- 1Centre for Environmental and Marine Studies and Department of Chemistry, University of Aveiro, Aveiro, Portugal
- 2Department of Agronomy, Faculty of Agriculture, Sher-e-Bangla Agricultural University, Dhaka, Bangladesh
- 3Department of Genetics and Plant Breeding, Bangladesh Agricultural University, Mymensingh, Bangladesh
- 4Department of Environmental Science, School of Life Sciences, Periyar University, Salem, India
- 5Post Graduate Department of Biotechnology, St. Xavier's College (Autonomous), Kolkata, India
- 6Stress Physiology and Molecular Biology Lab, Centre for Biotechnology, Maharshi Dayanand University, Rohtak, India
- 7Central European Institute of Technology, Brno University of Technology, Brno, Czech Republic
- 8Department of Chemistry and Biochemistry, Mendel University in Brno, Brno, Czech Republic
- 9Laboratory of Plant Stress Responses, Faculty of Agriculture, Kagawa University, Miki-cho, Japan
- 10Centre for Environmental and Marine Studies and Department of Biology, University of Aveiro, Aveiro, Portugal
Varied environmental compartments including soils are being contaminated by a myriad toxic metal(loid)s (hereafter termed as “metal/s”) mainly through anthropogenic activities. These metals may contaminate food chain and bring irreparable consequences in human. Plant-based approach (phytoremediation) stands second to none among bioremediation technologies meant for sustainable cleanup of soils/sites with metal-contamination. In turn, the capacity of plants to tolerate potential consequences caused by the extracted/accumulated metals decides the effectiveness and success of phytoremediation system. Chelation is among the potential mechanisms that largely govern metal-tolerance in plant cells by maintaining low concentrations of free metals in cytoplasm. Metal-chelation can be performed by compounds of both thiol origin (such as GSH, glutathione; PCs, phytochelatins; MTs, metallothioneins) and non-thiol origin (such as histidine, nicotianamine, organic acids). This paper presents an appraisal of recent reports on both thiol and non-thiol compounds in an effort to shed light on the significance of these compounds in plant-metal tolerance, as well as to provide scientific clues for the advancement of metal-phytoextraction strategies.
Introduction
Metal(loid)s and their Chelation Strategies in Plants
The Earth's crust harbors varying levels of different metals/metalloids (hereafter termed as “metal/s”). Though at optimum level, many metals (such as Cu, Fe, Mn, Ni, Zn) are essential for plant cells; however, the supra-optimum concentrations of these metals and even low concentrations of other metals such as Ag, Al, As, Cd, Cr, Cs, Hg, Pb, Sr, and U exhibit phytotoxicity. Thus, higher concentrations of all metals that have potential to cause detrimental consequences in human or environments can be considered as “contaminant” (reviewed by Anjum et al., 2015). Nevertheless, the inception of industrialization, metalliferous mining and smelting, sewage sludge treatment, warfare, and military training, waste disposal sites and indiscriminate agricultural fertilizer use have caused significant addition of previous toxic metals to soils (Padmavathiamma and Li, 2007; Hassan and Aarts, 2011; Alloway, 2013). Though, it remained technically a challenge for the global scientific community, the cleanup of metal-contaminated soils has been widely advocated to minimize their impact on human and environmental health (reviewed by Ali et al., 2013). In this context, compared to different physical, chemical and biological approaches employed for this purpose, plant and associated microbes based approach (phytoremediation) stands outstanding in terms its novelty, cost-effective, efficiency, environment- and eco-friendly, in situ applicability, and natural (solar-driven) (Mench et al., 2009; Hassan and Aarts, 2011; Anjum et al., 2012a; Ali et al., 2013). Basically, the phytoremediation approach is based on a number of strategies including: (a) phytoextraction, (b) rhizofiltration (phytofiltration), and (c) phytostabilization. Notably, plant types growing on contaminated or metalliferous soils were evidenced to develop metal-hyperaccumulation potential (reviewed by Baker and Whiting, 2002). Metal-hyperaccumulation, a process technically termed as phytoextraction, is a striking phenomenon exhibited by <0.2% of angiosperms, where a direct accumulation of metals into above-ground organs with subsequent removal/ processing of these plant-organs is possible. Nevertheless, metal-hyperaccumulators can exhibit extraordinarily high amounts of metals in their above-ground tissues to levels far exceeding those present in the soil or in non-accumulating plant species growing nearby (reviewed by Hassan and Aarts, 2011; Rascio and Navari-Izzo, 2011; Gill et al., 2012).
Understanding physiological and molecular defense strategies adopted by both hyperaccumulator and non-hyperaccumulator plants to cope with metal stress either during accumulation, degradation or elimination of metal pollutants in contaminated soils are of the utmost significance in phytoremediation studies. In particular, plant tolerance to potential toxicity caused by tissue-/organ-metal loads (during metal-hyperaccumulation/extraction) largely decides the efficiency and success of a metal remediation system (Vangronsveld et al., 2009; Maestri et al., 2010; Hassan and Aarts, 2011; Anjum et al., 2014a,b,c). Processes such as exclusion, compartmentalization, complexation, and the synthesis of metal-binding proteins and/or metal ion chelation are included in the list of defense strategies evidenced in plants under metal stress (Clemens, 2001; Mejáre and Bülow, 2001; reviewed by Hassan and Aarts, 2011). Plants have been credibly reported to avoid the damaging effects of metal toxicity, using strategies or mechanism involving: the binding of heavy metals to cell wall and immobilization (Mari and Lebrun, 2006; Kanneganti and Gupta, 2008; Bolan et al., 2014), exclusion of the plasma membrane (Lee et al., 2005; Arrivault et al., 2006), expression of more general stress response mechanisms such as stress proteins (heat shock proteins) (Song et al., 2012), and metal-chelation and -compartmentalization (Lal, 2010; Jozefczak et al., 2012; Seth et al., 2012; Lv et al., 2013). In particular, chelation is the most widespread intracellular mechanism for the maintenance of low concentrations and detoxification of free metals in plant cytoplasm that can be performed by thiol compounds (which contain sulfhydryl/thiol groups; such as a tripeptide glutathione, GSH, γ-Glu-Cys-Gly; phytochelatins, PCs; metallothioneins, MTs), and also by non-thiol compounds (such as organic acids, and amino acids) (Clemens, 2001; Mejáre and Bülow, 2001; Lal, 2010; Hassan and Aarts, 2011; Anjum et al., 2012b, 2014b,d; Jozefczak et al., 2012; Seth et al., 2012; Lv et al., 2013) (Figure 1).
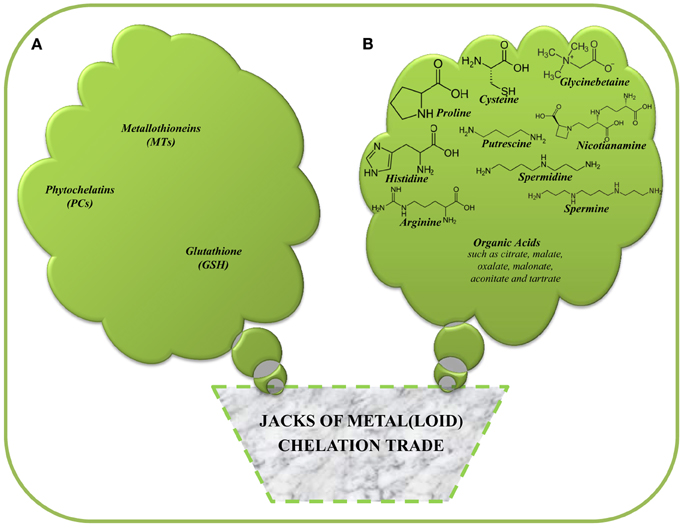
Figure 1. Major thiol- (A) and non-thiol compounds (B) involved in the chelation/detoxification of metal(loid)s in plants.
Based on recent reports, this paper discusses the basic physiology/molecular biology of metal-chelation by GSH, PCs, MTs, organic acids, amino acids—considered herein as jacks of metal-chelation trade in plants.
Thiol-Compounds and Metal(loid)-Chelation
Thiol compounds such as GSH, PCs, and MTs contain sulfhydryl (–SH) groups for binding a variety of metals (reviewed by Seth et al., 2012). GSH, a tripeptide (γ-Glu-Cys-Gly) with a wide distribution (0.5–10 mM) in plant cell compartments (namely cytosol, endoplasmic reticulum, vacuole, and mitochondria) is a major –SH compound. Apart from performing key roles in cellular redox homeostasis and the antioxidant defense, GSH is involved in the chelation and detoxification of free metal (reviewed by Anjum et al., 2010, 2012a, 2014d; Seth et al., 2012). The role of GSH in metal-chelation lies behind its significance as a precursor for the synthesis of phytochelatins (PCs, family of peptides structurally related to GSH) in metal-exposed plants (Clemens, 2006; Srivalli and Khanna-Chopra, 2008). The other important –SH compound, MTs [sulfur(S)-containing, cysteine (Cys)-rich, short, low molecular weight (4–8 kDa) gene-encoded polypeptides] have been reported to bind varied metals through the –SH of their Cys-residues (Cobbett and Goldsbrough, 2002; Verbruggen et al., 2009). Therefore, GSH, PCs, and MTs stand second to none in terms of their role in metal-chelation in plants (Figure 1; Table 1). GSH is briefly overviewed hereunder before discussing other –SH compounds and S-donor ligands—PCs and MTs in detail.
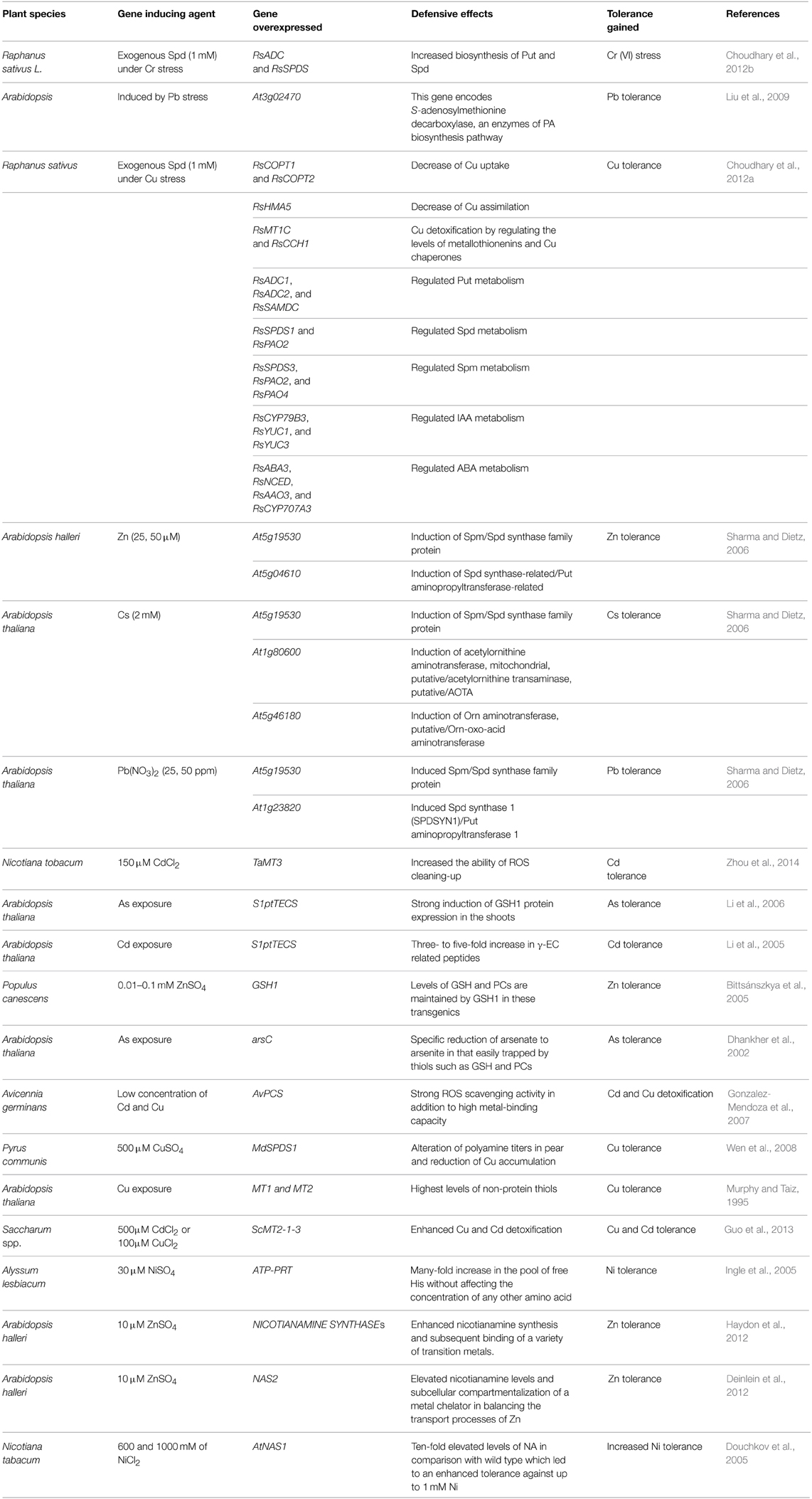
Table 1. Summary of representative studies on metal(loid)-tolerance in plants via expression of genes related with major thiol and non-thiol compounds.
GSH is recognized as an antioxidant that plays a key role in the defense mechanism of plants (Anjum et al., 2010, 2012a, 2014d; Seth et al., 2012; Nahar et al., 2015). Notably, since GSH is also an essential component in the synthesis of metal-binding peptides such as PCs, GSH has been the major metabolic/biochemical modulator of of PCs (Hall, 2002; Guo et al., 2012). The ability to maintain a high GSH level is therefore considered as an essential intrinsic feature, enabling the reduction of oxidative damage caused by accumulated metals in these species. Some metal-tolerant plants, e.g., Thlaspi goesingense, Thlaspi oxyceras, Thlaspi rosulare, and Holcus lanatus have a constitutively high GSH content, unlike e.g., Silene vulgaris or Pteris vittata (Ernst et al., 2008). The higher levels of GSH and Cys were also found in Cd-resistant mutant of Chlamydomonas reinhardtii than the wild type (Hu et al., 2001), Cu-resistant alga Stichococcus minor (Kalinowska and Pawlik-Skowrónska, 2010) and 276-4d strains of Desmodesmus armatus (Pokora et al., 2014). Cys could act as a defense mechanism not only for participating in PC biosynthesis but also directly as metal-chelator. Cys content was higher in freshwater microalga Chlamydomonas moewusii than that of GSH when Cd was present in the medium. Further, the amount of GSH decreased only slightly with the increase in the PC levels. This would suggest that the intracellular GSH was consumed during the PC biosynthesis and replaced quickly by new synthesis from the S of the medium (Mera et al., 2014). Estrella-Gómez et al. (2012) showed that Salvinia minima plants responded to Pb exposure by increasing the concentration of GSH, the activity of glutathione synthase (GS) and the expression levels of the SmGS gene. Similarly, GSH and glutathione reductase (GR) levels were also increased when tomato (Lycopersicon esculentum) plants are exposed to arsenite in which the activity of GR is essential for recharging the cells of GSH and the time of increased synthesis of PCs (Marmiroli et al., 2014). On the other hand, since Glu and Cys are important precursors in GSH biosynthesis, it is somewhat surprising that proteins involved in Gln and Cys synthesis were less abundant upon long term Cd-exposure (Dupae et al., 2014). Cd-accrued PCs-induction can cause elevation in the consumption of GSH as a result of utilization of GSH [and also the γ-GluCys (γ-EC) moiety from GSH by transpeptidation] in the production of PCs (Mendoza-Cózatl et al., 2008). In contrast, elevation in GSH pool, reported in some metal-exposed plants has been considered as a strategy to detoxify/tolerate metals(Cd)-accrued consequences either by direct binding or by synthesis of PCs (Cánovas et al., 2004; Thangavel et al., 2007; reviewed by Anjum et al., 2012b). Although the concentrations of GSH in ectomycorrhizal fungus are even in millimolar range (Schützendübel and Polle, 2002; Courbot et al., 2004) and GSH readily binds both to Cd and Zn (Feretti et al., 2007), it may provide a fast protection against the metal ion transients entering the cytoplasm.
Phytochelatins
Overview
Phytochelatins [PCs; γ-glutamyl (Glu)-cysteinyl (Cys)]n–X, where n = 2–11 and X is glycine (Gly), serine, β-alanine, glutamate or glutamine] are the principal non-protein, metal-binding (and metal-dotoxifying), S-rich, thiolate peptides (Cobbett and Goldsbrough, 2002). However, in iso-PCs (isoforms of PCs), the terminal amino acid consists of serine, glutamic acid, glutamine, or β-alanine (in the case of the homo-PCs, present in many legumes) (Oven et al., 2002). In fact, PCs are non-ribosomal peptides and are synthesized enzymatically (not by translation of mRNA owing to the presence of γ-carboxamide linkage between Glu and Cys) in response to varied metals from GSH by phytochelatin synthase (PCS), which is a γ-Glu-Cys dipeptidyl transpeptidase (E.C.2.3.2.15) (Vatamaniuk et al., 2004). Both GSH and hGSH can be the substrates for the synthesis of homo-PCs in Cd-exposed legume (Oven et al., 2002). Nevertheless, though, the amino acids Cys, Glu, and Gly constitute PCs (Zenk, 1996), PC variants without C-terminal Gly-residues can also be found (Oven et al., 2002). The occurrence of PC synthesis in metal-exposed plants was reported to follow different hierarchical levels from algae to higher plants including trees (Thangavel et al., 2007; Minocha et al., 2008). In earlier studies, PC production and the enzyme PCS were not found in land plants or bryophytes including liverworts, mosses, and hornworts (Bruns et al., 2001; Kopriva et al., 2007). Micrasterias denticulata is the only charophytic algae where the synthesis of PCs has until now been definitely detected (Volland et al., 2014). Recently, the PC-synthesis capability and the presence of constitutive and functional PCS were considered as ancestral (plesiomorphic) characters for basal land plants including bryophytes, charophytes, and lycophytes (Petraglia et al., 2014). A lower amount of PC produced under Cd stress (36 and 72 μ M of Cd for 72 h) in all the bryophyte lineages and in the lycophyte Selaginella denticulataprevious was suggested due to lower PCS activity as compared to angiosperms. Notably, potential toxicity of accumulated metals can be decreased as a result of the formation and subsequent sequestration of “metal-PC complexes” in vacuoles via transport across the tonoplast (Cobbett and Goldsbrough, 2002). Nevertheless, when expressed in an appropriate host the Caenorhabditis elegans PCS gene that control PCs production; however, knocking out the gene can increase the sensitivity of C. elegans to Cd (Vatamaniuk et al., 2001). Considering the previous roles of PCs as well as due to their significant role in the induction in plants under metal-exposure, the “status of PCs in plants” has been advocated as one of the major indicators of metal pollution (Dago et al., 2014).
Metal(loid)-Specificity and -Chelation Mechanisms
Synthesis of PCs can be plant-specific and/or metal-specific. Depending on the metal type, Hg, Cd, As, Ag, and Fe have been reported as strong inducers of PCs while Pb and Zn are weak inducers and Cu and Ni are moderate inducers (Zenk, 1996). Among metals, Cd has been detected as a strong inducer of PCs in various plant species (Ortega-Villasante et al., 2005; Clemens, 2006; Rellán-Álvarez et al., 2006; Thangavel et al., 2007; Sobrino-Plata et al., 2009; Gill et al., 2012; Guo et al., 2012; Dago et al., 2014). Compared to Zn (Thangavel et al., 2007) and Hg (Ortega-Villasante et al., 2005; Rellán-Álvarez et al., 2006; Sobrino-Plata et al., 2009) Cd was observed as a major PC-inducer. Opposite to GSH, which was more concentrated in Hg-exposed Hordeum vulgare aerial parts than in H. vulgare roots, longer-chain PCs (such as PC3, PC4, and PC5) were more abundant in H. vulgare roots than in aerial parts of H. vulgare and were increased with increase in phytoavailable Hg in soils (Dago et al., 2014). Moreover, the decreased concentrations of smaller thiols such as GSH and PC2 with increasing phytoavailable Hg in soils can be due to use of both GSH and PC2 as substrates for the synthesis of the said longer-chain PCs. Pb and Cd exposure can cause the production of PC2 and PC4, respectively, in Phaeodactylum tricornutum cells (Morelli and Scarano, 2001). Among different chains of PC synthesis (PC2–PC5), PC3 and PC4 were the major PCs exhibited in Cd-exposed microalga C. moewusii (Mera et al., 2014). Similarly, PC3 was the major peptide in both the 276-4d and B1-76 strains of green alga Desmodesmus armatus under 93 μ M Cd (Pokora et al., 2014). However, the other PC-oligomers (PC2, PC4 and unidentified P1, P2, and P3) were also higher in B1-76 strain in the first-phase of cell cycle. The unidentified thiol P1, found in green alga Stichococcus bacillaris may represent γ-Glu-Cys as the precursor of GSH and PC synthesis (Pawlik-Skowrónska, 2002). The remaining two unidentified non-protein thiols P2 and P3 were also found in the freshwater green alga Stigeoclonium tenue that differed from each other in one γ-Glu-Cys unit and contained an additional Cys-residue which was resistant to a mixture of heavy metals (Pawlik-Skowrónska, 2003). Although a linear relationship between Cd and PC production was previously observed in numerous algal species (Gekeler et al., 1988), the amount of synthesized PC after Cd-exposure may not reflect exactly the level of Cd-accumulation (Ahner et al., 1995; Nishikawa et al., 2006). Further, the mechanism underlying the Cd-efficiency for PC synthesis-activation remained unproven. PCs can also function as important chelators of Zn ions (Tennstedt et al., 2009). Song et al. (2014) suggested that essential metal ions, such as Zn(II), Cu(II), and Mn(II), can be transported into vacuoles as forms of “PC2-metal complexes” through the putative ABC transporter(s). However, the efficiency and speed of free Zn2+ chelation in the cytoplasm was higher in Cd/Zn hyperaccumulator Arabidopsis halleri than in Arabidopsis thaliana and these results are helpful to identify the metal sensitivity of the plants in terms of changes in the plasma membrane potential of root cortical cells (Ovečka and Takáč, 2014).
As highlighted also above that the –SH group of the Cys-residues help PCs to bind and generate strong “PC-metal complexes” in high metal-exposed plants. Subsequently, the “PC-metal complexes” are sequestered into vacuoles (via ABC type transporters, Verbruggen et al., 2009 or a group of organic solute transporters, Solanki and Dhankhar, 2011) for detoxification. Several studies have provided a strong evidence for the formation of Pb-PC complex and its role in Pb tolerance in plants (Zhang et al., 2008; Andra et al., 2010; Fernández et al., 2012). Spisso et al. (2014) also found Hg-PC complexes especially Hg-PC2, Hg-PC3, and Hg-PC4 in Vitis vinifera under 100 mg L−1 of Hg-exposure. The PC-As (III) complexation in rice leaves was reported to reduce translocation of As from leaves to grains (Duan et al., 2011). The chelation of Cd with PCs in the cytoplasm and compartmentalization of the PC-Cd complexes in the vacuole are generally considered as a “first line” of defense mechanism against Cd phytotoxicity (Inouhe, 2005). The PC-Cd complexes are up to 1000 times less toxic to many enzymatic proteins than the free Cd ions (Solt et al., 2003). The increase in the cytosolic pH of aquatic macrophyte Elodea canadensis after Cd addition could contribute to symplasmic Cd detoxification through PC-Cd complex formation (Tariq Javed et al., 2014), because PC-Cd complex stability increases with a rise in pH (Dorcak and Krezel, 2003). Further, such increase in cytosolic pH also activates vacuolar transporters as reported for Saccharomyces cerevisiae (Park et al., 2012). Mendoza-Cózatl et al. (2008) identified high concentrations of PCs, GSH, and Cd in the phloem sap of Brassica napus and suggested that, along with the xylem, the phloem is a channel for long-distance source-to-sink transport of Cd-PC and Cd-GSH complexes.
In contrast to the facts discussed above, overexpression of PCS gene does not always have beneficial effects on heavy metal tolerance. The heterologous overexpression of Triticum aestivum TaPCS1 in rice increased Cd sensitivity and significantly increased Cd accumulation in shoots but not in roots (Wang et al., 2012). On the contrary, expression of CdPCS1 from aquatic macrophyte Ceratophyllum demersum in tobacco (Nicotiana tabacum), Escherichia coli (Shukla et al., 2012) or Arabidopsis (Shukla et al., 2013) enhanced PC synthesis as well as Cd and As accumulation. As observed in the previous studies mentioned earlier in this section, higher level of PC accumulation was also detected in roots of cucumber (Cucumis sativus) plants treated with 25 or 50 μ M Cd, individually or simultaneously with selenium (Se) (Hawrylak-Nowak et al., 2014). PC4 and PC2 were predominant in individual Cd-exposed cucumber roots and leaves, respectively. However, a reduction of PCs accumulation was observed only in roots of cucumber when Cd-exposed plants with Se addition; whereas no change in GSH and PC contents in leaves (Hawrylak-Nowak et al., 2014). Because Se interferes with S metabolism and can replace S in the S-amino acids which results into the production of the corresponding Se-amino acids (seleno-Cys and selenomethionine), their subsequent incorporation into enzymatic proteins may affect catalytic activity (Ellis and Salt, 2003). The catalytic moiety of PCS enzyme contains the active Cys. Moreover, the C-terminal domain of PCs from different species shows low sequence conservation, but shares a common feature in that they all contain multiple Cys residues that bind Cd ions with high affinity and high capacity (Wang et al., 2009). Thus, the replacement of Cys by seleno-Cys in the PCs probably may affect the biosynthesis and accumulation of PCs in the plant tissues (Hawrylak-Nowak et al., 2014).
Metallothioneins
Overview
Another important metal chelator –SH compound and S-donor ligand, metallothioneins (MTs) are products of mRNA translation, characterized as low mass weight (4–14 kDa) Cys-rich metal-binding proteins, and are widely distributed in both prokaryotic and eukaryotic organisms (Cobbett and Goldsbrough, 2002). Margoshes and Vallee (1957) for the first time characterized MTs from horse kidneys as Cd-binding proteins. Based on sequence similarities and phylogenetic relationships plant MTs are divided into four subfamilies, type 1–4 (Freisinger, 2008; Hassinen et al., 2011). Type 1 and 2 sequences contain two Cys-rich domains separated by a central Cys-free spacer. In type 1 sequences the Cys residues are exclusively arranged in Cys-Xaa-Cys motifs (Xaa represents another amino acid) in both the N- and C-terminus, while type 2 have Cys-Cys, Cys-Xaa-Xaa-Cys, and Cys-Xaa-Cys sequences in the N-terminal domain and Cys-Xaa-Cys in the C-terminal domain. Each type of MT genes displays a distinct spatial and temporal expression pattern (Cobbett and Goldsbrough, 2002; Hassinen et al., 2011). Based on structural models, it can be assumed that the MT molecule is composed of two binding domains, α and β, which are composed of Cys-clusters. Covalent binding of metal atoms involves sulfhydryl cysteine residues. The N-terminal part of the peptide is designated as β-domain and has three binding sites for divalent ions, and the C-terminal part (the α-domain) has the ability to bind four divalent metal ions (Ruttkay-Nedecky et al., 2013).
Metal(loid)-Specificity and -Chelation Mechanisms
MTs exhibit their high affinity for both essential and non-essential metals, where MTs can provide thiols for metal chelation in their reduced state. High affinity of MTs for metals provides not only a mechanism for protection against the toxicity of different metals (such as Cd) but it is also significant for the maintenance of homeostasis of some essential metals such as Zn and Cu ions. A general structure for plant MT has been proposed for Triticum durum MT type I. This protein forms dimers or higher oligomers and adopts an extended conformation containing both α-helix and β-sheet structures. This model proposes an overall dumbbell shape similar to that reported for mammalian MTs (Bilecen et al., 2005), in agreement with dynamic data obtained on Fucus vesiculosus MTs (Merrifield et al., 2006). The involvement of peptide donor groups (S-thiol and N-imidazole) and non-protein ligands (as sulfide anions) in metal chelation, as well as secondary structure elements, was demonstrated. The protein accommodates up to six Cd2+ together with four S2−; while, less than four Zn2+ could bind the protein (Zimeri et al., 2005). The combination of high thermodynamic but low kinetic stability is one of the main features of the metal-MT complexes, which bind the metals very tightly but a part of the metal ions is easily exchanged for other proteins (Hassinen et al., 2011). The MT superfamily combines a large variety of small Cys-rich proteins that have the ability to coordinate various transition metal ions, including Zn2+, Cd2+, and Cu+ (Freisinger, 2011). Metal ions can also be coordinated through His residues, but the impact of this ligand on the metal binding properties and function of MTs is not clear(Blindauer, 2008).
There exists inconsistency in the literature available on major stimuli capable of MTs induction/expression in plants. Though abiotic stresses such as drought, salinity, heat, cold light, wounding and senescence can modulate MT gene expression in plants (references cited in Sekhar et al., 2011), among metals, Cu, Cd, Pb, and Zn can strongly induce the plant MT gene expression (reviewed by Mehes-Smith et al., 2013). Cu-induced expression of a Type 1 MT gene in Arabidopsis. Guo et al. (2003) were the first to describe the expression of the complete MT gene family in Arabidopsis and their responses to Cu treatment. In non-accumulator plants, like A. thaliana, MT1a and MT1b are expressed at high levels in roots during exposure to Cd, Cu, and Zn (Maestri et al., 2010). In Thlaspi caerulescens, the levels of MT1mRNA were found in leaves constitutively higher than in roots; the levels increased with exposure to Cu. The primary sequence of the type 3 MT of T. caerulescens displays modifications that are proposed to increase its Cu-binding properties when compared to the non-hyperaccumulator, A. thaliana ortholog. A. halleri and T. caerulescens have a constitutively high expression of MT2. The expression of MT3 genes increases during leaf aging and upon exposure to Cu in non-accumulator plants (Roosens et al., 2004). MT4 is highly expressed in seeds of A. thaliana. It plays a role in metal homeostasis during seed development and seed germination rather than in metal decontamination (Roosens et al., 2004; Maestri et al., 2010). The analysis of the expression of MT2a in A. thaliana, a non-Pb tolerant species, showed that were specifically over-expressed in roots by Pb-treatment (Auguy et al., 2013). MTs are extremely diverse in plants and T. caerulescens seems to be an excellent model to understand the adaptive significance of this phenomenon. MT1, MT2, and MT3-related cDNAs were isolated in T. caerulescens and both TcMT1- and TcMT3-deduced protein sequences display modifications in their Cys domains when compared to their homologs in A. thaliana. Functional tests in yeast indicated that such modifications may alter the metal chelation of the plant MT proteins. Roosens et al. (2005) showed that the drastic decrease in the Cys number of domain 1 of TcMT1 is associated with a lower tolerance to Cd and Zn of the yeast expressing TcMT1 when compared to AtMT1. Ectopic expression of MT1 and MT2 (from Brassica campestris) in A. thaliana enhanced the tolerance to Cd and Cu and increased the Cu concentration in the shoots of the transgenic plants. Transgenic Arabidopsis accumulated less reactive oxygen species (ROS) than wild-type plants. BcMT1 and BcMT2 increased Cd and Cu tolerance in transgenic Arabidopsis, and decreased production of Cd- and Cu-induced ROS, thereby protecting plants from oxidative damage (Lv et al., 2013). The expression levels of the genes MT2a, MT2b, and MT3 showed to be much higher in T. caerulescens than in non-metallophyte, non-hyperaccumulating reference species, as shown by microarray analyses. The expression of MT2a and MT2b in the roots is much higher in T. caerulescens than in A. thaliana (van de Mortel et al., 2006). Various MTs involved for As detoxification in Oryza sativa was also reported (Gautam et al., 2012). The authors have also shown that 11 class I MT genes in rice genome that are expressed differently during the growth and development (Gautam et al., 2012) which is influenced on Cu and Cd tolerance in plants (Zhou and Goldsbrough, 1994). Arabidopsis plants overexpressing pigeon pea CcMT1 were more tolerant to Cu and Cd (Sekhar et al., 2011), while the garlic MTs AsMT2b and the Colocasia esculenta protein CeMT2b simultaneously confer Cd tolerance and promote Cd accumulation (Zhang et al., 2006). MT-like protein has also been found in Chlorella vulgaris capable of detoxifying Cd and Zn toxicities (Huang et al., 2009a). Recently, Zn (100 μ M L−1) was reported cause a higher induction of Zn-MT-like proteins (1.65-fold than control) in C. vulgaris (Yang et al., 2014). In a recent study by Nath et al. (2014), MT1 and MT2 has strongly expressed in O. sativa during 5 days of As (V) exposure. The ability of MTs to bind and sequester with metals/metalloids depends upon the distribution and organization of Cys residues and their regulated expression during stress is a major option for metal detoxification and homeostasis (Usha et al., 2009; Singh et al., 2011; Gautam et al., 2012).
Non-Thiol Compounds and Metal(loid)-Chelation
A number of non-thiol compounds such as organic acid (OAs, including citrate, malate, oxalate, malonate, aconitate, and tartrate) and amino acids and their derivatives (including glycinebetaine/betaine; proline, Pro; histidine, His; cysteine, Cys; arginine, Arg; glutamate, Glu; nicotianamine, NA) in isolation and/or in coordination with thiol compounds have been credibly evidenced to contribute to metal-chelation in plants (Hall, 2002; Sharma and Dietz, 2006; Jakkeral and Kajjidoni, 2011) (Figure 2). Hereunder follows an appraisal of recent studies on chelation of metals in plants considering the mentioned above major non-thiol compounds.
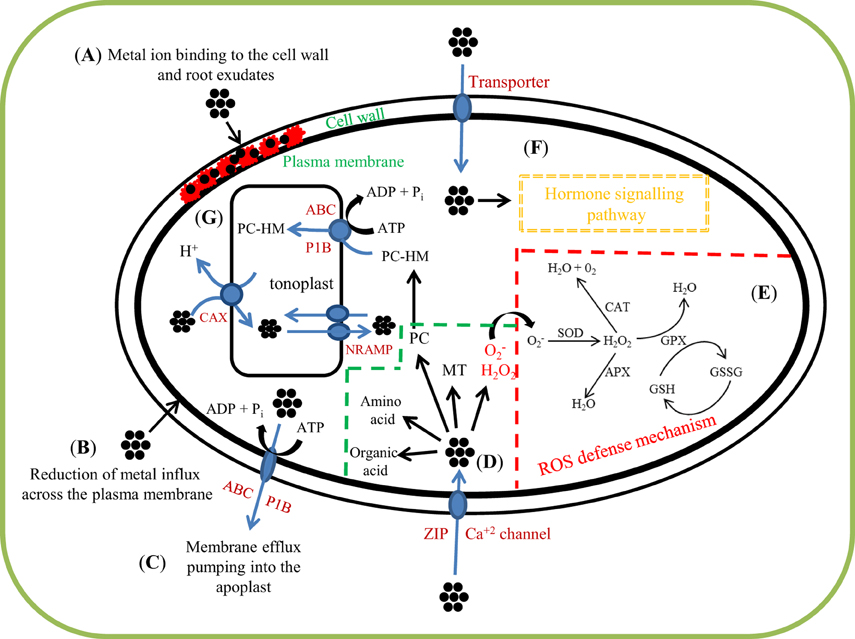
Figure 2. Schematic representation of major functions, interrelationships among thiol and non-thiol compounds, and their coordination with other defense system components in metal(loid)-exposed plants. As discussed in the text, plant responses to heavy metals include: (A) metal ion binding to the cell wall and root exudates; (B) reduction of metal influx across the plasma membrane; (C) membrane efflux pumping into the apoplast (ATP-binding-cassette (ABC) and P1B-ATPase transporter); (D) heavy metal (HM) chelation in the cytosol by ligands such as phytochelatins (PC), metallothioneins (MT), organic acids, and amino acids; (E) ROS defense mechanism [Antioxidant enzymes (SOD: superoxide dismutase, CAT: catalase, APX: ascorbate peroxidase, GPX: glutathione peroxidase, GSH: glutathione reduce and GSSG: glutathione oxidase)]; (F) hormone signaling pathway. (G) Transport and compartmentalization in the vacuole (ABC and P1B-ATPase transporter, NRAMP: natural resistance associated macrophage protein, CAX: cation/proton exchanger). Metal ions are shown as black dots.
Organic Acids
Overview
Organic acid (OAs) such as citrate, malate, oxalate, malonate, aconitate, and tartrate are low molecular weight weak-acidic compounds, possess at least one carboxyl group, and are termed as oxygen-donor metal ligands. Some of these compounds are present in all plant cells as intermediates of the tricarboxylic acid cycle (TCA), the main respiratory pathway involved in the oxidation of pyruvate (Trejo-Tellez et al., 2012). OAs potentially perform multiple functions in the rhizosphere. Contingent to the number of carboxylic groups and dissociation properties, OAs can carry varying negative charge, thereby allowing the complexation of metal cation in solution and displacement of anions from the soil-matrix (Jakkeral and Kajjidoni, 2011). Present in considerable amounts, citrate belongs to the key metabolites in plant cells. Lemons, wild strawberries and spinach leaves contain from 8 to 15% citrate, based on dry weight (Popova and Pinheiro de Carvalho, 1998). Metabolism of citrate in plants is carried out by several metabolic pathways located in different cellular compartments. Citrate has been extensively reported to be involved in carbon metabolism (as an intermediate) and plant-tolerance to varied stresses of abiotic (metals, nutrient deficiencies) and biotic (plant-microbe interactions operating at the rhizosphere) types (reviewed by Trejo-Tellez et al., 2012). Oxalate, C2 dicarboxilic acid anion is one of the strong OAs and a common constituents of plants. Though oxalate can be found in relatively small amounts in plants, it can be accumulated at high levels in several plants species (called extreme oxalate accumulators; can exhibit >3–18% oxalate based on their dry weight) from the families Caryophyllaceae, Chenopodiaceae, and Polygonaceae (Massey, 2003). Since oxalate can combine with various plant ions to from soluble or insoluble compounds, oxalate has been suggested to balance the excess of various inorganic cations (such as K+, Na+, NH+4, Ca++, and Mg++) over anions (such as NO−3, Cl−, H2PO−4, and SO2−4) (reviewed by Çalişkan, 2000). The role of oxalic acid in Al-tolerance has been well-documented (Furukawa et al., 2007; Liu et al., 2009; Maron et al., 2010; Yokosho et al., 2010). The dicarboxylic acid malate (an intermediate of the TCA cycle), an important plant metabolite, can be present in all cell types, and accumulated in plants to the levels up to 350 mM concentration. Malate in plants is involved in photosynthesis (C3, C4, and CAM plants), respiration and energy metabolism, fatty acid oxidation, stomatal, and pulvinual movement, lignin biosynthesis, nitrogen fixation, amino acid biosynthesis, ion balance, P- and Fe-uptake, and Al-tolerance (reviewed by Finkemeier and Sweetlove, 2009).
Metal(loid)-Specificity and -Chelation Mechanisms
Metal-complexation with OAs has been argued to have potential role in the long distance xylem transport of heavy metals (Rascio and Navari-Izzo, 2011). Among the various OAs studied in plants, citrate has a high capacity to chelate metal ions and has been well-documented in the case of Fe and Al (Clemens, 2001; Singh and Chauhan, 2011). However, other metals such as Zn, Co, Ni, and Cd also exhibit their strong affinity for citrate. Cd-citrate complexes were evidenced in the xylem sap of Arabidopsis hallerii (Ueno et al., 2008). Involvement of citrate was evindenced in Cu-exclusion mechanisms in non-accumulators (reviewed by Mehes-Smith et al., 2013) and Ni-exclusion (Hall, 2002). The role of Ni-complexation with citric acid in Ni-uptake and hyperaccumulation has been reported (Boominathan and Doran, 2003). Although exudation of OAs is common Al-tolerance mechanism in different plant species, there are species-specific peculiarities worth noting (Simões et al., 2012). In general, citrate has the maximum ability followed by malate and oxalate to alleviate Al-toxicity (Singh and Chauhan, 2011). The mechanism of Al-tolerance in Sorghum bicolor, Glycine max, Zea mays, and Hordeum vulgare involves mainly citrate exudation/release (Furukawa et al., 2007; Maron et al., 2010). Similarly, citrate exudation has also been found to contribute to Al-tolerance in T. aestivum, Arabidopsis, and rye (Liu et al., 2009; Yokosho et al., 2010). In O. sativa, citrate exudation (Yokosho et al., 2011) as well as symplastic mechanisms are likely to contribute to the extreme Al-tolerance in this species (Huang et al., 2009b). A correlation between citric acid exudation and Al-tolerance was detected by Miyasaka et al. (1991) in Phaseolus vulgaris. Co-occurrence of different Al-tolerance mechanisms has also been reported in some species. In Z. mays, root oxalate (Kidd et al., 2001) and citrate (Piñeros et al., 2002) exudation are likely involved in Al-tolerance. However, Piñeros et al. (2005) observed a low correlation between citrate exudation and Al tolerance in Z. mays, suggesting that this species has other complementary mechanisms enabling them to tolerate Al stress. Exposure of cells of the Co-hyperaccumulator Crotalaria cobalticola and non-accumulators Raufolia serpentina, and Silene cucubalus to Co-ions resulted in an increase of citrate, indicating the involvement OA in the complexation of metal ions (Oven et al., 2002). The Al-activated mechanism of malate exudation is well-described in a number of plants including B. napus (Ligaba et al., 2006), A. thaliana (Hoekenga et al., 2006), T. aestivum (Sasaki et al., 2004), and rye (Secale cereale) (Collins et al., 2008). Recently, Zhu et al. (2011) showed that Cd-induced oxalate secretion from root apex is associated with Cd exclusion and resistance in L. esculentum.
Considering mechanisms underlying OAs-assisted metal-chelation, OAs confer metal-tolerance by transporting metals through the xylem and sequestrating ions in the vacuole, but they have multiple additional roles in the cell (reviewed by Fernie and Martinoia, 2009; Finkemeier and Sweetlove, 2009; Yang et al., 2013). Mechanism of metal-tolerance and detoxification in plants can be divided into two categories: external exclusion and internal tolerance. In the external detoxification process, organic acids excreted from plant roots may form stable metal–ligand complexes with metal ion and change their mobility and bioavailability, thus preventing the metal ions from entering plants or avoiding their accumulation in the sensitive sites of roots. In internal metal-detoxification, OAs may chelate with metal in the cytosol, where the ions can be transformed into a non-toxic or less toxic form (Clemens, 2001; Hall, 2002). The chelation of metals with ligands, such as OAs, amino acids and thiols facilitates the movements of heavy metals from roots to shoots (Zacchini et al., 2009). The xylem cell wall has a high cation exchange capability, thus the movement of metal cations is severely retarded when the metals are not chelated by ligands. OAs are involved in the translocation of Cd in the species Brassica juncea (Salt et al., 1999). OA-mediated Al stress tolerance has been well-studied in plants (Ma et al., 2001; Singh and Chauhan, 2011; Delhaize et al., 2012).
Amino Acids and their Derivatives
Overview
Due to metal-binding capacity, amino acids and their derivatives may be deployed in response to metal-toxicity and in conferring to plants resistance to toxic levels of metal ions (Manara, 2012). However, a clear correlation between metal accumulation and the production of these compounds has not been established yet. Some of the amino acids, e.g., glycinebetaine (betaine), proline (Pro), histidine (His), cysteine (Cys), arginine (Arg), glutamate (Glu), nicotianamine (NA), and the polyamines (spermidine, Spd; spermine, Spm; putrescine, Put), are synthesized in the small (milimollar range) amount in response to metal stress (Figure 1). Thus, in many cases, nitrogen (N) metabolism is vital to the response of plants to metals (Sharma and Dietz, 2006). Based on several studies on different plants, chelation of metals by previous compounds and subsequent compartmentalization of the complexes formed with varied metals are well-established mechanisms for the detoxification of and tolerance to excess metals in plants (Hall, 2002; Sharma and Dietz, 2006).
Betaines are quaternary ammonium compounds (QACs) which contain a carboxylic acid group. They may be generally regarded as fully N-methylated amino or imino acids. There are several kinds of betaine among which glycine betaine (GB) is the most common. GB, also called as original betaine (N,N,N-trimethylglycine) was first discovered from Beta vulgaris which is later found to be distributed in microorganisms, plants and animals. It is one of the most abundant quaternary ammonium compounds those are accumulated in plants during dehydration-promoting conditions (Ashraf and Foolad, 2007). The role of GB as a significant osmoprotectant, ROS-scavenger, and metal-chelator has been reported in metal-exposed plants (Sharma and Dietz, 2006; Theriappan et al., 2011; Asgher et al., 2013; Kumchai et al., 2013; Gill et al., 2014). The other non-proteinogenic amino acid nicotianamine (NA) is ubiquitous in higher plants, and is considered as a key element in plant metal chelation and homeostasis (Takahashi et al., 2003; Rellán-Álvarez et al., 2008). The first step of NA biosynthesis is the formation of S-adenosylmethionine (SAM) from methionine by the action of S-adenosylmethionine synthetase (SAMS) (Mori et al., 2007). Arginine (Arg) is the most functionally diverse amino acid in living cells. Thus, role of Arg has come into light due to its multiple metabolic fates. Apart from serving as a constituent of proteins, Arg is a precursor for biosynthesis of other substances like polyamines (PAs), agmatine and Pro, Glu and nitric oxide (NO) those play vital role in metal stress tolerance (Liu et al., 2006; Nasibi et al., 2013). Arg biosynthesis in plant occurs through ornithine (Orn) which comes from few steps conversion of Glu involving five enzymes (Verma and Zhang, 1999). These compounds including NA, PAs, Pro, Glu, and NO play vital role in metal chelation and detoxification in plants (Hasanuzzaman and Fujita, 2013; Hasanuzzaman et al., 2013, 2014).
PAs are ubiquitous low molecular weight polycationic aliphatic amines have roles in plant growth, development and senescence. Diamine Put [NH2(CH2)4NH2], triamine Spd [NH2(CH2)3NH(CH2)4NH2], tetramine Spm [NH2(CH2)3NH(CH2)4NH(CH2)3NH2] are most common PAs in higher plants which exist as soluble conjugated, and insoluble bound forms and differential forms of PAs function differently (Lefevre et al., 2001). Some other PAs homospermidine, 1,3-diaminopropane, cadaverine, and canavalmine also common in some plants, animals, algae, and bacteria (Valero et al., 2002). Diversified properties of PAs including acid neutralizing, antioxidant properties, membrane and cell wall stabilizing abilities make those potent protectants against environmental stresses (Zhao and Yang, 2008). Both endogenous PAs and exogenous application of PAs confer tolerance against different stresses including metals (Groppa et al., 2003; Wang et al., 2007; Hasanuzzaman et al., 2014).
Metal(loid)-Specificity and -Chelation Mechanisms
Literature is scarce on the specificity of amino acids and their derivatives to varied metals. However, plants exposed to varied metals (such as Cd, Cu, Ni, and Zn) can accumulate and synthesize different N-containing metabolites including Pro, amino acids, and oligopeptides, betaine, PAs, and NA. Pro and His have metal-binding, antioxidant, and signaling functions and can be accumulated in plants in response to different metals including As, Cd, Cu, Hg, and Ni (Kerkeb and Krämer, 2003; Sharma and Dietz, 2006; Irtelli et al., 2009; Richau and Schat, 2009; Richau et al., 2009; Theriappan et al., 2011; Ahmad and Gupta, 2013; Anjum et al., 2014b; Gill et al., 2014). NA and His/Pro were among the most important Cu-chelators in xylem sap of Brassica carinata under conditions of Cu deficiency and excess, respectively (Irtelli et al., 2009). Elevated accumulation of NA was evident different plants exposed to a number of metals such as Cd, Cu, Fe, Ni, and Zn (Takahashi et al., 2003; Vacchina et al., 2003; Kim et al., 2005; Irtelli et al., 2009; Kawachi et al., 2009; reviewed by Hassan and Aarts, 2011 and Mehes-Smith et al., 2013). In fact, the presence of six functional groups in NA allows its octahedral coordination and an optimal structure ideal for chelation of metal ions (Rellán-Álvarez et al., 2008). Notably, the pK of the resulting “metal-NA complexes” as well as the pH of the solution (with most metals being chelated at neutral or basic pH values) were considered to significantly control the ability of NA to chelate metals (Rellán-Álvarez et al., 2008). Apart from acting as an antioxidant, PAs function mainly as signaling molecule and can activate metal detoxification mechanisms (Sharma and Dietz, 2006). PAs are strongly supported to stabilize and protect membrane from toxic effects of metal ions specially the redox active metals (Sharma and Dietz, 2006). As a cation PAs can mimic and compete for the binding with Mg2+ and Ca2+ on receptors, membranes and enzymes. Polyamines bind cations including Cu, Fe that protects cell. The roles of PAs as metal chelators are contradictory. But some other N-containing compounds like porphyrins and chlorophylls strongly bound Cu, Fe, Co, and Ni by forming complexes. Presences of complex groups in same molecule regulate chelation effects. That is why complexes of PAs with high number of N-groups supposed to enhance chelation mechanism (Løvaas, 1997).
Amino acids and their derivatives exhibit their affinity to different metals, play vital role in their chelation and subsequently confer metal stress tolerance in plants. However, potential mechanisms underlying amino acids and their derivatives-assisted metal-chelation are not so conclusive in the literature. Both Pro and GB are potential osmoprotectants and they are mostly studied in plants grown under salinity and drought stress. However, role of Pro and GB in osmoprotection, ROS-scavenging, and metal-chelation has also been studied in many metal-exposed plants (Sharma and Dietz, 2006; Theriappan et al., 2011; Asgher et al., 2013; Kumchai et al., 2013; Gill et al., 2014). In addition, involvement of the elevated level of Pro in regulation of expression of genes of PCS, metallothionine-2 (MT-2), glutathione reductase (GR), and glutathione synthetase (GS) was recently reported in As-exposed plants (Ahmad and Gupta, 2013). Though Pro and GB do not take part directly in metal-chelation, they stabilize native state of proteins by regulating their water and hence these osmoprotectants (especially Pro) help in maintaining the conformational characteristics and integrity of proteins (Paleg et al., 1981). It may happen due to the capacity of Pro to increase surface tension of water and thus can force water-protein interfaces into contact. This promotes proteins to maintain more native/folded configuration (Arakawa and Timasheff, 1985). Among the amino acids Pro accumulation is generally the highest under metal exposure which may be more than 20-fold in some species like S. vulgaris (Sharma and Dietz, 2006). However, their accumulation also varied depending on the metals where the plants were grown. Increased level of Pro was observed in B. oleracea in presence of Cd and Hg as reported by Theriappan et al. (2011). The role of Pro in the chelation of metals was reviewed by Sharma and Dietz (2006). One of the important role of Pro is the enhancement of endogenous GSH level from which PCs are synthesized, where the metal binds to the constitutively expressed enzyme PC synthase (PCS), thereby activating it to catalyze the conversion of GSH to PC (Zenk, 1996). Pro-mediated enzyme-protection can be possible via Pro-assisted reduction of free metal ion activity as a result of “metal(Zn/Cd)-Pro-complex” formation (Sharma et al., 1998).
Metal stress tolerance is enhanced by PAs as a result of their multiple roles such as the regulation of endogenous hormones (including IAA; abscisic acid, ABA) and antioxidants or ROS-scavengers (Groppa et al., 2007; Wang et al., 2007; Zhao et al., 2008; Choudhary et al., 2012a). Additionally, a number of PA-gene-expressing transgenic plants have been developed exhibiting enhanced metal-tolerance (Table 1). However, a direct role of PAs in metal-chelation has been least explored. To this end, Put-mediated activation of long-distance Ni-transport within the plant was argued to enhance plant-Ni tolerance (Shevyakova et al., 2011). Spd-induced mitigation of Cr-toxicity was related with reduced Cr-uptake and enhanced titers of PCs (Choudhary et al., 2012b). Put was reported to exhibit a selective effect on ion flux in Cd and Pb exposed plant leaves (Lakra et al., 2006). In many studies, NA was found to play a vital role in Cu-complexation in plants (Takahashi et al., 2003; Kim et al., 2005; Irtelli et al., 2009). Role of NA in the intracellular delivery of metals (and also plant reproductive development) has been reported (Takahashi et al., 2003). In Ni-hyperaccumulator, T. caerulescens, NA was found to chelate Ni in the xylem as a response to toxic levels of external Ni, and eventually provide Ni-tolerance (Vacchina et al., 2003). Additionally, NA was reported to perform the chelation and transportation of Fe and few divalent metal ions like Zn, Ni, and Cu in plants (reviewed by Hassan and Aarts, 2011). High levels of Zn, Cd, Cu, Fe, and/or Ni can significantly induce the expression of NA synthase genes (responsible for the synthesis of NA by trimerization of S-adenosylmethionine) (reviewed by Hassan and Aarts, 2011; Mehes-Smith et al., 2013). Over-expression of the T. caerulescens NAS3 gene in the Ni-excluder A. thaliana was reported to improve its Ni-tolerance and Ni-accumulation in their aerial organs (Pianelli et al., 2005). Similarly, overexpression of the NAS3 gene led to increased accumulation of Fe, Zn, and Cu in O. sativa (Kawachi et al., 2009).
Histidine (His) is a major N-donor ligand important for metal chelation in (metal hyperaccumulator) plants. Having carboxyl, amino, and imidazole groups as major structural components, His has been considered as a versatile chelator of metals (such as Ni) in plants (Callahan et al., 2006). Metal-chelation role of free His has been extensively reported in plants exposed to varied metals including Ni (Kerkeb and Krämer, 2003; Richau and Schat, 2009; Richau et al., 2009). Nevertheless, the role of elevated free root cell-His in reduced Ni-vacuolar sequestration and enhanced Ni-xylem loading was evidenced (Richau et al., 2009; Richau and Schat, 2009). To this end, higher free His concentration in roots but less Ni-in root vacuoles were evidenced in T. caerulescens (Ni-hyperaccumulator) when compared to Thlaspi arvense (non-Ni hyperaccumulator) (Richau et al., 2009). It was advocated that the His-Ni complexes were much less taken up by vacuoles than free Ni ions. Further, an inhibited vacuolar sequestration of “His-Ni complexes” in T. caerulescens roots was argued as a result of a higher increase in free His therein (hence an enhanced His-mediated Ni-xylem loading) compared to free Ni in non-Ni-hyperaccumulator T. arvense (Richau and Schat, 2009; Richau et al., 2009). In Ni-exposed Alyssum lesbiacum and B. juncea, Ni uptake was not highly correlated with His uptake but the release of Ni into the xylem was associated with a concomitant release of His from an increased root free His pool (Kerkeb and Krämer, 2003). However, in Alyssum montanum (and also in B. juncea), these authors evidenced a role of exogenously applied His in conferring enhanced Ni-tolerance possibly as a result of enhanced Ni-flux into the xylem. Enhanced tolerance to excess Ni in transgenic A. thaliana overexpressing StHisG (Salmonella typhimurium ATP phosphoribosyl transferase enzyme) was argued due to the accumulation of about 10-fold higher His level (vs. wild type) (Wycisk et al., 2004). A comprehensive study on the composition of amino acids under Cu stress was done by Irtelli et al. (2009). Among the amino acids His/Pro was found to be the most important Cu chelator in xylem sap of B. carinata under Cu toxicity. However, the accumulation of amino acids was dependent on the dose of Cu applied to plants. When B. carinata was treated with 5 μM CuSO4, the accumulation of His, threonine, Gln, glycine, Pro, and methionine was 140, 22, 7, 23, 6, and 13 μM, respectively which could make about 67, 42, 45, 52, 60, and 33% complexation of 0.94 μmol Cu under a pH of 5.8 (Irtelli et al., 2009). For every case, the accumulation of amino acids was pH dependent. More importantly, in the absence of His, Pro was found to play a very important role in Cu binding and the role of other amino acids (threonine, Gln, glycine, and methionine were negligible in presence of His and Pro (Irtelli et al., 2009). Although other amino acids such as NA was not efficiently involved in the response to excess of Cu but it participates in Cu-transport to the shoots in conditions of deficiency (Irtelli et al., 2009). An amino acid derivative namely 2-amino-3-(8-hydroxyquinolin-3-yl)propanoic acid (HQ-Ala) was reported to form highly stable complexes with most transition metal ions via its metal ion chelating group 8-hydroxyquinoline Lee et al. (2009). Cys can also perform a direct chelation of metals (such as Cd) by synthesizing methionine and GSH/PCs that in turn can sequester metals and provide a higher antioxidant defense in plants (Dominguez-Solis et al., 2004).
Conclusions and Future Prospects
Plant tolerance to metal-load-accrued impacts largely decides the efficiency and success of a metal-remediation system (Vangronsveld et al., 2009; Maestri et al., 2010; Anjum et al., 2014a,b,c). Hence, a sound knowledge is essential on the compounds that help plants to keep a tight control over concentrations of free metal(loid)s in cytoplasm. Considering recent reports, this paper attempted to present an orchestrated overview of both thiol- and non-thiol compounds that play significant roles in metal-chelation and maintain low concentrations of free metal(loid)s in cytoplasm. PCs and MTs are among the best characterized –SH compounds that strongly interact with metals, chelate them, reduce their concentrations in cytosol, and finally limit their potential toxicity (Cobbett and Goldsbrough, 2002; Solanki and Dhankhar, 2011; Hossain and Komatsu, 2013). To the other, OAs such as citrate, malate, oxalate, malonate, aconitate, and tartrate, and amino acids and their derivatives including GB, Pro, His, and NA are among non-GSH associated compounds and have also been extensively evidenced to contribute to metal-chelation in plants (Hall, 2002; Sharma and Dietz, 2006; Jakkeral and Kajjidoni, 2011). Both thiol- and non-thiol compounds are functionally related, and may coordinate with other defense system components in order to chelate and/or detoxify and tolerate potential metal impact in plants (Figure 2). The reports appraised herein evidenced extensive studies on thiol-compounds in particular context with metal-tolerance in plants; in contrast, reports on non-thiol compounds is rare. Nevertheless, the literature reviewed herein points toward the need of more molecular-genetic studies in order to get more insights into synthesis pathways, metal-specificity, and biological and non-biological factors responsible for the induction/expression of, and potential coordination among mechanisms underlying thiol- and non-thiol compounds-assisted metal-chelation. Together, the suggested studies will help to develop at large scale the transgenic plants with exceptional capacity to extract, chelate different metals and avert their potential toxic consequences.
Conflict of Interest Statement
The authors declare that the research was conducted in the absence of any commercial or financial relationships that could be construed as a potential conflict of interest.
Acknowledgments
NAA (SFRH/BPD/64690/2009), AD, EP, and IA are grateful to the Portuguese Foundation for Science and Technology (FCT) and the Aveiro University Research Institute/Centre for Environmental and Marine Studies (CESAM) for partial financial supports. SSG acknowledges the funds received from DST-SERB, CSIR and UGC, Government of India, New Delhi. Financial support generously provided by NANOSEMED KAN208130801 is greatly acknowledged by MR, VA, and RK. Authors apologize if some references related to the main theme of the current review could not be cited due to space constraint. AR acknowledges SERB-DST and CSIR, Government of India, for providing financial support.
References
Ahmad, M. A., and Gupta, M. (2013). Exposure of Brassica juncea (L) to arsenic species in hydroponic medium: comparative analysis in accumulation and biochemical and transcriptional alterations. Environ. Sci. Pollut. Res. 20, 8141–8150. doi: 10.1007/s11356-013-1632-y
PubMed Abstract | Full Text | CrossRef Full Text | Google Scholar
Ahner, B. A., Kong, S., and Morel, F. M. M. (1995). Phytochelatin production in marine algae. 1. An interspecies comparison. Limnol. Ocenogr. 40, 649–657. doi: 10.4319/lo.1995.40.4.0649
Ali, H., Khan, E., and Sajad, M. A. (2013). Phytoremediation of heavy metals–concepts and applications. Chemosphere 91, 869–881. doi: 10.1016/j.chemosphere.2013.01.075
PubMed Abstract | Full Text | CrossRef Full Text | Google Scholar
Alloway, B. (2013). “Heavy metals and metalloids as micronutrients for plants and animals,” in Heavy Metals in Soils, ed B. J. Alloway (Dordrecht: Springer), 195–209.
Andra, S. S., Datta, R., Sarkar, D., Makris, K. C., Mullens, C. P., Sahi, S. V., et al. (2010). Synthesis of phytochelatins in vetiver grass upon lead exposure in the presence of phosphorus. Plant Soil 326, 171–185. doi: 10.1007/s11104-009-9992-2
Anjum, N. A., Ahamd, I., Mohmood, I., Pacheco, M., Duarte, A. C., Pereira, E., et al. (2012b). Modulation of glutathione and its related enzymes in plants' responses to toxic metals and metalloids–a review. Environ. Exp. Bot. 75, 307–324. doi: 10.1016/j.envexpbot.2011.07.002
Anjum, N. A., Aref, I. M., Duarte, A. C., Pereira, E., Ahmad, I., and Iqbal, M. (2014d). Glutathione and proline can coordinately make plants withstand the joint attack of metal(loid) and salinity stresses. Front. Plant Sci. 5:662. doi: 10.3389/fpls.2014.00662
PubMed Abstract | Full Text | CrossRef Full Text | Google Scholar
Anjum, N. A., Gill, S. S., and Gill, R. (2014b). Plant Adaptation to Environmental Change: Significance of Amino Acids and their Derivatives, 1st Edn. Wallingford, UK: CABI.
Anjum, N. A., Israr, M., Duarte, A. C., Pereira, M. E., and Ahmad, I. (2014c). Halimione portulacoides (L.) physiological/biochemical characterization for its adaptive responses to environmental mercury exposure. Environ. Res. 131, 39–49. doi: 10.1016/j.envres.2014.02.008
PubMed Abstract | Full Text | CrossRef Full Text | Google Scholar
Anjum, N. A., Pereira, M. E., Ahmad, I., Duarte, A. C., Umar, S., and Khan, N. A. (2012a). Phytotechnologies: Remediation of Environmental Contaminants. Boca Raton, FL: CRC Press.
Anjum, N. A., Singh, H. P., Khan, M. I. R., Masood, A., Per, T. S., Negi, A. et al. (2015). Too much is bad – an appraisal of phytotoxicity of elevated plant-beneficial heavy metal ions. Environ. Sci. Pollut. Res. 22, 3361–3382. doi: 10.1007/s11356-014-3849-9
PubMed Abstract | Full Text | CrossRef Full Text | Google Scholar
Anjum, N. A., Umar, S., and Chan, M. T. (2010). Ascorbate-Glutathione Pathway and Stress Tolerance in Plants. Dordrecht: Springer. doi: 10.1007/978-90-481-9404-9
Anjum, N. A., Umar, S., and Iqbal, M. (2014a). Assessment of cadmium accumulation, toxicity, and tolerance in Brassicaceae and Fabaceae plants – implications for phytoremediation. Environ. Sci. Pollut. Res. 21, 10286–10293. doi: 10.1007/s11356-014-2889-5
PubMed Abstract | Full Text | CrossRef Full Text | Google Scholar
Arakawa, T., and Timasheff, S. N. (1985). The stabilization of proteins by osmolytes. Biophys. J. 47, 411–414. doi: 10.1016/S0006-3495(85)83932-1
PubMed Abstract | Full Text | CrossRef Full Text | Google Scholar
Arrivault, S., Senger, T., and Kramer, U. (2006). The Arabidopsis metal tolerance protein AtMTP3 maintains metal homeostasis by mediating Zn exclusion from the shoot under Fe deficiency and Zn oversupply. Plant J. 46, 861–879. doi: 10.1111/j.1365-313X.2006.02746.x
PubMed Abstract | Full Text | CrossRef Full Text | Google Scholar
Asgher, M., Khan, M. I. R., Iqbal, N., Masood, A., and Khan, N. A. (2013). Cadmium tolerance in mustard cultivars: dependence on proline accumulation and nitrogen assimilation. J. Funct. Environ. Bot. 3, 30–42. doi: 10.5958/j.2231-1750.3.1.005
Ashraf, M., and Foolad, M. R. (2007). Roles of glycine betaine and proline in improving plant abiotic stress resistance. Environ. Exp. Bot. 59, 206–216. doi: 10.1016/j.envexpbot.2005.12.006
Auguy, F., Fahr, M., Moulin, P., Brugel, A., Laplaze, L., El Mzibri, M., et al. (2013). Lead tolerance and accumulation in Hirschfeldia incana, a Mediterranean Brassicaceae from metalliferous mine spoils. PLoS ONE 8:e61932. doi: 10.1371/journal.pone.0061932
PubMed Abstract | Full Text | CrossRef Full Text | Google Scholar
Baker, A. J. M., and Whiting, S. N. (2002). In search of the Holy Grail: a further step in understanding metal hyperaccumulation. New Phytol. 155, 1–4. doi: 10.1046/j.1469-8137.2002.00449_1.x
Bilecen, K., Ozturk, U. H., Duru, A. D., Sutlu, T., Petoukhov, M. V., Svergun, D. I., et al. (2005). Triticum durum metallothionein–Isolation of the gene and structural characterization of the protein using solution scattering and molecular modeling. J. Biol. Chem. 280, 13701–13711. doi: 10.1074/jbc.M412984200
PubMed Abstract | Full Text | CrossRef Full Text | Google Scholar
Blindauer, C. A. (2008). Metallothioneins with unusual residues: histidines as modulators of zinc affinity and reactivity. J. Inorg. Biochem. 102, 507–521. doi: 10.1016/j.jinorgbio.2007.10.032
PubMed Abstract | Full Text | CrossRef Full Text | Google Scholar
Bolan, N., Kunhikrishnan, A., Thangarajan, R., Kumpiene, J., Park, J., Makino, T., et al. (2014). Remediation of heavy metal (loid) s contaminated soils – to mobilize or to immobilize? J. Hazard. Mater. 266, 141–166. doi: 10.1016/j.jhazmat.2013.12.018
PubMed Abstract | Full Text | CrossRef Full Text | Google Scholar
Boominathan, R., and Doran, P. M. (2003). Organic acid complexation, heavy metal distribution and the effect of ATPase inhibition in hairy roots of hyperaccumulator plant species. J. Biotechnol. 101, 131–146. doi: 10.1016/S0168-1656(02)00320-6
PubMed Abstract | Full Text | CrossRef Full Text | Google Scholar
Bruns, I., Sutter, K., Menge, S., Neumann, D., and Krauss, G. J. (2001). Cadmium lets increase the glutathione pool in bryophytes. J. Plant Physiol. 158, 79–89. doi: 10.1078/0176-1617-00071
Bittsánszkya, A., Kfmives, T., Gullner, G., Gyulai, G., Kiss, J., Heszky, L., et al. (2005). Ability of transgenic poplars with elevated glutathione content to tolerate zinc(2+) stress. Environ. Int. 31, 251–254. doi: 10.1016/j.envint.2004.10.001
PubMed Abstract | Full Text | CrossRef Full Text | Google Scholar
Callahan, D. L., Baker, A. J. M., Kolev, S. D., and Wedd, A. G. (2006). Metal ion ligands in hyperaccumulating plants. J. Biol. Inorg. Chem. 11, 2–12. doi: 10.1007/s00775-005-0056-7
PubMed Abstract | Full Text | CrossRef Full Text | Google Scholar
Cánovas, D., Vooijs, R., Schat, H., and De Lorenzo, V. (2004). The role of thiol species in the hypertolerance of Aspergillus sp. P37 to arsenic. J. Biol. Chem. 279, 51234–51240. doi: 10.1074/jbc.M408622200
PubMed Abstract | Full Text | CrossRef Full Text | Google Scholar
Choudhary, S. P., Kanwar, M., Bhardwaj, R., Yu, J. Q., and Tran, L. S. P. (2012b). Chromium stress mitigation by polyamine-brassinosteroid application involves phytohormonal and physiological strategies in Raphanus sativus L. PLoS ONE 7:e33210. doi: 10.1371/journal.pone.0033210
Choudhary, S. P., Oral, H. V., Bhardwaj, R., Yu, J. Q., and Tran, L. S. P. (2012a). Interaction of brassinosteroids and polyamines enhances copper stress tolerance in Raphanus sativus. J. Exp. Bot. 63, 5659–5675. doi: 10.1093/jxb/ers219
PubMed Abstract | Full Text | CrossRef Full Text | Google Scholar
Clemens, S. (2001). Molecular mechanisms of plant metal tolerance and homeostasis. Planta 212, 475–486. doi: 10.1007/s004250000458
PubMed Abstract | Full Text | CrossRef Full Text | Google Scholar
Clemens, S. (2006). Evolution and function of phytochelatin synthases. J. Plant Physiol. 163, 319–332. doi: 10.1016/j.jplph.2005.11.010
PubMed Abstract | Full Text | CrossRef Full Text | Google Scholar
Cobbett, C., and Goldsbrough, P. (2002). Phytochelatins and metallothioneins: roles in heavy metal detoxification and homeostasis. Annu. Rev. Plant Biol. 53, 159–182. doi: 10.1146/annurev.arplant.53.100301.135154
PubMed Abstract | Full Text | CrossRef Full Text | Google Scholar
Collins, N. C., Shirley, N. J., Saeed, M., Pallotta, M., and Gustafson, J. P. (2008). An ALMT1 gene cluster controlling aluminum tolerance at the Alt4 locus of rye (Secale cereale L.). Genetics 179, 669–682. doi: 10.1534/genetics.107.083451
PubMed Abstract | Full Text | CrossRef Full Text | Google Scholar
Courbot, M., Diez, L., Ruotola, R., Chalot, M., and Leroy, P. (2004). Cadmium-responsive thiols in the ectomycorrhizal fungus Paxillus involutus. Appl. Environ. Microbiol. 70, 7413–7417. doi: 10.1128/AEM.70.12.7413-7417.2004
PubMed Abstract | Full Text | CrossRef Full Text | Google Scholar
Dago, A., González, I., Ariño, C., Díaz-Cruz, J. M., and Esteban, M. (2014). Chemometrics applied to the analysis of induced phytochelatins in Hordeum vulgare plants stressed with various toxic non-essential metals and metalloids. Talanta 118, 201–209. doi: 10.1016/j.talanta.2013.09.058
PubMed Abstract | Full Text | CrossRef Full Text | Google Scholar
Deinlein, U., Weber, M., Schmidt, H., Rensch, S., Trampczynska, A., Hansen, T. H., et al. (2012). Elevated nicotianamine levels in Arabidopsis halleri roots play a key role in Zn hyperaccumulation. Plant Cell 24, 708–723. doi: 10.1105/tpc.111.095000
PubMed Abstract | Full Text | CrossRef Full Text | Google Scholar
Delhaize, E., Ma, J. F., and Ryan, P. R. (2012). Transcriptional regulation of aluminium tolerance genes. Trend Plant Sci. 17, 341–348. doi: 10.1016/j.tplants.2012.02.008
PubMed Abstract | Full Text | CrossRef Full Text | Google Scholar
Dhankher, O. P., Li, Y., Rosen, B. P., Shi, J., Salt, D., Senecoff, J. F., et al. (2002). Engineering tolerance and hyperaccumulation of arsenic in plants by combining arsenate reductase and -glutamylcysteine synthetase expression. Nat. Biotechnol. 20, 1140–1145. doi: 10.1038/nbt747
PubMed Abstract | Full Text | CrossRef Full Text | Google Scholar
Dominguez-Solis, J. R., Lopez-Martin, M. C., Ager, M. C., Ynsa, M. D., Romero, L. C., and Gotor, C. (2004). Increased cysteine availability is essential for cadmium tolerance and accumulation in Arabidopsis thaliana. Plant Biotechnol. J. 2, 469–476. doi: 10.1111/j.1467-7652.2004.00092.x
PubMed Abstract | Full Text | CrossRef Full Text | Google Scholar
Dorcak, V., and Krezel, A. (2003). Correlation of acide-base chemistry of phytochelatin PC2 with its coordination properties towards the toxic metal ion Cd (II). Dalton. Trans. 11, 2253–2259. doi: 10.1039/b301357j
Douchkov, D., Gryczka, C., Stephan, U. W., Hell, R., and Bäumlein, H. (2005). Ectopic expression of nicotianamine synthase genes results in improved iron accumulation and increased nickel tolerance in transgenic tobacco. Plant Cell Environ. 28, 365–374. doi: 10.1111/j.1365-3040.2005.01273.x
Duan, G. L., Hu, Y., Lui, W. J., Kneer, R., Zhao, F. J., and Zhu, Y. G. (2011). Evidence for a role of phytochelatins in regulating arsenic accumulation in rice grains. Environ. Exp. Bot. 71, 416–421. doi: 10.1016/j.envexpbot.2011.02.016
Dupae, J., Bohler, S., Noben, J. P., Carpentier, S., Vangronsveld, J., and Cuypers, A. (2014). Problems inherent to a meta-analysis of proteomics data: a case study on the plants' response to Cd in different cultivation conditions. J. Proteomics 108, 30–54. doi: 10.1016/j.jprot.2014.04.029
PubMed Abstract | Full Text | CrossRef Full Text | Google Scholar
Ellis, D. R., and Salt, D. E. (2003). Plants, selenium and human health. Curr. Opin. Plant Biol. 6, 273–279. doi: 10.1016/S1369-5266(03)00030-X
Ernst, W. H. O., Krauss, G. J., Verkleij, J. A. C., and Wesenberg, D. (2008). Interaction of heavy metals with the sulphur metabolism in angiosperms from an ecological point of view. Plant Cell Environ. 31, 123–143. doi: 10.1111/j.1365-3040.2007.01746.x
PubMed Abstract | Full Text | CrossRef Full Text | Google Scholar
Estrella-Gómez, N. E., Sauri-Duch, E., Zapata-Pérez, O., and Santamaría, J. M. (2012). Glutathione plays a role in protecting leaves of Salvinia minima from Pb2+ damage associated with changes in the expression of SmGS genes and increased activity of GS. Environ. Exp. Bot. 75, 188–194. doi: 10.1016/j.envexpbot.2011.09.001
Feretti, L., Elviri, L., Pelinghelli, M. A., Prediery, G., and Tegoni, M. (2007). Glutathione and N-acetylcysteinylglycine: protonation and Zn2+ complexation. J. Inorg. Biochem. 101, 1442–1456. doi: 10.1016/j.jinorgbio.2007.06.020
PubMed Abstract | Full Text | CrossRef Full Text | Google Scholar
Fernández, R., Bertrand, A., García, J. I., Tamés, R. S., and González, A. (2012). Lead accumulation and synthesis of non-protein thiolic peptides in selected clones of Melilotus alba and Melilotus officinalis. Environ. Exp. Bot. 78, 18–24. doi: 10.1016/j.envexpbot.2011.12.016
Fernie, A. R., and Martinoia, E. (2009). Malate. Jack of all trades or master of a few? Photochemistry 70, 828–832. doi: 10.1016/j.phytochem.2009.04.023
PubMed Abstract | Full Text | CrossRef Full Text | Google Scholar
Finkemeier, I., and Sweetlove, L. J. (2009). The role of malate in plant homeostasis. Biol. Rep. 1:47. doi: 10.3410/B1-47
PubMed Abstract | Full Text | CrossRef Full Text | Google Scholar
Freisinger, E. (2008). Plant MTs-long neglected members of the metallothionein superfamily. Dalton. Trans. 47, 6663–6675 doi: 10.1039/b809789e
PubMed Abstract | Full Text | CrossRef Full Text | Google Scholar
Freisinger, E. (2011). Structural features specific to plant metallothioneins. J. Biol. Inorg. Chem. 16, 1035–1045. doi: 10.1007/s00775-011-0801-z
PubMed Abstract | Full Text | CrossRef Full Text | Google Scholar
Furukawa, J., Yamaji, N., Wang, H., Mitani, N., Murata, Y., Sato, K., et al. (2007). An aluminum-activated citrate transporter in barley. Plant Cell Physiol. 48, 1081–1091. doi: 10.1093/pcp/pcm091
PubMed Abstract | Full Text | CrossRef Full Text | Google Scholar
Gautam, N., Verma, P. K., Verma, S., Tripathi, R. D., Trivedi, P. K., Adhikari, B., et al. (2012). Genome-wide identification of rice class I metallothionein gene: tissue expression patterns and induction in response to heavy metal stress. Funct. Integr. Genomics 12, 635–647. doi: 10.1007/s10142-012-0297-9
PubMed Abstract | Full Text | CrossRef Full Text | Google Scholar
Gekeler, W., Grill, E., Winnacker, E. L., and Zenk, M. H. (1988). Algae sequester heavy metals via synthesis of phytochelatin complexes. Arch. Microbiol. 150, 197–202. doi: 10.1007/BF00425162
Gill, S. S., Anjum, N. A., Ahmad, I., Pacheco, M., Duarte, A. C., Umar, S., et al. (2012). “Metal hyperaccumulation and tolerance in Alyssum, Arabidopsis and Thlaspi,” in The Plant Family Brassicaceae: Contribution Towards Phytoremediation, eds N. A. Anjum, I. Ahmad, E. Pereira, A. C. Duarte, S. Umar, and N. A. Khan (Dordrecht: Springer), 99–137.
Gill, S. S., Gill, R., and Anjum, N. A. (2014). “Target osmoprotectants for abiotic stress tolerance in crop plants – glycine betaine and proline,” in Plant Adaptation to Environmental Change: Significance of Amino Acids and Their Derivatives, eds N. A. Anjum, S. S. Gill, and R. Gill (Wallingford, CT: CAB International), 97–108.
Gonzalez-Mendoza, D., Moreno, A. Q., and Zapata-Perez, O. (2007). Coordinated responses of phytochelatin synthase and metallothionein genes in black mangrove, Avicennia germinans, exposed to cadmium and copper. Aqu. Toxicol. 83, 306–314. doi: 10.1016/j.aquatox.2007.05.005
Groppa, M. D., Benavides, M. P., and Tomaro, M. L. (2003). Polyamine metabolism in sunflower and wheat leaf discs under cadmium or copper stress. Plant Sci. 161, 481–488. doi: 10.1016/S0168-9452(01)00432-0
Groppa, M. D., Ianuzzo, M. P., Tomaro, M. L., and Benavides, M. P. (2007). Polyamine metabolism in sunflower plants under long-term cadmium or copper stress. Amino Acids 32, 265–275. doi: 10.1007/s00726-006-0343-9
PubMed Abstract | Full Text | CrossRef Full Text | Google Scholar
Guo, J., Xu, L., Su, Y., Wang, H., Gao, S., Xu, J., et al. (2013). ScMT2-1-3, a metallothionein gene of sugarcane, plays an important role in the regulation of heavy metal tolerance/accumulation. BioMed. Res. Int. 2013:904769. doi: 10.1155/2013/904769
Guo, J., Xu, W., and Ma, M. (2012). The assembly of metals chelation by thiols and vacuolar compartmentalization conferred increased tolerance to and accumulation of cadmium and arsenic in transgenic Arabidopsis thaliana. J. Hazard Mater. 199–200, 309–313. doi: 10.1016/j.jhazmat.2011.11.008
Guo, W. J., Bundithya, W., and Goldsbrough, P. B. (2003). Characterization of the Arabidopsis metallothionein gene family: tissue-specific expression and induction during senescence and in response to copper. New Phytol. 159, 369–381. doi: 10.1046/j.1469-8137.2003.00813.x
Hall, J. L. (2002). Cellular mechanisms for heavy metal detoxification and tolerance. J. Exp. Bot. 53, 1–11. doi: 10.1093/jexbot/53.366.1
PubMed Abstract | Full Text | CrossRef Full Text | Google Scholar
Hasanuzzaman, M., and Fujita, M. (2013). Exogenous sodium nitroprusside alleviate arsenic-induced oxidative stress in wheat seedlings by enhancing antioxidant defense and glyoxalase system. Ecotoxicology 22, 584–596. doi: 10.1007/s10646-013-1050-4
Hasanuzzaman, M., Gill, S. S., and Fujita, M. (2013). “Physiological role of nitric oxide in plants grown under adverse environmental conditions,” in Plant Acclimation to Environmental Stress, eds N. Tuteja and S. S. Gill (New York, NY: Springer), 269–322.
Hasanuzzaman, M., Nahar, K., and Fujita, M. (2014). “Regulatory role of polyamines in abiotic stress tolerance in plants,” in Plant Adaptation to Environmental Change: Significance of Amino Acids and Their Derivatives, eds N. A. Anjum, S. S. Gill, and R. Gill (Wallingford, CT: CAB International), 157–193.
Hassan, Z., and Aarts, M. G. M. (2011). Opportunities and feasibilities for biotechnological improvement of Zn, Cd or Ni tolerance and accumulation in plants. Environ. Exp. Bot. 72, 53–63. doi: 10.1016/j.envexpbot.2010.04.003
Hassinen, V. H., Tervahauta, A. I., Schat, H., and Kärenlampi, S. O. (2011). Plant metallothioneins–metal chelators with ROS scavenging activity? Plant Biol. 13, 225–232. doi: 10.1111/j.1438-8677.2010.00398.x
PubMed Abstract | Full Text | CrossRef Full Text | Google Scholar
Hawrylak-Nowak, B., Dresler, S., and Wójcik, M. (2014). Selenium affects physiological parameters and phytochelatins accumulation in cucumber (Cucumis sativus L.) plants grown under cadmium exposure. Sci. Hortic. 172, 10–18. doi: 10.1016/j.scienta.2014.03.040
Haydon, M. J., Kawachi, M., Wirtz, M., Hillmer, S., Hell, R., and Krämer, U. (2012). Vacuolar nicotianamine has critical and distinct roles under iron deficiency and for zinc sequestration in Arabidopsis. Plant Cell 24, 724–737. doi: 10.1105/tpc.111.095042
PubMed Abstract | Full Text | CrossRef Full Text | Google Scholar
Hoekenga, O. A., Maron, L. G., Piñeros, M. A., Cancado, G. M., and Shaff, J., et al. (2006). AtALMT1, which encodes a malate transporter, is identified as one of several genes critical for aluminum tolerance in Arabidopsis. Proc. Natl. Acad. Sci. U.S.A. 103, 9738–9743. doi: 10.1073/pnas.0602868103
PubMed Abstract | Full Text | CrossRef Full Text | Google Scholar
Hossain, Z., and Komatsu, S. (2013). Contribution of proteomic studies towards understanding plant heavy metal stress response. Front. Plant Sci. 3:310. doi: 10.3389/fpls.2012.00310
PubMed Abstract | Full Text | CrossRef Full Text | Google Scholar
Hu, S., Lau, K. W. K., and Wu, M. (2001). Cadmium sequestration in Chlamydomonas reinhardtii. Plant Sci. 161, 987–996. doi: 10.1016/S0168-9452(01)00501-5
Huang, C. F., Yamaji, N., Mitani, N., Yano, M., Nagamura, Y., and Ma, J. F. (2009b). A bacterial-type ABC transporter is involved in aluminum tolerance in rice. Plant Cell 21, 655–667. doi: 10.1105/tpc.108.064543
PubMed Abstract | Full Text | CrossRef Full Text | Google Scholar
Huang, Z. Y., Li, L. P., Huang, G. L., Yan, Q. P., Shi, B., and Xu, X. Q. (2009a). Growth-inhibitory and metal binding proteins in Chlorella vulgaris exposed to cadmium or zinc. Aquat. Toxicol. 91, 54–61. doi: 10.1016/j.aquatox.2008.10.003
PubMed Abstract | Full Text | CrossRef Full Text | Google Scholar
Ingle, R. A., Mugford, S. T., Rees, J. D., Campbell, M. M., and Smith, J. A. C. (2005). Constitutively high expression of the histidine biosynthetic pathway contributes to nickel tolerance in hyperaccumulator plants. Plant Cell, 17, 2089–2106. doi: 10.1105/tpc.104.030577
PubMed Abstract | Full Text | CrossRef Full Text | Google Scholar
Inouhe, M. (2005). Phytochelatins. Braz. J. Plant Physiol. 17, 65–78. doi: 10.1590/S1677-04202005000100006
Irtelli, B., Petrucci, W. A., and Navari-Izzo, F. (2009). Nicotianamine and histidine/proline are, respectively, the most important copper chelators in xylem sap of Brassica carinata under conditions of copper deficiency and excess. J. Exp. Bot. 60, 269–277. doi: 10.1093/jxb/ern286
PubMed Abstract | Full Text | CrossRef Full Text | Google Scholar
Jakkeral, S. A., and Kajjidoni, S. T. (2011). Root exudation of organic acids in selected genotypes under phosphorus deficient condition in blackgram (Vigna mungo L. Hepper). Karnataka J. Agric. Sci. 24, 316–319.
Jozefczak, M., Remans, T., Vangronsveld, J., and Cuypers, A. (2012). Glutathione is a key player in metal-induced oxidative stress defenses. Int. J. Mol. Sci. 13, 3145–3175. doi: 10.3390/ijms13033145
PubMed Abstract | Full Text | CrossRef Full Text | Google Scholar
Kalinowska, R., and Pawlik-Skowrónska, B. (2010). Response of two terrestrial green microalgae (Chlorophyta, Trebouxiophyceae) isolated from Cu-rich and unpolluted soils to copper stress. Environ. Pollut. 158, 2778–2785. doi: 10.1016/j.envpol.2010.03.003
Kanneganti, V., and Gupta, A. K. (2008). Wall associated kinases from plants – an overview. Physiol. Mol. Biol. Plant. 14, 109–118. doi: 10.1007/s12298-008-0010-6
PubMed Abstract | Full Text | CrossRef Full Text | Google Scholar
Kawachi, M., Kobae, Y., Mori, H., Tomioka, R., Lee, Y., and Maeshima, M. (2009). A mutant strain Arabidopsis thaliana that lacks vacuolar membrane zinc transporter MTP1 revealed the latent tolerance to excessive zinc. Plant Cell Physiol. 50, 1156–1170. doi: 10.1093/pcp/pcp067
Kerkeb, L., and Krämer, U. (2003). The role of free histidine in xylem loading of nickel in Alyssum lesbiacum and Brassica juncea. Plant Physiol. 131, 716–724. doi: 10.1104/pp102.010686
PubMed Abstract | Full Text | CrossRef Full Text | Google Scholar
Kidd, P. S., Llugany, M., Poschenrieder, C., Gunse, B., and Barcelo, J. (2001). The role of root exudates in aluminium resistance and silicon-induced amelioration of aluminium toxicity in three varieties of maize (Zea mays L.). J. Exp. Bot. 52, 1339–1352. doi: 10.1093/jexbot/52.359.1339
PubMed Abstract | Full Text | CrossRef Full Text | Google Scholar
Kim, S., Takahashi, M., Higuchi, K., Tsunoda, K., Nakanishi, H., Yoshimura, E., et al. (2005). Increased nicotianamine biosynthesis confers enhanced tolerance of high levels of metals, in particular nickel, to plants. Plant Cell Physiol. 46, 1809–1818. doi: 10.1093/pcp/pci196
PubMed Abstract | Full Text | CrossRef Full Text | Google Scholar
Kopriva, S., Wiedemann, G., and Reski, R. (2007). Sulfate assimilation in basal land plants–what does genomic sequencing tell us? Plant Biol. 9, 556–564. doi: 10.1055/s-2007-965430
PubMed Abstract | Full Text | CrossRef Full Text | Google Scholar
Kumchai, J., Huang, J. Z., Lee, C. Y., Chen, F. C., and Chin, S. W. (2013). Proline partially overcomes excess molybdenum toxicity in cabbage seedlings grown in vitro. Genet. Mol. Res. 12, 5589–5601. doi: 10.4238/2013.November.18.8
PubMed Abstract | Full Text | CrossRef Full Text | Google Scholar
Lakra, N., Mishra, S. N., Singh, D. B., and Tomar, P. C. (2006). Exogenous putrescine effect on cation concentration in leaf of Brassica juncea seedlings subjected to Cd and Pb along with salinity stress. J. Environ. Biol. 27, 263–269.
Lal, N. (2010). “Molecular mechanisms and genetic basis of heavy metal toxicity and tolerance in plants,” in Plant Adaptation and Phytoremediation, eds M. Ashraf, M. Ozturk, and M. S. A. Ahmad (Dordrecht: Springer), 35–58.
Lee, H. S., Spraggon, G., Schultz, P. G., and Wang, F. (2009). Genetic incorporation of a metal-ion chelating amino acid into proteins as a biophysical probe. J. Am. Chem. Soc. 131, 2481–2483. doi: 10.1021/ja808340b
PubMed Abstract | Full Text | CrossRef Full Text | Google Scholar
Lee, M., Lee, K., Lee, J., Noh, E. W., and Lee, Y. (2005). AtPDR12 contributes to lead resistance in Arabidopsis. Plant Physiol. 138, 827–836. doi: 10.1104/pp.104.058107
PubMed Abstract | Full Text | CrossRef Full Text | Google Scholar
Lefevre, I., Gratia, E., and Lutts, S. (2001). Discrimination between the ionic and osmotic components of salt stress in relation to free polyamine level in rice (Oryza sativa). Plant Sci. 16, 943–952. doi: 10.1016/S0168-9452(01)00485-X
Li, Y., Dankher, O. P., Carreira, L., Smith, A. P., and Meagher, R. B. (2006). The shoot-specific expression of γ-glutamylcysteine synthetase directs the longdistance transport of thiol-peptides to roots conferring tolerance to mercury and arsenic. Plant Physiol. 141, 288–298. doi: 10.1104/pp.105.074815
Li, Y., Dhankher, O., Carreira, L., Balish, R., and Meagher, R. (2005). Engineered overexpression of γ-glutamylcysteine synthetase in plants confers high level arsenic and mercury tolerance. Environ. Toxicol. Chem. 24, 1376–1386. doi: 10.1897/04-340R.1
Ligaba, A., Katsuhara, M., Ryan, P. R., Shibasaka, M., and Matsumoto, H. (2006). The BnALMT1 and BnALMT2 genes from rape encode aluminum-activated malate transporters that enhance the aluminum resistance of plant cells. Plant Physiol. 142, 1294–1303. doi: 10.1104/pp.106.085233
PubMed Abstract | Full Text | CrossRef Full Text | Google Scholar
Liu, J. H., Nada, K., Honda, C., Kitashiba, H., and Wen, X. P. (2006). Polyamine biosynthesis of apple callus under salt stress. Importance of the arginine decarboxylase pathway in stress responses. J. Exp. Bot. 57, 2589–2599. doi: 10.1093/jxb/erl018
PubMed Abstract | Full Text | CrossRef Full Text | Google Scholar
Liu, T., Liu, S., Guan, H., Ma, L., Chen, Z., Gu, H., et al. (2009). Transcriptional profiling of Arabidopsis seedlings in response to heavy metal lead (Pb). Environ. Exp. Bot. 67, 377–386. doi: 10.1016/j.envexpbot.2009.03.016
Løvaas, E. (1997). Antioxidant and metal-chelating effects of polyamines. Adv. Pharmacol. 38, 119–149. doi: 10.1016/S1054-3589(08)60982-5
Lv, Y., Deng, X., Quan, L., Xia, Y., and Shen, Z. (2013). Metallothioneins BcMT1 and BcMT2 from Brassica campestris enhance tolerance to cadmium and copper and decrease production of reactive oxygen species in Arabidopsis thaliana. Plant Soil 367, 507–519. doi: 10.1007/s11104-012-1486-y
Ma, J. F., Ryan, P. R., and Delhaize, E. (2001). Aluminium tolerance in plants and the complexing role of organic acids. Trends Plant Sci. 6, 273–278. doi: 10.1016/S1360-1385(01)01961-6
PubMed Abstract | Full Text | CrossRef Full Text | Google Scholar
Maestri, E., Marmiroli, M., Visioli, G., and Marmiroli, N. (2010). Metal tolerance and hyperaccumulation: costs and trade-offs between traits and environment. Environ. Exp. Bot. 68, 1–13. doi: 10.1016/j.envexpbot.2009.10.011
Manara, A. (2012). “Plant responses to heavy metal toxicity,” in Plants and Heavy Metals, ed A. Furini (New York, NY: Springer), 27–53.
Margoshes, M., and Vallee, B. L. (1957). A cadmium protein from equine kidney cortex. J. Am. Chem. Soc. 79, 4813–4814. doi: 10.1021/ja01574a064
Mari, S., and Lebrun, M. (2006). “Metal immobilization: where and how?,” in Molecular Biology of Metal Homeostasis and Detoxification: From Microbes to Man, eds M. J. Tamàs and E. Martinoia (Berlin: Springer), 273–298.
Marmiroli, M., Pigoni, V., Savo-Sardaro, M. L., and Marmiroli, N. (2014). The effect of silicon on the uptake and translocation of arsenic in tomato (Solanum lycopersicum L.). Environ. Exp. Bot. 99, 9–17. doi: 10.1016/j.envexpbot.2013.10.016
Maron, L. G., Piñeros, M. A., Guimarães, C. T., Magalhaes, J. V., Pleiman, J. K., Mao, C., et al. (2010). Two functionally distinct members of the MATE (multi-drug and toxic compound extrusion) family of transporters potentially underlie two major aluminum tolerance QTLs in maize. Plant J. 61, 728–740. doi: 10.1111/j.1365-313X.2009.04103.x
PubMed Abstract | Full Text | CrossRef Full Text | Google Scholar
Massey, L. (2003). Dietary influences on urinary oxalate and risk of kidney stones. Front. Biosci. 8:s584–94. doi: 10.2741/1082
PubMed Abstract | Full Text | CrossRef Full Text | Google Scholar
Mehes-Smith, M., Nkongolo, K., and Cholewa, E. (2013). “Coping mechanisms of plants to metal contaminated soil,” in Environmental Change and Sustainability, ed S. Steven (InTech-Open). doi: 10.5772/55124
Mejáre, M., and Bülow, L. (2001). Metal-binding proteins and peptides in bioremediation and phytoremediation of heavy metals. Trends Biotechnol. 19, 67–73. doi: 10.1016/S0167-7799(00)01534-1
PubMed Abstract | Full Text | CrossRef Full Text | Google Scholar
Mench, M., Schwitzguebel, J. P., Schroeder, P., Bert, V., Gawronski, S., and Gupta, S. (2009). Assessment of successful experiments and limitations of phytotechnologies: contaminant uptake, detoxification and sequestration, and consequences for food safety. Environ. Sci. Pollut. Res. 16, 876–900. doi: 10.1007/s11356-009-0252-z
PubMed Abstract | Full Text | CrossRef Full Text | Google Scholar
Mendoza-Cózatl, D. G., Butko, E., Springer, F., Torpey, J. W., Komives, E. A., Kehr, J., et al. (2008). Identification of high levels of phytochelatins, glutathione and cadmium in the phloem sap of Brassica napus. A role for thiol-peptides in the long-distance transport of cadmium and the effect of cadmium on iron translocation. Plant J. 54, 249–259. doi: 10.1111/j.1365-313X.2008.03410.x
Mera, R., Torres, E., and Abalde, J. (2014). Sulphate, more than a nutrient, protects the microalga Chlamydomonas moewusii from cadmium toxicity. Aquat. Toxicol. 148, 92–103. doi: 10.1016/j.aquatox.2013.12.034
PubMed Abstract | Full Text | CrossRef Full Text | Google Scholar
Merrifield, M. E., Chaseley, J., Kille, P., and Stillman, M. J. (2006). Determination of the Cd/S cluster stoichiometry in Fucus vesiculosus metallothionein. Chem. Res. Toxicol. 19, 365–375. doi: 10.1021/tx050206j
PubMed Abstract | Full Text | CrossRef Full Text | Google Scholar
Minocha, R., Thangavel, P., Dhankher, O. P., and Long, S. (2008). Separation and quantification of monothiols and phytochelatins from a wide variety of cell cultures and tissues of trees and other plants using high performance liquid chromatography. J. Chromatogr. A 1207, 72–83. doi: 10.1016/j.chroma.2008.08.023
PubMed Abstract | Full Text | CrossRef Full Text | Google Scholar
Miyasaka, S. C., George Buta, J., Howell, R. K., and Foy, C. D. (1991). Mechanism of aluminum tolerance in snapbeans: root exudation of citric acid. Plant Physiol. 96, 737–743. doi: 10.1104/pp.96.3.737
PubMed Abstract | Full Text | CrossRef Full Text | Google Scholar
Morelli, E., and Scarano, G. (2001). Synthesis and stability of phytochelatins induced by cadmium and lead in the marine diatom Phaeodactylum tricornutum. Mar. Environ. Res. 52, 383–395. doi: 10.1016/S0141-1136(01)00093-9
PubMed Abstract | Full Text | CrossRef Full Text | Google Scholar
Mori, S., Higuchi, K., Suzuki, K., Nishizawa, N., and Nakanishi, H. (2007). Nicitianamine Synthase and Gene Encoding the Same. United States Patent No. 7,192,755B1.
Murphy, A., and Taiz, T. (1995). Comparison of metallothionein gene expression and nonprotein thiols in ten Arabidopsis ecotypes. Plant Physiol. 109, 945–954 doi: 10.1104/pp.109.3.945
PubMed Abstract | Full Text | CrossRef Full Text | Google Scholar
Nahar, K., Hasanuzzaman, M., Alam, M. M., and Fujita, M. (2015). Exogenous glutathione confers high temperature stress tolerance in mung bean (Vigna radiata L.) by modulating antioxidant defense and methylglyoxal detoxification system. Environ. Exp. Bot. 112, 44–54. doi: 10.1016/j.envexpbot.2014.12.001
Nasibi, F., Heidari, T., Asrar, Z., and Mansoori, H. (2013). Effect of arginine pre-treatment on nickel accumulation and alleviation of the oxidative stress in Hyoscyamus niger. J. Soil Sci. Plant Nutr. 13, 680–689. doi: 10.4067/S0718-95162013005000054
Nath, S., Panda, P., Mishra, S., Dey, M., Choudhury, S., Sahoo, L., et al. (2014). Arsenic stress in rice: redox consequences and regulation by iron. Plant Physiol. Biochem. 80, 203–210. doi: 10.1016/j.plaphy.2014.04.013
PubMed Abstract | Full Text | CrossRef Full Text | Google Scholar
Nishikawa, K., Onodera, A., and Tominaga, N. (2006). Phytochelatins do not correlate with the level of Cd accumulation in Chlamydomonas spp. Chemosphere 63, 1553–1559. doi: 10.1016/j.chemosphere.2005.09.056
PubMed Abstract | Full Text | CrossRef Full Text | Google Scholar
Ortega-Villasante, C., Rellán-Álvarez, R., del Campo, F. F., Carpena-Ruiz, R. O., and Hernández, L. E. (2005). Cellular damage induced by cadmium and mercury in Medicago sativa. J. Exp. Bot. 56, 2239–2251. doi: 10.1093/jxb/eri223
PubMed Abstract | Full Text | CrossRef Full Text | Google Scholar
Ovečka, M., and Takáč, T. (2014). Managing heavy metal toxicity stress in plants: biological and biotechnological tools. Biotechnol. Adv. 32, 73–86. doi: 10.1016/j.biotechadv.2013.11.011
PubMed Abstract | Full Text | CrossRef Full Text | Google Scholar
Oven, M., Grill, E., Golan-Goldhirsh, A., Kutchan, T. M., and Zenk, M. H. (2002). Increase of free cysteine and citric acid in plant cells exposed to cobalt ions. Phytochemistry 60, 467–474. doi: 10.1016/S0031-9422(02)00135-8
PubMed Abstract | Full Text | CrossRef Full Text | Google Scholar
Padmavathiamma, P. K., and Li, L. Y. (2007). Phytoremediation technology: hyper-accumulation metals in plants. Water Air Soil Pollut. 184, 105–126. doi: 10.1007/s11270-007-9401-5
Paleg, L. G., Douglas, T. J., Van Daal, A., and Keech, D. B. (1981). Proline and betaine protect enzymes against heat inactivation. Aust. J. Plant Physiol. 9, 47–57.
Park, J., Song, W. Y., Ko, D., Eom, Y., Hansen, T. H., Schiller, M., et al. (2012). The phytochelatin transporters AtABCC1 and AtABCC2 mediate tolerance to cadmium and mercury. Plant J. 69, 278–288. doi: 10.1111/j.1365-313X.2011.04789.x
PubMed Abstract | Full Text | CrossRef Full Text | Google Scholar
Pawlik-Skowrónska, B. (2002). Correlations between toxic Pb effects and production of Pb-induced thiol peptides in the microalga Stichococcus bacillaris. Environ. Pollut. 119, 119–127. doi: 10.1016/S0269-7491(01)00280-9
PubMed Abstract | Full Text | CrossRef Full Text | Google Scholar
Pawlik-Skowrónska, B. (2003). When adapted to high Zn concentrations the periphytic greenalga Stigeoclonium tenue produces high amounts of novel phytochelatin-related peptides. Aquat. Toxicol. 62, 155–163. doi: 10.1016/S0166-445X(02)00080-2
PubMed Abstract | Full Text | CrossRef Full Text | Google Scholar
Petraglia, A., De Benedictis, M., Degola, F., Pastore, G., Calcagno, M., Ruotolo, R., et al. (2014). The capability to synthesize phytochelatins and the presence of constitutive and functional phytochelatin synthases are ancestral (plesiomorphic) characters for basal land plants. J. Exp. Bot. 65, 1153–1163. doi: 10.1093/jxb/ert472
PubMed Abstract | Full Text | CrossRef Full Text | Google Scholar
Pianelli, K., Mari, S., Marquès, L., Lebrun, M., and Czernic, P. (2005). Nicotianamine over-accumulation confers resistance to nickel in Arabidopsis thaliana. Transgenic Res. 14, 739–748. doi: 10.1007/s11248-005-7159-3
PubMed Abstract | Full Text | CrossRef Full Text | Google Scholar
Piñeros, M. A., Magalhaes, J. V., Carvalho Alves, V. M., and Kochian, L. V. (2002). The physiology and biophysics of an aluminum tolerance mechanism based on root citrate exudation in maize. Plant Physiol. 129, 1194–1206. doi: 10.1104/pp.002295
PubMed Abstract | Full Text | CrossRef Full Text | Google Scholar
Piñeros, M. A., Shaff, J. E., Manslank, H. S., Carvalho, A. V. M., and Kochian, L. (2005). Aluminum resistant in maize cannot be solely explained by root organic acid exudation. A comparative physiological study. Plant Physiol. 137, 231–241. doi: 10.1104/pp.104.047357
PubMed Abstract | Full Text | CrossRef Full Text | Google Scholar
Pokora, W., Baścik-Remisiewicz, A., Tukaj, S., Kalinowska, R., Pawlik-Skowrońska, B., Dziadziuszko, M., et al. (2014). Adaptation strategies of two closely related Desmodesmus armatus (green alga) strains contained different amounts of cadmium: a study with light-induced synchronized cultures of algae. J. Plant Physiol. 171, 69–77. doi: 10.1016/j.jplph.2013.10.006
PubMed Abstract | Full Text | CrossRef Full Text | Google Scholar
Popova, T. N., and Pinheiro de Carvalho, M. A. A. (1998). Citrate and isocitrate in plant abolism. Biochim. Biophys. Acta 1364, 307–325. doi: 10.1016/S0005-2728(98)00008-5
PubMed Abstract | Full Text | CrossRef Full Text | Google Scholar
Rascio, N., and Navari-Izzo, F. (2011). Heavy metal hyperaccumulating plants: how and why do they do it? And what makes them so interesting? Plant Sci. 180, 169–181. doi: 10.1016/j.plantsci.2010.08.016
PubMed Abstract | Full Text | CrossRef Full Text | Google Scholar
Rellán-Álvarez, R., Abadía, J., and Álvarez-Fernández, A. (2008). Formation of metal-nicotianamine complexes as affected by pH, ligand exchange with citrate and metal exchange. A study by electrospray ionization time-of-flight mass spectrometry. Rapid Commun. Mass Spectrom. 22, 1553–1562. doi: 10.1002/rcm.3523
PubMed Abstract | Full Text | CrossRef Full Text | Google Scholar
Rellán-Álvarez, R., Ortega-Villasante, C., Aìlvarez-Hernaìndez, A., Del Campo, F. F., and Hernaìndez, L. E. (2006). Stress responses of Zea mays to cadmium and mercury. Plant Soil 279, 41–50. doi: 10.1007/s11104-005-3900-1
Richau, K. H., Kozhevnikova, A. D., Seregin, I. V., Vooijs, R., Koevoets, P. L. M., Smith, J. A. C., et al. (2009). Chelation by histidine inhibits the vacuolar sequestration of nickel in roots of the hyperaccumulator Thlaspi caerulescens. New Phytol. 183, 106–116. doi: 10.1111/j.1469-8137.2009.02826.x
PubMed Abstract | Full Text | CrossRef Full Text | Google Scholar
Richau, K. H., and Schat, H. (2009). Intraspecific variation of nickeland zinc accumulation and tolerance in the hyperaccumulatoro Thlaspi caerulescens. Plant Soil 314, 253–262. doi: 10.1007/s11104-008-9724-z
Roosens, N. H., Bernard, C., Leplae, R., and Verbruggen, N. (2004). Evidence for copper homeostasis function of metallothionein (MT3) in the hyperaccumulator Thlaspi caerulescens. FEBS Lett. 577, 9–16. doi: 10.1016/j.febslet.2004.08.084
PubMed Abstract | Full Text | CrossRef Full Text | Google Scholar
Roosens, N. H., Leplae, R., Bernard, C., and Verbruggen, N. (2005). Variations in plant metallothioneins: the heavy metal hyperaccumulator Thlaspi caerulescens as a study case. Planta 222, 716–729. doi: 10.1007/s00425-005-0006-1
PubMed Abstract | Full Text | CrossRef Full Text | Google Scholar
Ruttkay-Nedecky, B., Nejdl, L., Gumulec, J., Zitka, O., Masarik, M., Eckschlager, T., et al. (2013). The role of metallothionein in oxidative stress. Int. J. Mol. Sci. 14, 6044–6066. doi: 10.3390/ijms14036044
PubMed Abstract | Full Text | CrossRef Full Text | Google Scholar
Salt, D. E., Prince, R. C., Baker, A. J. M., Raskin, I., and Pickering, I. J. (1999). Zinc legands in the metal hyperaccumulator Thalspi caerulescens as determined using X-absorption spectroscopy. Environ. Sci. Technol. 33, 713–717. doi: 10.1021/es980825x
Sasaki, T., Yamamoto, Y., Ezaki, B., Katsuhara, M., Ahn, S. J., Ryan, P. R., et al. (2004). A wheat gene encoding an aluminum-activated malate transporter. Plant J. 37, 645–653. doi: 10.1111/j.1365-313X.2003.01991.x
PubMed Abstract | Full Text | CrossRef Full Text | Google Scholar
Schützendübel, A., and Polle, A. (2002). Plant responses to abiotic stresses: heavy metal induced oxidative stress and protection by mycorrhization. J. Exp. Bot. 53, 1351–1365. doi: 10.1093/jexbot/53.372.1351
PubMed Abstract | Full Text | CrossRef Full Text | Google Scholar
Sekhar, K., Priyanka, B., Reddy, V. D., and Rao, K. V. (2011). Metallothionein 1 (CcMT1) of pigeonpea (Cajanus cajan L.) confers enhanced tolerance to copper and cadmium in Escherichia coli and Arabidopsis thaliana. Environ. Exp. Bot. 72, 131–139. doi: 10.1016/j.envexpbot.2011.02.017
Seth, C., Remans, T., Keunen, E., Jozefczak, M., Gielen, H., Opdenakker, K., et al. (2012). Phytoextraction of toxic metals: a central role for glutathione. Plant Cell Environ. 35, 334–346. doi: 10.1111/j.1365-3040.2011.02338.x
PubMed Abstract | Full Text | CrossRef Full Text | Google Scholar
Sharma, S. S., and Dietz, K. J. (2006). The significance of amino acids and amino acid-derived molecules in plant responses and adaptation to heavy metal stress. J. Exp. Bot. 57, 711–726. doi: 10.1093/jxb/erj073
PubMed Abstract | Full Text | CrossRef Full Text | Google Scholar
Sharma, S. S., Schat, H., and Vooijs, R. (1998). In vitro alleviation of heavy metal-induced enzyme inhibition by proline. Phytochemistry 49, 1531–1535. doi: 10.1016/S0031-9422(98)00282-9
PubMed Abstract | Full Text | CrossRef Full Text | Google Scholar
Shevyakova, N. I., Il'ina, E. N., Stetsenko, L. A., and Kuznetsov, V. V. (2011). Nickel accumulation in rape shoots (Brassica napus L.) increased by putrescine. Int. J. Phytoremed. 13, 345–356. doi: 10.1080/15226514.2010.495147
PubMed Abstract | Full Text | CrossRef Full Text | Google Scholar
Shukla, D., Kesari, R., Mishra, S., Dwivedi, S., Tripathi, R. D., Nath, P., et al. (2012). Expression of phytochelatin synthase from aquatic macrophyte Ceratophyllum demersum L. enhances cadmium and arsenic accumulation in tobacco. Plant Cell Rep. 31, 1687–1699. doi: 10.1007/s00299-012-1283-3
PubMed Abstract | Full Text | CrossRef Full Text | Google Scholar
Shukla, D., Kesari, R., Tiwari, M., Dwivedi, S., Tripathi, R. D., Nath, P., et al. (2013). Expression of Ceratophyllum demersum phytochelatin synthase, CdPCS1, in Escherichia coli and Arabidopsis enhances heavy metal(loid)s accumulation. Protoplasma 250, 1263–1272. doi: 10.1007/s00709-013-0508-9
PubMed Abstract | Full Text | CrossRef Full Text | Google Scholar
Simões, C. C., Melo, J. O., Magalhaes, J. V., and Guimarães, C. T. (2012). Genetic and molecular mechanisms of aluminum tolerance in plants. Genet. Mol. Res. 11, 1949–1957. doi: 10.4238/2012.July.19.14
PubMed Abstract | Full Text | CrossRef Full Text | Google Scholar
Singh, D., and Chauhan, S. K. (2011). Organic acids of crop plants in aluminium detoxification. Curr. Sci. 100, 1109–1515.
Singh, R. K., Anandhan, S., Singh, S., Patade, V. Y., Ahmed, Z., and Pande, V. (2011). Metallothionein-like gene from Cicer microphyllum is regulated by multiple abiotic stresses. Protoplasma 248, 839–847. doi: 10.1007/s00709-010-0249-y
PubMed Abstract | Full Text | CrossRef Full Text | Google Scholar
Sobrino-Plata, J., Ortega-Villasante, C., Flores-Cáceres, M. L., Escobar, C., Del Campo, F. F., and Hernández, L. E. (2009). Differential alterations of antioxidant defenses as bioindicators of mercury and cadmium toxicity in alfalfa. Chemosphere 77, 946–954. doi: 10.1016/j.chemosphere.2009.08.007
PubMed Abstract | Full Text | CrossRef Full Text | Google Scholar
Solanki, R., and Dhankhar, R. (2011). Biochemical changes and adaptive strategies of plants under heavy metal stress. Biologia 66, 195–204. doi: 10.2478/s11756-011-0005-6
Solt, J. P., Sneller, F. E. C., Bryngelsson, T., Lundborg, T., and Schat, H. (2003). Phytochelatin and cadmium accumulation in wheat. Environ. Exp. Bot. 49, 21–28. doi: 10.1016/S0098-8472(02)00045-X
Song, H. M., Wang, H. Z., and Xu, X. B. (2012). Overexpression of AtHsp90.3 in Arabidopsis thaliana impairs plant tolerance to heavy metal stress. Biol. Plant 56, 197–199. doi: 10.1007/s10535-012-0042-1
Song, W. Y., Mendoza-Cózatl, D. G., Lee, Y., Schroeder, J. I., Ahn, S. N., Lee, H. S., et al. (2014). Phytochelatin-metal(loid) transport into vacuoles shows different substrate preferences in barley and Arabidopsis. Plant Cell Environ. 37, 1192–1201. doi: 10.1111/pce.12227
PubMed Abstract | Full Text | CrossRef Full Text | Google Scholar
Spisso, A. A., Cerutti, S., Silva, F., Pacheco, P. H., and Martinez, L. D. (2014). Characterization of Hg-phytochelatins complexes in vines (Vitis vinifera cv. Malbec) as defense mechanism aginst metal stress. Biometals 27, 591–599. doi: 10.1007/s10534-014-9732-9
PubMed Abstract | Full Text | CrossRef Full Text | Google Scholar
Srivalli, S., and Khanna-Chopra, R. (2008). Delayed wheat flag leaf senescence due to the removal of spikelets is associated with increased activities of leaf antioxidant enzymes, reduced glutathione/oxidized glutathione ratio and oxidative damage to mitochondrial proteins. Plant Physiol. Biochem. 47, 663–670. doi: 10.1016/j.plaphy.2009.03.015
PubMed Abstract | Full Text | CrossRef Full Text | Google Scholar
Takahashi, M., Terada, Y., Nakai, I., Nakanishi, H., Yoshimura, E., Mori, S., et al. (2003). Role of nicotianamine in the intracellular delivery of metals and plant reproductive development. Plant Cell 15, 1263–1280. doi: 10.1105/tpc.010256
PubMed Abstract | Full Text | CrossRef Full Text | Google Scholar
Tariq Javed, M., Lindberg, S., and Greger, M. (2014). Cellular proton dynamics in Elodea canadensis leaves induced by cadmium. Plant Physiol. Biochem. 77, 15–22. doi: 10.1016/j.plaphy.2014.01.009
PubMed Abstract | Full Text | CrossRef Full Text | Google Scholar
Tennstedt, P., Peisker, D., Bottcher, C., Trampczynska, A., and Clemens, S. (2009). Phytochelatin synthesis is essential for the detoxification of excess zinc and contributes significantly to the accumulation of zinc. Plant Physiol. 149, 938–948. doi: 10.1104/pp.108.127472
PubMed Abstract | Full Text | CrossRef Full Text | Google Scholar
Thangavel, P., Long, S., and Minocha, R. (2007). Changes in phytochelatins and their biosynthetic intermediates in red spruce (Picea rubens Sarg.) cell suspension cultures under cadmium and zinc stress. Plant Cell Tissue Organ Cult. 88, 201–216. doi: 10.1007/s11240-006-9192-1
Theriappan, P., Gupta, A. K., and Dhasarathan, P. (2011). Accumulation of proline under salinity and heavy metal stress in cauliflower seedlings. J. Appl. Sci. Environ. Manag. 15, 251–255. doi: 10.4314/jasem.v15i2.68497
Trejo-Tellez, L. I., Gomez-Merino, F. C., and Schmitt, J. M. (2012). “Citric acid: biosynthesis, properties and applications on higher plants,” in Citric Acid, eds D. A. Vargas and J. V. Medina (New York, NY: Nova Science Publishers, Inc.), 43–70.
Ueno, D., Iwashita, T., Zhao, F. J., and Ma, J. F. (2008). Characterization of Cd translocation and identification of the Cd form in xylem sap of the Cd-hyperaccumulator Arabidopsis halleri. Plant Cell Physiol. 49, 540–548. doi: 10.1093/pcp/pcn026
PubMed Abstract | Full Text | CrossRef Full Text | Google Scholar
Usha, B., Venkataraman, G., and Parida, A. (2009). Heavy metal and abiotic stress inducible metallothionein isoform from Populus juliflora (SW) D.C. show differences in binding to heavy metals in vitro. Mol. Genet. Genomics 281, 99–108. doi: 10.1007/s00438-008-0398-2
PubMed Abstract | Full Text | CrossRef Full Text | Google Scholar
Vacchina, V., Mari, S., Czernic, P., Marquès, L., Pianelli, K., Schaumlöffel, D., et al. (2003). Speciation of nickel in a hyperaccumulating plant by high-performance liquid chromatography-inductively coupled plasma mass spectrometry and electrospray MS/MS assisted by cloning using yeast complementation. Anal. Chem. 75, 2740–2745. doi: 10.1021/ac020704m
PubMed Abstract | Full Text | CrossRef Full Text | Google Scholar
Valero, D., Pérez-Vicente, A., Martínez-Romero, D., Castillo, S., Guillén, F., and Serrano, M. (2002). Plum storability improved after calcium and heat postharvest treatments: role of polyamines. J. Food Sci. 67, 2571–2575. doi: 10.1111/j.1365-2621.2002.tb08778.x
van de Mortel, J. E., Almar Villanueva, L., Schat, H., Kwekkeboom, J., Coughlan, S., Moerland, P. D., et al. (2006). Large expression differences in genes for iron and zinc homeostasis, stress response, and lignin biosynthesis distinguish roots of Arabidopsis thaliana and the related metal hyperaccumulator Thlaspi caerulescens. Plant Physiol. 142, 1127–1147. doi: 10.1104/pp.106.082073
PubMed Abstract | Full Text | CrossRef Full Text | Google Scholar
Vangronsveld, J., Herzig, R., Weyens, N., Boulet, J., Adriaensen, K., Ruttens, A., et al. (2009). Phytoremediation of contaminated soils and groundwater: lessons from the field. Environ. Sci. Pollut. Res. 16, 765–794. doi: 10.1007/s11356-009-0213-6
PubMed Abstract | Full Text | CrossRef Full Text | Google Scholar
Vatamaniuk, O. K., Bucher, E. A., Ward, J. T., and Rea, P. A. (2001). A new pathway for heavy metal detoxification in animals. Phytochelatin synthase is required for cadmium tolerance in Caenorhabditis elegans. J. Biol. Chem. 276, 20817–20820. doi: 10.1074/jbc.C100152200
PubMed Abstract | Full Text | CrossRef Full Text | Google Scholar
Vatamaniuk, O. K., Mari, S., Lang, A., Chalasani, S., Demkiv, L. O., and Rea, P. A. (2004). Phytochelatin synthase, a dipeptidyltransferase that undergoes multisite acylation with γ-glutamylcysteine during catalysis. Stoichiometric and site-directed mutagenic analysis of Arabidopsis thaliana PCS1-catalyzed phytochelatin synthesis. J. Biol. Chem. 279, 22449–22460. doi: 10.1074/jbc.M313142200
PubMed Abstract | Full Text | CrossRef Full Text | Google Scholar
Verbruggen, N., Hermans, C., and Schat, H. (2009). Molecular mechanisms of metal hyperaccumulation in plants. New Phytol. 181, 759–776. doi: 10.1111/j.1469-8137.2008.02748.x
PubMed Abstract | Full Text | CrossRef Full Text | Google Scholar
Verma, D. P. S., and Zhang, C. S. (1999). “Regulation of proline and arginine biosynthesis inn plants,” in Plant Amno Acids: Biochemistry and Biotechnology, ed B. K. Singh (New York, NY: Marcel Dekker), 249–266.
Volland, S., Bayer, E., Baumgartner, V., Andosch, A., Lütz, C., Sima, E., et al. (2014). Rescue of heavy metal effects on cell physiology of the algal model system Micrasterias by divalent ions. J. Plant Physiol. 171, 154–163. doi: 10.1016/j.jplph.2013.10.002
PubMed Abstract | Full Text | CrossRef Full Text | Google Scholar
Wang, F., Wang, Z., and Zhu, C. (2012). Heteroexpression of the wheat phytochelatin synthase gene (TaPCS1) in rice enhances cadmium sensitivity. Acta Biochim. Biophys. Sin. 44, 886–893. doi: 10.1093/abbs/gms073
PubMed Abstract | Full Text | CrossRef Full Text | Google Scholar
Wang, H. C., Wu, J. S., Chia, J. C., Yang, C. C., Wu, Y. J., and Juang, R. H. (2009). Phytochelatin synthase is regulated by protein phosphorylation at a threonine residue near its catalytic site. J. Agric. Food Chem. 57, 7348–7355. doi: 10.1021/jf9020152
PubMed Abstract | Full Text | CrossRef Full Text | Google Scholar
Wang, X., Shi, G., Xu, Q., and Hu, J. (2007). Exogenous polyamines enhance copper tolerance of Nymphoides peltatum. J. Plant Physiol. 164, 1062–1070. doi: 10.1016/j.jplph.2006.06.003
PubMed Abstract | Full Text | CrossRef Full Text | Google Scholar
Wen, X. P., Pang, X. M., Matsuda, N., Kita, M., Inoue, H., Hao, Y. J., et al. (2008). Over-expression of the apple spermidine synthase gene in pear confers multiple abiotic stress tolerance by altering polyamine titers. Transgenic Res. 17, 251–263. doi: 10.1007/s11248-007-9098-7
PubMed Abstract | Full Text | CrossRef Full Text | Google Scholar
Wycisk, K., Kim, E. J., Schroeder, J. I., and Krämer, U. (2004). Enhancing the first enzymatic step in the histidine biosynthesis pathway increases the free histidine pool and nickel tolerance in Arabidopsis thaliana. FEBS Lett. 578, 128–134. doi: 10.1016/j.febslet.2004.10.086
PubMed Abstract | Full Text | CrossRef Full Text | Google Scholar
Yang, H., Huang, Z. Y., Li, J., and Hu, Y. (2014). MT-like proteins: potential bio-indicators of Chlorella vulgaris for zinc contamination in water environment. Ecol. Ind. 45, 103–109. doi: 10.1016/j.ecolind.2014.03.017
Yang, L. T., Qi, Y. P., Jiang, H. X., and Chen, L. S. (2013). Roles of organic acid anion secretation in aluminum tolerance of higher plants. BioMed. Res. Int. 2013:173682. doi: 10.1155/2013/173682
PubMed Abstract | Full Text | CrossRef Full Text | Google Scholar
Yokosho, K., Yamaji, N., and Ma, J. F. (2010). Isolation and characterization of two MATE genes in rye. Funct. Plant Biol. 37, 296–303. doi: 10.1071/FP09265
PubMed Abstract | Full Text | CrossRef Full Text | Google Scholar
Yokosho, K., Yamaji, N., and Ma, J. F. (2011). An Al-inducible MATE gene is involved in external detoxification of Al in rice. Plant J. 68, 1061–1069. doi: 10.1111/j.1365-313X.2011.04757.x
PubMed Abstract | Full Text | CrossRef Full Text | Google Scholar
Zacchini, M., Pietrini, F., Mugnozza, G. S., Iori, V., Pietrosanti, L., and Massacci, A. (2009). Metal tolerance, accumulation and translocation in poplar and willow clones treated with cadmium in hydroponics. Water Air Soil Pollut. 197, 23–34. doi: 10.1007/s11270-008-9788-7
Zenk, M. H. (1996). Heavy metal detoxification in higher plants–a review. Gene 179, 21–30. doi: 10.1016/S0378-1119(96)00422-2
PubMed Abstract | Full Text | CrossRef Full Text | Google Scholar
Zhang, H., Xu, W., Dai, W., He, Z., and Ma, M. (2006). Functional characterization of cadmium-responsive garlic gene AsMT2b: a new member of metallothionein family. Chin. Sci. Bull. 51, 409–416. doi: 10.1007/s11434-006-0409-9
Zhang, Z., Gao, X., and Qiu, B. (2008). Detection of phytochelatins in the hyperaccumulator Sedum alfredii exposed to cadmium and lead. Phytochemistry 69, 911–918. doi: 10.1016/j.phytochem.2007.10.012
PubMed Abstract | Full Text | CrossRef Full Text | Google Scholar
Zhao, H., and Yang, H. (2008). Exogenous polyamines alleviate the lipid peroxidation induced by cadmium chloride stress in Malus hupehensis Rehd. Sci. Hortic. 116, 442–447. doi: 10.1016/j.scienta.2008.02.017
Zhao, J., Shi, G. X., and Yuan, Q. H. (2008). Polyamines content and physiological and biochemical responses to ladder concentration of nickel stress in Hydrocharis dubia (Bl.) Backer leaves. Biometals 21, 665–674. doi: 10.1007/s10534-008-9151-x
PubMed Abstract | Full Text | CrossRef Full Text | Google Scholar
Zhou, B., Yao, W., Wang, S., Wang, Y., and Jiang, T. (2014). The metallothionein gene, TaMT3, from Tamarix androssowii confers Cd2+ tolerance in tobacco. Int. J. Mol. Sci. 15, 10398–10409. doi: 10.3390/ijms150610398
PubMed Abstract | Full Text | CrossRef Full Text | Google Scholar
Zhou, J., and Goldsbrough, P. B. (1994). Functional homologs of fungal metallothionein genes from Arabidopsis. Plant Cell 6, 875–884. doi: 10.1105/tpc.6.6.875
PubMed Abstract | Full Text | CrossRef Full Text | Google Scholar
Zhu, X. F., Zheng, C., Hu, Y. T., Jiang, T., Liu, Y., Dong, N. Y., et al. (2011). Cadmium-induced oxalate secretion from root apex is associated with cadmium exclusion and resistance in Lycopersicon esulentum. Plant Cell Environ. 34, 1055–1064. doi: 10.1111/j.1365-3040.2011.02304.x
PubMed Abstract | Full Text | CrossRef Full Text | Google Scholar
Zimeri, A. M., Dhankher, O. P., McCaig, B., and Meagher, R. B. (2005). The plant MT1 metallothioneins are stabilized by binding cadmiums and are required for cadmium tolerance and accumulation. Plant Mol. Biol. 58, 839–855. doi: 10.1007/s11103-005-8268-3
PubMed Abstract | Full Text | CrossRef Full Text | Google Scholar
Keywords: metal/metalloids, plant tolerance, chelation, thiol compounds, glutathione, organic acid, metallothioneins, phytochelatins
Citation: Anjum NA, Hasanuzzaman M, Hossain MA, Thangavel P, Roychoudhury A, Gill SS, Rodrigo MAM, Adam V, Fujita M, Kizek R, Duarte AC, Pereira E and Ahmad I (2015) Jacks of metal/metalloid chelation trade in plants—an overview. Front. Plant Sci. 6:192. doi: 10.3389/fpls.2015.00192
Received: 30 October 2014; Paper pending published: 13 January 2015;
Accepted: 10 March 2015; Published: 02 April 2015.
Edited by:
Richard Sayre, New Mexico Consortium at Los Alamos National Labs, USAReviewed by:
Vasileios Fotopoulos, Cyprus University of Technology, CyprusHannetz Roschzttardtz, University of Wisconsin-Madison, USA
Copyright © 2015 Anjum, Hasanuzzaman, Hossain, Thangavel, Roychoudhury, Gill, Rodrigo, Adam, Fujita, Kizek, Duarte, Pereira and Ahmad. This is an open-access article distributed under the terms of the Creative Commons Attribution License (CC BY). The use, distribution or reproduction in other forums is permitted, provided the original author(s) or licensor are credited and that the original publication in this journal is cited, in accordance with accepted academic practice. No use, distribution or reproduction is permitted which does not comply with these terms.
*Correspondence: Mirza Hasanuzzaman, Department of Agronomy, Faculty of Agriculture, Sher-e-Bangla Agricultural University, Dhaka 1207, BangladeshbWh6c2F1YWdAeWFob28uY29t