- 1Department of Marine Biology, Texas A&M University at Galveston, Galveston, TX, USA
- 2Red de Ecología Funcional, Instituto de Ecología, A.C., Xalapa, México
Over the last several decades, the distribution of the black mangrove Avicennia germinans in the Gulf of Mexico has expanded, in part because it can survive the occasional freeze events and high soil salinities characteristic of the area. Vessel architecture may influence mangrove chilling and salinity tolerance. We surveyed populations of A. germinans throughout the Gulf to determine if vessel architecture was linked to field environmental conditions. We measured vessel density, hydraulically weighted vessel diameter, potential conductance capacity, and maximum tensile fracture stress. At each sampling site we recorded mangrove canopy height and soil salinity, and determined average minimum winter temperature from archived weather records. At a subset of sites, we measured carbon fixation rates using a LI-COR 6400XT Portable Photosynthesis System. Populations of A. germinans from cooler areas (Texas and Louisiana) had narrower vessels, likely reducing the risk of freeze-induced embolisms but also decreasing water conductance capacity. Vessels were also narrower in regions with high soil salinity, including Texas, USA and tidal flats in Veracruz, Mexico. Vessel density did not consistently vary with temperature or soil salinity. In abiotically stressful areas, A. germinans had a safe hydraulic architecture with narrower vessels that may increase local survival. This safe architecture appears to come at a substantial physiological cost in terms of reduction in conductance capacity and carbon fixation potential, likely contributing to lower canopy heights. The current distribution of A. germinans in the Gulf is influenced by the complex interplay between temperature, salinity, and vessel architecture. Given the plasticity of A. germinans vessel characters, it is likely that this mangrove species will be able to adapt to a wide range of potential future environmental conditions, and continue its expansion in the Gulf of Mexico in response to near-term climate change.
Introduction
Coastal wetland environments in the tropics are primarily comprised of mangrove forests, but salt marshes are dominant in temperate latitudes (Saintilan et al., 2014). In the northern half of the western hemisphere, there is a transition between these two ecosystems in the Gulf of Mexico along the roughly 2200 km stretch of shoreline between La Pesca, Tamaulipas (Mexico) and Tampa, FL (USA). Over the last several decades, populations of mangroves (e.g., Avicennia spp.) have begun to expand into areas of salt marsh (Spartina alterniflora and other species) along the Texas, Louisiana, and Florida coasts, as well as in Australia and other regions of the world (Perry and Mendelssohn, 2009; Everitt et al., 2010; Krauss et al., 2011; Montagna et al., 2011; Williamson et al., 2011; Raabe et al., 2012; Bianchi et al., 2013; Cavanaugh et al., 2014). In some regions, forests of Avicennia germinans have completely replaced S. alterniflora (Sherrod and McMillan, 1981). The distribution of A. germinans is expected to continue expanding over the next several decades in response to climate change, which may alter the frequency of freezing temperatures, the amount of rainfall, and the rate of sea level rise (Osland et al., 2013; Cavanaugh et al., 2014).
Many species of salt marsh plants can survive in both temperate and tropical climates, but mangrove forests are found exclusively in tropical and subtropical latitudes (Tomlinson, 1994; Duke et al., 1998; Spalding et al., 2010). Avicennia germinans is the only mangrove tree species found in the subtropical northern Gulf of Mexico. Thus, the anatomical and physiological adaptations of A. germinans may play an important role in determining the range of mangrove forest distribution, and consequently of salt marsh distribution, in the northern Gulf of Mexico, and will influence the potential for further mangrove expansion in the region. Given the important global role of mangroves as ecosystem engineers, this expansion may have implications for a variety of ecosystem services (Ellison et al., 2005).
Temperature is widely recognized as a major driver of these coastal changes (e.g., McMillan and Sherrod, 1986; Osland et al., 2013; Cavanaugh et al., 2014). Interactions between biotic and abiotic characteristics of mangrove trees and their surrounding environment are also likely to influence the rate of change. For example, freezing temperatures may be fatal to mangroves, especially in arid and saline soils, contributing to low soil water potential (Quisthoudt et al., 2012); this creates more negative xylem pressures, increasing the likelihood of catastrophic embolism formation (Stuart et al., 2007). Embolisms are pockets of air that can form following cavitation, when sudden changes in hydraulic tension (e.g., due to a rapid drop in temperature or water stress in drought conditions) in the xylem cause liquid water to vaporize. After a period of time, air will fill these vapor-filled conduits, forming embolisms that disrupt the transport of xylem sap (Tyree and Sperry, 1989). Embolisms can sometimes be repaired when pressure within the xylem forces air out of the conduit through pits (pores) in the lateral sides of the vessels and back into solution (Holbrook and Zwieniecki, 1999), though in severe cases, embolism formation can cause plant mortality (Urli et al., 2013). Therefore, plants with better resistance to embolism are more likely to survive conditions, such as freezing temperatures, that cause rapid changes in xylem pressure. Laboratory studies have demonstrated that several mangrove tree species with narrower vessel elements are better able to prevent the formation of freeze-induced embolisms and consequently have a greater chilling tolerance (Stuart et al., 2007), though vessel plasticity and chilling tolerance vary widely among mangrove species (Chave et al., 2009).
Based on previous laboratory work on mangroves (Stuart et al., 2007), we expected that in field populations, A. germinans xylem vessel architecture (using hydraulically weighted vessel diameter and density as proxies) would vary with winter temperature minima and soil salinity. Hydraulically weighted vessel diameter (Davis et al., 1999; Stuart et al., 2007; Charrier et al., 2013) is a relatively unexplored trait in any mangrove species across a large spatial scale. Therefore, our objective was to investigate how xylem vessel characteristics influence mangrove distribution by systematically comparing A. germinans vessel anatomy across its distribution in the Gulf of Mexico. We hypothesized that vessel architecture would vary across the Gulf of Mexico, with narrower vessels and greater vessel densities in more northern latitudes.
Materials and Methods
Field sites were coastal or estuarine A. germinans (black mangrove) populations distributed throughout the Gulf of Mexico. Sites were grouped into four regions of the Gulf: Veracruz (Mexico), and Texas, Louisiana, and FL (USA; Table 1; Figure 1). Each region was considered to be a separate population for statistical analyses. Average winter temperature minima were determined from climatic records (previous 10–30 years) archived in the National Oceanic and Atmospheric Administration’s National Climatic Data Center (Table 1). Entire pieces of stem (1–1.5 cm thick and ≈30 cm long) were clipped from three or more mature (reproductive) A. germinans trees at each site (one per tree, n ≥ 3). Within each site, all sampled trees were approximately the same height and at least 10 m apart. At the USA sites, samples were collected from A. germinans stands in the mid- to high intertidal zone. In Veracruz, wood samples were collected from two distinct zones. In high salinity mudflat or sand flat areas, termed “tidal flats,” A. germinans typically had dwarf morphology (<3 m tall). In low to moderate salinity interdistributary flood basins, trees were typically over 10 m tall. The Veracruz tidal flat and flood basin localities were treated as separate populations in statistical analyses. Samples were collected at chest height for taller trees or at a height of about one meter for shorter trees. We chose stems with fresh, healthy, green leaves. Each sample was placed in a sealed plastic bag, transported to the laboratory, and dried at 60°C for 7–10 days.
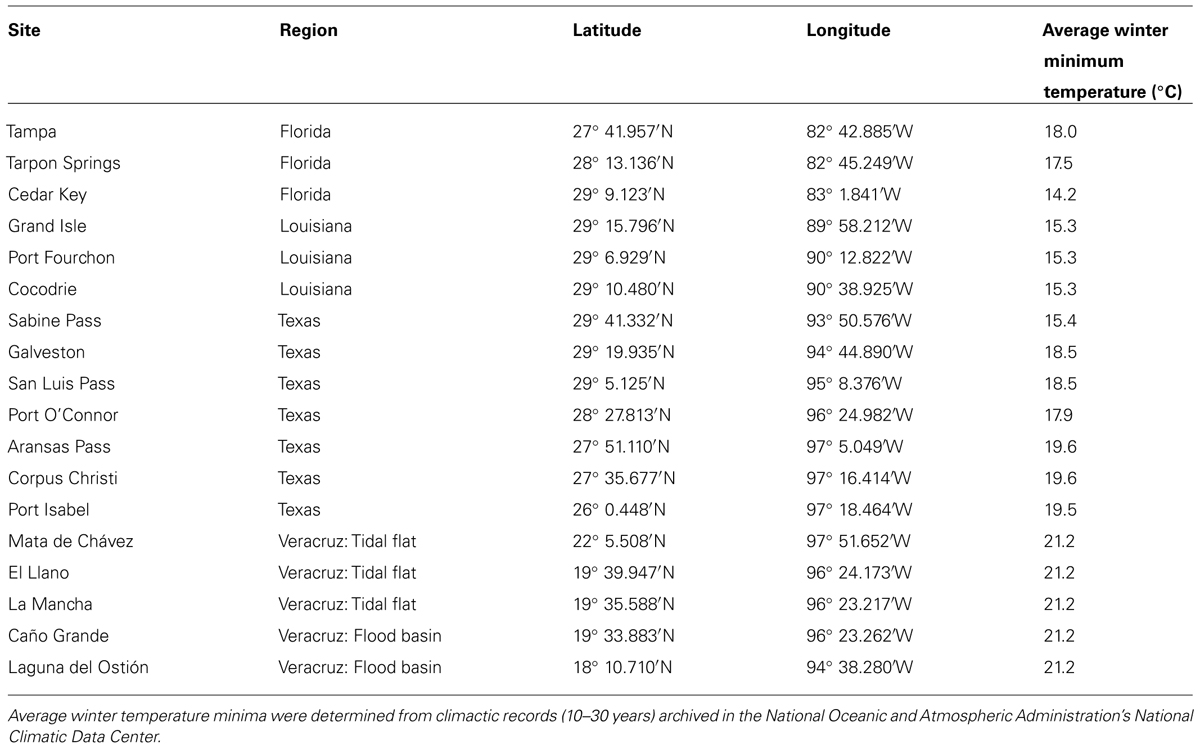
TABLE 1. Site locations and average minimum winter (December-February) temperatures over a 10–30 year period.
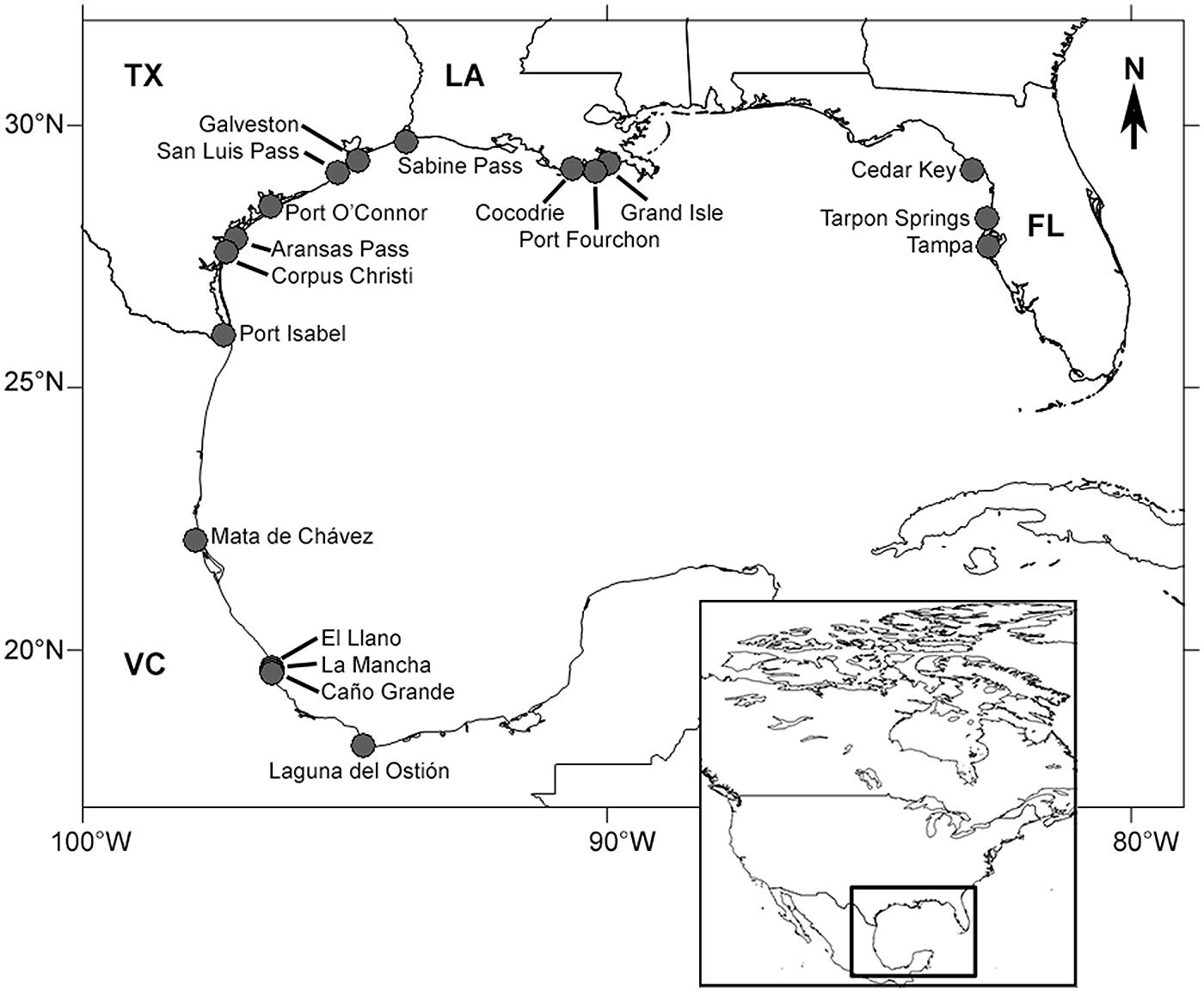
FIGURE 1. Mangrove collection sites in the Gulf of Mexico. Sites were located in Florida (FL, USA), Louisiana (LA, USA), Texas (TX, USA), and Veracruz (VC, Mexico).
The transverse edge of each wood sample presented a cross section of the mangrove stem. This edge was ground with progressively finer grades of sandpaper (200, 400, 800 grit) and cut into sections 0.5–1.0 mm thick. Each section was dehydrated through a graded ethanol series, immersed in Histo-Clear (National Diagnostics, Atlanta, GA, USA), placed on a slide with the sanded side facing upward, and mounted to a slide and coverslip with Permount mounting medium (Fisher Scientific, Fair Lawn, NJ, USA). Slides were viewed on an Axiophot microscope (Carl Zeiss Microscopy, LLC, Thornwood, NY, USA) at 200x magnification with differential interference contrast optics. Three equally spaced areas of 8.12 × 10-2 mm2 in each wood section were photographed with an Axiophot camera, and xylem characteristics were calculated with Zeiss Axiocam software. The radial growth of Avicennia is relatively unique among mangroves in that it occurs through several vascular cambia that are arranged in patches, creating non-concentric groups of xylem tissue surrounded by internal phloem tissue (Robert et al., 2014). The cross sections we examined were large enough to capture the heterogeneity of this complex patchiness (Robert et al., 2011). We focused our examinations on the outermost portion of the stems, which contained the youngest xylem and were most likely to be related to the environmental conditions we measured in the soil samples. Hydraulically weighted vessel diameter (hereafter “vessel diameter”) was calculated as: Dh = ∑ D5/∑ D4 where D was the diameter of each individual xylem vessel (Sobrado, 2007; Charrier et al., 2013). Total cross-sectional vessel area (sum of the cross-sectional areas of all xylem vessels per field of view, scaled to mm2 of stem) was calculated as a relative measure of potential conductance capacity (Davis et al., 1999). Vessel density was calculated as the number of xylem vessels per mm2 of stem, not excluding internal phloem from the stem area measurements.
One soil core (2 cm wide, 10 cm deep) was taken at the base of every sampled tree. The section of the core between 2.5 and 5.0 cm from the surface of the soil was removed, placed in a sealed bag, and transported to the laboratory for further analysis. In the laboratory, each soil sample was dried at 70°C for at least 72 h. Soil salinity was measured by filtering a portion of a saturated soil paste (Richards, 1954) through two layers of No. 2 Whatman filter paper onto a Leica® temperature-compensated refractometer. The saturated soil paste technique was preferred over measurements of pore water salinity because salinities in tidally influenced brackish and salt marshes frequently change and soil paste-derived measurements of soil salinity provide a better long-term indication of relative salinity history across large distances than single “snapshot” measurements of porewater salinity.
Mangrove canopy height was calculated around trees where wood samples were collected (n ≥ 3/site). A digital photograph was taken parallel to the ground at a height of ∼1.5 m; each photograph contained a benchmark of known height (e.g., a one meter tall marker pole). The pixel height of the benchmark was determined. Pixel length was converted to meters and used as a coarse but consistent calculation of canopy height.
Biomechanics tests were performed on three or more wood samples per site after sections had been prepared for vessel diameter measurements. Each sample was dried for several days before analysis in order to desiccate the xylem parenchyma. Bark was peeled away to expose the xylem before each fracture test. In each test, a wood sample (standardized to 25 cm long) was placed in an MTS Insight 30 kN (kilonewton) tensile tester (MTS Systems Corporation, Eden Prairie, MN, USA) configured to conduct a three-point flexural test. The sample supports had a span of 20 cm and the head moved at a speed of 0.03 cm/s. Maximum tensile fracture stress (hereafter “maximum stress;” N/mm2) at fracture was calculated as: Smax = 3PL/πR3 where Smax was the maximum stress at fracture, P was the maximum load (lbf) at fracture, L was the support span (mm), and R (mm) was the average radius of the sample at its center and each edge.
Photosynthetic performance was measured on mangroves at two sites in Texas: Galveston and Sabine Pass (Figure 1). Real-time photosynthetic measurements and fluorescence light curves were collected on cloud- and rain-free days between May 5 and May 20, 2011. Preliminary observations indicated that rates of carbon fixation in A. germinans were positive between 0900 and 1300, but all measurements for this study were collected between 0900 and 1100 to avoid mid-day photosynthetic depression. Data were collected with a LI-6400XT Portable Photosynthesis System (LI-COR, Lincoln, NE, USA) following Madrid et al. (2012). In brief, carbon fixation, as estimated by chlorophyll fluorescence, was measured simultaneously with oxygen consumption at 1800, 1500, 1000, 500, 250, 100, 50, 25, 15, and 0 μmol m-2 s-1 PAR on five mature trees/site (one tree/day). Oxygen consumption potential and carbon fixation rates were compared with previously published values in order to assess the relative photosynthetic performance of A. germinans in the northern Gulf of Mexico.
To reduce the heteroscedasticity of variances, all dependent variables (canopy height, soil salinity, hydraulically weighted vessel diameter, vessel density, total vessel area, and maximum stress) were square root transformed and normalized to a mean of zero and a standard deviation of one. Data were analyzed with one-way Analysis of Variance (ANOVA), with region (Florida, Louisiana, Texas, Veracruz flood basin, and Veracruz tidal flat) as the independent variable. Differences among regions were assessed with post hoc Tukey tests. Principal components analysis (PCA) was used to explore which variables contributed to differences among regions, and to assess relationships among the dependent variables.
Results
Mangrove canopy height varied significantly among study regions (Table 2). The tallest trees exceeded 20 m in height and were found in the southernmost interdistributary flood basin sites in Veracruz, Mexico (Figure 2A). In contrast, trees in Texas and Louisiana were often less than one meter tall. Trees in Florida and the Veracruz tidal flat areas were intermediate in height, about three meters tall.
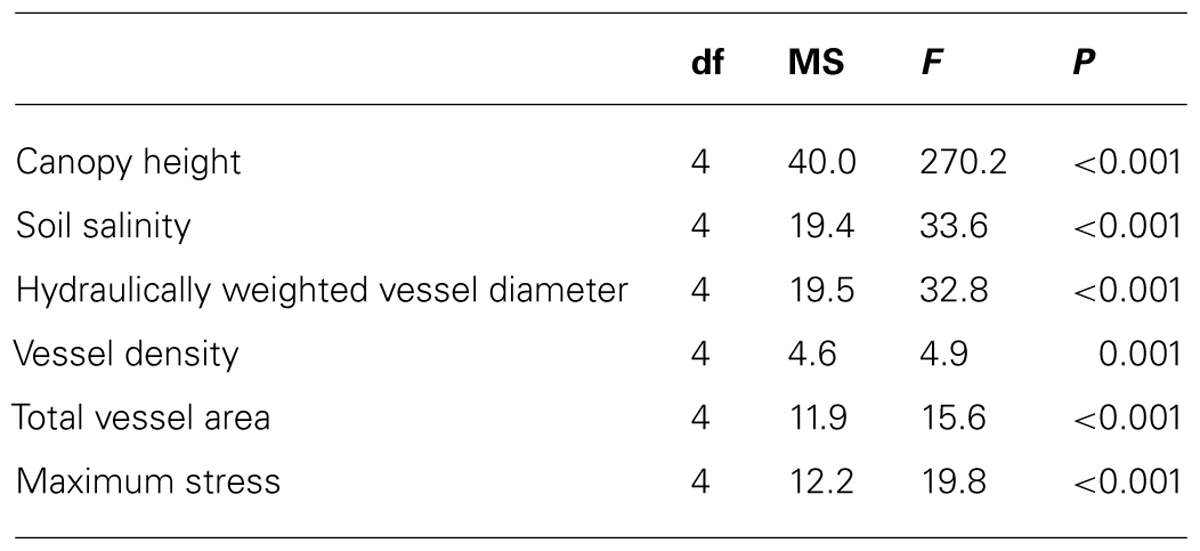
TABLE 2. Results of one-way ANOVA comparing tree and environmental characteristics among five regions of the Gulf of Mexico.
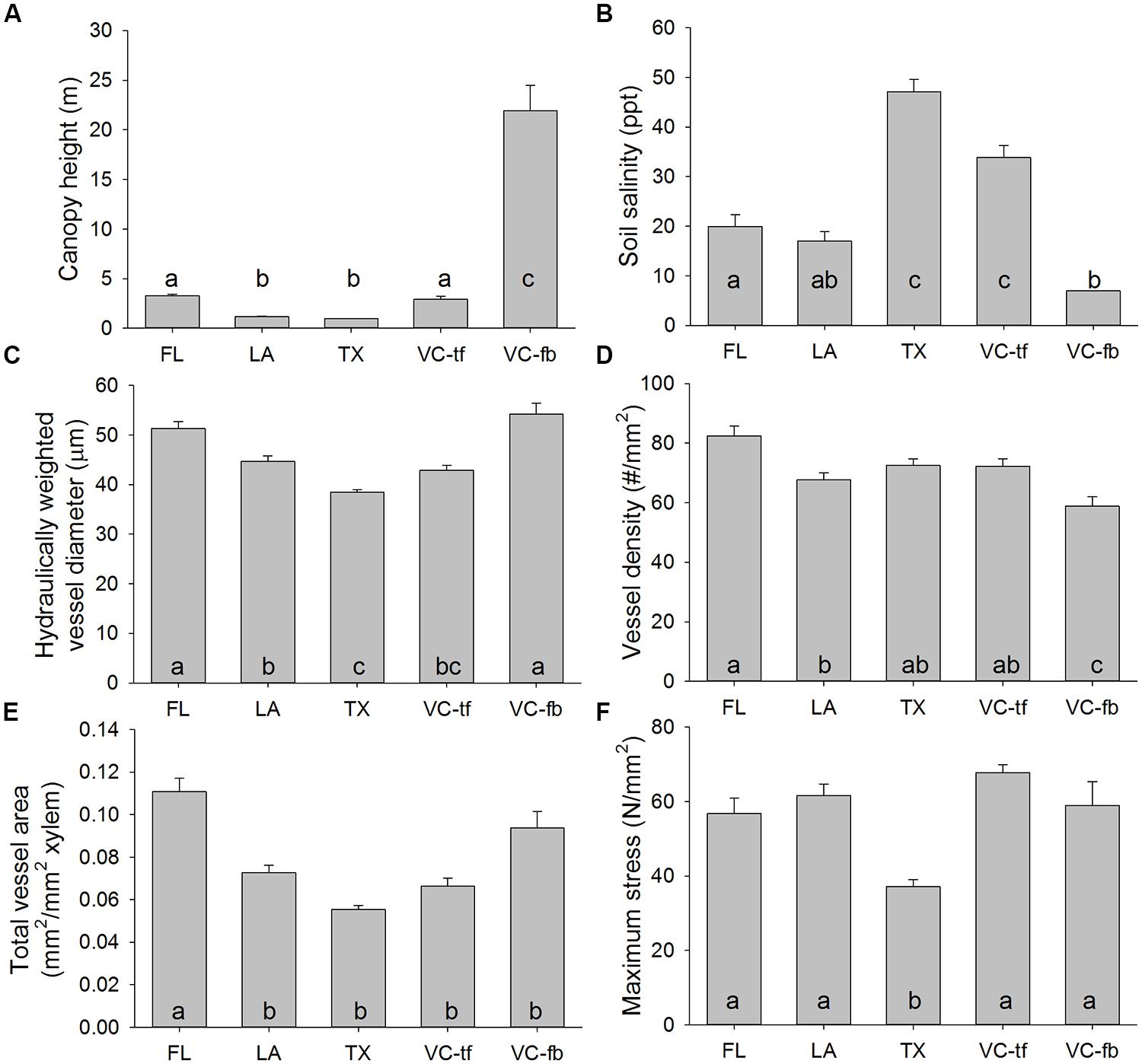
FIGURE 2. Anatomical, biomechanical, soil, and canopy features of Avicennia germinans throughout the Gulf of Mexico: Florida (FL), Louisiana (LA), Texas (TX), Veracruz tidal flats (VC-tf), and Veracruz interdistributary flood basin (VC-fb). (A) Mangrove canopy height; (B) Soil salinity; (C) Hydraulically weighted xylem vessel diameter; (D) Xylem vessel density; (E) Total xylem vessel area; (F) Maximum tensile fracture stress of mangrove branches. Error bars represent one SE. Letters indicate significant differences among regions from Tukey post hoc tests.
Soil salinity varied significantly among regions (Table 2). Soil salinity exceeded 30 parts per thousand (ppt) at sites in Texas and the Veracruz tidal flat areas, but was less than 10 ppt in the Veracruz interdistributary flood basins (Figure 2B).
Vessel characteristics varied significantly among regions (Table 2), but not all characteristics had the same spatial patterns. Hydraulically weighted vessel diameters were lowest in Texas and up to 30% wider in Florida and the Veracruz flood basins (Figure 2C). Vessel density was relatively similar among sites, though it was higher in Florida than in Louisiana or the Veracruz flood basins (Figure 2D). Total cross-sectional vessel area was up to 50% higher in Florida than in all other study regions (Figure 2E). Maximum stress at fracture was more than 50% lower in Texas mangroves than at all other sites (Figure 2F; Table 2).
Principal components analysis revealed three significant principal components (PC; eigenvalue >1) that accounted for 76.8% of the variability among samples. The first PC (34.8%) was positively correlated (coefficient ≥0.35) with vessel diameter, total vessel area, and maximum stress at fracture, and inversely correlated with soil salinity (Table 3; Figure 3). The second PC (22.9%) was inversely correlated with vessel density, soil salinity, canopy height, and minimum winter temperature. The third PC (19.1%) was positively correlated with canopy height and total vessel area, and inversely correlated with canopy height and minimum winter temperature.
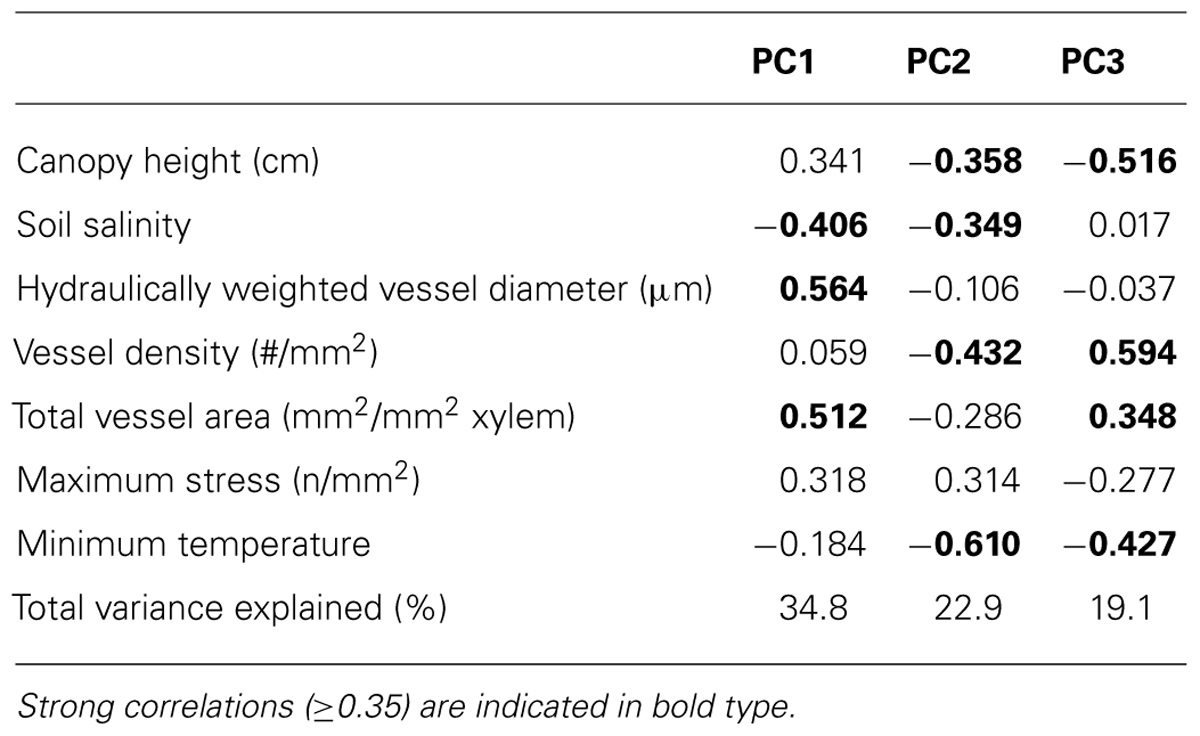
TABLE 3. Summary of results from principal components analysis, showing the correlation coefficients of each response variable with the three significant principal components.
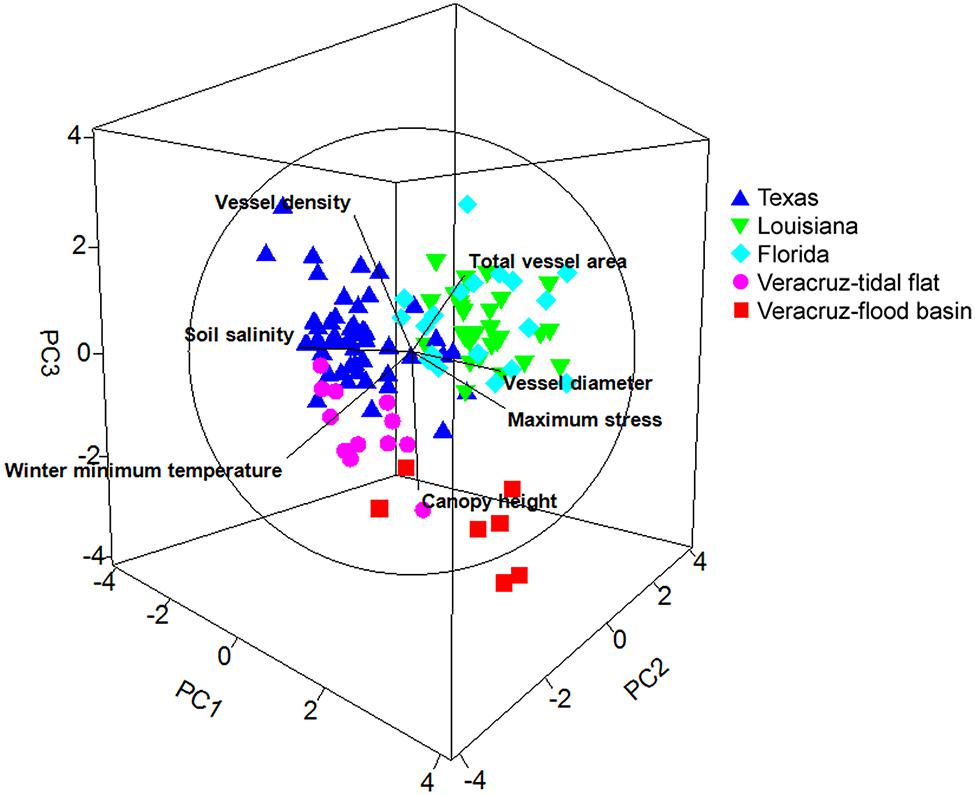
FIGURE 3. Principal components analysis depicting the three significant principal components and the relative relationships among study regions and among plant and environmental characters. Units follow Figure 2.
All five regions had distinct tree characteristics and environmental conditions (Figure 3). Trees in Florida were generally aligned with PC1, indicating that they tended to grow in moderate salinity soils and had wider hydraulically weighted vessel diameters and higher total vessel area (Table 3; Figure 2). Trees in Louisiana were correlated with PC2 and PC3, indicating that they grew in a cooler climate and had shorter canopies and narrower vessels. Trees in Texas were inversely related to PC1, indicating that they grew in higher soil salinities and had narrower vessels, lower vessel area, and shorter canopies (Table 3; Figure 2). Trees in the Veracruz flood basins were directly related to PC1 and inversely related to PC3, suggesting that they grew in a warmer climate with lower salinity soils and had taller canopies, wider vessels, lower vessel density, and higher total vessel area. Trees in the Veracruz tidal flat areas were inversely related to PC3, suggesting that they had lower vessel density, lower total vessel area, a relatively tall canopy, and grew in a warmer climate.
Avicennia germinans photochemical oxygen consumption (JO2) potential at two Texas sites neared maximum at 500 PAR (Figure 4A). Rates of carbon fixation (PN) were less than those of oxygen consumption, and were still increasing at the maximum PAR used in this study (1800; Figure 4B). To assess overall photosynthetic efficiency, the rates of oxygen consumption and carbon fixation were compared. The relationship between JO2 and PN was non-linear (Figure 4C), indicating inefficient carbon fixation.
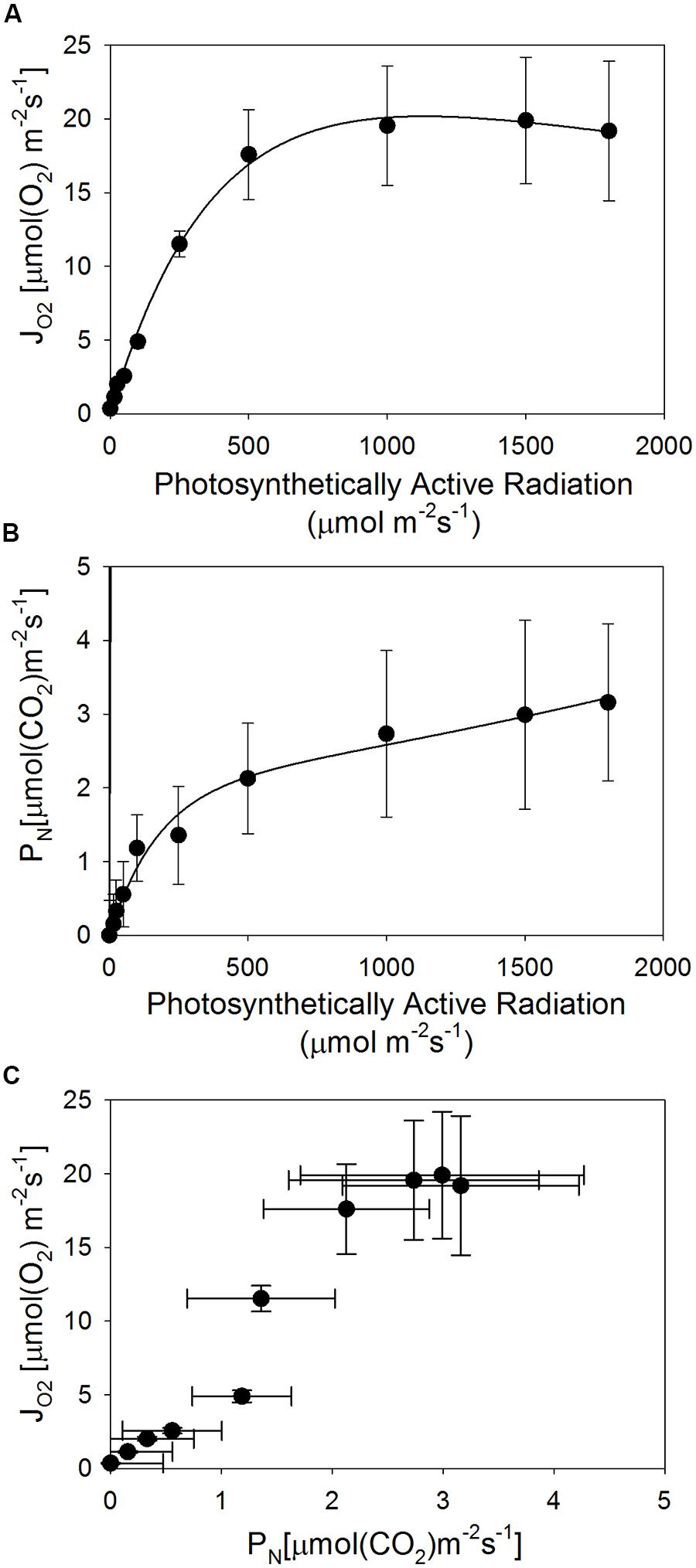
FIGURE 4. Average photosynthetic performance of A. germinans across two sites in Texas. (A) Oxygen consumption potential (JO2); (B) carbon fixation rates (PN); (C) the relationship between oxygen consumption potential and carbon fixation rates, as a measure of overall photosynthetic efficiency. Error bars represent one SD.
Discussion
Populations of A. germinans from cooler areas, namely those populations in Texas and Louisiana, had narrower vessels than populations from warmer habitats, particularly those in Florida and the Mexico fringe zones. At relatively high hydraulic tension, wider vessels will cavitate more than narrower vessels – as air bubbles come out of solution during the freezing and thawing process, they coalesce more easily in wider vessels, thus causing embolisms (Davis et al., 1999). Therefore, narrower vessels can reduce the frequency of freeze-induced embolisms and potentially increase chilling tolerance (Stuart et al., 2007; Charrier et al., 2013). Our study confirmed the link between vessel diameter and freezing risk in the field. Vessel characteristics in trees are often plastic (Chave et al., 2009), sometimes responding to environmental conditions on seasonal time scales (Arnold and Mauseth, 1999) and influencing cavitation risk in response to environmental stressors including freezing temperatures and drought (Choat et al., 2012). Recent physiological studies in Avicennia marina demonstrate that patchy xylem growth in this clade has allowed for constant and purposeful changes in wood anatomy in response to abiotic factors including salinity and drought (Robert et al., 2011, 2014). Our lab methodology sought to capture the variability introduced by xylem patchiness within the stem, and sampling a large number of trees across many study sites made our dataset relatively robust for drawing regional-level conclusions. Therefore, our study provides convincing field evidence that A. germinans may use plastic vessel architecture to tolerate the cooler subtropical climate of the northern Gulf of Mexico.
Our findings also provide an explanation for the results observed by McMillan (1975), Markley et al. (1982), and McMillan and Sherrod (1986) in their transplant experiments. These authors collected A. germinans from some of the localities where we collected our samples (Port Isabel, Aransas Pass, Galveston, Port Fourchon, Cedar Key, Tampa) and demonstrated through freezing and transplant experiments that plants from Texas had greater freeze tolerance than those from Louisiana, and that plants from Louisiana had greater freeze tolerance than those from Florida. Accordingly, we found that vessels were narrower in Texas than in Louisiana, and narrower in Louisiana than in Florida. Therefore, it is likely that the different A. germinans freezing tolerances among regions (McMillan, 1975; Markley et al., 1982; McMillan and Sherrod, 1986) are at least partially driven by differences in vessel diameters.
Although hydraulically weighted vessel diameter was linked to local temperature minima, it was also related to other environmental factors, namely soil salinity. In fact, our analysis revealed that soil salinity was more strongly correlated with the first two principal components, suggesting that vessel characteristics may be more strongly linked to soil salinity than to winter temperature. Vessels were narrowest in the regions with higher soil salinity, including Texas and Veracruz tidal flats. The Veracruz tidal flat was among the warmer sites, with no freezing risk. Therefore, the small vessel diameter in that region was likely driven by the extremely high soil salinity. This inverse relationship between salinity and vessel diameter had not been previously reported in A. germinans, though other mangrove species, including Laguncularia racemosa (Sobrado, 2007) and A. marina (Robert et al., 2009) have narrower vessels in saline conditions.
In contrast to the link between soil salinity and vessel diameter, we did not observe a strong relationship between vessel density and soil salinity. In species from warmer climates where freezing events are less likely, mangroves often have higher vessel densities at higher salinities (Schmitz et al., 2006; Sobrado, 2007; Robert et al., 2009). In these cases, vessel density increases at high salinity as a form of “safe hydraulic architecture,” where vessel redundancy helps protect the plant from the effects of cavitation, thus preserving the capacity of the water transport system (Robert et al., 2009). In the case of high salinity, a portion of the vessels may fail due to freeze- or salinity-induced embolism, but if vessel density is high, then there are likely to be other vessels to compensate for the loss, and hydraulic conduction capacity is maintained (Schmitz et al., 2006; Ewers et al., 2007). In our case study, A. germinans formed narrower vessels that are less susceptible to embolism formation in response to cooler temperatures, but did not produce them at an increased density. Consequently, as soil salinity increases and temperature goes down, water conduction capacity in A. germinans will decrease.
Lower water conductance capacity is likely linked to a decrease in productivity. Accordingly, we observed very low rates of carbon fixation in mangrove populations with high soil salinity and low temperature minima. The carbon fixation potentials we measured in A. germinans from Galveston and Sabine Pass, Texas were near 3 μmol CO2 m-2 s-1. In contrast, previous studies documented rates of carbon fixation (reported in μmol CO2 m-2 s-1) from 10 to 15 in populations of A. germinans (Krauss et al., 2006), 15 in A. marina (Naidoo, 2006) and 10 in L. racemosa (Sobrado, 2005) from the tropics. Carbon fixation is the primary source of energy derived through photochemistry, and efficient fixation would be indicated by a linear relationship between JO2 and PN with a slope of one. Instead, we detected a non-linear relationship between JO2 and PN and values of PN were much less than JO2. Thus, energy transfer to the carbon fixation reactions was inefficient, lowering the overall rates of carbon fixation. This is a typical water stress response (Chaves et al., 2009) and is likely due to the extremely low water potentials of the highly saline soils in which the plants were growing, the reduced diameter and relatively low density of their vessels that result in low water transport capacities, or both.
Mangrove canopy height is frequently linked to temperature, with shorter canopies at higher latitudes (Méndez-Alonzo et al., 2008). This geographic pattern is often directly attributed to tree dieback in freeze-prone areas (Stevens et al., 2006; Soares et al., 2012). Our study demonstrated that vessel architecture also played a role in limiting canopy height. In particular, A. germinans in cooler areas had developed a safe hydraulic architecture with a higher density of narrower vessels, and these vessel features also occurred in high salinity areas. Although these vessel characteristics are likely effective at increasing survival in abiotically stressful areas, they have substantial physiological costs in terms of reduction in xylem conductivity and carbon fixation potential. Therefore, areas with frequent freezing events or extremely saline soils will require vessels with such a narrow diameter that photosynthetic output may not be sufficient for long-term growth, reproduction, and survival. Thus, while A. germinans vessel architecture is conducive to survival in cooler climates, there is a limit to this capacity. The populations we surveyed in the northwestern Gulf of Mexico likely have the most extreme vessel sizes and density and are in the most stressful abiotic conditions that A. germinans is capable of tolerating.
The current distribution of A. germinans in the Gulf of Mexico is likely limited by the complex interplay between temperature, hydroperiod, and salinity, and vessel architecture. However, environmental conditions in the Gulf are expected to change in response to near-term climate change. Likely outcomes include increases in temperature (Keim et al., 2011), thus decreasing freezing risk for mangroves. Decreases in precipitation and increased urban and agricultural demand for freshwater are likely to increase estuarine salinity (Gibson et al., 2005; Keim et al., 2011), possibly increasing salinity stress. Several models predict that changes in temperature, salinity, and sea level will cause mangrove expansion into higher latitudes (Doyle et al., 2010; Osland et al., 2013). This expansion has already been documented in many regions, including the Gulf of Mexico (e.g., Saintilan et al., 2014). Given the wide variation range of A. germinans vessel characters, it is likely that this mangrove species will be able to thrive in a wide range of potential future environmental conditions, and continue its near-term expansion at the expense of salt marshes in the Gulf of Mexico.
Conflict of Interest Statement
The authors declare that the research was conducted in the absence of any commercial or financial relationships that could be construed as a potential conflict of interest.
Acknowledgments
This material is based upon work supported by the Texas Institute of Oceanography at TAMUG and the Texas A&M University and Consejo Nacional de Ciencia y Tecnología (CONACYT) Collaborative Research Grant Program. Jorge López-Portillo also acknowledges support by the Coastal Research Network on Environmental Changes (CREC), classified as a Marie Curie Action (FP7-PEOPLE-2009-IRSES #247514). Antonietta Quigg generously provided the LI-COR 6400XT for use in this study. Robin Brinkmeyer kindly provided the microscope we used to measure vessel diameter. We thank Amanda Thronson and Víctor Vasquez for their valuable assistance in the field, and Cody Hales and Ted Driscoll for their help with sample processing. The open access publishing fees for this article have been covered by the Texas A&M University Online Access to Knowledge (OAK) Fund, supported by the University Libraries and the Office of the Vice President for Research.
References
Arnold, D. H., and Mauseth, J. D. (1999). Effects of environmental factors on development of wood. Am. J. Bot. 86, 367–371. doi: 10.2307/2656758
Bianchi, T. S., Allison, M. A., Zhao, J., Li, X. X., Comeaux, R. S., Feagin, R. A.,et al. (2013). Historical reconstruction of mangrove expansion in the Gulf of Mexico: linking climate change with carbon sequestration in coastal wetlands. Estuar. Coast. Shelf Sci. 119, 7–16. doi: 10.1016/j.ecss.2012.12.007
Cavanaugh, K. C., Kellner, J. R., Forde, A. J., Gruner, D. S., Parker, J. D., Rodriguez, W.,et al. (2014). Poleward expansion of mangroves is a threshold response to decreased frequency of extreme cold events. Proc. Natl. Acad. Sci. U.S.A. 111, 723–727. doi: 10.1073/pnas.1315800111
Pubmed Abstract | Pubmed Full Text | CrossRef Full Text | Google Scholar
Charrier, G., Cochard, H., and Améglio, T. (2013). Evaluation of the impact of frost resistances on potential altitudinal limit of trees. Tree Physiol. 33, 891–902. doi: 10.1093/treephys/tpt062
Pubmed Abstract | Pubmed Full Text | CrossRef Full Text | Google Scholar
Chave, J., Coomes, D., Jansen, S., Lewis, S. L., Swenson, N. G., and Zanne, A. E. (2009). Towards a worldwide wood economics spectrum. Ecol. Lett. 12, 351–366. doi: 10.1111/j.1461-0248.2009.01285.x
Pubmed Abstract | Pubmed Full Text | CrossRef Full Text | Google Scholar
Chaves, M. M., Flexas, J., and Pinheiro, C. (2009). Photosynthesis under drought and salt stress: regulation mechanisms from whole plant to cell. Ann. Bot. 103, 551–560. doi: 10.1093/aob/mcn125
Pubmed Abstract | Pubmed Full Text | CrossRef Full Text | Google Scholar
Choat, B., Jansen, S., Brodribb, T. J., Cochard, H., Delzon, S., Bhaskar, R.,et al. (2012). Global convergence in the vulnerability of forests to drought. Nature 491, 752–755. doi: 10.1038/nature11688
Pubmed Abstract | Pubmed Full Text | CrossRef Full Text | Google Scholar
Davis, S. D., Sperry, J. S., and Hacke, U. G. (1999). The relationship between xylem conduit diameter and cavitation caused by freezing. Am. J. Bot. 86, 1367–1372. doi: 10.2307/2656919
Doyle, T. W., Krauss, K. W., Conner, W. H., and From, A. S. (2010). Predicting the retreat and migration of tidal forests along the northern Gulf of Mexico under sea-level rise. For. Ecol. Manag. 259, 770–777. doi: 10.1016/j.foreco.2009.10.023
Duke, N. C., Ball, M. C., and Ellison, J. C. (1998). Factors influencing biodiversity and distributional gradients in mangroves. Global Ecol. Biogeogr. Lett. 7, 27–47. doi: 10.2307/2997695
Ellison, A. M., Bank, M. S., Clinton, B. D., Colburn, E. A., Elliott, K., Ford, C. R.,et al. (2005). Loss of foundation species: consequences for the structure and dynamics of forested ecosystems. Front. Ecol. Environ. 3:479–486. doi: 10.1890/1540-9295(2005)003[0479:LOFSCF]2.0.CO;2
Everitt, J. H., Yang, C., Judd, F. W., and Summy, K. R. (2010). Use of archive aerial photography for monitoring black mangrove populations. J. Coast. Res. 26, 649–653. doi: 10.2112/JCOASTRES-D-09-00133.1
Ewers, F. W., Ewers, J. M., Jacobsen, A. L., and López-Portillo, J. (2007). Vessel redundancy: modeling safety in numbers. IAWA J. 28, 373–388. doi: 10.1163/22941932-90001650
Gibson, C. A., Meyer, J. L., Poff, N. L., Hay, L. E., and Georgakakos, A. (2005). Flow regime alterations under changing climate in two river basins: implications for freshwater ecosystems. River Res. Appl. 21, 849–864. doi: 10.1002/rra.855
Holbrook, N. M., and Zwieniecki, M. A. (1999). Embolism repair and xylem tension: Do we need a miracle? Plant Physiol. 120, 7–10. doi: 10.1104/pp.120.1.7
Pubmed Abstract | Pubmed Full Text | CrossRef Full Text | Google Scholar
Keim, B. D., Fontenot, R., Tebaldi, C., and Shankman, D. (2011). Hydroclimatology of the U.S. Gulf Coast under global cliamte change scenarios. Phys. Geogr. 32, 561–582. doi: 10.2747/0272-3646.32.6.561
Krauss, K. W., From, A. S., Doyle, T. W., Doyle, T. J., and Barry, M. J. (2011). Sea-level rise and landscape change influence mangrove encroachment onto marsh in the Ten Thousand Islands region of Florida, USA. J. Coast. Conserv. 15, 629–638. doi: 10.1007/s11852-011-0153-4
Krauss, K. W., Twilley, R. R., Doyle, T. W., and Gardiner, E. S. (2006). Leaf gas exchange characteristics of three neotropical mangrove species in response to varying hydroperiod. Tree Physiol. 26, 959–968. doi: 10.1093/treephys/26.7.959
Pubmed Abstract | Pubmed Full Text | CrossRef Full Text | Google Scholar
Madrid, E. N., Armitage, A. R., and Quigg, A. (2012). The response of photosystem II to soil salinity and nutrients in wetland plant species of the northwestern Gulf of Mexico. J. Coast. Res. 28, 1197–1207. doi: 10.2112/JCOASTRES-D-11-00142.1
Markley, J. L., McMillan, C., and Thompson, G. A. Jr. (1982). Latitudinal differentiation in response to chilling temperatures among populations of three mangroves, Avicennia germinans, Laguncularia racemosa, and Rhizophora mangle, from the western tropical Atlantic and Pacific Panama. Can. J. Bot. 60, 2704–2715. doi: 10.1139/b82-330
McMillan, C. (1975). “Adaptive differentiation to chilling in mangrove populations,” in Proceedings of the International Symposium on the Biology and Management of Mangroves, eds G. E. Walsh, S. C. Snedaker, and H. J. Teas (Gainesville, FL: University of Florida), 62–68.
McMillan, C., and Sherrod, C. L. (1986). The chilling tolerance of black mangrove, Avicennia germinans, from the Gulf of Mexico coast of Texas, Louisiana and Florida. Contrib. Mar. Sci. 29, 9–16.
Méndez-Alonzo, R., López-Portillo, J., and Rivera-Monroy, V. H. (2008). Latitudinal variation in leaf and tree traits of the mangrove Avicennia germinans (Avicenniaceae) in the central region of the Gulf of Mexico. Biotropica 40, 449–456. doi: 10.1111/j.1744-7429.2008.00397.x
Montagna, P. A., Brenner, J., Gibeaut, J. C., and Morehead, S. (2011). “Coastal impacts,” in The Impact of Global Warming on Texas, 2nd Edn, eds J. Schmandt, G. R. North, and J. Clarkson (Austin, TX: University of Texas Press).
Naidoo, G. (2006). Factors contributing to dwarfing in the mangrove Avicennia marina. Ann. Bot. 97, 1095–1101. doi: 10.1093/aob/mcl064
Pubmed Abstract | Pubmed Full Text | CrossRef Full Text | Google Scholar
Osland, M. J., Enwright, N., Day, R. H., and Doyle, T. W. (2013). Winter climate change and coastal wetland foundation species: salt marshes versus mangrove forests in the southeastern U.S. Glob. Chang Biol. 19, 1482–1494. doi: 10.1111/gcb.12126
Pubmed Abstract | Pubmed Full Text | CrossRef Full Text | Google Scholar
Perry, C. L., and Mendelssohn, I. A. (2009). Ecosystem effects of expanding populations of Avicennia germinans in a Louisiana salt marsh. Wetlands 29, 396–406. doi: 10.1672/08-100.1
Quisthoudt, K., Schmitz, N., Randin, C. F., Dahdouh-Guebas, F., Robert, E. M., and Koedam, N. (2012). Temperature variation among mangrove latitudinal range limits worldwide. Trees 26, 1919–1931. doi: 10.1007/s00468-012-0760-1
Raabe, E. A., Roy, L. C., and McIvor, C. C. (2012). Tampa Bay coastal wetlands: nineteenth to twentieth century tidal marsh-to-mangrove conversion. Est. Coasts 35, 1145–1162. doi: 10.1007/s12237-012-9503-1
Richards, L. A. (1954). Diagnosis and improvement of saline and alkali soils. Agriculture handbook no. 60. Washington, DC: U.S. Department of Agriculture.
Robert, E. M. R., Jambia, A. H., Schmitz, N., De Ryck, D. J. R., De Mey, J., Kairo, J. G.,et al. (2014). How to catch the patch? A dendrometer study of the radial increment through successive cambia in the mangrove Avicennia. Ann. Bot. 113, 741–752. doi: 10.1093/aob/mcu001
Pubmed Abstract | Pubmed Full Text | CrossRef Full Text | Google Scholar
Robert, E. M. R., Koedam, N., Beeckman, H., and Schmitz, N. (2009). A safe hydraulic architecture as wood anatomical explanation for the difference in distribution of the mangroves Avicennia and Rhizophora. Funct. Ecol. 23, 649–657. doi: 10.1111/j.1365-2435.2009.01551.x
Robert, E. M. R., Schmitz, N., Boeren, I., Driessens, T., Herremans, K., De Mey, J.,et al. (2011). Successive cambia: a developmental oddity or an adaptive structure? PLoS ONE 6:e16558. doi: 10.1371/journal.pone.0016558
Pubmed Abstract | Pubmed Full Text | CrossRef Full Text | Google Scholar
Saintilan, N., Wilson, N. C., Rogers, K., Rajkaran, A., and Krauss, K. W. (2014). Mangrove expansion and salt marsh decline at mangrove poleward limits. Glob. Chang Biol. 20, 147–157. doi: 10.1111/gcb.12341
Pubmed Abstract | Pubmed Full Text | CrossRef Full Text | Google Scholar
Schmitz, N., Verheyden, A., Beeckman, H., Kairo, J. G., and Koedam, N. (2006). Influence of a salinity gradient on the vessel characters of the mangrove species Rhizophora mucronata. Ann. Bot. 98, 1321–1330. doi: 10.1093/aob/mcl224
Pubmed Abstract | Pubmed Full Text | CrossRef Full Text | Google Scholar
Sherrod, C. L., and McMillan, C. (1981). Black mangrove, Avicennia germinans, in Texas: past and present distribution. Contrib. Mar. Sci. 24, 115–131.
Soares, M. L. G., Estrada, G. C. D., Fernandez, V., and Tognella, M. M. P. (2012). Southern limit of the Western South Atlantic mangroves: assessment of the potential effects of global warming from a biogeographical perspective. Estuar. Coast. Shelf Sci. 101, 44–53. doi: 10.1016/j.ecss.2012.02.018
Sobrado, M. A. (2005). Leaf characteristics and gas exchange of the mangrove Laguncularia racemosa as affected by salinity. Photosynthetica 43, 217–221. doi: 10.1007/s11099-005-0036-8
Sobrado, M. A. (2007). Relationship of water transport to anatomical features in the mangrove Laguncularia racemosa grown under contrasting salinities. New Phytol. 173, 584–591. doi: 10.1111/j.1469-8137.2006.01927.x
Pubmed Abstract | Pubmed Full Text | CrossRef Full Text | Google Scholar
Stevens, P. W., Fox, S. L., and Montague, C. L. (2006). The interplay between mangroves and saltmarshes at the transition between temperate and subtropical climate in Florida. Wetl. Ecol. Manag. 14, 435–444. doi: 10.1007/s11273-006-0006-3
Stuart, S. A., Choat, B., Martin, K. C., Holbrook, N. M., and Ball, M. C. (2007). The role of freezing in setting the latitudinal limits of mangrove forests. New Phytol. 173, 576–583. doi: 10.1111/j.1469-8137.2006.01938.x
Pubmed Abstract | Pubmed Full Text | CrossRef Full Text | Google Scholar
Tyree, M. T., and Sperry, J. S. (1989). Vulnerablity of xylem to cavitation and embolism. Ann. Rev. Plant Physiol. Plant Mol. Biol. 40, 19–36. doi: 10.1146/annurev.pp.40.060189.000315
Urli, M., Porté, A. J., Cochard, H., Guengant, Y., Burlett, R., and Delzon, S. (2013). Xylem embolism threshold for catastrophic hydraulic failure in angiosperm trees. Tree Physiol. 33, 672–683. doi: 10.1093/treephys/tpt030
Pubmed Abstract | Pubmed Full Text | CrossRef Full Text | Google Scholar
Keywords: elasticity, evolution, phenotypic plasticity, safe xylem hydraulic architecture, salt marsh, Spartina alterniflora vessel architecture, wetland
Citation: Madrid EN, Armitage AR and López-Portillo J (2014) Avicennia germinans (black mangrove) vessel architecture is linked to chilling and salinity tolerance in the Gulf of Mexico. Front. Plant Sci. 5:503. doi: 10.3389/fpls.2014.00503
Received: 30 May 2014; Accepted: 09 September 2014;
Published online: 26 September 2014.
Edited by:
Ülo Niinemets, Estonian University of Life Sciences, EstoniaReviewed by:
Jens Kattge, Max-Planck-Institute for Biogeochemistry, GermanyElisabeth M. R. Robert, Vrije Universiteit Brussel, Belgium
Copyright © 2014 Madrid, Armitage and López-Portillo. This is an open-access article distributed under the terms of the Creative Commons Attribution License (CC BY). The use, distribution or reproduction in other forums is permitted, provided the original author(s) or licensor are credited and that the original publication in this journal is cited, in accordance with accepted academic practice. No use, distribution or reproduction is permitted which does not comply with these terms.
*Correspondence: Anna R. Armitage, Department of Marine Biology, Texas A&M University at Galveston, P.O. Box 1675, Galveston, TX 77553, USA e-mail:YXJtaXRhZ2FAdGFtdWcuZWR1