- 1Maize Research Institute, Sichuan Agricultural University, Chengdu, China
- 2Department of Molecular, Cellular, and Developmental Biology, University of Michigan, Ann Arbor, MI, United States
The patterning of root-hair and non-hair epidermal cells in the Arabidopsis root is governed by a network of transcriptional regulators. The central MYB-bHLH-WD40 (MBW) transcriptional complex includes the WD40-repeat protein TRANSPARENT TESTA GLABRA1 (TTG1). To clarify the role of TTG1, we describe the identification and analysis of two new ttg1 mutants. Each of these mutants contains a single nucleotide change in the TTG1 gene, which causes a single amino-acid substitution in the predicted TTG1 protein and alters root-hair pattern formation. Surprisingly, these new ttg1 mutants exhibit decreased root-hair formation, particularly in the caprice (cpc) mutant background, rather than increased root-hair formation as reported for strong ttg1 mutants. We show that the unique phenotype of these mutants is due to differential effects of the altered TTG1 proteins on target gene expression, associated with a weakened ability to interact with its GLABRA3 bHLH partner. These findings demonstrate the crucial role of TTG1 for the appropriate balance of target gene activation to achieve the proper pattern of epidermal cell types during Arabidopsis root development.
Introduction
The development of the Arabidopsis root epidermis has been widely used as a simple model for understanding cell-type specification and pattern formation in plants (Duckett et al., 1994; Schiefelbein et al., 2009). There are two distinct cell types produced in the root epidermis, root-hair cells and non-hair cells, and these are arranged in a position-dependent pattern (Grebe, 2012). Developing epidermal cells located between two underlying cortical cells (the “H” cell position) are specified as root-hair cells, and epidermal cells located over a single cortical cell (the “N” cell position) are specified as non-hair cells (Cormack, 1962; Dolan and Roberts, 1995; Berger et al., 1998). Over the past 20 years, a large collection of studies have uncovered a highly orchestrated network of transcriptional regulators responsible for establishing position-dependent gene expression leading to the two cell fates (Bruex et al., 2012; Schiefelbein et al., 2014). The basic component of this network is a central complex containing a WD40-repeat protein encoded by TRANSPARENT TESTA GLABRA 1 (TTG1) (Galway et al., 1994; Walker et al., 1999), bHLH proteins encoded by the functionally redundant GLABRA3 and ENHANCER OF GLABRA3 (GL3/EGL3) (Payne et al., 2000; Bernhardt et al., 2003, 2005), and an R2R3-type MYB protein encoded by WEREWOLF (WER) (Lee and Schiefelbein, 1999). This MYB-bHLH-WD40 (MBW) complex preferentially accumulates in non-hair cells where it directly promotes transcription of GLABRA 2 (GL2) (Di Cristina et al., 1996; Masucci et al., 1996). GL2 encodes an HD-ZIP transcription factor that activates transcription of non-hair cell differentiation genes and inhibits the expression of root-hair promoting genes such as ROOT HAIR DEFECTIVE 6 (RHD6) (Masucci and Schiefelbein, 1994; Yi et al., 2010). As a result, null mutations of WER, GL3/EGL3, and TTG1, or GL2 cause a lack of non-hair cells and exhibit a hairy root phenotype.
The MBW complex also mediates lateral inhibition by promoting transcription of the single-repeat R3-type MYB genes CAPRICE (CPC), TRIPTYCHON (TRY), and ENHANCER OF TRY AND CPC 1 (ETC1) preferentially in the N-position cells (Wada et al., 1997; Schellmann et al., 2002; Kirik et al., 2004; Simon et al., 2007). The resulting proteins then appear to move through plasmodesmata to accumulate in the adjacent H-position cells where they compete with WER for binding to GL3/EGL3 bHLHs and generate a non-functional complex (Lee and Schiefelbein, 2002; Kurata et al., 2005; Song et al., 2011). As a result, the H-position cells express GL2 at a relatively low level and RHD6 at a relatively high level, which specifies the root-hair cell fate. The CPC, TRY, and ETC1 proteins are functionally redundant, although CPC is most abundant and plays the major role in root-hair patterning (Simon et al., 2007). Null mutations of CPC, TRY, and/or ETC1 produce less root-hair cells and more non-hair cells. In addition to the movement of CPC/TRY/ETC1 from N cells to H cells, the GL3/EGL3 transcripts are found to preferentially accumulate in the H cells rather than the N cells, due to the negative transcriptional regulation of these genes by the MBW complex (Bernhardt et al., 2005; Kang et al., 2013). The GL3/EGL3 proteins are translocated from the H cells to the N cells. The opposite movement of CPC/TRY/ETC1 and GL3/EGL3 forms an intercellular mutual reinforcing loop and provides a robust root-hair patterning system (Schiefelbein et al., 2014). The patterning system is initiated by position cue(s) acting through the SCRAMBLED (SCM) LRR receptor-like kinase to influence the relative abundance of the MBW complex in the H and N positions (Kwak et al., 2005; Kwak and Schiefelbein, 2007, 2008).
In addition to root-hair patterning, the TTG1 gene is involved in several other developmental and biochemical pathways in Arabidopsis including trichome patterning, anthocyanin accumulation, seed coat pigmentation, and seed mucilage production (Walker et al., 1999; Miller et al., 2016). For each pathway, TTG1 appears to participate via a MBW complex, although different MYB and bHLH components are used for the five pathways (Zhang and Schrader, 2017). In trichome patterning, GLABROUS1 (GL1) is functionally equivalent to WER and forms a GL1-GL3/EGL3-TTG1 complex to promote trichome differentiation (Larkin et al., 1999; Kirik et al., 2005). Interestingly, the TTG1 exhibits an opposite effect on trichome formation when compared to its effect on root-hair formation. The ttg1 mutants produce no trichomes (a glabrous phenotype), but it produces excess root hairs (a hairy root phenotype) (Galway et al., 1994; Larkin et al., 1999). The proanthocyanidin biosynthetic pathway is blocked in ttg1 mutants causing a transparent testa phenotype in the seed coat (Haughn and Chaudhury, 2005; Gonzalez et al., 2009). In wild type seedlings, purple anthocyanin pigments are present in the hypocotyl, while ttg1 mutants completely lack the accumulation (Baudry et al., 2004; Gonzalez et al., 2008). The TTG1 gene encodes a protein of 341 amino acid residues with four WD40 repeats (Walker et al., 1999), and many ttg1 alleles have been identified, such as ttg1-1 (Q317stop) (Koornneef, 1981), ttg1-9 (S282F) (Larkin et al., 1994b), ttg1-10 (G→A in 5’UTR) (Larkin et al., 1994a), and ttg1-13 (deletion) (Larkin et al., 1999). These mutant lines exhibit pleiotropic phenotypes, including excessive (ectopic) root hairs, glabrous leaves, and yellow seed coat, although the severity of the mutant alleles varies (Bouyer et al., 2008; Zhang and Schrader, 2017).
To further investigate the cell fate determination mechanism in the Arabidopsis root epidermis, we sought to identify new genes or alleles that control the cell-type specification process. In this study, two novel mutant alleles of TTG1, designated ttg1-23 and ttg1-24, were identified from an enhancer screen in the cpc-1 mutant background. Both of these mutant ttg1 alleles were found to contain a single nucleotide change causing a single-residue substitution in the TTG1 protein and generating a novel root epidermis phenotype. The detailed analysis of these mutants provide new insights into the function of TTG1 and its role in root-hair cell patterning.
Materials and Methods
Plant Materials and Growth Conditions
The following mutant and transgenic lines have been previously described: cpc-1 (Wada et al., 1997), ttg1-1 (Koornneef, 1981), ttg1-13 (Larkin et al., 1999), ttg1-9 (Galway et al., 1994), and GL2:GUS (Masucci et al., 1996). Seeds were surface sterilized with 30% bleach and 0.02% Triton-X100 and sown on mineral nutrient mix media solidified with 0.3% Gelrite as described (Schiefelbein and Somerville, 1990). Four-day-old seedlings incubated vertically at 23°C under continuous light were used for all experiments. For plant propagation, 10-day-old seedlings were transplanted to soil and grown in growth chambers under long-day light cycle at 23°C (16 h day) and 18°C (8 h night).
Genetic Screening, Positional Mapping, and Whole Genome Sequencing
Seed mutagenesis of the cpc-1 GL2:GUS line (Wassilewskija [Ws] ecotype) with ethyl methanesulfonate (EMS) was performed as previously described (Estelle and Somerville, 1987). The cpc-1 ttg1-23 and cpc-1 ttg1-24 mutants were identified from the M2 population by visually screening for reduced root hair density using a dissection microscope. The F2 and F3 offspring from a cross between cpc-1 ttg1-23 and a Columbia wild-type plant were analyzed using multiple simple sequence length polymorphism (SSLP) markers (Bell and Ecker, 1994), and strong linkage was identified with marker NGA139 (position 8.4 Mb on chromosome 5). At the same time, a pool of 122 individuals of F3 offspring together with cpc-1 and Columbia were collected for whole genome sequencing. The genomic DNA was prepared with DNeasy Plant Maxi Kit (Qiagen) and samples were sequenced on an Illumina HiSeq 4000 platform in a 150-bp paired end run. The alignment of reads and variants calling were performed with MiModD 0.1.9.
Genotyping of ttg1-23 and ttg1-24 was performed with the Derived Cleaved Amplified Polymorphic Sequencing (dCAPS) (Neff et al., 2002) technique, using primers listed in Supplementary Table S1.
Transgene Construction and Plant Transformation
The TTG1 genomic DNA including 3-kb 5’ promotor sequence, 1-kb gene sequence and 1-kb 3’ terminal sequence was cloned using Phusion (NEB) and integrated into the pCB302 binary vector (digested with XbaI and BamHI) (Xiang et al., 1999) using the HiFi assembly system (NEB). Cloning primers are listed in Supplementary Table S1. The verified TTG1:TTG1 construct was then transformed into ttg1-23, ttg1-24, cpc-1 ttg1-23, and cpc-1 ttg1-24 mutant plants through floral dipping as previously described (Clough and Bent, 1998).
To construct the TTG1:TTG1-EYFP transgene, the TTG1 genomic segment including 3-kb 5’ promotor sequence, 1-kb stop codon deleted coding sequence, 1-kb 3’ terminal sequence and EYFP fragment were combined using Phusion (NEB) and integrated into the pCB302 binary vector (digested with XbaI and BamHI) using the HiFi assembly system (NEB). The TTG1:TTG1(23)-EYFP and TTG1:TTG1(24)-EYFP transgenic constructs were created in the same manner, but the wild-type coding sequence was replaced with the ttg1-23 and ttg1-24 mutants, respectively. Cloning primers are listed in Supplementary Table S1. Sequence-verified TTG1:TTG1-EYFP, TTG1:TTG1(23)-EYFP, and TTG1:TTG1(24)-EYFP constructs were then transformed into ttg1-13 mutant plants through the floral dipping method. After plant transformation, T0 plants were grown and T1 seeds were harvested and subjected to glufosinate-ammonium (PESTANAL®, Sigma-Aldrich) selection. The resistant T1 seedlings were grown and selfed to obtain T2 seeds, and single insertion lines were identified by analyzing the segregation of individual T2 populations for their chemical resistance and root-hair pattern. From each transformation experiment, homozygous T3 populations from at least three independent single-insertion lines were identified and used for further experiments.
Microscopy and Image Analysis
The quantification of root epidermal cell types was performed using a bright field compound microscope, following brief staining with toluidine blue as described (Wang et al., 2019). Cell positions were determined by inspecting the underlying cortical cells, and hair cells were defined by the presence of a visible protrusion regardless of its length. For each genotype, three independent biological replicates were performed. For each replicate, a total of 10 seedlings were analyzed and 10 cells were scored in both the H and N positions in each seedling (total of 100 cells).
Histochemical analysis of GUS fusion reporter lines was performed essentially as previously described (Masucci et al., 1996; Wang et al., 2019). Specifically, 10 μl/mL of X-Gluc (Gold Biotechnology) substrate was used for GL2:GUS seedling incubation (20 min at 37°C). To generate histograms representing the GL2:GUS signal distribution, wild-type, ttg1-23 and ttg1-24 roots were stained and photographed under the same conditions. For each root, 10 continuous cells in one file were analyzed from the oldest cell prior to rapid elongation (i.e., the cell’s length exceeds its width) and continuing toward the root tip. For all cells, the GUS signal was measured using the same region of interest frame and the mean values were plotted onto histograms. For each genotype, three independent biological replicates were performed.
Fluorescence imaging was performed using a TCS SP5 DM6000B broadband confocal microscope (Leica) with 20× dry lens. Seedlings roots were briefly stained in propidium iodide (PI) for cell wall visualization. Default excitement and emission settings for YFP and PI signals were used for imaging. Care was taken to ensure each root was imaged on similar Z-axis positions marked by the maximum nucleus size.
Pigment Accumulation and Trichome Analysis
Photographs of seed coat color were taken with a Canon EOS Rebel XSi. The hypocotyls of three-day-old seedlings grown on mineral nutrient mix media were examined and photographed for their anthocyanin accumulation using a Wild Makroskop M420 photomacroscope. Trichomes from one of the first two postembryonic leaves (referred to as the “first true leaves”) were analyzed using a Leica MS5 stereomicroscope (Larkin et al., 1999). Only trichomes that clearly protruded from the surface of the leaf were counted. For each genotype, three independent biological replicates were performed. For each replicate, trichomes were counted on one of the first true leaves from 10 independent plants. A trichome cluster was defined as two or more trichomes located immediately adjacent to each other, without intervening adjacent cells, and these were analyzed as described (Larkin et al., 1994a).
RNA Extraction and Reverse Transcriptase Quantitative PCR (RT-qPCR)
Root tips including the meristematic zone, elongation zone, and early maturation zone were used for total RNA extraction with the RNeasy Plant Mini kit (QIAGEN) (Huang and Schiefelbein, 2015). RNA was treated with the RQ1 DNase (Promega), and cDNA was synthesized using the SuperScript First-Strand Synthesis System (Invitrogen). The qPCR experiments used the Radiant Green Hi-Rox qPCR Kit (Alkali Scientific Inc.) and was conducted with the StepOnePlus real-time PCR system (Applied Biosystems). The relative transcript amounts were determined using the Delta-Delta-Ct method (Livak and Schmittgen, 2001). The GAPCP2 gene (AT1G16300, encoding a GAPDH isoform) was used as the internal reference gene. For each genotype, three independent biological replicates were performed. Primers used for RT-qPCR are listed in Supplementary Table S1.
Yeast Two-Hybrid Assays
Yeast two-hybrid assays were conducted as previously described (Lee and Schiefelbein, 1999; Bernhardt et al., 2003). The entire coding region of the GL3 cDNA was joined as a C-terminal fusion to the yeast GAL4 DNA-binding domain in pGBT9 to generate the in-frame protein fusion BD-GL3. The GAL4 transcriptional activation domain in pGAD424 was fused to the full-length TTG1-coding region of wild-type, ttg1-23 and ttg1-24 to generate AD-TTG1(WT), AD-TTG1(ttg1-23), and AD-TTG1(ttg1-24), respectively. After transformation into yeast strain AH109, the β-galactosidase assays were performed on at least three individual transformants (3 biological replicates) for each combination of constructs using the Yeast β-Galactosidase Assays Kit (Thermo Fisher Scientific).
Results
Isolation of a Novel Root Epidermis Development Mutant
The cpc-1 mutant produces approximately 40% of the number of root hairs found in wild-type roots (Lee and Schiefelbein, 2002), which provides a perturbed root-hair pattern useful for secondary genetic screens to discover new root epidermis development genes. We performed an ethyl methanesulfonate (EMS)-based enhancer screen in the cpc-1 GL2:GUS background and identified seedlings in subsequent generations exhibiting a more extreme reduced root-hair phenotype. One of the resulting lines, ultimately designated as cpc-1 ttg1-23 (see below), generated very few root hairs (approximately 4% of the wild type number; Figure 1A and Table 1) and exhibited increased ectopic expression of GL2:GUS in differentiating H-position cells as compared to cpc-1 (Figures 1B, 2). Further, we observed a 3:1 segregation ratio of the cpc-like phenotype to the double mutant-like phenotype (hairless) among offspring from a self-pollinated cpc-1/cpc-1 ttg1-23/+ population, indicating that the ttg1-23 mutation is recessive. These results indicate that the new cpc-1 enhancing mutation acts at an early developmental stage (upstream of GL2) to cause a change in root epidermal cell fate (from root-hair cells to non-hair cells).
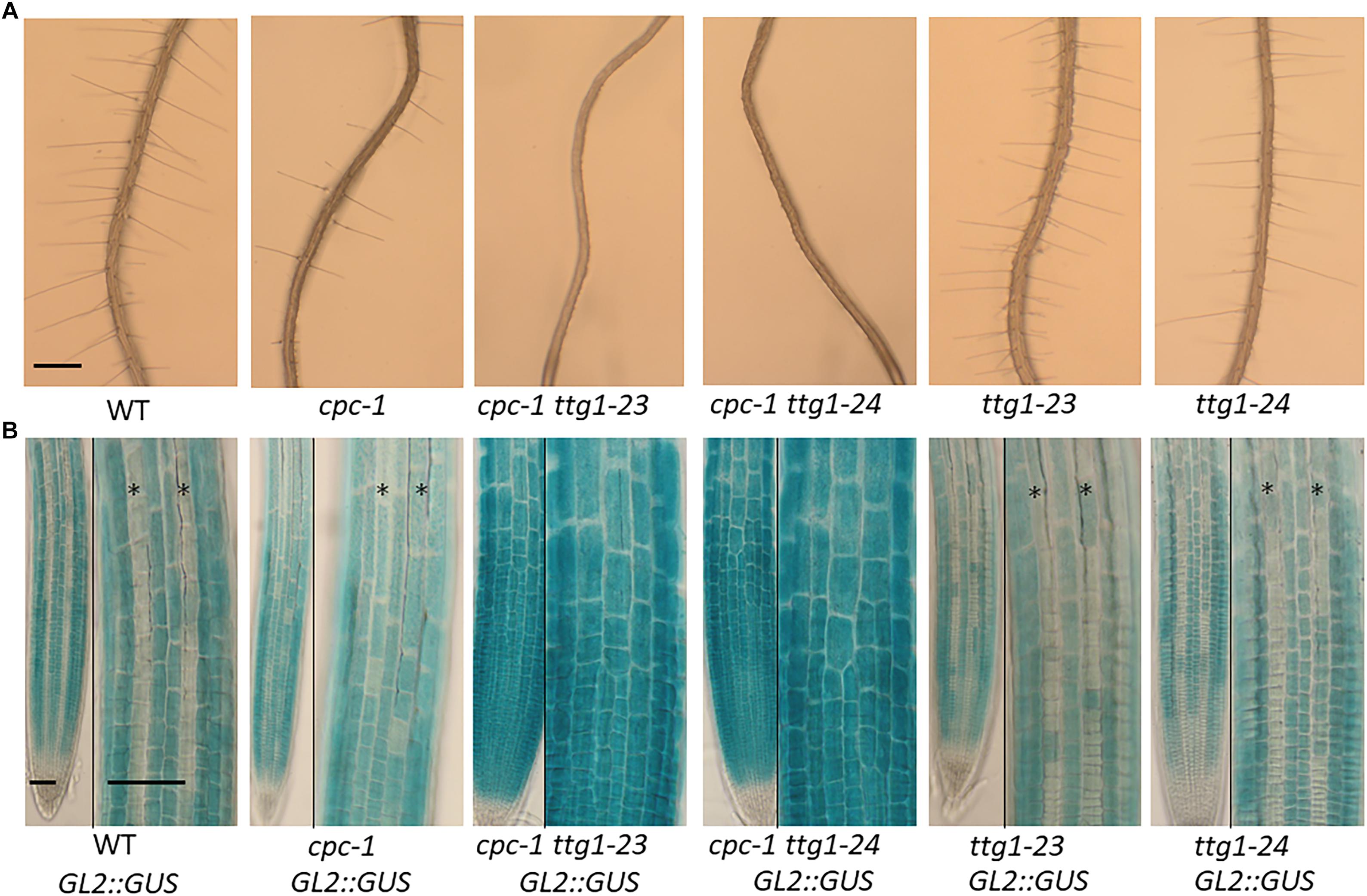
Figure 1. Characterization of the ttg1-23 and ttg1-24 mutants. (A) Seedling roots of wild type, cpc-1, cpc-1 23, cpc-1 24, 23, and 24 displaying their root hair phenotypes. Bar = 200 μm. (B) GL2:GUS reporter expression in seedling root tips of wild type, cpc-1, cpc-1 23, cpc-1 24, 23, and 24. Stars indicate H-position epidermal cell files. For each panel, the left and right images show the same root under different magnification. Bar = 50 μm.
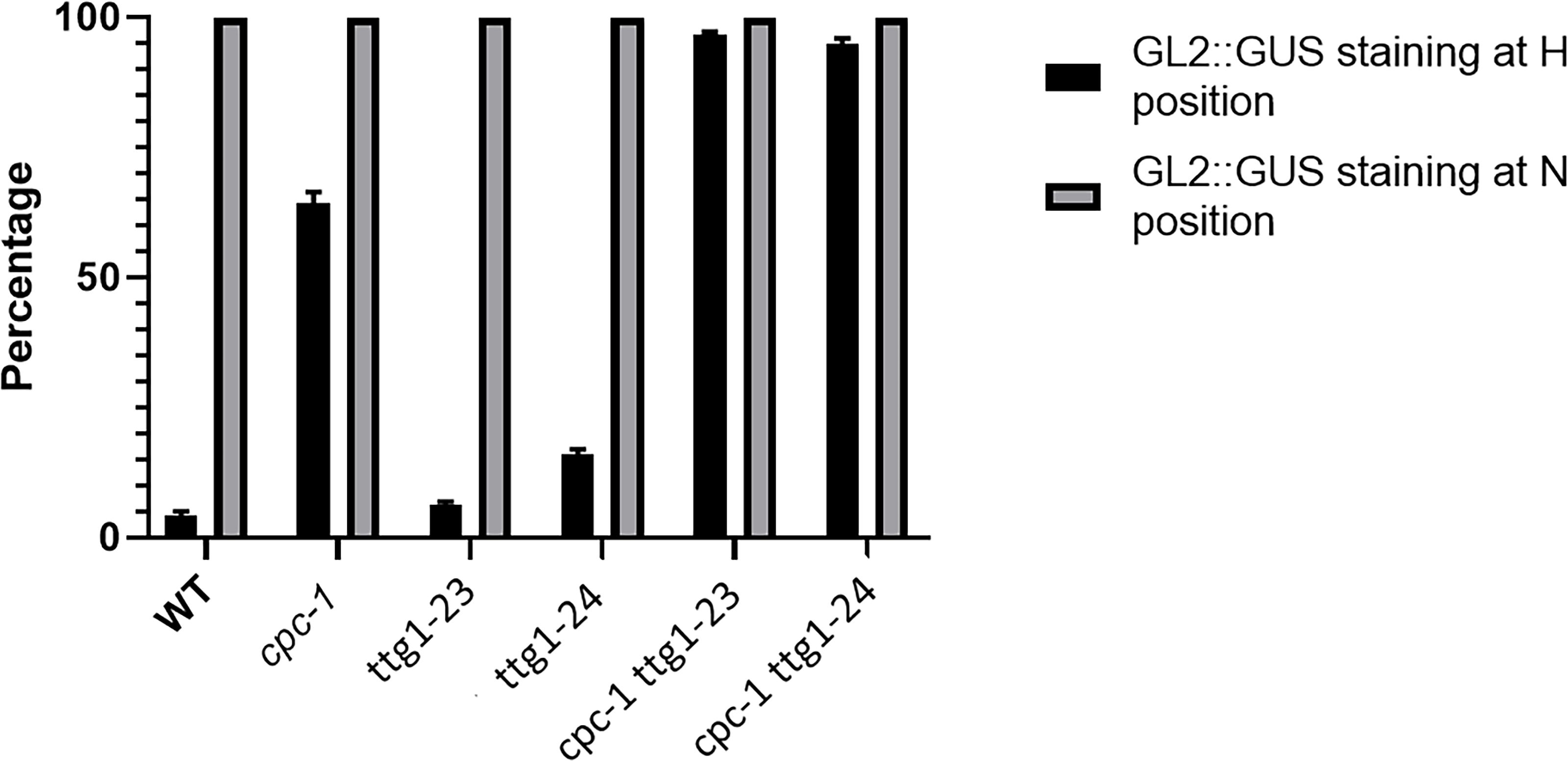
Figure 2. Histograms of GL2:GUS expression in H-position and N-position root epidermal cells of WT, cpc-1, ttg1-23, ttg1-24, cpc-1 ttg1-23, and cpc-1 ttg1-24 seedling root tips. Ten cells from each of the H and N positions in each root were measured. Ten seedling roots were analyzed for each replicate. Three independent biological replicates were performed for each genotype. Data shown are means (+SD).
The ttg1-23 single mutant, separated genetically from cpc-1, does not exhibit an obvious defect in root epidermis development. It produces approximately 93% root-hair cells at the H-cell position and 99% non-hair cells at the N-cell position, which is comparable to the wild type (Table 1). It also exhibits a spatial distribution of GL2:GUS expressing cells and GL2:GUS non-expressing cells that is similar to the wild type (Figures 1B, 2). Overall, we conclude that the ttg1-23 strongly enhances the reduced root-hair phenotype of cpc-1, but in a wild-type background, it does not significantly alter root epidermal cell-type specification.
Identification of TTG1 as the Mutated Gene in the Enhancer Mutant
To identify the mutated gene in the ttg1-23 line, we conducted genetic mapping with molecular markers and whole genome sequencing (see section “Materials and Methods”). We narrowed the genetic location of the ttg1-23 mutation to a region on chromosome 5 near the marker NGA139 at 8.4 Mb (Bell and Ecker, 1994). Upon analyzing the ttg1-23 genome sequence in this region, we detected a single C-G to T-A substitution within the TTG1 gene, which changes the serine encoded by the 197th codon to phenylalanine (S197F) in the predicted TTG1 protein (Figure 3). This amino acid substitution is located near the end of the third WD40 repeat (among four total WD40 repeats) of the TTG1 protein (Figure 3). Accordingly, we designated this new mutant ttg1 allele as ttg1-23. Consistent with a mutation in the TTG1 gene, the ttg1-23 line also exhibited reduced seed coat pigmentation, reduced trichome formation, and diminished seedling anthocyanin production (Figure 4, Table 2, and Supplementary Figure S1).
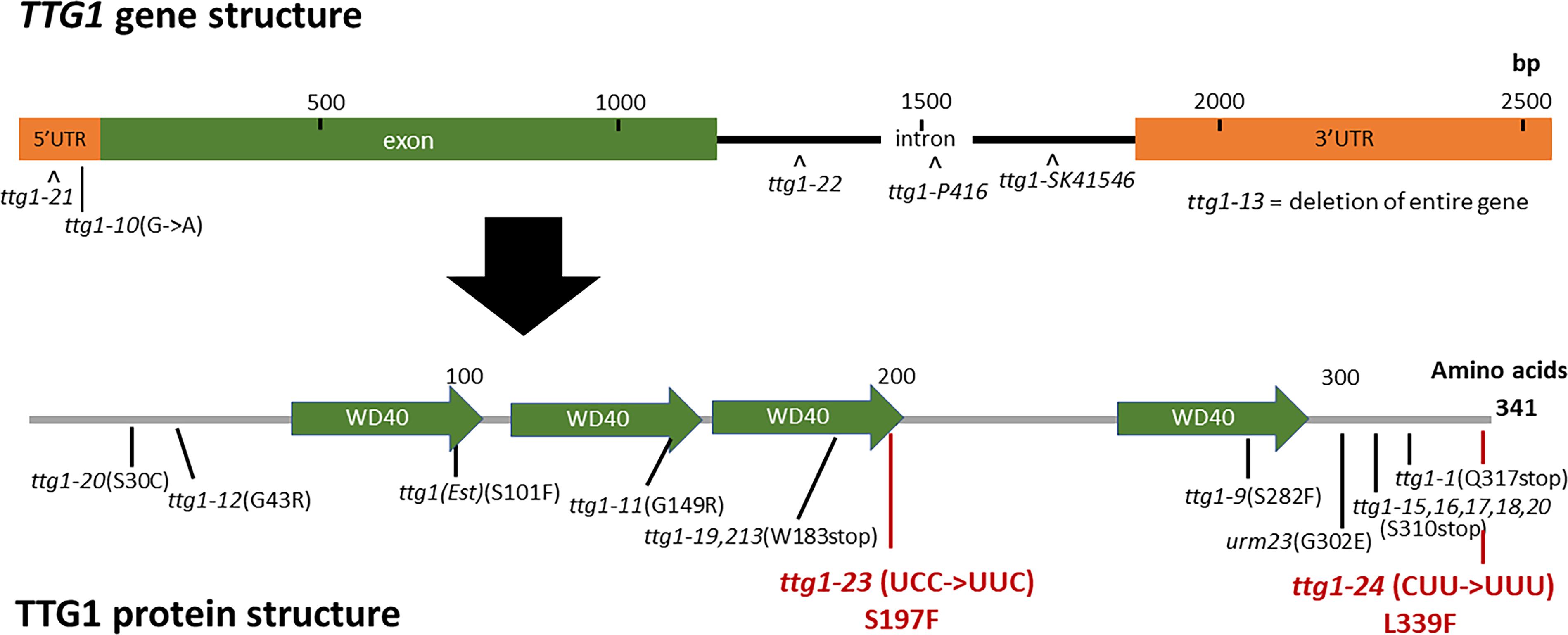
Figure 3. TTG1 gene structure, protein structure, and mutations. The TTG1 gene structure was retrieved from TAIR. The protein domains and WD40 repeats were annotated by SMART. Previously characterized mutations are indicated in black; new mutations found in this study are indicated in red.
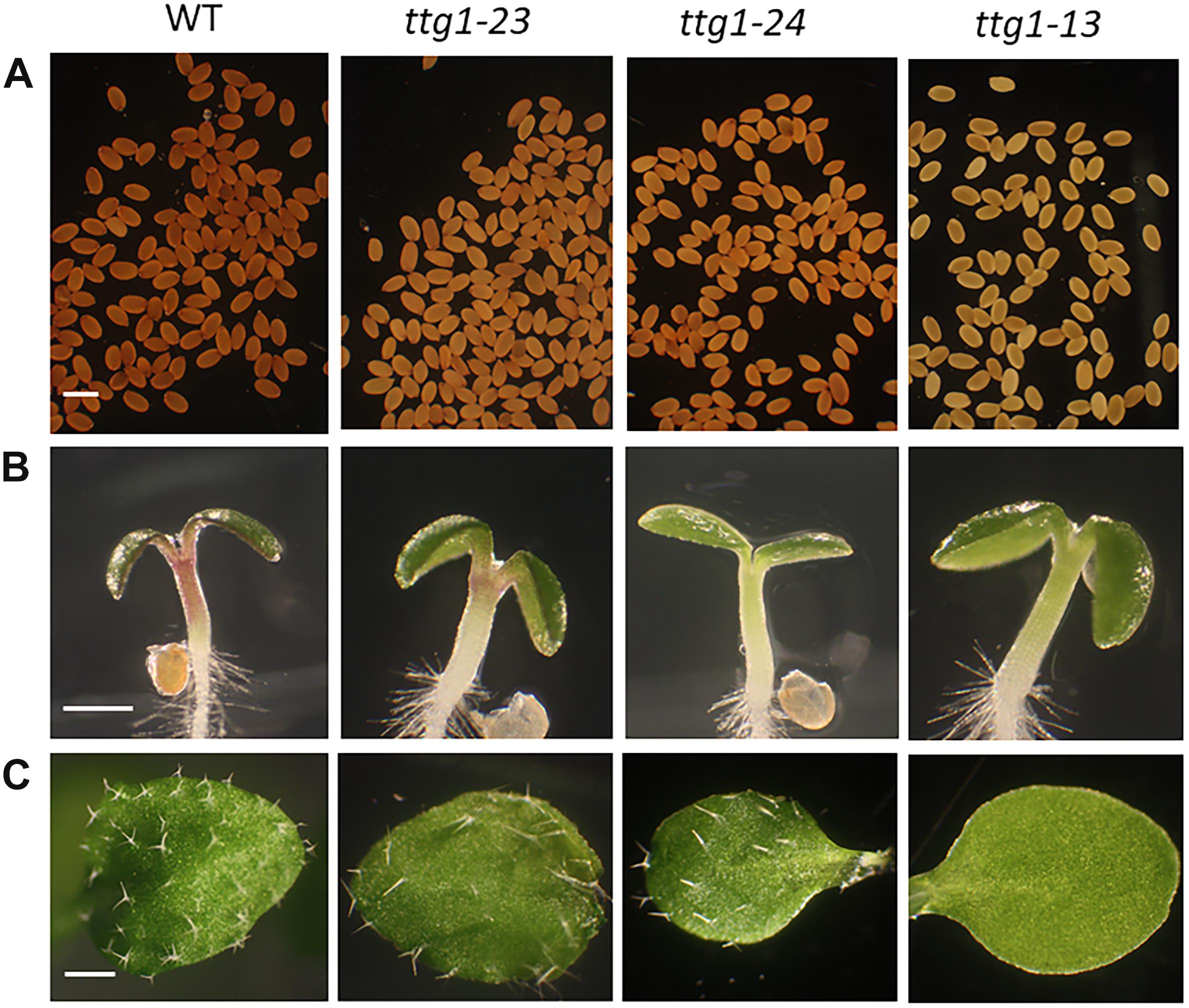
Figure 4. TTG1-related phenotypes in WT, ttg1-23, ttg1-24, and ttg1-13. (A) Proanthocyanidin accumulation in the seed. Bar = 1 mm. (B) Anthocyanin accumulation in the hypocotyl of 3-day-old seedlings. Bar = 10 mm. (C) Trichome production on one of the first true leaves. Bar = 2 mm.
In the same EMS-based genetic screen, we identified a second cpc-1 enhancer that resembled the cpc-1 ttg1-23 line. This second line produced approximately 12.5% root-hair cells at the H-cell position and exhibited increased ectopic expression of GL2:GUS relative to cpc-1 (Figures 1, 2, and Table 1). A line homozygous for the enhancer mutation alone, separated genetically from the cpc-1 mutation, produced a significant root epidermal mutant phenotype, with more non-hair cells (15%) and a greater proportion of GL2:GUS expressing cells (16%) at the H position than wild type or ttg1-23 (Figures 1, 2, and Table 1). Like the ttg1-23 line, we found that this second enhancer mutant also exhibits reduced seed pigmentation, diminished trichome production, and reduced seedling anthocyanin (Figure 4, Table 2, and Supplementary Figure S1). Given the similar phenotypes, we sequenced the TTG1 gene in this line and detected a single C-G to T-A substitution in codon 339, which changes a leucine to phenylalanine (L339F) near the C-terminus of the predicted 341 amino acid TTG1 protein (Figure 3). We designated the mutation in this second enhancer line as ttg1-24.
To determine whether the identified TTG1 mutations in the ttg1-23 and ttg1-24 lines are responsible for their root epidermal phenotypes, we introduced a wild-type 5-kb TTG1 genomic DNA fragment (TTG1:TTG1 transgene, including 5’ and 3’ flanking sequences) into the cpc-1 ttg1-23 and cpc-1 ttg1-24 mutants. The resulting cpc-1 ttg1-23 TTG1:TTG1 and cpc-1 ttg1-24 TTG1:TTG1 plants each exhibit a root-hair phenotype that is comparable to the cpc-1 single mutant (Table 1 and Supplementary Figure S2). These results indicate that the two single nucleotide changes (affecting codons 197 and 339) in the TTG1 gene are the mutations responsible for enhancing the root epidermal phenotype of cpc-1 in these two lines.
Weak ttg1 Mutant Alleles Confer a Similar Root Epidermal Phenotype
Our finding that the ttg1-23 and ttg1-24 mutations enhance the cpc-1 phenotype (i.e., increase the production of non-hair cells) was unexpected, because previous studies have shown that ttg1 mutants produce more root-hair cells and thus TTG1 is likely involved in non-hair cell specification (Galway et al., 1994). To investigate this discrepancy, we examined additional mutant alleles of the TTG1 gene which have not been analyzed in detail for their root-hair phenotypes (Zhang and Schrader, 2017). We selected three previously described ttg1 mutant lines: ttg1-1, ttg1-9, and ttg1-13. The ttg1-1 bears a single C-to-T mutation that introduces a stop codon in place of a glutamine codon at position 317 (Figure 3), leading to a truncated TTG1 protein lacking the C-terminal 25 amino acid residues (Walker et al., 1999). The ttg1-13 mutation is a large deletion (>4 kb) encompassing the entire TTG1 gene (Larkin et al., 1999). Consistent with the nature of their mutations, both ttg1-1 and ttg1-13 are known to exhibit strong (presumed null) mutant phenotypes for other TTG1-related processes. In contrast, the ttg1-9 mutant is reported to exhibit relatively weak TTG1-related phenotypes, and it contains a C-to-T substitution causing an amino acid change from serine to phenylalanine at position 282 (S282F) (Figure 3; Larkin et al., 1994b; Walker et al., 1999).
To compare the effects of these three ttg1 mutations (ttg1-1, ttg1-9, and ttg1-13) with ttg1-24, ttg1-23, and wild-type, we quantified the root epidermis cell types produced in each single mutant and each cpc-1 double mutant. We found that the ttg1-1 and ttg1-13 roots exhibit a strong hairy phenotype, with root-hair cells produced throughout the H position (100% hair cells) and in most of the N position (91 and 93% hair cells) (Table 1 and Figure 5). In contrast, the ttg1-9 roots exhibit a minor cell-type defect, with only a small fraction of ectopic hair cells in the N-position (3%) and ectopic non-hair cells in the H position (6%) (Table 1 and Figure 5). This ttg1-9 phenotype is comparable to the mild root epidermal phenotypes exhibited by the ttg1-23 and ttg1-24 mutants (Table 1). These results suggest that strong defects in TTG1 function (e.g., truncated protein or gene deletion) essentially prevent non-hair cell specification, whereas weak TTG1 mutations (e.g., missense mutations) have a mild effect on root epidermal cell specification.
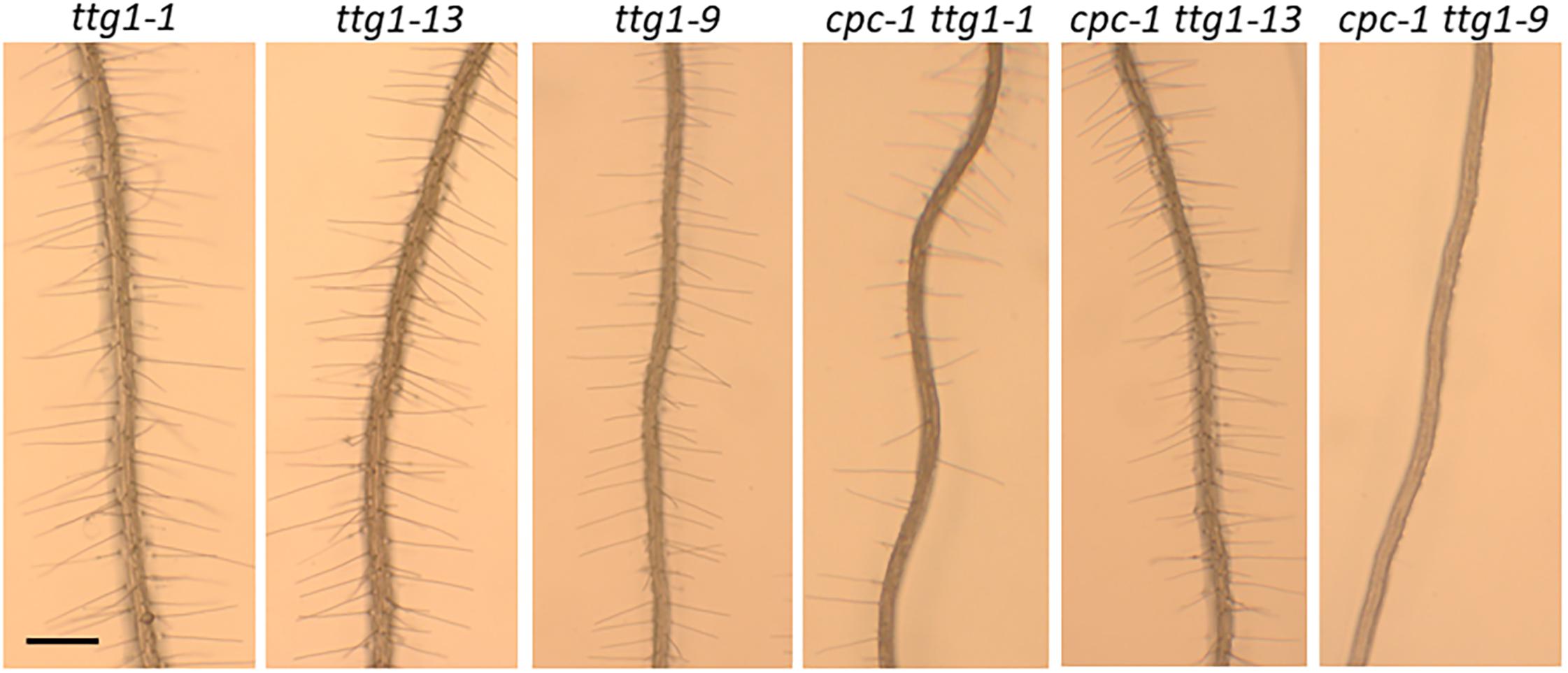
Figure 5. Seedling roots of ttg1-1, ttg1-13, ttg1-9, cpc-1 ttg1-1, cpc-1 ttg1-13, and cpc-1 ttg1-9 displaying their root hair phenotypes. Bar = 200 μm.
The analysis of double mutants with cpc-1 showed a similar trend. The cpc-1 ttg1-1 double mutant produced 57% root-hair cells at the H position and 9% root-hair cells at N position, and cpc-1 ttg1-13 produced 100% root-hair cells at the H position and 72% root-hair cells at the N position (Table 1 and Figure 5). This indicates that these two strong ttg1 mutations do not enhance cpc-1 but instead, they suppress the cpc-1 mutant phenotype. Interestingly, the cpc-1 ttg1-9 double mutant exhibits a nearly hairless root phenotype, similar to cpc-1 ttg1-23 and cpc-1 ttg1-24 (Table 1 and Figure 5), indicating that ttg1-9 enhances the cpc-1 mutant phenotype.
We also examined the relative effect of these ttg mutants and cpc ttg double mutants on trichome formation. Consistent with the root epidermal phenotypes, we observed two distinct classes of trichome mutant phenotypes. The ttg1-1 and ttg1-13 produce glabrous leaves, and inclusion of cpc-1 into these mutants (i.e., cpc-1 ttg1-1 and cpc-1 ttg1-13) does not alter this phenotype (Table 2). On the other hand, ttg1-9, ttg1-23, and ttg1-24 are able to produce a substantial number of leaf trichomes (approximately 50–80% of the wild-type number), with a fraction of these present in clusters (Table 2). The cpc-1 ttg1-9, cpc-1 ttg1-23, and cpc-1 ttg1-24 double mutants produce a similar number of trichomes and fraction of trichome clusters, but significantly less than the cpc-1 mutant (Table 2).
These results show that the ttg1-9, ttg1-23, and ttg1-24 mutations generate similar effects on epidermal cell differentiation, which are distinct from the effects of the strong ttg1-1 and ttg1-13 mutations. Given that ttg1-9, ttg1-23, and ttg1-24 alter different residues of the TTG1 protein, it is likely that their common phenotypes represent the general impact of a partially functional TTG1 protein.
The ttg1 Mutations Differentially Affect Target Gene Expression
Next, we sought to understand how the various ttg1 mutants yield their distinct effects on root epidermal patterning and enhancement of the cpc-1 phenotype. As a component of the central MBW complex that specifies root epidermal cell fates, TTG1 is believed to participate in transcriptional activation of multiple target genes. These include the presumed direct activation of four target genes: GL2, MYB23, CPC, and TRY genes (Schiefelbein et al., 2014). We analyzed the transcript level for these four genes in the developing root from the five ttg1 mutants and wild type using quantitative real-time PCR.
The most striking result from this analysis is the different effects on GL2 expression. The GL2 transcript level is dramatically reduced in the strong ttg1-1 and ttg1-13 mutants, whereas the weak ttg1-9, ttg1-23 and ttg1-24 show near-normal GL2 levels (Figure 6A). Given the critical role of GL2 in specifying the non-hair cell type, these differences in GL2 expression among the ttg1 mutants are consistent with the root epidermal phenotypes of the corresponding mutants. Specifically, the ttg1-1 and ttg1-13 mutants essentially lack non-hair cells (Table 1) and ttg1-1 produces a very low level of GL2:GUS transcriptional reporter expression (Hung et al., 1998). On the other hand, the ttg1-9, ttg1-23, and ttg1-24 produce near-normal amounts of non-hair cells (Table 1), and ttg1-23 and ttg1-24 generate near-normal GL2:GUS transcriptional reporter expression in the developing root epidermis (Figure 1). The strong correlation between GL2 gene expression and epidermal phenotypes suggest that differences in the ability of the various ttg1 mutant proteins to activate GL2 expression is primarily responsible for their different root epidermis phenotypes.
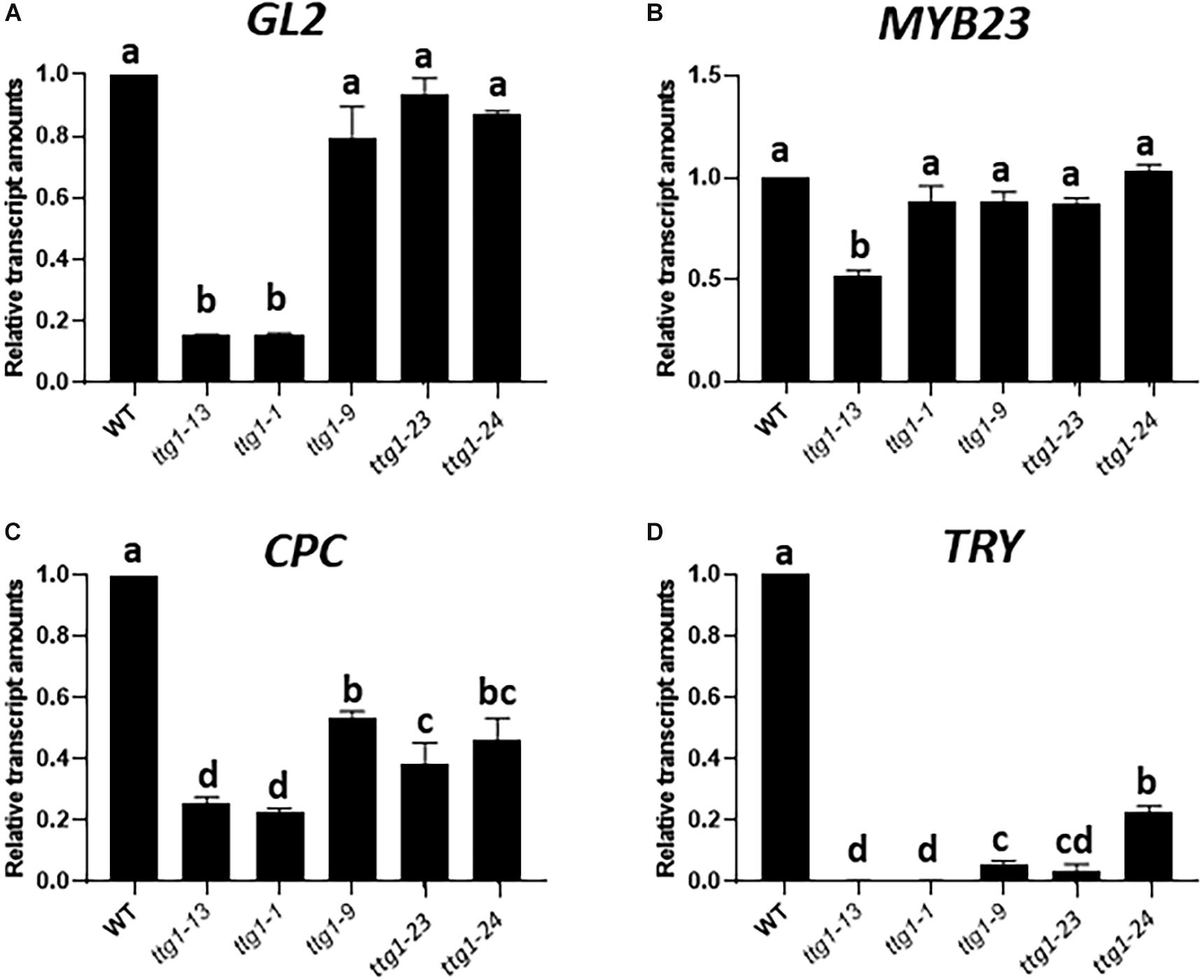
Figure 6. Relative transcript amounts from (A) GL2, (B) MYB23, (C) CPC, and (D) TRY genes from seedling root tips of WT, ttg1-13, ttg1-1, ttg1-9, ttg1-23, and ttg1-24, determined with RT-qPCR. Three independent biological replicates were performed for each genotype. Error bars represent standard deviations. For each panel, bars marked with the same letter indicate values not significantly different; bars marked with different letters indicate values showing statistically significant differences (P < 0.05; ANOVA testt).
The transcript levels of CPC and TRY, the two genes encoding lateral inhibitors, are decreased in each of the ttg1 mutants (Figures 6C,D). Interestingly, the weak ttg1 mutants (ttg1-9, ttg1-23, and ttg1-24), possess a higher level of CPC RNA than the strong ttg1 mutants (ttg1-1, ttg1-13), which may enable the weak ttg1 mutants to achieve lateral inhibition and explain why they exhibit a near-normal root-hair pattern. Indeed, the effect of cpc-1 on the root epidermis phenotype of these weak ttg1 mutants (Table 1) demonstrates that they possess CPC function because the root-hair production and pattern formation in these weak ttg1 mutants is CPC-dependent. It is notable that TRY transcript levels are strongly diminished, relative to CPC, in all ttg1 mutants (Figure 6D). The relatively low level of TRY expression compared to CPC in the weak ttg1 mutants may provide an explanation for the ability of the weak ttg1 mutants to enhance the cpc-1 phenotype (see section “Discussion”). Finally, we observe substantial MYB23 transcript accumulation in the ttg1 mutants and wild type, indicating that TTG1 is perhaps not essential for transcription of MYB23 (Figure 6B).
Mutant TTG1 Proteins Exhibit Altered Interaction With GL3
In the MBW complex for root epidermal patterning, TTG1 is reported to directly interact with the GL3 bHLH protein (Payne et al., 2000). To determine whether the mutated TTG1 proteins in ttg1-23 and ttg1-24 have altered interaction with GL3, we conducted a yeast two-hybrid assay. The GL3 cDNA was fused to the sequence encoding the GAL4 DNA binding domain (BD), and three different TTG1 cDNAs (representing wild type TTG1, ttg1-23, and ttg1-24 gene products) were fused to sequences encoding the GAL4 activation domain (AD). The introduction of BD-GL3 and AD-empty constructs into yeast cells yielded some lacZ reporter expression, but higher reporter expression was achieved in yeast co-expressing BD-GL3 fusion together with the AD-TTG1 fusion (Figure 7). Significantly, lower β-galactosidase activities were detected in yeast cells co-expressing BD-GL3 fusions with AD-TTG1(ttg1-23) and AD-TTG1(ttg1-24) than those co-expressing BD-GL3 with AD-TTG1(WT) (Figure 7). As controls, yeast cells co-expressing BD-empty together with AD-empty, AD-TTG1(WT), AD-TTG1(ttg1-23), or AD-TTG1(ttg1-24) were not able to grow on the selection plate, and very low β-galactosidase activities were measured in these combinations (Figure 7). These results indicate that all three tested TTG1 proteins are able to physically interact with the GL3 bHLH protein in yeast, but the mutated TTG1 proteins (from ttg1-23 and ttg1-24) have a diminished ability to interact. This suggests that the amino acid changes in ttg1-23 and ttg1-24 alter protein-protein binding of TTG with GL3, which may reduce the effectiveness of the MBW complex and lead to the observed root epidermis defects.
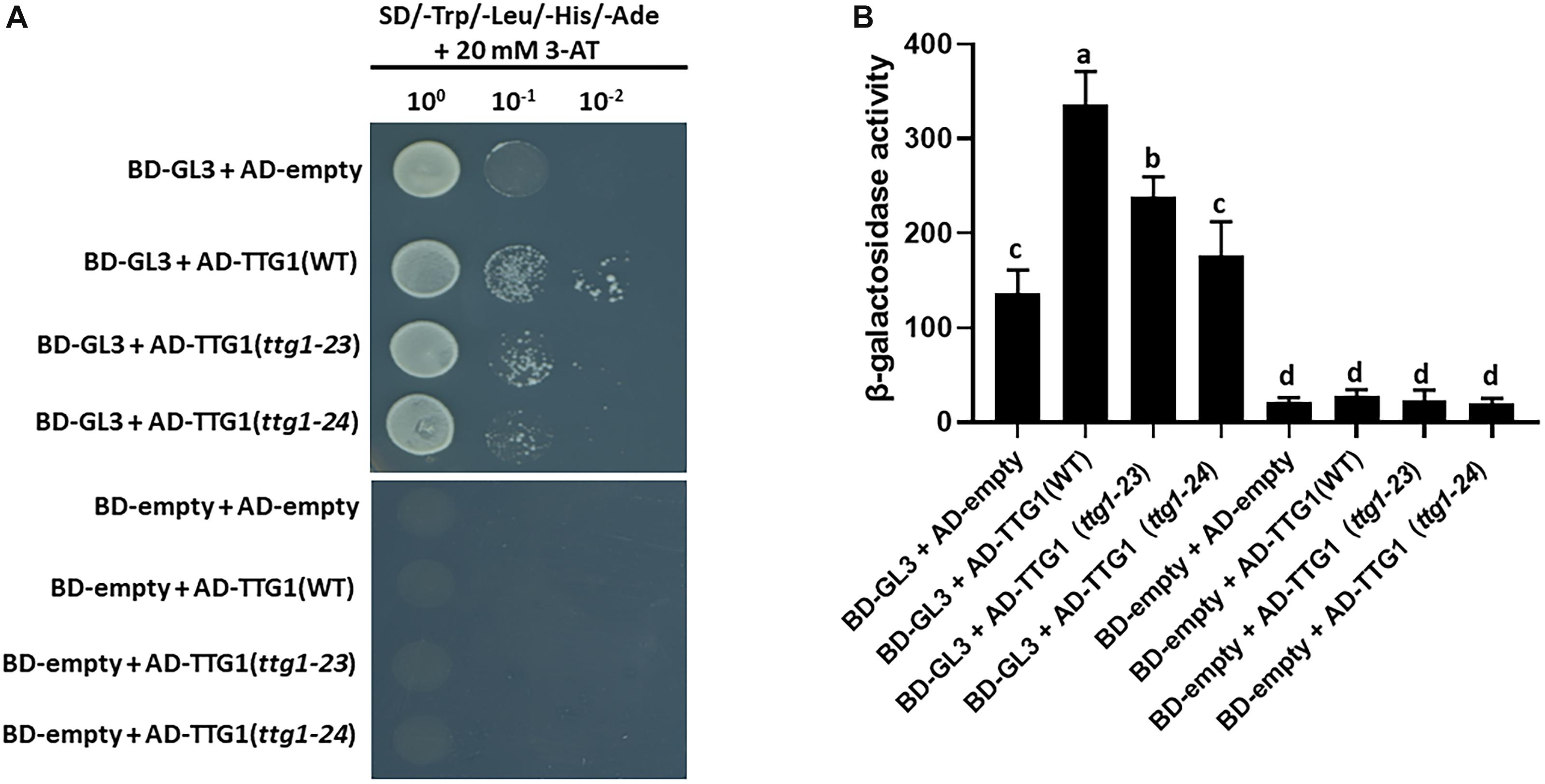
Figure 7. Yeast two-hybrid protein interaction assays. (A) The growth of yeast cells harboring the GL3 fused to the GAL4 DNA-binding domain (BD-GL3) or the GAL4 DNA-binding domain alons (BD-empty), and TTG1(WT), TTG1(ttg1-23), or TTG1(ttg1-24) fused to the GAL4 activation domain (AD) are shown. The yeast cells are grown on the SD/-Trp/-Leu/-His/-Ade selection medium with 20 mM 3-aminotriazole (3-AT). For serial dilution analysis, yeast cells were collected and adjusted to OD600 = 1.0, and diluted to 100, 10–1, and 10–2. (B) Yeast two-hybrid β-galactosidase activity assay quantifying the interaction between GL3 and TTG1(WT), TTG1(ttg1-23) or TTG1(ttg1-24). Three independent biological replicates were performed for each combination. Error bars represent standard deviations from the three replicates. Bars marked with the same letter indicate values not significantly different; bars marked with different letters indicate values showing statistically significant differences (P < 0.05; ANOVA test).
Accumulation of Wild-Type and Mutated TTG1 Proteins in the Root Epidermis
To determine whether the ttg1-23 and ttg1-24 mutations alter the accumulation of TTG1 protein in the root epidermal cells, we generated EYFP translational fusions to three versions of TTG1 (from wild type, ttg1-23, and ttg1-24) in the ttg1-13 mutant background (which bears a deletion of TTG1). Transgenic plants bearing the wild-type TTG1:TTG1-EYFP construct showed TTG1-EYFP accumulation in all epidermal cells (both H- and N-position) and predominantly in the cytoplasm (Figure 8). Both the TTG1:ttg1-23-EYFP and TTG1:ttg1-24-EYFP proteins exhibit a similar EYFP accumulation pattern as TTG1-EYFP (Figure 8), indicating that the ttg1-23 and ttg1-24 mutations have little if any effect on the cellular accumulation of the TTG1 protein.
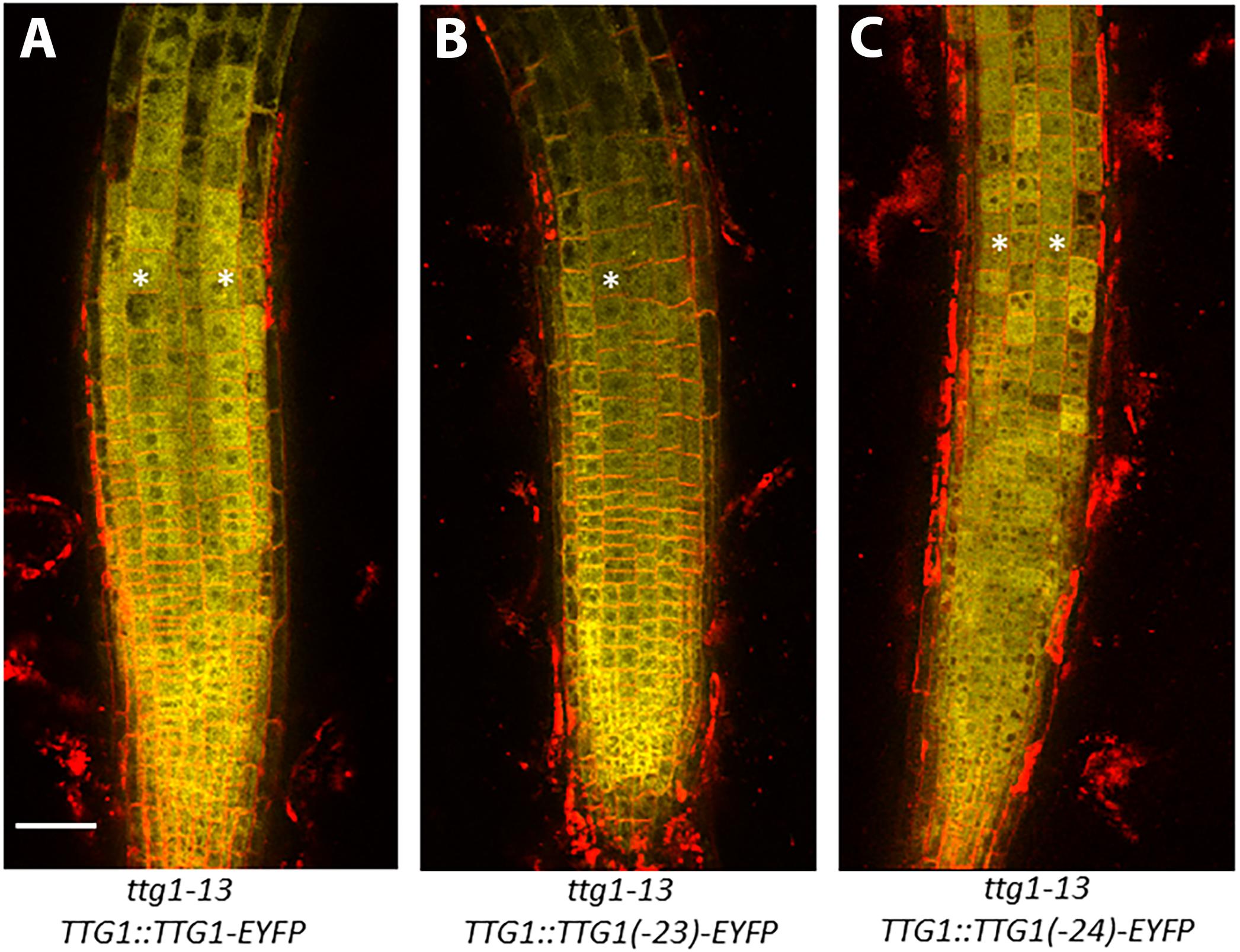
Figure 8. Accumulation of TTG1-EYFP fusion proteins in ttg1-13 seedling roots bearing the (A) TTG1:TTG1(WT)-EYFP, (B) TTG1:TTG1(ttg1-23)-EYFP, or (C) TTG1:TTG1(ttg1-24)-EYFP transgene. White stars mark the H-position epidermal cell files. The red color represents propidium iodide and the yellow color represents YFP. Representative images are shown from the analysis of at least 3 independent single-insertion lines for each transgenic experiment. Bar = 50 μm.
Discussion
The TTG1 protein has been known to be a component of the MBW complex that regulates root-hair patterning in Arabidopsis (Galway et al., 1994). However, the precise role and importance of TTG1 for the function of the MBW complex has not been defined. Previously reported strong ttg1 mutants were found to produce all root-hair cells in their root epidermis, suggesting that TTG1 is involved in specifying the non-hair cell type (Galway et al., 1994). In another study, ectopic overexpression of the bHLH factors GL3 and/or EGL3 in the ttg1 mutant background yielded an entirely hairless root (Bernhardt et al., 2003), implying that TTG1 is not essential for the MYB-bHLH induction of non-hair gene transcription but may influence its level or activity. Here, we surprisingly found that two new ttg1 mutants cause an increase in non-hair cells and enhance the cpc-1 mutation, which is distinct from the hairy root phenotype of the strong ttg1-1 and ttg1-13 mutants. Our analysis of target gene expression in these lines provides an explanation for these different ttg1 mutant phenotypes. Although strong ttg1 mutants have very low levels of GL2, CPC, and TRY transcripts, our new ttg1 mutants exhibit differences in the expression of the various MBW target genes. GL2 gene expression is not significantly reduced, yet CPC and TRY gene expression is substantially affected. This means that, in our new ttg1 mutants, the non-hair cell fate will tend to be induced to a greater extent than normal, which is consistent with the observed phenotypes. Taken together, our study suggests that TTG1 is best considered as a component of the MBW complex that is necessary to ensure the proper relative activation of downstream target genes to generate the appropriate proportion and pattern of the root-hair and non-hair cell types.
In addition to the two new ttg1 mutants identified in this study (ttg1-23 and ttg1-24), we also discovered that a previously isolated ttg1 missense mutant (ttg1-9) produced a similar effect on root epidermis development. Thus, we found that three independent amino-acid substitution variants of the TTG1 protein generate essentially the same phenotype, including a mild defect in root epidermal pattern, an ability to enhance the cpc-1 reduced-hair phenotype, and a distortion in the relative expression of MBW target genes. The locations of the amino acid substitutions in these three mutants are dispersed along the TTG1 protein (residues 197, 282, and 339), suggesting that their common phenotype is not due to alteration of a particular protein motif or domain. Our yeast two-hybrid analysis of the ttg1-23 and ttg1-24 proteins showed a reduction in their ability to interact with their partner bHLH (GL3) protein (Figure 7). Also, we observed a comparable accumulation of EYFP-tagged proteins for the ttg1-23 and ttg1-24 variants as for the wild-type TTG1 (Figure 8). Together, these findings suggest that the similar phenotype of these ttg1 missense mutants is the result of a common negative effect of these amino acid substitutions on TTG1-bHLH interaction, which leads to reduced MBW complex formation and altered target gene expression. This explanation implies that multiple residues/regions of the TTG1 protein are likely to be important for proper TTG1-bHLH interaction. In a previous study, the C-terminal 25 amino acids of the TTG1 protein were shown to be essential for interaction with GL3 in yeast (Payne et al., 2000). Our results also imply that the MBW target genes possess differential sensitivity to the level of the MBW complex. In particular, among the four target genes tested, expression of the TRY gene appears to be most sensitive to MBW level, whereas GL2 is least sensitive (Figure 6). In this respect, it is notable that the requirement for TTG1 (i.e., the requirement for a MBW complex) for non-hair cell differentiation can be bypassed by overexpression of GL3 or EGL3 (Bernhardt et al., 2003), suggesting that high levels of the bHLH and MYB components are sufficient to induce target gene expression. In future studies, it will be informative to analyze the presumed differential protein-DNA interactions in greater detail to evaluate the importance of MBW complex on its various downstream targets.
The ability of the ttg1-23 and ttg1-24 mutations to enhance, rather than suppress, the cpc-1 mutant phenotype was initially puzzling. However, given the effect of these mutations on MBW target gene expression, we can provide the following explanation. First, it is known that TRY encodes a CPC-like R3-type MYB protein, which is partially functionally redundant with CPC, and mutations in TRY are known to enhance the cpc-1 phenotype (Simon et al., 2007). We showed that each of the weak ttg1 mutants are defective in TRY gene expression (Figure 6). Therefore, in the cpc-1 mutant background, these weak ttg1 mutants will essentially lack the ability to produce the CPC/TRY lateral inhibitory proteins, due to the combination of the cpc mutation and their inability to activate TRY transcription. However, these weak ttg1 mutants retain substantial capacity to activate GL2 and MYB23 gene expression, which promote the non-hair cell fate. Thus, with respect to the root epidermal patterning process, these cpc-1 ttg1 mutants essentially behave like a cpc try mutant and, accordingly, result in a hairless root phenotype.
From an evolutionary point of view, it is interesting that single amino acid substitutions in the TTG1 protein are able to modify the pattern of epidermal cell types in the Arabidopsis root. In particular, the ttg1-24 mutant exhibits a novel distribution of root-hair cells and non-hair cells, significantly different from the wild-type (Table 1). This suggests that TTG1 could provide a target for mutations that can yield novel and potentially beneficial distributions of root-hair cells and non-hair cells in plants. It is notable that none of the sequenced natural accessions of Arabidopsis thaliana exhibit differences in their predicted TTG1 protein product1. The future molecular characterization of root hair patterns in a broad array of plant species will likely uncover the mechanisms responsible for the evolution of new cell type patterns in plant roots.
Data Availability Statement
The datasets used in this study can be found in the NCBI using accession number PRJNA610815.
Author Contributions
YL conducted the experiments. Both authors analyzed the data, designed the experiments, wrote the manuscript, and approved the final version of the manuscript.
Funding
This work was supported by a grant from the U.S. National Science Foundation (IOS-1444400).
Conflict of Interest
The authors declare that the research was conducted in the absence of any commercial or financial relationships that could be construed as a potential conflict of interest.
Acknowledgments
We thank the Arabidopsis Biological Resource Center for providing seed stocks of multiple mutant lines. We also thank members of the Schiefelbein laboratory for helpful suggestions and discussions.
Supplementary Material
The Supplementary Material for this article can be found online at: https://www.frontiersin.org/articles/10.3389/fpls.2020.00383/full#supplementary-material
Footnotes
References
Baudry, A., Heim, M. A., Dubreucq, B., Caboche, M., Weisshaar, B., and Lepiniec, L. (2004). TT2, TT8, and TTG1 synergistically specify the expression of BANYULS and proanthocyanidin biosynthesis in Arabidopsis thaliana. Plant J. 39, 366–380. doi: 10.1111/j.1365-313x.2004.02138.x
Bell, C. J., and Ecker, J. R. (1994). Assignment of 30 microsatellite loci to the linkage map of Arabidopsis. Genomics 19, 137–144. doi: 10.1006/geno.1994.1023
Berger, F., Haseloff, J., Schiefelbein, J., and Dolan, L. (1998). Positional information in root epidermis is defined during embryogenesis and acts in domains with strict boundaries. Curr. Biol. 8, 421–430. doi: 10.1016/s0960-9822(98)70176-9
Bernhardt, C., Lee, M. M., Gonzalez, A., Zhang, F., Lloyd, A., and Schiefelbein, J. (2003). The bHLH genes GLABRA3 (GL3) and ENHANCER OF GLABRA3 (EGL3) specify epidermal cell fate in the Arabidopsis root. Development 130, 6431–6439. doi: 10.1242/dev.00880
Bernhardt, C., Zhao, M., Gonzalez, A., Lloyd, A., and Schiefelbein, J. (2005). The bHLH genes GL3 and EGL3 participate in an intercellular regulatory circuit that controls cell patterning in the Arabidopsis root epidermis. Development 132, 291–298. doi: 10.1242/dev.01565
Bouyer, D., Geier, F., Kragler, F., Schnittger, A., Pesch, M., Wester, K., et al. (2008). Two-dimensional patterning by a trapping/depletion mechanism: the role of TTG1 and GL3 in Arabidopsis trichome formation. PLoS Biol. 6:e141. doi: 10.1371/journal.pbio.0060141
Bruex, A., Kainkaryam, R. M., Wieckowski, Y., Kang, Y. H., Bernhardt, C., Xia, Y., et al. (2012). A gene regulatory network for root epidermis cell differentiation in Arabidopsis. PLoS Genet. 8:e1002446. doi: 10.1371/journal.pgen.1002446
Clough, S. J., and Bent, A. F. (1998). Floral dip: a simplified method for Agrobacterium-mediated transformation of Arabidopsis thaliana. Plant J. 16, 735–743. doi: 10.1046/j.1365-313x.1998.00343.x
Cormack, R. (1962). Development of root hairs in angiosperms. II. Bot. Rev. 28, 446–464. doi: 10.1007/bf02868690
Di Cristina, M., Sessa, G., Dolan, L., Linstead, P., Baima, S., Ruberti, I., et al. (1996). The Arabidopsis Athb-10 (GLABRA2) is an HD-Zip protein required for regulation of root hair development. Plant J. 10, 393–402. doi: 10.1046/j.1365-313x.1996.10030393.x
Dolan, L., and Roberts, K. (1995). The development of cell pattern in the root epidermis. Philos. Trans. R. Soc. Lond. B Biol. Sci. 350, 95–99. doi: 10.1098/rstb.1995.0143
Duckett, C. M., Grierson, C., Linstead, P., Schneider, K., Lawson, E., Dean, C., et al. (1994). Clonal relationships and cell patterning in the root epidermis of Arabidopsis. Development 120, 2465–2474.
Estelle, M. A., and Somerville, C. (1987). Auxin-resistant mutants of Arabidopsis thaliana with an altered morphology. Mol. Gen. Genet. 206, 200–206. doi: 10.1007/bf00333575
Galway, M. E., Masucci, J. D., Lloyd, A. M., Walbot, V., Davis, R. W., and Schiefelbein, J. W. (1994). The TTG gene is required to specify epidermal cell fate and cell patterning in the Arabidopsis root. Dev. Biol. 166, 740–754. doi: 10.1006/dbio.1994.1352
Gonzalez, A., Mendenhall, J., Huo, Y., and Lloyd, A. (2009). TTG1 complex MYBs, MYB5 and TT2, control outer seed coat differentiation. Dev. Biol. 325, 412–421. doi: 10.1016/j.ydbio.2008.10.005
Gonzalez, A., Zhao, M., Leavitt, J. M., and Lloyd, A. M. (2008). Regulation of the anthocyanin biosynthetic pathway by the TTG1/bHLH/Myb transcriptional complex in Arabidopsis seedlings. Plant J. 53, 814–827. doi: 10.1111/j.1365-313x.2007.03373.x
Grebe, M. (2012). The patterning of epidermal hairs in Arabidopsis—updated. Curr. Opin. Plant Biol. 15, 31–37. doi: 10.1016/j.pbi.2011.10.010
Haughn, G., and Chaudhury, A. (2005). Genetic analysis of seed coat development in Arabidopsis. Trends Plant Sci. 10, 472–477. doi: 10.1016/j.tplants.2005.08.005
Huang, L., and Schiefelbein, J. (2015). Conserved gene expression programs in developing roots from diverse plants. Plant Cell 27, 2119–2132. doi: 10.1105/tpc.15.00328
Hung, C. Y., Lin, Y., Zhang, M., Pollock, S., Marks, M. D., and Schiefelbein, J. (1998). A common position-dependent mechanism controls cell-type patterning and GLABRA2 regulation in the root and hypocotyl epidermis of Arabidopsis. Plant Physiol. 117, 73–84. doi: 10.1104/pp.117.1.73
Kang, Y. H., Song, S.-K., Schiefelbein, J., and Lee, M. M. (2013). Nuclear trapping controls the position-dependent localization of CAPRICE in the root epidermis of Arabidopsis. Plant Physiol. 163, 193–204. doi: 10.1104/pp.113.221028
Kirik, V., Lee, M. M., Wester, K., Herrmann, U., Zheng, Z., Oppenheimer, D., et al. (2005). Functional diversification of MYB23 and GL1 genes in trichome morphogenesis and initiation. Development 132, 1477–1485. doi: 10.1242/dev.01708
Kirik, V., Simon, M., Huelskamp, M., and Schiefelbein, J. (2004). The ENHANCER OF TRY AND CPC1 gene acts redundantly with TRIPTYCHON and CAPRICE in trichome and root hair cell patterning in Arabidopsis. Dev. Biol. 268, 506–513. doi: 10.1016/j.ydbio.2003.12.037
Kurata, T., Ishida, T., Kawabata-Awai, C., Noguchi, M., Hattori, S., Sano, R., et al. (2005). Cell-to-cell movement of the CAPRICE protein in Arabidopsis root epidermal cell differentiation. Development 132, 5387–5398. doi: 10.1242/dev.02139
Kwak, S.-H., and Schiefelbein, J. (2007). The role of the SCRAMBLED receptor-like kinase in patterning the Arabidopsis root epidermis. Dev. Biol. 302, 118–131. doi: 10.1016/j.ydbio.2006.09.009
Kwak, S.-H., and Schiefelbein, J. (2008). A feedback mechanism controlling SCRAMBLED receptor accumulation and cell-type pattern in Arabidopsis. Curr. Biol. 18, 1949–1954. doi: 10.1016/j.cub.2008.10.064
Kwak, S.-H., Shen, R., and Schiefelbein, J. (2005). Positional signaling mediated by a receptor-like kinase in Arabidopsis. Science 307, 1111–1113. doi: 10.1126/science.1105373
Larkin, J. C., Oppenheimer, D. G., Lloyd, A. M., Paparozzi, E. T., and Marks, M. D. (1994a). Roles of the GLABROUS1 and TRANSPARENT TESTA GLABRA genes in Arabidopsis trichome development. Plant Cell 6, 1065–1076. doi: 10.1105/tpc.6.8.1065
Larkin, J. C., Oppenheimer, D. G., and Marks, M. D. (1994b). “The GL1 gene and the trichome developmental pathway in Arabidopsis thaliana,” in Plant Promoters and Transcription Factors Results and Problems in Cell Differentiation (A Series of Topical Volumes in Developmental Biology), Vol. 20, ed. L. Nover, (Berlin: Springer), 259–275. doi: 10.1007/978-3-540-48037-2_12
Larkin, J. C., Walker, J. D., Bolognesi-Winfield, A. C., Gray, J. C., and Walker, A. R. (1999). Allele-specific interactions between ttg and gl1 during trichome development in Arabidopsis thaliana. Genetics 151, 1591–1604.
Lee, M. M., and Schiefelbein, J. (1999). WEREWOLF, a MYB-related protein in Arabidopsis, is a position-dependent regulator of epidermal cell patterning. Cell 99, 473–483. doi: 10.1016/s0092-8674(00)81536-6
Lee, M. M., and Schiefelbein, J. (2002). Cell pattern in the Arabidopsis root epidermis determined by lateral inhibition with feedback. Plant Cell 14, 611–618. doi: 10.1105/tpc.010434
Livak, K. J., and Schmittgen, T. D. (2001). Analysis of relative gene expression data using real-time quantitative PCR and the 2- ΔΔCT method. Methods 25, 402–408. doi: 10.1006/meth.2001.1262
Masucci, J. D., Rerie, W. G., Foreman, D. R., Zhang, M., Galway, M. E., Marks, M. D., et al. (1996). The homeobox gene GLABRA2 is required for position-dependent cell differentiation in the root epidermis of Arabidopsis thaliana. Development 122, 1253–1260.
Masucci, J. D., and Schiefelbein, J. W. (1994). The rhd6 mutation of Arabidopsis thaliana alters root-hair initiation through an auxin-and ethylene-associated process. Plant Physiol. 106, 1335–1346. doi: 10.1104/pp.106.4.1335
Miller, J. C., Chezem, W. R., and Clay, N. K. (2016). Ternary WD40 repeat-containing protein complexes: evolution, composition and roles in plant immunity. Front. Plant Sci. 6:1108. doi: 10.3389/fpls.2015.01108
Neff, M. M., Turk, E., and Kalishman, M. (2002). Web-based primer design for single nucleotide polymorphism analysis. Trends Genet. 18, 613–615. doi: 10.1016/s0168-9525(02)02820-2
Payne, C. T., Zhang, F., and Lloyd, A. M. (2000). GL3 encodes a bHLH protein that regulates trichome development in Arabidopsis through interaction with GL1 and TTG1. Genetics 156, 1349–1362.
Schellmann, S., Schnittger, A., Kirik, V., Wada, T., Okada, K., Beermann, A., et al. (2002). TRIPTYCHON and CAPRICE mediate lateral inhibition during trichome and root hair patterning in Arabidopsis. EMBO J. 21, 5036–5046. doi: 10.1093/emboj/cdf524
Schiefelbein, J., Huang, L., and Zheng, X. (2014). Regulation of epidermal cell fate in Arabidopsis roots: the importance of multiple feedback loops. Front. Plant Sci. 5:47. doi: 10.3389/fpls.2014.00047
Schiefelbein, J., Kwak, S.-H., Wieckowski, Y., Barron, C., and Bruex, A. (2009). The gene regulatory network for root epidermal cell-type pattern formation in Arabidopsis. J. Exp. Bot. 60, 1515–1521. doi: 10.1093/jxb/ern339
Schiefelbein, J. W., and Somerville, C. (1990). Genetic control of root hair development in Arabidopsis thaliana. Plant Cell 2, 235–243. doi: 10.1105/tpc.2.3.235
Simon, M., Lee, M. M., Lin, Y., Gish, L., and Schiefelbein, J. (2007). Distinct and overlapping roles of single-repeat MYB genes in root epidermal patterning. Dev. Biol. 311, 566–578. doi: 10.1016/j.ydbio.2007.09.001
Song, S.-K., Ryu, K. H., Kang, Y. H., Song, J. H., Cho, Y.-H., Yoo, S.-D., et al. (2011). Cell fate in the Arabidopsis root epidermis is determined by competition between WEREWOLF and CAPRICE. Plant Physiol. 157, 1196–1208. doi: 10.1104/pp.111.185785
Wada, T., Tachibana, T., Shimura, Y., and Okada, K. (1997). Epidermal cell differentiation in Arabidopsis determined by a Myb homolog, CPC. Science 277, 1113–1116.
Walker, A. R., Davison, P. A., Bolognesi-Winfield, A. C., James, C. M., Srinivasan, N., Blundell, T. L., et al. (1999). The TRANSPARENT TESTA GLABRA1 locus, which regulates trichome differentiation and anthocyanin biosynthesis in Arabidopsis, encodes a WD40 repeat protein. Plant Cell 11, 1337–1349. doi: 10.1105/tpc.11.7.1337
Wang, W., Ryu, K. H., Barron, C., and Schiefelbein, J. (2019). Root epidermal cell patterning is modulated by a critical residue in the WEREWOLF transcription factor. Plant Physiol. 181, 1239–1256. doi: 10.1104/pp.19.00458
Xiang, C., Han, P., Lutziger, I., Wang, K., and Oliver, D. J. (1999). A mini binary vector series for plant transformation. Plant Mol. Biol. 40, 711–717.
Yi, K., Menand, B., Bell, E., and Dolan, L. (2010). A basic helix-loop-helix transcription factor controls cell growth and size in root hairs. Nat. Genet. 42, 264–267. doi: 10.1038/ng.529
Keywords: cell differentiation, cell fate, pattern formation, root development, root hair, transcriptional network
Citation: Long Y and Schiefelbein J (2020) Novel TTG1 Mutants Modify Root-Hair Pattern Formation in Arabidopsis. Front. Plant Sci. 11:383. doi: 10.3389/fpls.2020.00383
Received: 14 January 2020; Accepted: 17 March 2020;
Published: 07 April 2020.
Edited by:
Munetaka Sugiyama, The University of Tokyo, JapanReviewed by:
Anthony Bishopp, University of Nottingham, United KingdomHyung-Taeg Cho, Seoul National University, South Korea
Copyright © 2020 Long and Schiefelbein. This is an open-access article distributed under the terms of the Creative Commons Attribution License (CC BY). The use, distribution or reproduction in other forums is permitted, provided the original author(s) and the copyright owner(s) are credited and that the original publication in this journal is cited, in accordance with accepted academic practice. No use, distribution or reproduction is permitted which does not comply with these terms.
*Correspondence: John Schiefelbein, c2NoaWVmZWxAdW1pY2guZWR1