- Department of Plant Agriculture, University of Guelph, Guelph, ON, Canada
1-Methylcyclopropene (1-MCP) delays ethylene-meditated ripening of apple (Malus domestica Borkh.) fruit during controlled atmosphere (CA) storage. Here, we tested the hypothesis that 1-MCP and CA storage enhances the levels of polyamines (PAs) and 4-aminobutyrate (GABA) in apple fruit. A 46-week experiment was conducted with “Empire” apple using a split-plot design with four treatment replicates and 3°C, 2.5 kPa O2, and 0.03 or 2.5 kPa CO2 with or without 1 μL L-1 1-MCP. Total PA levels were not elevated by the 1-MCP treatment. Examination of the individual PAs revealed that: (i) total putrescine levels tended to be lower with 1-MCP regardless of the CO2 level, and while this was mostly at the expense of free putrescine, large transient increases in soluble conjugated putrescine were also evident; (ii) total spermidine levels tended to be lower with 1-MCP, particularly at 2.5 kPa CO2, and this was mostly at the expense of soluble conjugated spermidine; (iii) total spermine levels at 2.5 kPa CO2 tended to be lower with 1-MCP, and this was mostly at the expense of both soluble and insoluble conjugated spermine; and (iv) total spermidine and spermine levels at 0.03 kPa were relatively unaffected, compared to 2.5 kPa CO2, but transient increases in free spermidine and spermine were evident. These findings might be due to changes in the conversion of putrescine into higher PAs and the interconversion of free and conjugated forms in apple fruit, rather than altered S-adenosylmethionine availability. Regardless of 1-MCP and CO2 treatments, the availability of glutamate showed a transient peak initially, probably due to protein degradation, and this was followed by a steady decline over the remainder of the storage period which coincided with linear accumulation of GABA. This pattern has been attributed to the stimulation of glutamate decarboxylase activity and inhibition of GABA catabolism, rather than a contribution of PAs to GABA production.
Introduction
Over the last decade, 1-methylcyclopropene (1-MCP) has been adopted by the apple industry as a means of delaying ethylene-mediated fruit ripening and senescence, especially in combination with storage under controlled atmosphere (CA) conditions (i.e., 0–3°C, 2–2.5 kPa O2, 2–4 kPa CO2; DeEll et al., 2008; Fawbush et al., 2008; Watkins, 2008). 1-MCP inhibits ethylene binding and production in apple fruit held at ambient or chilling temperature, and reduces the expression of genes responsible for ethylene biosynthesis (Dal Cin et al., 2006; Pang et al., 2006; Vilaplana et al., 2007; Figure 1). Under commercial CA conditions, these findings are accompanied by a decline in the level of 1-aminocyclopropane-1-carboxylic acid (ACC), and the level of its precursor S-adenosylmethionine (SAM) is not directly linked to the rate of ethylene production and does not appear to be limiting (Bulens et al., 2012).
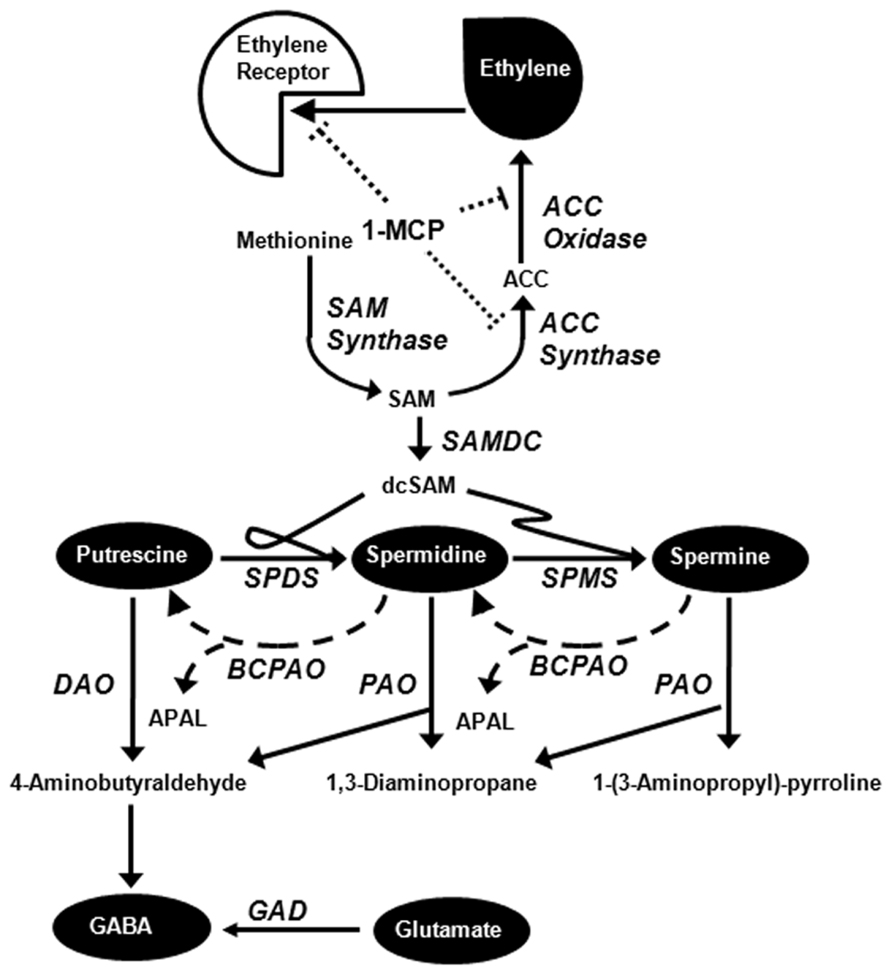
FIGURE 1. Simplified diagram of metabolic relationships among ethylene, polyamines, and GABA [adapted from Shelp et al. (2012c)]. 1-MCP inhibits ethylene binding to its receptor and the expression of both ACC synthase and ACC oxidase (see text for detailed explanation). Abbreviations: ACC, 1-aminocyclopropane-1-carboxylic acid; APAL, 3-aminopropionaldehyde; BCPAO, back-conversion polyamine oxidase; dcSAM, decarboxylated S-adenosylmethionine; DAO, diamine oxidase; GABA, 4-aminobutyrate; GAD, glutamate decarboxylase; 1-MCP, 1-methylcyclopropene; PAO, polyamine oxidase; SAM, S-adenosylmethionine; SAMDC, SAM decarboxylase; SPDS, spermidine synthase; SPMS, spermine synthase.
S-adenosylmethionine is essential for conversion of putrescine (Put) into the higher polyamines (PAs) spermidine (Spd) and spermine (Spm), as well the production of ethylene (Figure 1). During the ripening of a bulky fruit such as tomato, there is an inverse relationship between the production of higher PAs and ethylene (Lasanajak et al., 2014), 1-MCP treatment inhibits autocatalytic ethylene production without affecting SAM levels (Van de Poel et al., 2013), and decreased levels of ethylene in RNAi-1-aminocyclpropane-1-carboxylate synthase fruits enhance PA levels and upregulate PA biosynthesis genes (Gupta et al., 2013). Research with non-bulky fruits such as rice grain also suggests interaction between PAs and ethylene in regulating plant growth and in response to environmental stress (Chen et al., 2013). Notably, there is a transient increase in the level of Put only, and no changes in the level of Spd, Spm, or total PAs in apple fruit stored at 24°C, even though the expression of only one of two SAM decarboxylases (SAMDCs) is repressed (Pang et al., 2006).
During CA storage 4-aminobutyrate (GABA) accumulates in apple fruit (Deewatthanawong and Watkins, 2010; Lee et al., 2012; Trobacher et al., 2013a), but it is uncertain whether this GABA is derived from PAs or glutamate (Shelp et al., 2012c; Figure 1). Notably, the levels of both PAs and GABA can be stimulated by abiotic stresses such as chilling, O2 deficiency and elevated CO2, conditions that are the basis of CA storage (Alcázar et al., 2010; Bitrián et al., 2012; Shelp et al., 2012a,b,c; Trobacher et al., 2013a).
Here, we tested the hypothesis that 1-MCP and CA storage enhances the levels of PAs and GABA in apple fruit imposed by abiotic stresses associated with CA storage. Since 1-MCP has been shown to increase the incidence of CO2-induced physiological injury in “Empire” fruit during CA storage (Watkins, 2006; Fawbush et al., 2008; Jung and Watkins, 2011), we utilized two levels of CO2 (2.5 and 0.03 kPa).
Materials and Methods
Apple Source and Controlled Atmosphere Storage Trials
Sixteen boxes (~20 kg each) of apple (Malus domestica Borkh. cv. Empire) fruit were harvested in the morning from a commercial orchard within 15 km of Simcoe, Ontario on 29 September 2011, and immediately transported to our post-harvest facility at the University of Guelph. On arrival, four fruit were randomly chosen, frozen as quickly as is practical in liquid N2 and stored at -80°C for future metabolite analysis. Then the boxes were divided into two treatments without or with 1 μL L-1 1-MCP (SmartFreshSM, AgroFresh Inc., Spring House, PA, supplied in sealed polybags) and stored at approximately 23°C for 1 day.
A split-plot design was used to eliminate the possibility of chamber effects. Fruit were stored in two random chambers that were set at 3°C; within each chamber, two rooms were supplied with 2.5 kPa O2 and either 2.5 kPa or 0.03 kPa CO2, giving a total of four rooms, each containing fruit treated without or with 1-MCP. The four rooms in each chamber were treated as biological replicates in this nested design.
About 90 fruit were stored in individual boxes in the CA rooms, which were sealed and flushed with 99 kPa N2. When the O2 concentration declined to 2.5 kPa, N2 flushing was replaced with CO2 until 2.5 or 0.03 kPa CO2 was achieved. Four fruit were collected from each box at eight different time points over the storage period (17 days, and 4, 8, 10, 14, 23, 33, and 46 weeks) following harvest, frozen in liquid N2 and stored at -80°C prior to metabolite analysis. Fruit were also collected for assessment of fruit quality, but these data will be reported elsewhere.
Metabolite Composition of Whole Apple Fruit
Polyamine analysis
For each treatment replicate, four whole frozen apples were cryogenically pulverized, taking care to ensure that the apple tissue did not thaw during the procedure (Trobacher et al., 2013a). PAs were essentially extracted and analyzed as described by Smith and Davies (1985) and Shiozaki et al. (2000). Briefly, 100 mg of fine frozen powder (comprised of 25 mg from each of the four apple subsamples in each treatment replicate) was homogenized in cold 5% perchloric acid (PCA, 100 mg mL-1), placed on ice for 30 min and then spiked with 10 μL of the internal standard 1,6 hexanediamine (0.25 nmol μL-1; Fisher Scientific, Whitby, ON, Canada). The sample was centrifuged for 20 min at 14,000 g at room temperature, and the supernatant was transferred to a 2-mL microfuge tube and maintained on ice for 30 min. The pellet was extracted again in 1 mL of 5% PCA and treated as above. For analysis of free and conjugated soluble polyamines, the two supernatants were combined and dried in vacuo at 60°C or under a stream of filtered air at room temperature, then redissolved in 0.4 mL of 5% PCA. One half of this extract was transferred to a 5-mL amber reacti-vial and derivatized for analysis of free PAs as described below. The remaining 0.2 mL was hydrolyzed with HCl as described below before derivatization. The soluble conjugated soluble polyamines were estimated as the difference between the hydrolyzed and original supernatants.
The final pellet was resuspended in 0.2 mL of 1 M NaOH and centrifuged for 5 min at 10,000 g. The supernatant was transferred to a 2-mL amber Eppendorf tube and hydrolyzed with 0.2 mL of 12 M HCl at room temperature for 18 h. The sample was then dried as above, resuspended in 0.2 mL of 5% PCA and derivatized for analysis of conjugated insoluble polyamines.
A 0.6 mL aliquot of dansyl chloride (Sigma Aldrich, Oakville, ON, Canada) in acetone (7.5 mg mL-1 acetone) was added to a 0.2-mL aliquot of the various PCA extracts. Then 0.3 mL of a saturated sodium carbonate solution was added with brief vortexing to give a pH of 10. The mixture was placed in a 60°C waterbath for 30 min (Gennaro et al., 1988). A 0.1-mL aliquot of proline (0.1 g mL-1; Sigma Aldrich) was added to the solution to remove excess dansyl chloride, and then the mixture was incubated in the dark for 15 min at room temperature. Dansylated PAs were extracted from the mixture by adding 0.5 mL toluene (Fisher Scientific, Whitby, ON, Canada) and vortexing for 1 min. Then, 0.4 mL was taken from the organic layer and dried under filtered air for 30 min. The dry residue was dissolved in 0.2 mL methanol and stored at 4°C in the dark for up to 2 weeks.
Dansylated PAs were passed through 0.45 um syringe filter and 20-μL aliquots injected onto a reverse-phase column (Agilent Zorbax ODS 5 μm, 4.6 mm × 150 mm) linked to an Agilent 1100 HPLC system (Allan and Shelp, 2006). Initially, 60% methanol was provided for 4 min at a flow rate of 1.5 mL min-1, followed by a linear increase to 95% methanol over 10 min and then 95% methanol for a further 5 min. The PAs were quantified with a fluorescence detector set at excitation and emission wavelengths of 254 and 500 nm, respectively (Gennaro et al., 1988). Although other peaks might appear on the chromatogram, they eluted before all dansylated PAs of interest, allowing for good resolution of Put (RT = 12.6 min), 1,6 hexanediamine (RT = 13.8 min), Spd (RT = 16.4 min), and Spm (RT = 18.5 min). Each treatment replicate was considered to be the average of the four subsamples.
The linearity of the standard curves up to 120 pmol for Put, Spd, Spm and 1,6 hexanediamine (Sigma Aldrich, Oakville, ON, Canada) was not affected by the addition of frozen apple powder tissue to the extraction procedure. The recovery of the internal standard across apple samples was approximately 60%; all samples were corrected for the actual loss during preparation. Furthermore, reverse-phase HPLC analysis of the PA standards and apple samples showed baseline separation of all PAs of interest. For routine analysis, a suite of external standards (80 pmol each) was run every sixth sample. The detection limit for the overall method was approximately 0.10 nmol g-1 fresh mass (FM).
Amino acid analysis
The amino acid composition of each apple was determined essentially as described previously (Allan and Shelp, 2006). Briefly, 1 g of the fine frozen powder was ground in four volumes of 30 g L-1 sulfosalicylic acid using a chilled mortar and pestle and fine silica sand, and 1.0 mL of the solution was centrifuged. The supernatant was adjusted to neutrality with NaOH, and then passed through a 0.45-μm syringe filter prior to on-line derivatization with o-phthalaldehyde. Aliquots (0.5 μL) of the supernatant were analyzed by reverse-phase HPLC. Each treatment replicate was considered to be the average of the four subsamples analyzed and each mean was the average of four treatment replicates. A suite of external amino acid standards (125 pmol each) derived from protein hydrolysate and the individual amino acids GABA, asparagine and glutamine were run every sixth sample.
Statistical analysis of data
All statistical analyses were conducted using SAS 9.2 at the α = 0.05 level (SAS Institute Inc., Cary, NC, USA). Replicate room effects were analyzed using analysis of variances (ANOVAs; proc mixed method), which partitioned variance into the fixed effects (1-MCP, temperature, CO2, storage time) and their interactions, and the random effect of chambers. In cases where interactions were significant (P ≤ 0.05), treatment means were compared within weeks (slice option) over the period from 17 days to 46 weeks using a Fisher’s protected least significant difference test. For experiments where interactions were significant (P ≤ 0.05), data were pooled across repeated measures to determine differences among treatments and over storage period using the Tukey’s test. All data were arcsine square root transformed to ensure a normal distribution of variance and the treatment means were back-transformed for presentation.
Results
Total apple PAs consisted of free, soluble conjugated, and insoluble conjugated forms, with free and soluble conjugated forms being at much higher concentrations than the insoluble conjugated forms (Figures 2A–D). The concentrations of all forms fluctuated considerably during the 1-MCP treatment and the initial collection periods at 17 days and 4 weeks, but were much steadier from 8 to 46 weeks. Therefore, statistical analysis of the main effects and interactions was conducted for the entire treatment period, whereas detailed comparisons between the 1-MCP treatments were made over the 8–46 week period. Furthermore, the total PAs were comprised primarily of total Put and total Spd, followed by total Spm (Figures 3–5, panel A). The concentrations of individual PAs consisted mainly of the free form, followed closely by the soluble conjugated form, and more distantly by the insoluble conjugated form (Figures 3–5, panels B–D). Notably, the concentrations of the free forms tended to fluctuate less than the conjugated forms.
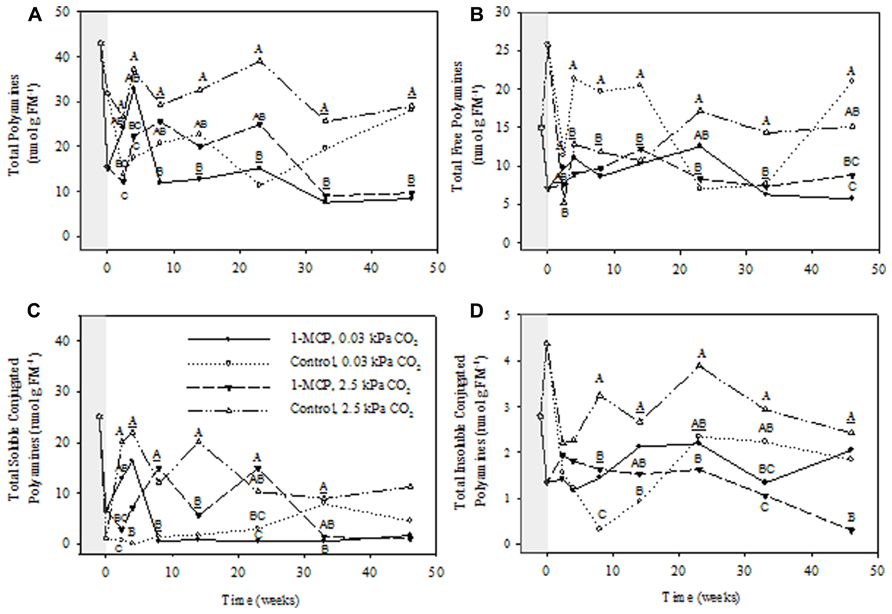
FIGURE 2. Effects of 1-MCP and elevated CO2 on total (A), total free (B), total soluble conjugated (C), and total insoluble conjugated (D) polyamines in “Empire” fruit during controlled atmosphere storage. With the exception of the beginning and end of 1-MCP treatment (-1 day to 0 week, the shaded area), all data are mean estimates of four storage replicates. Different letter groupings indicate significant differences between treatments within weeks (P ≤ 0.05). Underlined letters indicate a shared letter for overlapping data; where letters are absent at a time point, there were no significant differences.
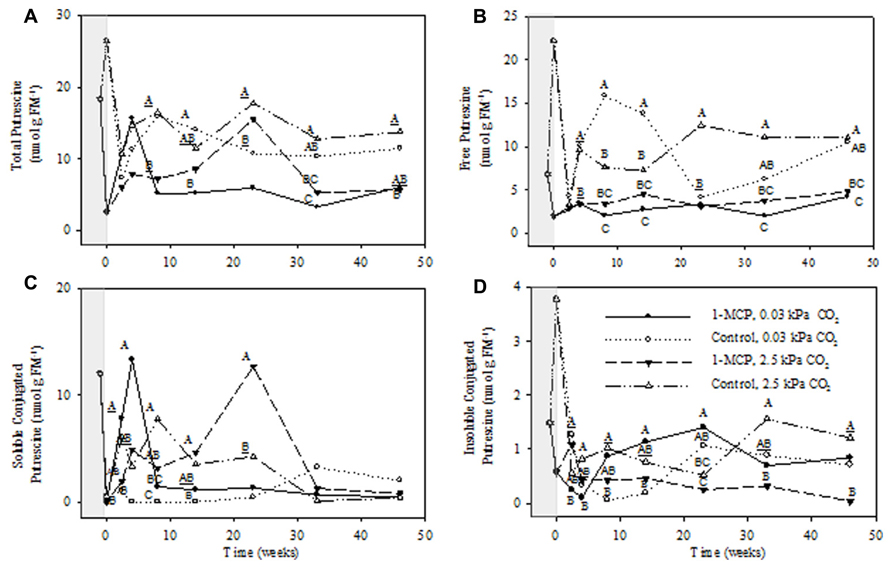
FIGURE 3. Effects of 1-MCP and elevated CO2 on total (A), free (B), soluble conjugated (C), and insoluble conjugated (D) putrescine in “Empire” fruit during controlled atmosphere storage. With the exception of the beginning and end of 1-MCP treatment (-1 day to 0 week, the shaded area), all data are mean estimates of four storage replicates. Different letter groupings indicate significant differences between treatments within weeks (P ≤ 0.05). Underlined letters indicate a shared letter for overlapping data; where letters are absent at a time point, there were no significant differences.
The concentrations of total PAs showed significant storage time, CO2 and 1-MCP main effects, as well as storage time × CO2, 1-MCP × CO2, and storage time × CO2 × 1-MCP interactions (P ≤ 0.05, ANOVA table not shown). During the period from 8 to 46 weeks, the total PA concentrations in 1-MCP-treated fruit at 2.5 kPa CO2 were, with the exception of 8 and 23 weeks, 33–61% of those in control fruit (i.e., not treated with 1-MCP), whereas at 0.03 kPa CO2 they were 29–39% of those at 33 and 46 weeks (Figure 2A). The concentrations of total free PAs showed significant storage time and CO2 main effects, as well as storage time × 1-MCP, and storage time × 1-MCP × CO2 interactions (P ≤ 0.05, ANOVA table not shown). At 2.5 kPa CO2 the total free PA concentrations in 1-MCP-treated fruit were 49–51% of those in control fruit at 23 and 33 weeks, whereas at 0.03 kPa CO2 they were, with the exception of weeks 23 and 33, 27–52% of those in control fruit (Figure 2B). The concentrations of total soluble conjugated PAs showed significant CO2 and 1-MCP main effects, as well as CO2 × 1-MCP, storage time × CO2 × 1-MCP interactions (P ≤ 0.05, ANOVA table not shown). At 2.5 kPa CO2, the concentrations of total soluble conjugated PAs in 1-MCP-treated fruit were similar to those in control fruit, whereas at 0.03 kPa CO2 they were 6% of that in the control at 33 weeks (Figure 2C). The concentrations of total insoluble conjugated PAs showed significant CO2 and 1-MCP main effects, and storage time × 1-MCP, CO2 × 1-MCP interactions (P ≤ 0.05, ANOVA table not shown). At 2.5 kPa CO2 the concentrations of total insoluble conjugated PA concentrations in 1-MCP- treated fruit were, with the exception of week 14, 13–57% of those in control fruit, whereas at 0.03 kPa CO2 they were 130–350% greater at 8 and 14 weeks (Figure 2D).
The concentrations of total Put displayed significant CO2 main effects, but no interactions (P ≤ 0.05, ANOVA table not shown). The total Put concentrations at 2.5 and 0.03 kPa CO2 in 1-MCP-treated fruit were 41–44% and 32–37% of those in control fruit at 8, 33, and 46 weeks and at 8, 14, and 33 weeks, respectively (Figure 3A). There were significant storage time and CO2 main effects on the concentrations of free Put, as well as storage time × CO2 × 1-MCP interaction (P ≤ 0.05, ANOVA table not shown). At 2.5 kPa CO2 the free Put concentrations in 1-MCP-treated fruit were 25–44% of those in control fruit at 23, 33, and 46 weeks, whereas at 0.03 kPa CO2 they were, with the exception of week 23, 13–40% of the controls (Figure 3B). The concentrations of soluble conjugated Put showed significant 1-MCP and CO2 main effects, as well as storage time × 1-MCP and storage time × CO2 × 1-MCP interactions (P ≤ 0.05, ANOVA table not shown). At 2.5 kPa CO2 the concentrations of soluble conjugated Put in 1-MCP-treated fruit were 200% greater than those in control fruit at 23 weeks, whereas at 0.03 kPa CO2 there were no significant differences among treatments (Figure 3C). The concentrations of insoluble conjugated Put showed significant CO2 main effects, as well as storage time × CO2, storage time × 1-MCP, CO2 × 1-MCP and storage time × CO2 × 1-MCP interactions (P ≤ 0.05, ANOVA table not shown). At 2.5 kPa CO2 the concentrations of insoluble conjugated Put in 1-MCP-treated fruit were 3–20% of those in control fruit at 33 and 46 weeks, whereas at 0.03 kPa CO2 they were 490–1420% greater than controls at 8 and 14 weeks (Figure 3D).
The total Spd concentrations displayed significant storage time, CO2 and 1-MCP main effects, as well as storage time × CO2, storage time × 1-MCP, and CO2 × 1-MCP interactions (P ≤ 0.05, ANOVA table not shown). At 2.5 kPa CO2 total Spd concentrations in 1-MCP-treated fruit were 25–52% of those in control fruit at 14, 33, and 46 weeks, whereas at 0.03 kPa CO2 there were no significant differences between treatments (Figure 4A). Free Spd concentrations showed significant storage time, CO2, and 1-MCP main effects, as well as storage time × CO2, storage time × 1-MCP, and storage time × CO2 × 1-MCP interactions (P ≤ 0.05, ANOVA table not shown). At 2.5 kPa CO2 free Spd concentrations in 1-MCP-treated fruit were 121% greater than those in control fruit at 14 weeks, whereas at 0.03 kPa CO2 they were 230–350% greater at 8, 23, and 33 weeks and 49% of the control at 46 weeks (Figure 4B). The concentrations of soluble conjugated Spd displayed CO2 and 1-MCP main effects, and storage time × CO2, CO2 × 1-MCP and storage time × CO2 × 1-MCP interactions (P ≤ 0.05, ANOVA table not shown). At 2.5 kPa CO2 the concentrations of soluble conjugated Spd in 1-MCP-treated fruit never exceeded 4% of those at 14, 33, and 46 weeks of storage, whereas at 0.03 kPa CO2, they were undetectable at 33 weeks of storage (Figure 4C). The concentrations of insoluble conjugated Spd showed significant storage time and 1-MCP main effects and storage time × 1-MCP interactions (P ≤ 0.05, ANOVA table not shown). At 2.5 and 0.03 kPa CO2, there were no significant differences between the 1-MCP treatments (Figure 4D).
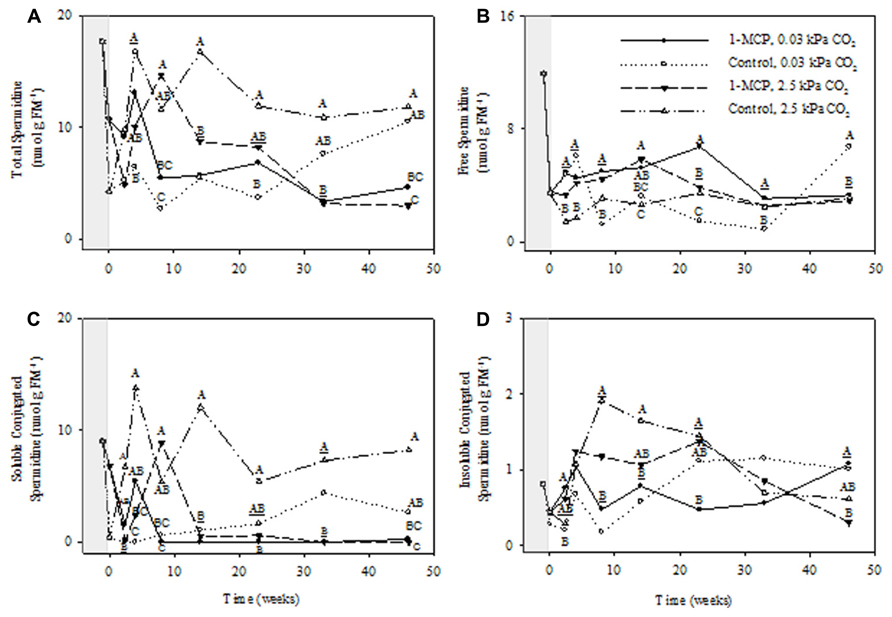
FIGURE 4. Effects of 1-MCP and elevated CO2 on total (A), free (B), soluble conjugated (C), and insoluble conjugated (D) spermidine in “Empire” fruit during controlled atmosphere storage. With the exception of the beginning and end of 1-MCP treatment (-1 day to 0 week, the shaded area), all data are mean estimates of four storage replicates. Different letter groupings indicate significant differences between treatments within weeks (P ≤ 0.05). Underlined letters indicate a shared letter for overlapping data; where letters are absent at a time point, there were no significant differences.
The concentrations of total Spm showed significant storage time and CO2 main effects, as well as CO2 × 1-MCP and storage time × CO2 × 1-MCP interactions (P ≤ 0.05, ANOVA table not shown). At 2.5 kPa CO2 total Spm concentrations in 1-MCP-treated fruit were 140% greater than those that in control fruit at 8 weeks, but 34% of the control at 23 weeks (Figure 5A). There were no differences between treatments at 0.03 kPa CO2. The concentration of free Spm showed significant storage time and 1-MCP main effects (P ≤ 0.05, ANOVA table not shown). At 2.5 kPa CO2 there was no significant differences between the treatments, whereas at 0.03 kPa CO2 the free Spm concentration was 260% greater in 1-MCP-treated fruit than that in control fruit at 23 weeks (Figure 5B). There were significant CO2 and 1-MCP main effects, as well as storage time × CO2, CO2 × 1-MCP and storage time × CO2 × 1-MCP interactions, for concentrations of soluble conjugated Spm (P ≤ 0.05, ANOVA table not shown). At 2.5 kPa CO2 the concentration of soluble conjugated Spm in 1-MCP-treated fruit was much greater than that in control fruit at 8 weeks, but less than 3% of those at 14 and 23 weeks, whereas at 0.03 kPa CO2 there were no differences between 1-MCP treatments (Figure 5C). The concentrations of insoluble conjugated Spm showed significant CO2 and 1-MCP main effects, as well as storage time × CO2, CO2 × 1-MCP interactions (P ≤ 0.05, ANOVA table not shown). At 2.5 kPa CO2 the concentrations of insoluble conjugated Spm in 1-MCP-treated fruit never exceeded 18% of those in control fruit over the 8- to 46-week period; however, at 0.03 kPa CO2 the concentrations were 280–1250% greater at 8, 14, and 23 weeks (Figure 5D).
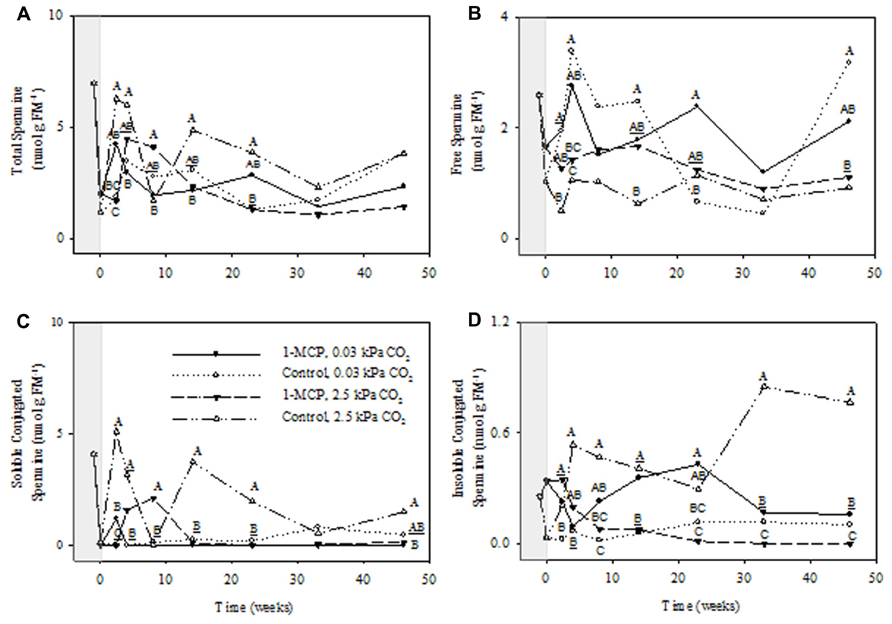
FIGURE 5. Effects of 1-MCP and elevated CO2 on total (A), free (B), soluble conjugated (C), and insoluble conjugated (D) spermine in “Empire” fruit during controlled atmosphere storage. With the exception of the beginning and end of 1-MCP treatment (-1 day to 0 week, the shaded area), all data are mean estimates of four storage replicates. Different letter groupings indicate significant differences between treatments within weeks (P ≤ 0.05). Underlined letters indicate a shared letter for overlapping data; where letters are absent at a time point, there were no significant differences.
Regardless of 1-MCP and CO2 treatments GABA accumulated in a linear fashion over the storage period (Figure 6A), whereas glutamate declined after an initial rise (Figure 6B). Notably, fruit receiving 2.5 kPa CO2 and 1-MCP accumulated twice as much GABA (~2 nmol g-1 FM week-1) as fruit receiving 0.03 kPa CO2 only, accounting for approximately one half of the decline in glutamate over the same period. The GABA concentrations displayed significant storage time, CO2 and 1-MCP main effects, as well as storage time × 1-MCP interactions (P ≤ 0.05, ANOVA table not shown). At 2.5 and 0.03 kPa CO2 the GABA concentrations in 1-MCP-treated fruit were 19 and 25% greater, respectively, than those in control fruit at 33 weeks (Figure 6A). Glutamate showed significant storage time, CO2 and 1-MCP main effects, as well as storage time × 1-MCP, CO2 × 1-MCP interactions (P ≤ 0.05, ANOVA table not shown). At 2.5 kPa CO2 glutamate concentrations in 1-MCP-treated fruit were 34–38% greater than those in control fruit at 14 and 23 weeks, whereas at 0.03 kPa CO2 they were 8–57% greater than those in control fruit over the storage period (Figure 6B).
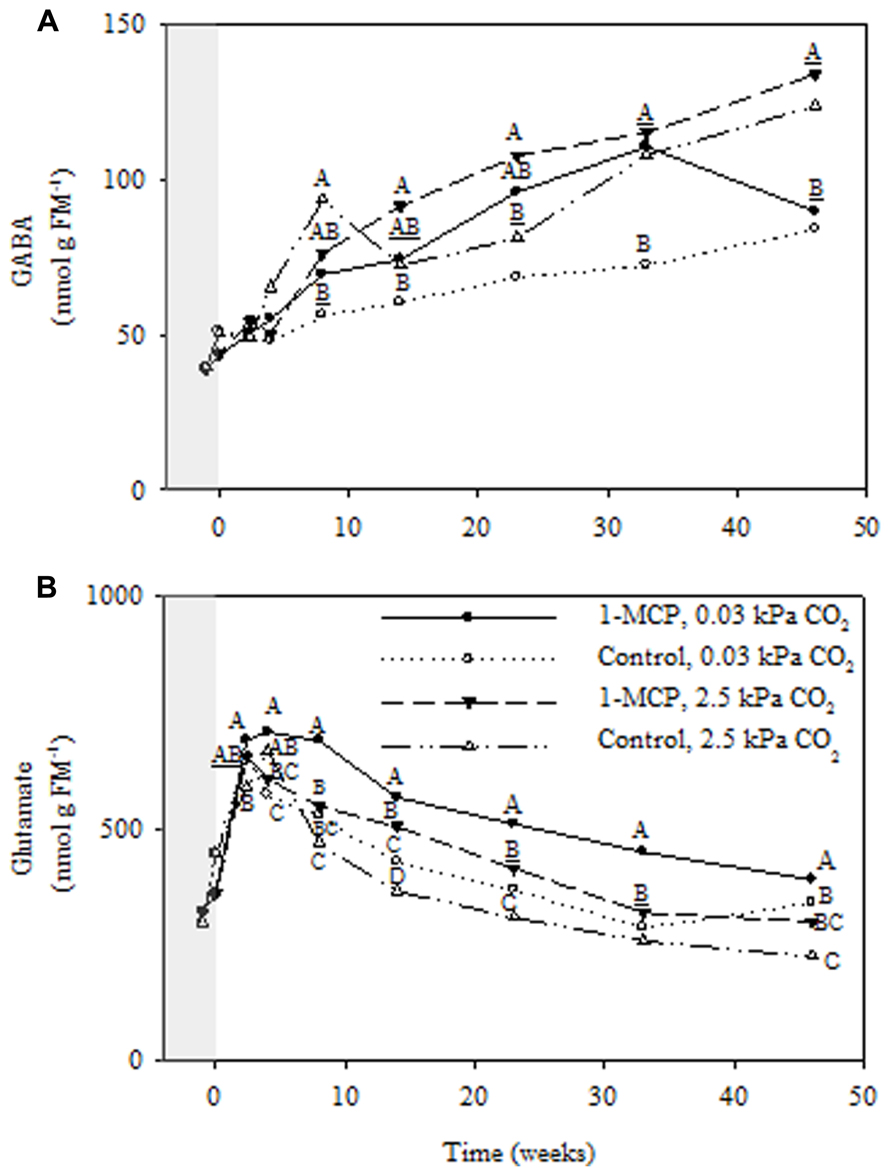
FIGURE 6. Effects of 1-MCP and elevated CO2 on GABA (A) and glutamate (B) levels in “Empire” fruit during controlled atmosphere storage. With the exception of the beginning and end of 1-MCP treatment (-1 day to 0 week, the shaded area), all data are mean estimates of four storage replicates. Different letter groupings indicate significant differences between treatments within weeks (P ≤ 0.05). Underlined letters indicate a shared letter for overlapping data; where letters are absent at a time point, there were no significant differences.
Discussion
Both PAs and GABA appear to function in various physiological processes such as stress responses and growth and development (Alcázar et al., 2010; Fincato et al., 2012; Moschou et al., 2012; Shelp et al., 2012b,c). The presence and interconversion of free forms of Put, Spd and Spm have been the focus of PA research, but soluble (i.e., bound to small molecules such as phenolic acids) and insoluble (i.e., bound to macromolecules such as nucleic acids and proteins) conjugated forms also exist. The ratios between free and conjugated PAs vary among plant species (Bagni and Tassoni, 2001), and some evidence exists for interconversion between free and conjugated PAs (Bassard et al., 2010), which could influence our interpretation of the impact of 1-MCP and CA on PAs and possibly GABA in apple fruit (Figure 1). Interestingly, it has been suggested that the stress hormone abscisic acid contributes to the conversion of conjugated forms of PA to the free forms (Ben Hassine et al., 2009).
In the present study, the concentrations of free and conjugated forms of PAs were determined in ripening 1-MCP-treated “Empire” apple fruit over a 46-week storage period under CA conditions. Initially, there were marked fluctuations in the concentrations of these PAs as the fruit acclimated to the shift in environmental conditions. Steady-state levels were evident after approximately 8 weeks of storage. It is well known that 1-MCP treatment should inhibit the autocatalytic production of ethylene in apple fruit (Pang et al., 2006; DeEll et al., 2008; Fawbush et al., 2008; Jung and Watkins, 2011), reduce the expression of genes involved in ethylene biosynthesis (Dal Cin et al., 2006; Pang et al., 2006; Vilaplana et al., 2007), and decrease the level of ACC (Bulens et al., 2012). However, we found no evidence for elevated levels of total PAs, Put, Spd, or Spm during the steady-state period (8–46 weeks) in response to 1-MCP treatment; indeed the levels tended to be lower regardless of CO2 (Figures 2–5), suggesting that the availability of SAM for PA biosynthesis in apple fruit was not influenced by the 1-MCP treatment. These findings are consistent with previous studies of ripening apple and tomato fruits, which suggest that the requirement for SAM in PA biosynthesis is not limited by the requirement in ethylene biosynthesis (Van de Poel et al., 2013; Lasanajak et al., 2014), and suggest an unknown biochemical or transcriptional mechanism, possibly altering the PA biosynthetic rates from glutamate (Alcázar et al., 2010; Majumdar et al., 2013), was responsible for the lower total PA levels in 1-MCP-treated fruit under CA storage. However, these findings are at odds with the generally accepted view that PAs, especially Spd and Spm, accumulate in respond to abiotic stress (Groppa and Benavides, 2008; Bassard et al., 2010; Shelp et al., 2012c), which could be due to a variety of reasons: that view is generally based on free PA levels, rather than total PAs; apple fruit experience multiple stresses during CA storage; and, ripening apple fruit, like tomato fruit (Mattoo et al., 2010), are at a terminal developmental stage.
1-Methylcyclopropene application changed the levels of individual PAs and the relative proportions of free and conjugated forms. Total Put levels tended to be lower with 1-MCP regardless of the CO2 level, and while this was mostly at the expense of free Put, large transient increases in soluble conjugated Put were also evident (Figure 3). Total Spd levels tended to be lower with 1-MCP, particularly at 2.5 kPa CO2, and this was mostly at the expense of soluble conjugated Spd (Figure 4). Total Spm levels at 2.5 kPa CO2 tended to be lower with 1-MCP, and this was mostly at the expense of both soluble and insoluble conjugated Spm (Figure 5). Overall, total Spd and Spm levels at 0.03 kPa were relatively unaffected, compared to 2.5 kPa CO2, but transient increases in free Spd and Spm were evident. Thus, 1-MCP treatment reduced the accumulation of individual PAs with elevated CO2 under CA conditions; however, there was a general decrease and increase in the ratio of free:conjugated forms for Put and Spd plus Spm, respectively. The accumulation of PAs was unaffected under low CO2, but there was some evidence for accumulation of conjugated Spd and Spm. Since total PA levels declined with 1-MCP (Figure 2), this differential response of Put and Spd plus Spm in the partitioning between free and conjugated forms could not be attributed to altered SAM availability. The response might be attributed, at least in part, to changes in the conversion and back-conversion of PAs, and the interconversion of free and conjugated forms in apple fruit, although the activities of polyamine oxidases (PAOs) could be limited by the low O2 status of intact apple fruit stored under CA conditions (Ho et al., 2011; Shelp et al., 2012c).
Glutamate serves a precursor for biosynthesis of both GABA and PAs, raising the possibility that GABA in CA-stored apple fruit can be derived directly from glutamate or indirectly from free Put or free Spd (Figure 1). Regardless of 1-MCP and CO2 treatments, the availability of glutamate showed a transient peak initially, probably due to protein degradation (Magné et al., 1997; Sugimoto et al., 2011), and this was followed by a steady decline over the remainder of the storage period which coincided with accumulating GABA (Figure 6). This pattern could be attributed due to the stimulation of glutamate decarboxylase (GAD) activities via bound Ca2+/calmodulin or lower cytosolic pH (Shelp et al., 2012a; Trobacher et al., 2013b), and product inhibition of GABA transaminase activity (Clark et al., 2009) due to the restricted activity of succinic semialdehyde dehydrogenase activity under O2 deficiency (Busch and Fromm, 1999; Shelp et al., 1999). GABA accumulation was lowest at 0.03 kPa CO2 and noticeably greater with either 1-MCP or 2.5 kPa CO2. These findings are consistent with previous research showing that GABA accumulates in tissues of “Empire” apple fruit during CA storage with elevated CO2 and is then catabolized when the fruit are transferred to ambient conditions (Deewatthanawong and Watkins, 2010; Lee et al., 2012; Trobacher et al., 2013a). Relatively higher levels of free Put and Spd than free Spm are also consistent with studies of other species and tissues (Mattoo et al., 2010). Interestingly, the changing levels of Spd seemed to be more highly correlated than those of Put with the rate of GABA production regardless of the 1-MCP and CO2 treatments, suggesting that Spd could be in steady-state equilibrium with GABA. It has been argued that changes in O2 availability and cellular redox balance in apple fruit stored under CA conditions would directly influence the activity of 4-aminobutyraldehyde dehydrogenase, as well as diamine oxidases (DAO), thereby restricting GABA formation from both Spd and Put (Shelp et al., 2012c). Unfortunately, pool sizes do not indicate the flux through a pathway, mutants of the metabolic routes for GABA and PAs are not readily available for apple, and radiolabelled precursors cannot be supplied to intact apples without perturbing the internal gaseous environment, which would most certainly affect GABA formation from glutamate. Useful information about the relative contributions of glutamate and PAs to GABA production in intact apple fruit could be gained via a combination of metabolite and gene transcript analyses. Recently, use of the DAO inhibitor aminoguanidine suggested that approximately 30% of the GABA accumulated in fava bean seed germinating under hypoxia is derived from PAs (Yang et al., 2013). Notably, the free levels of the three PAs, especially Put, increased, unlike the case reported here, and this was accompanied by some loss of GAD activity, which was attributed to growth inhibition.
While uncertainty continues regarding the relationship between PA and ethylene biosynthesis in fruits, especially those exposed to multiple abiotic stresses, our research indicates that the requirement for SAM in PA biosynthesis in CA-stored apple fruit was probably not limited by the requirement in ethylene biosynthesis. Moreover, the differential response of PA partitioning between free and conjugated forms could be attributed to changes in the interconversion of free and conjugated forms and the conversion and back-conversion of PAs. Also, the data could be interpreted as preliminary evidence for a relationship between Spd level and GABA production, but it is argued that the biochemical reactions involved would be limited by the in vivo O2 level, and that elevated GAD activity and product inhibition of GABA transaminase activity would be responsible for GABA accumulation in these fruit.
Conflict of Interest Statement
This research was funded in part by AgroFresh Inc., the manufacturer of SmartFreshSM.
Acknowledgments
The authors thank Dr. Gordon J. Hoover for technical assistance with HPLC analysis. The financial support of the Natural Sciences and Engineering Research Council (NSERC) of Canada (Strategic Project and Individual Discovery Grants), the Ontario Ministry of Agriculture Food and Rural Affairs, and Rohm and Haas Canada LP (AgroFresh Inc.) is gratefully acknowledged.
References
Alcázar, R., Altabella, T., Marco, F., Bortolotti, C., Reymond, M., Koncz, C., et al. (2010). Polyamines: molecules with regulatory functions in plant abiotic stress tolerance. Planta 231, 1237–1249. doi: 10.1007/s00425-010-1130-0
Allan, W. L., and Shelp, B. J. (2006). Fluctuations of γ-aminobutyrate, γ-hydroxybutyrate, and related amino acids in Arabidopsis leaves as a function of the light-dark cycle, leaf age, and N stress. Can. J. Bot. 84, 1339–1346. doi: 10.1139/b06-093
Bagni, N., and Tassoni, A. (2001). Biosynthesis, oxidation and conjugation of aliphatic polyamines in higher plants. Amino Acids 20, 301–317. doi: 10.1007/s007260170046
Bassard, J. E., Ullmann, P., Bernier, F., and Werck-Reichhart, D. (2010). Phenolamides: bridging polyamines to the phenolic metabolism. Phytochemistry 71, 1808–1824. doi: 10.1016/j.phytochem.2010.08.003
Ben Hassine, A., Ghanem, M. E., Bouzid, S., and Lutts, S. (2009). Abscisic acid has contrasting effects on salt excretion and polyamine concentrations of an inland and a coastal population of the Mediterranean xero-halophyte species Atriplex halimus. Ann. Bot. 104, 925–936. doi: 10.1093/aob/mcp174
Bitrián, M., Zarza, X., Altabella, T., Tiburico, A. F., and Alcázar, R. (2012). Polyamines under abiotic stress: metabolic crossroads and hormonal crosstalks in plants. Metabolites 2, 516–528. doi: 10.3390/metabo2030516
Bulens, B., Van de Poel, B., Hertog, M. L. A. T. M., De Proft, M. P., Geeraerd, A. H., and Nicolai, B. M. (2012). Influence of harvest time and 1-MCP application on postharvest ripening and ethylene biosynthesis of ‘Jonagold’ apple. Postharvest Biol. Technol. 72, 11–19. doi: 10.1016/j.postharvbio.2012.05.002
Busch, K. B., and Fromm, H. (1999). Plant succinic semialdehyde dehydrogenase. Cloning, purification, localization in mitochondria, and regulation by adenine nucleotides. Plant Physiol. 121, 589–597. doi: 10.1104/pp.121.2.589
Chen, T., Xu, Y., Wang, J., Wang, Z., Yang, J., and Zhang, J. (2013). Polyamines and ethylene interact in rice grains in response to soul drying during grain filling. J. Exp. Bot. 64, 2523–2538. doi: 10.1093/jxb/ert115
Clark, S. M., Di Leo, R., Dhanoa, P. K., Van Cauwenberghe, O. R., Mullen, R. T., and Shelp, B. J. (2009). Biochemical characterization, mitochondrial localization, expression, and potential functions for an Arabidopsis γ-aminobutyrate transaminase that utilizes both pyruvate and glyoxylate. J. Exp. Bot. 60, 1743–1757. doi: 10.1093/jxb/erp044
Dal Cin, V., Rizzini, F. M., Botton, A., and Tonutti, P. (2006). The ethylene biosynthetic and signal transduction pathways are differently affected by 1-MCP in apple and peach fruit. Postharvest Biol. Technol. 42, 125–133. doi: 10.1016/j.postharvbio.2006.06.008
DeEll, J., Ayres, J., and Murr, D. (2008). 1-Methylcyclopropene concentration and timing of postharvest application alters the ripening of ‘McIntosh’ apples during storage. Horttechnology 18, 624–630.
Deewatthanawong, R., and Watkins, C. B. (2010). Accumulation of γ-aminobutyric acid in apple, strawberry and tomato fruit in response to postharvest treatments. Acta Hortic. 877, 947–952.
Fawbush, F., Nock, J. F., and Watkins, C. B. (2008). External carbon dioxide injury and 1-methylcyclopropene (1-MCP) in the ‘Empire’ apple. Postharvest Biol. Technol. 48, 92–98. doi: 10.1016/j.postharvbio.2007.09.005
Fincato, P., Moschou, P. N., Ahou, A., Angelini, R., Roubelakis, K. A., Federico, R., et al. (2012). The members of Arabidopsis thaliana PAO gene family exhibit distinct tissue- and organ-specific expression pattern during seedling growth and flower development. Amino Acids 42, 831–841. doi: 10.1007/s00726-011-0999-7
Gennaro, M. C., Mentasti, E., Sarzanini, C., and Porta, V. (1988). Aliphatic monoamines, diamines, polyamines: an HPLC method for their identification and separation by a dansylation reaction; the study of separation factors in homologous series. Chromatographia 25, 117–124. doi: 10.1007/BF02259027
Groppa, M. D., and Benavides, M. P. (2008). Polyamines and abiotic stress: recent advances. Amino Acids 34, 35–43. doi: 10.1007/s00726-007-0501-8
Gupta, A., Pal, R. K., and Rajam, M. V. (2013). Delayed ripening and improved fruit processing in tomato by RNAi-mediated silencing of three homologs of 1-aminopropane-1-carboxylate synthase gene. J. Plant Physiol. 170, 987–995. doi: 10.1016/j.jplph.2013.02.003
Ho, Q. T., Verboven, P., Verlinden, B. E., Herremans, E., Wevers, M., Carmeliet, J., et al. (2011). A three dimensional multiscale model for gas exchange in fruit. Plant Physiol. 155, 1158–1168. doi: 10.1104/pp.110.169391
Jung, S. K., and Watkins, C. B. (2011). Involvement of ethylene in browning development of controlled atmosphere-stored ‘Empire’ apple fruit. Postharvest Biol. Technol. 59, 219–226. doi: 10.1016/j.postharvbio.2010.08.019
Lasanajak, Y., Minocha, R., Minocha, S. C., Goyal, R., Fatima, T., Handa, A. K., et al. (2014). Enhanced flux of substrates into polyamine but not ethylene in tomato fruit engineered with yeast S-adenosylmethionine decarboxylase gene. Amino Acids 46, 729–742. doi: 10.1007/s00726-013-1624-8
Lee, J., Rudell, D. R., Davies, P. J., and Watkins, C. B. (2012). Metabolic changes in 1-methylcyclopropene (1-MCP)-treated ‘Empire’ apple fruit during storage. Metabolomics 8, 742–753. doi: 10.1007/s11306-011-0373-5
Magné, C., Bonenfant-Magné, M., and Audran, J.-C. (1997). Nitrogenous indicators of postharvest ripening and senescence in apple fruit (Malus domestica Borkh. cv. Granny Smith). Int. J. Plant Sci. 158, 811–817. doi: 10.1086/297494
Majumdar, R., Shao, L., Miocha, R., Long, S., and Minocha, S. C. (2013). Ornithine: the overlooked molecule in the regulation of polyamine metabolism. Plant Cell Physiol. 54, 990–1004. doi: 10.1093/pcp/pct053
Mattoo, A. K., Minocha, S. C., Minocha, R., and Handa, A. K. (2010). Polyamines and cellular metabolism in plants: transgenic approaches reveal different responses to diamine putrescine versus higher polyamines spermidine and spermine. Amino Acids 38, 405–413. doi: 10.1007/s00726-009-0399-4
Moschou, P. N., Wu, J., Cona, A., Tavladoraki, P., Angelini, R., and Roubelakis-Angelakis, K. A. (2012). The polyamines and their catabolic products are significant players in the turnover of nitrogenous molecules in plants. J. Exp. Bot. 63, 5003–5015. doi: 10.1093/jxb/ers202
Pang, X.-M., Nada, K., Liu, J.-H., Kitashiba, H., Honda, C., Yamashita, H., et al. (2006). Interrrelationships between polyamine and ethylene in 1-methylcycopropene treated apple fruits after harvest. Physiol. Plant. 128, 351–359. doi: 10.1111/j.1399-3054.2006.00748.x
Shelp, B. J., Bown, A. W., and McLean, M. D. (1999). Metabolism and functions of gamma-aminobutyric acid. Trends Plant Sci. 4, 446–452. doi: 10.1016/S1360-1385(99)01486-7
Shelp, B. J., Bozzo, G. G., Trobacher, C. P., Chiu, G., and Bajwa, V. S. (2012a). Strategies and tools for studying the metabolism and function of γ-aminobutyrate in plants: I. Pathway structure. Botany 90, 651–668. doi: 10.1139/b2012-030
Shelp, B. J., Bozzo, G. G., Zarei, A., Simpson, J. P., Trobacher, C. P., and Allan, W. L. (2012b). Strategies and tools for studying the metabolism and function of γ-aminobutyrate in plants: II. Integrated analysis. Botany 90, 781–793. doi: 10.1139/b2012-041
Shelp, B. J., Bozzo, G. G., Trobacher, C. P., Zarei, A., Deyman, K. L., and Brikis, C. J. (2012c). Hypothesis/review: contribution of putrescine to 4-aminobutyrate (GABA) production in response to abiotic stress. Plant Sci. 193–194, 130–135. doi: 10.1016/j.plantsci.2012.06.001
Shiozaki, S., Ogata, T., and Horiuchi, S. (2000). Endogenous polyamines in the pericap and seed of the grape berry during development and ripening. Sci. Hortic. 83, 33–41. doi: 10.1016/S0304-4238(99)00064-3
Smith, M. A., and Davies, P. J. (1985). Separation and quantitation of polyamines in plant tissue by high performance liquid chromatography of their dansyl derivatives. Plant Physiol. 78, 89–91. doi: 10.1104/pp.78.1.89
Sugimoto, N., Jones, A. D., and Beaudry, R. (2011). Changes in free amino acid content in ‘Jonagold’ apple fruit as related to branched-chain ester production, ripening and senescence. J. Am. Soc. Hortic. Sci. 136, 429–440.
Trobacher, C. P., Clark, S. M., Bozzo, G. G., Mullen, R. T., DeEll, J. R., and Shelp, B. J. (2013a). Catabolism of GABA in apple fruit: subcellular localization and biochemical characterization of two γ-aminobutyrate transaminases. Postharvest Biol. Technol. 75, 106–113. doi: 10.1016/j.postharvbio.2012.08.005
Trobacher, C. P., Zarei, A., Liu, J., Clark, S. M., Bozzo, G. G., and Shelp, B. J. (2013b). Calmodulin-dependent and calmodulin-independent glutamate decarboxylases in apple fruit. BMC Plant Biol. 13:144. doi: 10.1186/1471-2229-13-144
Van de Poel, B., Bulens, I., Oppermann, Y., Hertog, M. L. A. T. M., Nicolai, B. M., Sauter, M., et al. (2013). S-adenosyl-L-methionine usage during climacteric ripening of tomato in relation to ethylene and polyamine biosynthesis and transmethylation capacity. Physiol. Plant. 148, 176–188. doi: 10.1111/j.1399-3054.2012.01703.x
Vilaplana, R., Soria, Y., Valentines, M. C., and Larrigaudière, C. (2007). Specific response of apple skin and pulp tissues to cold stress and 1-MCP treatment. Postharvest Biol Technol. 43, 215–220. doi: 10.1016/j.postharvbio.2006.09.003
Watkins, C. B. (2006). The use of 1-methylcyclopropene (1-MCP) on fruits and vegetables. Biotechnol. Adv. 24, 389–409. doi: 10.1016/j.biotechadv.2006.01.005
Watkins, C. B. (2008). Overview of 1-methylcyclopropene trials and uses for edible horticultural crops. HortScience 43, 86–94.
Keywords: abiotic stress, apple fruit, controlled atmosphere storage, 4-aminobutyrate (GABA), 1-methylcyclopropene (1-MCP), high performance liquid chromatography, polyamines
Citation: Deyman KL, Brikis CJ, Bozzo GG and Shelp BJ (2014) Impact of 1-methylcyclopropene and controlled atmosphere storage on polyamine and 4-aminobutyrate levels in “Empire” apple fruit. Front. Plant Sci. 5:144. doi: 10.3389/fpls.2014.00144
Received: 11 January 2014; Accepted: 25 March 2014;
Published online: 10 April 2014.
Edited by:
Antonio F. Tiburcio, Universitat de Barcelona, SpainReviewed by:
Subhash C. Minocha, University of New Hampshire, USAManchikatla Venkat Rajam, University of Delhi South Campus, India
Copyright © 2014 Deyman, Brikis, Bozzo and Shelp. This is an open-access article distributed under the terms of the Creative Commons Attribution License (CC BY). The use, distribution or reproduction in other forums is permitted, provided the original author(s) or licensor are credited and that the original publication in this journal is cited, in accordance with accepted academic practice. No use, distribution or reproduction is permitted which does not comply with these terms.
*Correspondence: Barry J. Shelp, Department of Plant Agriculture, Bovey Building, University of Guelph, 50 Stone Road East, Guelph, ON N1G 2W1, Canada e-mail:YnNoZWxwQHVvZ3VlbHBoLmNh