- 1Department of Biology, University of Padova, Padova, Italy
- 2Botanical Garden, University of Padova, Padova, Italy
Calcium has long been known to regulate the metabolism of chloroplasts, concerning both light and carbon reactions of photosynthesis, as well as additional non photosynthesis-related processes. In addition to undergo Ca2+ regulation, chloroplasts can also influence the overall Ca2+ signaling pathways of the plant cell. Compelling evidence indicate that chloroplasts can generate specific stromal Ca2+ signals and contribute to the fine tuning of cytoplasmic Ca2+ signaling in response to different environmental stimuli. The recent set up of a toolkit of genetically encoded Ca2+ indicators, targeted to different chloroplast subcompartments (envelope, stroma, thylakoids) has helped to unravel the participation of chloroplasts in intracellular Ca2+ handling in resting conditions and during signal transduction. Intra-chloroplast Ca2+ signals have been demonstrated to occur in response to specific environmental stimuli, suggesting a role for these plant-unique organelles in transducing Ca2+-mediated stress signals. In this mini-review we present current knowledge of stimulus-specific intra-chloroplast Ca2+ transients, as well as recent advances in the identification and characterization of Ca2+-permeable channels/transporters localized at chloroplast membranes. In particular, the potential role played by cMCU, a chloroplast-localized member of the mitochondrial calcium uniporter (MCU) family, as component of plant environmental sensing is discussed in detail, taking into account some specific structural features of cMCU. In summary, the recent molecular identification of some players of chloroplast Ca2+ signaling has opened new avenues in this rapidly developing field and will hopefully allow a deeper understanding of the role of chloroplasts in shaping physiological responses in plants.
Introduction
Calcium is a fundamental intracellular messenger involved in a wide range of different signaling pathways in all eukaryotes. In plants, Ca2+ has been shown to participate in the transduction of a large variety of environmental stimuli of both abiotic and biotic nature (Dodd et al., 2010). A complex Ca2+ homeostatic and signaling machinery allows for a tight regulation of the intracellular concentration of the ion ([Ca2+]) and its variations during signal transduction (Kudla et al., 2018). Plant organellar Ca2+ signaling is a rapidly expanding field of investigation, also thanks to the increasing availability of novel genetically encoded Ca2+ indicators, specifically targeted to different intracellular compartments (Costa et al., 2018). In addition to the vacuole, considered as the main stimulus-releasable Ca2+ store in the plant cell, other organelles, i.e. chloroplasts, have recently come to the fore. The detection of stimulus-specific intra-chloroplast Ca2+ signals in response to different environmental cues has highlighted the contribution of chloroplasts to shaping cytosolic Ca2+ signatures. In this mini-review we present the most recent research works dealing with the monitoring of chloroplast Ca2+ concentration and its changes during signal transduction events. Moreover, we focus on the recently reported identification and biochemical characterization of some molecular players involved in chloroplast Ca2+ handling. Current evidence for a crucial role of chloroplasts as stress sensors and future avenues of investigation in this promising field are also discussed.
The Emerging Role of Chloroplast Calcium Signaling in the Transduction of Biotic and Abiotic Stress Signals
Chloroplasts have long been known to be involved in intracellular Ca2+ homeostasis and signaling. The regulatory role played by these organelles on intracellular Ca2+ handling is two-fold: i) a tight control of intra-organellar [Ca2+] is essential for the proper functioning of the chloroplast physiology, e.g. the regulation of photosynthesis, as well as other chloroplast-localized processes (Stael et al., 2012b; Rocha and Vothknecht, 2012; Nomura and Shiina, 2014; Hochmal et al., 2015); ii) transient changes in stromal [Ca2+] ([Ca2+]str), evoked in response to different stress stimuli, in turn can shape intracellular Ca2+ signals, thereby affecting Ca2+-mediated signaling circuits.
After the pioneering work conducted by Johnson et al. (Johnson et al., 1995) and Sai and Johnson (Sai and Johnson, 2002), who monitored [Ca2+] in the chloroplast stroma by means of a chloroplast-targeted aequorin chimera, precise measurements of Ca2+ levels inside the different chloroplast subcompartments have been lacking for a long time. However, in the last few years the increasing availability of specifically targeted Ca2+ reporters has rapidly expanded the possibility of accurately monitoring organellar Ca2+ dynamics. The set up of a toolkit of aequorin-based probes targeted to the different subcompartments of chloroplasts (outer and inner envelope membranes, stroma, thylakoids) has allowed for the elucidation of stimulus-specific intra-organellar Ca2+ signals and their contribution to fine-tuning cytosolic Ca2+ signatures (Mehlmer et al., 2012; Sello et al., 2016; Sello et al., 2018). A complementary approach based on the design of a cameleon probe directed to the chloroplast stroma further permitted Ca2+ imaging in single chloroplasts, highlighting organelle-autonomous Ca2+ transients (Loro et al., 2016). The establishment of aequorin reporters targeted to the thylakoid lumen and thylakoid membrane highlighted the ability of thylakoids to store 3- to 5-fold higher [Ca2+] with respect to the stroma (about 500 nM in the thylakoid lumen versus 100÷150 nM in the stroma, in resting conditions in the dark), as well as their contribution to the modulation of intra-chloroplast Ca2+ signals (Sello et al., 2018).
Chloroplast Ca2+ signals have been shown to be triggered by a large number of different stimuli of both biotic and abiotic nature. Elicitors of plant defence responses, such as the fungal-derived protein cryptogein and the plant cell wall-derived pectin fragments oligogalacturonides, were found to evoke transient Ca2+ elevations in the chloroplast stroma of Nicotiana tabacum and Arabidopsis thaliana plant cell suspension cultures (Manzoor et al., 2012; Sello et al., 2018). Moreover, the bacterial flagellin peptide flg22 was demonstrated to trigger a chloroplast Ca2+ response in the chloroplast stroma of Arabidopsis rosette leaves, peaking later than the cytosolic Ca2+ elevation (Nomura et al., 2012; Nomura and Shiina, 2014). In this latter work, a striking chloroplast-mediated transcriptional reprogramming during plant immune responses was demonstrated, uncovering an unanticipated link between chloroplast and nuclear plant innate immunity via ROS and Ca2+ signaling (Stael et al., 2015). The calcium-sensing receptor CAS, a thylakoid-localized protein of not yet well-defined function, was found to be involved in the generation of the flg22-induced stromal Ca2+ transient and chloroplast-mediated activation of defence gene expression (Nomura et al., 2012).
Different abiotic cues, such as cold, oxidative, salt and osmotic stresses were found to evoke stimulus-specific Ca2+ signals in the chloroplast stroma (Nomura et al., 2012; Sello et al., 2016; Sello et al., 2018; Teardo et al, 2019). Whereas these stimuli were shown to activate Ca2+ responses in both chloroplasts and non-green plastids (Sello et al., 2016), the light-to-dark transition was found to elicit a chloroplast-specific response (Sello et al., 2016; Loro et al., 2016). Although the precise mechanisms underlying dark-induced chloroplast Ca2+ fluxes remain to be unravelled, the circadian gating of dark-induced chloroplast and cytosolic Ca2+ elevations has recently been demonstrated (Martí Ruiz et al., 2020), uncovering an intriguing link between eukaryotic circadian clocks and chloroplasts.
In contrast to the above-mentioned stimuli, that have been demonstrated to trigger Ca2+ transients in both chloroplasts and the cytosol, increases in absolute temperature were found to evoke Ca2+ responses specific to chloroplasts, as no corresponding elevations were detected in the cytosol (Lenzoni and Knight, 2019). Interestingly, also in this case the chloroplast Ca2+ response was found to be partially dependent on CAS (Lenzoni and Knight, 2019).
Taken together, the above findings strongly highlight the ability of chloroplasts to perceive and transduce environmental signals in a Ca2+-dependent manner. However, compared to the large amount of information progressively cumulating on the generation of chloroplast Ca2+ signals, information about Ca2+-permeable channels/transporters localized at chloroplast membranes has long lagged behind.
Current Knowledge of the Molecular Players Involved in Ca2+ Handling in Chloroplasts
The extent, duration and frequency (i.e. signature) of free Ca2+ elevation in the cytosol ([Ca2+]cyt) acts as a signal to be implemented in the transducing machinery of the cell. Different stimuli are followed by different Ca2+ signatures, leading in turn to different specific responses, in terms of gene expression, protein activity and localization. The Ca2+ signature is shaped by the activity of Ca2+-permeable channels and transporters regulating the ion entry into and exit from the cytosol, respectively. Ca2+-permeable channels are grouped in five families: cyclic nucleotide-gated channels (CNGCs), glutamate receptors-like channels (GLRs), two-pore channels (TPCs), mechanosensitive channels (MCAs), hyperosmolality gated channels (OSCAs) (Demidchik et al., 2018). Ca2+ transport off the cytosol to restore the resting [Ca2+]cyt is mediated by energy-driven pumps/transporters belonging to the P-type ATPases, such as P1B-type calcium/heavy metal cation-transporting ATPase (AtHMA1), P2A-type calcium cation-transporting ATPase (ECAs) and P2B-type calcium cation-transporting ATPase (ACAs) (García Bossi et al., 2020). Other Ca2+ transporters are grouped in the CaCA family (CAX-type proton:calcium cation exchanger, CCX-type cation:calcium cation exchanger, MHX-type proton:magnesium cation exchanger, NCL/EF-CAX-type cation exchanger, EF-CAX-type cation exchanger) (Pittman and Hirschi, 2016) and CaCA2 family (PAM71-type manganese/calcium cation transporter).
The transduction of the Ca2+ signal is mediated by Ca2+ -dependent/binding proteins. The Arabidopsis genome encodes for 250 proteins harbouring at least one Ca2+ binding domain (EF-hand), hence acting as putative Ca2+ sensors [e.g. (Ranty et al., 2016)]. Calmodulins (CaMs), calmodulin-like (CaMLs), calcineurin B-like proteins (CBLs) and Ca2+-dependent protein kinases (CPKs) all harbour EF hand motifs. Ca2+ sensors directly (CPKs) or indirectly (CaMs, CaMLs, CBLs) [e.g. (Sanyal et al., 2015; Kudla et al., 2018)] modulate protein activity (e.g. ion channels, metabolic enzymes) and/or protein subcellular localization (e.g. transcription factors). The redundancy of sensor isoforms allows the discrimination between different signals and carry the specificity of the message brought by the Ca2+ signature.
To our knowledge, Ca2+-binding proteins acting as buffers in the chloroplast have not yet been identified. Nevertheless, organellar Ca2+ buffering mechanisms are likely to play an essential role, generating heterogeneity in local Ca2+ concentrations inside chloroplasts. How Ca2+ is stored in the chloroplast remains an open question for future investigations, aimed to unravel whether Ca2+ interacts with specific Ca2+ binding proteins or with the thylakoid surface, which harbours a significant amount of phosphorylated proteins that have been suggested to bind calcium ions (Rocha and Vothknecht, 2012; Stael et al., 2012a; Stael et al., 2012b).
The major part of research carried out so far has focused on the analysis of the cytosolic Ca2+ signature, but the possibility to study Ca2+ dynamics in organelles by targeting Ca2+ probes to plastids has recently allowed the understanding of the existence of organellar Ca2+ transients in response to external stimuli. These findings pose the question of the identity of players involved in shaping and transducing the Ca2+ signal coming from organelles. The existence of peculiar and dedicated pathways for Ca2+ handling in organelles can be a possibility, and/or the machinery may comprise some already known players that may localize to chloroplasts as well (Finazzi et al., 2015; Pottosin and Shabala, 2015; Carraretto et al., 2016).
Recently, two proteins belonging to the family of the mitochondrial calcium uniporter (MCU) have been found to mediate Ca2+ transport across the mitochondria and chloroplast membranes, respectively AtMCU1 (Teardo et al, 2017) and AtMCU6 (later renamed AtcMCU (Teardo et al., 2019). In animal cells the only isoform, MCU (De Stefani et al., 2011; Baughman, 2011) is responsible for Ca2+ loading into mitochondria, thus helping recovery of resting [Ca2+]cyt. New evidence supports the involvement of MCU isoforms in shaping the organellar Ca2+ signatures in plants as well (Wagner et al., 2015; Teardo et al., 2017; Selles et al., 2018; Teardo et al., 2019). In particular, cMCU is involved in the generation of the stromal Ca2+ transient specific for the osmotic stress and mutants lacking cMCU showed an improved drought tolerance (Stael, 2019; Teardo et al., 2019).
It is now commonly acknowledged that a protein can localize to different cell compartments (Karniely and Pines, 2005), as it has been proven also for proteins involved in Ca2+ handling (Table 1). AtGLR3.4 and AtGLR3.5, two Ca2+ -permeable channels belonging to the GLR family, have a dual localization, at the plasma membrane and chloroplasts the former (Teardo et al., 2010; Teardo et al., 2011), in mitochondria and chloroplasts the latter (Teardo et al., 2015). Both seem to play a role in ABA signaling under abiotic stress (Cheng et al., 2018; Ju et al., 2020), although their direct involvement in organellar Ca2+ signaling under abiotic stress has to be investigated more in depth.
Querying the protein databases Uniprot (The UniProt Consortium, 2019), SUBA4 (Hooper et al., 2017) and Aramemnon (Schwacke et al., 2003) for A. thaliana records with plastidial localization and using “calcium” as keyword, 682 hits can be found in SUBA4, only 43 in Aramemnon and 42 in Uniprot. Table 1 shows all those proteins belonging to the above-mentioned classes of channels/transporters, sensors and kinases involved in Ca2+ signature formation and signaling, whose plastidial localization has been predicted or demonstrated by MS/MS or by fusion to fluorescent proteins (FP).
23 out of 47 proteins belong to Ca2+ channels/transporters: 6 are confirmed to be located in plastid membranes either by biochemical and cell biology methods or by mass spectrometry. Among them, for AtcMCU, AtGLR3.4 and AtGLR3.5 a role in stress response was suggested. Altogether, these channels/transporters can be involved in the formation of the plastidial Ca2+ transients, along with the putative calcium-transporting protein PAM71/BICAT (Frank et al., 2019). However, this latter protein seems to play a prevalent role in manganese homeostasis rather than in calcium homeostasis (Schneider et al., 2016; Zhang et al., 2018). In addition to Ca2+ channels and transporters, Ca2+ sensors, namely 21 proteins, are predicted to be located in plastids. However, only three have been confirmed so far: AtCPK20, AtCPK31, and AtCAS. It is worth to mention that CPK20, besides the plastidial localization that was confirmed by MS/MS approaches (Behrens et al., 2013), showed a plasma membrane localization when fused to reporter genes or co-expressed with other CPK members (Gutermuth et al., 2013). CPK31 has also been shown to localize at the plasma membrane when interacting with the arsenite transporter NIP1;1 (Ji et al., 2017). In addition, localization of many CPKs with chloroplast-targeting sequence can be affected by N-acylation. For example, AtCPK20 and 31 are located in the chloroplast, only if its N-acylation is prevented (Stael et al., 2011). Interestingly, AtGRF9, a Ca2+-regulated 14-3-3 protein, although not predicted to be located in chloroplasts, has been demonstrated to be present in many compartments, including plastids. This regulatory protein is involved in root and leaf development under water stress (He et al., 2015) and leaf development in general (Omidbakhshfard et al., 2018), but its role in chloroplasts has not yet been explored.
The presence of members of protein families involved in Ca2+ transport/sensing supports the idea of a core-machinery determining the observed Ca2+ transients in the chloroplast stroma, and putatively in the thylakoid lumen as well. Ca2+ sensors are indeed present in plastids, although their activity in deciphering organellar Ca2+ signatures has not been fully demonstrated so far. Nevertheless, a recent work points to CAS as mediator of light response and photoacclimation (Cutolo et al., 2019).
The multiple localizations shown by some proteins in Table 1 awaits further investigation. Recent evidence is pointing to the hypothesis of an inter-connection between organelles and nucleus for material exchanging or signal propagation (Kmiecik et al., 2016). The presence of the Ca2+ handling machinery in multiple positions can be part of the retrograde signaling in response to adverse environmental conditions (Pornsiriwong et al., 2017).
Structural and Functional Comparison Between MCU Isoforms From Different Organisms and the Chloroplast-Localized Homologue in Plants
As mentioned above, AtcMCU is one of the very few molecular entities among the plastidial Ca2+ channels/transporters shown to work as a Ca2+-permeable ion channel, to mediate indeed Ca2+ flux across chloroplast envelope and to participate in the drought stress response in Arabidopsis. While many organisms have only one MCU isoform (Bick et al., 2012), Arabidopsis harbours 6 different isoforms: 5 with clear predicted subcellular localization to mitochondria, whereas AtMCU6/At5g66650 has a predicted localization to either chloroplasts and/or to mitochondria. Localization prediction was confirmed for AtMCU1/At1g09575 (Teardo et al., 2017), AtMCU2/At1g57610 (Wagner et al., 2015; Selles et al., 2018), AtMCU3/At2g23790 (Carraretto et al., 2016). For AtMCU6 an interesting situation was observed: in tissues harbouring mature chloroplasts, AtMCU6 was efficiently targeted to these photosynthetic organelles, whereas in roots the protein was found in mitochondria (Teardo et al., 2019). Thus, either plastid-specific partners promote targeting of AtMCU6/AtcMCU or targeting depends on the metabolic state of a given cell. However, among the possible partners (https://string-db.org/network/3702.AT5G66650.1) no proteins with unique localization to chloroplasts are present. Thus, the mechanism by which dual localization occurs awaits clarification.
The N-terminal domain (NTD) of AtcMCU harbours motifs rich in acidic residues, one of which (107-118) playing a role in Ca2+ uptake by cMCU, as demonstrated by mutagenesis studies (D107A/E118K mutant) and Ca2+ uptake assays in an aequorin-based E. coli system (Teardo et al., 2019). Two groups independently set up the same system to study MCU activity, namely that exploiting E. coli stably expressing aequorin (Teardo et al., 2019) or the fluorescent Ca2+ reporter GCaMP2 (Fan et al., 2018). This valuable tool allows a quick screening of the effect of MCU residues' mutations and of chemical modulators on the Ca2+ flux-mediating activity and may become a method of choice for further structure-function studies.
One common feature of MCU homologs from fungi and Arabidopsis is that they can function as Ca2+-permeable channels on their own in contrast to vertebrates, where the uniporter is a complex (MCUC) consisting of multiple subunits, including: 1) the channel forming unit (MCU) with two transmembrane segments and a conserved DXXE sequence forming the Ca2+ selectivity filter (see Figure 1); 2) regulatory EF-hand proteins MICU1-3; 3) a small, single-pass transmembrane protein, EMRE (Essential MCU REgulator) [for review see e.g.(Wagner et al., 2016)]. The structure of MCU homologs from various organisms has been recently solved: 1) from Fusarium graminearum and Metarhizium acridum revealing a dimer assembly of MCU (Fan et al., 2018); 2) from Neurospora crassa (Yoo et al., 2018); 3) from Neosartorya fischeri (Nguyen et al., 2018b); and from 4) zebrafish and Cyphellophora europaea (Baradaran et al., 2018). All these homologues share high sequence similarity in their transmembrane domains, show a similar pore architecture and a high structural similarity of the NTDs (despite relatively low sequence homology). The amino acid sequence is more similar between Arabidopsis and Dictyostelium discoideum than between AtMCUs and human MCU (Teardo et al., 2017). This similarity apparently translates also to the tertiary structure of the two proteins, at least regarding the N-terminal domain, whose structure has been recently resolved for Dictyostelium MCU, proving its divergent evolution (doi: https://doi.org/10.1101/848002) (see Figure 1).
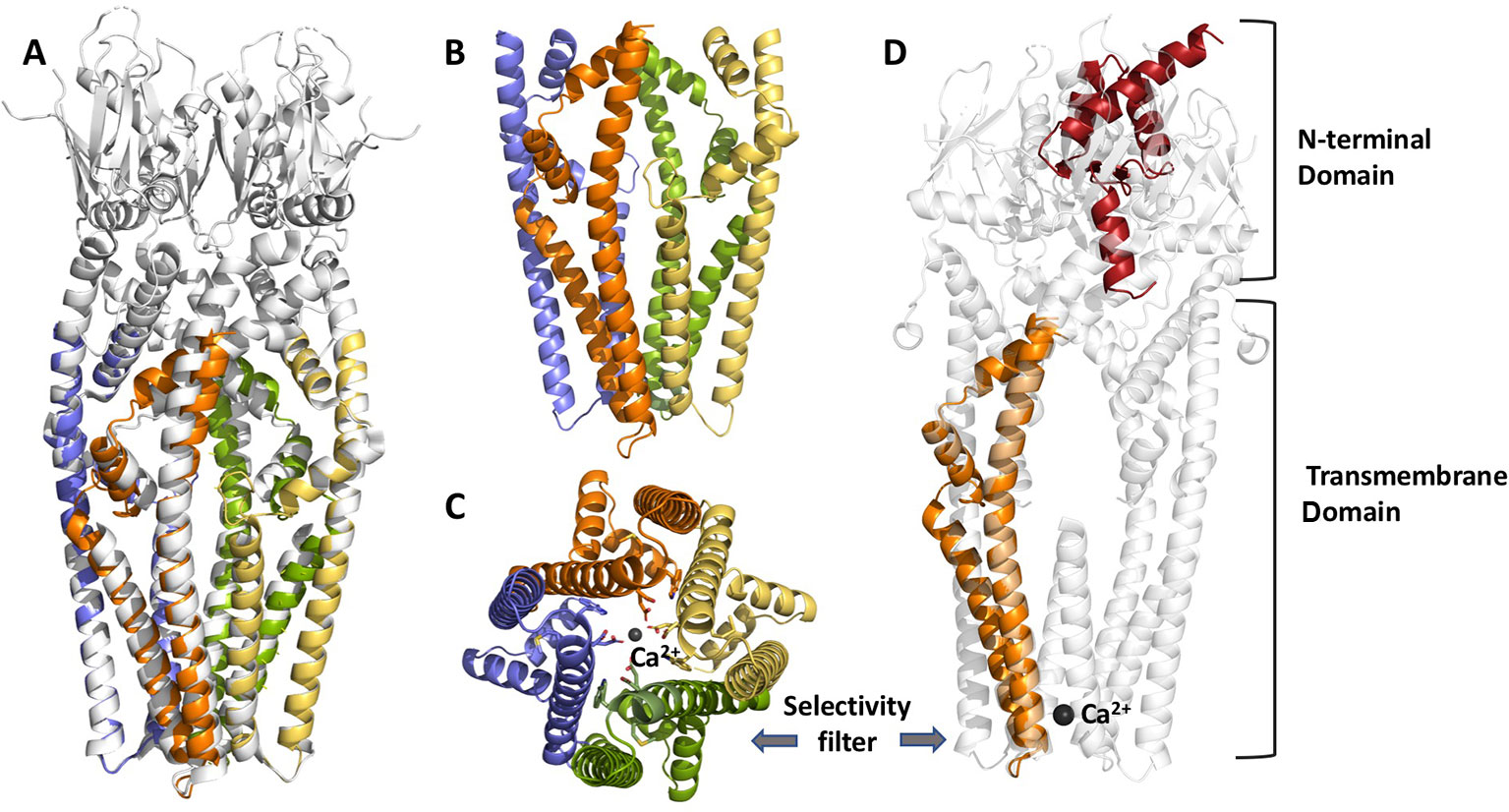
Figure 1 Structural features of chloroplast MCU from Arabidopsis thaliana, modelled by Phyre V 2.0. From the left to the right: (panel A) cartoon view of the superposition of MCU structure from Neurospora crassa (cryoEM, 3.7 Å resolution, PDB:6DT0, grey), used as a reference, and predicted A. thaliana cMCU transmembrane tetrameric assembly (coloured chains); (panel B, C) details of the transmembrane (TM) and coiled-coil domain (CCD) tetrameric assembly and selectivity filter (panel C), where the four chains are shown in yellow, orange, pale violet and green. The key acidic residues within the highly conserved motif (WDXXEP, where X is any hydrophobic residue) of cMCU are highlighted in sticks, as well as the coordinated calcium ion, shown as dark grey sphere; (panel D) superposition of the model of one monomer of A. thaliana cMCU channel (orange and red) and N. crassa MCU tetramers (light grey); cMCU model shown here includes the transmembrane domain (TM), part of the coil-coiled region and the N-terminal domain (NTD), the last predicted according to our previous homology searches and its similarity toward Dictyostelium discoideum NTD (PDB:5Z2H, doi: https:/doi.org/10.1101/848002). The superposition underlines the divergence from metazoan NTDs and other structure-known fungal homologues such as NcMCU, CeMCU, MaMCU, and NfMCU.
In plants and fungi, the pore-forming unit MCU alone is able to allow Ca2+ flux, without the need of EMRE, as confirmed by different groups (Tsai et al., 2016; Teardo et al., 2017; Fan et al., 2018; Teardo et al., 2019). In fact, homologs of EMRE are not present in these organisms. The cryo-EM structure of the human MCU-EMRE complex (Wang et al., 2019) suggests that NTD mediates the dimerization of two human MCU tetramers, thereby modulating the function of the channel [although deletion of NTD does not affect Ca2+ flux (Lee et al., 2015)]. In contrast to other MCUs, an (R/K)/Q/(R/K/D)/K/L motif is found in the L2 (Oxenoid et al., 2016) (now called CC2a for coiled-coiled domain 2a) (Wang et al., 2019) region of Arabidopsis, Dictyostelium and NfMCU (Teardo et al., 2017; Wang et al., 2019), all being able to form functional MCU without EMRE. It has been proposed that the extended side chain of HsMCU R297 (missing in the above MCUs) on CC2a connects the gate-forming juxtamembrane loop (JML) of MCU to EMRE by forming hydrogen bonds with the hydroxyl group of highly conserved T285 (on the JML of MCU) and a valine residue of EMRE. Interaction between CC2a and EMRE has been proposed as a crucial factor determining the conductivity of the channel formed by MCU tetramers. On the other hand, in the EMRE-independent Dictyostelium MCU, deletion of either CC1 or CC2 caused the loss of function of MCU (Yamamoto et al., 2019), suggesting that these two domains are crucial for MCU function independently of their ability to bind EMRE. Altogether, determination of structural differences among various MCUs accounting for the requirement of EMRE for channel function requires further work.
Conclusions and Perspectives
In these last few years there has been a surge of papers on Ca2+ signaling in chloroplasts, witnessing the crucial role increasingly attributed to these plant-unique organelles in the orchestration of the complex Ca2+ signaling network of the plant cell. We foresee that the newly available experimental tools to investigate the role of thylakoids in Ca2+-mediated signal transduction, the molecular identification of Ca2+ channels/transporters in chloroplast membranes and the determination of the structure of transmembrane proteins by cryo-EM will lead to a rapid development of this exciting field of plant research. Future plant organellar Ca2+ signaling studies should also focus on non-photosynthetic plastids, which have recently been proposed to trigger tissue-specific signaling involved in mounting plant systemic stress response (Beltran et al., 2018). Furthermore, the potential interplay of chloroplasts with other intracellular Ca2+-mobilizable stores should also be taken into consideration, in view of the well-known structural and functional interactions established by plastids with other organelles (Mathur et al., 2012).
Author Contributions
LN, EF, and IS jointly contributed to the writing of this manuscript. LC designed the structural model of cMCU presented in Figure 1. All authors reviewed and approved the final version of the submitted manuscript.
Funding
This work was supported by HFSP RG0052 to IS and the University of Padova (PRID 2018, BIRD180317) to LN.
Conflict of Interest
The authors declare that the research was conducted in the absence of any commercial or financial relationships that could be construed as a potential conflict of interest.
References
Alexandersson, E., Saalbach, G., Larsson, C., Kjellbom, P. (2004). Arabidopsis plasma membrane proteomics identifies components of transport, signal transduction and membrane trafficking. Plant Cell Physiol. 45, 1543–1556. doi: 10.1093/pcp/pch209
Anil, V. S., Rajkumar, P., Kumar, P., Mathew, M. K. (2008). A plant Ca2+ pump, ACA2, relieves salt hypersensitivity in yeast. Modulation of cytosolic calcium signature and activation of adaptive Na+ homeostasis. J. Biol. Chem. 283 (6), 3497–3506. doi: 10.1074/jbc.M700766200
Astegno, A., Bonza, M. C., Vallone, R., La Verde, V., D'Onofrio, M., Luoni, L., et al. (2017). Arabidopsis calmodulin-like protein CML36 is a calcium Ca2+ sensor that interacts with the plasma membrane Ca(2+)-ATPase isoform ACA8 and stimulates its activity. J. Biol. Chem. 292, 15049–15061. doi: 10.1074/jbc.M117.787796
Baliardini, C., Meyer, C. L., Salis, P., Saumitou-Laprade, P., Verbruggen, N. (2015). CATION EXCHANGER1 Cosegregates with Cadmium tolerance in the metal hyperaccumulator Arabidopsis halleri and plays a role in limiting oxidative stress in Arabidopsis Spp. Plant Physiol. 169, 549–559. doi: 10.1104/pp.15.01037
Baradaran, R., Wang, C., Siliciano, A. F., Long, S. B. (2018). Cryo-EM structures of fungal and metazoan mitochondrial calcium uniporters. Nature 559, 580–584. doi: 10.1038/s41586-018-0331-8
Baughman, J. M. (2011). Integrative genomics identifies MCU as an essential component of the mitochondrial calcium uniporter. Nature 476, 341–345. doi: 10.1038/nature10234
Behrens, C., Blume, C., Senkler, M., Eubel, H., Peterhansel, C., Braun, H. P. (2013). The ‘protein complex proteome’ of chloroplasts in Arabidopsis thaliana. J. Proteomics 91, 73–83. doi: 10.1016/j.jprot.2013.07.001
Beltran, J., Wamboldt, Y., Sanchez, R., Labrant, E. W., Kundariya, H., Virdi, K. S., et al. (2018). Specialized plastids trigger tissue-specific signaling for systemic stress response in plants. Plant Physiol. 178, 672–683. doi: 10.1104/pp.18.00804
Benschop, J. J., Mohammed, S., O'Flaherty, M., Heck, A. J., Slijper, M., Menke, F. L. (2007). Quantitative phosphoproteomics of early elicitor signaling in Arabidopsis. Mol. Cell Proteomics 6, 1198–1214. doi: 10.1074/mcp.M600429-MCP200
Bernfur, K., Larsson, O., Larsson, C., Gustavsson, N. (2013). Relative abundance of integral plasma membrane proteins in Arabidopsis leaf and root tissue determined by metabolic labeling and mass spectrometry. PloS One 8, e71206. doi: 10.1371/journal.pone.0071206
Bick, A. G., Calvo, S. E., Mootha, V. K. (2012). Evolutionary diversity of the mitochondrial calcium uniporter. Science 336, 886. doi: 10.1126/science.1214977
Carraretto, L., Teardo, E., Checchetto, V., Finazzi, G., Uozumi, N., Szabo, I. (2016). Ion channels in plant bioenergetic organelles chloroplast and mitochondria: from molecular identification to function. Mol. Plant 9, 371–395. doi: 10.1016/j.molp.2015.12.004
Chen, Y., Hoehenwarter, W., Weckwerth, W. (2010). Comparative analysis of phytohormone-responsive phosphoproteins in Arabidopsis thaliana using TiO2-phosphopeptide enrichment and mass accuracy precursor alignment. Plant J. 63 (1), 1–17. doi: 10.1111/j.1365-313X.2010.04218.x
Cheng, N. H., Pittman, J. K., Shigaki, T., Hirschi, K. D. (2002). Characterization of CAX4, an Arabidopsis H(+)/cation antiporter. Plant Physiol. 128, 1245–1254. doi: 10.1104/pp.010857
Cheng, N. H., Pittman, J. K., Barkla, B. J., Shigaki, T., Hirschi, K. D. (2003). The Arabidopsis cax1 mutant exhibits impaired ion homeostasis, development, and hormonal responses and reveals interplay among vacuolar transporters. Plant Cell 15 (2), 347–364. doi: 10.1105/tpc.007385
Cheng, Y., Zhang, X., Sun, T., Tian, Q., Zhang, W. H. (2018). Glutamate receptor Homolog3.4 is involved in regulation of seed germination under salt stress in Arabidopsis. Plant Cell Physiol. 59, 978–988. doi: 10.1093/pcp/pcy034
Chigri, F., Flosdorff, S., Pilz, S., Kolle, E., Dolze, E., Gietl, C., et al. (2012). The Arabidopsis calmodulin-like proteins AtCML30 and AtCML3 are targeted to mitochondria and peroxisomes, respectively. Plant Mol. Biol. 78, 211–222. doi: 10.1007/s11103-011-9856-z
Cho, D., Kim, S. A., Murata, Y., Lee, S., Jae, S. K., Nam, H. G., et al. (2009). De-regulated expression of the plant glutamate receptor homolog AtGLR3.1 impairs long-term Ca2+-programmed stomatal closure. Plant J. 58 (3), 437–449. doi: 10.1111/j.1365-313X.2009.03789.x
Cho, D., Villiers, F., Kroniewicz, L., Lee, S., Seo, Y. J., Hirschi, K. D., et al. (2012). Vacuolar CAX1 and CAX3 influence auxin transport in guard cells via regulation of apoplastic pH. Plant Physiol. 160 (3), 1293–1302. doi: 10.1104/pp.112.201442
Conn, S. J., Gilliham, M., Athman, A., Schreiber, A. W., Baumann, U., Moller, I., et al. (2011). Cell-specific vacuolar calcium storage mediated by CAX1 regulates apoplastic calcium concentration, gas exchange, and plant productivity in Arabidopsis. Plant Cell 23, 240–257. doi: 10.1105/tpc.109.072769
Costa, A., Navazio, L., Szabo, I. (2018). The contribution of organelles to plant intracellular Calcium signaling. J. Exp. Bot. 69, 4175–4193. doi: 10.1093/jxb/ery185
Cutolo, E., Parvin, N., Ruge, H., Pirayesh, N., Roustan, V., Weckwerth, W., et al. (2019). The high light response in Arabidopsis requires the Calcium sensor protein CAS, a target of STN7- and STN8-Mediated phosphorylation. Front. Plant Sci. 10, 974. doi: 10.3389/fpls.2019.00974
Dammann, C., Ichida, A., Hong, B., Romanowsky, S. M., Hrabak, E. M., Harmon, A. C., et al. (2003). Subcellular targeting of nine calcium-dependent protein kinase isoforms from Arabidopsis. Plant Physiol. 132, 1840–1848. doi: 10.1104/pp.103.020008
De Michele, R., McFarlane, H. E., Parsons, H. T., Meents, M. J., Lao, J., Gonzalez Fernandez-Nino, S. M., et al. (2016). Free-flow electrophoresis of plasma membrane vesicles enriched by two-phase partitioning enhances the quality of the proteome from arabidopsis seedlings. J. Proteome Res. 15, 900–913. doi: 10.1021/acs.jproteome.5b00876
De Stefani, D., Raffaello, A., Teardo, E., Szabo, I., Rizzuto, R. (2011). A forty-kilodalton protein of the inner membrane is the mitochondrial calcium uniporter. Nature 476, 336–340. doi: 10.1038/nature10230
Demidchik, V., Shabala, S., Isayenkov, S., Cuin, T. A., Pottosin, I. (2018). Calcium transport across plant membranes: mechanisms and functions. New Phytol. 220, 49–69. doi: 10.1111/nph.15266
Demir, F., Horntrich, C., Blachutzik, J. O., Scherzer, S., Reinders, Y., Kierszniowska, S., et al. (2013). Arabidopsis nanodomain-delimited ABA signaling pathway regulates the anion channel SLAH3. Proc. Natl. Acad. Sci. U. S. A 110, 8296–8301. doi: 10.1073/pnas.1211667110
Dodd, A. N., Kudla, J., Sanders, D. (2010). The language of calcium signaling. Annu. Rev. Plant Biol. 61, 593–620. doi: 10.1146/annurev-arplant-070109-104628
Drakakaki, G., van de Ven, W., Pan, S., Miao, Y., Wang, J., Keinath, N. F., et al. (2012). Isolation and proteomic analysis of the SYP61 compartment reveal its role in exocytic trafficking in Arabidopsis. Cell Res. 22, 413–424. doi: 10.1038/cr.2011.129
Dunkley, T. P., Hester, S., Shadforth, I. P., Runions, J., Weimar, T., Hanton, S. L., et al. (2006). Mapping the Arabidopsis organelle proteome. Proc. Natl. Acad. Sci. U. S. A 103, 6518–6523. doi: 10.1073/pnas.0506958103
Eisenhut, M., Hoecker, N., Schmidt, S. B., Basgaran, R. M., Flachbart, S., Jahns, P., et al. (2018). The plastid envelope CHLOROPLAST MANGANESE TRANSPORTER1 is essential for manganese homeostasis in Arabidopsis. Mol. Plant 11 (7), 955–969. doi: 10.1016/j.molp.2018.04.008
Elmore, J. M., Liu, J., Smith, B., Phinney, B., Coaker, G. (2012). Quantitative proteomics reveals dynamic changes in the plasma membrane during Arabidopsis immune signaling. Mol. Cell Proteomics 11, M111.014555. doi: 10.1074/mcp.M111.014555
Fakih, Z., Ahmed, M. B., Letanneur, C., Germain, H. (2016). An unbiased nuclear proteomics approach reveals novel nuclear protein components that participates in MAMP-triggered immunity. Plant Signal Behav. 11, e1183087. doi: 10.1080/15592324.2016.1183087
Fan, C., Fan, M., Orlando, B. J., Fastman, N. M., Zhang, J., XU, Y., et al. (2018). X-ray and cryo-EM structures of the mitochondrial calcium uniporter. Nature 559, 575–579. doi: 10.1038/s41586-018-0330-9
Ferro, M., Brugiere, S., Salvi, D., Seigneurin-Berny, D., Court, M., Moyet, L., et al. (2010). AT_CHLORO, a comprehensive chloroplast proteome database with subplastidial localization and curated information on envelope proteins. Mol. Cell Proteomics 9 (6), 1063–1084. doi: 10.1074/mcp.M900325-MCP200
Finazzi, G., Petroutsos, D., Tomizioli, M., Flori, S., Sautron, E., Villanova, V., et al. (2015). Ions channels/transporters and chloroplast regulation. Cell Calcium 58, 86–97. doi: 10.1016/j.ceca.2014.10.002
Frank, J., Happeck, R., Meier, B., Hoang, M. T. T., Stribny, J., Hause, G., et al. (2019). Chloroplast-localized BICAT proteins shape stromal calcium signals and are required for efficient photosynthesis. New Phytol. 221, 866–880. doi: 10.1111/nph.15407
Fromm, S., Senkler, J., Eubel, H., Peterhansel, C., Braun, H. P. (2016). Life without complex I: proteome analyses of an Arabidopsis mutant lacking the mitochondrial NADH dehydrogenase complex. J. Exp. Bot. 67 (10), 3079–3093. doi: 10.1093/jxb/erw165
García Bossi, J., Kumar, K., Barberini, M. L., Domínguez, G. D., Rondón Guerrero, Y. D. C., Marino-Buslje, C., et al. (2020). The role of P-type IIA and P-type IIB Ca2+-ATPases in plant development and growth. J. Exp. Bot. 71, 1239–1248. doi: 10.1093/jxb/erz521
Geiger, D., Scherzer, S., Mumm, P., Marten, I., Ache, P., Matschi, S., et al. (2010). Guard cell anion channel SLAC1 is regulated by CDPK protein kinases with distinct Ca2+ affinities. Proc. Natl. Acad. Sci. U. S. A 107, 8023–8028. doi: 10.1073/pnas.0912030107
Guo, H., Mockler, T., Duong, H., Lin, C. (2001). SUB1, an Arabidopsis Ca2+-binding protein involved in cryptochrome and phytochrome coaction. Science 291, 487–490. doi: 10.1126/science.291.5503.487
Gutermuth, T., Lassig, R., Portes, M. T., Maierhofer, T., Romeis, T., Borst, J. W., et al. (2013). Pollen tube growth regulation by free anions depends on the interaction between the anion channel SLAH3 and calcium-dependent protein kinases CPK2 and CPK20. Plant Cell 25, 4525–4543. doi: 10.1105/tpc.113.118463
Hamada, T., Nagasaki-Takeuchi, N., Kato, T., Fujiwara, M., Sonobe, S., Fukao, Y., et al. (2013). Purification and characterization of novel microtubule-associated proteins from Arabidopsis cell suspension cultures. Plant Physiol. 163, 1804–1816. doi: 10.1104/pp.113.225607
He, Y., Wu, J., Lv, B., Li, J., Gao, Z., Xu, W., et al. (2015). Involvement of 14-3-3 protein GRF9 in root growth and response under polyethylene glycol-induced water stress. J. Exp. Bot. 66 (8), 2271–2281. doi: 10.1093/jxb/erv149
Heard, W., Sklenar, J., Tome, D. F., Robatzek, S., Jones, A. M. (2015). Identification of Regulatory and Cargo Proteins of Endosomal and Secretory Pathways in Arabidopsis thaliana by Proteomic Dissection. Mol. Cell Proteomics 14, 1796–1813. doi: 10.1074/mcp.M115.050286
Helm, S., Dobritzsch, D., Rodiger, A., Agne, B., Baginsky, S. (2014). Protein identification and quantification by data-independent acquisition and multi-parallel collision-induced dissociation mass spectrometry (MS(E)) in the chloroplast stroma proteome. J. Proteomics 98, 79–89. doi: 10.1016/j.jprot.2013.12.007
Higuchi, M., Ozaki, H., Matsui, M., Sonoike, K. (2009). A T-DNA insertion mutant of AtHMA1 gene encoding a Cu transporting ATPase in Arabidopsis thaliana has a defect in the water-water cycle of photosynthesis. J. Photochem. Photobiol. B. 94 (3), 205–213. doi: 10.1016/j.jphotobiol.2008.12.002
Hochmal, A. K., Schulze, S., Trompelt, K., Hippler, M. (2015). Calcium-dependent regulation of photosynthesis. Biochim. Biophys. Acta 1847, 993–1003. doi: 10.1016/j.bbabio.2015.02.010
Hocking, B., Conn, S. J., Manohar, M., Xu, B., Athman, A., Stancombe, M. A., et al. (2017). Heterodimerization of Arabidopsis calcium/proton exchangers contributes to regulation of guard cell dynamics and plant defense responses. J. Exp. Bot. 68, 4171–4183. doi: 10.1093/jxb/erx209
Hooper, C. M., Castleden, I. R., Tanz, S. K., Aryamanesh, N., Millar, A. H. (2017). SUBA4: the interactive data analysis centre for Arabidopsis subcellular protein locations. Nucleic Acids Res. 45, D1064–d1074. doi: 10.1093/nar/gkw1041
Huang, L., Berkelman, T., Franklin, A. E., Hoffman, N. E. (1993). Characterization of a gene encoding a Ca(2+)-ATPase-like protein in the plastid envelope. Proc. Natl. Acad. Sci. U. S. A 90 (21), 10066–10070. doi: 10.1073/pnas.90.21.10066
Huang, K., Peng, L., Liu, Y., Yao, R., Liu, Z., Li, X., et al. (2018). Arabidopsis calcium-dependent protein kinase AtCPK1 plays a positive role in salt/drought-stress response. Biochem. Biophys. Res. Commun. 498 (1), 92–98. doi: 10.1016/j.bbrc.2017.11.175
Inzè, A., Vanderauwera, S., Hoeberichts, F. A., Vandorpe, M., van Gaever, T., van Breusegem, F. (2012). A subcellular localization compendium of hydrogen peroxide-induced proteins. Plant Cell Environ. 35, 308–320. doi: 10.1111/j.1365-3040.2011.02323.x
Ito, J., Batth, T. S., Petzold, C. J., Redding-Johanson, A. M., Mukhopadhyay, A., Verboom, R., et al. (2011). Analysis of the Arabidopsis cytosolic proteome highlights subcellular partitioning of central plant metabolism. J. Proteome Res. 10 (4), 1571–1582. doi: 10.1021/pr1009433
Ji, R., Zhou, L., Liu, J., Wang, Y., Yang, L., Zheng, Q., et al. (2017). Calcium-dependent protein kinase CPK31 interacts with arsenic transporter AtNIP1;1 and regulates arsenite uptake in Arabidopsis thaliana. PloS One 12, e0173681. doi: 10.1371/journal.pone.0173681
Johnson, C. H., Knight, M. R., Kondo, T., Masson, P., Sedbrook, J., Haley, A., et al. (1995). Circadian oscillations of cytosolic and chloroplastic free calcium in plants. Science 269, 1863–1865. doi: 10.1126/science.7569925
Ju, C., Kong, D., Lee, Y., Ge, G., Song, Y., Liu, J., et al. (2020). Methionine Synthase 1 Provides Methionine for Activating AtGLR3.5 Ca2+ Channel and Regulating Germination in Arabidopsis. J. Exp. Bot. 71, 178–187. doi: 10.1093/jxb/erz431
Karniely, S., Pines, O. (2005). Single translation–dual destination: mechanisms of dual protein targeting in eukaryotes. EMBO Rep. 6, 420–425. doi: 10.1038/sj.embor.7400394
Keinath, N. F., Kierszniowska, S., Lorek, J., Bourdais, G., Kessler, S. A., Shimosato-Asano, H., et al. (2010). PAMP (pathogen-associated molecular pattern)-induced changes in plasma membrane compartmentalization reveal novel components of plant immunity. J. Biol. Chem. 285 (50), 39140–39149. doi: 10.1074/jbc.M110.160531
Kmiecik, P., Leonardelli, M., Teige, M. (2016). Novel connections in plant organellar signaling link different stress responses and signaling pathways. J. Exp. Bot. 67, 3793–3807. doi: 10.1093/jxb/erw136
Kong, D., Hu, H. C., Okuma, E., Lee, Y., Lee, H. S., Munemasa, S., et al. (2016). L-Met Activates Arabidopsis GLR Ca(2+) Channels Upstream of ROS Production and Regulates Stomatal Movement. Cell Rep. 17 (10), 2553–2561. doi: 10.1016/j.celrep.2016.11.015
Kudla, J., Becker, D., Grill, E., Hedrich, R., Hippler, M., Kummer, U., et al. (2018). Advances and current challenges in calcium signaling. New Phytol. 218, 414–431. doi: 10.1111/nph.14966
Latz, A., Mehlmer, N., Zapf, S., Mueller, T. D., Wurzinger, B., Pfister, B., et al. (2013). Salt stress triggers phosphorylation of the Arabidopsis vacuolar K+ channel TPK1 by calcium-dependent protein kinases (CDPKs). Mol. Plant 6, 1274–1289. doi: 10.1093/mp/sss158
Lee, T. A., Bailey-Serres, J. (2019). Integrative Analysis from the Epigenome to Translatome Uncovers Patterns of Dominant Nuclear Regulation during Transient Stress. Plant Cell 31, 2573–2595. doi: 10.1105/tpc.19.00463
Lee, D., Polisensky, D. H., Braam, J. (2005). Genome-wide identification of touch- and darkness-regulated Arabidopsis genes: a focus on calmodulin-like and XTH genes. New Phytol. 165, 429–444. doi: 10.1111/j.1469-8137.2004.01238.x
Lee, J., Lee, H., Kim, J., Lee, S., Kim, D. H., Kim, S., et al. (2011). Both the hydrophobicity and a positively charged region flanking the C-terminal region of the transmembrane domain of signal-anchored proteins play critical roles in determining their targeting specificity to the endoplasmic reticulum or endosymbiotic organelles in Arabidopsis cells. Plant Cell 23 (4), 1588–1607. doi: 10.1105/tpc.110.082230
Lee, Y., Min, C. K., Kim, T. G., Song, H. K., Lim, Y., Kim, D., et al. (2015). Structure and function of the N-terminal domain of the human mitochondrial calcium uniporter. EMBO Rep. 16, 1318–1333. doi: 10.15252/embr.201540436
Lenzoni, G., Knight, M. R. (2019). Increases in Absolute Temperature Stimulate Free Calcium Concentration Elevations in the Chloroplast. Plant Cell Physiol. 60, 538–548. doi: 10.1093/pcp/pcy227
Li, B., Takahashi, D., Kawamura, Y., Uemura, M. (2012). Comparison of plasma membrane proteomic changes of Arabidopsis suspension-cultured cells (T87 Line) after cold and ABA treatment in association with freezing tolerance development. Plant Cell Physiol. 53 (3), 543–554. doi: 10.1093/pcp/pcs010
Li, G., Boudsocq, M., Hem, S., Vialaret, J., Rossignol, M., Maurel, C., et al. (2015). The calcium-dependent protein kinase CPK7 acts on root hydraulic conductivity. Plant Cell Environ. 38 (7), 1312–1320. doi: 10.1111/pce.12478
Li, P., Zhang, G., Gonzales, N., Guo, Y., Hu, H., Park, S., et al. (2016). Ca(2+) -regulated and diurnal rhythm-regulated Na(+) /Ca(2+) exchanger AtNCL affects flowering time and auxin signaling in Arabidopsis. Plant Cell Environ. 39 (2), 377–392. doi: 10.1111/pce.12620
Li, Z., Takahashi, Y., Scavo, A., Brandt, B., Nguyen, D., Rieu, P., et al. (2018). Abscisic acid-induced degradation of Arabidopsis guanine nucleotide exchange factor requires calcium-dependent protein kinases. Proc. Natl. Acad. Sci. U. S. A 115, E4522–e4531. doi: 10.1073/pnas.1719659115
Liu, N., Hake, K., Wang, W., Zhao, T., Romeis, T., Tang, D. (2017). CALCIUM-DEPENDENT PROTEIN KINASE5 associates with the truncated nlr protein TIR-NBS2 to contribute to exo70B1-mediated immunity. Plant Cell 29, 746–759. doi: 10.1105/tpc.16.00822
Loro, G., Wagner, S., Doccula, F. G., Behera, S., Weinl, S., Kudla, J., et al. (2016). Chloroplast-specific in Vivo Ca2+ imaging using yellow cameleon fluorescent protein sensors reveals organelle-autonomous Ca2+ signatures in the Stroma. Plant Physiol. 171, 2317–2330. doi: 10.1104/pp.16.00652
Ma, X., Gai, W. X., Qiao, Y. M., Ali, M., Wei, A. M., Luo, D. X., et al. (2019). Identification of CBL and CIPK gene families and functional characterization of CaCIPK1 under Phytophthora capsici in pepper (Capsicum annuum L.). BMC Genomics 20, 775. doi: 10.1186/s12864-019-6125-z
Manohar, M., Shigaki, T., Mei, H., Park, S., Marshall, J., Aguilar, J., et al. (2011). Characterization of Arabidopsis Ca2+/H+ exchanger CAX3. Biochemistry 50, 6189–6195. doi: 10.1021/bi2003839
Manzoor, H., Chiltz, A., Madani, S., Vatsa, P., Schoefs, B., Pugin, A., et al. (2012). Calcium signatures and signaling in cytosol and organelles of tobacco cells induced by plant defense elicitors. Cell Calcium 51, 434–444. doi: 10.1016/j.ceca.2012.02.006
Marmagne, A., Ferro, M., Meinnel, T., Bruley, C., Kuhn, L., Garin, J., et al. (2007). A high content in lipid-modified peripheral roteins and integral receptor kinases features in the arabidopsis plasma membrane proteome. Mol. Cell Proteomics 6, 1980–1996. doi: 10.1074/mcp.M700099-MCP200
Martí Ruiz, M. C., Jung, H. J., Webb, A. A. R. (2020). Circadian gating of dark-induced increases in chloroplast- and cytosolic-free calcium in Arabidopsis. New Phytol. 225, 1993–2005 doi: 10.1111/nph.16280
Mathur, J., Mammone, A., Barton, K. A. (2012). Organelle extensions in plant cells. J. Integr. Plant Biol. 54, 851–867. doi: 10.1111/j.1744-7909.2012.01175.x
Matschi, S., Hake, K., Herde, M., Hause, B., Romeis, T. (2015). The calcium-dependent protein kinase CPK28 regulates development by inducing growth phase-specific, spatially restricted alterations in jasmonic acid levels independent of defense responses in Arabidopsis. Plant Cell 27 (3), 591–606. doi: 10.1105/tpc.15.00024
Mayfield, J. D., Paul, A. L., Ferl, R. J. (2012). The 14-3-3 proteins of Arabidopsis regulate root growth and chloroplast development as components of the photosensory system. J. Exp. Bot. 63 (8), 3061–3070. doi: 10.1093/jxb/ers022
Mehlmer, N., Parvin, N., Hurst, C. H., Knight, M. R., Teige, M., Vothknecht, U. C. (2012). A toolset of aequorin expression vectors for in planta studies of subcellular calcium concentrations in Arabidopsis thaliana. J. Exp. Bot. 63, 1751–1761. doi: 10.1093/jxb/err406
Mei, H., Cheng, N. H., Zhao, J., Park, S., Escareno, R. A., Pittman, J. K., et al. (2009). Root development under metal stress in Arabidopsis thaliana requires the H+/cation antiporter CAX4. New Phytol. 183, 95–105. doi: 10.1111/j.1469-8137.2009.02831.x
Melonek, J., Oetke, S., Krupinska, K. (2016). Multifunctionality of plastid nucleoids as revealed by proteome analyses. Biochim. Biophys. Acta 1864, 1016–1038. doi: 10.1016/j.bbapap.2016.03.009
Meyerhoff, O., Muller, K., Roelfsema, M. R., Latz, A., Lacombe, B., Hedrich, R., et al. (2005). AtGLR3.4, a glutamate receptor channel-like gene is sensitive to touch and cold. Planta 222, 418–427. doi: 10.1007/s00425-005-1551-3
Mitra, S. K., Walters, B. T., Clouse, S. D., Goshe, M. B. (2009). An efficient organic solvent based extraction method for the proteomic analysis of Arabidopsis plasma membranes. J. Proteome Res. 8, 2752–2767. doi: 10.1021/pr801044y
Monaghan, J., Matschi, S., Shorinola, O., Rovenich, H., Matei, A., Segonzac, C., et al. (2014). The calcium-dependent protein kinase CPK28 buffers plant immunity and regulates BIK1 turnover. Cell Host. Microbe 16, 605–615. doi: 10.1016/j.chom.2014.10.007
Monaghan, J., Matschi, S., Romeis, T., Zipfel, C. (2015). The calcium-dependent protein kinase CPK28 negatively regulates the BIK1-mediated PAMP-induced calcium burst. Plant Signal Behav. 10, e1018497. doi: 10.1080/15592324.2015.1018497
Mori, I. C., Murata, Y., Yang, Y., Munemasa, S., Wang, Y. F., Andreoli, S., et al. (2006). CDPKs CPK6 and CPK3 function in ABA regulation of guard cell S-type anion- and Ca(2+)-permeable channels and stomatal closure. PloS Biol. 4, e327. doi: 10.1371/journal.pbio.0040327
Morris, J., Tian, H., Park, S., Sreevidya, C. S., Ward, J. M., Hirschi, K. D. (2008). AtCCX3 is an Arabidopsis endomembrane H+ -dependent K+ transporter. Plant Physiol. 148, 1474–1486. doi: 10.1104/pp.108.118810
Myers, C., Romanowsky, S. M., Barron, Y. D., Garg, S., Azuse, C. L., Curran, A., et al. (2009). Calcium-dependent protein kinases regulate polarized tip growth in pollen tubes. Plant J. 59, 528–539. doi: 10.1111/j.1365-313X.2009.03894.x
Nelson, C. J., Hegeman, A. D., Harms, A. C., Sussman, M. R. (2006). A quantitative analysis of Arabidopsis plasma membrane using trypsin-catalyzed (18)O labeling. Mol. Cell Proteomics 5 (8), 1382–1395. doi: 10.1074/mcp.M500414-MCP200
Nguyen, C. T., Kurenda, A., Stolz, S., Chetelat, A., Farmer, E. E. (2018a). Identification of cell populations necessary for leaf-to-leaf electrical signaling in a wounded plant. Proc. Natl. Acad. Sci. U. S. A 115, 10178–10183. doi: 10.1073/pnas.1807049115
Nguyen, N. X., Armache, J. P., Lee, C., Yang, Y., Zeng, W., Mootha, V. K., et al. (2018b). Cryo-EM structure of a fungal mitochondrial calcium uniporter. Nature 559, 570–574. doi: 10.1038/s41586-018-0333-6
Nguyen-Kim, H., San Clemente, H., Balliau, T., Zivy, M., Dunand, C., Albenne, C., et al. (2016). Arabidopsis thaliana root cell wall proteomics: Increasing the proteome coverage using a combinatorial peptide ligand library and description of unexpected Hyp in peroxidase amino acid sequences. Proteomics 16, 491–503. doi: 10.1002/pmic.201500129
Nikolovski, N., Rubtsov, D., Segura, M. P., Miles, G. P., Stevens, T. J., Dunkley, T. P., et al. (2012). Putative glycosyltransferases and other plant Golgi apparatus proteins are revealed by LOPIT proteomics. Plant Physiol. 160, 1037–1051. doi: 10.1104/pp.112.204263
Nomura, H., Shiina, T. (2014). Calcium signaling in plant endosymbiotic organelles: mechanism and role in physiology. Mol. Plant 7, 1094–1104. doi: 10.1093/mp/ssu020
Nomura, H., Komori, T., Uemura, S., Kanda, Y., Shimotani, K., Nakai, K., et al. (2012). Chloroplast-mediated activation of plant immune signaling in Arabidopsis. Nat. Commun. 3, 926. doi: 10.1038/ncomms1926
Nühse, T. S., Stensballe, A., Jensen, O. N., Peck, S. C. (2003). Large-scale analysis of in vivo phosphorylated membrane proteins by immobilized metal ion affinity chromatography and mass spectrometry. Mol. Cell Proteomics 2 (11), 1234–1243. doi: 10.1074/mcp.T300006-MCP200
Nühse, T. S., Stensballe, A., Jensen, O. N., Peck, S. C. (2004). Phosphoproteomics of the Arabidopsis plasma membrane and a new phosphorylation site database. Plant Cell 16 (9), 2394–2405. doi: 10.1105/tpc.104.023150
Omidbakhshfard, M. A., Fujikura, U., Olas, J. J., Xue, G. P., Balazadeh, S., Mueller-Roeber, B. (2018). GROWTH-REGULATING FACTOR 9 negatively regulates arabidopsis leaf growth by controlling ORG3 and restricting cell proliferation in leaf primordia. PloS Genet. 14, e1007484. doi: 10.1371/journal.pgen.1007484
Oxenoid, K., Dong, Y., Cao, C., Cui, T., Sancak, Y., Markhard, A. L., et al. (2016). Architecture of the mitochondrial calcium uniporter. Nature 533, 269–273. doi: 10.1038/nature17656
Parsons, H. T., Christiansen, K., Knierim, B., Carroll, A., Ito, J., Batth, T. S., et al. (2012). Isolation and proteomic characterization of the Arabidopsis Golgi defines functional and novel components involved in plant cell wall biosynthesis. Plant Physiol. 159, 12–26. doi: 10.1104/pp.111.193151
Pittman, J. K., Hirschi, K. D. (2016). CAX-ing a wide net: Cation/H(+) transporters in metal remediation and abiotic stress signaling. Plant Biol. (Stuttg) 18, 741–749. doi: 10.1111/plb.12460
Pornsiriwong, W., Estavillo, G. M., Chan, K. X., Tee, E. E., Ganguly, D., Crisp, P. A., et al. (2017). A chloroplast retrograde signal, 3'-phosphoadenosine 5'-phosphate, acts as a secondary messenger in abscisic acid signaling in stomatal closure and germination. Elife 6, e23361. doi: 10.7554/eLife.23361
Pottosin, I., Shabala, S. (2015). Transport across chloroplast membranes: optimizing photosynthesis for adverse environmental conditions. Mol. Plant 9, 356–370. doi: 10.1016/j.molp.2015.10.006
Ranty, B., Aldon, D., Cotelle, V., Galaud, J. P., Thuleau, P., Mazars, C. (2016). Calcium sensors as key hubs in plant responses to biotic and abiotic stresses. Front. Plant Sci. 7, 327. doi: 10.3389/fpls.2016.00327
Reumann, S., Quan, S., Aung, K., Yang, P., Manandhar-Shrestha, K., Holbrook, D., et al. (2009). In-depth proteome analysis of Arabidopsis leaf peroxisomes combined with in vivo subcellular targeting verification indicates novel metabolic and regulatory functions of peroxisomes. Plant Physiol. 150, 125–143. doi: 10.1104/pp.109.137703
Rocha, A. G., Vothknecht, U. C. (2012). The role of calcium in chloroplasts–an intriguing and unresolved puzzle. Protoplasma 249, 957–966. doi: 10.1007/s00709-011-0373-3
Rodriguez Milla, M. A., Uno, Y., Chang, I. F., Townsend, J., Maher, E. A., Quilici, D., et al. (2006). A novel yeast two-hybrid approach to identify CDPK substrates: characterization of the interaction between AtCPK11 and AtDi19, a nuclear zinc finger protein. FEBS Lett. 580 (3), 904–911. doi: 10.1016/j.febslet.2006.01.013
Ruge, H., Flosdorff, S., Ebersberger, I., Chigri, F., Vothknecht, U. C. (2016). The calmodulin-like proteins AtCML4 and AtCML5 are single-pass membrane proteins targeted to the endomembrane system by an N-terminal signal anchor sequence. J. Exp. Bot. 67, 3985–3996. doi: 10.1093/jxb/erw101
Sai, J., Johnson, C. H. (2002). Dark-stimulated calcium ion fluxes in the chloroplast stroma and cytosol. Plant Cell 14, 1279–1291. doi: 10.1105/tpc.000653
Sanyal, S. K., Pandey, A., Pandey, G. K. (2015). The CBL-CIPK signaling module in plants: a mechanistic perspective. Physiol. Plant 155, 89–108. doi: 10.1111/ppl.12344
Schiott, M., Romanowsky, S. M., Baekgaard, L., Jakobsen, M. K., Palmgren, M. G., Harper, J. F. (2004). A plant plasma membrane Ca2+ pump is required for normal pollen tube growth and fertilization. Proc. Natl. Acad. Sci. U. S. A 101 (25), 9502–9507. doi: 10.1073/pnas.0401542101
Schneider, A., Steinberger, I., Herdean, A., Gandini, C., Eisenhut, M., Kurz, S., et al. (2016). The evolutionarily conserved protein PHOTOSYNTHESIS AFFECTED MUTANT71 is required for efficient manganese uptake at the Thylakoid Membrane in Arabidopsis. Plant Cell 28, 892–910. doi: 10.1105/tpc.15.00812
Scholz, S. S., Vadassery, J., Heyer, M., Reichelt, M., Bender, K. W., Snedden, W. A., et al. (2014). Mutation of the Arabidopsis calmodulin-like protein CML37 deregulates the jasmonate pathway and enhances susceptibility to herbivory. Mol. Plant 7, 1712–1726. doi: 10.1093/mp/ssu102
Scholz, S. S., Reichelt, M., Vadassery, J., Mithofer, A. (2015). Calmodulin-like protein CML37 is a positive regulator of ABA during drought stress in Arabidopsis. Plant Signal Behav. 10 (6), e1011951. doi: 10.1080/15592324.2015.1011951
Schwacke, R., Schneider, A., van der Graaff, E., Fischer, K., Catoni, E., Desimone, M., et al. (2003). ARAMEMNON, a novel database for Arabidopsis integral membrane proteins. Plant Physiol. 131, 16–26. doi: 10.1104/pp.011577
Seigneurin-Berny, D., Gravot, A., Auroy, P., Mazard, C., Kraut, A., Finazzi, G., et al. (2006). HMA1, a new Cu-ATPase of the chloroplast envelope, is essential for growth under adverse light conditions. J. Biol. Chem. 281, 2882–2892. doi: 10.1074/jbc.M508333200
Selles, B., Michaud, C., Xiong, T. C., Leblanc, O., Ingouff, M. (2018). Arabidopsis pollen tube germination and growth depend on the mitochondrial calcium uniporter complex. New Phytol. 219, 58–65. doi: 10.1111/nph.15189
Sello, S., Perotto, J., Carraretto, L., Szabo, I., Vothknecht, U. C., Navazio, L. (2016). Dissecting stimulus-specific Ca2+ signals in amyloplasts and chloroplasts of Arabidopsis thaliana cell suspension cultures. J. Exp. Bot. 67, 3965–3974. doi: 10.1093/jxb/erw038
Sello, S., Moscatiello, R., Mehlmer, N., Leonardelli, M., Carraretto, L., Cortese, E., et al. (2018). Chloroplast Ca(2+) Fluxes into and across Thylakoids revealed by Thylakoid-Targeted Aequorin Probes. Plant Physiol. 177, 38–51. doi: 10.1104/pp.18.00027
Senkler, J., Senkler, M., Eubel, H., Hildebrandt, T., Lengwenus, C., Schertl, P., et al. (2017). The mitochondrial complexome of Arabidopsis thaliana. Plant J. 89, 1079–1092. doi: 10.1111/tpj.13448
Stael, S., Bayer, R. G., Mehlmer, N., Teige, M. (2011). Protein N-acylation overrides differing targeting signals. FEBS Lett. 585, 517–522. doi: 10.1016/j.febslet.2011.01.001
Stael, S., Rocha, A. G., Wimberger, T., Anrather, D., Vothknecht, U. C., Teige, M. (2012a). Cross-talk between calcium signaling and protein phosphorylation at the thylakoid. J. Exp. Bot. 63, 1725–1733. doi: 10.1093/jxb/err403
Stael, S., Wurzinger, B., Mair, A., Mehlmer, N., Vothknecht, U. C., Teige, M. (2012b). Plant organellar calcium signaling: an emerging field. J. Exp. Bot. 63, 1525–1542. doi: 10.1093/jxb/err394
Stael, S., Kmiecik, P., Willems, P., van der Kelen, K., Coll, N. S., Teige, M., et al. (2015). Plant innate immunity–sunny side up? Trends Plant Sci. 20, 3–11. doi: 10.1016/j.tplants.2014.10.002
Stael, S. (2019). Chloroplast calcium signaling quenches a thirst. Nat. Plants 5, 559–560. doi: 10.1038/s41477-019-0435-7
Stephens, N. R., Qi, Z., Spalding, E. P. (2008). Glutamate receptor subtypes evidenced by differences in desensitization and dependence on the GLR3.3 and GLR3.4 genes. Plant Physiol. 146, 529–538. doi: 10.1104/pp.107.108134
Szymanski, W. G., Zauber, H., Erban, A., Gorka, M., Wu, X. N., Schulze, W. X. (2015). Cytoskeletal components define protein location to membrane microdomains. Mol. Cell Proteomics 14, 2493–2509. doi: 10.1074/mcp.M114.046904
Teardo, E., Segalla, A., Formentin, E., Zanetti, M., Marin, O., Giacometti, G. M., et al. (2010). Characterization of a plant glutamate receptor activity. Cell Physiol. Biochem. 26, 253–262. doi: 10.1159/000320525
Teardo, E., Formentin, E., Segalla, A., Giacometti, G. M., Marin, O., Zanetti, M., et al. (2011). Dual localization of plant glutamate receptor AtGLR3.4 to plastids and plasmamembrane. Biochim. Biophys. Acta 1807, 359–367. doi: 10.1016/j.bbabio.2010.11.008
Teardo, E., Carraretto, L., de Bortoli, S., Costa, A., Behera, S., Wagner, R., et al. (2015). Alternative splicing-mediated targeting of the Arabidopsis GLUTAMATE RECEPTOR3.5 to mitochondria affects organelle morphology. Plant Physiol. 167, 216–227. doi: 10.1104/pp.114.242602
Teardo, E., Carraretto, L., Wagner, S., Formentin, E., Behera, S., de Bortoli, S., et al. (2017). Physiological characterization of a plant mitochondrial Calcium Uniporter in Vitro and in Vivo. Plant Physiol. 173, 1355–1370. doi: 10.1104/pp.16.01359
Teardo, E., Carraretto, L., Moscatiello, R., Cortese, E., Vicario, M., Festa, M., et al. (2019). A chloroplast-localized mitochondrial calcium uniporter transduces osmotic stress in Arabidopsis. Nat. Plants 5, 581–588. doi: 10.1038/s41477-019-0434-8
Tomizioli, M., Lazar, C., Brugiere, S., Burger, T., Salvi, D., Gatto, L., et al. (2014). Deciphering thylakoid sub-compartments using a mass spectrometry-based approach. Mol. Cell Proteomics 13, 2147–2167. doi: 10.1074/mcp.M114.040923
Tsai, M. F., Phillips, C. B., Ranaghan, M., Tsai, C. W., Wu, Y., Willliams, C., et al. (2016). Dual functions of a small regulatory subunit in the mitochondrial calcium uniporter complex. Elife 5, e15545. doi: 10.7554/eLife.15545
Uno, Y., Rodriguez Milla, M. A., Maher, E., Cushman, J. C. (2009). Identification of proteins that interact with catalytically active calcium-dependent protein kinases from Arabidopsis. Mol. Genet. Genomics 281 (4), 375–390. doi: 10.1007/s00438-008-0419-1
Urao, T., Katagiri, T., Mizoguchi, T., Yamaguchi-Shinozaki, K., Hayashida, N., Shinozaki, K. (1994). An Arabidopsis thaliana cDNA encoding Ca(2+)-dependent protein kinase. Plant Physiol. 105, 1461–1462. doi: 10.1104/pp.105.4.1461
Vainonen, J. P., Sakuragi, Y., Stael, S., Tikkanen, M., Allahverdiyeva, Y., Paakkarinen, V., et al. (2008). Light regulation of CaS, a novel phosphoprotein in the thylakoid membrane of Arabidopsis thaliana. FEBS J. 275, 1767–1777. doi: 10.1111/j.1742-4658.2008.06335.x
van Kleeff, P. J. M., Gao, J., Mol, S., Zwart, N., Zhang, H., Li, K. W., et al. (2018). The Arabidopsis GORK K(+)-channel is phosphorylated by calcium-dependent protein kinase 21 (CPK21), which in turn is activated by 14-3-3 proteins. Plant Physiol. Biochem. 125, 219–231. doi: 10.1016/j.plaphy.2018.02.013
Vanderbeld, B., Snedden, W. A. (2007). Developmental and stimulus-induced expression patterns of Arabidopsis calmodulin-like genes CML37, CML38 and CML39. Plant Mol. Biol. 64, 683–697. doi: 10.1007/s11103-007-9189-0
Vincill, E. D., Clarin, A. E., Molenda, J. N., Spalding, E. P. (2013). Interacting glutamate receptor-like proteins in Phloem regulate lateral root initiation in Arabidopsis. Plant Cell 25, 1304–1313. doi: 10.1105/tpc.113.110668
Wagner, S., Behera, S., de Bortoli, S., Logan, D. C., Fuchs, P., Carraretto, L., et al. (2015). The EF-Hand Ca2+ binding protein MICU choreographs mitochondrial Ca2+ dynamics in Arabidopsis. Plant Cell 27, 3190–3212. doi: 10.1105/tpc.15.00509
Wagner, S., de Bortoli, S., Schwarzlander, M., Szabo, I. (2016). Regulation of mitochondrial calcium in plants versus animals. J. Exp. Bot. 67, 3809–3829. doi: 10.1093/jxb/erw100
Wang, P., Li, Z., Wei, J., Zhao, Z., Sun, D., Cui, S. (2012). A Na+/Ca2+ exchanger-like protein (AtNCL) involved in salt stress in Arabidopsis. J. Biol. Chem. 287 (53), 44062–44070. doi: 10.1074/jbc.M112.351643
Wang, W. H., Chen, J., Liu, T. W., Chen, J., Han, A. D., Simon, M., et al. (2014). Regulation of the calcium-sensing receptor in both stomatal movement and photosynthetic electron transport is crucial for water use efficiency and drought tolerance in Arabidopsis. J. Exp. Bot. 65 (1), 223–234. doi: 10.1093/jxb/ert362
Wang, C., Xu, W., Jin, H., Zhang, T., Lai, J., Zhou, X., et al. (2016). A putative chloroplast-localized Ca(2+)/H(+) Antiporter CCHA1 is involved in calcium and pH Homeostasis and required for PSII function in Arabidopsis. Mol. Plant 9 (8), 1183–1196. doi: 10.1016/j.molp.2016.05.015
Wang, Y., Nguyen, N. X., She, J., Zeng, W., Yang, Y., Bai, X. C., et al. (2019). Structural mechanism of EMRE-dependent gating of the human mitochondrial Calcium Uniporter. Cell 177, 1252–1261.e13. doi: 10.1016/j.cell.2019.03.050
Weinl, S., Held, K., Schlücking, K., Steinhorst, L., Kuhlgert, S., Hippler, M., et al. (2008). A plastid protein crucial for Ca2+-regulated stomatal responses. New Phytol. 179, 675–686. doi: 10.1111/j.1469-8137.2008.02492.x
Whiteman, S. A., Serazetdinova, L., Jones, A. M., Sanders, D., Rathjen, J., Peck, S. C., et al. (2008). Identification of novel proteins and phosphorylation sites in a tonoplast enriched membrane fraction of Arabidopsis thaliana. Proteomics 8 (17), 3536–3547. doi: 10.1002/pmic.200701104
Yamamoto, T., Ozono, M., Watanabe, A., Maeda, K., Nara, A., Hashida, M., et al. (2019). Functional analysis of coiled-coil domains of MCU in mitochondrial calcium uptake. Biochim. Biophys. Acta Bioenerg. 1860, 148061. doi: 10.1016/j.bbabio.2019.148061
Yang, Y., Zhang, C., Tang, R. J., Xu, H. X., Lan, W. Z., Zhao, F., et al. (2019). Calcineurin B-Like proteins CBL4 and CBL10 mediate two independent salt tolerance pathways in Arabidopsis. Int. J. Mol. Sci. 20, pii: E2421. doi: 10.3390/ijms20102421
Yoo, J., Wu, M., Yin, Y., Herzik, M. A., Jr., Lander, G. C., Lee, S. Y. (2018). Cryo-EM structure of a mitochondrial calcium uniporter. Science 361, 506–511. doi: 10.1126/science.aar4056
Yoshida, K., Ohnishi, M., Fukao, Y., Okazaki, Y., Fujiwara, M., Song, C., et al. (2013). Studies on vacuolar membrane microdomains isolated from Arabidopsis suspension-cultured cells: local distribution of vacuolar membrane proteins. Plant Cell Physiol. 54, 1571–1584. doi: 10.1093/pcp/pct107
Yuan, F., Yang, H., Xue, Y., Kong, D., Ye, R., Li, C., et al. (2014). OSCA1 mediates osmotic-stress-evoked Ca2+ increases vital for osmosensing in Arabidopsis. Nature 514, 367–371. doi: 10.1038/nature13593
Zargar, S. M., Kurata, R., Inaba, S., Oikawa, A., Fukui, R., Ogata, Y., et al. (2015). Quantitative proteomics of Arabidopsis shoot microsomal proteins reveals a cross-talk between excess zinc and iron deficiency. Proteomics 15, 1196–1201. doi: 10.1002/pmic.201400467
Zhang, Z. J., Peck, S. C. (2011). Simplified enrichment of plasma membrane proteins for proteomic analyses in Arabidopsis thaliana. Proteomics 11, 1780–1788. doi: 10.1002/pmic.201000648
Zhang, B., Zhang, C., Liu, C., Jing, Y., Wang, Y., Jin, L., et al. (2018). Inner envelope CHLOROPLAST MANGANESE TRANSPORTER 1 supports manganese homeostasis and phototrophic growth in Arabidopsis. Mol. Plant 11, 943–954. doi: 10.1016/j.molp.2018.04.007
Zhao, L. N., Shen, L. K., Zhang, W. Z., Zhang, W., Wang, Y., Wu, W. H. (2013). Ca2+-dependent protein kinase11 and 24 modulate the activity of the inward rectifying K+ channels in Arabidopsis pollen tubes. Plant Cell 25 (2), 649–661. doi: 10.1105/tpc.112.103184
Zhu, S. Y., Yu, X. C., Wang, X. J., Zhao, R., Li, Y., Fan, R. C., et al. (2007). Two calcium-dependent protein kinases, CPK4 and CPK11, regulate abscisic acid signal transduction in Arabidopsis. Plant Cell 19, 3019–3036. doi: 10.1105/tpc.107.050666
Zou, J. J., Wei, F. J., Wang, C., Wu, J. J., Ratnasekera, D., Liu, W. X., et al. (2010). Arabidopsis calcium-dependent protein kinase CPK10 functions in abscisic acid- and Ca2+-mediated stomatal regulation in response to drought stress. Plant Physiol. 154, 1232–1243. doi: 10.1104/pp.110.157545
Zou, J. J., Li, X. D., Ratnasekera, D., Wang, C., Liu, W. X., Song, L. F., et al. (2015). Arabidopsis CALCIUM-DEPENDENT PROTEIN KINASE8 and CATALASE3 function in abscisic acid-mediated signaling and H2O2 homeostasis in stomatal guard cells under drought stress. Plant Cell 27 (5), 1445–1460. doi: 10.1105/tpc.15.00144
Keywords: chloroplasts, organellar calcium signaling, calcium-permeable channels, calcium transporters, calcium binding proteins, genetically encoded calcium indicators, chloroplast calcium uniporter
Citation: Navazio L, Formentin E, Cendron L and Szabò I (2020) Chloroplast Calcium Signaling in the Spotlight. Front. Plant Sci. 11:186. doi: 10.3389/fpls.2020.00186
Received: 09 December 2019; Accepted: 07 February 2020;
Published: 11 March 2020.
Edited by:
Jürgen Soll, Ludwig Maximilian University of Munich, GermanyReviewed by:
Markus Teige, University of Vienna, AustriaCornelia Spetea, University of Gothenburg, Sweden
Copyright © 2020 Navazio, Formentin, Cendron and Szabò. This is an open-access article distributed under the terms of the Creative Commons Attribution License (CC BY). The use, distribution or reproduction in other forums is permitted, provided the original author(s) and the copyright owner(s) are credited and that the original publication in this journal is cited, in accordance with accepted academic practice. No use, distribution or reproduction is permitted which does not comply with these terms.
*Correspondence: Ildikò Szabò, ildiko.szabo@unipd.it
†These authors have contributed equally to this work