.5% glutaraldehyde and 2% polyformaldehyde at 4°C for more than 12 h. The leaf fragments were then dehydrated with an ethanol concentration gradient (30–100%) every 20 min. The dehydrated leaf fragments were critical-point dried with CO2 and then sputtered with a 30-nm gold layer. The stomata were subsequently observed via a scanning electron microscope (SEM) (Q25, FEI, USA).
Hydrogen Peroxide and Superoxide Histochemical Staining
3,3’-Diaminobenzidine (DAB) and nitroblue tetrazolium (NBT) staining were performed to detect the accumulation of hydrogen peroxide and superoxide, respectively (Liu et al., 2007). After, they were treated at 4°C for 12 h; leaves and stems were dipped quickly into potassium phosphate buffer (50 mM, pH 7.0) that contained 0.5 mg ml−1 DAB and potassium phosphate buffer (50 mM, pH 6.4) that contained 1 mg ml−1 NBT under vacuum for 30 min, after which they were incubated at room temperature for 8 or 12 h (stained with DAB or NBT, respectively) in the dark. Subsequently, methanol:HCl (99:1, v/v) and 80% acetone solutions were used to clarify anthocyanins and Chl in the stained plant samples, respectively. Images of the stained plant samples were taken with a digital camera.
Biomass Statistics
The red and green branches of M. micrantha were marked. After 10 days, the marked branches were removed, heated at 105°C for 20 min, and then, dried at 80°C for 72 h. The M. micrantha dry matter that accumulated during 10 days was determined via an electronic balance. The diameter of M. micrantha was measured using a Vernier caliper.
Statistical Analysis
Statistical significance was determined by one-way analysis of variance (ANOVA) followed by Duncan’s post hoc test or Student’s t-test using SPSS Statistics 19.0 (IBM, NY, USA). The means were considered significantly different at P < 0.05. SigmaPlot 12.5 (Systat Software Inc., USA) was used to conduct linear regression analysis and to plot the data. All the data are shown as the means ± standard errors (SEs).
Results
Accumulation of Anthocyanins
During winter, M. micrantha exhibited different colors, with some plants turning red and some remaining green (Figure 1A). The spectral absorption peaks of the anthocyanin extracts from the leaves and stems occurred at 530 nm (Figure 1B), demonstrating the presence of anthocyanins. The anthocyanin contents in red leaves and stems were significantly greater than those in green leaves and stems, and the highest anthocyanin content was detected in red leaves (Figure 1C). The anthocyanin content in red leaves was more than 60 times that in green leaves, and the content in red stems was more than six times that in green stems. The contents of flavonoids and total phenols were the same: these contents were greatest in red leaves, lowest in green stems, and moderate in both red stems and green leaves, and there was no significant difference in flavonoid or total phenol contents between red stems and green leaves (Figures 1D, E). The total antioxidant capability of red leaves and stems was significantly greater than that of green leaves and stems, and the total antioxidant capability of the leaves was significantly greater than that of the stems (Figure 1F). The expression of genes related to anthocyanin synthesis (CHS, CHI, F3H, DFR, and ANS) in red leaves and stems was significantly greater than that in green leaves and stems, and the expression of the downstream gene ANS in red leaves and stems was nearly 1,000 times greater than that in green leaves and stems. These results indicated that anthocyanins were synthesized vigorously in red leaves (Figures 1G–K). A schematic of anthocyanin biosynthesis is shown in Figure 1L.
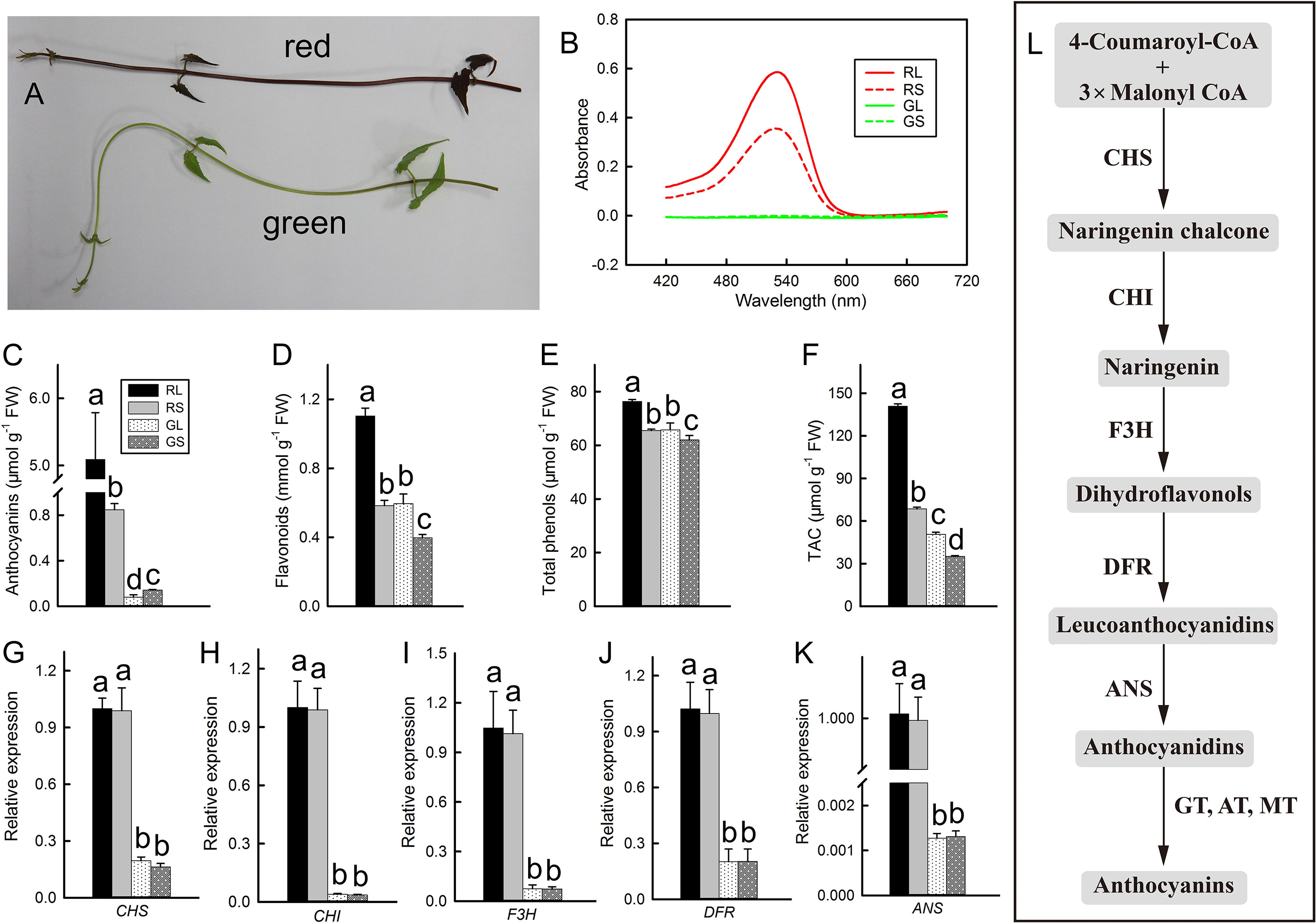
Figure 1 Phenotypes of different-colored M. micrantha (A). Absorbance spectra of anthocyanin extracts from red leaves (RL), red stems (RS), green leaves (GL), and green stems (GS) of M. micrantha (n = 5) (B). Contents of anthocyanins (C), flavonoids (D), and total phenols (E) and the total antioxidant capability (F) in the RL, RS, GL, and GS of M. micrantha (n = 8). Relative expression of the anthocyanin biosynthetic pathway genes chalcone synthase (CHS), chalcone isomerase (CHI), flavanone 3-hydroxylase (F3H), dihydroflavonol 4-reductase (DFR), and anthocyanidin synthase (ANS) (G–K, respectively) in the RL, RS, GL, and GS of M. micrantha (n = 6). The actin gene was used for normalization. The schematic picture of anthocyanin biosynthesis (L). The error bars represent the standard errors (SEs) of five to eight biological replicates. One-way analysis of variance (ANOVA) was used to evaluate the statistical significance; the bars with different letters (a, b, c, d) indicate significant differences between the means (least significant difference, P < 0.05).
Chl Fluorescence and Localization of Reactive Oxygen Species
According to the previously discussed results, M. micrantha exhibited different colors under low temperatures during winter: red and green. The accumulation of anthocyanins in leaves is associated with resistance to low-temperature stress (Ahmed et al., 2014). To detect differences in low-temperature tolerance between the red and green M. micrantha plants, we treated the two different-colored M. micrantha plants with low temperature (4°C). The value of Fv/Fm in plants will decrease significantly after stress. In this study, the Chl fluorescence results revealed no significant differences in the Fv/Fm values between the red and green tissue in the control group. Compared with the control group, the value of Fv/Fm in red leaves had no significant decline, and it decreased significantly in red stems after 4°C treatment. The value of Fv/Fm in green leaves and stems decreased significantly after 4°C treatment (Figures 2A, B). These results suggest that anthocyanins can improve plant tolerance to low temperature. DAB and NBT were used to detect the localization of hydrogen peroxide and superoxide anions in the leaves and stems of M. micrantha after 4°C treatment, respectively. The accumulation of hydrogen peroxide and superoxide anions was relatively low in red leaves and stems, but a large amount of hydrogen peroxide and superoxide anions accumulated in green leaves and stems (Figures 2C, D).
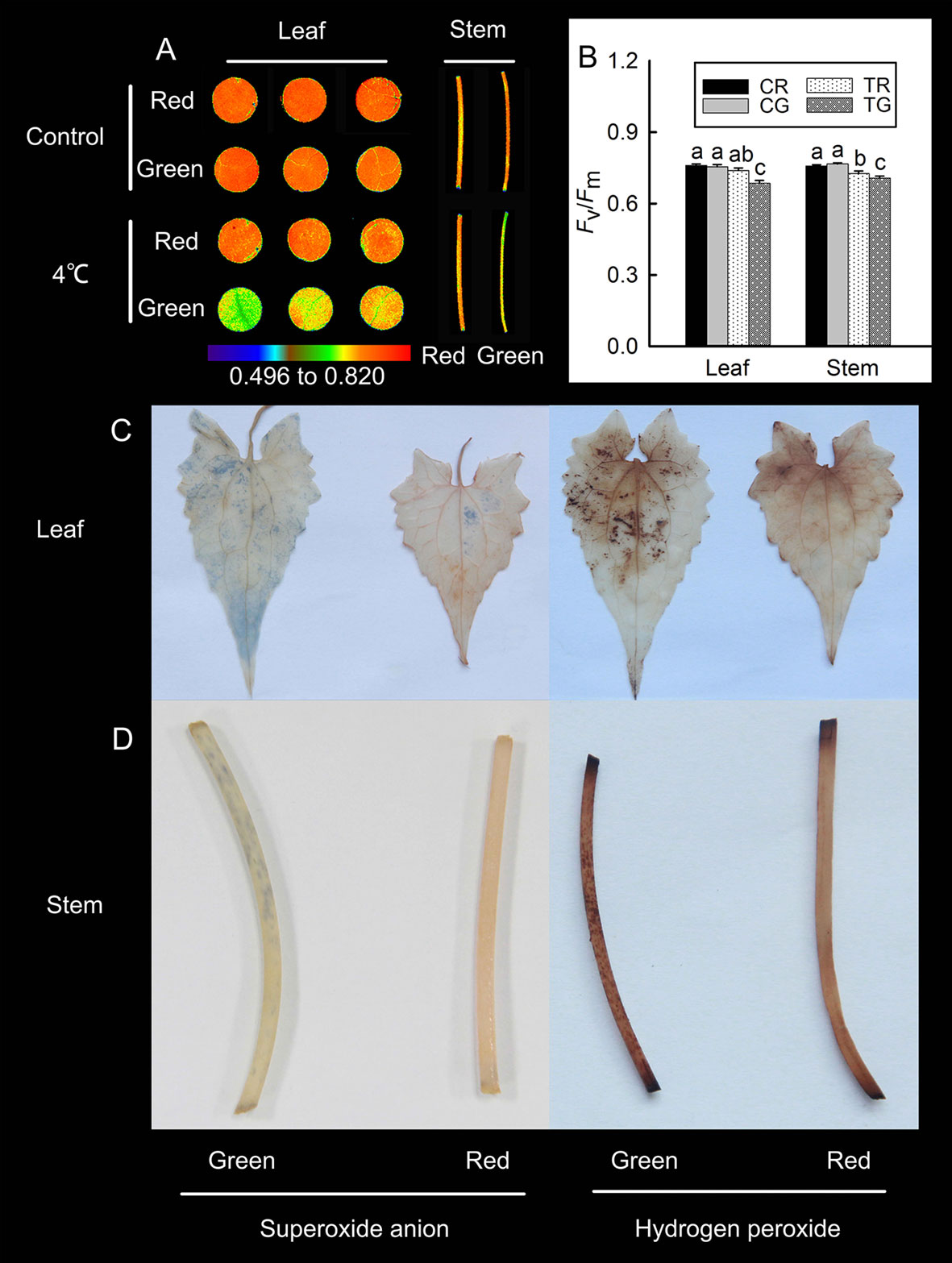
Figure 2 Changes in the maximum photochemical efficiency (Fv/Fm) of the red leaves (RL), red stems (RS), green leaves (GL), and green stems (GS) of M. micrantha after 4°C treatment (A), the Fv/Fm value of red and green in control (CR, CG) and 4°C treatment (TR, TG) (B). Tissue localization of superoxide anions and hydrogen peroxide in the RL, RS, GL, and GS of M. micrantha was observed after 4°C treatment (C, D). The error bars represent the standard errors (SEs) of three biological replicates. One-way analysis of variance (ANOVA) was used to evaluate the statistical significance; the bars with different letters (a, b, c, d) indicate significant differences between the means (least significant difference LSD, P < 0.05).
Gas Exchange Parameters and Biomass
The previously discussed results showed that, compared with green M. micrantha plants, red plants could tolerate lower temperature. Both the accumulation of anthocyanins in leaves and low temperature are associated with photosynthetic capability (Hughes et al., 2007; Sun et al., 2018). Photosynthetic capability is the basis of the biomass accumulation of M. micrantha. Whether this species can accumulate additional biomass during winter is a reflection of its adaptability to low-temperature environments. Therefore, we compared indicators related to photosynthesis. Stomatal aperture directly affects leaf gas exchange. The stomata of M. micrantha leaves were comparatively examined by SEM. During winter, the stomata of green leaves were not fully open and most of them were even closed, while the stomata of red leaves were partially open. To understand their relevance with other physiological activities, the density and aperture of stomata were further estimated. The resulting data showed that the density and aperture of stomata on red leaves were higher and larger than those on green leaves (Figures 3A and B). Compared with that of green leaves, the stomatal aperture of red leaves is relatively large. The Gs parameters related to stomatal aperture showed that Gs were significantly greater in red leaves than in green leaves (Figure 3E). The results concerning the Pn and Tr were similar to those concerning Gs, and all three parameters were significantly greater in the red leaves than in the green leaves (Figures 3C, D). In contrast, the Ci results were different from those of the other gas exchange parameters. The Ci of the green leaves was significantly greater than that of the red leaves (Figure 3F). The contents of Chl and Rubisco, both of which are related to photosynthesis, were relatively low in the green leaves (Figures 3G, H), which were consistent with the results of the Pn. Western blotting analysis on Rubisco LS (Figure 3J) and the result of Western blot membrane stained by Ponceau S (Figure 3I) were consistent with SDS-PAGE analysis (Figure 3H). The stem diameter and biomass results showed that the diameter of the red stems was significantly greater than that of the green stems. The dry matter accumulation of the red M. micrantha plants during the same time period was also significantly greater than that of the green plants (Figures 3K, L).
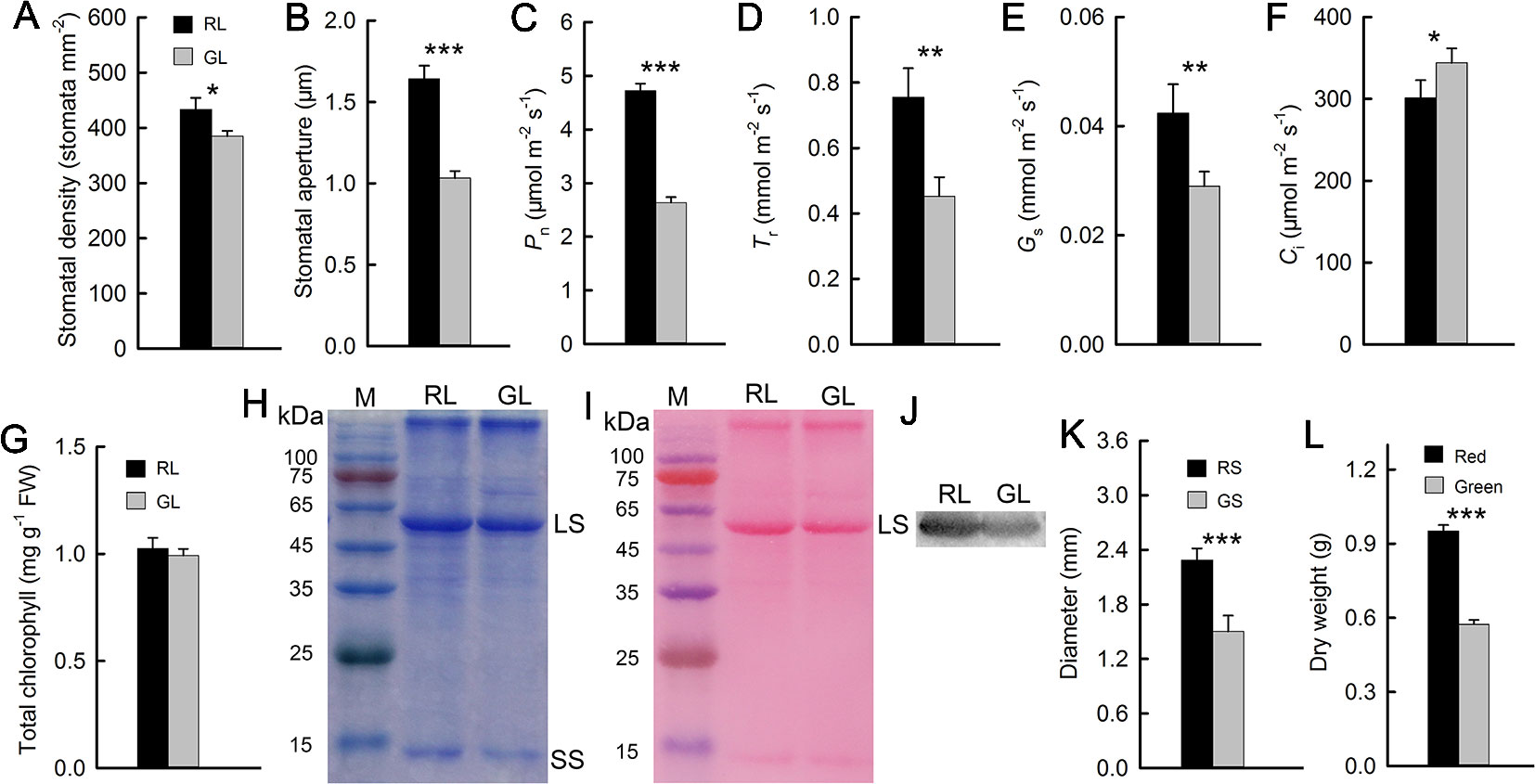
Figure 3 Stomatal density and aperture of red (RL) and green leaves (GL) (A, B). Gas exchange parameters of red and green leaves, including the net photosynthetic rate (Pn) (C), transpiration rate (Tr) (D), stomatal conductance (Gs) (E), and intercellular CO2 concentration (Ci) (F) (n = 10). Chlorophyll (Chl) content in RL and GL (G) (n = 8). Rubisco LS and small subunit (SS) were separated in 12.5% SDS-PAGE, and the polypeptides were stained by Coomassie Brilliant Blue R-250 (H). The membrane of the Western blot was stained by Ponceau S (I), and the Western blotting showed LS in RL and GL (J). Diameter of red (RS) and green stems (GS) (K) (n = 15) and dry weight of red and green M. micrantha plants (L) (n = 8). The error bars represent the standard errors (SEs) of 8 to 15 biological replicates, and the asterisks indicate significant differences (two-sided Student’s t-test, *P < 0.05, **P < 0.01, ***P < 0.001).
Discussion
As a highly invasive weed, M. micrantha is native to parts of South America that have a tropical, high-temperature environment. However, South China, one of the areas infested with M. micrantha, is a subtropical region with a low-temperature environment (below 15°C) during winter (Yan et al., 2013). To adapt to the low-temperature environment during winter, the leaves of M. micrantha plants exhibit a striking redness due to the presence of anthocyanins. Spectroscopic results show that the extracts from the stems and leaves of M. micrantha have an absorption peak at 530 nm, and the absorption peak of red M. micrantha plants is significantly higher than that of green M. micrantha plants. Anthocyanins are induced by a number of environmental factors, including high light and UV-B radiation and cold temperatures, and anthocyanins have been proposed to be important compounds involved in the abiotic stress tolerance response (Lorenc-Kukula et al., 2005; Zhang et al., 2016; Sivankalyani et al., 2016; Liu et al., 2018). There are two main functions of anthocyanins in plants: one involves photoprotection by functioning as a light screen, and the other involves antioxidation by scavenging ROS. The results of this study showed that anthocyanins in M. micrantha leaves were more likely to have antioxidant effects in winter.
Studies have shown that low temperatures can induce anthocyanin formation (Lo Piero et al., 2005; Li et al., 2017b; Carmona et al., 2017). During winter, a large amount of anthocyanins, total phenols, and flavonoids accumulated in red M. micrantha (Figures 1C–E). This result is consistent with that of woody plants during winter (Zhu et al., 2018). Low temperatures during winter lead to decreased fluidity of plant cell membranes, decreased activity of enzymes, oxidative stress, and the accumulation of ROS. As toxic substances, ROS can destroy biological macromolecules, attack cell membranes, and accelerate leaf damage. Anthocyanins, total phenols, and flavonoids can improve the antioxidant capability of plants. The results showed that the total antioxidant capability of the leaves and stems of red M. micrantha was significantly greater than that of green M. micrantha. A study of sweet potato showed that the hydrogen peroxide content in the leaves increased significantly and that membrane lipid peroxidation increased at 4°C (Jin et al., 2017). In the present study, red and green M. micrantha plants were placed in a 4°C incubator for 12 h. The results showed that more ROS (superoxide anions and hydrogen peroxide) accumulated in the stems and leaves of green M. micrantha than in red M. micrantha. This finding indicated that the accumulation of anthocyanins could effectively eliminate ROS under low-temperature stress.
To test the tolerance of the different phenotypes of M. micrantha to low-temperature stress, red and green M. micrantha plants were cultivated in a 4°C incubator. After 12 h, the Fv/Fm was measured using a Chl fluorescence imaging system (Technologica, UK). The results showed that the Fv/Fm value of the red leaves did not decrease significantly after low-temperature exposure; however, the Fv/Fm value of the red stems, green leaves, and green stems decreased significantly. The greatest decrease in Fv/Fm value was detected in green leaves and stems. The Fv/Fm is an important parameter of Chl fluorescence and does not change significantly when a plant is under normal conditions. However, the Fv/Fm will decrease significantly when a plant is under stress (drought, high light, low temperature, etc.) or is aging (Zhang et al., 2016; Guadagno et al., 2017; Jin et al., 2017; Yu et al., 2017; Sun et al., 2018). Thus, the results showed that anthocyanin accumulation could improve plant tolerance to low temperature.
Low temperatures limit photosynthesis in many plant species. As the only source of organic matter in higher plants, photosynthesis plays a decisive role in the accumulation of plant biomass. Low-temperature environments can reduce the activity of enzymes and affect the activity of Rubisco, which is related to photosynthesis, thus reducing the photosynthetic rate. Low temperature also decreases membrane fluidity, affects stomatal activity, and limits gas exchange. Comparing the Pn and Gs, we found that the values in red leaves were significantly higher than those in green leaves. The Gs of plant leaves is closely related to stomatal opening and closing. Studies have shown that under drought stress, stomatal closure can reduce the Gs of leaves (Li et al., 2017; Takahashi et al., 2018). In the present study, SEM revealed that the stomatal aperture of the red leaves was larger than that of the green leaves. Photosynthesis involves “energy capture” (light reactions), in which light energy is converted to chemical energy, and “energy utilization” (carbon reactions), in which chemical energy is used to convert CO2 to carbohydrates. Chl and Rubisco proteins play key roles in these two reactions, and their contents directly affect photosynthesis. In this experiment, Chl and Rubisco proteins in red leaves were significantly higher than those in green leaves, which was consistent with the Pn results. It has been reported that Chl and Rubisco protein contents in leaves are low and that the photosynthetic system is fragile when plant leaves are not fully mature. To reduce photooxidative damage, anthocyanin contents in leaves are increased (Zhang et al., 2016). Other studies have shown that plants grown in high-light environments present increased anthocyanin contents to filter excess light energy. The main role of anthocyanins in both cases is to reduce the absorption of light by leaves, resulting in a decrease in the photosynthetic rate of plant leaves (Lev-Yadun and Gould, 2009). However, the Pn and Chl and Rubisco protein contents in this study were significantly greater in red leaves than those in green leaves. This finding shows that anthocyanin function mainly as an antioxidant, which is consistent with results reported in mango (Sivankalyani et al., 2016).
As an invasive plant, the adaptation strategy of M. micrantha to its environment is obviously to increase its invasiveness. M. micrantha is known as “mile-a-minute grass,” so its rapid growth is a reflection of its strong invasiveness. Biomass accumulation depends on the rapid growth of plants. The results show that the biomass of red M. micrantha is significantly greater than that of green M. micrantha during the same period of winter. Moreover, the stems of red M. micrantha are thicker than those of the green type. The accumulation of anthocyanins in M. micrantha under low temperature during winter resulted in improved antioxidant capability and reduced oxidative stress, increasing its adaptability to low-temperature environments and subsequently increasing its photosynthetic rate and biomass.
Data Availability
All datasets for this study are included in the manuscript and/or the Supplementary Files.
Author Contributions
QZ and JZ designed the experiments. LS and WL performed all experiments. QZ and JZ analyzed the data. QZ wrote the manuscript. CP revised the manuscript. All the authors approved the final version of the manuscript.
Funding
The study was supported by the National Basic Research Program of China (2017YFC1200105) and the National Natural Science Foundation of China (31570398, 31870374). This work was also supported by Guangdong Province Natural Science Foundation (2017A030313167), and the study was also supported by the Innovation Project of Graduate School of South China Normal University.
Conflict of Interest Statement
The authors declare that the research was conducted in the absence of any commercial or financial relationships that could be construed as a potential conflict of interest.
Acknowledgments
We thank Zhidan Xiao (South China Normal University) for the help in conducting the experiments of Western blotting.
References
Ahmed, N. U., Park, J. I., Jung, H. J., Yang, T. J., Hur, Y., Nou, I. S. (2014). Characterization of dihydroflavonol 4-reductase (DFR) genes and their association with cold and freezing stress in Brassica rapa. Gene 550, 46–55. doi: 10.1016/j.gene.2014.08.013
Ainsworth, E. A., Gillespie, K. M. (2007). Estimation of total phenolic content and other oxidation substrates in plant tissues using Folin–Ciocalteu reagent. Nat. Protoc. 2, 875–877. doi: 10.1038/nprot.2007.102
Allan, A. C., Hellens, R. P., Laing, W. A. (2008). MYB transcription factors that colour our fruit. Trends Plant Sci. 13, 99–102. doi: 10.1016/j.tplants.2007.11.012
Arnholdt-Schmitt, B., Costa, J. H., de Melo, D. F. (2006). AOX—a functional marker for efficient cell reprogramming under stress? Trends Plant Sci. 11, 281–287. doi: 10.1016/j.tplants.2006.05.001
Baek, K. H., Skinner, D. Z. (2003). Alteration of antioxidant enzyme gene expression during cold acclimation of near-isogenic wheat lines. Plant Sci. 165, 1221–1227. doi: 10.1016/S0168-9452(03)00329-7
Carmona, L., Alquezar, B., Marques, V. V., Pena, L. (2017). Anthocyanin biosynthesis and accumulation in blood oranges during postharvest storage at different low temperatures. Food Chem. 237, 7–14. doi: 10.1016/j.foodchem.2017.05.076
Chinnusamy, V., Zhu, J., Zhu, J. K. (2007). Cold stress regulation of gene expression in plants. Trends Plant Sci. 12, 444–451. doi: 10.1016/j.tplants.2007.07.002
Field, T. S., Lee, D. W., Holbrook, N. M. (2001). Why leaves turn red in autumn. The role of anthocyanins in senescing leaves of redosier dogwood. Plant Physiol. 127, 566–574. doi: 10.1104/pp.010063
Guadagno, C. R., Ewers, B. E., Speckman, H. N., Aston, T. L., Huhn, B. J., DeVore, S. B., et al. (2017). Dead or alive? Using membrane failure and chlorophyll a fluorescence to predict plant mortality from drought. Plant Physiol. 175, 223–234. doi: 10.1104/pp.16.00581
Gusta, L. V., Wisniewski, M. (2013). Understanding plant cold hardiness: an opinion. Physiol. Plant 147, 4–14. doi: 10.1111/j.1399-3054.2012.01611.x
Heimler, D., Vignolini, P., Dini, M. G., Romani, A. (2005). Rapid tests to assess the antioxidant activity of Phaseolus vulgaris L. dry beans. J. Agr. Food Chem. 53, 3053–3056. doi: 10.1021/jf049001r
Holm, L. G., Plucknett, D. L., Pancho, J. V., Herberger, J. P. (1977). The World’s Worst Weeds: Distribution and Biology. Honolulu: East-West Center and University Press of Hawaii.
Hughes, N. M., Morley, C. B., Smith, W. K. (2007). Coordination of anthocyanin decline and photosynthetic maturation in juvenile leaves of three deciduous tree species. New Phytol. 175, 675–685. doi: 10.1111/j.1469-8137.2007.02133.x
Jin, R., Kim, B. H., Ji, C. Y., Kim, H. S., Li, H. M., Ma, D. F., et al. (2017). Overexpressing IbCBF3 increases low temperature and drought stress tolerance in transgenic sweetpotato. Plant Physiol. Biochem. 118, 45–54. doi: 10.1016/j.plaphy.2017.06.002
Lev-Yadun, S., Gould, K. S. (2009). “Role of anthocyanins in plant defence,” in Anthocyanins: Biosynthesis, Functions, and Applications. Eds. Gould, K., Davies, K., Winefield, C. (New York: Springer), 21–48. doi: 10.1007/978-0-387-77335-3_2
Li, J., Li, Y., Yin, Z., Jiang, J., Zhang, M., Guo, X., et al. (2017). OsASR5 enhances drought tolerance through a stomatal closure pathway associated with ABA and H2O2 signaling in rice. Plant Biotechnol. J. 15, 183–196. doi: 10.1111/pbi.12601
Li, P., Li, Y. J., Zhang, F. J., Zhang, G. Z., Jiang, X. Y., Yu, H. M., et al. (2017b). The Arabidopsis UDP-glycosyltransferases UGT79B2 and UGT79B3, contribute to cold, salt and drought stress tolerance via modulating anthocyanin accumulation. Plant J. 89, 85–103. doi: 10.1111/tpj.13324
Liu, Y., Tikunov, Y., Schouten, R. E., Marcelis, L. F. M., Visser, R. G. F., Bovy, A. (2018). Anthocyanin biosynthesis and degradation mechanisms in solanaceous vegetables: a review. Front. Chem. 6, 52. doi: 10.3389/fchem.2018.00052
Liu, Y., Ren, D., Pike, S., Pallardy, S., Gassmann, W., Zhang, S. (2007). Chloroplast-generated reactive oxygen species are involved in hypersensitive response-like cell death mediated by a mitogen-activated protein kinase cascade. Plant J. 51, 941–954. doi: 10.1111/j.1365-313X.2007.03191.x
Livak, K. J., Schmittgen, T. D. (2001). Analysis of relative gene expression data using real-time quantitative PCR and the 2–DDCT method. Methods 25, 402–408. doi: 10.1006/meth.2001.1262
Lo Piero, A. R., Puglisi, I., Rapisarda, P., Petrone, G. (2005). Anthocyanins accumulation and related gene expression in red orange fruit induced by low temperature storage. J. Agric. Food Chem. 53, 9083–9088. doi: 10.1021/jf051609s
Lorenc-Kukula, K., Jafra, S., Oszmianski, J., Szopa, J. (2005). Ectopic expression of anthocyanin 5-o-glucosyltransferase in potato tuber causes increased resistance to bacteria. J. Agric. Food Chem. 53, 272–281. doi: 10.1021/jf048449p
Manetas, Y., Petropoulou, Y., Psaras, G. K., Drinia, A. (2003). Exposed red (anthocyanic) leaves of Quercus coccifera display shade characteristics. Funct. Plant Biol. 30, 265–270. doi: 10.1071/FP02226
Mega, R., Abe, F., Kim, J. S., Tsuboi, Y., Tanaka, K., Kobayashi, H., et al. (2019). Tuning water-use efficiency and drought tolerance in wheat using abscisic acid receptors. Nat. Plants 5, 153–159. doi: 10.1038/s41477-019-0361-8
Naing, A. H., Ai, T. N., Lim, K. B., Lee, I. J., Kim, C. K. (2018). Overexpression of Rosea1 from snapdragon enhances anthocyanin accumulation and abiotic stress tolerance in transgenic tobacco. Front. Plant Sci. 9, 1070. doi: 10.3389/fpls.2018.01070
Oxborough, K., Baker, N. R. (1997). Resolving chlorophyll a fluorescence images of photosynthetic efficiency into photochemical and non-photochemical components-calculation of qP and Fv’/Fm’; without measuring Fo’. Photosynth. Res. 54, 135–142. doi: 10.1023/A:1005936823310
Pojer, E., Mattivi, F., Johnson, D., Stockley, C. S. (2013). The case for anthocyanin consumption to promote human health: a review. Comp. Rev. Food Sci. Food Saf. 12, 483–508. doi: 10.1111/1541-4337.12024
Ruelland, E., Vaultier, M. N., Zachowski, A., Hurry, V. (2009). Cold signaling and cold acclimation in plants. Adv. Bot. Res. 49, 35–150. doi: 10.1016/S0065-2296(08)00602-2
Saha, M. R., Hasan, R., Akter, R., Hossain, M. (2008). In vitro free radical scavenging activity of methanol extract of the leaves of Mimusops elengi Linn. Bangl. J. Vet. Med. 6, 197–202. doi: 10.3329/bjvm.v6i2.2336
Sivankalyani, V., Feygenberg, O., Diskin, S., Wright, B., Alkan, N. (2016). Increased anthocyanin and flavonoids in mango fruit peel are associated with cold and pathogen resistance. Postharvest Biol. Technol. 111, 132–139. doi: 10.1016/j.postharvbio.2015.08.001
Siwakoti, M. (2007). Mikania Weed: A Challenge for Conservationists. Our Nat. 5, 70–74. doi: 10.3126/on.v5i1.801
Sun, L., Li, X., Wang, Z., Sun, Z., Zhu, X., Liu, S., et al. (2018). Cold priming induced tolerance to subsequent low temperature stress is enhanced by melatonin application during recovery in wheat. Molecules 23, 1091. doi: 10.3390/molecules23051091
Takahashi, F., Suzuki, T., Osakabe, Y., Betsuyaku, S., Kondo, Y., Dohmae, N. (2018). A small peptide modulates stomatal control via abscisic acid in long-distance signalling. Nature 556, 235–238. doi: 10.1038/s41586-018-0009-2
Tanaka, Y., Ohmiya, A. (2008). Seeing is believing: engineering anthocyanin and carotenoid biosynthetic pathways. Curr. Opin. Biotechnol. 19, 190–197. doi: 10.1016/j.copbio.2008.02.015
Tanaka, Y., Sasaki, N., Ohmiya, A. (2008). Biosynthesis of plant pigments: anthocyanins, betalains and carotenoids. Plant J. 54, 733–749. doi: 10.1111/j.1365-313X.2008.03447.x
Wang, T., Wang, Z., Chen, G. P., Wang, C. B., Su, Y. J. (2016). Invasive chloroplast population genetics of Mikania micrantha in China: no local adaptation and negative correlation between diversity and geographic distance. Front. Plant Sci. 7, 1426. doi: 10.3389/fpls.2016.01426
Wellburn, A. R. (1994). The spectral determination of chlorophylls a and b, as well as total carotenoids, using various solvents with spectrophotometers of different resolution. J. Plant. Physiol. 144, 307–313. doi: 10.1016/S0176-1617(11)81192-2
Yan, J., Zhang, Y., Yu, G., Zhou, G., Zhang, L., Li, K., et al. (2013). Seasonal and inter-annual variations in net ecosystem exchange of two old-growth forests in Southern China. Agric. Forest Meteorol. 182-183, 257–265. doi: 10.1016/j.agrformet.2013.03.002
Yang, Q., Ye, W., Deng, X., Cao, H., Zhang, Y., Xu, K. (2005). Seed germination eco-physiology of Mikania micrantha H.B.K. Bot. Bull. Acad. Sin. 46, 293–299 doi: 10.1016/j.aquabot.2005.06.004
Yang, S. L., Lan, S. S., Deng, F. F., Gong, M. (2016). Effects of calcium and calmodulin antagonists on chilling stress-induced proline accumulation in Jatropha curcas L. J. Plant Growth Regul. 35, 815–826. doi: 10.1007/s00344-016-9584-3
Yu, L., Liu, Y., Lu, L., Zhang, Q., Chen, Y., Zhou, L., et al. (2017). Ascorbic acid deficiency leads to increased grain chalkiness in transgenic rice for suppressed of L-GalLDH. J. Plant Physiol. 211, 13–26. doi: 10.1016/j.jplph.2016.11.017
Zhang, B. Q., Yang, L. T., Li, Y. R. (2014). Comparison of physiological and biochemical characteristics related to cold resistance in sugarcane under field conditions. Sugar Tech. 17, 496–505. doi: 10.3724/SP.J.1006.2011.00496
Zhang, T. J., Chow, W. S., Liu, X. T., Zhang, P., Liu, N., Peng, C. L. (2016). A magic red coat on the surface of young leaves: anthocyanins distributed in trichome layer protect Castanopsis fissa leaves from photoinhibition. Tree Physiol. 36, 1296–1306. doi: 10.1093/treephys/tpw080
Zhang, L. Y., Ye, W. H., Cao, H. L., Feng, H. L. (2004). Mikania micrantha H. B. K. in China–anoverview. Weed Res. 44, 42–49. doi: 10.1111/j.1365-3180.2003.00371.x
Zhu, H., Zhang, T. J., Zheng, J., Huang, X. D., Yu, Z. C., Peng, C. L., et al. (2018). Anthocyanins function as a light attenuator to compensate for insufficient photoprotection mediated by nonphotochemical quenching in young leaves of Acmena acuminatissima in winter. Photosynthetica 56, 445–454. doi: 10.1007/s11099-017-0740-1
Keywords: anthocyanins, antioxidant activity, gas exchange, Mikania micrantha, winter
Citation: Zhang Q, Zhai J, Shao L, Lin W and Peng C (2019) Accumulation of Anthocyanins: An Adaptation Strategy of Mikania micrantha to Low Temperature in Winter. Front. Plant Sci. 10:1049. doi: 10.3389/fpls.2019.01049
Received: 30 April 2019; Accepted: 29 July 2019;
Published: 29 August 2019.
Edited by:
Wim Van den Ende, KU Leuven, BelgiumReviewed by:
Yi Li, University of Southern California, United StatesRudy Dolferus, Commonwealth Scientific and Industrial Research Organisation (CSIRO), Australia
Copyright © 2019 Zhang, Zhai, Shao, Lin and Peng. This is an open-access article distributed under the terms of the Creative Commons Attribution License (CC BY). The use, distribution or reproduction in other forums is permitted, provided the original author(s) and the copyright owner(s) are credited and that the original publication in this journal is cited, in accordance with accepted academic practice. No use, distribution or reproduction is permitted which does not comply with these terms.
*Correspondence: Changlian Peng, pengchl@scib.ac.cn