- 1College of Horticulture, Qingdao Agricultural University, Qingdao, China
- 2Qingdao Key Laboratory of Genetic Improvement and Breeding in Horticulture Plants, Qingdao Agricultural University, Qingdao, China
Brassinolide (BL) mediates various physiological processes and improves plant tolerance to abiotic stresses. However, the effects and mechanism of exogenous BL on the salt tolerance of apple seedlings remain unclear. Herein, we investigated the role of BL in the salt stress response of Malus hupehensis Rehd., a widely grown apple rootstock. Salt-stressed apple seedlings showed significant decline in chlorophyll content and photosynthetic rate, and the application of 0.2 mg/L exogenous BL alleviated salt stress and maintained photosynthetic capacity. Exogenous BL application can strengthen the activities of superoxide dismutase and catalase and thereby eliminates reactive oxygen species (ROS) production induced by salt stress and promote the accumulation of proline and soluble sugar, thus maintaining osmotic balance. Furthermore, exogenous BL application decreased Na+ accumulation and increased K+ content in shoots and roots under salt stress by regulating the expression levels of Na+(K+)/H+ antiporter genes (MhNHXs). MhBZR1 and MhBZR2, which are the key transcription factors in the BR signal transduction pathway, can directly bind to the promoter of MhSOS1 and MhNHX4-1, respectively, and inhibit their expression. Our findings would provide a theoretical basis for analyzing the mechanism of exogenous BL application on the salt tolerance of apples.
Introduction
Soil salinity is a major type of abiotic stress that limits crop productivity and affects plant growth and development. More than 800 million hectares of land is affected by salinity, and this problem continues to worsen (Liang et al., 2018; Yang and Guo, 2018; Feng et al., 2019). Apples (Malus domestica Borkh.) are one of the most valuable horticultural fruit crops cultivated worldwide. The growth and productivity of apple trees is severely limited by salt stress in China, especially in the Yellow River Delta. The negative effects of salt stress on apple trees, as perennial crops, not only are limited to the current year but also extends to subsequent years (Navarro et al., 2003; An et al., 2018). Therefore, strategies for improving the salt tolerance of apple trees should be explored.
Under salt stress conditions, plants are typically stressed in three ways: osmotic stress, ionic stress, and oxidative damage (Yang and Guo, 2018). A low water potential causes water deficits in roots (Hasegawa et al., 2000). Excessive external and internal Na+ accumulation in the cytoplasm disrupts the homeostasis of Na+:K+ and evokes toxic ion effects. Oxidative damage is usually triggered by osmotic and ionic stress. Given that plants are sessile, they are inevitably affected by salt stress. After prolonged adaptation, plants gradually evolve a precise mechanism as a response to salt stress (Sun et al., 2015a; Mao et al., 2017). For example, a series of salt-responsive genes involved in salt stress signal transduction are activated in response to salt stress (Lopez-Gomez et al., 2016). Reactive oxygen species (ROS) induced by salt stress is alleviated by antioxidant systems, including antioxidants and antioxidant enzymes (Tofighi et al., 2017; Wu et al., 2017). The accumulation of osmolytes, such as sorbitol, proline, and lycine, is critical to osmotic balance (Shahid et al., 2014; Yusuf et al., 2017). Maintaining homeostasis of Na+ and K+ in cells by activating Na+ and K+ transporters is vital for plant salt stress tolerance (Azhar et al., 2017).
Cytosolic Na+:K+ ratio is one of the key features conferring salinity stress tolerance in plants, and this trait is often considered a potential screening tool for plant breeders (Poustini and Siosemardeh, 2004). The salt overly sensitive (SOS) pathway, which is essential for maintaining Na+ and K+ homeostasis in the cytoplasm and defense against salt stress, has been well identified and characterized (Zhu, 2002; Zhu, 2016). The SOS pathway is relatively specific for ionic transportation, in which high Na+ stress initiates a calcium signal that stimulates the SOS3–SOS2 protein kinase complex, which then activates the Na+/H+ exchange transporter SOS1, resulting in the exclusion of excess Na+ from plant cells, thereby enhancing salt detoxification (Qiu et al., 2004; Hu et al., 2016a). SOS1 belongs to the NHX-type Na+(K+)/H+ exchange family genes (NHXs), and the NHXs family is essential for Na+ and K+ balance in plants.
NHX proteins serve a primary role in ion homeostasis, which is an essential cellular process for salt stress response. The Arabidopsis genome encodes eight NHX homologs that have been grouped based on sequence similarities and functions into three distinct classes, namely, plasma membrane (NHX7 and NHX8), endosomal, or vesicular (NHX5 and NHX6; Bassil et al., 2011a; Bassil et al., 2011b), and into four vacuolar homologs, namely, NHX1, NHX2, NHX3, and NHX4 (Leidi et al., 2010). In apple trees, the overexpression of MdSOS1 can enhance salt tolerance and reduce Na+ content (Liu et al., 2012). Besides transport of Na+, AtNHX1 and AtNHX2 are reported to control vacuolar K+ homeostasis to regulate growth, flower development, and reproduction (Bassil et al., 2011a), and they are essential for active K+ uptake in the tonoplast for stomatal function (Barragan et al., 2012).
Plant hormones are essential endogenous molecules and signals involved in regulating plant development and tolerance to diverse stresses, including salinity stress (Ryu and Cho, 2015). The application of plant growth regulators, such as gibberellin 3 (GA3), salicylic acid (SA), and jasmonic acid (JA), is an effective approach for improving the salt tolerance of crops (Shahzad et al., 2018). Brassinolide (BL), as a highly active synthetic analog of brassinosteroids (BRs), play numerous important roles in plant growth and development (Clouse, 1996; Kim et al., 2009). The exogenous application of BL also has major effects on plants and mitigates the adverse effects of salt stress (Yusuf et al., 2012). Exogenous BL application can improve photosynthetic efficiency in different plant species under salt stress by increasing the levels of hormones, especially ZR, iPA, IAA, and SA, increasing gas exchange, enhancing antioxidant enzyme activities, and delaying plant senescence (Yusuf et al., 2012; Wu et al., 2017). In addition, exogenous BL can increase the protein levels and the expression of various salt-responsive genes to different levels (Sharma et al., 2013), but the mechanisms in which exogenous BL application induces the expression of salt-responsive genes remain largely unexplored.
Over the past decade, the BR signaling pathway has been explored in plants (Wang et al., 2012; Ye et al., 2012). In the presence of BRs, brassinosteroid-insensitive 2 (BIN2) is dephosphorylated by bri1-suppressor 1 (BSU1) and degraded by the 26S proteasome, which subsequently suppresses brassinazole resistant1 (BZR1) and bri1-ems-suppressor1 (BES1)/BZR2 by BIN2 (Yin et al., 2002). BZR1 and BES1 are the key transcription factors in the BR signaling pathway, and dephosphorylated BZR1 and BES1 can directly bind the E-BOX (CANNTG) and BRRE elements (CGTGT/CG) of the BR-related gene promoters to regulate the expression of these genes (Shahnejat-Bushehri et al., 2016). However, the molecular mechanism in which BZR1 and BES1 responds to salt stress remain unknown.
Apple trees are often subject to severe salt stress, resulting in a remarkable loss of apple production and quality. Several agronomic practices are employed to mitigate the adverse effects of salt stress. The exogenous application of BL can improve plant tolerance to salt stress in rice and tomato. However, the effects and mechanism by which BL affects the tolerance of woody plants to salt stress are not well documented. In the present study, we investigated the effects of different concentrations of exogenous BL on apple seedlings under salt stress. Then, we explored the potential physiological and molecular mechanisms by which BL influences antioxidative activity, osmotic balance, and ion homeostasis. This finding will enhance the applications and examination of the physiological role of BL under salt stress.
Materials and Methods
Plant Materials and Growth Conditions
Apple seeds (Malus hupehensis) were sown in wet vermiculite after cold stratification. Apple seedlings were grown at 23 ± 2°C in a 16/8 h light/dark cycle. Light intensity was approximately 100 µmol·m−2·s−1. Apple calli induced from “Orin” embryos were grown on MS medium containing 1.5 mg·L–1 2,4-dichlorophenoxyacetic acid and 0.4 mg·L–1 6-BA at 23 ± 2°C in darkness. The calli were subcultured every 2 weeks.
Salt Stress and Exogenous BL Treatment
One hundred eighty 1-month-old apple seedlings were randomly divided into five groups. The apple seedlings in group I were watered with a complete nutrient solution as the control, and group II was treated with 200 mM NaCl. Except for the same salt stress with group II, the seedlings in groups III, IV, and V were sprayed with 0.05, 0.2, and 1.0 mg/L of BL, respectively. BL (Solarbio, Beijing, China) was sprayed every 2 days. Each experiment was independently repeated three times.
Measurements of Chlorophyll Content and Photosynthetic Rate
For determination of chlorophyll content, a SPAD-502 chlorophyll meter (Konica Minolta, Tokyo, Japan) was used. The photosynthetic rate was measured using a LI-6400XT meter (LI-COR, Lincoln, USA). The light intensity was set at 500 µmol·m−2·s−1 at an approximately 50% humidity. The temperature was set at 22°C.
Measurements of ROS Levels and Malondialdehyde Content
H2O2 and O2·− staining was conducted as described by Zheng et al. (2017). The malondialdehyde (MDA) content of the leaves was detected using a plant MDA extraction kit (Nanjing Jiancheng Bioengineering Institute, Nanjing, China).
Detection of Antioxidant Enzyme Activities
A total of 0.1 g of leaves from apple seedlings were used for the detection of antioxidant enzyme activities. Superoxide dismutase (SOD), peroxidase (POD), and catalase (CAT) activities were detected according to the plant SOD, POD, and CAT extraction kits (Nanjing Jiancheng Bioengineering Institute, Nanjing, China) following the manufacturer’s instructions.
Determination of Electrolyte Leakage and Osmolytes
A total of 0.5 g of leaves from apple seedlings were used for the detection of electrolyte leakage and osmolytes. Electrolyte leakage was determined as described previously (Ahmad et al., 2016). The proline content was determined as described by Wani et al. (2017). The absorbance was obtained at 520 nm by using a spectrophotometer (Yoke, Shanghai, China). Soluble protein and soluble sugar contents were determined as described by Qiu et al. (2019) and Sharma et al., 2019, respectively.
Determinations of Na+ and K+
A total of 0.5 g (dry weight) of shoots and roots from apple seedlings were used for the detection of Na+ and K+ contents. The concentrations of Na+ and K+ were measured by inductively coupled plasma-optical emission spectrometry (PerkinElmer, Waltham Massachusetts, USA) as described by Liang et al. (2017).
Quantitative Reverse Transcription Polymerase Chain Reaction Assay
Total RNA extraction and quantitative polymerase chain reaction (qPCR) assay were conducted as described by Zheng et al. (2017). MhActin (accession number: MDP0000774288) was used as an internal control. Primer sequences for qPCR were designed according to the coding sequence of MhNHXs and MhBZRs by using the Primer 5 software and checked using BLAST search in the apple genomic database. The primer sequences are shown in Table S1.
Cloning of MhBZRs Genes and the Prokaryotic Expression of Their Proteins in Escherichia coli
The MhBZR1 (MDP0000157809), MhBZR2 (MDP0000306427), MhBZR3 (MDP0000203462), MhBZR4 (MDP0000218271), MhBZR5 (MDP0000344348), and MhBZR6 (MDP0000130173) coding regions were cloned from M. hupehensis. The coding sequences of MhBZRs were amplified and ligated into the pGEX-6P-1 vector containing a GST tag. The primers are shown in Table S1. The GST-MhBZRs fusion proteins were expressed in E. coli (BL21) and purified using glutathione-sepharose 4B (GE Healthcare, Little Chalfont, UK).
Electrophoretic Mobility Shift Assay
Electrophoretic mobility shift assays (EMSA) were employed to detect a combination of the GST-MhBZRs proteins with the promoters of MhNHXs in which the MhNHXs promoter fragments containing the E-box (CANNTG) were produced using biotin-labeled (Invitrogen, Shanghai, China) or unlabeled oligonucleotide (to be used as a competitor). The primers are shown in Table S1. The EMSA reaction was performed as described by Zheng et al. (2018).
Detection of GUS and LUC Expression Assays in the Transiently Transformed Apple Calli
The MhSOS1 and MhNHX4-1 promoters were cloned from M. hupehensis and ligated into the pCAMBIA1301 vector, and the coding sequences of MhBZR1/MhBZR2 were amplified and ligated into the pMDC83 vector. The primers are shown in Table S1. 35S::MhBZR1/MhBZR2-GFP, proMhSOS1/MhNHX4-1::GUS (β-glucuronidase), and 35S::LUC were transiently transformed together into the Agrobacterium strain EHA105 for the transformation of apple calli. EHA105 carrying 35S::GFP, proMhSOS1/MhNHX4-1::GUS, and 35S::LUC was used as the control. The transient transformation of apple calli and the GUS and LUC expression assays were performed as described previously (Zheng et al., 2018).
Experimental Design and Statistical Analysis
All experiments were repeated three times according to a completely randomized design. The data were analyzed by ANOVA followed by Fisher’s LSD or Student’s t-test analysis. Statistically significant differences were indicated by P < 0.05. Statistical computations were conducted using SPSS (IBM, Armonk, NY, USA).
Results
Exogenous BL Treatment Improved the Salt Tolerance of Apple Seedlings
BL at different concentrations was sprayed on the leaves of M. hupehensis seedlings under 200 mM NaCl stress. As shown in Figure 1, the apple seedlings were seriously damaged, showing withered and chlorotic leaves under salt stress. At low (0.05 mg/L) and high BL concentrations (1.0 mg/L), the growth vigor of the seedlings was much better than those without BL treatment, but the leaves still withered noticeably. However, when 0.2 mg/L BL was applied to the apple seedlings, the growth of the seedlings under salt stress was similar to that of the control seedlings under normal conditions. Therefore, 0.2 mg/L BL was selected for further experiments.
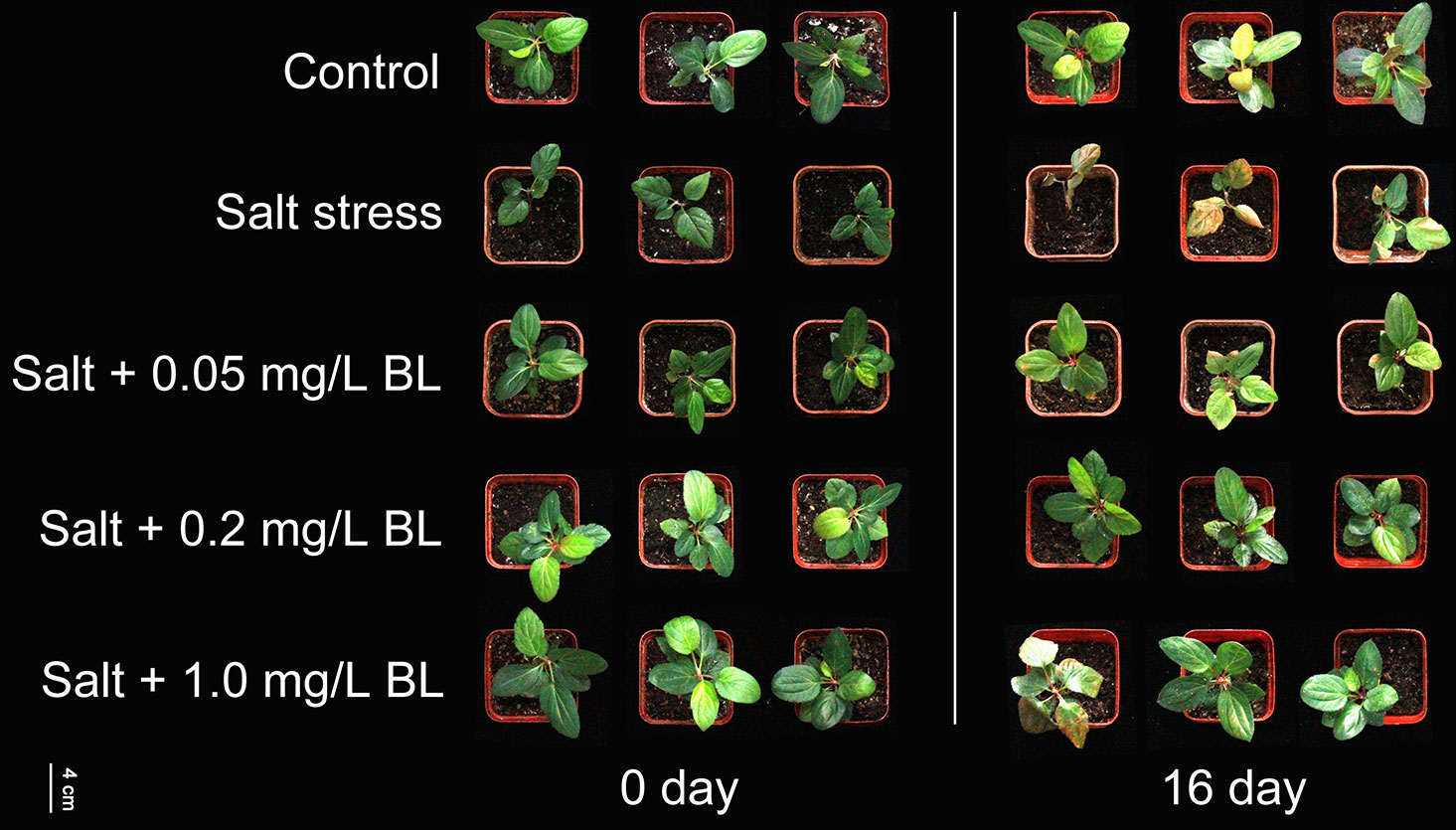
Figure 1 The phenotype resulting from the application of different concentrations of exogenous brassinolide (BL) (0.05, 0.2, and 1.0 mg/L) to Malus hupehensis under 200 mM NaCl stress at day 0 and day 16. Exogenous BL was sprayed on the leaves of apple seedlings every 2 days. Each experiment was independently repeated three times.
Effects of Exogenous BL Application on Chlorophyll Content and Photosynthetic Rate Under Salt Stress
As shown in Figure 2A, salt stress dramatically reduced the chlorophyll content from 41.2 SPAD to 30.0 SPAD but increased chlorophyll content to 37.6 SPAD upon exogenous BL application. The photosynthetic rate of apple seedlings sharply decreased from 8.0 to 1.2 µmol·m−2·s−1 under salt stress. When exogenous BL was applied, the photosynthetic rate of the apple seedlings reached 4.1 µmol·m−2·s−1 (Figure 2B).
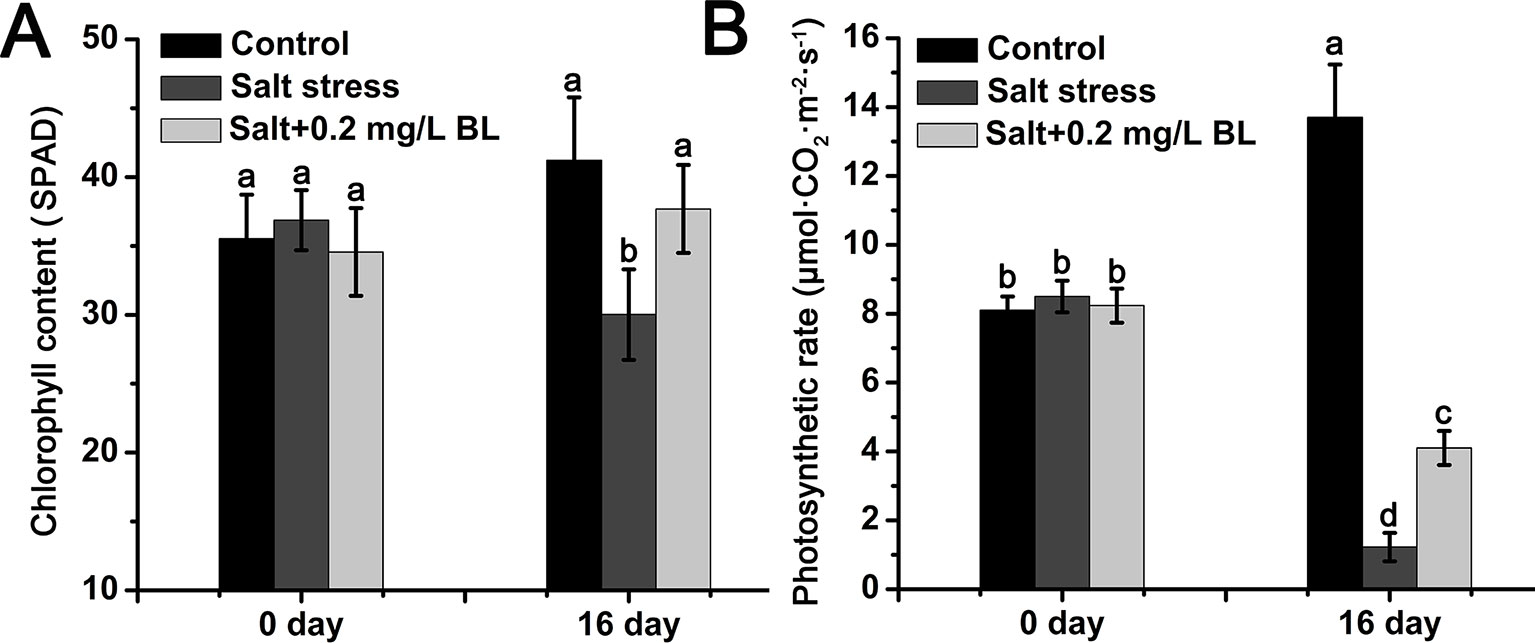
Figure 2 Effects of exogenous BL application on the chlorophyll content (A) and photosynthetic rate (B) of apple seedlings before and after salt stress. Each experiment was independently repeated three times. Data represent the means ± SD of three biological replicates. Different lowercase letters indicate significant differences, according to Fisher’s LSD (P < 0.05).
Effects of Exogenous BL Application on Oxidative Damage and Antioxidant Enzyme Activities Under Salt Stress
Salt stress significantly elevated the O2·− and H2O2 levels in the leaves of apple seedlings, whereas exogenous BL significantly reduced these levels (Figure 3A). Consistently, the MDA content under salt stress was twice that of the control group. When exogenous BL was applied, the MDA content recovered to the control level (Figure 3B).
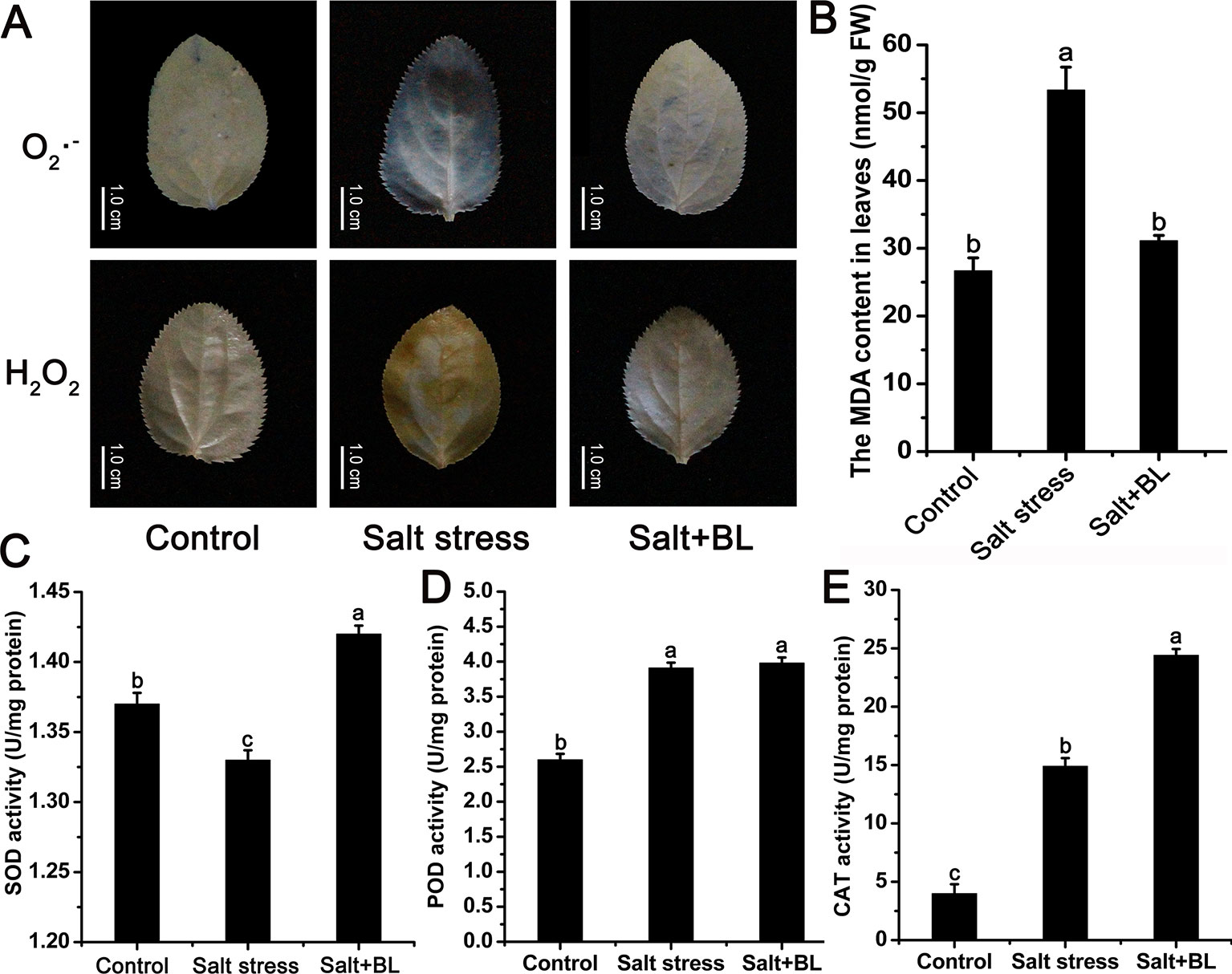
Figure 3 Effects of exogenous BL application on the levels of O2·− and H2O2 (A) and malondialdehyde (MDA) contents (B) under salt stress. Effects of exogenous BL application on the activities of superoxide dismutase (SOD) (C), peroxidase (POD) (D), and catalase (CAT) (E) under salt stress. The scale bars in (A) represent 1.0 cm. Each experiment was independently repeated three times. Data represent the means ± SD of three biological replicates. Different lowercase letters indicate significant differences, according to Fisher’s LSD (P < 0.05).
The activities of antioxidant enzymes SOD, POD, and CAT were measured. SOD activity was 1.33 U/mg protein under salt stress. When exogenous BL was applied, SOD activity increased to 1.42 U/mg protein (Figure 3C). CAT activity was only 14.9 U/mg protein after salt stress, and exogenous BL treatment increased CAT activity to 24.4 U/mg protein (Figure 3E). During POD activity, no difference was observed in the seedlings treated with or without exogenous BL (Figure 3D).
Effects of Exogenous BL Application on the Electrolyte Leakage and Osmolytes Under Salt Stress
As shown in Figure 4A, electrolyte leakage was sharply induced by salt stress but was reduced by 43.18% compared with that in the salt-treated plants alone when exogenous BL was applied.
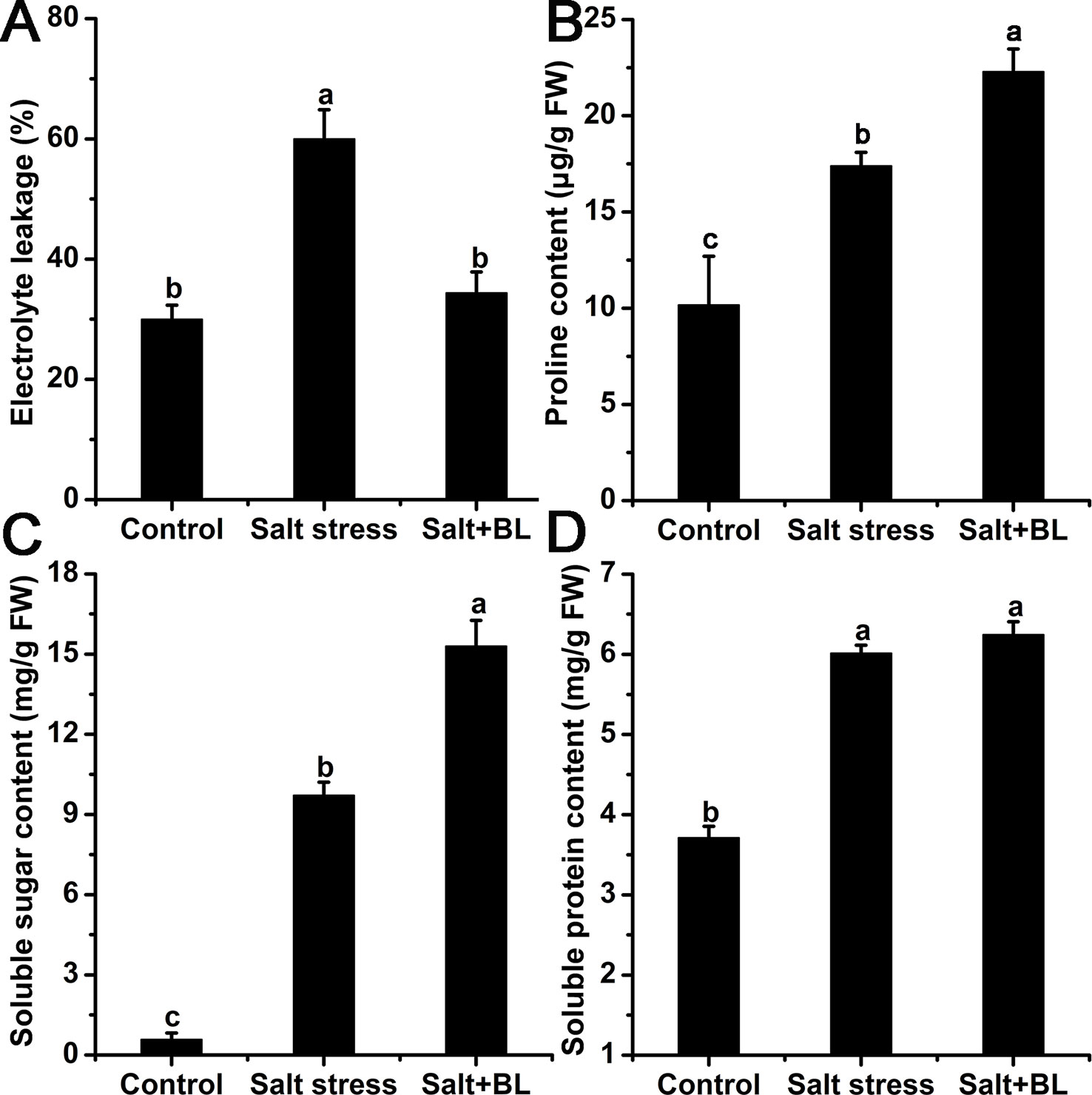
Figure 4 Effects of exogenous BL application on electrolyte leakage (A), proline content (B), soluble sugar content (C), and soluble protein content (D) under salt stress. Each experiment was independently repeated three times. Data represent the means ± SD of triplicate experiments. Different lowercase letters indicate significant differences, according to Fisher’s LSD (P < 0.05).
We measured the contents of osmolytes, including proline, soluble sugar, and soluble protein. The contents of proline, soluble sugar, and soluble protein were all significantly induced by salt stress (Figure 4). When exogenous BL was applied, the contents of proline and soluble sugar were increased compared with the apple seedlings under salt stress, and the content of soluble protein had no change.
Effects of Exogenous BL Application on the Na+ and K+ Contents Under Salt Stress
The Na+ and K+ contents in the shoots and roots were measured. As shown in Figure 5A, the content of Na+ was sharply induced by salt stress both in the shoots and roots. When exogenous BL was applied, the Na+ content decreased significantly. The K+ content increased in the shoots and roots due to salt stress and exogenous BL (Figure 5B). The Na+:K+ ratio was significantly increased under salt stress, and exogenous BL reduced sodium concentrations, increased potassium concentrations, and reduced the Na+:K+ ratio under salt stress in the shoots and roots (Figure 5C).
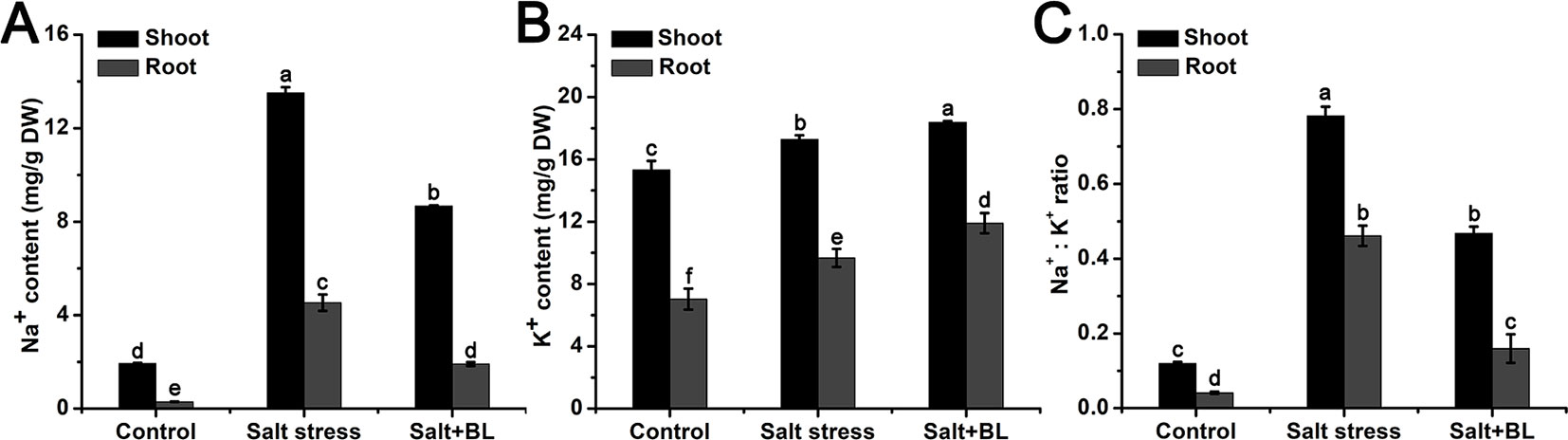
Figure 5 Effects of exogenous BL application on Na+ content (A), K+ content (B), and the Na+:K+ ratio (C) in shoots and roots under salt stress. Each experiment was independently repeated three times. Data represent the means ± SD of three biological replicates. Different lowercase letters indicate significant differences, according to Fisher’s LSD (P < 0.05).
Effects of Exogenous BL Application on the Expression of the MhNHXs Family of Genes Under Salt Stress
A phylogenetic tree was constructed according to the amino acid sequences of MhNHXs and AtNHXs. As shown in Figure 6A, five MhNHXs genes homologous with AtNHX1, 2, 3, and 4; two MhNHXs genes homologous with AtNHX5 and 6; and the MhNHX7-1 (MhSOS1) gene, which is homologous with AtNHX7 (AtSOS1) and AtNHX8 were observed.
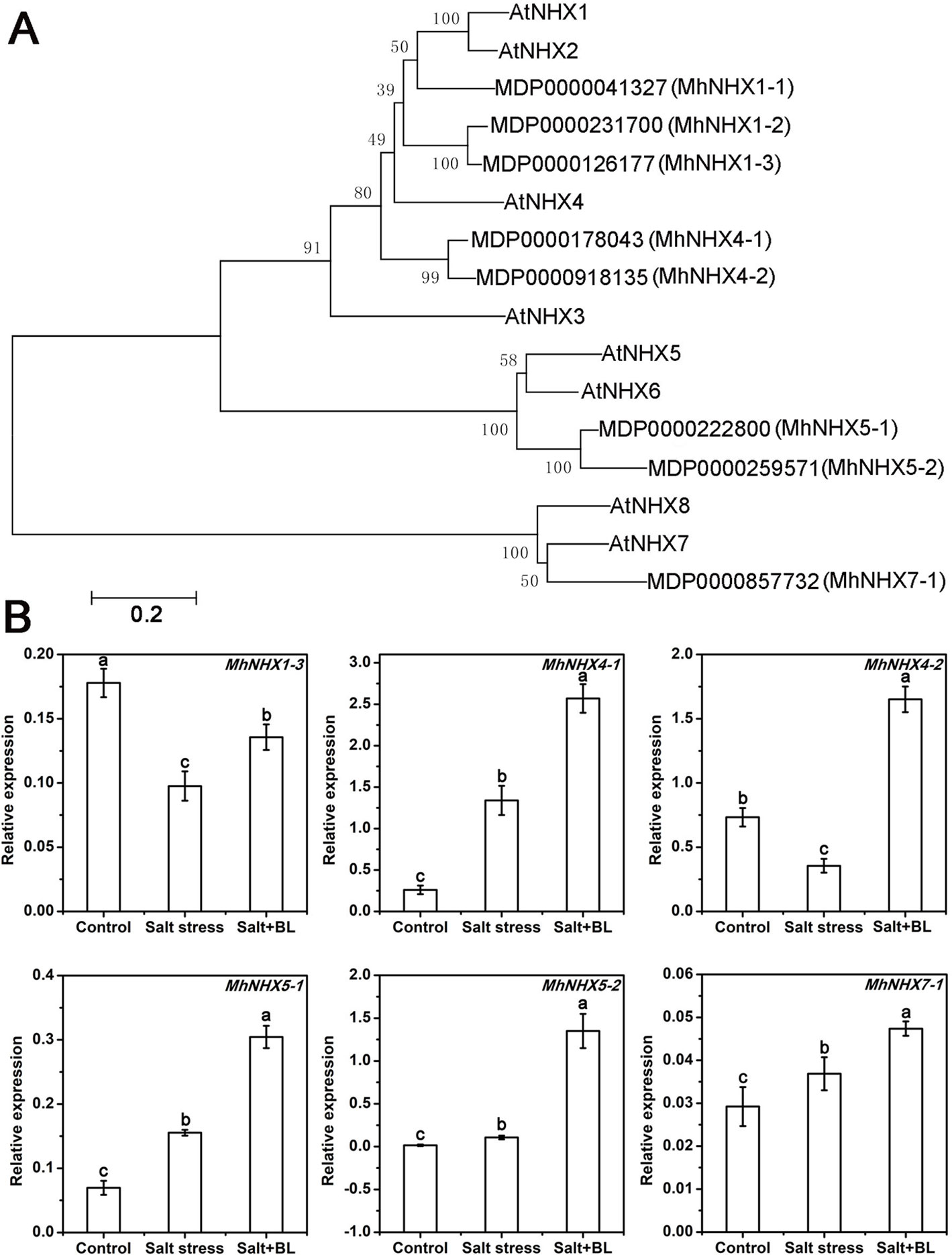
Figure 6 Phylogenetic tree of the amino acid sequences of the NHX family in apple and Arabidopsis and effects of exogenous BL application on the expression of MhNHXs family genes in apple seedlings under salt stress. (A) The phylogenetic tree was constructed using MEGA5.2 software with the neighbor-joining method and a bootstrap test with 1000 iterations based on the amino acid sequences of NHXs in apple and Arabidopsis. (B) The effects of exogenous BL application on the expression level of MhNHXs (MhNHX1-3, MhNHX4-1, MhNHX4-2, MhNHX5-1, MhNHX5-2, and MhSOS1) in apple seedlings under salt stress. Each experiment was independently repeated three times. Data represent the means ± SD of three biological replicates. Different lowercase letters indicate significant differences according to Fisher’s LSD (P < 0.05).
The expression of the MhNHXs gene family was detected. The expression of MhNHX4-1, MhNHX5-1, MhNHX5-2, and MhNHX7-1 was induced by salt stress, whereas the expression of MhNHX1-3 and MhNHX4-2 was suppressed by salt stress. Notably, the expression of MhNHX1-3, MhNHX4-1, MhNHX4-2, MhNHX5-1, MhNHX5-2, and MhNHX7-1 was induced by exogenous BL (Figure 6B).
Effects of Exogenous BL Application on the Expression of the MhBZRs Gene Family Under Salt Stress
Six MhBZRs genes that were homologous with AtBZR1 and AtBZR2 were identified. qPCR results showed that MhBZR1 and MhBZR2 were suppressed by salt stress, and MhBZR3, 4, 5, and 6 were induced by salt stress. When exogenous BL was applied under salt stress, the expression levels of MhBZR1, 2, 3, and 5 were suppressed, whereas that of MhBZR4 was increased compared with the treatment without BL (Figure 7 and Figure S1).
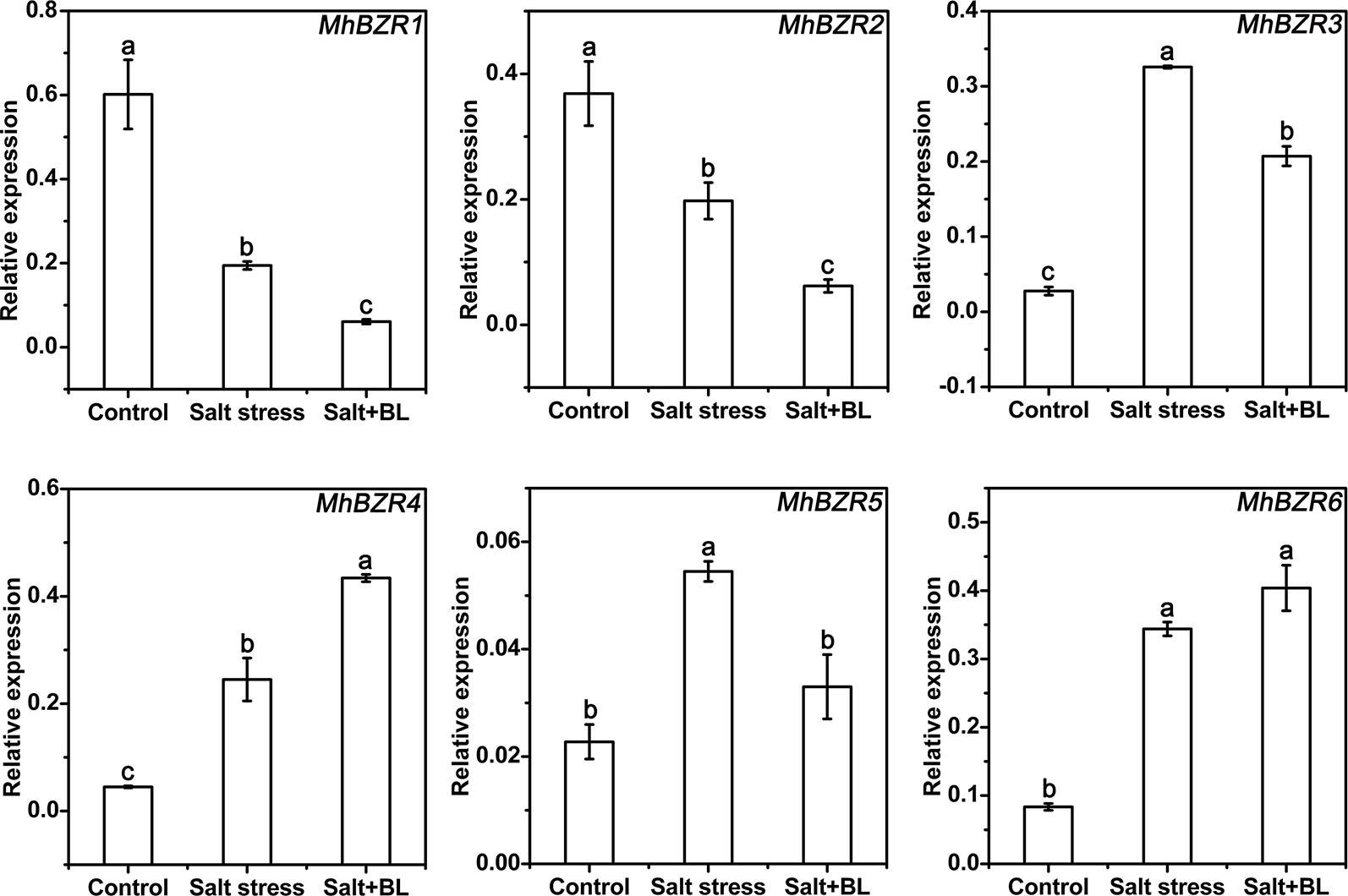
Figure 7 Effects of exogenous BL application on the expression levels of the MhBZRs gene family (MhBZR1, MhBZR2, MhBZR3, MhBZR4, MhBZR5, and MhBZR6) in apple seedlings under salt stress. Each experiment was independently repeated three times. Data represent the means ± SD of three biological replicates. Different lowercase letters indicate significant differences, according to Fisher’s LSD (P < 0.05).
MhBZR1 and MhBZR2 Directly Bind to the Promoter of MhSOS1 or MhNHX4-1 and Repress Their Transcription
EMSA was performed to examine the binding of the promoter of MhNHXs by MhBZRs. MhBZR1 was bound to the MhSOS1 promoter in vitro. The addition of 50×, 100×, and 200× unlabeled competitor reduced the detected binding of MhBZR1. Similar results confirmed that MhBZR2 can bind to the MhNHX4-1 promoter in vitro (Figure 8A).
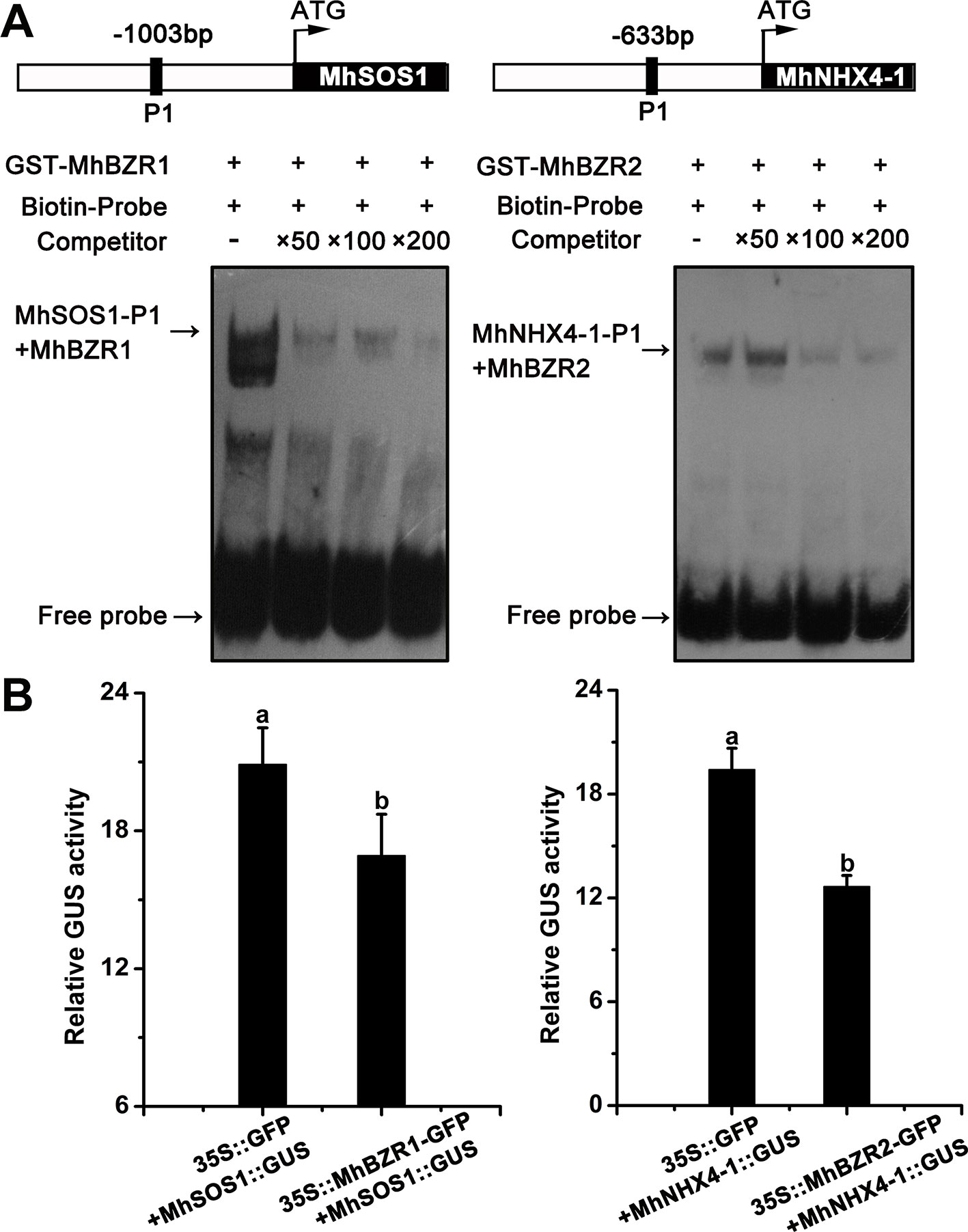
Figure 8 MhBZR1 and MhBZR2 bind to the promoter of MhSOS1 or MhNHX4-1 and inhibits their transcription, respectively. (A) Electrophoretic mobility shift assay (EMSA) results show the in vitro binding of MhBZR1 and MhBZR2 to the MhSOS1 and MhNHX4-1 promoters. The arrow indicates the position of a protein/DNA complex after the incubation of a biotin-labeled DNA probe and the GST-MhBZR1/MhBZR2 protein. Both the probes containing an E-box (CANNTG) was synthesized according to the sequence of the MhSOS1/MhNHX4-1 promoter. (B) The relative GUS activity normalized to luciferase (LUC) activity in transiently transformed apple calli overexpressing 35S::MhBZR1/MhBZR2-GFP, proMhSOS1/MhNHX4-1::GUS and 35S::LUC; 35S::GFP, proMhSOS1/MhNHX4-1::GUS and 35S::LUC were used as controls. Each experiment was independently repeated three times. Data represent the means ± SD of three biological replicates. Different lowercase letters indicate significant differences, according to Fisher’s LSD (P < 0.05).
As shown in Figure 8B, the GUS : LUC activity ratio was significantly lower in transgenic apple calli expressing proMhSOS1::GUS and 35S::MhBZR1-GFP than in transgenic apple calli expressing proMhSOS1::GUS and 35S::GFP. Similar results were observed in MhBZR2 to MhNHX4-1. Hence, MhBZR1 and MhBZR2 can directly bind to the promoter of MhSOS1 and MhNHX4-1 and repress their transcription.
Discussion
Soil salinization negatively affects crop growth and is one of the major limiting factors of agricultural production (Zhu, 2016). Increased salinity in soil has severely hampered the development of apple plantations and resulted in economic losses for orchardists (Hu et al., 2018). The application of BL is a promising method for improving salt tolerance in plants (Shahzad et al., 2018; Tanveer et al., 2018). However, in woody plants, such as apple, the effect of exogenous BL application on seedlings under salt stress has not been reported. In our study, we applied different concentrations of BL to salt-stressed apple seedlings and found that the effects of BL application was much better at 0.2 mg/L than at 0.05 and 1.0 mg/L (Figure 1). Plant hormones are usually trace and efficient, and plant growth regulators affect plant growth and development usually in a dose-dependent manner. Low BL concentrations (0.1 and 0.01 µg/L) promote root elongation and lateral root development, whereas high BL concentrations (1–100 µg/L) inhibit root elongation (Hu et al., 2016b). Our result indicated that 0.2 mg/L BL would be an appropriate concentration for enhancing salt tolerance of M. hupehensis seedlings.
Salt toxicity to plants generally causes reduction in their photosynthetic performance due to the negative effects of salt stress on chlorophyll content and photosynthetic machinery (Wu et al., 2017). Wani et al. (2017) reported that salt stress inhibits the synthesis of 5-aminolaevulinic acid, a precursor of chlorophyll. Sadeghi and Shekafandeh (2014) reported the enhanced activity of chlorophyllase, the enzyme catalyzes the degradation of chlorophyll molecules. The present study demonstrated that salinity stress can significantly reduce the chlorophyll content of apple seedlings, and exogenous BL treatment can recover the content to normal levels (Figure 2A). The recovery of chlorophyll contents after the exogenous application of BL might be due to the modulation of transcription and translation processes by BR signaling, which ultimately stimulates chlorophyll biosynthesis and inhibits the breakdown of chlorophyll molecules (Sharma et al., 2016; Dong et al., 2017). Moreover, the present study indicated that the photosynthetic rate of the apple seedlings was reduced by salt stress, and exogenous BL application significantly improved photosynthetic rate under salt stress (Figure 2B). The application of BL enhances the leaf area of plants under salt stress (Sadeghi and Shekafandeh, 2014). In cucumber, BL increases photosynthesis by increasing chlorophyll content (Yuan et al., 2012). The application of BL improves photosynthesis by positively regulating synthesis and the activation of rubisco enzyme, thus increasing the gaseous exchange parameters of plants under salt stress (Fariduddin et al., 2014; Yusuf et al., 2017). It can be inferred that exogenous BL improves the photosynthetic rate of the apple seedlings under salt stress mainly by increasing chlorophyll content, leaf area, stomatal conductance, and rubisco enzyme activity.
Three main challenges are encountered by plants under salt stress, namely, osmotic stress, ion toxicity, and oxidative damage. Osmotic stress and ionic stress usually result in the oxidative damage with the accumulation of ROS, including O2·− and H2O2 (Miller et al., 2010). In our study, the O2·−, H2O2, and MDA contents were sharply induced by salt stress, and exogenous BL application can markedly diminish them under salt stress (Figure 3). Exogenous BL application can reduce oxidative damage and decrease the production of ROS (Fariduddin et al., 2013; Lopez-Gomez et al., 2016). To prevent oxidative damages induced by salt stress, plants have developed an antioxidant system to maintain a dynamic balance of ROS. The antioxidant system comprises antioxidants and antioxidant enzymes. Enzymatic ROS scavenging mechanisms mainly include SOD, POD, and CAT (Ismail et al., 2014). The present study demonstrated that the accumulation of O2·− and H2O2 and the increased concentration of MDA under salt stress were associated with the change of SOD, POD, and CAT activities. When exogenous BL was applied, the activities of SOD and CAT were significantly increased by 7% and 64%, respectively (Figure 3). The exogenous application of BL triggers the antioxidant enzyme systems of plants under salt stress, resulting in decreased oxidative stress (Tofighi et al., 2017). Wani et al. (2017) reported that exogenous BL application can increase the activities of SOD (75%) and CAT (52%) under salt stress in Cicer arietinum. This may be caused by that BL promotes the transcription and/or translation of the specific genes of antioxidant enzymes (Bajguz and Hayat, 2009). In addition, BL is also involved in the upregulation of genes enhancing the level of antioxidant enzymes subjected to salt stress (Cao et al., 2005; Ali et al., 2007).
Osmotic stress results from the effect of high salt concentrations in soil. Excess soluble salts in soil reduce the water potential at the root surface, which leads to water deficit in plants (Yang and Guo, 2018). Leaf electrolyte leakage is an important indicator of plant cell permeability. Our results indicated that electrolyte leakage was sharply induced by salt stress, and exogenous BL can decrease electrolyte leakage and protect apple seedlings from osmotic stress (Figure 4A). Under salt stress, plants accumulate various osmolytes in the cytoplasm, including soluble sugar and proline, through which they sustain their osmotic equilibrium and support the absorption of water (Liang et al., 2018). Our experiments revealed that salt stress increased the contents of proline, soluble sugar, and soluble protein. The application of exogenous BL enhanced the contents of proline and soluble sugar but did not influence the levels of soluble protein (Figure 4). In Triticum aestivum, a significant increase in proline content and soluble sugar was noticed after the application of BL, thus protecting plants from the adverse effects of salt toxicity (Talaat and Shawky, 2012; Yusuf et al., 2017). Our results indicated that exogenous BL can protect apple seedlings from osmotic stress mainly through the accumulation of proline and soluble sugar.
The primary challenge for plants under salt stress is ionic toxicity, which is caused by the excessive accumulations of sodium in the cytoplasm. This ionic toxicity can lead to an imbalance in cytosolic Na+:K+ ratio and disrupt normal plant growth (Dai et al., 2018). In the present study, Na+ and K+ contents in the shoots were increased under salt stress. When exogenous BL was applied to the apple leaves, Na+ content decreased with increased K+ content, and thus the leaf Na+/K+ ratio decreased (Figure 5). This condition is consistent with the findings of Sun et al. (2015b) in which 10 nM BL treatment reduced leaf Na+/K+ and increased K+ content in perennial ryegrass under salt stress. However, in the roots, exogenous BL notably increased K+ content. Given that exogenous BL was applied to the leaves, we inferred that the increased K+ content in roots was caused by the transportation and distribution of K+ between shoots and roots, which was affected by exogenous BL to the leaves. Recently, Azhar et al. (2017) showed that BL in the growth medium prevented salt-induced K+ leak from shoots and roots. They also noted that BL decreased K+ efflux under salt stress by controlling depolarization-activated GORK channels. Our results indicated that exogenous BL can enhance the exclusion of Na+ and increase the absorption of K+ both in shoots and roots under salt stress.
The physiological mechanism of exogenous BL on plants has been widely explored. However, limited information is available regarding the transcriptional changes and the molecular mechanism in response to BL under salt stress. Na+(K+)/H+ antiporters (NHX) are essential compounds and are critical for modulating plant Na+:K+ homeostasis (Carden et al., 2003). The overexpression of apple MdSOS1 can enhance salt tolerance and reduce the content of Na+ (Liu et al., 2012). In the present study, MhSOS1 was induced by salt stress and enhanced by exogenous BL treatment. Thus, the decrease in Na+ content following exogenous BL treatment under salt stress was mainly caused by the induced expression level of MhSOS1. Furthermore, AtNHX1, 2, 3, and 4 are located in vacuoles and regulate K+ homeostasis between the cytoplasm and vacuoles (Craig Plett and Moller, 2010; Barragan et al., 2012). In apple, the overexpression of MdNHX1 significantly enhances salt tolerance (Li et al., 2010). Our results showed that MhNHX1-3, MhNHX4-1, and MhNHX4-2 expression levels were increased by exogenous BL treatment (Figure 6). We assumed that exogenous BL application increased K+ content mainly through the regulation of transcriptional changes of these genes.
BR regulates a wide spectrum of cellular activities and biological processes, which depend, at least in part, on the direct regulation of gene expression via the BZR1/BES1 family of transcription factors, thus eliciting various BR responses. BZR1 and BES1 are the key transcription factors in the BR signaling pathway that directly regulates the expression of many target genes (Sun et al., 2010; Kim et al., 2017). Information about the transcriptional regulation of the NHX family is still limited. The sugar beet gene BvNHX1 is regulated by the MYB transcription factor (Diedhiou et al., 2009; Adler et al., 2010). We assume that exogenous BL application can regulate transcriptional changes in MhNHXs through MhBZRs. In our study, EMSA results indicated that in vitro, MhBZR1 and MhBZR2 can directly bind to the E-box of the MhSOS1 or MhNHX4-1 promoter, respectively (Figure 8A). The transiently transformed calli expressing the GUS reporter indicated that in vivo, MhBZR1 and MhBZR2 can repress the expression of MhSOS1 and MhNHX4-1, respectively (Figure 8B). Overall, our study revealed the partial molecular mechanism of the effect of exogenous BL application on salt stress in apple.
Data Availability Statement
The datasets generated for this study are available on request to the corresponding author.
Author Contributions
CW and YT planned and designed the research. QS and XZ performed experiments, conducted fieldwork, analyzed data, etc. QS, XZ, and CW wrote the manuscript.
Funding
This study was supported by the Funds for Modern Agricultural Industry Technology System in Shandong Province, China (SDAIT-06-06) and Shandong Provincial Natural Science Foundation, China (ZR2019BC038).
Conflict of Interest
The authors declare that the research was conducted in the absence of any commercial or financial relationships that could be construed as a potential conflict of interest.
Supplementary Material
The Supplementary Material for this article can be found online at: https://www.frontiersin.org/articles/10.3389/fpls.2020.00038/full#supplementary-material
References
Adler, G., Blumwald, E., Bar-Zvi, D. (2010). The sugar beet gene encoding the sodium/proton exchanger 1 (BvNHX1) is regulated by a MYB transcription factor. Planta. 232, 187–195. doi: 10.1007/s00425-010-1160-7
Ahmad, P., Abdel Latef, A. A., Hashem, A., Abd Allah, E. F., Gucel, S., Tran, L. S. (2016). Nitric oxide mitigates salt stress by regulating levels of osmolytes and antioxidant enzymes in chickpea. Front. Plant Sci. 7, 347. doi: 10.3389/fpls.2016.00347
Ali, B., Hayat, S., Ahmad, A. (2007). 28-Homobrassinolide ameliorates the saline stress in chickpea (Cicer arietinum L.). Environ. Exp. Bot. 59, 217–223. doi: 10.1016/j.envexpbot.2005.12.002
An, J. P., Yao, J. F., Xu, R. R., You, C. X., Wang, X. F., Hao, Y. J. (2018). An apple NAC transcription factor enhances salt stress tolerance by modulating the ethylene response. Physiol. Plantarum. 164, 279–289. doi: 10.1111/ppl.12724
Azhar, N., Su, N., Shabala, L., Shabala, S. (2017). Exogenously applied 24-epibrassinolide (EBL) ameliorates detrimental effects of salinity by reducing K+ efflux via depolarization-activated K+ channels. Plant Cell Physiol. 58, 802–810. doi: 10.1093/pcp/pcx026
Bajguz, A., Hayat, S. (2009). Effects of brassinosteroids on the plant responses to environmental stresses. Plant Physiolo. Bioch. 47, 1–8. doi: 10.1016/j.plaphy.2008.10.002
Barragan, V., Leidi, E. O., Andres, Z., Rubio, L., De Luca, A., Fernandez, J. A., et al. (2012). Ion exchangers NHX1 and NHX2 mediate active potassium uptake into vacuoles to regulate cell turgor and stomatal function in Arabidopsis. Plant Cell. 24, 1127–1142. doi: 10.1105/tpc.111.095273
Bassil, E., Ohto, M. A., Esumi, T., Tajima, H., Zhu, Z., Cagnac, O., et al. (2011a). The Arabidopsis intracellular Na+/H+ antiporters NHX5 and NHX6 are endosome associated and necessary for plant growth and development. Plant Cell. 23, 224–239. doi: 10.1105/tpc.110.079426
Bassil, E., Tajima, H., Liang, Y. C., Ohto, M. A., Ushijima, K., Nakano, R., et al. (2011b). The Arabidopsis Na+/H+ antiporters NHX1 and NHX2 control vacuolar pH and K+ homeostasis to regulate growth, flower development, and reproduction. Plant Cell. 23, 3482–3497. doi: 10.1105/tpc.111.089581
Cao, S., Xu, Q., Cao, Y., Qian, K., An, K., Zhu, Y., et al. (2005). Loss-of-function mutations in DET2 gene lead to an enhanced resistance to oxidative stress in Arabidopsis. Physiol. Plantarum 123, 57–66. doi: 10.1111/j.1399-3054.2004.00432.x
Carden, D. E., Walker, D. J., Flowers, T. J., Miller, A. J. (2003). Single-cell measurements of the contributions of cytosolic Na+ and K+ to salt tolerance. Plant Physiol. 131, 676–683. doi: 10.1104/pp.011445
Clouse, S. D. (1996). Molecular genetic studies confirm the role of brassinosteroids in plant growth and development. Plant J. 10, 1–8. doi: 10.1046/j.1365-313X.1996.10010001.x
Craig Plett, D., Moller, I. S. (2010). Na+ transport in glycophytic plants: what we know and would like to know. Plant Cell Environ. 33, 612–626. doi: 10.1111/j.1365-3040.2009.02086.x
Dai, W., Wang, M., Gong, X., Liu, J. H. (2018). The transcription factor FcWRKY40 of Fortunella crassifolia functions positively in salt tolerance through modulation of ion homeostasis and proline biosynthesis by directly regulating SOS2 and P5CS1 homologs. New Phytol. 219, 972–989. doi: 10.1111/nph.15240
Diedhiou, C. J., Popova, O. V., Golldack, D. (2009). Transcript profiling of the salt-tolerant Festuca rubra ssp. litoralis reveals a regulatory network controlling salt acclimatization. J. Plant Physiol. 166, 697–711. doi: 10.1016/j.jplph.2008.09.015
Dong, Y., Wang, W., Hu, G., Chen, W., Zhuge, Y., Wang, Z., et al. (2017). Role of exogenous 24-epibrassinolide in enhancing the salt tolerance of wheat seedlings. J. Soil Sci. Plant Nutt. 17, 554–569. doi: 10.4067/S0718-95162017000300001
Fariduddin, Q., Khalil, R. R., Mir, B. A., Yusuf, M., Ahmad, A. (2013). 24-Epibrassinolide regulates photosynthesis, antioxidant enzyme activities and proline content of Cucumis sativus under salt and/or copper stress. Environ. Monit. Assess. 185, 7845–7856. doi: 10.1007/s10661-013-3139-x
Fariduddin, Q., Mir, B., Yusuf, M., Ahmad, A. (2014). 24-epibrassinolide and/or putrescine trigger physiological and biochemical responses for the salt stress mitigation in Cucumis sativus L. Photosynthetica 52, 464–474. doi: 10.1007/s11099-014-0052-7
Feng, Y., Liu, J., Zhai, L., Gan, Z., Zhang, G., Yang, S., et al. (2019). Natural variation in cytokinin maintenance improves salt tolerance in apple rootstocks. Plant Cell Environ. 42, 424–436. doi: 10.1111/pce.13403
Hasegawa, P. M., Bressan, R. A., Zhu, J. K., Bohnert, H. J. (2000). Plant cellular and molecular responses to high salinity. Annu. Rev. Plant Boil. 51, 463–499. doi: 10.1146/annurev.arplant.51.1.463
Hu, D. G., Ma, Q. J., Sun, C. H., Sun, M. H., You, C. X., Hao, Y. J. (2016a). Overexpression of MdSOS2L1, a CIPK protein kinase, increases the antioxidant metabolites to enhance salt tolerance in apple and tomato. Physiol. Plantarum. 156, 201–214. doi: 10.1111/ppl.12354
Hu, Y., Xia, S., Su, Y., Wang, H., Luo, W., Su, S., et al. (2016b). Brassinolide increases potato root growth in vitro in a dose-dependent way and alleviates salinity stress. Biomed. Res. Int. 2016, 8231873. doi: 10.1155/2016/8231873
Hu, L., Zhou, K., Li, Y., Chen, X., Liu, B., Li, C., et al. (2018). Exogenous myo-inositol alleviates salinity-induced stress in Malus hupehensis Rehd. Plant Physiol. Biochem. 133, 116–126. doi: 10.1016/j.plaphy.2018.10.037
Ismail, A., Takeda, S., Nick, P. (2014). Life and death under salt stress: same players, different timing? J. Exp. Bot. 65, 2963–2979. doi: 10.1093/jxb/eru159
Kim, T. W., Guan, S., Sun, Y., Deng, Z., Tang, W., Shang, J. X., et al. (2009). Brassinosteroid signal transduction from cell-surface receptor kinases to nuclear transcription factors. Nat. Cell Biol. 11, 1254–1260. doi: 10.1038/ncb1970
Kim, Y., Song, J. H., Park, S. U., Jeong, Y. S., Kim, S. H. (2017). Brassinosteroid-induced transcriptional repression and dephosphorylation-dependent protein degradation negatively regulate BIN2-interacting AIF2 (a BR signaling-negative regulator) bHLH transcription factor. Plant Cell Physiol. 58, 227–239. doi: 10.1093/pcp/pcw223
Leidi, E. O., Barragan, V., Rubio, L., El-Hamdaoui, A., Ruiz, M. T., Cubero, B., et al. (2010). The AtNHX1 exchanger mediates potassium compartmentation in vacuoles of transgenic tomato. Plant J. 61, 495–506. doi: 10.1111/j.1365-313X.2009.04073.x
Li, Y., Zhang, Y., Feng, F., Liang, D., Cheng, L., Ma, F., et al. (2010). Overexpression of a Malus vacuolar Na+/H+ antiporter gene (MdNHX1) in apple rootstock M.26 and its influence on salt tolerance. Plant Cell Tiss. Org. 102, 337–345. doi: 10.1007/s11240-010-9738-0
Liang, B., Li, C., Ma, C., Wei, Z., Wang, Q., Huang, D., et al. (2017). Dopamine alleviates nutrient deficiency-induced stress in Malus hupehensis. Plant Physiol. Bioch. 119, 346. doi: 10.1016/j.plaphy.2017.09.012
Liang, W., Ma, X., Wan, P., Liu, L. (2018). Plant salt-tolerance mechanism: A review. Biochem. Bioph. Res. Co. 495, 286–291. doi: 10.1016/j.bbrc.2017.11.043
Liu, C., Li, C., Liang, D., Wei, Z., Zhou, S., Wang, R., et al. (2012). Differential expression of ion transporters and aquaporins in leaves may contribute to different salt tolerance in Malus species. Plant Physiol. Biochem. 58, 159–165. doi: 10.1016/j.plaphy.2012.06.019
Lopez-Gomez, M., Hidalgo-Castellanos, J., Lluch, C., Herrera-Cervera, J. A. (2016). 24-Epibrassinolide ameliorates salt stress effects in the symbiosis Medicago truncatula-Sinorhizobium meliloti and regulates the nodulation in cross-talk with polyamines. Plant Physiol. Bioch. 108, 212–221. doi: 10.1016/j.plaphy.2016.07.017
Mao, K., Dong, Q., Li, C., Liu, C., Ma, F. (2017). Genome wide identification and characterization of apple bHLH transcription factors and expression analysis in response to drought and salt stress. Front. Plant Sci. 8, 480. doi: 10.3389/fpls.2017.00480
Miller, G., Suzuki, N., Ciftci-Yilmaz, S., Mittler, R. (2010). Reactive oxygen species homeostasis and signalling during drought and salinity stresses. Plant Cell Environ. 33, 453–467. doi: 10.1111/j.1365-3040.2009.02041.x
Navarro, J. M., Garrido, C., Martínez, V., Carvajal, M. (2003). Water relations and xylem transport of nutrients in pepper plants grown under two different salts stress regimes. Plant Growth Regul. 41, 237–245. doi: 10.1023/B:GROW.0000007515.72795.c5
Poustini, K., Siosemardeh, A. (2004). Ion distribution in wheat cultivars in response to salinity stress. Field Crop Res. 85, 125–133. doi: 10.1016/S0378-4290(03)00157-6
Qiu, Q. S., Guo, Y., Quintero, F. J., Pardo, J. M., Schumaker, K. S., Zhu, J. K. (2004). Regulation of vacuolar Na+/H+ exchange in Arabidopsis thaliana by the salt-overly-sensitive (SOS) pathway. J. Biol. Chem. 279, 207–215. doi: 10.1074/jbc.M307982200
Qiu, Y., An, K., Sun, J., Chen, X., Gong, X., Ma, L., et al. (2019). Investigating the effect of methyl jasmonate and melatonin on resistance of Malus crabapple ‘Hong Jiu’ to ozone stress. Environ. Sci. Pollut. R. 26, 27761–27768. doi: 10.1007/s11356-019-05946-w
Ryu, H., Cho, Y. G. (2015). Plant hormones in salt stress tolerance. J. Plant Biol. 58, 147–155. doi: 10.1007/s12374-015-0103-z
Sadeghi, F., Shekafandeh, A. (2014). Effect of 24-epibrassinolide on salinity-induced changes in loquat (Eriobotrya japonica Lindl). J. Appl. Bot. Food Qual. 87, 182–189. doi: 10.5073/JABFQ.2014.087.026
Shahid, M. A., Balal, R. M., Pervez, M. A., Garcia-Sanchez, F., Gimeno, V., Abbas, T., et al. (2014). Treatment with 24-epibrassinolide mitigates NaCl-induced toxicity by enhancing carbohydrate metabolism, osmolyte accumulation, and antioxidant activity in Pisum sativum. Turk. J. Bot. 38, 511–525. doi: 10.3906/bot-1304-45
Shahnejat-Bushehri, S., Tarkowska, D., Sakuraba, Y., Balazadeh, S. (2016). Arabidopsis NAC transcription factor JUB1 regulates GA/BR metabolism and signalling. Nat. Plants. 2, 16013. doi: 10.1038/nplants.2016.13
Shahzad, B., Tanveer, M., Che, Z., Rehman, A., Cheema, S. A., Sharma, A., et al. (2018). Role of 24-epibrassinolide (EBL) in mediating heavy metal and pesticide induced oxidative stress in plants: a review. Ecotox. Environ. Safe 147, 935–944. doi: 10.1016/j.ecoenv.2017.09.066
Sharma, I., Bhardwaj, R., Pati, P. K. (2013). Stress modulation response of 24-epibrassinolide against imidacloprid in an elite indica rice variety Pusa Basmati-1. Pestic. Biochem. Phys. 105, 144–153. doi: 10.1016/j.pestbp.2013.01.004
Sharma, A., Thakur, S., Kumar, V., Kanwar, M. K., Kesavan, A. K., Thukral, A. K., et al. (2016). Pre-sowing seed treatment with 24-epibrassinolide ameliorates pesticide stress in Brassica juncea L. through the modulation of stress markers. Front. Plant Sci. 7, 1569. doi: 10.3389/fpls.2016.01569
Sharma, V., Goel, P., Kumar, S., Singh, A. K. (2019). An apple transcription factor, MdDREB76, confers salt and drought tolerance in transgenic tobacco by activating the expression of stress-responsive genes. Plant Cell Rep. 38, 221–241. doi: 10.1007/s00299-018-2364-8
Sun, Y., Fan, X. Y., Cao, D. M., Tang, W., He, K., Zhu, J. Y., et al. (2010). Integration of brassinosteroid signal transduction with the transcription network for plant growth regulation in Arabidopsis. Dev. Cell 19, 765–777. doi: 10.1016/j.devcel.2010.10.010
Sun, H., Fan, H. J., Ling, H. Q. (2015a). Genome-wide identification and characterization of the bHLH gene family in tomato. BMC Genomics 16, 9. doi: 10.1186/s12864-014-1209-2
Sun, S., An, M., Han, L., Yin, S. (2015b). Foliar application of 24-epibrassinolide improved salt stress tolerance of perennial ryegrass. Hortic. Sci. 50, 1518–1523. doi: 10.21273/HORTSCI.50.10.1518
Talaat, N. B., Shawky, B. T. (2012). 24-Epibrassinolide ameliorates the saline stress and improves the productivity of wheat (Triticum aestivum L.). Environ. Exp. Bot. 82, 80–88. doi: 10.1016/j.envexpbot.2012.03.009
Tanveer, M., Shahzad, B., Sharma, A., Biju, S., Bhardwaj, R. (2018). 24-Epibrassinolide; an active brassinolide and its role in salt stress tolerance in plants: a review. Plant Physiol. Bioch. 130, 69–79. doi: 10.1016/j.plaphy.2018.06.035
Tofighi, C., Khavari-Nejad, R. A., Najafi, F., Razavi, K., Rejali, F. (2017). Responses of wheat plants to interactions of 24-epibrassinolide and Glomus mosseae in saline condition. Physiol. Mol. Boil. Pla. 23, 557–564. doi: 10.1007/s12298-017-0439-6
Wang, Z. Y., Bai, M. Y., Oh, E., Zhu, J. Y. (2012). Brassinosteroid signaling network and regulation of photomorphogenesis. Annu. Rev. Genet. 46, 701–724. doi: 10.1146/annurev-genet-102209-163450
Wani, A. S., Tahir, I., Ahmad, S. S., Dar, R. A., Nisar, S. (2017). Efficacy of 24-epibrassinolide in improving the nitrogen metabolism and antioxidant system in chickpea cultivars under cadmium and/or NaCl stress. Sci. Hortic. 225, 48–55. doi: 10.1016/j.scienta.2017.06.063
Wu, W., Zhang, Q., Ervin, E. H., Yang, Z., Zhang, X. (2017). Physiological mechanism of enhancing salt stress tolerance of perennial ryegrass by 24-epibrassinolide. Front. Plant Sci. 8, 1017. doi: 10.3389/fpls.2017.01017
Yang, Y., Guo, Y. (2018). Elucidating the molecular mechanisms mediating plant salt-stress responses. New Phytol. 217, 523–539. doi: 10.1111/nph.14920
Ye, H., Li, L., Guo, H., Yin, Y. (2012). MYBL2 is a substrate of GSK3-like kinase BIN2 and acts as a corepressor of BES1 in brassinosteroid signaling pathway in Arabidopsis. Proc. Natl. Acad. Sci. U. S. A. 109, 20142–20147. doi: 10.1073/pnas.1205232109
Yin, Y., Wang, Z. Y., Mora-Garcia, S., Li, J., Yoshida, S., Asami, T., et al. (2002). BES1 accumulates in the nucleus in response to brassinosteroids to regulate gene expression and promote stem elongation. Cell. 109, 181–191. doi: 10.1016/S0092-8674(02)00721-3
Yuan, L., Shu, S., Sun, J., Guo, S., Tezuka, T. (2012). Effects of 24-epibrassinolide on the photosynthetic characteristics, antioxidant system, and chloroplast ultrastructure in Cucumis sativus L. under Ca(NO3)2 stress. Photosynth. Res. 112, 205–214. doi: 10.1007/s11120-012-9774-1
Yusuf, M., Fariduddin, Q., Ahmad, A. (2012). 24-epibrassinolide modulates growth, nodulation, antioxidant system, and osmolyte in tolerant and sensitive varieties of Vigna radiata under different levels of nickel: a shotgun approach. Plant Physiol. Bioch. 57, 143–153. doi: 10.1016/j.plaphy.2012.05.004
Yusuf, M., Fariduddin, Q., Khan, T., Hayat, S. (2017). Epibrassinolide reverses the stress generated by combination of excess aluminum and salt in two wheat cultivars through altered proline metabolism and antioxidants. S. Afr. J. Bot. 112, 391–398. doi: 10.1016/j.sajb.2017.06.034
Zheng, X., Zhou, J., Tan, D. X., Wang, N., Wang, L., Shan, D., et al. (2017). Melatonin improves waterlogging tolerance of Malus baccata (Linn.) Borkh. seedlings by maintaining aerobic respiration, photosynthesis and ROS migration. Front. Plant Sci. 8, 483. doi: 10.3389/fpls.2017.00483
Zheng, X., Zhao, Y., Shan, D., Shi, K., Wang, L., Li, Q., et al. (2018). MdWRKY9 overexpression confers intensive dwarfing in the M26 rootstock of apple by directly inhibiting brassinosteroid synthetase MdDWF4 expression. New Phytol. 217, 1086–1098. doi: 10.1111/nph.14891
Zhu, J. K. (2002). Salt and drought stress signal transduction in plants. Annu. Rev. Plant Boil. 53, 247–273. doi: 10.1146/annurev.arplant.53.091401.143329
Keywords: brassinolide, oxidative stress, plant hormone, ion homeostasis, MhNHXs
Citation: Su Q, Zheng X, Tian Y and Wang C (2020) Exogenous Brassinolide Alleviates Salt Stress in Malus hupehensis Rehd. by Regulating the Transcription of NHX-Type Na+(K+)/H+ Antiporters. Front. Plant Sci. 11:38. doi: 10.3389/fpls.2020.00038
Received: 19 June 2019; Accepted: 13 January 2020;
Published: 06 February 2020.
Edited by:
Jayakumar Bose, University of Adelaide, AustraliaReviewed by:
Kamrun Nahar, Sher-e-Bangla Agricultural University, BangladeshChanghai Liu, Northwest A&F University, China
Copyright © 2020 Su, Zheng, Tian and Wang. This is an open-access article distributed under the terms of the Creative Commons Attribution License (CC BY). The use, distribution or reproduction in other forums is permitted, provided the original author(s) and the copyright owner(s) are credited and that the original publication in this journal is cited, in accordance with accepted academic practice. No use, distribution or reproduction is permitted which does not comply with these terms.
*Correspondence: Caihong Wang, chw6068@126.com
†These authors have contributed equally to this work