- 1Volpe National Transportation Systems Center, Cambridge, MA, United States
- 2Federal Aviation Administration, Washington, DC, United States
- 3National Institute of Food and Agriculture (USDA), Washington, DC, United States
- 4Bioenergy Technologies Office (DOE), Washington, DC, United States
- 5Washington State University, Pullman, WA, United States
- 6LanzaTech, Skokie, IL, United States
Editorial on the Research Topic
The motivations for and the value proposition of sustainable aviation fuels
Drivers of aviation sector interest in sustainable aviation fuels
All forecasts for aviation expect continued growth in the sector over time. It will take some time for the aviation sector to return to pre-pandemic levels of activity, with some estimates putting this recovery to 2024 and beyond (Airlines for America, 2021a), and this recovery will likely be affected by traveler willingness, workforce availability, and other factors. Nevertheless, the long-term perspective suggests that aviation will continue to grow based on its value proposition to society of safe, efficient, high-speed movement of goods and people. This growth in traffic and locations of service will result in accompanying increases in fuel demand (Fleming & de Lépinay, 2019). Under current practices, this expansion of traffic would lead to increased carbon emissions. However, the aviation sector has made several commitments to addressing carbon-dioxide emissions.
The first of these was the first industrial-sector-wide commitment to carbon-neutral growth, in which increases in aviation activity must be de-coupled from increases in greenhouse gas emissions. Meeting these emissions goals relies on what the International Civil Aviation Organization (ICAO) refers to as a “basket of measures” for reducing carbon emissions associated with aviation (ICAO Secretariat, 2019), including improved operations, new technology, alternative fuels, and other market-based measures (see Figure 1). The ICAO Carbon Offsetting and Reduction Scheme for International Aviation (CORSIA) caps carbon emissions from international aviation at 2019 levels out to 2035. Airline operators of participating countries must report their emissions each year and, if emissions exceed the baseline, offset those emissions (ICAO, 2021a). The emissions obligation associated with fuel burn can be reduced by replacing standard petroleum-based jet fuel with low carbon CORSIA-eligible fuel, including SAF or fossil-based “lower carbon aviation fuels.” The aviation sector is currently in the pilot phase of CORSIA, which is expected to continue to drive interest in carbon-beneficial alternatives to standard petroleum-based jet fuel.
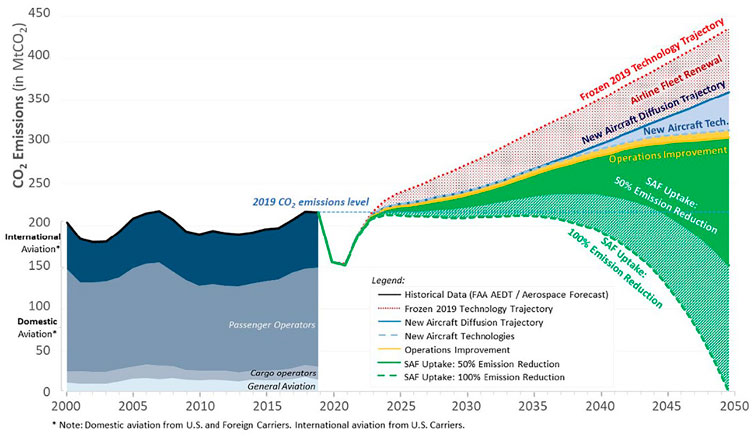
FIGURE 1. Projection of future annual emissions of aviation out to 2050 with and without the application of the “basket of measures” of operational and technological advancements and SAF deployment. Without SAF, the aviation sector will not meet the net-zero emissions goals they have set. Source (United States, 2021) used with permission.
Additionally, in March 2021, Airlines for America (A4A), a trade association of U.S. airlines, announced that all its members have committed to net zero carbon emissions by 2050, a commitment that can be achieved with the successful deployment of the full basket of measures (Airlines for America, 2021b). At the 77th Annual General Meeting of the International Air Transport Association (IATA) in Boston, United States, on 4 October 2021, a resolution was passed by IATA member airlines committing them to achieving net-zero carbon emissions from their operations by 2050. This pledge brings air transport in line with the objectives of the Paris agreement to limit global warming to 1.5°C. In November 2021, the U.S. federal government released the 2021 Aviation Climate Action Plan (United States, 2021) committing to net zero carbon emissions for the U.S. aviation sector by 2050 and detailing the strategy to achieve this goal. In the U.S., the White House’s SAF Grand Challenge is a new effort to facilitate the deployment of SAF, setting U.S. SAF production goals of three billion gallons by 2030 and 100% of U.S. aviation fuel need (or 35 billion gallons) by 2050. A federal agency roadmapping effort is underway to identify key governmental actions to facilitate coordinated support for industry-led SAF production expansion (U.S. White House, 2021).
The aviation sector has made tremendous strides in technology and operational efficiency since the inception of jet-powered air travel, increasing fuel efficiency of aircraft by 85% since the 1950s and operational efficiency by 55% (Air Transport Action Group, 2020). Recent efforts to integrate real-time satellite and geospatial information into flight routing and operational controls in the U.S. through the NextGen program has led to improvements of 17%, reduction in fuel burn and 21% reductions in aircraft operating costs (FAA, 2021). Many of the easiest efficiency gains may have already been made, and future improvements to operations and infrastructure will be more challenging. ICAO estimates that even in an optimistic scenario, fuel burn improvements from technology and operations will be 0.98 and 0.39%, respectively per annum out to 2050 (Fleming & de Lépinay, 2019). Therefore, to achieve greater decarbonization, other measures from the basket will be needed. The expanded use of SAF is one lever the aviation sector can use to start decarbonization immediately and will be an important contributor in the future (Jain et al.).
The pursuit of drop-in sustainable aviation fuels
The unique power requirements for flight and longevity of the commercial fleets, as well as the global infrastructure compatibility requirements of aircraft and airports means that drop-in, low carbon fuel that can leverage the existing global aircraft fleet and fueling system infrastructure to improve environmental performance, while maintaining energy security and price certainty, can facilitate near-term and lowest cost decarbonization of aviation. Drop-in SAFs have essentially identical composition, performance, and safety to existing petroleum-based kerosene fuels and are fungible with existing fuels and in existing fueling systems.
Fuel suitability and safety is assured through detailed production specifications, handling procedures, and certification testing protocols established by the aviation industry. These specifications and operating practices are published by specification bodies such as ASTM International and recognized by national regulatory authorities as well as original equipment manufacturers and academic institutions (Rumizen).
Until 2009, jet fuel used for turbine powered aircraft was produced from petroleum. Furthermore, aircraft and other equipment are certified for use with petroleum-based jet fuel, so to avoid recertifying all equipment, the alternative fuels must be considered interchangeable with standard jet fuel. The commonly used specification of conventional aviation turbine fuel is ASTM D1655 (Standard Specification for Aviation Turbine Fuels (ASTM International, 2020)) although there are similar, equivalent standards used internationally (e.g., DEF STAN 91-091 (MODUK, 2020)). Alternative fuels (non-petroleum origination) with comparable properties that have been approved for use are defined in a specification under the ASTM D7566. If testing demonstrates that a fuel has the required physical and fit-for-purpose characteristics to be considered usable as jet fuel, the specification body will issue an annex to ASTM D7566 that outlines the characteristics of the novel fuel type including feedstock, conversion process parameters, and fuel characteristics. Once a fuel is qualified under D7566 and blended at specified levels with petroleum-based jet fuel, it is redesignated as jet fuel under D1655. This fungibility is a critical aspect of the ASTM qualification that allows new fuels to be brought into the marketplace without the recertification of equipment and aircraft. Further information about the specification of synthetic aviation fuels can be found in (Rumizen). Drop-in, synthesized fuel production also has the potential to meet environmental, social, and economic sustainability criteria (such as those required by voluntary and/or regulatory frameworks, as described in Section 5), resulting in SAF.
Short overview of the range of fuels available
There are many ways to make alternative fuels from resources other than petroleum. Most of these processes produce, not only alternative jet fuel (C7 to C17 hydrocarbons), but also renewable diesel (C12 hydrocarbons and above) and naphtha or reformate blending components for gasoline (C12 and below). First-generation biofuels such as ethanol and biodiesel still contain oxygen from the original biomass, whereas advanced alternative fuels are strictly hydrocarbons and therefore chemically like the petroleum counterparts they seek to replace.
Biomass can be converted into fuels using thermochemical or biochemical processes, or combinations of the two. The thermochemical processes utilize heat, pressure, catalysts, and a reactor to decompose the biomass to varying degrees (e.g., gasification, pyrolysis, or hydrothermal liquefaction), and then recompose the constituent molecules (syngas or bio-oil intermediates) into pure hydrocarbons in the jet fuel range. In contrast, biochemical processes use microbial processing (e.g., enzymatic hydrolysis, fermentation, anaerobic digestion) to produce a primarily hydrocarbon intermediate. In either process, the liquid hydrocarbon intermediate is catalytically converted to hydrocarbons which may require subsequent upgrading steps to produce fuel distillates (Huber, Iborra, & Corma, 2006). Wastes, such as used cooking oil, and purpose-grown lipids can be directly upgraded to hydrocarbons using commercially available hydro-treatment and upgrading processes. The most cost-effective sources of these lipids include waste fats, oils, and greases (FOGs); however, vegetable oils from corn or oil-seed crops, and even oil derived from algae can be converted into SAF using this same technology.
As of August 2022, seven alternative aviation fuel production pathways were defined in specifications under Annexes of ASTM D7566-20c (CAAFI, 2021a; ASTM International, 2021). Furthermore, an annex has been added to D1655 to allow for the coprocessing of either fatty acids, fatty acid esters, or Fischer-Tropsch (FT) biocrude within a traditional petroleum refinery at up to 5% by volume.
As of this time, additional fuel pathways to produce aromatics and more diversified fuel molecules are also undergoing evaluation and testing in the ASTM specification development process (CAAFI, 2021b). These include pyrolysis, hydrothermal liquefaction and additional alcohol-to-jet pathways, and biomass-based pathways similar to those already included in D7566. Task force groups are in place to progress these fuels through the ASTM specification development and approval process. Additional research is being performed to better understand both the potential for conversion pathway optimization and the chemical and combustion characteristics of the products of various pathways (e.g., (Boehm et al.; Landera et al.; Mehl et al.; Moore et al.) and to effectively leverage these data and make them available to facilitate ASTM prescreening and fuel testing (Blakey et al.). An additional Task Force is currently active at ASTM to define how to enable higher blend levels up to 100% SAF to be used in aircraft, including what technical and performance characteristics are needed and to what extent backward compatibility with existing aircraft will be required (Kramer et al.).
Additional SAF production pathways that have received significant attention and potential investment, particularly in Europe, include “power-to-liquids” or “e-fuel” technologies. These approaches utilize renewable electricity to power electrocatalysis processes to produce a liquid intermediate which is subsequently converted into a hydrocarbon. One common approach is to electrolyze water to produce renewable hydrogen and convert waste or atmospheric carbon dioxide (CO2) into syngas (carbon monoxide + hydrogen gas). The syngas can then be converted to hydrocarbons using the FT process or to alcohols to be used in an alcohol-to-jet process (Schmidt, Weindorf, Roth, Batteiger, & Riegel, 2016). However, the supply of these fuels is dependent on a significant ramp up in production and availability of low cost, low carbon renewable energy (Holladay, Male, Rousseau, & Weber, 2020; Male et al.) to achieve the targeted very low fuel carbon intensities. These e-fuels, outside of those that might be produced via FT conversion of syngas, are not yet being evaluated as part of the ASTM qualification process.
There is currently no mechanism for incorporating non-drop-in fuels into the aviation sector by ASTM or other specification processes. Hydrogen fuel cells or direct combustion of hydrogen are possible long-term options for aviation but would require significant redesign of aircraft, engines, ground equipment, airports, fueling infrastructure, and safety procedures to be accommodated. The Air Transport Action Group (ATAG) estimates that hydrogen-fuel cell regional aircraft could enter the market in 2030 and that some hydrogen-combustion powered short haul flights could happen by the 2040 timeframe, but medium- and long-haul flights are unlikely to be powered by hydrogen until 2050 or beyond (Air Transport Action Group, 2020). On the other hand, renewable hydrogen could be immediately used to significantly reduce the life-cycle carbon footprint of alternative fuels if used in place of fossil-based hydrogen in SAF production processes. In the near term, this may be the best use for hydrogen in aviation.
Another alternative energy source that has received significant recent attention for aviation is electrification. However, electrification is challenging for aviation due to the size and weight of batteries, as even the best lithium-ion batteries have a lower mass-specific energy density by a factor of 60 compared to standard kerosene (Hepperle, 2012). It is currently anticipated that only small aircraft and flights under 200 nautical miles would be feasible with current technology, and future envisioned technologies necessary for extending range and passengers.
Sustainability is a key value proposition for SAF
ASTM specifications are focused on the physical and fit-for-performance characteristics of turbine fuels, but do not provide any evaluation of environmental, social, or economic impacts. However, the drivers outlined above have motivated airlines and other end users to see sustainability as a key component of the value proposition of non-petroleum aviation fuels. Given their cost, many of these fuels would not be competitive with standard petroleum-based jet fuel without the added value of verifiable environmental and social outcomes.
Three key issues previously raised regarding first generation biofuels continue to challenge advanced biofuels options: 1) concerns about induced land use change in which production of feedstocks for biofuels leads to displacement of another crop, followed by conversion of land from forest or other natural, carbon sequestering systems to agriculture or forestry to compensate, 2) potential impacts on food prices associated with reallocation of land to alternative fuels and 3) resource availability or how much SAF can be produced given sustainability constraints. Airline fuel purchasers are sensitive to these concerns and seek assurance that the SAF they purchase will not lead to sustainability issues. On the other hand, sustainable aviation fuel production—including from biomass—has the potential to contribute positively to economic and social sustainability outcomes such as rural economic development, creation of skilled jobs, and energy security.
Therefore, to be successful, SAF must:
1) Be economically viable.
2) Avoid environmental damage and/or improve environmental sustainability.
3) Meet social sustainability goals (e.g., land-use change, food security, local economic sustainability, energy security etc.).
Existing regulatory schemes that provide requirements and incentives for renewable/low carbon fuels, such as the European Union’s Renewable Energy Directive (EU RED), the U.S. Renewable Fuel Standard (RFS2), and California Low Carbon Fuel Standard (CA LCFS), include criteria for one or more of these sustainability pillars. Under the E.U. RED fuels are certified by voluntary and national certification schemes to meet the requirements for carbon reduction, reducing carbon stock depletion, and avoiding highly biodiverse lands. Other sustainability criteria may also be included in the future (European Commission, 2020). Under RFS as defined in the Energy Independence and Security Act (U.S. Public Law 110-140, 2007), fuels that are produced according to a process defined and accepted by the Environmental Protection Agency (EPA) are assigned a carbon reduction level based on their category. EPA is responsible for determining fuel volume requirements under RFS based on impacts on “air quality, climate change, conversion of wetlands, ecosystems, wildlife habitat, water quality, and water supply,” as well as “job creation, the price and supply of agricultural commodities, rural economic development, and food prices.” EPA is responsible for periodically assessing the impact of the program on “soil conservation, water availability, and ecosystem health and biodiversity, including impacts on forests, grasslands, and wetlands,” “hypoxia, pesticides, sediment, nutrient and pathogen levels in waters, acreage and function of waters, and soil environmental quality” as well as potential “growth and use of cultivated invasive or noxious plants.” Producers have to meet a limited number of specific criteria regarding their pathway definition and restriction of land use conversion after 19 December 2007.
In the context of ICAO, SAF are a “renewable or waste-derived aviation fuel that meets the CORSIA Sustainability Criteria under [Annex 16, Volume IV of the Convention on International Civil Aviation]” (ICAO, 2018). In the pilot phase (2021-2023) there are only two themes and three criteria approved that define SAF: lifecycle greenhouse gas emissions (including a 10% reduction threshold) and carbon stock (safeguarding high carbon stock lands and providing requirements for land conversion after a threshold date of 1 January 2008) (ICAO, 2021b). An expanded set of sustainability criteria will be used under CORSIA during the “Voluntary” phase from 1 January 2024 to 31 December 2026 (ICAO Council, 2021). These criteria include environmental, social, and economic indicators and principles addressing greenhouse gases, carbon stock/land use change, water, soil, air, conservation, wastes and chemicals, human and labor rights, land use rights and land use, water use rights, local and social development, and food security (ICAO, 2021c). The expanded CORSIA Sustainability Criteria were developed by drawing upon existing voluntary sustainability certification scheme themes, principles, and criteria. ICAO relies on approved sustainability certification schemes to execute the certification of SAF under CORSIA (ICAO, 2020).
The interest in SAF from airlines and other fuel purchasers (e.g., business aviation, air framers) as well as existing and emerging regulatory requirements and mandates indicates that sustainability improvements will continue to be a critical component of the value proposition of alternative aviation fuels.
Tools for evaluating SAF and SAF supply chains—Reducing costs and enabling supply
While technical opportunities remain and new pathways are constantly emerging, the key challenges for commercial scale SAF deployment include cost, supply chain development and risk management, and demonstration of sustainability.
While selling prices for produced SAF are not disclosed, SAF currently appears to cost more than conventional petroleum-based jet fuel. Absorbing this cost differential is a significant challenge for airlines seeking to reduce their carbon footprint, as many airlines are unwilling or unable to pay extra for SAF given their existing expenses and competitive environment. However, while the energy content of SAF is completely fungible with conventional fuels, the environmental services of carbon reduction overcome problems from its conventional counterpart and must be considered as part of its value.
Technoeconomic analysis (TEA) can be a useful tool to evaluate the economic factors affecting fuel selling price and identify opportunities to reduce costs within the supply chain. To compare among fuel production pathways, consistent TEAs for a range of options are extremely valuable. The U.S. Dept of Energy has developed one set of biofuel TEAs that focus on potential to drive down costs based on DOE targets (Kinchin, 2020). Another set of harmonized TEAs for the seven certified SAF pathways have been developed with consistent approaches to economic, finance, feedstock preparation, and support system (Brandt et al.). These models have been used to evaluate the influence of federal and state policies that evaluate environmental services provided by SAF. For real world costs as currently understood, researchers are implementing stochastic and deterministic TEA analysis assuming various policies and technology maturation rates to aid in thinking about uncertain future conditions (Tanzil, et al.; Trejo-Pech et al.). Other researchers are analyzing the influence of technoeconomics, technology maturation rates, and policies on deployment potential for SAF and other fuels (Newes, Han, & Peterson, 2017; Lewis, et al., 2018).
Life cycle analysis (LCA) of greenhouse gas emissions is critical for ensuring that the SAF that is commercially produced are carbon-beneficial. Highly rigorous and reviewed tools such as the Argonne National Laboratory’s GREET model (ANL, 2021) provide reliable and transparent GHG accounting that demonstrates emissions reductions for SAF. ICAO publishes a standard set of GHG LCA values for SAF used under CORSIA (ICAO, 2021d) as well as a standardized methodology for calculating core GHG LCA values for SAF (ICAO, 2021a). The California Air Resources Board similarly has a set of default carbon intensities published for fuels under the Low Carbon Fuel Standard (CARB, 2022).
Because new supply chains must be established to support SAF deployment, risk management is a critical issue for supply chain participants and investors. The Commercial Aviation Alternative Fuels Initiative’s Feedstock Readiness Level, Fuel Readiness Level, and Environmental Progression frameworks provide consistent scoring and communication approach and clearly identify stages of development for feedstocks and fuels (CAAFI, 2021a) to facilitate communication about technical performance of SAF.
Understanding potential and probable availability of SAF feedstock is another critical aspect of supply chain development that requires detailed analyses of different potential species or feedstock opportunities by location and potential economic performance (e.g., Bach et al.; Kubic et al.; Sharma et al.; Trejo-Pech et al.; Field et al.; Tumuluru, et al.). SAF feedstock performance must also be characterized to ensure that both feedstocks and preparatory processes are suitable for fuel production (Bach et al.; Tumuluru, et al.). Waste feedstocks (e.g., municipal solid waste, waste fats, oils, and greases, wet wastes, or waste gases from industrial processes) are often less expensive than dedicated energy crops and considered advantageous for SAF production. Cover crops are also seen as a potentially beneficial feedstock type, with the potential to be integrated into existing crop rotations and provide benefits in the form of reduced erosion and disruption of pathogen and pest cycles and reduced land use demand (Taheripour et al.; Field et al.). Integrated analyses of potential supply chains based on agricultural and forestry products have been established through the US Dept. of Agriculture’s Coordinated Agriculture projects, which focus on convening regional stakeholders to “facilitate the development of regionally-based industries producing advanced biofuels, industrial chemicals, and other biobased products,” (National Institute of Food and Agriculture, 2021). SAF-relevant projects include the Southeast Partnership for Advanced Renewables from Carinata (Field et al.), the Integrated Pennycress Research Enabling Farm and Energy Resilience (IPREFER) project (Phippen et al.) and eight other projects so far.
Other tools have been developed to identify supply chain development and deployment opportunities, including tools for siting biorefineries based on geographic variation in capital and operational costs and resource availability (e.g., (English et al.) and how geography and transportation scenarios and disruptions influence supply chain performance (Lewis, et al., 2018). Social criteria, including social capital and cultural capital, should be considered when identifying the potential suitability of biorefinery candidate locations (Anderson et al.). These criteria should be assessed through mixed methods approaches, especially ongoing outcomes of biofuel development, as quantitative assessment does not adequately measure many of these impacts, especially at a more local level (Anderson et al.).
Policy can be a critical enabler for the development of new industries and the establishment of supply chains Nevertheless, for sound policy to be developed and implemented, it is crucial to develop an understanding of how past policies have worked and their impact on this and similar industries. This issue contains several papers that provide insight into the benefits and hurdles posed by various alternative fuel- and SAF-related policies (Korkut and Fowler; Brandt et al.; Taheripour et al.; Wang, et al.).
Given the key role of sustainability in the value proposition of SAF, reliable and consistent ways to measure the environmental, social, and economic outcomes of SAF are needed to build confidence in the marketplace. Compliance with CORSIA sustainability criteria is one definition of sustainability (ICAO, 2021b). Only three sustainability criteria are currently required for SAF under CORSIA, which most airlines and stakeholders would deem incomplete; however, as indicated above, ICAO has established additional sustainability criteria for the post-pilot phase. Whether under CORSIA or separately, voluntary certification by an independent sustainability certification scheme (e.g,. RSB, ISCC) can provide assurance of environmental, social, and economic sustainability. Compliance with regulatory schemes such as the U.S. Renewable Fuel Standard, California’s Low Carbon Fuel Standard, and others can also provide both sustainability assurance and economic benefits (e.g., sellable Renewable Identification Numbers). Enhancement of ecosystem services are another way to demonstrate environmental benefits and potentially add revenue for feedstock producers (Gasparatos, et al., 2018; Brandt et al.).
This special issue in Frontiers in Energy Research
Sustainable aviation fuels are a key component of the basket of measures being pursued by the aviation sector to meet environmental, social, and economic goals, particularly to address climate change. A broad array of fuel production pathways are currently in development that need to be assessed for their technical production, performance, supply chain viability, and sustainability. Stakeholders from across the aviation and alternative fuels sectors, including government, academic, and private entities, have been researching, developing, and deploying a wide range of potential alternative jet fuel options, and along the way have also developed tools, data, and models to help with alternative jet fuel assessment and supply chain development. In this special topic, the contributors highlight some of the key research tools, models, and outcomes to provide a better overall understanding of the current state of play for alternative jet fuels. We have highlighted these papers in the preceding sections. Each paper provides an important perspective on the technologies and feedstocks to produce SAF, development of specifications to ensure safety and performance of SAF, the development and deployment of SAF supply chains, and sustainability. Future directions for SAF research include reducing the cost of SAF production via innovative biochemical, thermochemical, and hybrid approaches, the development of a specification for high blend levels/100% synthetic fuels that would enable simplified logistics and greater deployment, and enhanced understanding of the non-CO2 impacts of aviation (e.g., contrails) and the potential for SAF to address these issues.
Author contributions
The authors served as co-editors of the Research Topic on Sustainable Aviation Fuels, led by MW. KL led the drafting of the paper, and all authors contributed to the development and revision of the paper to reflect the state of SAF development and deployment.
Funding
FAA supported this work by KL under contract 693KA9-20-N-00013, and by MW and SH through the ASCENT Center of Excellence under FAA Award #13-C-AJFE-WaSU-013. Any opinions, findings, conclusions, or recommendations expressed in this material are those of the authors and do not necessarily reflect the views of the FAA nor other agencies of the U.S. Government.
Acknowledgments
The authors thank S. Csonka of CAAFI for reviewing this paper before submission.
Conflict of interest
The authors declare that the research was conducted in the absence of any commercial or financial relationships that could be construed as a potential conflict of interest.
Publisher’s note
All claims expressed in this article are solely those of the authors and do not necessarily represent those of their affiliated organizations, or those of the publisher, the editors and the reviewers. Any product that may be evaluated in this article, or claim that may be made by its manufacturer, is not guaranteed or endorsed by the publisher.
References
Air Transport Action Group (2020). Waypoint 2050. Available at: https://aviationbenefits.org/media/167187/w2050_full.pdf.
Airlines for America (2021b). Letter to secretary-designate buttigieg, january 19, 2021. Washington, DC: Bloomberg News. Available at: https://www.bloomberg.com/news/articles/2021-01-19/wave-of-senate-confirmation-hearings-begins-inaugural-update (January 20, 2021).
Airlines for America (2021a). A4A climate change commitment and flight path: Innovative industry and government action to achieve net zero carbon emissions. Washington, DC, USA. Available at: https://www.airlines.org/wp-content/uploads/2021/03/A4A-Climate-Change-Commitment-Flight-Path-to-Net-Zero-FINAL-3-30-21.pdf.
ANL (2021). GREET model. Argonne, IL. Available at: https://greet.es.anl.gov/index.php (April 29, 2022).
ASTM International (2020). D1655-20d: Standard specification for aviation turbine fuels. West Conshohocken, PA, USA: ASTM International.
ASTM International (2021). D7566-20c: Standard specification for aviation turbine fuel containing synthesized hydrocarbons. West Conshohocken, PA, USA. doi:10.1520/D7566-20C
CAAFI (2021a). Commercial aviation alternative fuels initiative. Path to Alternative Jet Fuel Readiness:Available at: https://www.caafi.org/tools/Path_to_Alternative_Jet_Fuel_Readiness.html.
CAAFI (2021b). Current fuels in the D4054 qualification process. Available at: https://www.caafi.org/focus_areas/fuel_qualification.html#qualification (April 1, 2021).
CARB (2022). LCFS pathway certified carbon intensities. State of CA. Available at: https://ww2.arb.ca.gov/resources/documents/lcfs-pathway-certified-carbon-intensities (April 29, 2022).
English, B. C., Menard, R. J., and Wilson, B. (2022). The economic impact of a renewable biofuels/energy industry supply chain using the renewable energy economic analysis layers modeling system. Front. Energy Res. 10. doi:10.3389/fenrg.2022.780795
European Commission (2020). Voluntary schemes. Available at: https://ec.europa.eu/energy/topics/renewable-energy/biofuels/voluntary-schemes_en (April 05, 2021).
FAA (2021). NextGen benefits. from Federal Aviation Administration:,Available at: https://www.faa.gov/nextgen/benefits/ (July 20, 2022).
Fleming, G. G., and de Lépinay, I. (2019). “Environmental trends in aviation to 2050,” in Environmental report. Editor I. C. Organization (Montreal, Canada: International Civil Aviation Organization).
Gasparatos, A., Romeu-Dalmau, C., von Maltitz, G., Johnson, F. X., Jumbe, C. B., Stromberg, P., et al. (2018). Using an ecosystem services perspective to assess biofuel sustainability. Biomass Bioenergy. doi:10.1016/j.biombioe.2018.01.025
Hepperle, M. (2012). Electric flight – potential and limitations. AVT-209 workshop on energy efficient technologies and concepts operation. Lisbon, Portugal. (pp. STO-MP-AVT-209) Available at: https://www.mh-aerotools.de/company/paper_14/MP-AVT-209-09.pdf.
Holladay, J. E., Male, J. L., Rousseau, R., and Weber, R. S. (2020). Synthesizing clean transportation fuels from CO2 will at least quintuple the demand for non-carbogenic electricity in the United States. Energy fuels. 34, 15433–15442. doi:10.1021/acs.energyfuels.0c02595
Huber, G. W., Iborra, S., and Corma, A. (2006). Synthesis of transportation fuels from biomass: Chemistry, catalysts, and engineering. Chem. Rev. 106, 4044–4098. doi:10.1021/cr068360d
ICAO (2018). Annex 16 to the convention on international Civil aviation: Environmental protection - volume IV. Carbon offsetting and reduction scheme for international aviation (CORSIA). Montreal, ontario, Canada. Available at: https://elibrary.icao.int/home/product-details/229739.
ICAO (2021c). Carbon offsetting and reduction scheme for international aviation (CORSIA). Montreal, quebec, Canada. Available at: https://www.icao.int/environmental-protection/CORSIA/Pages/default.aspx.
ICAO (2020). CORSIA approved sustainability certification schemes. Montreal, Ontario, Canada: ICAO. Available at: https://www.icao.int/environmental-protection/CORSIA/Documents/ICAO%20document%2004%20-%20Approved%20SCSs.pdf.
ICAO (2021d). CORSIA sustainability criteria for CORSIA eligible fuels. Montreal, ON, Canada. Available at: https://www.icao.int/environmental-protection/CORSIA/Documents/ICAO%20document%2005%20-%20Sustainability%20Criteria%20-%20November%202021.pdf.
ICAO Council (2021). Council 222nd session, twelfth meeting summary of decisions. C-DEC 222/12. Montreal, Canada: ICAO. Available at: https://www.icao.int/about-icao/Council/Council%20Documentation/222/C-DECs/C.222.DEC.12.EN.pdf.
ICAO Secretariat (2019). “Introduction to the ICAO basket of measures to mitigate climate change,” in ICAO, ICAO environmental report 2019 (Montreal: ICAO), 111–115. Available at: https://www.icao.int/environmental-protection/Documents/EnvironmentalReports/2019/ENVReport2019_pg111-115.pdf.
ICAO (2021a). CORSIA default life cycle emissions values for CORSIA eligible fuels. Montreal, ON, Canada. Available at: https://www.icao.int/environmental-protection/CORSIA/Pages/CORSIA-Eligible-Fuels.aspx.
ICAO (2021b). CORSIA methodology for calculating actual life cycle emissions values. Montreal, ON, Canada. Available at: https://www.icao.int/environmental-protection/CORSIA/Pages/CORSIA-Eligible-Fuels.aspx.
Kinchin, C. (2020). BETO biofuels TEA database. Washington, DC: Dept. of Energy. Available at: https://bioenergykdf.net/content/beto-biofuels-tea-database (April 29, 2022).
Lewis, K. C., Newes, E. K., Peterson, S. O., Pearlson, M. N., Lawless, E. A., Brandt, K., et al. (2018). December 7) US alternative jet fuel deployment scenario analyses identifying key drivers and geospatial patterns for the first billion gallons. BioFPR. doi:10.1002/bbb.1951
National Institute of Food and Agriculture (2021). AFRI Regional Bioenergy System Coordinated Agricultural Projects. Washington, DC: US Department of Agriculture. Available at: https://nifa.usda.gov/afri-regional-bioenergy-system-coordinated-agricultural-projects.
Newes, E., Han, J., and Peterson, S. (2017). “NREL/TP-6A20-67482: Potential avenues for significant biofuels penetration in the U.S. Aviation market,” in Golden, CO: Nrel. Available at: https://www.nrel.gov/docs/fy17osti/67482.pdf.
Schmidt, P., Weindorf, W., Roth, A., Batteiger, V., and Riegel, F. (2016). Background/september 2016 - power-to-liquids: Potentials and perspectives for the future supply of renewable aviation fuel. Munich: German Environment Agency.
United States (2021). United States 2021 aviation climate action plan. Washington, D.C.: United States Government. Available at: https://www.faa.gov/sites/faa.gov/files/2021-11/Aviation_Climate_Action_Plan.pdf.
U.S. Public Law 110-140 (2007). Energy independence and security Act of 2007. USA. Available at: https://www.govinfo.gov/content/pkg/BILLS-110hr6enr/pdf/BILLS-110hr6enr.pdf.
White House, U. S. (2021). Fact sheet: Biden administration advances the future of sustainable fuels in American aviation. Washington, DC, USA. Available at: https://www.whitehouse.gov/briefing-room/statements-releases/2021/09/09/fact-sheet-biden-administration-advances-the-future-of-sustainable-fuels-in-american-aviation/ (December 07, 2021).
Keywords: decarbonization, drop-in fuel, sustainable aviation fuel, SAF, sustainability, aviation, carbon-neutral aviation
Citation: Lewis KC, Brown NL, Goldner WR, Haq Z, Hoard S, Holladay JE and Wolcott MP (2022) Editorial: The motivations for and the value proposition of sustainable aviation fuels. Front. Energy Res. 10:1005493. doi: 10.3389/fenrg.2022.1005493
Received: 28 July 2022; Accepted: 05 September 2022;
Published: 26 September 2022.
Edited by:
Haiping Yang, Huazhong University of Science and Technology, ChinaReviewed by:
Fernano Israel Gómez-Castro, University of Guanajuato, MexicoLuis Cortez, State University of Campinas, Brazil
Copyright © 2022 Lewis, Brown, Goldner, Haq, Hoard, Holladay and Wolcott. This is an open-access article distributed under the terms of the Creative Commons Attribution License (CC BY). The use, distribution or reproduction in other forums is permitted, provided the original author(s) and the copyright owner(s) are credited and that the original publication in this journal is cited, in accordance with accepted academic practice. No use, distribution or reproduction is permitted which does not comply with these terms.
*Correspondence: Kristin C. Lewis, a3Jpc3Rpbi5sZXdpc0Bkb3QuZ292