- 1 Department of Plant Biology, Complex Carbohydrate Research, The University of Georgia, Athens, GA, USA
- 2 Complex Carbohydrate Research Center, The University of Georgia, Athens, GA, USA
There is compelling evidence showing that the structurally complex pectic polysaccharide rhamnogalacturonan II (RG-II) exists in the primary cell wall as a borate cross-linked dimer and that this dimer is required for the assembly of a functional wall and for normal plant growth and development. The results of several studies have also established that RG-II structure and cross-linking is conserved in vascular plants and that RG-II likely appeared early in the evolution of land plants. Two features that distinguish RG-II from other plant polysaccharides are that RG-II is composed of 13 different glycoses linked to each other by up to 22 different glycosidic linkages and that RG-II is the only polysaccharide known to contain both apiose and aceric acid. Thus, one key event in land plant evolution was the emergence of genes encoding nucleotide sugar biosynthetic enzymes that generate the activated forms of apiose and aceric acid required for RG-II synthesis. Many of the genes involved in the generation of the nucleotide sugars used for RG-II synthesis have been functionally characterized. By contrast, only one glycosyltransferase involved in the assembly of RG-II has been identified. Here we provide an overview of the formation of the activated sugars required for RG-II synthesis and point to the possible cellular and metabolic processes that could be involved in assembling and controlling the formation of a borate cross-linked RG-II molecule. We discuss how nucleotide sugar synthesis is compartmentalized and how this may control the flux of precursors to facilitate and regulate the formation of RG-II.
Introduction
The pectic matrix of the plant primary cell wall is comprised of at least three polysaccharide domains, homogalacturonan, rhamnogalacturonan I, and rhamnogalacturonan II (RG-II), that are believed to be covalently linked to one another (Caffall and Mohnen, 2009). Rhamnogalacturonan II is a low-molecular-weight (see Figure 1A), structurally complex pectic polysaccharide that is released when plant cell walls are treated with endo-α-(1,4)-polygalacturonase (Darvill et al., 1978). RG-II contains 13 different monosaccharides (Darvill et al., 1978; Spellman et al., 1983a; York et al., 1985; Stevenson et al., 1988a) and has a backbone composed of 1,4-linked α-D-GalpA residues (Puvanesarajah et al., 1991; see Figure 1A). A series of structural studies (Spellman et al., 1983b; York et al., 1985; Melton et al., 1986; Stevenson et al., 1988b; Thomas et al., 1989; Puvanesarajah et al., 1991; Whitcombe et al., 1995; Pellerin et al., 1996; Vidal et al., 2000; Glushka et al., 2003) established that four different oligosaccharide side chains (A–D, see Figure 1A) are linked to this backbone, although their order along the backbone is not known with certainty (du Penhoat et al., 1999; Glushka et al., 2003; Pérez et al., 2003). RG-II has also been isolated from the cells walls of gymnosperms (Edashige and Ishii, 1998; Shimokawa et al., 1999), lycopodiophytes, and pteridophytes (Matsunaga et al., 2004). Thus, RG-II is likely present in the walls of all vascular plants. Nevertheless, RG-II remained an enigmatic component of the cell wall with no known function for almost 20 years after its discovery.
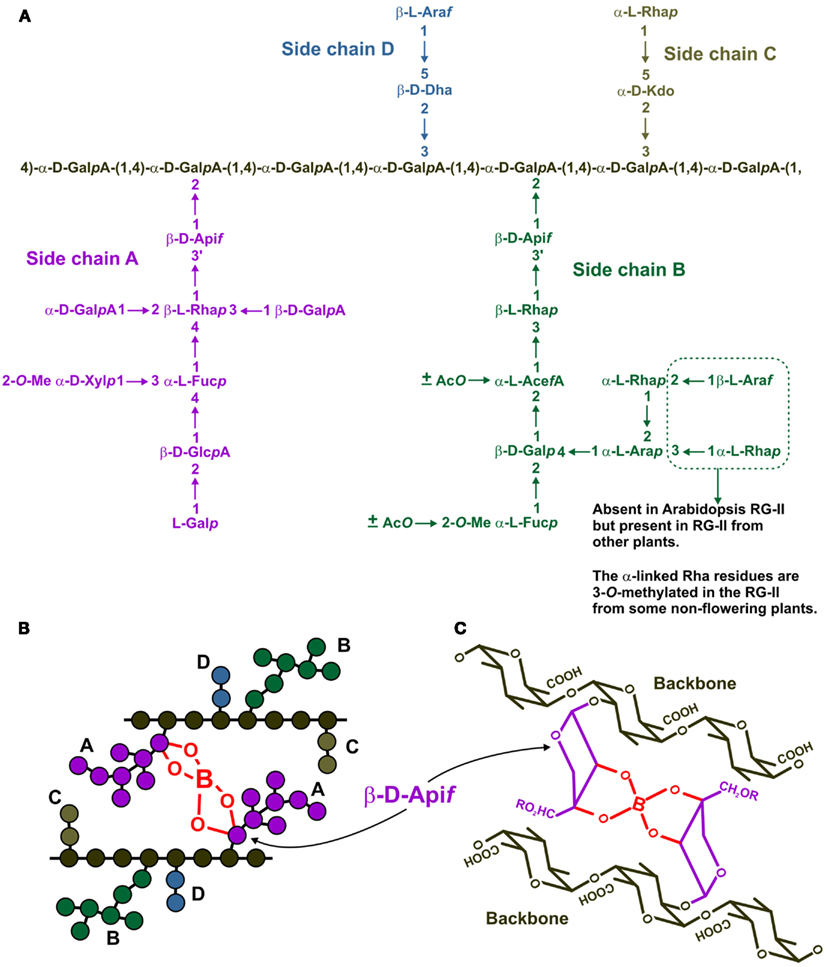
Figure 1. Rhamnogalacturonan II. (A) The glycosyl sequence of RG-II. The nominal mass for the RG-II monomer is 4,612, assuming a backbone of 7 GalA residues and fully decorated side chains. (B) Cartoon showing the cross-linking of two RG-II molecules. (C) The borate diester cross-link. When two apiosyl residues are linked by a borate diester, the boron atom is chiral, and two diastereoisomers (bis[3-C-(hydroxymethyl)-β-L threo-furanoside]-(R)-2,3:2′,3′ borate) and (bis[3-C-(hydroxymethyl)-β-L- threo-furanoside]-(S)-2,3:2′,3′ borate may form (O’Neill et al., 2004). It is not known if one or both diastereoisomers are formed when RG-II is cross-linked in the cell wall.
The results of several studies had led to the suggestion that an interaction between borate and pectin is important for wall structure and for plant growth and development (Matoh et al., 1993; Hu and Brown, 1994; Hu et al., 1996). The first clues that this interaction involved RG-II began to emerge when it was shown that the amounts of RG-II present in the cell wall are correlated with the boron requirements of flowering plants (Matoh et al., 1996) and that two RG-II molecules are cross-linked to one another by a borate diester (Kobayashi et al., 1996; see Figures 1B,C). This diester bond is located between the apiosyl residue of side chain A in two separate RG-II molecules (Ishii et al., 1999; see Figures 1B,C). Together the studies of borate cross-linking of RG-II in vitro (O’Neill et al., 1996, 2001; Fleischer et al., 1999; Ishii et al., 1999; Kobayashi et al., 1999) led to the hypothesis that borate diester formation and stability in vivo are likely influenced by the primary structure of RG-II, wall pH, and calcium ions.
At least 90% of the RG-II in the primary cell wall is cross-linked when plants are grown with an adequate supply of borate (Kobayashi et al., 1996; O’Neill et al., 1996; Matoh et al., 2000). By contrast, virtually none of the RG-II is cross-linked when suspension-cultured Chenopodium album cells are adapted to grow in the absence of added borate (Fleischer et al., 1999). Moreover, the walls of these cells have pore sizes that are ∼60% larger than their borate-grown counterparts. Pore size and RG-II cross-linking return to near-normal values when the cells are supplied with borate suggesting that RG-II cross-linking affects the size of molecules that can diffuse through the wall (Fleischer et al., 1999). The lack of RG-II cross-linking also reduces the strength of the wall, which ruptures when the borate-deficient cells enter the stationary growth phase (Fleischer et al., 1999). Reduced borate cross-linking of RG-II is associated with reduced growth, brittle tissues, and swollen cell walls when pumpkin plants are grown in the absence of added borate (Ishii et al., 2001, 2002). Similarly, tobacco cells adapted to grow in low-borate media have much thicker walls than is normal and most of the RG-II is not cross-linked (Matoh et al., 2000). Mutations that alter RG-II structure and thereby decrease its ability to cross-link with borate have also been shown to be deleterious to plant growth and development (O’Neill et al., 2001; Noguchi et al., 2003; Reuhs et al., 2004; Ahn et al., 2006; Delmas et al., 2008; Voxeur et al., 2011). Such results when taken together with evidence that RG-II, homogalacturonan, and rhamnogalacturonan I are covalently linked to one another (Ishii and Matsunaga, 2001), are consistent with the hypothesis that cross-linking of RG-II results in the formation of a pectic network within the primary cell wall (Brown and Hu, 1997; Matoh and Kobayashi, 1998; O’Neill et al., 2004). The inability to form this network likely alters the mechanical and physical properties of the cell wall and may account for many of the growth defects observed when vascular plants do not have an adequate supply of boron (Loomis and Durst, 1992).
The conserved structure of RG-II likely ensures that two RG-II molecules can interact with one another and with borate to self-assemble into a dimer. This dimer apparently is essential for the formation of a functional wall. Nevertheless, there is evidence that the glycosyl sequences of RG-II isolated from different plants are not identical. Such natural differences typically involve variations in the external monosaccharides of a side chain (see Figure 1A) and have no discernible effect on borate cross-linking or plant growth. For example, side chain B exists predominantly as a heptasaccharide in Arabidopsis (Glushka et al., 2003) and rice (Thomas et al., 1989) RG-II and predominantly as a nonasaccharide in sycamore (Whitcombe et al., 1995), wine (Glushka et al., 2003), and citrus (Séveno et al., 2009). The B side chain of the RG-II isolated from the walls of several non-flowering plants contains terminal non-reducing 3-O-methyl rhamnose rather than terminal non-reducing rhamnose (Matsunaga et al., 2004). There are no published data showing structural variations in side chains A, C, and D. Nevertheless, other glycoforms may exist as RG-II has only been isolated from a limited number of plants and structurally characterized. Furthermore, the possibility cannot be discounted that RG-II structure varies in different plant tissues and cell types. If other RG-II glycoforms do exist they may provide insight into the minimum glycosyl sequence that is required for borate cross-linking and for a functional wall.
RG-II is the only glycan known in nature to consist of such a large number of different sugars linked to one another by so many different glycosidic linkages. However, it is not known if this structural complexity is required solely to ensure that RG-II can interact with borate to form a functional wall or if RG-II has other as yet unidentified functions. To fully define the role(s) of RG-II in the cell wall requires in-depth knowledge of the molecular processes leading to the initiation of RG-II synthesis, the elongation of the backbone, and the formation and modification of the four side chains. Here we briefly review recent genetic and biochemical studies that have contributed to a better understanding of some of the factors involved in RG-II formation.
Nucleotide Sugars Required for RG-II Biosynthesis
RG-II synthesis is believed to occur in the Golgi apparatus (Mohnen, 2008) and, due to its structural complexity, requires a large number of different activated sugars, specific glycosyltransferases and additional enzymes required for methylation and acetylation of side chain residues. RG-II formation requires the following nucleotide sugars: UDP-D-Xylp, UDP-D-Galp, UDP-D-GalpA, UDP-D-GlcpA, CMP-D-Kdo (2-keto-3-deoxy-D-manno-octulosonic acid), UDP-L-Rhap, UDP-L-Arap, UDP-L-Araf, GDP-L-Fucp, and GDP-L-Galp (Figure 2). In addition, UDP-D-Apif [3-C-(hydroxymethyl)-D-glycero-tetrose], a plant-specific activated branched-chain sugar (Mølhøj et al., 2003; Guyett et al., 2009), and the as yet unidentified activated forms of Dha (2-keto-3-deoxy-D-lyxo-heptulosaric acid) and aceric acid (3-C-carboxy-5-deoxy-L-xylose) are also necessary. Several studies have shown that mutations that affect the formation of nucleotide sugars involved in RG-II synthesis may severely impact plant growth and development. For example, the lack of UDP-Api (Ahn et al., 2006) or CMP-Kdo (Delmas et al., 2008; Kobayashi et al., 2011) is lethal. By contrast, reducing the amounts of GDP-L-Fuc (O’Neill et al., 2001) and GDP-L-Gal (Voxeur et al., 2011) that are formed results in decreased plant growth. This is likely due to reduced RG-II cross-linking as plant growth is rescued by feeding the plants with exogenous supply of borate (O’Neill et al., 2001; Voxeur et al., 2011).
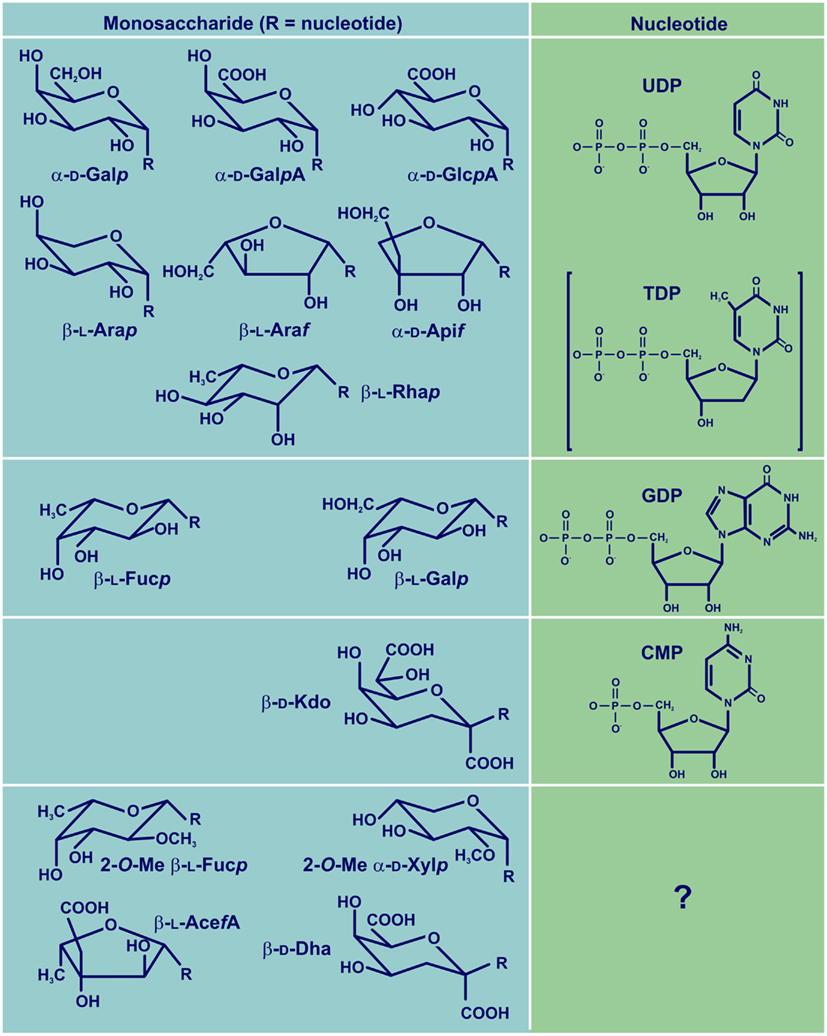
Figure 2. The monosaccharides of RG-II and their corresponding nucleotides. TDP-Rha is the activated form of rhamnose in bacteria. It is not known if fucose and xylose are O-methylated when they exist as nucleotide sugars or after they have been transferred to the side chains of RG-II. The activated forms of aceric acid and Dha have not been determined.
Glycosyltransferases (GTs) are enzymes that transfer a sugar from the nucleotide sugar in a linkage-specific manner to extend an acceptor. We estimate that at least 22 specific GTs are needed to synthesize RG-II, based on the assumption that one GT is required for the formation of each different glycosidic linkage. Additionally, two O-methyl transferases, two O-acetyl transferases and the enzymes that generate their respective donors are required for the modification of side chain monosaccharides.
Many of the activated sugars used for the synthesis of RG-II are also used for the synthesis of other plant glycans including polysaccharides, glycoproteins, proteoglycans, glycolipids, and low-molecular-weight glycosides. By contrast, RG-II and apiogalacturonan, which has a backbone composed of 1,4-linked α-D-GalpA residues that are substituted at O-2 with apiosyl and di-apiosyl side chains (Hart and Kindel, 1970; Ovodov et al., 1971; Longland et al., 1989; Gloaguen et al., 2010), are the only polysaccharides known to require UDP-Api for their formation. To date, apiogalacturonans have only detected in the cell walls of selected monocots including Lemna and plants referred to as “seagrasses” (Hart and Kindel, 1970; Ovodov et al., 1971; Gloaguen et al., 2010).
CMP-Kdo is required for the formation of RG-II and may also be used for the synthesis of glycolipids (Li et al., 2011), whereas the activated forms of AceA and Dha appear to be used only for the synthesis of RG-II. Thus, for RG-II to be produced by early land plants, either existing NDP-sugar biosynthetic genes/pathways evolved new functions to allow for the formation of different sugars or new genes were recruited to expand the repertoire of activated sugars. With the exception of UDP-Api and the activated form of AceA, sequence data provides evidence that genes involved in the formation of the remaining nucleotide sugars were present in green plants prior to the existence of RG-II (see Table 1). For example, CMP-Kdo synthetase has been proposed to have appeared in plants through an ancient horizontal gene transfer event from endoparasitic eubacteria similar to the genus Chlamydia (Royo et al., 2000). The development of hypotheses describing the sequence of molecular events that allowed plants to synthesize RG-II requires further phylogenetic analysis of NDP-sugar biosynthetic pathway enzymes from taxonomically diverse plants and prokaryotes.
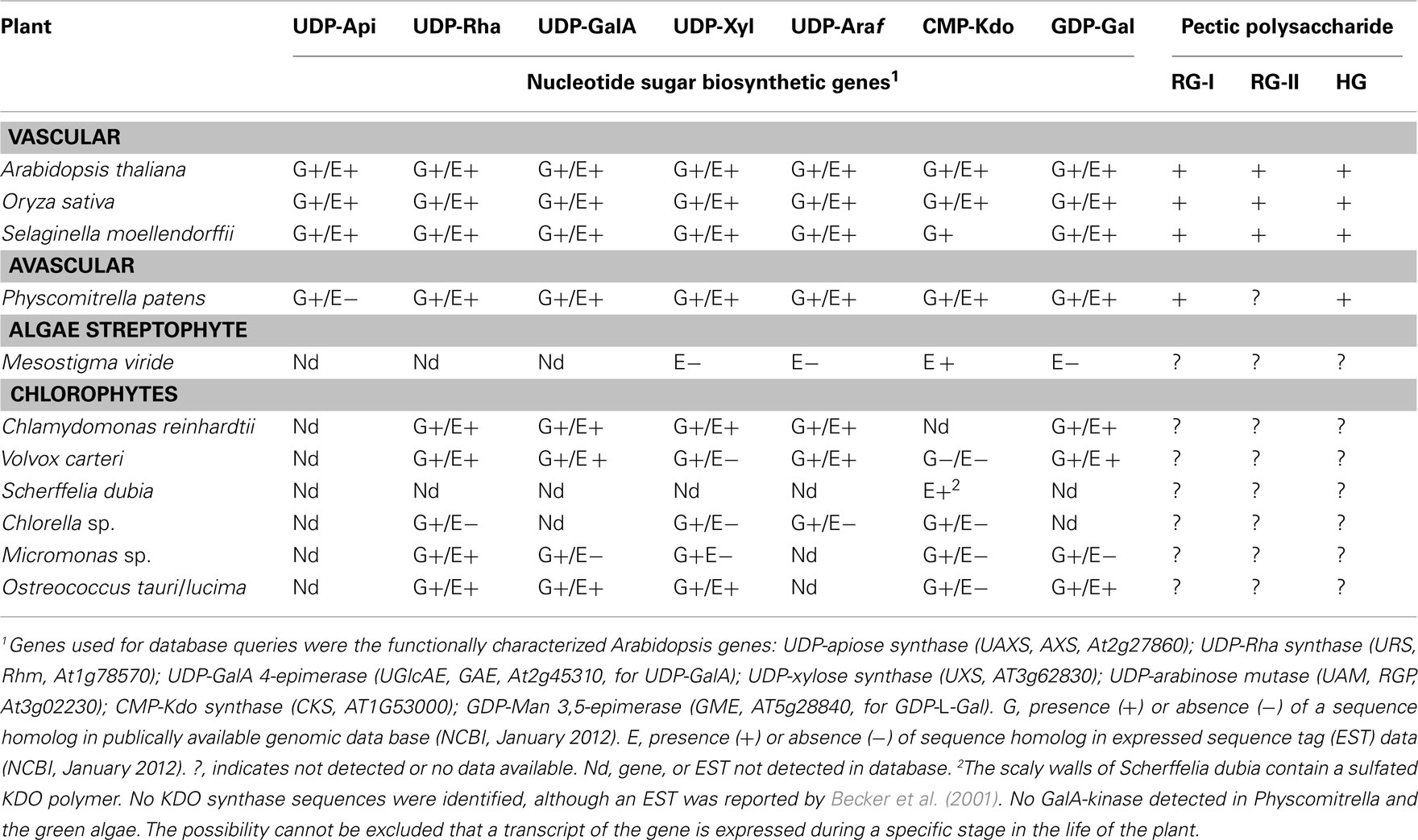
Table 1. The occurrence of selected nucleotide sugar biosynthetic genes and pectic polysaccharides in green plants.
The Subcellular Distribution of Nucleotide Sugars
Nucleotide sugars are used in diverse cellular compartments to form glycans and low-molecular-weight glycosides. For example, UDP-Rha is likely used for the synthesis of RG-II and rhamnogalacturonan I (RG-I) in the Golgi (Mohnen, 2008) but is also used in the cytosol for the formation of rhamnose-containing secondary metabolites including flavonoids, and terpenoids (Bar-Peled et al., 1991). The enzymes that form UDP-Rha are present in the cytosol, therefore mechanisms must exist to transport this activated sugar to the appropriate organelle. By contrast, other nucleotide sugars including UDP-Xyl, UDP-Arap, and UDP-GalA are made in both the cytosol and in the lumen of the Golgi (Bar-Peled and O’Neill, 2011). For example, UDP-GalA is formed from UDP-GlcA by six 4-epimerase isoforms (UGlcAe) that reside in the Golgi lumen (Gu and Bar-Peled, 2004). However, UDP-GalA is also generated from GalA by a cytosolic GalA-kinase and a UDP-sugar pyrophosphorylase referred to as “Sloppy” (Yang et al., 2009). This raises the question of whether the pools of UDP-GalA formed in the cytosol and in the Golgi are used for the synthesis of specific polysaccharides. Some Golgi-localized glycosyltransferases have been shown to have their catalytic domain facing the cytosol and thus likely use cytosolic nucleotide sugars for glycan synthesis (Davis et al., 2010), whereas others including a GalA transferase have their catalytic domain in the Golgi lumen (Sterling et al., 2001). Additional studies are required to determine the biological significance of segregating the formation of some but not all UDP-sugars in plants. Such compartmentalization may provide an additional level of regulation to control the channel of sugars for the synthesis of specific polysaccharides.
Recent reports suggest that the catalytic domain of CMP-Kdo synthetase is localized to the lumen of mitochondria (Kobayashi et al., 2011). Thus, mitochondria are a site of CMP-Kdo formation, where it is presumably used for the synthesis of Kdo-containing glycolipids (Séveno et al., 2010; Li et al., 2011). If mitochondria are the only site of CMP-Kdo formation then mechanisms must exist to export CMP-Kdo to the Golgi for RG-II synthesis. Interestingly, there are reports that there is vesicular trafficking between the mitochondria, the endoplasmic reticulum and the Golgi (Jouhet et al., 2009). Yet, the possibility cannot be discounted that CMP-Kdo is transported to the Golgi via the cytosol or that an altogether different CMP-Kdo synthase exists in the Golgi.
Do Nucleotide Sugars Formed in the Cytosol and Golgi Contribute Equally to RG-II Synthesis?
There is compelling evidence that plants contain multiple genes encoding enzymes involved in the synthesis of the same NDP-sugar (Bar-Peled and O’Neill, 2011). Several hypotheses can been put forward for the existence of multiple isoforms: (i) Isoforms are redundant with one another, (ii) isoforms are expressed in a cell and tissue specific manner, (iii) each isoform is localized to a specific compartment within a single cell, (iv) individual isoforms can function in different Golgi cisternae or within subdomains of an individual cisternae, (v) individual isoforms are required for the formation of glycan synthase complexes, and (vi) the activity of individual isoforms may be differentially affected by other nucleotide sugars. One cannot exclude the possibility that not all Golgi are “created equal” since they possess different complements of isoforms.
The interconversion of UDP-Arap and UDP-Araf is catalyzed by a mutase. At least two mutase isoforms are found in rice (Konishi et al., 2007) and at least three mutases exist in Arabidopsis (Rautengarten et al., 2011). These mutases have been shown to exist predominantly in the cytosol although they may also be closely associated with the cytosolic face of the Golgi (Konishi et al., 2007; Rautengarten et al., 2011). Multiple isoforms located exclusively in the cytosol generate pools of UDP-Rha, UDP-Api, UDP-Gal, and GDP-Fuc. Thus, these activated sugars must be imported into the lumen of the Golgi by nucleotide sugar transporters (Reyes and Orellana, 2008) if they are to be used as substrates by those glycosyltransferases that have their catalytic domains facing the Golgi lumen. UDP-Xyl, UDP-GalA, and UDP-Arap are also formed by multiple isoforms. However these isoform exist in both the cytosol and in the Golgi lumen (Bar-Peled and O’Neill, 2011). Such subcellular compartmentalization may provide a mechanism to regulate the channeling of precursor sugars for the synthesis of specific polysaccharides.
Enzymes involved in plant N-glycan biosynthesis and processing (Schoberer and Strasser, 2011) and cell wall polysaccharide formation (Chevalier et al., 2010) have been reported to be targeted to and localized in different Golgi sub-compartments. For example, the glycosyltransferases involved in forming the side chains of xyloglucan are differentially distributed across the compartments of Golgi stacks. The XylT that adds Xyl to the glucan backbone resides in the cis and medial Golgi cisternae, the GalT the adds Gal to this Xyl resides in the medial cisternae and the FucT that fucosylates the Gal resides in the trans cisternae (Chevalier et al., 2010). Thus, it is probable that specifically localized transporters import UDP-Gal and GDP-Fuc into the cisternae where the distinct glycosyltransferase activities exist. However, the source of the UDP-Xyl used by the XylT is not known as UDP-Xyl is produced in both the cytosol and in the Golgi lumen.
Based on analyses of transcriptomic data for individual root cells (Birnbaum et al., 2003; Brady et al., 2007), the genes encoding the multiple isoforms of the NDP-sugar-forming enzymes are in large part expressed in all cells, albeit at different levels (unpublished results of the authors). This suggests that multiple isoforms of the same enzyme activity are essential for the normal functioning of a single cell. The challenge will be to decipher the role of each isoform.
The flux of precursors between and within different subcellular compartments is likely to have an important role in regulating the synthesis of RG-II as well as other cell wall glycans. Sugars may be channeled to different glycan metabolic routes through different pools of nucleotide sugars. Alternatively, partitioning may provide a mechanism to control, at the metabolite level (e.g., UDP-GalA), the amounts of RG-II, or other polysaccharides produced. Another possibility is that the amount of an activated sugar available is a bottleneck for wall polysaccharide synthesis. For example, the amounts of UDP-apiose present in a cell may be key to controlling the amounts of RG-II formed. Altering the balance of nucleotide sugars may also have multiple effects on wall compositions. For example, analyses of the developing endosperm in the ADP-Glc transporter mutant (lys) of barley showed that the amounts of mixed-linkage β-glucan increased, presumably due to a shift of the ADP-Glc pool via Glc-1-P to UDP-Glc (Christensen and Scheller, 2012). Interestingly, rice plants carrying a null mutation in a gene encoding a Golgi-localized UDP-Glc transporter (OsNST1) have walls that are thinner than normal and contain reduced amounts of cellulose and xyloglucan and increased amounts of arabinoxylan and pectin (Zhang et al., 2011).
UDP-GlcNAc is used as a donor substrate for the formation of glycoproteins (Kornfeld and Kornfeld, 1985). In animals, UDP-GlcNAc is also used by O-GlcNAc transferases for the post-translational modification of regulatory proteins (Comer and Hart, 2000). Such O-GlcNAc modification has been shown to affect gene expression and contributes to changes in metabolism and growth (Slawson et al., 2010). Plants contain two O-GlcNAc transferases referred to as SECRET AGENT (SEC) and SPINDLY (SPY) that are functionally similar to their animal counterparts (Hartweck et al., 2002; Olszewski et al., 2010). Mutant analysis has indicated that SEC and SPY-dependent O-GlcNAcylation may have a role in multiple plant metabolic pathways and growth processes, although their target proteins remain largely unknown. SPY has been proposed to modify transcription factors, which in turn affect a plants response to cytokinin (Steiner et al., 2012). Thus, plants may be utilizing a O-GlcNAc modification to sense and control diverse plant biochemical pathways. It will be of interest to determine if the activities of wall biosynthetic enzymes are directly or indirectly affected by O-GlcNAc modification. It also remains to be determined if other nucleotide sugars have a role in as yet unidentified signaling pathways.
RG-II Biosynthesis – Initiation and Assembly
Current models of pectin structure depict a macromolecule composed of homogalacturonan, rhamnogalacturonan I and RG-II domains linked to one another by covalent bonds (Caffall and Mohnen, 2009), although the precise arrangement of each component remains a subject of debate (Vincken et al., 2003). Irrespective of how the individual components are arranged, the pectin macromolecules are believed to be cross-linked to one another in the walls of growing cells by the formation of borate diesters between RG-II molecules. Other types of molecular interactions including Ca2+ cross-links between GalA residues and hydrophobic interactions between methyl-esterified GalA residues likely stabilize the pectin network. Such a model of pectin structure, if correct, raises numerous questions regarding pectin synthesis in general and RG-II synthesis in particular.
In aqueous environments, pectic polysaccharides typically exist as large extended molecules. Light scattering and atomic force microscopy studies suggest that a single pectin molecule may have sizes in the range of 20–170 nm (Round et al., 2001, 2010) whereas a RG-II molecule may have a radius of up to 18 nm (Pérez et al., 2003). Pectic polysaccharides are believed to be synthesized in Golgi cisternae, which are typically flattened disk-like organelles with a diameter of up to a 1000-nm long and a height of 40 nm (Seguí-Simarro and Staehelin, 2006; Staehelin and Kang, 2008). How many pectin molecules can such a cisternae accommodate? Is this number increased by packing or folding of pectin molecules in a manner comparable to the compacting of DNA? Are pectins assembled on molecules that anchor them to the Golgi membrane or are they free to diffuse throughout the cisternae? Are the individual pectin domains synthesized together or in separate zones of the same cisternae or in different cisternae? If the individual domains are synthesized separately, when are they linked to one another and when does borate cross-linking of RG-II occur? Clearly, the volume of the Golgi, the dimensions of the polysaccharides being synthesized, the copy numbers of nucleotide sugar-forming enzymes, glycosyltransferases, and modifying enzymes along with all the other molecules that transit through this organelle must be taken into account to understand how pectin is assembled.
Here, we will for convenience, consider the synthesis of RG-II as occurring in three distinct steps: first, initiation and extension of the homogalacturonan backbone, followed by side chain formation and addition to the backbone, and finally, side chain modification. The borate cross-link may form during RG-II synthesis or during the incorporation of RG-II into the growing wall. Under normal growth conditions the apoplast likely contains adequate amounts of borate for cross-linking RG-II. If the borate cross-link is formed during RG-II synthesis, then mechanisms must exist to transport borate into the Golgi. Although several borate transporters have been identified in plants, they are present in the plasma membrane and are believed to be involved in boron uptake from the soil (Miwa and Fujiwara, 2010). Irrespective of where cross-linking of RG-II occurs, the currently available evidence suggests that borate diester formation is a spontaneous self-assembly process that does not require enzymes (Ishii et al., 1999).
Numerous genes that encode putative GalA transferases have been identified (Sterling et al., 2006; Yin et al., 2010). Only one of these (GAUT1) has been shown to be involved in the formation of the homogalacturonan backbone (Sterling et al., 2006). To date no GalAT has been identified and shown to be involved in the initiation and elongation of the RG-II backbone. Moreover, it is not known if RG-II side chains are attached to a pre-existing homogalacturonan, or if backbone extension and side chain addition are coupled to one another.
The formation of RG-II side chains and their attachment to the backbone may occur through at least two different mechanisms. The side chains may be assembled directly on the backbone by addition of one sugar at a time or the side chains could be assembled on a lipid intermediate and then transferred en bloc to the backbone. Lipid-linked oligosaccharides are known to be used for the formation of N- and O-linked oligosaccharides of glycoproteins (Sharma et al., 1974; Weerapana and Imperiali, 2006). Arabinose and xylose-containing lipids have been reported to be formed by pea membranes (Hayashi and Maclachlan, 1984), but their role in the formation of cell wall polysaccharides remains to be demonstrated.
Rhamnogalacturonan II xylosyltransferase (RGXT) is the only glycosyltransferase identified to date that has been predicted to be involved in the synthesis of an RG-II side chain (Egelund et al., 2006; Liu et al., 2011). The C-terminal catalytic domain of RGXT2 has been shown to be localized to the lumen of the Golgi apparatus (Søgaard et al., 2012). Recombinant versions of this transferase have been reported to catalyze the formation of the α-Xyl-1,3-Fuc linkage of side chain A (see Figure 1) using RG-II as an acceptor. Thus, at least in vitro, xylose can be added to a pre-existing side chain attached to RG-II and suggests that O-methylation of xylose occurs after it has been added to side chain A. Identifying other GTs involved in RG-II synthesis is hampered by the lack of viable RG-II-defective plants. Reverse-genetic approaches have also been of limited success. Thus, it is likely that identifying the genes and the corresponding enzymes involved in RG-II biosynthesis will require the use of classical biochemistry.
Studies of the structure of RG-II synthesized by the fucose-deficient mur1 mutant suggest that in vivo, the transferase that forms the α-Xyl-1,3-Fuc linkage is less effective when the L-Fuc is replaced by L-Gal (Reuhs et al., 2004; Séveno et al., 2009). By contrast, the transferase that adds β-GlcA to the same Fuc residue does not appear to be affected by the mur1 mutation. This GlcA serves as the acceptor for the addition of a L-Gal residue (see Figure 1). Interestingly, silencing genes involved in GDP-L-Gal formation results in reduced amounts of this L-Gal, decreased borate cross-linking of RG-II and reduced seedling growth (Voxeur et al., 2011). However, when these same mutants are grown in the presence of borate, seedling growth, and RG-II cross-linking is restored to wild-type. This result when taken together with studies of the mur1 mutant (O’Neill et al., 2001) indicate that altering RG-II structure results in reduced borate cross-linking of apiose, which is detrimental to plant growth. Knowledge of the three-dimensional structure of RG-II together with insight into how the side chains interact with one another and with borate and how these interactions are affected by changes in side chain structure are required to understand the structure-function relationships of RG-II and borate (Pérez et al., 2003).
Some of the sugars present in the side chains of RG-II are O-methylated and/or O-acetylated (see Figure 1). However, it is not known if these modifications affect the structure-function relationship of RG-II, since little is known about the genes and enzymes involved in such modifications. Early studies of the synthesis of grass 4-O-methyl glucuronoxylans indicated that the GlcA is O-methylated after it has been transferred to the xylan backbone (Kauss and Hassid, 1967). Acetyl-CoA has been shown to be a donor for O-acetylation of plant cell wall polysaccharides (Pauly and Scheller, 2000), although no protein has been isolated and shown to acetylate a specific plant polysaccharide. However, recent genetic studies have begun to shed light on some of the genes that may be involved in O-acetylation of plant polysaccharides (Gille et al., 2011; Manabe et al., 2011).
RG-II Disassembly
During plant cell growth and development a portion of the wall is believed to be recycled. Some tissues including, germinating seeds, developing fruits, and senescing leaves as well as pollen tubes have higher polysaccharide hydrolytic activity than other tissues and cells (Hadfield and Bennett, 1998; Buckeridge et al., 2000; Vicente et al., 2007). The released monosaccharides are believed to be made available for other metabolic processes or for conversion to nucleotide sugars and subsequent reincorporation into the growing wall. Nevertheless, the amounts of cell wall polysaccharides that are salvaged and the significance of these processes for plant growth remains to be determined. Arabidopsis mutants that are unable to salvage fucose by converting it to GDP-Fuc, accumulate large amounts of free fucose but appear to grow normally (Kotake et al., 2008). By contrast, Arabidopsis mutants that lack arabinose kinase and thus are unable to convert Ara to Ara-1-P germinate in the presence of arabinose but fail to grow (Dolezal and Cobbett, 1991). Numerous enzymes and genes involved in the salvage of GalA, Gal, Fuc, Ara, and GlcA have been described suggesting that numerous sugar salvage pathways exist in plants (Bar-Peled and O’Neill, 2011). However, it is not known if any of the RG-II sugars are recycled. It will be of interest to determine if plants produce enzymes that fragment the RG-II backbone and the side chains. Such enzymes would also be of value for structure-function studies of RG-II, and for the generation of acceptor molecules for biosynthesis studies, since very few microbial enzymes have been indentified that fragment RG-II (Vidal et al., 1999).
When did RG-II First Appear in Green Plants – Insight from Nucleotide Sugar Biosynthetic Genes
There is persuasive evidence that RG-II is present in the cell walls of all major groups of extant vascular plants including lycopodiophytes, pteridophytes, gymnosperms, and angiosperms (Matsunaga et al., 2004). By contrast, it is far from clear whether RG-II is present in the cell walls of avascular land plants (mosses, liverworts, and hornworts), and green algae (Matsunaga et al., 2004; Sørensen et al., 2011). Bryophytes have been reported to contain over 100-fold less RG-II than dicots on the basis of the boron contents of their cell walls (Matsunaga et al., 2004). Interestingly, the genome of the moss Physcomitrella patens contains a gene encoding a protein with high sequence identity (73%) to Arabidopsis UDP-Api synthase (see Table 1). We identified no transcripts for this gene in publically available EST databases, nor have we been able to detect apiose in the cell walls isolated from Physcomitrella leafy gametophores or protonema (unpublished results of the authors). Thus, the function of the product of this gene in Physcomitrella and the biosynthetic pathways that it is involved in remains to be determined. No monosaccharides characteristic of RG-II have been detected in the cell walls of charophycean green algae (Sørensen et al., 2011). By contrast, there is evidence that the cell walls of these algae and the cell walls bryophytes do contain homogalacturonan (Moller et al., 2007; Sørensen et al., 2011), whereas rhamnogalacturonan I may be restricted to land plant cell walls (Sørensen et al., 2011).
The walls of several monocots (Alismatales) including Lemna and the “seagrasses” contain apiogalacturonans (Hart and Kindel, 1970; Golovchenko et al., 2002; Gloaguen et al., 2010), but the relationship between RG-II and these polysaccharides is not known. Apiogalacturonans appear to have a limited distribution in vascular plants and may be a relatively recent addition to the cell wall as there are no studies describing their presence in the walls of non-flowering vascular plants. Nevertheless, “absence of evidence” is not “evidence of absence”. Indeed, mixed-linkage β-glucans were once believed to be present only in grass cell walls, but are now known to be a component of the walls of horsetails, a non-flowering vascular plant (Fry et al., 2008; Sørensen et al., 2008).
Rhamnose is a component of RG-II as well as rhamnogalacturonan I and plant glycosides. It is likely that in plants UDP-Rha is the predominate activated form of rhamnose (Bar-Peled and O’Neill, 2011). Genes encoding enzymes for UDP-Rha exist in diverse algae and avascular plants (Table 1). Somewhat unexpectedly, genes with high sequence similarity to plant UDP-Rha biosynthetic genes have been identified in viruses that inhabit both Chlorella and ameba and in selected fungi (Parakkottil Chothi et al., 2010; Martinez et al., 2012). Moreover, many of these organisms have been shown to produce UDP-Rha. By contrast prokaryotes utilize dTDP-Rha in the synthesis of Rha-containing glycans (Trezer et al., 1999). Interestingly the plant enzymes that form UDP-Rha can also form TDP-Rha in vitro, albeit at lower efficiency (Watt et al., 2004). Such studies suggest that using different forms of activated rhamnose for the synthesis of rhamnose-containing glycans provides some, as yet unidentified, evolutionary advantages for prokaryotes and eukaryotes.
Currently available data is consistent with the notion that RG-II appeared early in the evolution of land plants (Matsunaga et al., 2004; Sørensen et al., 2011). However, this data is insufficient to determine if this occurred before or after the divergence of avascular and vascular plants. Irrespective of when RG-II appeared in plants, the question remains as to whether the activated sugars required for RG-II synthesis appeared at the same time as RG-II or were already present in plants but used for the formation of other carbohydrate-containing molecules. An examination of publically available sequence data (genomic and expression sequence tags) revealed that genes with homology to UDP-apiose synthase (UAXS/AXS) exist in land plants but not in green algae (Table 1). By contrast, genes for the formation of CMP-Kdo are widely distributed in green plants (Table 1). However, this alone is not evidence for the presence of RG-II. For example, the walls of some members of the Prasinophyceaen algae (Chlorophyta) contain sulfated polysaccharides composed of Kdo, 5-O-methyl Kdo, Dha, and GalA (Becker et al., 1989, 1991, 1998, 2001). Dha has also been reported to be a present in the scales of the Streptophyte alga Mesostigma viride (Domozych et al., 1991). It is believed that these ancient green algae or their ancestors acquired the genes for the formation of the activated forms of Kdo and Dha from prokaryotes (Royo et al., 2000). Interestingly, the Prasinophyte polysaccharide, which has been reported to have a backbone composed of 2,8-linked Kdo residues, has structural features similar to the polysialic acids produced by animals and bacteria (Muhlenhoff et al., 1998). The presence in land plants of genes encoding Kdo transferases can also no longer be considered a marker for RG-II, as the studies by Raetz and colleagues have shown that Arabidopsis has orthologs of several enzymes involved in the biosynthesis bacterial Kdo-containing glycolipids (Li et al., 2011).
Clearly, our knowledge of the changes in the types and amounts of polysaccharides in the cell wall during the evolution of land plants is rudimentary (Niklas, 2004; Popper and Tuohy, 2010; Sørensen et al., 2010; Popper et al., 2011). How such changes affected wall architecture and function also need to be elucidated. If substantial advances are to be made in plant cell wall biology we will require a combination of chemical, biochemical, and genetic data from a much wider taxonomic range of Chlorophyta and Streptophyta (Viridiplantae) than is currently available. Such metadata can then be evaluated using computational biology to reveal candidate genes and proteins involved in wall biology in general as well as in RG-II formation.
Future Challenges
Although there has been some progress in understanding RG-II structure and function, numerous challenges remain for the current and future generations of cell wall researchers. Clearly, a complete description of RG-II formation will require the identification of the activated forms of aceric acid and Dha as well as the functional characterization of the myriad genes encoding the glycosyl, acetyl, and methyl transferases involved in RG-II assembly. Progress in this area will be dependent on the ability to generate the appropriate acceptor substrates and to develop facile assays for the individual transferases activities. Success in these endeavors will provide an opportunity to determine if RG-II is either formed “one sugar at a time” or built in manner analogous to the N-linked oligosaccharides of glycoproteins. With this information in hand it will be possible to identify the factors that initiate RG-II synthesis and to determine the order of side chain assembly. This in turn will lead to insight into whether the amounts of RG-II formed and inserted into the wall is controlled by the activities of individual glycosyltransferases, the availability of specific nucleotide sugars or other yet to be discovered mechanisms. Since RG-II cross-linking is sensitive to changes in RG-II structure, it is important to determine if there are “quality control processes” in place to ensure that only the correct type of RG-II is deposited in the cell wall. Additional studies are required to address if ester formation in vivo is an entirely spontaneous chemical reaction or a reaction catalyzed by other molecules. Irrespective of where borate cross-linking occurs, there is a need to determine how two RG-II molecules “find one another” and then align so that the borate ester can form. Molecular modeling studies are likely to provide clues as to the ability of RG-II molecules to form complementary structures that interact with each other and thereby facilitate and stabilize cross-linking. In addition, studies are required to determine how plant cells detect changes in cross-linking of wall pectins and how such changes are translated into altered growth. Another major challenge will be to identify the mechanisms that coordinate the synthesis of RG-II and the other pectic polysaccharides present in the cell wall. There is increasing awareness that plant cell wall pectic polysaccharides may be formed by enzymes complexes (Atmodjo et al., 2011). If such complexes do exist, plant scientists must determine if all three pectin domains are synthesized by a “mega-complex” in the Golgi and then secreted into the apoplast. Alternatively, there may be complexes within the Golgi that synthesize the individual pectin domains. These domains would then need to be linked to one another by enzymes located either in the Golgi or the apoplast. A combination of genetic, biochemical, and physicochemical studies will be required to understand the biology of RG-II and will allow researchers to determine if the structural complexity of RG-II evolved solely to ensure the “correct” borate cross-linking of pectin in the primary wall or if this unusual polysaccharide has other as yet unidentified functions in plant growth and development.
Conflict of Interest Statement
The authors declare that the research was conducted in the absence of any commercial or financial relationships that could be construed as a potential conflict of interest.
Acknowledgments
Research in the authors laboratories is funded in part by US National Science Federation grant IOB-0453664 (Maor Bar-Peled), by United States-Israel Binational Agricultural Research and Development Fund Grant IS-4141-08 (Maor Bar-Peled) and by U.S. Department of Energy grant DE-FG02-96ER20220 (Malcolm A. O’Neill). The authors research is also funded as part of the Bioenergy Science Center (BESC), a U.S. Department of Energy Bioenergy Research Center supported by the Office of Biological and Environmental Research in the DOE Office of Science (grant DE-PS02-06ER64304).
References
Ahn, J. W., Verma, R., Kim, M., Lee, J. Y., Kim, Y. K., Bang, J. W., Reiter, W. D., and Pai, H. S. (2006). Depletion of UDP-D-apiose/UDP-D-xylose synthases results in rhamnogalacturonan-II deficiency, cell wall thickening, and cell death in higher plants. J. Biol. Chem. 281, 13708–13718.
Atmodjo, M. A., Sakuragi, Y., Zhu, X., Burrell, J. A., Mohanty, S. S., Atwood, J. A. III, Orlando, R., Scheller, H. V., and Mohnen, D. (2011). Galacturonosyltransferase (GAUT)1 and GAUT7 are the core of a plant cell wall pectin biosynthetic homogalacturonan:galacturonosyltransferase complex. Proc. Nat. Acad. Sci. U.S.A. 108, 20225–20230.
Bar-Peled, M., Lewinsohn, E., Fluhr, R., and Gressel, J. (1991). UDP-rhamnose: flavanone-7-O-glucoside-2-O-rhamnosyltransferase. Purification and characterization of an enzyme catalyzing the production of bitter compounds in citrus. J. Biol. Chem. 266, 20953–20960.
Bar-Peled, M., and O’Neill, M. A. (2011). Plant nucleotide sugar formation, interconversion, and salvage by sugar recycling. Annu. Rev. Plant Biol. 62, 127–155.
Becker, B., Becker, D., Kamerling, J. P., and Melkonian, M. (1991). 2-keto-sugar acids in green flagellates: a chemical marker for Prasinophycean scales. J. Phycol. 27, 498–504.
Becker, B., Feja, N., and Melkonian, M. (2001). Analysis of expressed sequence tags (ESTs) from the scaly green flagellate Scherffelia dubia Pascher emend. Melkonian et Preisig. Protist 152, 139–147.
Becker, B., Hard, K., Melkonian, M., Kamerling, J. P., and Vligenthart, F. G. V. (1989). Identification of 3-deoxy-manno-2-octulosonic acid, 3-deoxy-5-O-methyl-manno-2-octulosonic acid and 3-deoxy-lyxo-2-heptulosaric acid in the cell wall (theca) of the green alga Tetraselmis striata Buthcher (Prasinophyceae). Eur. J. Biochem. 182, 153–160.
Becker, B., Melkonian, M., and Kamerling, J. P. (1998). The cell wall (theca) of Tetraselmis striata (Chlorophyta): macromolecular composition and structural elements of the complex polysaccharides. J. Phycol. 34, 779–787.
Birnbaum, K., Shasha, D. E., Wang, J. Y., Jung, J. W., Lambert, G. M., Galbraith, D. W., and Benfey, P. N. (2003). A gene expression map of the Arabidopsis root. Science 302, 1956–1960.
Brady, S. M., Orlando, D. A., Lee, J. Y., Wang, J. Y., Koch, J., Dinneny, J. R., Mace, D., Ohler, U., and Benfey, P. N. (2007). A high-resolution root spatiotemporal map reveals dominant expression patterns. Science 318, 801–806.
Brown, P. H., and Hu, H. (1997). Does boron play only a structural role in the growing tissues of higher plants? Plant Soil 196, 211–215.
Buckeridge, M. S., Pessoa dos Santos, H., and Tiné, M. A. S. (2000). Mobilisation of storage cell wall polysaccharides in seeds. Plant Physiol. Biochem. 38, 141–156.
Caffall, K. H., and Mohnen, D. (2009). The structure, function, and biosynthesis of plant cell wall pectic polysaccharides. Carbohydr. Res. 344, 1879–1900.
Chevalier, L., Bernard, S., Ramdani, Y., Lamour, R., Bardor, M., Lerouge, P., Follet-Gueye, M. L., and Driouich, A. (2010). Subcompartment localization of the side chain xyloglucan-synthesizing enzymes within Golgi stacks of tobacco suspension-cultured cells. Plant J. 64, 977–989.
Christensen, U., and Scheller, H. V. (2012). Regulation of (1, 3;1, 4) β-d-glucan synthesis in developing endosperm of barley lys mutants. J. Cereal Sci. 55, 69–76.
Comer, F. I., and Hart, G. W. (2000). O-Glycosylation of nuclear and cytosolic proteins. J. Biol. Chem. 275, 29179–29182.
Darvill, A. G., McNeil, M., and Albersheim, P. (1978). Structure of plant cell walls: VIII. A new pectic polysaccharide. Plant Physiol. 62, 418–427.
Davis, J., Brandizzi, F., Liepman, A. H., and Keegstra, K. (2010). Arabidopsis mannan synthase CSLA9 and glucan synthase CSLC4 have opposite orientations in the Golgi membrane. Plant J. 64, 1028–1037.
Delmas, F., Séveno, M., Northey, J. G. B., Hernould, M., Lerouge, P., McCourt, P., and Chevalier, C. (2008). The synthesis of the rhamnogalacturonan II component 3-deoxy-D-manno-2-octulosonic acid (Kdo) is required for pollen tube growth and elongation. J. Exp. Bot. 59, 2639–2647.
Dolezal, O., and Cobbett, C. S. (1991). Arabinose kinase-deficient mutant of Arabidopsis thaliana. Plant Physiol. 96, 1255–1260.
Domozych, D. S., Wells, B., and Shaw, P. J. (1991). Basket scales of the green alga, Mesostigma viride: chemistry and ultrastructure. J. Cell Sci. 100, 397–407.
du Penhoat, C. H., Gey, C., Pellerin, P., and Perez, S. (1999). An NMR solution study of the mega-oligosaccharide, rhamnogalacturonan II. J. Biomol. NMR 14, 253–271.
Edashige, Y., and Ishii, T. (1998). Rhamnogalacturonan II from cell walls of Cryptomeria japonica. Phytochemistry 49, 681–690.
Egelund, J., Petersen, B. L., Motawia, M. S., Damager, I., Faik, A., Olsen, C. E., Ishii, T., Clausen, H., Ulvskov, P., and Geshi, N. (2006). Arabidopsis thaliana RGXT1 and RGXT2 encode Golgi-localized (1,3)-α-D-xylosyltransferases involved in the synthesis of pectic rhamnogalacturonan-II. Plant Cell 18, 2593–2607.
Fleischer, A., O’Neill, M. A., and Ehwald, R. (1999). The pore size of non-graminaceous plant cell walls is rapidly decreased by borate ester cross-linking of the pectic polysaccharide rhamnogalacturonan II. Plant Physiol. 121, 829–839.
Fry, S. C., Nesselrode, B. H. W. A., Miller, J. G., and Mewburn, B. R. (2008). Mixed-linkage (1→3, 1→4)-β-D-glucan is a major hemicellulose of Equisetum (horsetail) cell walls. New Phytol. 179, 104–115.
Gille, S., de Souza, A., Xiong, G., Benz, M., Cheng, K., Schultink, A., Reca, I. B., and Pauly, M. (2011). O-acetylation of Arabidopsis hemicellulose xyloglucan requires AXY4 or AXY4L, proteins with a TBL and DUF231 domain. Plant Cell 23, 4041–4053.
Gloaguen, V., Brudieux, V., Closs, B., Barbat, A., Krausz, P., Sainte-Catherine, O., Kraemer, M., Maes, E., and Guerardel, Y. (2010). Structural characterization and cytotoxic properties of an apiose-rich pectic polysaccharide obtained from the cell wall of the marine phanerogam Zostera marina. J. Nat. Prod. 73, 1087–1092.
Glushka, J. N., Terrell, M., York, W. S., O’Neill, M. A., Gucwa, A., Darvill, A. G., Albersheim, P., and Prestegard, J. H. (2003). Primary structure of the 2-O-methyl-α-fucose-containing side chain of the pectic polysaccharide, rhamnogalacturonan II. Carbohydr. Res. 338, 341–352.
Golovchenko, V. V., Ovodova, R. G., Shashkov, A. S., and Ovodov, Y. S. (2002). Structural studies of the pectic polysaccharide from duckweed Lemna minor L. Phytochemistry 60, 89–97.
Gu, X., and Bar-Peled, M. (2004). The biosynthesis of UDP-galacturonic acid in plants. Functional cloning and characterization of Arabidopsis UDP-D-glucuronic acid 4-epimerase. Plant Physiol. 136, 4256–4262.
Guyett, P., Glushka, J., Gu, X., and Bar-Peled, M. (2009). Real-time NMR monitoring of intermediates and labile products of the bifunctional enzyme UDP-apiose/UDP-xylose synthase. Carbohydr. Res. 344, 1072–1078.
Hadfield, K. A., and Bennett, A. B. (1998). Polygalacturonases: many genes in search of a function. Plant Physiol. 117, 337–343.
Hart, D. A., and Kindel, P. K. (1970). Isolation and partial characterization of apiogalacturonans from the cell wall of Lemna minor. Biochem. J. 116, 569–579.
Hartweck, L. M., Scott, C. L., and Olszewski, N. E. (2002). Two O-linked N-acetylglucosamine transferase genes of Arabidopsis thaliana L. Heynh. Have overlapping functions necessary for gamete and seed development. Genetics 161, 1279–1291.
Hayashi, T., and Maclachlan, G. (1984). Biosynthesis of pentosyl lipids by pea membranes. Biochem. J. 217, 791–803.
Hu, H., and Brown, P. H. (1994). Localization of boron in cell walls of squash and tobacco and its association with pectin (evidence for a structural role of boron in the cell wall). Plant Physiol. 105, 681–689.
Hu, H., Brown, P. H., and Labavitch, J. M. (1996). Species variability in boron requirement is correlated with cell wall pectin. J. Exp. Bot. 47, 227–232.
Ishii, T., and Matsunaga, T. (2001). Pectic polysaccharide rhamnogalacturonan II is covalently linked to homogalacturonan. Phytochemistry 57, 969–974.
Ishii, T., Matsunaga, T., and Hayashi, N. (2001). Formation of rhamnogalacturonan II-borate dimer in pectin determines cell wall thickness of pumpkin tissue. Plant Physiol. 126, 1698–1705.
Ishii, T., Matsunaga, T., Iwai, H., Satoh, S., and Taoshita, J. (2002). Germanium does not substitute for boron in cross-linking of rhamnogalacturonan II in pumpkin cell walls. Plant Physiol. 130, 1967–1973.
Ishii, T., Matsunaga, T., Pellerin, P., O’Neill, M. A., Darvill, A., and Albersheim, P. (1999). The plant cell wall polysaccharide rhamnogalacturonan II self-assembles into a covalently cross-linked dimer. J. Biol. Chem. 274, 13098–13104.
Jouhet, J., Dubots, E., Marechal, E., and Block, M. A. (2009). “Lipid trafficking in plant photosynthetic cells,” in Lipids in Photosynthesis: Essential and Regulatory Functions, eds W. Hajime and M. Norio (Berlin: Springer Science), 349–372.
Kauss, H., and Hassid, W. (1967). Biosynthesis of the 4-O-methyl-D-glucuronic acid unit of hemicellulose B by transmethylation from S-adenosyl-L-methionine. J. Biol. Chem. 242, 1680–1685.
Kobayashi, M., Kouzu, N., Inami, A., Toyooka, K., Konishi, Y., Matsuoka, K., and Matoh, T. (2011). Characterization of Arabidopsis CTP: 3-deoxy-D-manno-2-octulosonate cytidylyltransferase (CMP-KDO synthetase), the enzyme that activates KDO during rhamnogalacturonan II biosynthesis. Plant Cell Physiol. 52, 1832–1843.
Kobayashi, M., Matoh, T., and Azuma, J. (1996). Two chains of rhamnogalacturonan II are cross-linked by borate-diol ester bonds in higher plant cell walls. Plant Physiol. 110, 1017–1020.
Kobayashi, M., Nakagawa, H., Asaka, T., and Matoh, T. (1999). Borate-rhamnogalacturonan II bonding reinforced by Ca2+ retains pectic polysaccharides in higher-plant cell walls. Plant Physiol. 119, 199–204.
Konishi, T., Takeda, T., Miyazaki, Y., Ohnishi-Kameyama, M., Hayashi, T., O’Neill, M. A., and Ishii, T. (2007). A plant mutase that interconverts UDP-arabinofuranose and UDP-arabinopyranose. Glycobiology 17, 345–352.
Kornfeld, R., and Kornfeld, S. (1985). Assembly of asparagine-linked oligosaccharides. Annu. Rev. Biochem. 54, 631–664.
Kotake, T., Hojo, S., Tajima, N., Matsuoka, K., Koyama, T., and Tsumuraya, Y. (2008). A bifunctional enzyme with L-fucokinase and GDP-L-fucose pyrophosphorylase activities salvages free L-fucose in Arabidopsis. J. Biol. Chem. 283, 8125–8135.
Li, C., Guan, Z., Liu, D., and Raetz, C. R. H. (2011). Pathway for lipid A biosynthesis in Arabidopsis thaliana resembling that of Escherichia coli. Proc. Nat. Acad. Sci. U.S.A. 108, 11387–11392.
Liu, X. L., Liu, L., Niu, Q. K., Xia, C., Yang, K. Z., Li, R., Chen, L. Q., Zhang, X. Q., Zhou, Y., and Ye, D. (2011). MALE GAMETOPHYTE DEFECTIVE 4 encodes a rhamnogalacturonan II xylosyltransferase and is important for growth of pollen tubes and roots in Arabidopsis. Plant J. 65, 647–660.
Longland, J. M., Fry, S. C., and Trewavas, A. J. (1989). Developmental control of apiogalacturonan biosynthesis and UDP-apiose production in a duckweed. Plant Physiol. 90, 972–978.
Manabe, Y., Nafisi, M., Verhertbruggen, Y., Orfila, C., Gille, S., Rautengarten, C., Cherk, C., Marcus, S. E., Somerville, S., and Pauly, M. (2011). Loss-of-function mutation of REDUCED WALL ACETYLATION2 in Arabidopsis leads to reduced cell wall acetylation and increased resistance to Botrytis cinerea. Plant Physiol. 155, 1068–1078.
Martinez, V., Ingwers, M., Smith, J., Glushka, J., Yang, T., and Bar-Peled, M. (2012). Biosynthesis of UDP-4-keto-6-deoxyglucose and UDP-rhamnose in the pathogenic fungi Magnaporthe grisea and Botryotinia fuckeliana. J. Biol. Chem. 287, 879–892.
Matoh, T., Ishigaki, K., Ohno, K., and Azuma, J. (1993). Isolation and characterization of a boron-polysaccharide complex from radish roots. Plant Cell Physiol. 34, 639–642.
Matoh, T., Kawaguchi, S., and Kobayashi, M. (1996). Ubiquity of a borate-rhamnogalacturonan II complex in the cell walls of higher plants. Plant Cell Physiol. 37, 636–644.
Matoh, T., and Kobayashi, M. (1998). Boron and calcium, essential inorganic constituents of pectic polysaccharides in higher plant cell walls. J. Plant Res. 111, 179–190.
Matoh, T., Takasaki, M., Kobayashi, M., and Takabe, K. (2000). Boron nutrition of cultured tobacco BY-2 cells. III. Characterization of the boron-rhamnogalacturonan II complex in cells acclimated to low levels of boron. Plant Cell Physiol. 41, 363–366.
Matsunaga, T., Ishii, T., Matsumoto, S., Higuchi, M., Darvill, A., Albersheim, P., and O’Neill, M. A. (2004). Occurrence of the primary cell wall polysaccharide rhamnogalacturonan II in pteridophytes, lycophytes, and bryophytes. Implications for the evolution of vascular plants. Plant Physiol. 134, 339–351.
Melton, L. D., McNeil, M., Darvill, A. G., Albersheim, P., and Dell, A. (1986). Structural characterization of oligosaccharides isolated from the pectic polysaccharide rhamnogalacturonan II. Carbohydr. Res. 146, 279–305.
Miwa, K., and Fujiwara, T. (2010). Boron transport in plants: co-ordinated regulation of transporters. Ann. Bot. 105, 1103–1108.
Mølhøj, M., Verma, R., and Reiter, W. D. (2003). The biosynthesis of the branched-chain sugar D-apiose in plants: functional cloning and characterization of a UDPα-D-apiose/UDP-D-xylose synthase from Arabidopsis. Plant J. 35, 693–703.
Moller, I., Sørensen, I., Bernal, A. J., Blaukopf, C., Lee, K., Øbro, J., Pettolino, F., Roberts, A., Mikkelsen, J. D., and Knox, J. P. (2007). High-throughput mapping of cell-wall polymers within and between plants using novel microarrays. Plant J. 50, 1118–1128.
Muhlenhoff, M., Eckhardt, M., and Gerardy-Schahn, R. (1998). Polysialic acid: three-dimensional structure, biosynthesis and function. Curr. Opin. Struct. Biol. 8, 558–564.
Noguchi, K., Ishii, T., Matsunaga, T., Kakegawa, K., Hayashi, H., and Fujiwara, T. (2003). Biochemical properties of the cell wall in the Arabidopsis mutant bor1-1 in relation to boron nutrition. J. Plant Nutr. Soil Sci. 166, 175–178.
Olszewski, N. E., West, C. M., Sassi, S. O., and Hartweck, L. M. (2010). O-GlcNAc protein modification in plants: evolution and function. Biochim. Biophys. Acta 1800, 49–56.
O’Neill, M. A., Eberhard, S., Albersheim, P., and Darvill, A. G. (2001). Requirement of borate cross-linking of cell wall rhamnogalacturonan II for Arabidopsis growth. Science 294, 846.
O’Neill, M. A., Ishii, T., Albersheim, P., and Darvill, A. G. (2004). Rhamnogalacturonan II: structure and function of a borate cross-linked cell wall pectic polysaccharide. Annu. Rev. Plant Biol. 55, 109–139.
O’Neill, M. A., Warrenfeltz, D., Kates, K., Pellerin, P., Doco, T., Darvill, A. G., and Albersheim, P. (1996). Rhamnogalacturonan-II, a pectic polysaccharide in the walls of growing plant cells, forms a dimer that is covalently cross-linked by a borate ester. J. Biol. Chem. 271, 22923–22931.
Ovodov, Y. S., Ovodova, R., Bondarenko, O., and Krasikova, I. (1971). The pectic substances of zosteraceae: part IV. Pectinase digestion of zosterine. Carbohydr. Res. 18, 311–318.
Parakkottil Chothi, M., Duncan, G. A., Armirotti, A., Abergel, C., Gurnon, J. R., Van Etten, J. L., Bernardi, C., Damonte, G., and Tonetti, M. (2010). Identification of an L-rhamnose synthetic pathway in two nucleocytoplasmic large DNA viruses. J. Virol. 84, 8829–8838.
Pauly, M., and Scheller, H. V. (2000). O-Acetylation of plant cell wall polysaccharides: identification and partial characterization of a rhamnogalacturonan O-acetyl-transferase from potato suspension-cultured cells. Planta 210, 659–667.
Pellerin, P., Doco, T., Vida, S., Williams, P., Brillouet, J. M., and O’Neill, M. A. (1996). Structural characterization of red wine rhamnogalacturonan II. Carbohydr. Res. 290, 183–197.
Pérez, S., Rodriguez-Carvajal, M., and Doco, T. (2003). A complex plant cell wall polysaccharide: rhamnogalacturonan II. A structure in quest of a function. Biochimie 85, 109–121.
Popper, Z. A., Michel, G., Hervé, C., Domozych, D. S., Willats, W. G. T., Tuohy, M. G., Kloareg, B., and Stengel, D. B. (2011). Evolution and diversity of plant cell walls: from algae to flowering plants. Annu. Rev. Plant Biol. 62, 567–590.
Popper, Z. A., and Tuohy, M. G. (2010). Beyond the green: understanding the evolutionary puzzle of plant and algal cell walls. Plant Physiol. 153, 373–383.
Puvanesarajah, V., Darvill, A. G., and Albersheim, P. (1991). Structural characterization of two oligosaccharide fragments formed by the selective cleavage of rhamnogalacturonan II: evidence for the anomeric configuration and attachment sites of apiose and 3-deoxy-2-heptulosaric acid. Carbohydr. Res. 218, 211–222.
Rautengarten, C., Ebert, B., Herter, T., Petzold, C. J., Ishii, T., Mukhopadhyay, A., Usadel, B., and Scheller, H. V. (2011). The interconversion of UDP-arabinopyranose and UDP-arabinofuranose is indispensable for plant development in Arabidopsis. Plant Cell 23, 1373–1390.
Reuhs, B. L., Glenn, J., Stephens, S. B., Kim, J. S., Christie, D. B., Glushka, J. G., Zablackis, E., Albersheim, P., Darvill, A. G., and O’Neill, M. A. (2004). L-Galactose replaces L-fucose in the pectic polysaccharide rhamnogalacturonan II synthesized by the L-fucose-deficient mur1 Arabidopsis mutant. Planta 219, 147–157.
Reyes, F., and Orellana, A. (2008). Golgi transporters: opening the gate to cell wall polysaccharide biosynthesis. Curr. Opin. Plant Biol. 11, 244–251.
Round, A. N., Rigby, N. M., MacDougall, A. J., and Morris, V. J. (2010). A new view of pectin structure revealed by acid hydrolysis and atomic force microscopy. Carbohydr. Res. 345, 487–497.
Round, A. N., Rigby, N. M., MacDougall, A. J., Ring, S. G., and Morris, V. J. (2001). Investigating the nature of branching in pectin by atomic force microscopy and carbohydrate analysis. Carbohydr. Res. 331, 337–342.
Royo, J., Gimez, E., and Hueros, G. (2000). CMP-KDO synthetase: a plant gene borrowed from gram-negative eubacteria. Trends Genet. 16, 432–433.
Schoberer, J., and Strasser, R. (2011). Sub-compartmental organization of Golgi-resident N-glycan processing enzymes in plants. Mol. Plant 4, 220–228.
Seguí-Simarro, J. M., and Staehelin, L. A. (2006). Cell cycle-dependent changes in Golgi stacks, vacuoles, clathrin-coated vesicles and multivesicular bodies in meristematic cells of Arabidopsis thaliana: a quantitative and spatial analysis. Planta 223, 223–236.
Séveno, M., Séveno-Carpentier, E., Voxeur, A., Menu-Bouaouiche, L., Rihouey, C., Delmas, F., Chevalier, C., Driouich, A., and Lerouge, P. (2010). Characterization of a putative 3-deoxy-D-manno-2-octulosonic acid (Kdo) transferase gene from Arabidopsis thaliana. Glycobiology 20, 617–628.
Séveno, M., Voxeur, A., Rihouey, C., Wu, A. M., Ishii, T., Chevalier, C., Ralet, M. C., Driouich, A., Marchant, A., and Lerouge, P. (2009). Structural characterisation of the pectic polysaccharide rhamnogalacturonan II using an acidic fingerprinting methodology. Planta 230, 947–957.
Sharma, C. B., Babczinksi, P., Lehle, L., and Tanner, W. (1974). The role of dolichol monophosphate in glycoprotein biosynthesis in Saccharomyces cerevisiae. Eur. J. Biochem. 46, 35–41.
Shimokawa, T., Ishii, T., and Matsunaga, T. (1999). Isolation and structural characterization of rhamnogalacturonan II-borate complex from Pinus densiflora. J. Wood Sci. 45, 435–439.
Slawson, C., Copeland, R., and Hart, G. (2010). O-GlcNAc signaling: a metabolic link between diabetes and cancer? Trends Biochem. Sci. 35, 547–555.
Søgaard, C., Stenbæk, A., Bernard, S., Hadi, M., Driouich, A., Scheller, H. V., and Sakuragi, Y. (2012). GO-PROMTO illuminates protein membrane topologies of glycan biosynthetic enzymes in the Golgi apparatus of living tissues. PLoS ONE 7, e31324. doi:10.1371/journal.pone.0031324
Sørensen, I., Domozych, D., and Willats, W. G. T. (2010). How have plant cell walls evolved? Plant Physiol. 153, 366–372.
Sørensen, I., Pettolino, F. A., Bacic, A., Ralph, J., Lu, F., O’Neill, M. A., Fei, Z., Rose, J. K. C., Domozych, D. S., and Willats, W. G. T. (2011). The Charophycean green algae provide insights into the early origins of plant cell walls. Plant J. 68, 201–211.
Sørensen, I., Pettolino, F. A., Wilson, S. M., Doblin, M. S., Johansen, B., Bacic, A., and Willats, W. G. T. (2008). Mixed linkage (1→3),(1→4)-β-D-glucan is not unique to the Poales and is an abundant component of Equisetum arvense cell walls. Plant J. 54, 510–521.
Spellman, M. W., McNeil, M., Darvill, A. G., Albersheim, P., and Henrick, K. (1983a). Isolation and characterization of 3-C-carboxy-5-deoxy-L-xylose, a naturally occurring, branched-chain, acidic monosaccharide. Carbohydr. Res. 122, 115–129.
Spellman, M. W., McNeil, M., Darvill, A. G., Albersheim, P., and Dell, A. (1983b). Characterization of a structurally complex heptasaccharide isolated from the pectic polysaccharide rhamnogalacturonan II. Carbohydr. Res. 122, 131–153.
Staehelin, L. A., and Kang, B. H. (2008). Nanoscale architecture of endoplasmic reticulum export sites and of Golgi membranes as determined by electron tomography. Plant Physiol. 147, 1454–1468.
Steiner, E., Efroni, I., Gopalraj, M., Saathoff, K., Tseng, T. S., Kieffer, M., Eshed, Y., Olszewski, N., and Weiss, D. (2012). The Arabidopsis O-linked N-acetylglucosamine transferase SPINDLY interacts with class I TCPs to facilitate cytokinin responses in leaves and flowers. Plant Cell 24, 96–108.
Sterling, J. D., Atmodjo, M. A., Inwood, S. E., Kumar Kolli, V., Quigley, H. F., Hahn, M. G., and Mohnen, D. (2006). Functional identification of an Arabidopsis pectin biosynthetic homogalacturonan galacturonosyltransferase. Proc. Nat. Acad. Sci. U.S.A. 103, 5236–5241.
Sterling, J. D., Quigley, H. F., Orellana, A., and Mohnen, D. (2001). The catalytic site of the pectin biosynthetic enzyme (-1,4-galacturonosyltransferase is located in the lumen of the Golgi. Plant Physiol. 127, 360–371.
Stevenson, T. T., Darvill, A. G., and Albersheim, P. (1988a). 3-Deoxy-D-lyxo-2-heptulosaric acid, a component of the plant cell-wall polysaccharide rhamnogalacturonan-II. Carbohydr. Res. 179, 269–288.
Stevenson, T. T., Darvill, A. G., and Albersheim, P. (1988b). Structural features of the plant cell-wall polysaccharide rhamnogalacturonan-II. Carbohydr. Res. 182, 207–226.
Thomas, J. R., Darvill, A. G., and Albersheim, P. (1989). Isolation and structural characterization of the pectic polysaccharide rhamnogalacturonan II from walls of suspension-cultured rice cells. Carbohydr. Res. 185, 261–277.
Trezer, A., Salas, J. A., and Bechtold, A. (1999). Genes and enzymes involved in deoxysugar biosynthesis in bacteria. Nat. Prod. Rep. 16, 283–299.
Vicente, A. R., Saladie, M., Rose, J. K. C., and Labavitch, J. M. (2007). The linkage between cell wall metabolism and fruit softening: looking to the future. J. Sci. Food Agric. 87, 1435–1448.
Vidal, S., Doco, T., Williams, P., Pellerin, P., York, W. S., O’Neill, M. A., Glushka, J., Darvill, A. G., and Albersheim, P. (2000). Structural characterization of the pectic polysaccharide rhamnogalacturonan II: evidence for the backbone location of the aceric acid-containing oligoglycosyl side chain. Carbohydr. Res. 326, 277–294.
Vidal, S., Salmon, J. M., Williams, P., and Pellerin, P. (1999). Penicillium daleae, a soil fungus able to degrade rhamnogalacturonan II, a complex pectic polysaccharide. Enzyme Microb. Technol. 24, 283–290.
Vincken, J. P., Schols, H. A., Oomen, R. J. F. J., McCann, M. C., Ulvskov, P., Voragen, A. G. J., and Visser, R. G. F. (2003). If homogalacturonan were a side chain of rhamnogalacturonan I. Implications for cell wall architecture. Plant Physiol. 132, 1781–1789.
Voxeur, A., Gilbert, L., Rihouey, C., Driouich, A., Rothan, C., Baldet, P., and Lerouge, P. (2011). Silencing of the GDP-D-mannose 3,5-epimerase affects the structure and cross-linking of the pectic polysaccharide rhamnogalacturonan II and plant growth in tomato. J. Biol. Chem. 286, 8014–8023.
Watt, G., Leoff, C., Harper, A. D., and Bar-Peled, M. (2004). A bifunctional 3, 5-epimerase/4-keto reductase for nucleotide-rhamnose synthesis in Arabidopsis. Plant Physiol. 134, 1337–1346.
Weerapana, E., and Imperiali, B. (2006). Asparagine-linked protein glycosylation: from eukaryotic to prokaryotic systems. Glycobiology 16, 91R–101R.
Whitcombe, A. J., O’Neill, M. A., Steffan, W., Albersheim, P., and Darvill, A. G. (1995). Structural characterization of the pectic polysaccharide, rhamnogalacturonan-II. Carbohydr. Res. 271, 15–29.
Yang, T., Bar-Peled, L., Gebhart, L., Lee, S. G., and Bar-Peled, M. (2009). Identification of galacturonic acid-1-phosphate kinase, a new member of the GHMP kinase superfamily in plants, and comparison with galactose-1-phosphate kinase. J. Biol. Chem. 284, 21526–21532.
Yin, Y., Chen, H., Hahn, M. G., Mohnen, D., and Xu, Y. (2010). Evolution and function of the plant cell wall synthesis-related glycosyltransferase family 8. Plant Physiol. 153, 1729–1746.
York, W. S., Darvill, A. G., McNeil, M., and Albersheim, P. (1985). 3-Deoxy-D-manno-2-octulosonic acid (KDO) is a component of rhamnogalacturonan II, a pectic polysaccharide in the primary cell walls of plants. Carbohydr. Res. 138, 109–126.
Keywords: RG-II biosynthesis, UDP-apiose, CMP-kdo, aceric acid, Golgi, wall evolution, borate, dimer
Citation: Bar-Peled M, Urbanowicz BR and O’Neill MA (2012) The synthesis and origin of the pectic polysaccharide rhamnogalacturonan II – insights from nucleotide sugar formation and diversity. Front. Plant Sci. 3:92. doi: 10.3389/fpls.2012.00092
Received: 07 March 2012; Paper pending published: 24 March 2012;
Accepted: 23 April 2012; Published online: 11 May 2012.
Edited by:
Seth DeBolt, University of Kentucky, USAReviewed by:
Henrik Scheller, Lawrence Berkeley National Laboratory, USAJesper Harholt, University of Copenhagen, Denmark
Copyright: © 2012 Bar-Peled, Urbanowicz and O’Neill. This is an open-access article distributed under the terms of the Creative Commons Attribution Non Commercial License, which permits non-commercial use, distribution, and reproduction in other forums, provided the original authors and source are credited.
*Correspondence: Maor Bar-Peled, Department of Plant Biology, Complex Carbohydrate Research Center, University of Georgia, 315 Riverbend Road, Athens, GA 30602, USA. e-mail:cGVsZWRAY2NyYy51Z2EuZWR1