- 1Department of Resource and Environment, College of Resource and Environmental Science, Fujian Agriculture and Forestry University, Fuzhou, China
- 2Institute of Horticultural Plant Physiology, Biochemistry, and Molecular Biology, Fujian Agriculture and Forestry University, Fuzhou, China
- 3Institute of Materia Medica, Fujian Academy of Medical Sciences, Fuzhou, China
- 4Department of Horticultural Science, Kyungpook National University, Daegu, South Korea
- 5The Higher Educational Key Laboratory of Fujian Province for Soil Ecosystem Health and Regulation, Fujian Agriculture and Forestry University, Fuzhou, China
Seedlings of Citrus sinensis (L.) Osbeck were supplied with boron (B)-deficient (without H3BO3) or -sufficient (10 μM H3BO3) nutrient solution for 15 weeks. We identified 54 (38) and 38 (45) up (down)-regulated cDNA-AFLP bands (transcript-derived fragments, TDFs) from B-deficient leaves and roots, respectively. These TDFs were mainly involved in protein and amino acid metabolism, carbohydrate and energy metabolism, nucleic acid metabolism, cell transport, signal transduction, and stress response and defense. The majority of the differentially expressed TDFs were isolated only from B-deficient roots or leaves, only seven TDFs with the same GenBank ID were isolated from the both. In addition, ATP biosynthesis-related TDFs were induced in B-deficient roots, but unaffected in B-deficient leaves. Most of the differentially expressed TDFs associated with signal transduction and stress defense were down-regulated in roots, but up-regulated in leaves. TDFs related to protein ubiquitination and proteolysis were induced in B-deficient leaves except for one TDF, while only two down-regulated TDFs associated with ubiquitination were detected in B-deficient roots. Thus, many differences existed in long-term B-deficiency-responsive genes between roots and leaves. In conclusion, our findings provided a global picture of the differential responses occurring in B-deficient roots and leaves and revealed new insight into the different adaptive mechanisms of C. sinensis roots and leaves to B-deficiency at the transcriptional level.
Introduction
Boron (B), as an essential micronutrient required for higher plants, is absorbed from soil solution by plant roots mainly in the form of boric acid. As boric acid in soils is easily leached under high rainfall conditions, B-deficient symptoms are often observed in many important agricultural crops, including citrus (Chen et al., 2012). According to investigation, a fair number of cultivated soils in southern and eastern China had very low concentration of hot water soluble B (less than 0.25 mg kg−1 DW) (Liu et al., 1989). Up to 45.5 and 9.0% of “Guanximiyou” pummelo (Citrus grandis) orchards in Pinghe, Zhangzhou, China were deficient in soil water-soluble B and leaf B, respectively (Huang et al., 2001).
In plants, B-deficiency affects many physiological and biochemical processes, such as gas exchange, nucleic acid metabolism, protein and amino acid (AA) biosynthesis, carbohydrate transport and accumulation, organic acid (OA) metabolism, cell division and elongation, cell wall structure, vascular development, phenolic metabolism, biosynthesis and transport of plant hormones, membrane integrity and oxidoreductase activity (Kouchi and Kumazawa, 1975; Tang and Dela Fuente, 1986; Han et al., 2008, 2009; Camacho-Cristóbal et al., 2008a; Chen et al., 2012; Hajiboland et al., 2012; Lu et al., 2014a).
B-deficiency causes remarkable alterations in the expression profiles of genes for various processes during plant growth and development, including cell wall modification (Camacho-Cristóbal et al., 2008b; Redondo-Nieto et al., 2012; Zhou et al., 2015), B uptake and translocation (Camacho-Cristóbal et al., 2008a), cell transport (Camacho-Cristóbal and González-Fontes, 2007; Redondo-Nieto et al., 2012; Zhou et al., 2015), vascular development (Yang et al., 2013a), stress response and defense (Redondo-Nieto et al., 2012; Zhou et al., 2015), protein and AA metabolism (Beato et al., 2010; Zhou et al., 2015), transcription, DNA metabolism, cell cycle, and signal transduction (Redondo-Nieto et al., 2012) in tobacco roots, leaves, and cells, citrus roots, and leaf veins, Medicago truncatula root nodules, Arabidopsis roots, shoots, and seedlings. Most research, however, has focused on roots (Camacho-Cristóbal et al., 2011), because the inhibition of root growth is one of the most rapid responses of plants to B-deficiency (Bohnsack and Albert, 1977). Less is known about the effects of B-deficiency on leaf transcriptomics. Also, limited data are available on B-deficiency-responsive genes in woody plants.
There are several comparative studies showing that the effects of B-deficiency on gas exchange, carbohydrates and OAs and related metabolic enzymes, nitrogen and phenolic metabolisms differ between roots and leaves (Stavrianakou et al., 2006; Camacho-Cristóbal et al., 2008a; Hajiboland et al., 2013; Lu et al., 2014a). Thus, B-deficiency-induced changes in transcriptomics should be different between roots and leaves. To our knowledge, such data are not yet available in woody plants.
In this study, we first compared B-deficiency-responsive genes in Citrus sinensis roots and leaves using cDNA-amplified fragment length polymorphism (cDNA-AFLP) in order to (a) determine the mechanisms of plants to deal with B-deficiency at the transcriptional level and (b) understand the differences in B-deficiency-induced alterations in gene expression between roots and leaves.
Materials and Methods
Plant Materials
This study was conducted at Fujian Agriculture and Forestry University, Fuzhou, China (26°5′ N, 119°14′ E). “Xuegan” (Citrus sinensis) seedlings were used in this study, because C. sinensis is polyembryonic seed development, an apomictic process in which many embryos are initiated directly from the maternal nucellar cells surrounding the embryo sac containing a developing zygotic embryo (Aleza et al., 2010).
Experimental Design
Plant culture and B-treatments were performed according to Yang et al. (2013b). In late-May (5 weeks after germination), uniform seedlings were transplanted into 6 L pots (two per pot) containing river sand and grown in a greenhouse under natural photoperiod. Ten weeks after transplanting, each pot were irrigated every other day until dripping with B-deficient (without H3BO3) or -sufficient (10 μM H3BO3) nutrient solution for 15 weeks. There were 20 pots per treatment in a completely randomized design. At the end of the experiment, fully expanded (about 7-week-old) leaves (midribs and petioles removed) and ca. 5-mm-long root apices were collected at noon under full sun from different replicates and treatments and immediately frozen in liquid N2. Both leaf and root samples were stored at −80°C until they were used for cDNA-AFLP and qRT-PCR analysis. The remaining seedlings that were not sampled were used to measure root, stem, and leaf dry weights (DWs) and root and leaf concentration of B.
Measurements of Root, Stem, and Leaf DWs, and Root and Leaf B Concentration
Ten plants per treatment from different replicates were harvested and divided into roots, stems and leaves. After being dried 70°C for 48 h, their DWs were weighted.
For the determination of B concentration, about 7-week-old leaves (midribs and petioles removed) and fibrous roots were collected and dried at 70°C for 48 h. Dried samples were ground in a mortar to pass a 40-mesh sieve, then ashed at 500°C for 5 h, finally dissolved in 0.1 M HCl. B concentration in the solution was assayed by the modified curcumin method (Kowalenko and Lavkulich, 1976). There were six replicates per treatment.
RNA Preparation, cDNA Synthesis and CDNA-AFLP Analysis
Equal amounts of frozen leaf (root) samples collected from five plants (one per pot) were mixed as a biological replicate. There were three biological replicates for each treatment. Total RNA was independently extracted three times from the frozen samples using Recalcitrant Plant Total RNA Extraction Kit (Centrifugal column type, Bioteke Corporation, China) according to manufacturer's instructions. cDNA synthesis and cDNA-AFLP analysis were performed according to Zhou et al. (2013). After the integrity and quantity of total RNA being checked, first-strand cDNA was synthesized. The resulting double-stranded cDNA was purified using equal volume of phenol: chloroform: isoamyl alcohol (25: 24: 1). Double-stranded cDNA (600 ng) was digested with restriction enzymes: 5 U each of EcoR I (Thermo Scientific, Massachusetts, USA; 3 h at 37°C) and Mse I (Tru1I, Thermo Scientific, Massachusetts, USA; 3 h at 65°C). The resulting restricted fragments were ligated to adaptors (EcoR I, 0.2 μM forward primer: 5′- CTCGTAGACTGC GTACC-3′ and reverse primer: 3′- CATCTG ACGCATGGTTAAP -5′; Mse I, 2 μM forward primer: 5′-GACGAT GAGTCCTGAG-3′ and reverse primer: 3′-TA CTCAGGACTCATP-5′) with T4-DNA ligase (Thermo Scientific, Massachusetts, USA) for 10–16 h at 16°C. The resulting ligated products were pre-amplified with the corresponding pre-amplification primers: EcoR I, 5′-GACTGCGATCCAATTC-3′ and Mse I, 5′-GATGAG TCCTGAGTAA-3′. From a 100-fold dilution of the pre-amplified samples, a 5 μL diluted sample was used for the selective amplification using 256 combinations of the following primers: 16 derivatives of EcoR I primers 5′-GACTGCGATCCAATT CEE-3′ and 16 derivatives of Mse I primers 5′-GATGAG TCCTGAGTAAMM-3′; where EE and MM represented AA, AT, AC, AG, TA, TC, TT, TG, CA, CT, CG, CC, GA, GC, GT, and GG. The selective amplification products were separated on a 6% (w/v) polyacrylamide gel run at 50 W for 2.5 h. The gels were silver stained to visualize the cDNA bands. Samples for cDNA-AFLP analysis were run in three replicates at least.
The up- or down-regulated cDNA-AFLP bands (transcript-derived fragments, TDFs) were selected based on their presence, absence, or differential intensity and cut out with a scalpel, and incubated in 50 μL of dd H2O for 30 min in a boiling water bath, then centrifuged at 10000 rpm (Eppendorf 5418R, Hamburg, Germany). The supernatant (eluted DNA) was re-amplified by PCR using the same primer combinations. The re-amplified products were checked on 1% (w/v) agarose gels, each band was isolated and eluted using DNA Agarose Gel Recovery Kit (Solarbio, China). Before being sequenced by BGI Technology Corporation (Shenzhen, China), these TDFs fragments were ligated to pGEM-T EASY vector according to usage information of pGEM®-T Easy Vector System I (Promega, USA), then transduced into Escherichia coli (DH5α) competent cells using ampicillin as the selecting agent. All sequences were input into the VecScreen (http://www.ncbi.nlm.nih.gov/VecScreen/VecScreen) to identify and remove all of the vector sequence. Homology of TDFs' sequences was analyzed using the BLASTX and BLASTN searching engines (http://www.blast.ncbi.nlm.nih.gov/Blast). Their functional categories were assigned based on the analysis of information reported for each sequence by The Gene Ontology (http://amigo.geneontology.org/cgi-bin/amigo/blast) and Uniprot (http://www.uniprot.org/).
qRT-PCR Analysis
Both sample collecting and total RNA extraction were performed as described above. qRT-PCR analysis was performed according to Zhou et al. (2013). Specific primers were designed from the sequences of 51 differentially expressed TDFs using Primer Premier Version 5.0 (PREMIER Biosoft International, CA, USA). The sequences of the F and R primers used were listed in Table S1. Samples for qRT-PCR were run in three biological replicates with two technical replicates. Relative gene expression was calculated using ddCt algorithm. For the normalization of gene, citrus actin (GU911361.1) was used as an internal standard and the sample from B-sufficient treated plants was used as reference sample, which was set to 1.
Statistical Analysis
Results represented the mean ± SD. Statistical analyses of data were carried out by unpaired t-test at P < 0.05 level.
Results
Plant Growth and B Concentration in Roots and Leaves
Seedlings treated without H3BO3 had slower growth and less leaf and root level of B than those treated with 10 μM H3BO3 (Figure S1). B concentration in leaves from seedlings treated without H3BO3 was lower than the sufficient range of 30–100 μg g−1 DW (Chapman, 1968). Also, a typical B-deficient symptom (i.e., corky split veins) was observed in leaves from seedlings treated without H3BO3 (Han et al., 2008). Thus, seedlings treated without H3BO3 are considered B-deficient, and those treated with 10 μM H3BO3 are considered B-sufficient.
Differentially Expressed Genes in B-deficient Roots and Leaves
A total of 256 selective primer combinations were used to isolate the differentially expressed TDFs from B-deficient roots and leaves. Figure S2 displayed the typical picture of a silver-stained cDNA-AFLP gel. We amplified a total of 5247 (5579) reproducible, clear, and unambiguous cDNA-AFLP bands (TDFs) from B-deficient roots (leaves), with an average of 20.5 (21.8) TDFs in roots (leaves) for each primer combination. A TDF with both a P-value of less than 0.05 and an average fold change of more than 1.5 was considered differentially expressed. Here, 131 and 165 differentially expressed and reproducible TDFs were obtained from B-deficient roots and leaves, respectively. After all these TDFs were reamplified, cloned, and sequenced, 114 and 129 TDFs from roots and leaves produced useable sequence data. All these data were blasted against the sequence data available in GenBank. Eighty-three root TDFs and 92 leaf TDFs showed significant homology to genes encoding known, putative uncharacterized, hypothetical and unknown proteins, and the remaining 31 root TDFs and 37 leaf TDFs did not share homologous with any nucleotide or AA sequence in the public databases.
Functions of the Differentially Expressed Genes in Roots and Leaves
Among the 83 matched root TDFs, 38 TDFs were up-regulated and 45 TDFs were down-regulated by B-deficiency. According to the biological properties, these TDFs were associated with carbohydrate and energy metabolism (11), nucleic acid metabolism (13), protein and AA metabolism (10), cell transport (9), signal transduction (7), stress response and defense (8), lipid metabolism (4), cell wall modification (2), and others (19) (Table 1; Figure S3). For the 92 matched leaf TDFs, 54 TDFs were increased and 38 TDFs were decreased by B-deficiency. These TDFs were classified into the following categories: carbohydrate and energy metabolism (12), nucleic acid metabolism (11), protein and AA metabolism (19), cell transport (10), signal transduction (5), stress response and defense (8), lipid metabolism (6), cell wall modification (1), and others (20) (Table 2; Figure S3). As shown in Figure S3, the majority of the differentially expressed TDFs were isolated only from B-deficient roots or leaves, only seven TDFs with the same GenBank ID were isolated from the both.
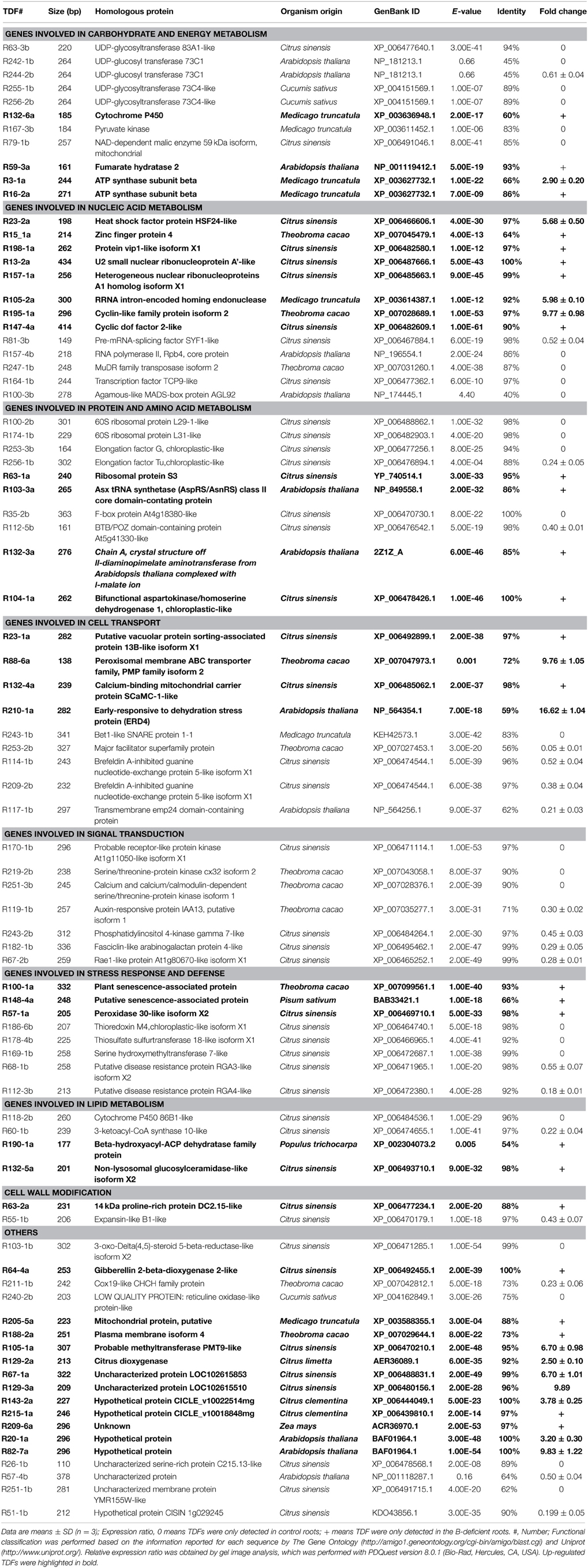
Table 1. Homologies of differentially expressed cDNA-AFLP fragments with known gene sequences in database using BLASTN algorithm along with their expression patterns in B-deficient Citrus sinensis roots.
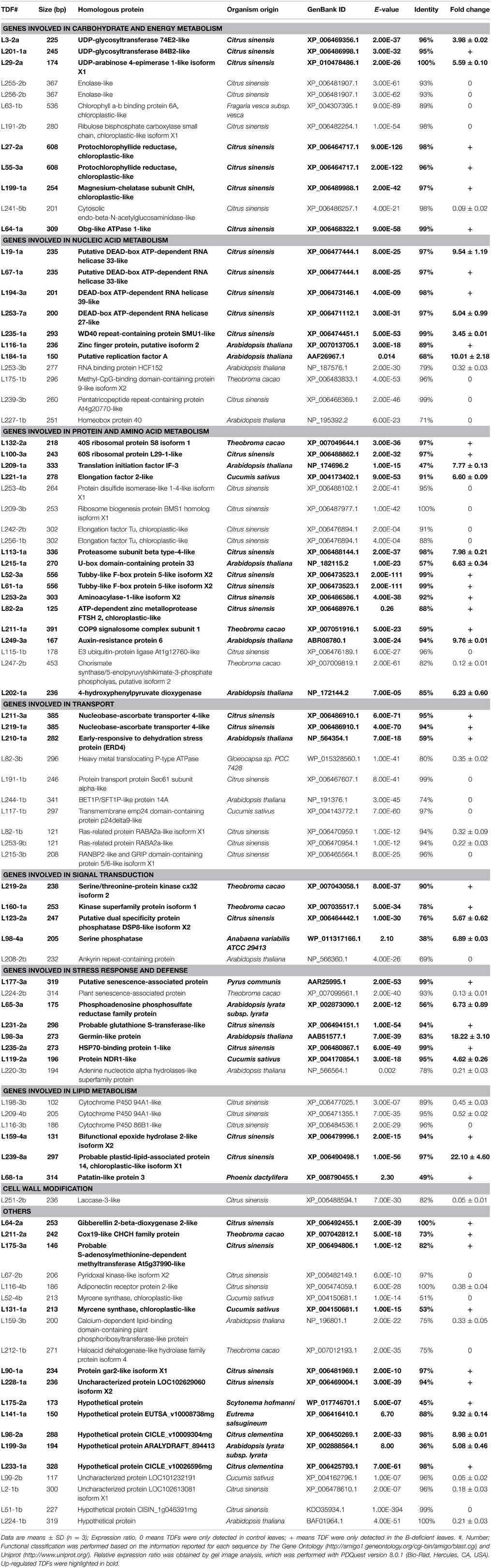
Table 2. Homologies of differentially expressed cDNA-AFLP fragments with known gene sequences in database using BLASTN algorithm along with their expression patterns in B-deficient Citrus sinensis leaves.
Validation of cDNA-AFLP Data
Twenty-five TDFs from roots and 26 TDFs from leaves were selected for qRT-PCR to check their expression patterns obtained by cDNA-AFLP. The expression profiles of all these TDFs produced by qRT-PCR well-matched with the expression patterns revealed by cDNA-AFLP except for three TDFs (i.e., TDFs #R67-2b, R190-1a and L199-1a; Figure 1). Thus, this technique was validated in 94% of cases.
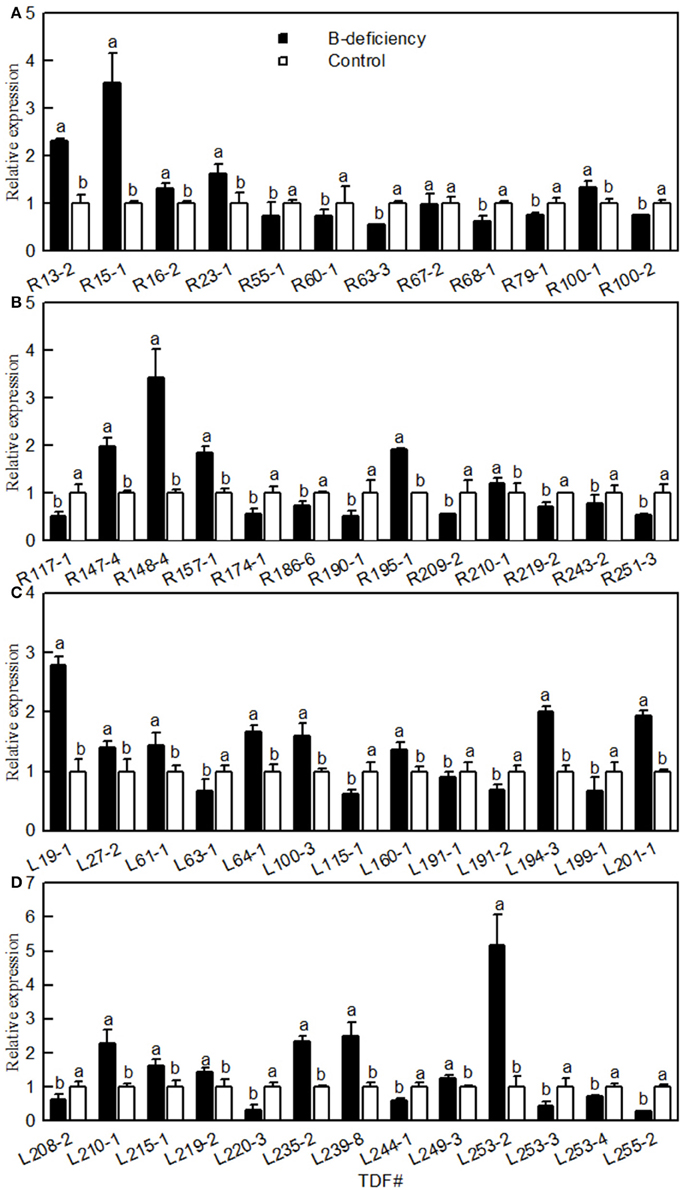
Figure 1. Effects of B-deficiency on gene expression of Citrus sinensis roots (A,B) and leaves (C,D). qRT-PCR was run in three biological replicates with two technical replicates. For the normalization of gene, citrus actin (GU911361.1) was used as an internal standard and the sample from B-sufficient plants was used as reference sample, which was set to 1. Bars represent means ± SD. Different letters above the bars indicate a significant difference at P < 0.05.
Discussion
B-deficiency-responsive-genes Differed between Roots and Leaves
We isolated less up-regulated TDFs from B-deficient roots than from B-deficient leaves, and more down-regulated TDFs from the former than from the latter (Tables 1, 2; Figure S3). This agrees with our report that mitochondrial respiration, OA metabolism and AA biosynthesis were increased in B-deficient C. sinensis leaves with more accumulation of carbohydrates, but decreased in B-deficient C. sinensis roots with less accumulation of carbohydrates (Lu et al., 2014a). Furthermore, the vast majority of the differentially expressed TDFs only presented in B-deficient roots or leaves, only seven TDFs [i.e., XP_006484536.1 (TDFs #R118-2b and L116-3b), XP_006488862.1 (TDFs #R100-2b and L100-3a), XP_007042812.1 (TDFs #R211-1b and L211-2a), XP_007043058.1 (TDFs #R219-2b and L219-2a), NP_564354.1 (TDFs #R210-1a and L210-1a), XP_006492455.1 (TDFs #R64-4a and L64-2a) and BAF01964.1 (TDFs #R20-1a, R82-7a and L224-1b)] with the same GenBank ID presented in the both. Except for XP_006484536.1, NP_564354.1, and XP_006492455.1, the remaining four TDFs in roots and leaves displayed different responses to B-deficiency (Tables 1, 2; Figure S3). To conclude, B-deficiency-induced changes in gene expression differed between roots and leaves.
Genes Involved in Carbohydrate and Energy Metabolism
The expression levels of many carbohydrate and energy metabolism-related TDFs were altered in B-deficient roots and leaves (Tables 1, 2; Figure S3). Plant UDP-glycosyltransferases (UGTs) play important parts in enhancing the tolerance of plants to environmental stresses (Bowles et al., 2006). Over-expression of UGT85A5 conferred salt tolerance in tobacco (Sun et al., 2013). However, over-expression of UGT73B2 lowered oxidative stress tolerance in Arabidopsis (Kim et al., 2010). Our results showed that the expression levels of UGTs were decreased in B-deficient roots (i.e., TDFs #R255-1b, R256-2b, R242-1b, R244-2b, and 63-3b) and increased in B-deficient leaves (i.e., TDFs #L201-1a, L3-2a and L29-2a) (Tables 1, 2), which might be related with the less and more accumulation of carbohydrates in B-deficient C. sinensis roots and leaves, respectively (Lu et al., 2014a). Thus, we proposed that the adaptive responses of UGPs differed between B-deficient roots and leaves.
The expression of cytochrome P450s (CytoP450s) was increased in Vicia sativa seedlings when exposed to plant hormone methyl jasmonate (Pinot et al., 1998) and in B-toxic C. grandis leaves (Guo et al., 2014). Transgenic tobacco and potato plants over-expressing CytoP450 displayed increased monooxygenase activity and enhanced tolerance of oxidative stress after herbicide treatment (Gorinova et al., 2005). Therefore, the up-regulation of CytoP450 (TDF #R132-6a) in B-deficient roots (Table 1) might contribute to the tolerance of plants to B-deficiency.
Root expression levels of genes related to glycolysis [i.e., pyruvate kinase (TDF #R167-3b)] and tricarboxylic acid (TCA) cycle [i.e., NAD-dependent malic enzyme 59 kDa isoform, mitochondrial (TDF #R79-1b)] were decreased by B-deficiency (Table 1). This agrees with our report that mitochondrial respiration and OA metabolism were down-regulated in B-deficient C. sinensis roots (Lu et al., 2014a). However, B-deficiency induced root expression of fumarate hydratase 2 (TDF #R59-3a; Table 1). Interestingly, enolase-like (i.e., TDFs #L255-2b and L256-2b) associated with glycolysis was repressed in B-deficient leaves (Table 2), which disagrees with our report that B-deficient C. sinensis leaves had higher mitochondrial respiration and activities of enzymes in glycolysis and TCA cycle (Lu et al., 2014a).
Hamilton et al. (2001) observed that both root vacuolar ATPase and mitochondrial ATP synthase were induced by aluminum (Al) in an Al-resistant wheat cultivar and suggested that increased ATP synthase activity was required for supporting V-ATPase induction and other energy-dependent processes associated with Al-resistance. Over-expression of a mitochondrial ATP synthase small subunit gene enhanced the salt-tolerance of transgenic tobacco plants (Zhang et al., 2006). Thus, the up-regulation of ATP synthase subunit β (i.e., TDFs #R3-1a and R16-2a) in B-deficient roots (Table 1) might be advantage to maintaining energy balance by enhancing ATP biosynthesis, when ATP synthesis was reduced due to decreased root respiration (Lu et al., 2014a).
Genes Involved in Nucleic Acid Metabolism
We isolated eight up-regulated (i.e., TDFs #R23-2a, R15-1a, R198-1a, R13-2a, R157-1a, R105-2a, R195-1a, and R147-4a) and five down-regulated (i.e., TDFs #R81-3b, R157-4b, R247-1b, R164-1b, and R100-3b) nucleic acid metabolism-related TDFs from B-deficient roots (Table 1), which disagrees with our report that the abundances of 60 B-deficiency-responsive protein species associated with nucleic acid metabolism in C. sinensis roots were decreased by B-deficiency except for argonaute family protein (Yang et al., 2013b). The difference between the two studies might be caused by post-translational modifications (PTMs).
Heat shock transcription factors (HSFs) play a role in various stresses, including oxidative stress. Davletova et al. (2005) demonstrated that HSFs were indispensable in the early sensing of H2O2 stress in Arabidopsis. Mukhopadhyay et al. (2004) reported that transgenic tobacco plants over-expressing a zinc finger protein (ZFP) gene from rice had enhanced tolerance to cold, dehydration, and salt stress. Our results showed that HSF24-like (TDF #R23-2a) and ZFP4 (TDF #R15-1a) were induced in B-deficient roots (Table 1), indicating a possible role of the two genes in B-deficiency-tolerance.
Like roots, 11 leaf TDFs (i.e., TDFs #L19-1a, L67-1a, L194-3a, L253-7a, L235-1a, L116-1a, L184-1a, L253-3b, L175-1b, L239-3b, and L227-1b) associated with nucleic acid metabolism were affected by B-deficiency (Table 2). DEAD box RNA helicases play important roles in plant stress responses. Over-expression of rice OsBIRH1, which encodes a functional DEAD-box RNA helicase, in Arabidopsis led to up-regulation of defense-related genes and enhanced disease resistance and oxidative stress tolerance (Li et al., 2008). Mishra et al. (2012) showed that WD40 proteins played a key role in stress tolerance of foxtail millet. Transgenic Arabidopsis plants over-expressing CCCH-type ZFP gene (AtSZF1) were more tolerant to salt stress (Sun et al., 2007). Therefore, the induction of DEAD box RNA helicases (i.e., TDFs #L19-1a, L67-1a, L194-3a, L253-7a), WD40 repeat-containing protein SMU1-like (TDF #L235-1a) and zinc finger CCCH domain-containing protein 22 (TDF #L116-1a) in B-deficient leaves (Table 2) might be an adaptive response of plants to B-deficiency.
Plant methyl-CpG-binding domain (MBD) proteins, which control chromatin structure mediated by CpG methylation, play crucial roles in plant development (Grafi et al., 2007). Peng et al. (2006) observed that the mbd9 mutants had more shoot branches by increasing the outgrowth of axillary buds than wild-type Arabidopsis. Our results showed that B-deficiency down-regulated the expression of MBD-containing protein 9-like isoform X2 (TDF#L175-1b) in leaves (Table 2), indicating that DNA methylation and plant development were probably impaired, thus increasing shoot branching. This agrees with the report that B-deficient plants displayed a relatively weak apical dominance, and a subsequent sprouting of lateral buds (Wang et al., 2006).
Jacobs and Kück (2011) demonstrated that RNA-binding proteins (RBPs) could modulate chloroplast RNA stability and chloroplast splicing, facilitate RNA editing. Lezhneva and Meurer (2004) showed that photosystem I (PSI) function was specifically affected in the high Chl fluorescence (HCF) 145 mutant of Arabidopsis due to the lack of the two PSI core proteins. The defect seemed to be a result of increased instability of the tricistronic psaA, psaB, and rps14 transcript (Jacobs and Kück, 2011). We observed that leaf expression of RBPHCF152 (TDF#L253-3b, Table 2), which functions in the processing of polycistronic chloroplast psbB-psbT-psbH-petB-petD transcript, was repressed by B-deficiency. Thus, it is very likely that PSI was impaired in B-deficient leaves, as observed on B-deficient C. grandis leaves (Han et al., 2009).
Genes Involved in Protein and AA Metabolism
In roots, all differentially expressed TDFs [i.e., 60S ribosomal protein L29-1-like (TDF #R100-2b), 60S ribosomal protein L31-like (TDF #R174-1b), elongation factor G (EF-G), chloroplastic-like (TDF #R253-3b) and EF-Tu, chloroplastic-like (TDF #R256-1b)] associated with protein biosynthesis were down-regulated by B-deficiency except for ribosomal protein S3 (TDF #R63-1a) and Asx tRNA synthetase (AspRS/AsnRS) class II core domain-containing protein (TDF #R103-3a) (Table 1). This agrees with our report that the levels of all the differentially expressed ribosomal proteins and EFs were decreased in B-deficient C. sinensis roots (Yang et al., 2013b). Therefore, it is likely that protein biosynthesis was impaired in B-deficient roots, thus decreasing root concentration of proteins (Lu et al., 2014a). Similarly, the expression levels of two TDFs [i.e., F-box protein At4g18380-like (TDF #R35-2b) and BTB/POZ domain-containing protein At5g41330-like (TDF # R112-5b)] related to protein ubiquitination were inhibited in B-deficient roots (Table 1), indicating that the ubiquitination of some proteins might be impaired in these roots.
Unlike roots, we isolated four up-regulated (i.e., TDFs #L132-2a, L100-3a, L209-1a, and L221-1a) and four down-regulated (i.e., TDF #L253-4b, L209-3b, L242-2b, and L256-1b) TDFs related to protein biosynthesis from B-deficient leaves (Table 2). Thus, B-deficiency-induced decrease in leaf concentration of total soluble proteins (Lu et al., 2014a) could not be explained alone by decreased biosynthesis. Our results showed that the expression levels of eight TDFs (i.e., TDFs #L113-1a, L215-1a, L52-3a, L61-1a, L253-2a, L82-2a, L211-1a, and L249-3a) associated with protein ubiquitination and proteolysis was induced in B-deficient leaves. This indicated that protein degradation might be accelerated in B-deficient leaves, thus lowering leaf concentration of total soluble proteins (Lu et al., 2014a).
Plant proteases play crucial roles in keeping strict protein quality control and degrading specific sets of proteins in response to diverse (a)biotic stimuli (García-Lorenzo et al., 2006). FtsH-mediated repairment of the PSII complex thylakoid is required for the light-induced turnover of the PSII D1 protein (Lindahl et al., 2000). Ubiquitin-mediated regulation of protein stability functions in regulating plant responses to abiotic stresses. The substrate-recruiting E3 ubiquitin ligases control numerous cellular processes through influencing the specificity of the ubiquitination pathway (Lyzenga and Stone, 2012). Thus, the up-regulation of eight TDFs (i.e., TDFs #L113-1a, L215-1a, L52-3a, L61-1a, L253-2a, L82-2a, L211-1a, and L249-3a) related to protein degradation in B-deficient leaves might contribute to the B-deficiency tolerance. However, the expression of E3 ubiquitin-protein ligase At1g12760-like (TDF #L115-1b) was down-regulated in B-deficient leaves (Table 2).
The expression levels of two TDFs (i.e., TDFs #R132-3a and R104-1a) in AA biosynthesis were decreased in B-deficient roots (Table 1), which had less accumulation of total free AAs (Lu et al., 2014a). However, we identified one down-regulated TDF (TDF #L247-2b) in AA biosynthesis and one up-regulated TDF (TDF #L202-1a) in AA degradation in B-deficient leaves (Table 2), which had more accumulation of total free AAs (Lu et al., 2014a).
Genes Involved in Cell Transport
Membrane traffic not only is a constitutive housekeeping process, but also plays a role in plant tolerance to environmental stresses (Ohno, 2006). The up-regulation of three TDFs [i.e., putative vacuolar protein sorting-associated protein 13B-like isoform X1 (TDF #R23-1a), peroxisomal membrane ABC transporter family, PMP family isoform 2 (TDF#R88-6a) and calcium-binding mitochondrial carrier protein SCaMC-1-like (TDF#R132-4a)] associated with membrane traffic in B-deficient roots (Table 1) might be an adaptive response to B-deficiency by facilitating the cell transport. Yang et al. (2013b) reported that the abundances of many membrane traffic-related protein species in C. sinensis roots were increased by B-deficiency. Besides, the expression of gene encoding early-responsive to dehydration stress protein (ERD4; TDF #R210-1a), a transmembrane (ion channel) protein, was induced in B-deficient roots. However, the expression levels of five transport-related TDFs (i.e., TDFs #R243-1b, R253-2b, R114-1b, R209_2b, and R117-1b) were down-regulated in B-deficient roots (Table 1). Similarly, we obtained three up-regulated (i.e., TDFs #L211-3a, L219-1a, and L210-1a) and seven down-regulated (i.e., TDFs #L82-3b, L191-1b, L244-1b, L117-1b, L82-1b, L253-9b, and L215-3b) transport-related TDFs from B-deficient leaves (Table 2). Obviously, cell transport was affected in B-deficient roots and leaves, thus impairing the transport of some substances, which agrees with the reports that B played a role in cell transport including membrane transport, sugar transport, and hormone transport (Tang and Dela Fuente, 1986; Brown et al., 2002; Zhou et al., 2015).
Genes Involved in Signal Transduction
Protein phosphorylation and dephosphorylation play a key role in plant stress signal transduction pathways. Transgenic Arabidopsis over-expressing TaSnRK2.4 encoding SNF1-type serine/threonine protein kinase had enhanced tolerance to drought, salt, and freezing stresses (Mao et al., 2010). Arabidopsis dual-specificity protein tyrosine phosphatase 2 (AtDsPTP2) plays a role in the tolerance of plants to oxidative stress generated by ozone (Lee and Ellis, 2007). AtPTP1 has been suggested to function in stress responses of higher plants (Xu et al., 1998). Thus, the up-regulation of four TDFs related to phosphorylation (i.e., TDFs #L219-2a and L160-1a) and phosphatases (i.e., TDFs #L123-2a and L98-4a) (Table 2) in B-deficient leaves indicated that protein phosphorylation and dephosphorylation probably played a role in B-deficiency-tolerance.
Unlike leaves, the expression levels of three phosphorylation-related TDFs (i.e., TDFs #R170-1b, R219-2b, and R251-3b) were down-regulated in B-deficient roots (Table 1), indicating that the phosphorylation of some proteins might be impaired in these roots. This agrees with our report that B-deficiency decreased the abundances of protein species in phosphorylation such as protein kinase superfamily protein with octicosapeptide/Phox/Bem1p domain (AT1G79570.1), calcium-dependent protein kinase 21 (AT4G04720.1) and kinase-related protein of unknown function (DUF1296, AT1G29370.1, and AT3G07660.1) in C. sinensis roots (Yang et al., 2013b). Also, the expression levels of three TDFs (i.e., TDFs # R119-1b, R243-2b, R182-1b, and R67-2b) in other signal pathway were decreased in B-deficient roots (Table 1). To conclude, signal transduction might be impaired in B-deficient roots.
Genes Involved in Stress Response and Defense
Senescence is a genetically programmed process governed by the developmental age and induced by (a) biotic stresses. The up-regulation of plant senescence-associated protein (TDF #R100-1a) and putative senescence-associated protein (i.e., TDFs #R148-4a and L177-3a) in B-deficient roots and leaves (Tables 1, 2) indicated that senescence might be accelerated in these tissues. However, the expression of plant senescence-associated protein (TDF # L224-2b) was repressed in B-deficient leaves (Table 2).
To deal with oxidative damage caused by reactive oxygen species (ROS), plants have evolved a scavenging system composed of antioxidants and antioxidant enzymes. We found that the expression of TDFs encoding peroxidase (POD) 30-like isoform X2 (TDF #R57-1a), phosphoadenosine phosphosulfate reductase (thioredoxin) family protein (TDF # L65-3a) and probable glutathione glutathione S-transferase (GST)-like (TDF #L231-2a) was induced in B-deficient roots (Table 1) and leaves (Table 2). This agrees with the reports that the total ability of ROS scavenging was enhanced in B-deficient citrus roots (Yang et al., 2013b) and leaves (Han et al., 2008, 2009). However, the expression of three TDFs [i.e., thioredoxin (Trx) M4, chloroplastic-like isoform X1 (TDF #R186-6b), thiosulfate sulfurtransferase 18-like isoform X1 (TDF #R178-4b) and serine hydroxymethyltransferase 7-like (TDF #R169-1b)] associated ROS scavenging was inhibited in B-deficient roots (Table 1).
Germin-like proteins (GLPs), which have different enzyme functions, including oxalate oxidase (OXO) and superoxide dismutase (SOD), play a role in plant development and various abiotic stress responses (Dunwell et al., 2008). Transgenic Arabidopsis plants overexpressing Arachis hypogaea GLP2 and 3 displayed enhanced salt-tolerance (Wang et al., 2013). Plant heat shock protein 70s (HSP 70s) function in various cellular processes including protein import into organelles (Shi and Theg, 2010) and folding of de novo-synthesized polypeptides (Hartl, 1996). A nuclear-localized HSP70 conferred heat and drought tolerance on tobacco plants (Cho and Choi, 2009). The up-regulation of GLP (TDF #L98-3a) and HSP70-binding protein 1-like (TDF #L235-2a) in B-deficient leaves demonstrated the possible involvement of the two genes in B-deficiency-tolerance.
Our finding that the expression of putative disease resistance protein RGA3-like isoform X2 (TDF # R68-1b) and putative disease resistance protein RGA4-like (TDF #R112-3b) was repressed in B-deficient roots (Table 1) agrees with our report that four disease resistance genes (i.e., AT3G14460.1, AT5G63020.1, AT4G27190.1, and AT1G12210.1) were down-regulated in B-deficient C. sinensis roots (Lu et al., 2014b). Thus, it is likely that the disease resistance was lowered in these roots, which agrees with the report that B improved the disease resistance in plants (Frenkel et al., 2010). However, the expression of protein nonrace specific disease resistance 1 (NDR1)-like (TDF # L119-2a) was induced in B-deficient leaves (Table 2).
In conclusion, most of the differentially expressed TDFs in stress defense (tolerance) were up-regulated in B-deficient leaves, while the amount of the down-regulated TDFs was more than that of the up-regulated ones in B-deficient roots (Tables 1, 2; Figure S3). Obviously, great difference existed in B-deficiency-responsive genes related to stress defense between roots and leaves.
Genes Involved in Lipid Metabolism
Cytochrome P450 86B1 (CYP86B1) participates in the ω-hydroxylation of very long chain fatty acids (FAs), and the biosynthesis of both suberin and polyester monomer (Compagnon et al., 2009). Cytochrome P450 94A1 (CYP94A1), which has FA oxidation activity, functions in cutin biosynthesis (Tijet et al., 1998). Many 3-ketoacyl-CoA synthase (KCS) enzymes take part in FA elongation. In Arabidopsis, loss of KCS20 and KCS2/DAISY led to decreased level of total wax in stems and leaves by 20 and 15%, respectively, while an increase of 10–34% was observed in transgenic leaves over-expressing KCS20 or KCS2/DAISY (Lee et al., 2009). The down-regulation of cytochrome P450 86B1-like (i.e., TDFs #R118-2b and L116-3b), cytochrome P450 94A1-like (i.e., TDFs #L198-3b and TDF#L209-4b) and KCS 10-like, partial (TDF #R60-1b) in B-deficient roots and leaves indicated that B-deficiency might impaire FA metabolism and the biosynthesis of related metabolites in these tissues. However, the expression of four TDFs (i.e., TDFs #L159-4a, L239-8a, L68-1a, and R190-1a) associated with FA metabolism was induced in B-deficient roots and leaves (Tables 1, 2). Epoxide hydrolases (EHs) play important roles in cuticle formation, responses to stresses, and pathogen defenses (Morisseau, 2013). Thus, The up-regulation of bifunctional epoxide hydrolase 2-like isoform X2 (TDF #L159-4a) in B-deficient leaves (Table 2) might contribute to the B-deficiency-tolerance.
Genes Involved in Cell Wall Modification
In cell wall, proline-rich proteins (PRPs) cross-link each other or link to other components (i.e., saccharides and lignin) to form effective protection layer after pathogen infection or wounding (Brisson et al., 1994). Zhou et al. (2015) reported that the expression levels of two PRP2 genes (i.e., JK817586 and JK817604) in B-deficiency-sensitive Poncirus trifoliate roots were down-regulated by 24 and/or 6 h B-deficient treatments, while B-deficiency did not alter their expression in B-deficiency-tolerant Carrizo citrange (C. sinensis × P. trifoliata) roots. Thus, the up-regulation of 14 kDa PRP DC2.15-like in B-deficient roots might contribute to the B-deficiency-tolerance by reinforcing cell walls.
Expansins, which enable the growing cell wall to extend, are considered to be crucial regulators of wall extension during growth (Link and Cosgrove, 1998). The down-regulation of expansin-like B1-like (TDF #R55-1b) in B-deficient roots (Table 1) indicated that root cell elongation might be impaired (Lee and Kende, 2002), thus inhibiting root growth (Figure S1). Similar results have been obtained in B-deficient Arabidopsis roots (Camacho-Cristóbal et al., 2008b) and citrus roots (Zhou et al., 2015).
Laccase, which catalyzes the oxidation of phenolic substrates using oxygen as the electron acceptor, is required for lignin polymerization during vascular development in Arabidopsis (Zhao et al., 2013). Ranocha et al. (2002) reported that neither lignin level nor composition was affected due to a repression of laccase expression in poplar. However, the antisense transgenic population, lac3AS, had a two- to three-fold increase in total soluble phenolic level. In addition, lac3 suppression caused a dramatic alteration of xylem fiber cell walls. We observed that B-deficiency down-regulated the expression level of laccase-3-like (TDF #L251-2b) in leaves (Table 2), thus increasing leaf concentration of total soluble phenolics and altering cell wall structure. This agrees with the reports that B-deficient C. sinensis leaves had higher level of total phenolics (Lu et al., 2014a) and that B-deficiency altered cell wall structure, thus causing growth defects of C. sinensis leaves (Liu et al., 2014).
Others
VEP1, which encodes a short-chain dehydrogenase/reductase with 3-oxo-Δ4,5-steroid 5-β-reductase activity in Arabidopsis, is required for vascular strand development (Jun et al., 2002). The down-regulation of 3-oxo-Δ4,5-steroid 5-β-reductase-like isoform X2 (TDF #R103-1b) in B-deficient roots (Table 1) indicated that B-deficiency might impair root vascular development. This agrees with the report that B-deficiency affected vascular development in roots, stems, leaves, and nodules of plants (Kouchi and Kumazawa, 1975; Bolaños et al., 1994; Hajiboland et al., 2012).
Gibberellin (GA) 2-β-dioxygenase 2-like (GA2OX2; i.e., TDFs #R64-4a and L64-2a) functioned in the catabolism of GAs was induced in B-deficient roots and leaves (Tables 1, 2). This implied that the degradation of GAs might be enhanced in these tissues, thus reducing their concentrations. This agrees with the report that B-deficient plants had lower levels of GAs (Shi and Liu, 2002).
Conclusions
This is the first comparative investigation of B-deficiency-induced alterations in gene expression profiles in C. sinensis roots and leaves using cDNA-AFLP. We isolated more up-regulated TDFs from B-deficient leaves than from B-deficient roots, and less down-regulated TDFs from the former than from the latter, which agrees with our report that mitochondrial respiration, OA metabolism, and AA biosynthesis are enhanced in B-deficient leaves with more accumulation of carbohydrates, but reduced in B-deficient roots with less accumulation of carbohydrates. The majority of B-deficiency-responsive TDFs were isolated only from roots or leaves, only seven TDFs with the same GenBank ID were isolated from the both. Furthermore, only three differentially expressed TDFs shared by the both displayed similar expression trend in response to B-deficiency. Besides, UGTs were repressed in B-deficient roots, but were induced in B-deficient leaves; however, TDFs related to ATP biosynthesis were up-regulated in the former, but were unaffected in the latter. Most of the differentially expressed TDFs associated with signal transduction and stress defense were down-regulated in B-deficient roots, but up-regulated in B-deficient leaves. Eight (one) TDFs related to protein ubiquitination and proteolysis were induced (inhibited) in B-deficient leaves, while only two down-regulated TDFs involved in ubiquitination were detected in B-deficient roots. Through integration of the present results and the previous data available in the literatures, we presented a possible model for the responses of C. sinensis roots and leaves to B-deficiency (Figure 2). Obviously, many differences existed in long-term B-deficiency-responsive genes between roots and leaves. These findings presented an integrated view of the differential responses occurring in B-deficient roots and leaves and revealed new insight into the different adaptive mechanisms of C. sinensis roots and leaves to B-deficiency at the transcriptional level. Our results are also useful for obtaining the key genes responsible for citrus B-deficiency-tolerance and for improving citrus productivity and quality. Therefore, these results are of great importance from the citrus breeding and production point of view.
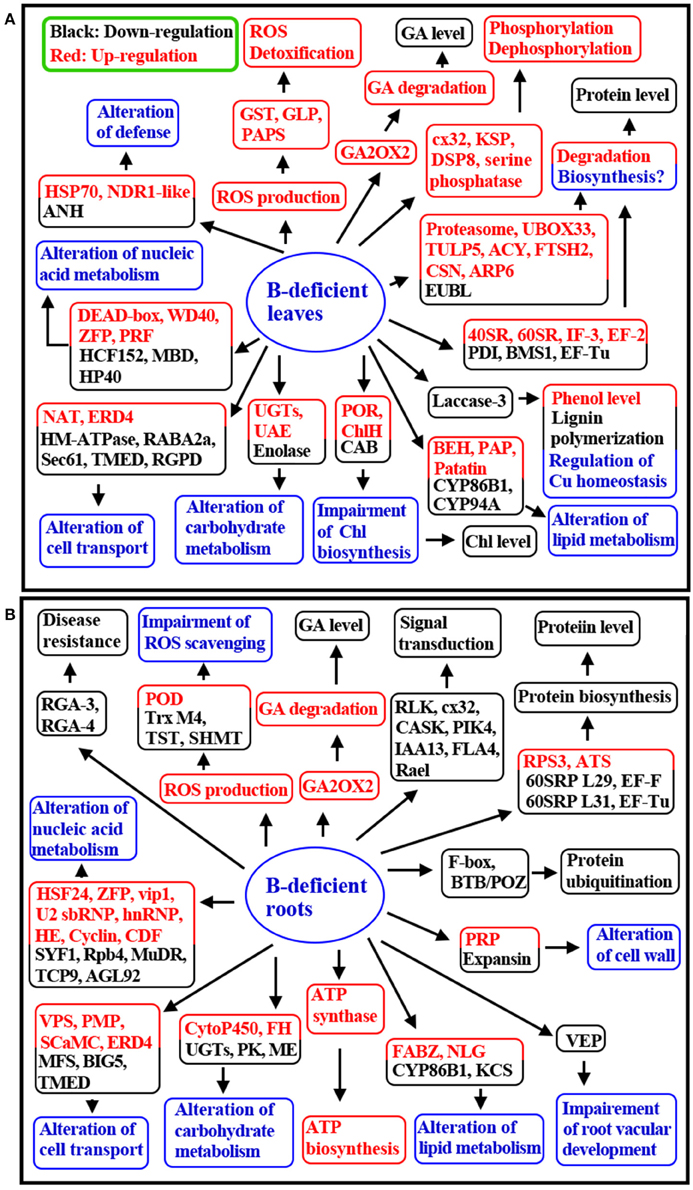
Figure 2. A proposed model for the responses of C. sinensis leaves (A) and roots (B) to B-deficiency. 40SRP, 40S ribosomal protein; 60SRP, 60S ribosomal protein; ACY, Aminoacylase; ANH, Adenine nucleotide α hydrolases-like superfamily protein; ARP6, Auxin-resistance protein 6; ATS, Asx tRNA synthetase (AspRS/AsnRS) class II core domain-contating protein; BEH, Bifunctional epoxide hydrolase; BIG5, Brefeldin A-inhibited guanine nucleotide-exchange protein 5-like; CAB, Chlorophyll a-b binding protein; CASK, Calcium and calcium/calmodulin-dependent serine/threonine-protein kinase; CDF, Cyclin-like family protein; ChlH, Mg-chelatase subunit ChlH, chloroplastic-like; CSN, COP9 signalosome complex subunit 1; cx32, Serine/threonine-protein kinase cx32 isoform 2; DSP8, Putative dual specificity protein phosphatase DSP8-like isoform X2; EF, Elongation factor; EUBL, E3 ubiquitin-protein ligase; FABZ, Beta-hydroxyacyl-ACP dehydratase family protein; FH, Fumarate hydratase 2; FLA4, Fasciclin-like arabinogalactan protein 4-like; FTSH2, ATP-dependent zinc metalloprotease FTSH 2; HE, RRNA intron-encoded homing endonuclease; HM-ATPase, Heavy metal translocating P-type ATPase; hnRNP, Heterogeneous nuclear ribonucleoprotein; HP40, Homeobox protein 40; IF, Translation initiation factor; KSP, Kinase superfamily protein; MFS, Major facilitator superfamily protein; NAT, Nucleobase-ascorbate transporter 4-like; NLG, Non-lysosomal glucosylceramidase-like; PAP, Probable plastid-lipid-associated protein; PAPS, Phosphoadenosine phosphosulfate reductase family protein; PIK4, Phosphatidylinositol 4-kinase; POR, Protochlorophyllide reductase; PRF, Putative replication factor; PRP, Proline-rich protein; RGPD, RANBP2-like and GRIP domain-containing protein; RLK, Receptor-like protein kinase; RPS3, Ribosomal protein S3; SHMT, Serine hydroxymethyltransferas; TMED, Transmembrane emp24 domain; TST, Thiosulfate sulfurtransferase; TULP5, Tubby-like F-box protein 5-like; U2 snRNP, U2 small nuclear ribonucleoprotein; UAE, UDP-arabinose 4-epimerase; UBOX33, U-box domain-containing protein 33; VPS, Vacuolar protein sorting-associated protein.
Conflict of Interest Statement
The authors declare that the research was conducted in the absence of any commercial or financial relationships that could be construed as a potential conflict of interest.
Acknowledgments
This study was financially supported by the National Natural Science Foundation of China (No. 31171947) and the earmarked fund for China Agriculture Research System (No. CARS-27).
Supplementary Material
The Supplementary Material for this article can be found online at: http://journal.frontiersin.org/article/10.3389/fpls.2015.00585
References
Aleza, P., Juárez, J., Ollitrault, P., and Navarro, L. (2010). Polyembryony in non-apomictic citrus genotypes. Ann Bot. 106, 533–545. doi: 10.1093/aob/mcq148
Beato, V. M., Rexach, J., Navarro-Gochicoa, M. T., Camacho-Cristóbal, J. J., Herrera-Rodríguez, M. B., Maldonado, J. M., et al. (2010). A tobacco asparagine synthetase gene responds to carbon and nitrogen status and its root expression is affected under boron stress. Plant Sci. 178, 289–298. doi: 10.1016/j.plantsci.2009.12.008
Bohnsack, C. W., and Albert, L. S. (1977). Early effects of boron deficiency on indoleacetic acid oxidase levels of squash root tips. Plant Physiol. 59, 1047–1050. doi: 10.1104/pp.59.6.1047
Bolaños, L., Esteban, E., de Lorenzo, C., Fernandez-Pascual, M., de Felipe, M. R., Garate, A., et al. (1994). Essentiality of boron for symbiotic dinitrogen fixation in pea (Pisum sativum) rhizobium nodules. Plant Physiol. 104, 85–90.
Bowles, D., Lim, E. K., Poppenberger, B., and Vaistij, F. E. (2006). Glycosyltransferases of lipophilic small molecules. Annu. Rev. Plant Biol. 57, 567–597. doi: 10.1146/annurev.arplant.57.032905.105429
Brisson, L. F., Tenhaken, R., and Lamb, C. (1994). Function of oxidative cross-Linking of cell wall structural proteins in plant disease resistance. Plant Cell 6, 1703–1712. doi: 10.1105/tpc.6.12.1703
Brown, P. H., Bellaloui, N., Wimmer, M. A., Bassil, E. S., Ruiz, J., Hu, H., et al. (2002). Boron in plant biology. Plant Biol. 4, 205–223. doi: 10.1055/s-2002-25740
Camacho-Cristóbal, J. J., and González-Fontes, A. (2007). Boron deficiency decreases plasmalemma H+-ATPase expression and nitrate uptake, and promotes ammonium assimilation into asparagine in tobacco roots. Planta 226, 443–451. doi: 10.1007/s00425-007-0494-2
Camacho-Cristóbal, J. J., Herrera-Rodríguez, M. B., Beato, V. M., Rexach, J., Navarro-Gochicoa, M. T., Maldonado, J. M., et al. (2008b). The expression of several cell wall-related genes in Arabidopsis roots is down-regulated under boron deficiency. Environ. Exp. Bot. 63, 351–358. doi: 10.1016/j.envexpbot.2007.12.004
Camacho-Cristóbal, J. J., Rexach, J., and González-Fontes, A. (2008a). Boron in plants: deficiency and toxicity. J. Integr. Plant Biol. 50, 1247–1255. doi: 10.1111/j.1744-7909.2008.00742.x
Camacho-Cristóbal, J. J., Rexach, J., Herrera-Rodríguez, M. B., Navarro-Gochicoa, M. T., and González-Fontes, A. (2011). Boron deficiency and transcript level changes. Plant Sci. 181, 85–89. doi: 10.1016/j.plantsci.2011.05.001
Chapman, H. D. (1968). “The mineral nutrition of citrus,” in The Citrus Industry, Division of Agricultural Sciences, Vol. 2, eds W. Reuther, H. J. Webber, and L. D. Batchelor (Berkeley, CA: University of California), 127–189.
Chen, L. S., Han, S., Qi, Y. P., and Yang, L. T. (2012). Boron stresses and tolerance in citrus. Afr. J. Biotech. 11, 5961–5969. doi: 10.5897/AJBX11.073
Cho, E. K., and Choi, Y. J. (2009). A nuclear-localized HSP70 confers thermoprotective activity and drought-stress tolerance on plants. Biotechnol. Lett. 31, 597–606. doi: 10.1007/s10529-008-9880-5
Compagnon, V., Diehl, P., Benveniste, I., Meyer, D., Schaller, H., Schreiber, L., et al. (2009). CYP86B1 is required for very long chain omega-hydroxyacid and alpha, omega-dicarboxylic acid synthesis in root and seed suberin polyester. Plant Physiol. 150, 1831–1843. doi: 10.1104/pp.109.141408
Davletova, S., Rizhsky, L., Liang, H., Zhong, S., Oliver, D. J., Coutu, J., et al. (2005). Cytosolic ascorbate peroxidase 1 is a central component of the reactive oxygen gene network of Arabidopsis. Plant Cell 17, 268–281. doi: 10.1105/tpc.104.026971
Dunwell, J. M., Gibbings, J. G., Mahmood, T., and Naqvi, S. M. (2008). Germin and germin-like proteins: evolution, structure, and function. Cri. Rev. Plant Sci. 27, 342–375. doi: 10.1080/07352680802333938
Frenkel, O., Yermiyahu, U., Forbes, G. A., Fry, W. E., and Shtienberg, D. (2010). Restriction of potato and tomato late blight development by sub-phytotoxic concentrations of boron. Plant Pathol. 59, 626–633. doi: 10.1111/j.1365-3059.2010.02301.x
García-Lorenzo, M., Sjödin, A., Jansson, S., and Funk, C. (2006). Protease gene families in Populus and Arabidopsis. BMC Plant Biol. 6:30. doi: 10.1186/1471-2229-6-30
Gorinova, N., Nedkovska, M., and Atanassov, A. (2005). Cytochrome P450 monooxygenases as a tool for metabolization herbicides in plants. Biotechnol. Biotechnol. Eq. 19, 105–115. doi: 10.1080/13102818.2005.10817290
Grafi, G., Zemach, A., and Pitto, L. (2007). Methyl-CpG-binding domain (MBD) proteins in plants. Biochim. Biophys. Acta 1769, 287–294. doi: 10.1016/j.bbaexp.2007.02.004
Guo, P., Qi, Y. P., Yang, L. T., Ye, X., Jiang, H. X., Huang, J. H., et al. (2014). cDNA-AFLP analysis reveals the adaptive responses of citrus to long-term boron-toxicity. BMC Plant Biol. 14:284. doi: 10.1186/s12870-014-0284-5
Hajiboland, R., Bahrami-Rad, S., and Bastani, S. (2013). Phenolics metabolism in boron-deficient tea [Camellia sinensis (L.) O. Kuntze] plants. Acta Biol. Hung. 64, 196–206. doi: 10.1556/ABiol.64.2013.2.6
Hajiboland, R., Farhanghi, F., and Aliasgharpour, M. (2012). Morphological and anatomical modifications in leaf, stem and roots of four plant species under boron deficiency conditions. Anal Biol. 34, 15–29. doi: 10.6018/analesbio.0.34.4
Hamilton, C. A., Good, A. G., and Taylor, G. J. (2001). Induction of vacuolar ATPase and mitochondrial ATP synthase by aluminum in an aluminum-resistant cultivar of wheat. Plant Physiol. 125, 2068–2077. doi: 10.1104/pp.125.4.2068
Han, S., Chen, L. S., Jiang, H. X., Smith, B. R., Yang, L. T., and Xie, C. Y. (2008). Boron deficiency decreases growth and photosynthesis, and increases starch and hexoses in leaves of citrus seedlings. J. Plant Physiol. 165, 1331–1341. doi: 10.1016/j.jplph.2007.11.002
Han, S., Tang, N., Jiang, H. X., Yang, L. T., Li, Y., and Chen, L. S. (2009). CO2 assimilation, photosystem II photochemistry, carbohydrate metabolism and antioxidant system of citrus leaves in response to boron stress. Plant Sci. 176, 143–153. doi: 10.1016/j.plantsci.2008.10.004
Hartl, F. U. (1996). Molecular chaperones in cellular protein folding. Nature 381, 571–579. doi: 10.1038/381571a0
Huang, Y. Z., Li, J., Wu, S. H., and Pang, D. M. (2001). Nutrition condition of the orchards in the main production areas of Guanxihoney pomelo trees (Pinhe county). J. Fujian Agri. Univ. 30, 40–43.
Jacobs, J., and Kück, U. (2011). Function of chloroplast RNA-binding proteins. Cell Mol. Life Sci. 68, 735–748. doi: 10.1007/s00018-010-0523-3
Jun, J. H., Ha, C. M., and Nam, H. G. (2002). Involvement of the VEP1 gene in vascular strand development in Arabidopsis thaliana. Plant Cell Physiol. 43, 323–330. doi: 10.1093/pcp/pcf042
Kim, I. A., Heo, J. O., Chang, K. S., Lee, S. A., Lee, M. H., Lim, C. E., et al. (2010). Overexpression and inactivation of UGT73B2 modulate tolerance to oxidative stress in Arabidopsis. J. Plant Biol. 53, 233–239. doi: 10.1007/s12374-010-9110-2
Kouchi, H., and Kumazawa, K. (1975). Anatomical responses of root tips to boron deficiency II. Effect of boron deficiency on the cellular growth and development in root tips. Soil Sci. Plant Nutr. 21, 137–150. doi: 10.1080/00380768.1975.10432630
Kowalenko, C. G., and Lavkulich, L. M. (1976). Modified curcumin method for boron analysis of soil extracts. Can. J. Soil Sci. 56, 537–539. doi: 10.4141/cjss76-068
Lee, J. S., and Ellis, B. E. (2007). Arabidopsis MAPK phosphatase 2 (MKP2) positively regulates oxidative stress tolerance and inactivates the MPK3 and MPK6 MAPKs. J. Biol. Chem. 282, 25020–25029. doi: 10.1074/jbc.M701888200
Lee, S. B., Jung, S. J., Go, Y. S., Kim, H. U., Kim, J. K., Cho, H. J., et al. (2009). Two Arabidopsis 3-ketoacyl CoA synthase genes, KCS20 and KCS2/DAISY, are functionally redundant in cuticular wax and root suberin biosynthesis, but differentially controlled by osmotic stress. Plant J. 60, 462–475. doi: 10.1111/j.1365-313X.2009.03973.x
Lee, Y., and Kende, H. (2002). Expression of α-expansin and expansin-like genes in deepwater rice. Plant Physiol. 130, 1396–1405. doi: 10.1104/pp.008888
Lezhneva, L., and Meurer, J. (2004). The nuclear factor HCF145 affects chloroplast psaA-psaB-rps14 transcript abundance in Arabidopsis thaliana. Plant J. 38, 740–753. doi: 10.1111/j.1365-313X.2004.02081.x
Li, D., Liu, H., Zhang, H., Wang, X., and Song, F. (2008). OsBIRH1, a DEAD-box RNA helicase with functions in modulating defence responses against pathogen infection and oxidative stress. J. Exp. Bot. 59, 2133–2146. doi: 10.1093/jxb/ern072
Lindahl, M., Spetea, C., Hundal, T., Oppenheim, A. B., Adam, Z., and Andersson, B. (2000). The thylakoid FtsH protease plays a role in the light-induced turnover of the photosystem II D1 protein. Plant Cell 12, 419–431. doi: 10.1105/tpc.12.3.419
Link, B. M., and Cosgrove, D. J. (1998). Acid-growth response and α-expansins in suspension cultures of bright yellow 2 tobacco. Plant Physiol. 118, 907–916. doi: 10.1104/pp.118.3.907
Liu, G., Deng, X., Liu, L., Wu, L., Peng, S., and Jiang, C. (2014). Boron deficiency is correlated with changes in cell wall structure that lead to growth defects in the leaves of navel orange plants. Sci. Hort. 176, 54–62. doi: 10.1016/j.scienta.2014.06.036
Liu, Z., Zhu, Q. Q., and Tong, L. H. (1989). Regularities of content and distribution of boron in soils. Acta Pedo. Sin. 26, 353–361.
Lu, Y. B., Yang, L. T., Li, Y., Xu, J., Liao, T. T., Chen, Y. B., et al. (2014a). Effects of boron deficiency on major metabolites, key enzymes and gas exchange in leaves and roots of Citrus sinensis seedlings. Tree Physiol. 34, 608–618. doi: 10.1093/treephys/tpu047
Lu, Y. B., Yang, L. T., Qi, Y. P., Li, Y., Li, Z., Chen, Y. B., et al. (2014b). Identification of boron-deficiency-responsive microRNAs in Citrus sinensis roots by Illumina sequencing. BMC Plant Biol. 14:123. doi: 10.1186/1471-2229-14-123
Lyzenga, W. J., and Stone, S. L. (2012). Abiotic stress tolerance mediated by protein ubiquitination. J. Exp. Bot. 63, 599–616. doi: 10.1093/jxb/err310
Mao, X. G., Zhang, H. Y., Tian, S. J., Chang, X. P., and Jing, R. L. (2010). TaSnRK2.4, an SNF1-type serine/threonine protein kinase of wheat (Triticum aestivum L.), confers enhanced multistress tolerance in Arabidopsis. J. Exp. Bot. 61, 683–896. doi: 10.1093/jxb/erp331
Mishra, A. K., Puranik, S., Bahadur, R. P., and Prasad, M. (2012). The DNA-binding activity of an AP2 protein is involved in transcriptional regulation of a stress-responsive gene, SiWD40, in foxtail millet. Genomics 100, 252–263. doi: 10.1016/j.ygeno.2012.06.012
Morisseau, C. (2013). Role of epoxide hydrolases in lipid metabolism. Biochimie 95, 91–95. doi: 10.1016/j.biochi.2012.06.011
Mukhopadhyay, A., Vij, S., and Tyagi, A. K. (2004). Overexpression of a zinc-finger protein gene from rice confers tolerance to cold, dehydration, and salt stress in transgenic tobacco. Proc. Natl. Acad. Sci. U.S.A. 101, 6309–6314. doi: 10.1073/pnas.0401572101
Ohno, H. (2006). Membrane traffic in multicellular systems: more than just a housekeeper. J. Biochem. 139, 941–942. doi: 10.1093/jb/mvj119
Peng, M., Cui, Y., Bi, Y. M., and Rothstein, S. J. (2006). AtMBD9: a protein with a methyl-CpG-binding domain regulates flowering time and shoot branching in Arabidopsis. Plant J. 46, 282–296. doi: 10.1111/j.1365-313X.2006.02691.x
Pinot, F., Benveniste, I., Salaün, J. P., and Durst, F. (1998). Methyl jasmonate induces lauric acid ω-hydroxylase activity and accumulation of CYP94A1 transcripts but does not affect epoxide hydrolase activities in Vicia sativa seedlings. Plant Physiol. 118, 1481–1486. doi: 10.1104/pp.118.4.1481
Ranocha, P., Chabannes, M., Chamayou, S., Danoun, S., Jauneau, A., Boudet, A. M., et al. (2002). Laccase down-regulation causes alterations in phenolic metabolism and cell wall structure in poplar. Plant Physiol. 129, 145–155. doi: 10.1104/pp.010988
Redondo-Nieto, M., Maunoury, N., Mergaert, P., Kondorosi, E., Bonilla, I., and Bolaños, L. (2012). Boron and calcium induce major changes in gene expression during legume nodule organogenesis. Does boron have a role in signalling? New Phytol. 195, 14–19. doi: 10.1111/j.1469-8137.2012.04176.x
Shi, L. X., and Theg, S. M. (2010). A stromal heat shock protein 70 system functions in protein import into chloroplasts in the moss Physcomitrella patens. Plant Cell 22, 205–220. doi: 10.1105/tpc.109.071464
Shi, Y. H., and Liu, P. (2002). A review of advances in physiological function of boron in plants. Subtropical Plant Sci. 31, 64–69. doi: 10.3969/j.issn.1009-7791.2002.02.015
Stavrianakou, S., Liakopoulos, G., and Karabourniotis, G. (2006). Boron deficiency effects on growth, photosynthesis and relative concentrations of phenolics of Dittrichia viscosa (Asteraceae). Environ. Exp. Bot. 56, 293–300. doi: 10.1016/j.envexpbot.2005.03.007
Sun, J., Jiang, H., Xu, Y., Li, H., Wu, X., Xie, Q., et al. (2007). The CCCH-type zinc finger proteins AtSZF1 and AtSZF2 regulate salt stress responses in Arabidopsis. Plant Cell Physiol. 48, 1148–1158. doi: 10.1093/pcp/pcm088
Sun, Y. G., Wang, B., Jin, S. H., Qu, X. X., Li, Y. J., and Hou, B. K. (2013). Ectopic expression of Arabidopsis glycosyltransferase UGT85A5 enhances salt stress tolerance in tobacco. PLoS ONE 8:e59924. doi: 10.1371/journal.pone.0059924
Tang, P. M., and Dela Fuente, R. K. (1986). The transport of indole-3-acetic acid in boron- and calcium-deficient sunflower hypocotyl segments. Plant Physiol. 81, 646–650. doi: 10.1104/pp.81.2.646
Tijet, N., Helvig, C., Pinot, F., Le Bouquin, R., Lesot, A., Durst, F., et al. (1998). Functional expression in yeast and characterization of a clofibrate-inducible plant cytochrome P-450 (CYP94A1) involved in cutin monomers synthesis. Biochem. J. 332, 583–589.
Wang, G., Römheld, V., Li, C., and Bangerth, F. (2006). Involvement of auxin and CKs in boron deficiency induced changes in apical dominance of pea plants (Pisum sativum L.). J. Plant Physiol. 163, 591–600. doi: 10.1016/j.jplph.2005.09.014
Wang, T., Chen, X., Zhu, F., Li, H., Li, L., Yang, Q., et al. (2013). Characterization of peanut germin-like proteins, AhGLPs in plant development and defense. PLoS ONE 8:e61722. doi: 10.1371/journal.pone.0061722
Xu, Q., Fu, H., Gupta, R., and Luan, S. (1998). Molecular characterization of a tyrosine-specific protein phosphatase encoded by a stress-responsive gene in Arabidopsis. Plant Cell 10, 849–857. doi: 10.1105/tpc.10.5.849
Yang, C. Q., Liu, Y. Z., An, J. C., Li, S., Jin, L. F., Zhou, G. F., et al. (2013a). Digital gene expression analysis of corky split vein caused by boron deficiency in ‘Newhall’ Navel Orange (Citrus sinensis Osbeck) for selecting differentially expressed genes related to vascular hypertrophy. PLoS ONE 8:e65737. doi: 10.1371/journal.pone.0065737
Yang, L. T., Qi, Y. P., Lu, Y. B., Guo, P., Sang, W., Feng, H., et al. (2013b). iTRAQ protein profile analysis of Citrus sinensis roots in response to long-term boron-deficiency. J. Proteomics 93, 179–206. doi: 10.1016/j.jprot.2013.04.025
Zhang, X., Takano, T., and Shenkui Liu, S. (2006). Identification of a mitochondrial ATP synthase small subunit gene (RMtATP6) expressed in response to salts and osmotic stresses in rice (Oryza sativa L.). J. Exp. Bot. 57, 193–200. doi: 10.1093/jxb/erj025
Zhao, Q., Nakashima, J., Chen, F., Yin, Y., Fu, C., Yun, J., et al. (2013). Laccase is necessary and nonredundant with peroxidase for lignin polymerization during vascular development in Arabidopsis. Plant Cell 25, 3976–3987. doi: 10.1105/tpc.113.117770
Zhou, C. P., Qi, Y. P., You, X., Yang, L. T., Guo, P., Ye, X., et al. (2013). Leaf cDNA-AFLP analysis of two citrus species differing in manganese tolerance in response to long-term manganese-toxicity. BMC Genomics 14:621. doi: 10.1186/1471-2164-14-621
Keywords: boron-deficiency, cDNA-AFLP, Citrus sinensis, leaves, roots, transcript-derived fragment
Citation: Lu Y-B, Qi Y-P, Yang L-T, Lee J, Guo P, Ye X, Jia M-Y, Li M-L and Chen L-S (2015) Long-term boron-deficiency-responsive genes revealed by cDNA-AFLP differ between Citrus sinensis roots and leaves. Front. Plant Sci. 6:585. doi: 10.3389/fpls.2015.00585
Received: 13 April 2015; Accepted: 13 July 2015;
Published: 28 July 2015.
Edited by:
Maria Carlota Vaz Patto, Instituto de Tecnologia Quimica e Biologica/Universidade Nova de Lisboa, PortugalReviewed by:
Pedro Martinez-Gomez, Centro de Edafología y Biología Aplicada del Segura-Consejo Superior de Investigaciones Científicas, SpainAgustín González-Fontes, Universidad Pablo de Olavide, Sevilla, Spain
Copyright © 2015 Lu, Qi, Yang, Lee, Guo, Ye, Jia, Li and Chen. This is an open-access article distributed under the terms of the Creative Commons Attribution License (CC BY). The use, distribution or reproduction in other forums is permitted, provided the original author(s) or licensor are credited and that the original publication in this journal is cited, in accordance with accepted academic practice. No use, distribution or reproduction is permitted which does not comply with these terms.
*Correspondence: Li-Song Chen, Department of Resource and Environment, College of Resource and Environmental Science, Fujian Agriculture and Forestry University, Boxue Building, No. 15 Shangxiadian Road, Cangshan District, Fuzhou 350002, China,bGlzb25nY2hlbjIwMDJAaG90bWFpbC5jb20=