- 1National Engineering Laboratory for Tree Breeding, College of Biological Sciences and Technology, Beijing Forestry University, Beijing, China
- 2Key Laboratory of Genetics and Breeding in Forest Trees and Ornamental Plants, College of Biological Sciences and Technology, Beijing Forestry University, Beijing, China
DNA methylation and miRNAs provide crucial regulation of the transcriptional and post-transcriptional responses to abiotic stress. In this study, we used methylation-sensitive amplification polymorphisms to identify 1066 sites that were differentially methylated in response to temperature stress in Populus simonii. Among these loci, BLAST searches of miRBase identified seven miRNA genes. Expression analysis by quantitative real-time PCR suggested that the methylation pattern of these miRNA genes probably influences their expression. Annotation of these miRNA genes in the sequenced genome of Populus trichocarpa found three target genes (Potri.007G090400, Potri.014G042200, and Potri.010G176000) for the miRNAs produced from five genes (Ptc-MIR396e and g, Ptc-MIR156i and j, and Ptc-MIR390c) respectively. The products of these target genes function in lipid metabolism to deplete lipid peroxide. We also constructed a network based on the interactions between DNA methylation and miRNAs, miRNAs and target genes, and the products of target genes and the metabolic factors that they affect, including H2O2, malondialdehyde, catalase (CAT), and superoxide dismutase. Our results suggested that DNA methylation probably regulates the expression of miRNA genes, thus affecting expression of their target genes, likely through the gene-silencing function of miRNAs, to maintain cell survival under abiotic stress conditions.
Introduction
Temperature has major effects on plant growth and development in the field, where temperatures can change frequently, potentially causing stress on the plant. Heat or cold stress can negatively affect many physiological processes; thus plants have evolved complex signaling pathways that perceive and transduce signals in response to particular stresses (You et al., 2014). These signals act through H2O2, malondialdehyde (MDA), catalase (CAT), and superoxide dismutase (SOD), which affect the degree of response to abiotic stress (Nie et al., 2015; Zhang et al., 2015). Some of these signals require transcription and are broadly regulated by a variety of factors, including cytosine methylation, covalent modification of DNA by 5-methylcytosines. For example, alterations in methylation might be critical to energy metabolism in the Antarctic polychaete Spiophanes tcherniai (Marsh and Pasqualone, 2014). Rakei et al. (2015) identified specific DNA sequences that play an important role in cold tolerance with possible responsive components correlated with cold stress in plants, suggesting that DNA methylation regulates cold stress signals. In addition, the altered methylation state of CycD3-1 and Nt-EXPA5 shifted their expression during heat stress in tobacco (Centomani et al., 2015).
MicroRNAs (miRNAs) also regulate expression of genes directly and indirectly related to the response to temperature stress. MiRNAs are small (21–24 nucleotides), non-coding, single-stranded RNAs derived predominantly from intergenic regions, and function as key regulators of gene expression (Sunkar and Zhu, 2004). For instance, work in Arabidopsis thaliana identified 29 miRNAs that regulate gene expression in response to drought stress (Hajdarpašić and Ruggenthaler, 2012). Also, in Larix leptolepis, four miRNA families (miR159, miRNA169, miRNA171, and miRNA172) are all induced by abiotic stress and their targets regulate genes crucial to cell development, including MYB transcription factors (miR159), an NF-YA transcription factor (miR169), a scarecrow-like transcription factor (miR171) and apetala2 (miR172; Zhang et al., 2010). In addition, Ishikawa et al. (2014) found that a genotoxic stress-responsive miRNA, miR574-3p, delays cell growth by suppressing the enhancer of a rudimentary homolog gene in vitro.
Thus, miRNAs and DNA methylation have crucial functions in regulating gene expression in response to abiotic stress in plants. However, so far, few studies have examined possible mutual adjustments between DNA methylation and miRNAs. Rykov et al. (2013) found that the methylation of MIR125b-1 and MIR137 genes was correlated with non-small-cell lung cancer progression. Also, repression of miRNAs was correlated to hypermethylation of their promoters in human cancer cells (Li et al., 2011). Additional research has begun to examine the potential interaction between DNA methylation and miRNAs in response to abiotic stress in plants, but so far this interaction remains unclear.
In comparison to annual plants, perennial plants undergo more temperature changes over their longer lives. Here, we chose Populus simonii as an experimental system to examine the possible interaction between cytosine methylation and miRNAs. The advantages of using Populus species as genomic models for tree molecular biology have been extensively reported. Among Populus species, P. simonii shows broad geographic distribution and a strong ability to survive, even in extreme temperatures (-41 to +43°C) and under other abiotic stresses. It is one of the most important native tree species in northern China, for its commercial and ecological value (Wang et al., 2012).
Considering global climate change and frequent extreme weather, including very low and high temperatures, studying the plant response to temperature stress may provide important information for future agricultural and ecological studies. Given the increasing evidence for miRNAs and DNA methylation as important regulators of gene expression in response to abiotic stresses, we hypothesized that the potential interaction of miRNAs and DNA methylation plays a critical role in stress-responsive gene expression. Here, we used P. simonii, a resistant and adaptable species, for methylation-sensitive amplification polymorphism (MSAP) and transcript level analysis through quantitative real-time PCR (qRT-PCR) to uncover changes of methylation and expression of miRNA genes in response to heat and cold stress. This study provides new insights on different DNA methylation-mediated regulatory mechanisms in the response to temperature stresses in plants.
Materials And Methods
Plant Materials and Treatments
Populus simonii ‘QL9’ was planted in pots under natural light conditions (1,250 l mol m-2 s-1 of photosynthetically active radiation), 25 ± 1°C, 50% ± 1 relative humidity and 12 h day/night in an air-conditioned greenhouse. In this study, thirty annual clones of the same size (50 cm in height) were divided into three groups; one group was chosen to act as the control group and other two groups were treated by heat or cold stress, respectively. These treatment groups were exposed to 42 and 4°C for 3, 6, 12, and 24 h for heat, and cold stress treatments, respectively. The 3- and 6-h time points were chosen to capture early responsive genes, and the 24-h time point for late responsive genes (Lee et al., 2005). Clones not exposed to abiotic stress were used as the control group. Three biological replicates were used for each treatment time point, including the control group. For physiological and gene expression analysis, fresh leaves were collected from the five groups, then immediately frozen in liquid nitrogen and stored at -80°C until analyzed.
Measurement of Physiological and Biochemical Characteristics
Endogenous H2O2 levels were detected by measuring luminol-dependent chemiluminescence according to the method described by Dat et al. (1998) and the H2O2-specific fluorescent probe H2DCF-DA (green; Molecular Probes, Eugene, OR, USA, prepared in a MES-KCl buffer, pH 5.7). The amount of malondialdehyde (MDA), and the activities of SOD and catalase (CAT) were measured by absorption photometry using a spectrophotometer. The details were as described by Giannopolitis and Ries (1977), Carrillo et al. (1992), and Song et al. (2013), respectively.
DNA and RNA Extraction
Plant materials were stored in liquid nitrogen and total genomic DNA was extracted using a DNeasy Plant Mini kit (Qiagen China, Shanghai), according to the manufacturer’s protocol. Total RNA was extracted using an RNeasy Plant Mini Kit (Qiagen, China, Shanghai) following the manufacturer’s protocol. Genomic DNA and RNA were measured with a Nano Vue UV/visible spectrophotometer (GE Healthcare Company) and stored at -80°C.
Methylation-sensitive Amplification Polymorphism (MSAP) Analysis
Methylation-sensitive amplification polymorphism analysis was carried out based on an established protocol (Sha et al., 2005; Peredo et al., 2006), and the isoschizomers Hpa II and Msp I were employed as frequent-cutter enzymes. During the selective PCR step, EcoR I and Hpa II/Msp I primers adding three additional selective nucleotides were used. The selective PCR products were resolved by electrophoresis on 6% sequencing gels and detected with silver staining (Tixier et al., 1997). The differentially amplified fragments represent stress-responsive differentially methylated regions.
The isolation of polymorphic bands was performed as described previously (Sha et al., 2005). Briefly, polymorphic fragments were excised from the gels, hydrated in 50 μl of water, and incubated at 42°C for 30 min. The eluted DNA was amplified with the same primer pairs and under the same conditions used for selective amplification. Sequence information was obtained by cloning the fragments into vector pMD18-T (Takara Bio, Inc., Tokyo, Japan), and three positive clones for each individual were selected for sequencing.
The methylation patterns of specific cytosine loci (5′-CCGG-3′) obtained by MSAP have four types: unknown, unmethylated, methylated at CG (mCG) and hemi-methylated at CNG (mCNG; Supplementary Figure S1). The relative methylation levels were calculated using the following equations: relative methylated level relative hemi-methylated level and relative total methylation level . In these formulas, mCG represents the number of MSAP markers with the mCG methylation pattern. Similarly, mCNG, unknown, and unmethylated represent the number of MSAP markers with mCNG, unknown, and unmethylated methylation patterns, respectively.
Bisulfite Sequencing of Candidate Differentially Methylated Sequences
For analysis of the candidate differentially methylated sequences (DMSs), genomic DNA was treated with bisulfite and used as template for amplification, which was carried out for 35 cycles. The assay primers span the region that contains the 5′-CCGG-3′ sites and are listed in Supplementary Table S3. PCR products were then purified using a Gel Extraction Kit (Qiagen, Hilden, Germany) and cloned into the vector pMD18-T (Takara Bio, Inc., Tokyo, Japan). 20 positive clones for each individual were selected for sequencing through an ABI sequencer (PRISM BigDye Terminator, ABI, Sunnyvale, CA, USA).
Annotation of miRNA and Target Genes
The DMSs were analyzed using miRBase1 to find the mapped miRNA genes and psRNATarget tools2 to map the target genes in the sequenced reference genome of P. trichocarpa. The annotation information for target genes was obtained from PopGenIE3 and pathway analysis of biological process from KEGG4.
Real-time Quantitative PCR of Mature miRNAs
Quantitative PCR analysis of miRNA was carried out following a high-stringency protocol where a poly A tail was added by using poly A polymerase. The Power SYBR Green PCR Master Mix (ABI) and the StepOne+ Real-Time PCR System (ABI) were used to perform quantitative PCR according to the standard protocol. The forward primers were designed based on miRNA sequences in miRBase 21.05. The reverse primer was designed based on the poly(T) adapter, which was always the same (5′-GTCGTATTAATTCTGTGCTCGC-3′). The internal reference gene was a 5.8S rRNA (forward primers: 5′-GTCTGCCTGGGTGTCACGCAA-3′; Supplementary Table S2).
Gene Expression Analysis by qRT-PCR
For quantitative PCR analysis, the TaKaRa ExTaq R PCR Kit, SYBR green dye (TaKaRa, Dalian, China) and a DNA Engine Opticon 2 machine (MJ Research) were used. Gene-specific primers were designed to target the 3′ UTR of each gene (Supplementary Table S1). A melting curve was used to check the specificity of each amplified fragment. For all reactions, triplicate technical and biological repetitions of each individual were performed; the PCR was performed according to Song et al. (2014). After amplification, the PCR products were sequenced to check the specificity of the primer sets. Relative expression levels of candidate genes were standardized to the transcript levels for PsiACTIN, which shows stable expression under abiotic stress calculated by the 2–ΔΔCt method.
Validation by Degradome Sequencing
We used the fastx toolkit to exclude low-quality reads from the degradome sequencing and to remove adapter sequences. The Cleaveland 2.0 software was used to further analyze the reads. Briefly, the P. trichocarpa transcripts database of JGI Phytozome V 7.0 was mapped with the reads. Next, the true miRNA cleavage site was distinguished from background noise with a target plot. We used the default parameters and performed 100 randomized sequence shuffles with Cleaveland 2.0. The cleaved targets were categorized into five categories using the following criteria: (1) the read abundance of the cleavage site had the maximum value on the transcript; (2) the read abundance of the cleavage site had the maximum value on the transcript but was not unique; (3) the read abundance of the cleavage site did not have the maximum value but was higher than the median on the transcript; (4) the read abundance of the cleavage site was equal to or less than the median on the transcript; and (5) only one raw read existed at the cleavage site. Only the targets that were verified by degradome sequencing were recorded in this study.
Statistical Analysis
Significance of differences in enzyme activities were determined with the Least Significant Difference test using SPSS 20 (Copyright IBM Corporation 1989). Differences were considered statistically significant when P < 0.05. Asterisks “∗” represent P < 0.05, “∗∗” represent P < 0.01 compared with control group.
Results
Changes of Physiological and Biological Parameters in Response to Temperature Stress
To evaluate the dynamic biological and physiological reactions that occur during temperature treatment, we treated 30-cm cuttings from 1-year-old branches at 42 or 4°C for 0, 3, 6, 12, and 24 h and measured four parameters of the cuttings: H2O2 contents, and activities of catalase (CAT), SOD, and malondialdehyde (MDA; Figure 1). In both heat and cold stress treatments, the activity of CAT and SOD reached a peak at 6 h; also, CAT and SOD activities were significantly higher under heat stress than cold at 6 h. In addition, the amounts of MDA and H2O2 significantly increased from 12 to 24 h in cold- and heat-treated plants (P < 0.01; Figure 1).
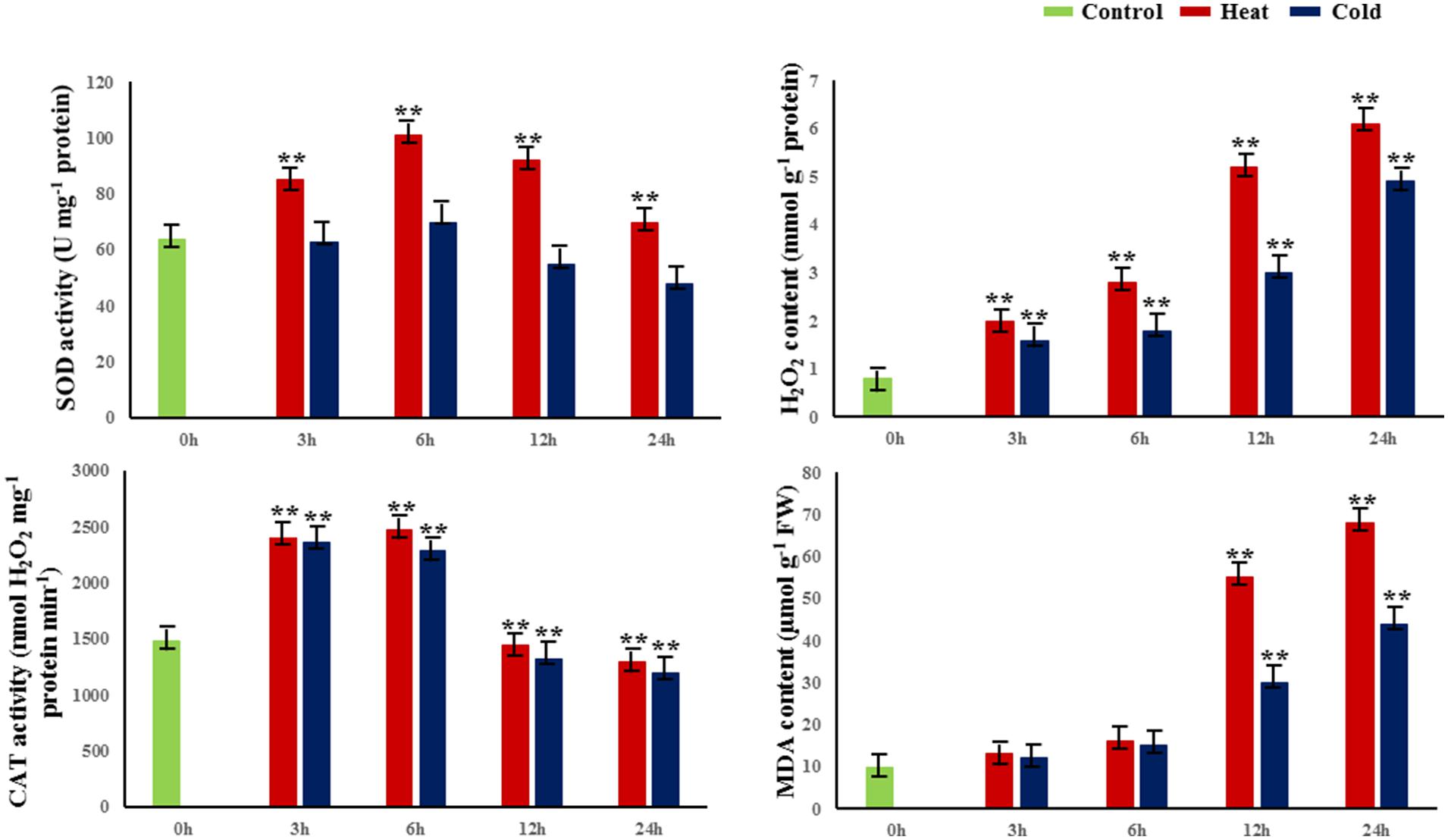
FIGURE 1. Changes in physiological and biochemical properties in response to abiotic stress. SOD superoxide dismutase, H2O2 hydrogen peroxide, MDA malondialdehyde, CAT catalase. 0 h indicates the control group without stress treatment. 3–24 h indicate different times of exposure to heat and cold stress. Error bars represent standard error. Activities are presented as mean ± SE, and n = 3. An asterisk indicates differences at ∗P < 0.05 compared with the control group, and two asterisks indicates significant differences at ∗∗P < 0.01 compared with the control group.
Relative Levels and Patterns of Cytosine Methylation under Temperature Stress
We used samples at 6 h of treatment to examine DNA methylation changes and gene expression responses underlying these treatments. MSAP analysis, which can reveal different methylation sites in the genome, was used to examine the genome-wide patterns of DNA methylation in response to temperature stress treatments. We used 240 primer combinations with 15 Hpa II/Msp I and 20 EcoR I primers (Excel S1) to detect the sites of DNA methylation at the 5′-CCGG-3′ sequence from P. simonii. In total, MSAP produced 4199 methylation bands, including 1066 polymorphic loci (25.39%; Figure 2A). Among these, 70.73 and 46.90% polymorphic loci were from cold stress and heat stress, respectively.
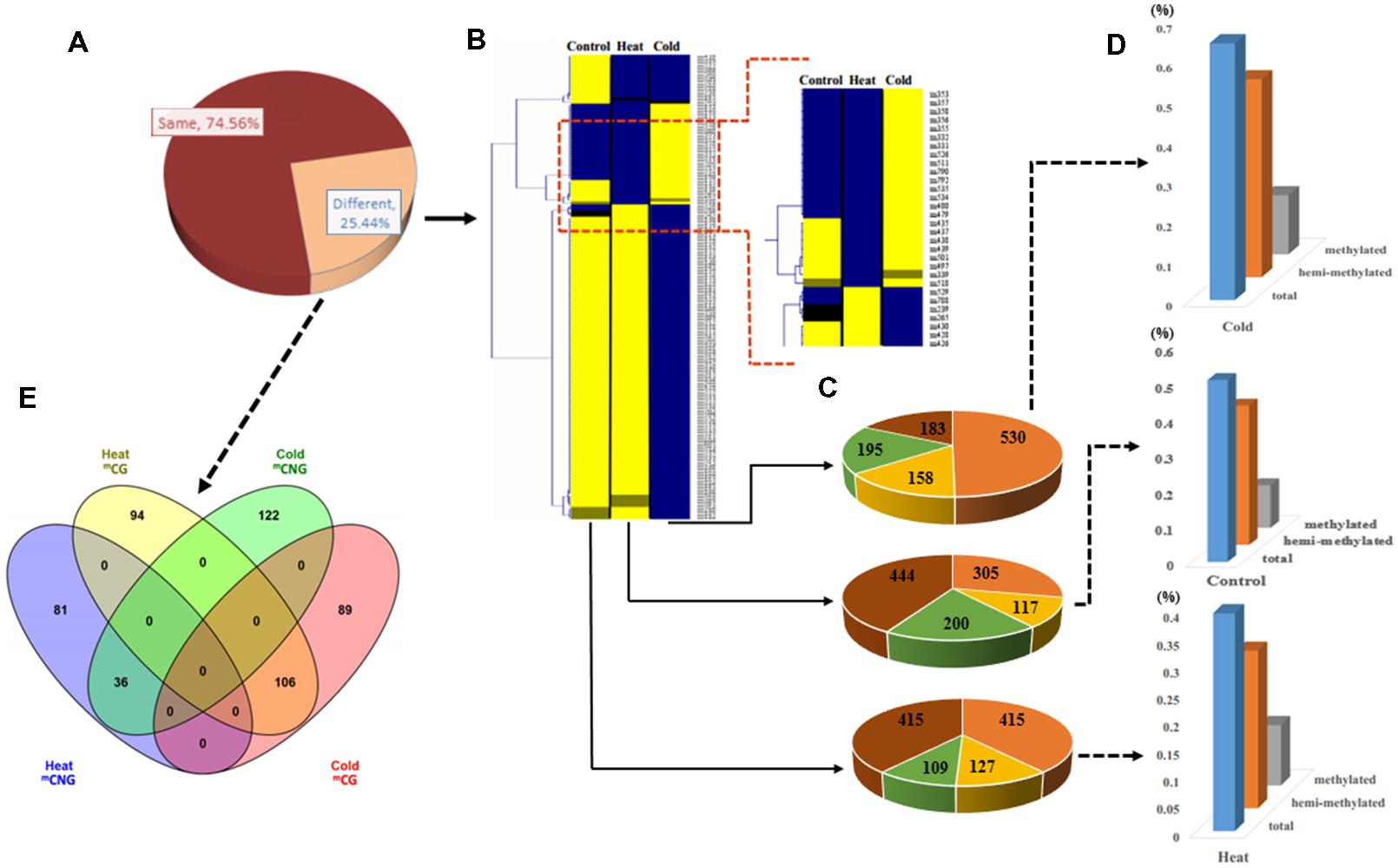
FIGURE 2. Analysis of methylation patterns and levels in Populus simonii under heat stress, cold stress, and in the control group. (A) The ratio of different MSAP band types: the dark red part represents MSAP bands with the same methylation pattern in heat, cold, and control groups; the orange part represents MSAP bands with different methylation patterns in heat, cold, and control groups. (B) Hierarchical clustering analysis of pattern of MSAP markers: represents unknown methylation pattern;
represents hemi-methylated pattern;
represents methylated pattern;
represents unmethylated pattern. (C) The number of four MSAP patterns:
represents unknown methylation pattern;
represents hemi-methylated pattern;
represents methylated pattern;
represents unmethylated pattern. (D) Relative methylation level, relative hemi-methylated level, and relative total methylation level (see Materials and Methods for calculations). (E) Venn diagram showing the number of heat stress and cold stress loci with stress-specific DNA methylation.
To estimate the relative methylation levels under different abiotic stresses, we next examined the methylation state (unknown, unmethylated, methylated at CG, or hemi-methylated at CNG) of the methylation bands (Supplementary Figure S1). Relative total methylation levels ranged from 39.59% (heat) to 64.54% (cold) and the relative total methylation level of the control group was 50.84%. The relative methylation level (mCG) was 10.98% in heat-treated plants, 14.82% in cold-treated plants, and 11.91% in control plants. In addition, the relative hemi-methylation level of P. simonii in different treatments was 28.61% (heat), 49.72% (cold), and 38.93% (control). These results showed that plants showed the highest relative methylation in all methylation patterns under cold stress treatment, and the lowest level under heat stress treatment (Figures 2B–D). Comparison of methylation band types under heat and cold stress treatments also detected differences in the cytosine methylation patterns under different abiotic stresses. This identified 175 heat-specific bands (94 mCG and 81 mCNG), 211 cold-specific methylated bands (89 mCG and 122 mCNG), and 142 bands present in both heat and cold (106 mCG and 36 mCNG; Figure 2E and Excel S1).
Identification of miRNA Genes Methylated in Response to Temperature Stress
Based on the polymorphisms observed in MSAP bands under different temperature stresses, we isolated and sequenced a subset of the stress-specific DMSs. We focused on two classes: Class I includes DMSs present in both heat and cold stresses, but not in the control group; Class II includes DMSs specific to heat or cold stress. From the 1066 differentially methylated bands, we isolated and sequenced 400 bands, including 150 stress-specific MSAP bands for each stress and 100 bands common to both stresses. After removing low-quality sequences and redundant fragments, we finally obtained 259 DMSs.
To identify miRNA genes potentially affected by methylation in response to temperature stress, the DMSs were annotated by BLAST searches against miRBase. This identified seven DMSs that mapped to conserved miRNA genes in the sequenced P. trichocarpa genome: Ptc-MIR156i, Ptc-MIR156j, Ptc-MIR167h, Ptc-MIR390c, Ptc-MIR393a, Ptc-MIR396e, and Ptc-MIR396g (Excel S2); these are homologs of the P. simonii miRNA genes. The psRNATarget algorithm predicted 111 targets of the miRNAs produced by these methylated miRNA genes (Excel S3) and gene ontology (GO) classification of these miRNA targets showed enrichment of hydrolase activity, acting on acid anhydrides, ribonucleotide binding, purine nucleotide binding, DNA binding, and purine nucleoside binding in molecular function GO terms (Supplementary Figure S3A). In biological process, regulation of cellular biosynthetic process, RNA metabolic process, regulations of macromolecule biosynthetic process, and regulation of gene expression were enriched (Supplementary Figure S3B).
Methylation of miRNA Genes in Response to Temperature Stress
Annotation analysis of all target genes based on their homologs in the sequenced reference genome of P. trichocarpa showed that of the three miRNA genes are located in genic regions. Examination of the local methylation patterns of these miRNA genes revealed that Ptc-MIR393a shows different cytosine methylation patterns among control group (mCNG), cold (mCG), and heat stress (mCNG) and is located in the 5′ untranslated regions (UTR) and first exon of Potri.008G062800, which has two exons and one intron. Also, Ptc-MIR396e is located in the 5′ UTR and first exon of Potri.018G127000, which has two exons and one intron and was unmethylated in the control group, mCG in cold-treated plants, and mCNG in heat-treated plants. Moreover, Ptc-MIR396g is located in the promoter of Potri.003G160700, which contains four exons and three introns, and was unmethylated in the control group, mCG in cold- and mCNG in heat-treated plants (Figure 3A and Table 1).
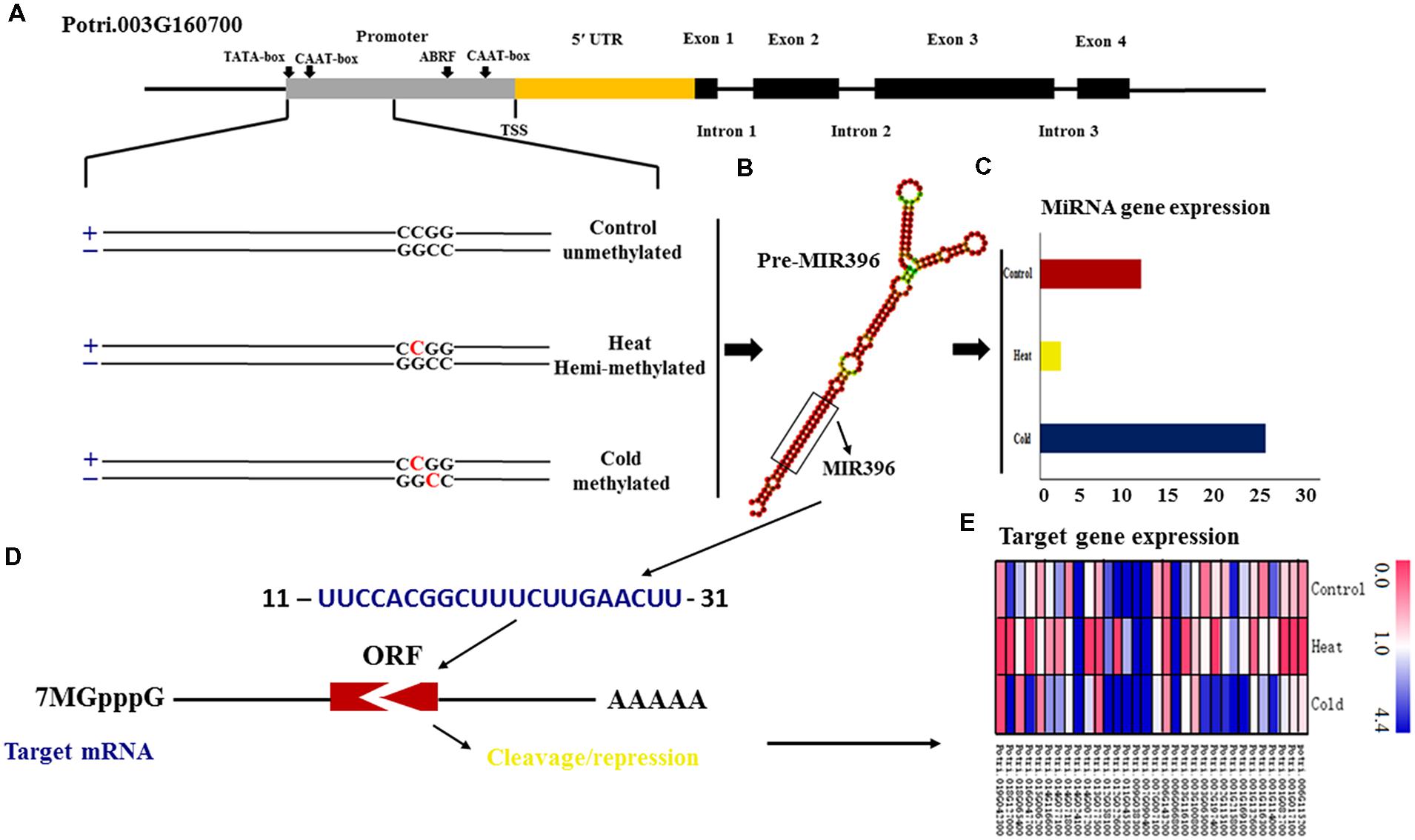
FIGURE 3. DNA methylation changes in miRNA genes in P. simonii under heat, cold and control conditions. (A) Schematic representation of the differentially methylated patterns of MIR396g, whose host gene is Potri.003G160700. Horizontal lines represent the gene sequence. The red C represents 5-methylcytosine and black C represents normal cytosine; (B) The secondary structure of the miRNA396g precursor. (C) The expression of the methylated Psi-MIR396g locus. (D) The interaction between miR396g and its target gene. (E) The expression of target genes regulated by miR396g in response to heat and cold, and in the control group.
The cytosine methylation sites cut by EcoR I and Hpa II/Msp I also are located in intergenic regions in Ptc-MIR156i, Ptc-MIR156j, Ptc-MIR167h, and Ptc-MIR390c. Therefore, we used these sites to assay methylation in these regions. The Ptc-MIR156i and Ptc-MIR156j loci were unmethylated in the control group, while Ptc-MIR156i had mCG in cold-treated plants, and mCNG in heat-treated plants, but Ptc-MIR156j had mCNG in cold- and heat-treated plants. In addition, Ptc-MIR167h was unmethylated in the control group, had mCG in cold-treated plants, and had mCNG in heat-treated plants. Also, Ptc-MIR390c was unmethylated in the control group and cold-treated plants, but had mCG in heat-treated plants (Table 1). Thus, these miRNA genes showed different patterns of methylation, but most of the miRNA genes (except for Ptc-MIR156j and Ptc-MIR390c) were methylated at CG sites under cold stress. By contrast, under heat stress, these miRNA genes (except for Ptc-MIR390c) were methylated at CNG sites.
To confirm the status of the methylation of DMSs, we performed bisulfite sequencing for each of the temperature stresses (treated for 6 h) and the control group. The methylation level of CHG on candidate DMSs ranged from 34.2 to 100.0% in the control, CHH ranged from 48.6 to 69.5%, and CG ranged from 51.3 to 90.6%. The methylation levels in the temperature treated samples are listed in Table 2, and the sequences contained less CG and CHG methylation than CHH methylation. In treated individuals, de novo methylation and demethylation occurred simultaneously (Excel S4 and Supplementary Figure S5), but methylation of these candidate sequences showed a decreasing trend, with the methylation under heat treatment declining more than under cold treatment, and the methylation level in mCNG context being lower than in mCG and unmethylated.
Expression of miRNA Genes in Response to Temperature Stress
To verify the possible relationship between DNA methylation pattern and expression of candidate miRNA genes, we used quantitative real-time PCR (qRT-PCR) to detect the expression levels of these miRNA genes in control and treated samples (Supplementary Table S2). Five miRNA genes (Ptc-MIR156i, Ptc-MIR167h, Ptc-MIR393a, Ptc-MIR396e, and Ptc-MIR396g) showed mCG modification under cold stress and mCNG modification under heat stress but were unmethylated in the control group (Table 1). The qRT-PCR analysis showed that the expression of miRNA genes with mCNG was significantly higher than the miRNA genes with mCG and also higher than the non-methylated genes under temperature stress. Ptc-MIR390c showed mCG under heat stress and was unmethylated in cold-treated and control groups and also showed lower expression when modified with mCG, compared with the unmethylated control (Table 1; Figures 3B,C). Thus, generally, under temperature stress, the candidate methylated miRNA genes that had mCNG showed higher expression than those that had mCG. However, miRNA genes with the same methylation pattern under heat and cold stresses showed different expression levels under these two conditions. For instance, MIR156j had the same methylation pattern (mCG) in both heat and cold stresses, but it showed higher expression under heat stress than under cold stress (Table 1).
Expression of Target Genes of miRNAs
After we measured the expression of the miRNA genes (Supplementary Table S1), we next measured the expression of their target genes to examine the regulatory relationship between the miRNAs and their targets. In total, we found 111 target genes, including 38 targets of Ptc-miR156i/j, 30 of Ptc-miR167h, 11 of Ptc-miR393a, and 32 of Ptc-miR396e/g. To detect whether the changes in miRNA gene expression affected target gene expression, we used qRT-PCR to measure the mRNA levels of the targets of these miRNA genes (Figure 3E and Excel S3). The results showed that the targets’ expression varied with the expression changes in the corresponding miRNA genes in response to heat and cold stresses. For example, Potri.001G294400 encoding a homolog of Arabidopsis VACUOLAR SORTING RECEPTOR 5 (VSR5), a target of Ptc-miR156i and j with mCG methylation modification, was downregulated in response to cold stress, when Ptc-MIR156i and j were upregulated. Potri.001G323100 encodes a homolog of Arabidopsis AUXIN SIGNALING F-BOX 2 (AFB2), is a target of Ptc-miR393a, showed mCNG, and was repressed under heat stress, in contrast to Ptc-MIR393a. In short, expression of the target genes decreased as the expression of the corresponding miRNA increased, consistent with the gene-silencing function of miRNAs (Figure 3D).
Regulatory Network of DNA Methylation in the Response to Temperature Stress
KEGG pathway analysis was used to characterize the functions of the target genes and indicated that target genes generally participate in lipid metabolism, including fatty acid degradation. For example, Potri.007G090400, a target of Ptc-miR396e and g, encodes a protein that functions in glycerophospholipid metabolism. Potri.014G042200, a target gene of Ptc-miR156i and j, encodes a protein involved in ether lipid metabolism. Other predicted products function in alpha-linolenic acid metabolism [ACYL-COA OXIDASE (ACOX1 and ACOX3)] and biosynthesis of unsaturated fatty acids [ACYL-COA OXIDASE (ACOX1 and ACOX3)], which all deplete lipid peroxide. Also, ISOCITRATE DEHYDROGENASE (IDH) participates in peroxisome biogenesis (a target gene of Ptc-miR390c; Biswas and Mano, 2015; Figure 4 and Excel S3). To validate the predicted target genes of miRNAs, degradome sequencing was performed, which confirmed LPCAT1/2, ACOX1/3, and IDH as targets of MIR156i/j, MIR396e/g, and MIR390c, respectively, in lipid metabolism or peroxidation (Supplementary Figure S4).
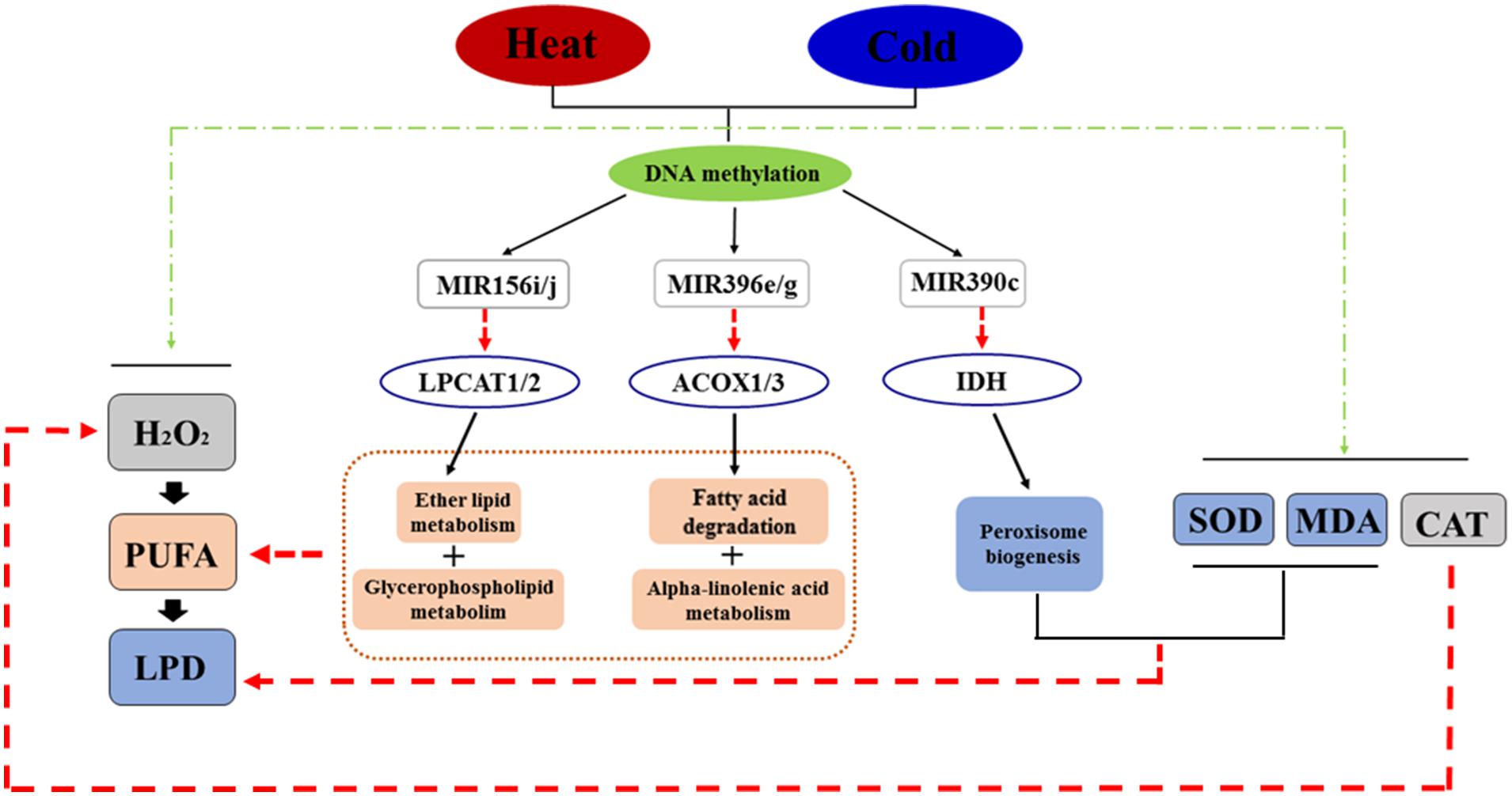
FIGURE 4. The regulatory network in response to temperature stress in P. simonii. Black line arrows represent regulatory effects (positive or negative regulation), red line arrows represent negative regulation, and green line arrows represent positive regulation.
Based on these pathways and the interactions between DNA methylation, miRNAs, and target genes, we constructed a regulatory network showing the changes of DNA methylation in response to temperature stress (Figure 4). In this network, temperature stress (heat and cold) induced production of H2O2, which produced more oxyradicals attacking polyunsaturated fatty acids, which induced lipid peroxidation to destroy cells. Moreover, heat and cold stresses induced SOD, MDA, and CAT activities to decrease superoxide, lipid peroxide, and H2O2 levels, respectively. Temperature stress also changed the methylation patterns of specific loci associated with miRNA genes responding to stress treatments, including Ptc-MIR156i and j, Ptc-MIR390c, and Ptc-MIR396e and g, which decrease the products of ACYL-COA OXIDASE (ACOX1 and ACOX3), PHOSPHOLIPID/GLYCEROL ACYLTRANSRASE FAMILY PROTEIN (LPCAT1 and LPCAT2), and ISOCITRATE DEHYDROGENASE (IDH) from Potri.007G090400, Potri. 014G042200, and Potri.010G176000, respectively (Excel S3). These products participate in lipid metabolism and peroxisome biogenesis; these reversible pathways can consume polyunsaturated fatty acids and lipid peroxides to prevent cell death (Figure 4).
Verification of MSAP Data
To make sure the MSAP data are reliable, we used methyl-sensitive PCR (MS-PCR) to confirm the data produced by MSAP. For this purpose, we design MS-PCR primers for 45 MSAP sequences for 15 stress-specific MSAP bands and 15 common MSAP bands in both heat and cold stress treatments (Supplementary Figure S2). The MS-PCR results indicate that MSAP is a stable, effective, and reproducible technology for detecting methylated sites that change in response to temperature stress in the genome of P. simonii.
Discussion
Catalase, SOD, and MDA are three important factors participating in general physiological and biochemical processes in plants. H2O2 produces oxyradicals that attack polyunsaturated fatty acids, thus inducing lipid peroxidation to destroy cells (Roach et al., 2015). CAT metabolizes H2O2 to prevent or reduce these harmful effects (Nie et al., 2015; Zhang et al., 2015), and provides a parameter allowing evaluation of the degree of physiological and biochemical effects of stress. The decomposition products of lipid peroxide induce cell damage and can be measured by the amount of MDA, which reflects the extent of lipid peroxidation and thus the extent of cell damage. Measurements of MDA and SOD complement each other, as the SOD activity indirectly reflects the oxygen free radical-scavenging ability of the cell, and the level of MDA indirectly reflects the severity of the effects of free radicals on the cell. In this study, we detected changes in CAT activity, SOD activity, MDA contents, and H2O2 contents in response to temperature stress. These results showed that the physiological reaction of poplar is the strongest after 6-h temperature stress treatment. So, we chose P. simonii after 6-h stress treatment as the experimental material for analysis of DNA methylation.
The Variation of DNA Methylation in Response to Heat and Cold Stress
In this study, we found that 25.38% of methylation sites changed in response to abiotic stress. This was consistent with the observations of Alonso et al. (2015), who examined 49 studies on different abiotic stresses in 18 species; in 78% of studies, the results agreed with the hypothesis that stress elicited changes in global DNA methylation. This suggests that DNA methylation might function in genomic regulation in response to abiotic stress. In addition, Tang et al. (2014) found that cytosine methylation at various loci decreased 10.28% due to drought exposure in Lolium perenne. Our results showed that 70.73 and 46.90% of differentially methylated loci responded to cold or heat stress, respectively, suggesting that methylated loci might respond differently to different abiotic stresses. The mCHG and mCHH modifications also showed site-specific methylation between male and female flowers, suggesting that different DNA methylation patterns might have different influences on flower development (Song et al., 2014). By contrast, in this study, we obtained 175 methylated bands (94 mCG and 81 mCNG) that were specific to heat treatment, and 211 methylated bands (89 mCG and 122 mCNG) that were specific to cold stress (Figure 2E and Excel S1), suggesting that methylation patterns might differ in the responses to heat and cold stress.
The Effect of Methylated miRNA Genes on the Expression of Target Genes
Different DNA methylation patterns have different effects on gene expression (Sunkar and Zhu, 2004). MIR164a associated with flower development showed significantly lower methylation levels in female flowers than in male flowers and induced expression in male flowers; by contrast, MIR164a was only methylated in male flowers and its CHH methylation level was higher than its CG and CHG levels (Song et al., 2014). These results indicated that the expression of miRNA genes might be regulated by their methylation level or pattern. Here, five candidate miRNA genes (MIR156i, MIR167h, MIR393a, MIR396e, and MIR396g) related to the response to temperature stress showed mCG modification and repressed expression under cold stress; these loci also showed mCNG modification and induced expression under heat stress, compared with the expression of miRNA genes that were unmethylated under control conditions (Table 1). This furthermore indicated that the different cytosine methylation patterns of MSAP markers probably associate with different expression levels of miRNA genes.
Moreover, the methylation levels under heat treatment declined more than under cold treatment, which might explain the observation that miRNA expression increased most in heat-treated samples. The CNG methylation was generally lower than the other methylation patterns (mCG and unmethylated), which indicated that the results of methylation sequencing support the results of the enzyme digestion. These findings suggest that in poplar, methylation regulates miRNA gene expression in the response to abiotic stress.
In addition, to detect the influence of these miRNA genes on their target genes, we used qRT-PCR to survey the transcript levels of target genes. The results showed that ACYL-COA OXIDASE 1 (ACOX1) and ACYL-COA OXIDASE 3 (ACOX3), targets of Ptc-miR396e and g, were induced in cold stress. The IDH gene, a target of Ptc-miR390c, was highly expressed in cold stress. Two targets of Ptc-miR156i and j, PHOSPHOLIPID/GLYCEROL ACYLTRANSFERASE FAMILY PROTEIN (LPCAT1 and LPCAT2), were repressed in heat stress (Excel S3). Degradome sequencing confirmed that these targets produce cleavage products consistent with regulation by the corresponding miRNAs. These variations of expression were consistent with the gene-silencing function of miRNAs, where miRNA levels negatively correlate with the transcript levels of their target genes. Our results suggested that the function of DNA methylation in response to temperature stress might be implemented by affecting expression of miRNAs and their targets.
The Regulatory Network of P. simonii in the Response to Abiotic Stress
Recent work showed that lipid peroxide-derived toxic carbonyl compounds mediate environment stress-induced damage of plants (Biswas and Mano, 2015), suggesting that lipid peroxide might also negatively affect plants in response to abiotic stress. Lipid peroxide derives from lipid peroxidation due to oxyradicals that attack polyunsaturated fatty acids when plants suffer temperature stress that induces oxidative stress. Abiotic stress signals result directly or indirectly from gene expression regulated by many factors, including DNA methylation (Rakei et al., 2015).
Here, we focused on the effect of DNA methylation on regulation of miRNAs as critical to changes in target gene expression to adapt to abiotic stress. Numerous genes related to oxidative stress play crucial roles in maintaining reactive oxygen species homeostasis and levels in organisms. In this study, we found Ptc-MIR156i and j, and Ptc-MIR396e and g affected genes related to lipid metabolism and depletion of polyunsaturated fatty acids preventing the production of lipid peroxide. However, miRNAs with different methylation patterns were differentially expressed under different stress treatments, which led to higher expression of ACOX1 and 3, and LPCAT1 and 2 under cold stress with mCG pattern than under heat stress with mCNG pattern. This pattern was reversed for IDH, which functions in peroxisome biogenesis directly reducing lipid peroxides. The ACOX gene encodes a peroxisomal acyl-CoA oxidase that is thought to catalyze the first reaction during biosynthesis of the fatty acid component of daumones (Joo et al., 2010). The transcript levels of ACOX did not show significant changes following oxidative stress induced by perfluorododecanoic acid (Liu et al., 2008). IDH is resistant to denaturation, which reduces its catalytic efficiency in high temperature regimes (Bergmann and Gregorius, 1993). Rzezniczak and Merritt (2012) found that IDH seem to be induced by oxidative stress in the enzyme network responsible for the reduction of nicotinamide adenine dinucleotide phosphate and further mentioned that biological network interactions were strongly influenced by environmental conditions. In our study, transcript levels of ACOX decreased 2.4-fold and IDH decreased over 5-fold in response to heat treatment (Excel S3); these changes of expression might relate to the variation in DNA methylation. Plant LPCAT enzymes are crucial in regulating the acyl-CoA composition of cells by transferring polyunsaturated and hydroxy fatty acids produced on phosphatidylcholine straight to the acyl-CoA pool for further metabolism or catabolism (Lager et al., 2013). Arabidopsis LPCATs were measured in the reverse reaction from which phosphatidylcholine was transferred to acyl-CoA to a similar extent. Here, LPCAT1/2 decreased 2.66-fold under heat treatment (Excel S3), while the methylation level of candidate sequences overlapping Ptc-MIR156i and Ptc-MIR156j declined (Table 2), suggesting that the variation of DNA methylation might suppress LPCAT correlated with reduced PC for fatty acid desaturation to protect cells from temperature stress. These results indicated that methylated miRNAs might play a key role in P. simonii under temperature stress. Our data also provide a series of candidate miRNA genes for research into epigenetic regulation of abiotic stress responses.
Author Contributions
DZ conceived and designed the experiment. DC performed the DNA and RNA extractions and performed MSAP analyses and drafted the manuscript. YS carried out the gene expression analysis. MT participated in the statistical analyses. All authors read and approved the final manuscript.
Conflict of Interest Statement
The authors declare that the research was conducted in the absence of any commercial or financial relationships that could be construed as a potential conflict of interest.
Acknowledgments
This work was supported by grants from the following sources: the Forestry Public Benefit Research Program (No. 201204306), the Specialized Research Fund for the Doctoral Program of Higher Education (No. 20110014110011), and Project of the National Natural Science Foundation of China (No. 31170622, 30872042).
Supplementary Material
The Supplementary Material for this article can be found online at: http://journal.frontiersin.org/article/10.3389/fpls.2015.00921
Footnotes
- ^ http://www.mirbase.org/search.shtml
- ^ http://plantgrn.noble.org/psRNATarget
- ^ http://popgenie.org
- ^ http://www.kegg.jp/kegg/tool/map_pathway2.html
- ^ http://www.mirbase.org/
References
Alonso, C., Pérez, R., Bazaga, P., Medrano, M., and Herrera, C. M. (2015). MSAP markers and global cytosine methylation in plants: a literature survey and comparative analysis for a wild-growing species. Mol. Ecol. Resour. doi: 10.1111/1755-0998.12426 [Epub ahead of print].
Bergmann, F., and Gregorius, H. R. (1993). Ecogeographical distribution and thermostability of isocitrate dehydrogenase (IDH) alloenzymes and European silver fir (Abies alba). Biochem. Syst. Ecol. 21, 597–605. doi: 10.1016/0305-1978(93)90059-Z
Biswas, M. S., and Mano, J. I. (2015). Lipid peroxide-derived short-chain carbonyls mediate H2O2-induced and NaCl-induced programmed cell death in plants. Plant Physiol. 168, 885–898. doi: 10.1104/pp.115.256834
Carrillo, M. C., Kanai, S., Sato, Y., and Kitani, K. (1992). Age-related changes in antioxidant enzyme activities are region and organ, as well as sex, selective in the rat. Mech. Ageing Dev. 65, 187–198. doi: 10.1016/0047-6374(92)90035-C
Centomani, I., Sgobba, A., D’Addabbo, P., Dipierro, N., Paradiso, A., De Gara, L., et al. (2015). Involvement of DNA methylation in the control of cell growth during heat stress in tobacco BY-2 cells. Protoplasma doi: 10.1007/s00709-015-0772-y [Epub ahead of print].
Dat, J. F., Lopez-Delgado, H., Foyer, C. H., and Scott, I. M. (1998). Parallel changes in H2O2 and catalase during thermotolerance induced by salicylic acid or heat acclimation in mustard seedlings. Plant Physiol. 116, 1351–1357. doi: 10.1104/pp.116.4.1351
Giannopolitis, C. N., and Ries, S. K. (1977). Superoxide dismutases I. Occurrence in higher plants. Plant Physiol. 59, 309–314.
Hajdarpašić, A., and Ruggenthaler, P. (2012). Analysis of miRNA expression under stress in Arabidopsis thaliana. Bosn. J. Basic Med. Sci. 12, 169–176.
Ishikawa, K. I., Ishikawa, A., Shoji, Y., and Imai, T. (2014). A genotoxic stress-responsive miRNA, miR-574-3p, delays cell growth by suppressing the enhancer of rudimentary homolog gene in vitro. Int. J. Mol. Sci. 15, 2971–2990. doi: 10.3390/ijms15022971
Joo, H. J., Kim, K. Y., Yim, Y. H., Jin, Y. X., Kim, H., Kim, M. Y., et al. (2010). Contribution of the peroxisomal acox gene to the dynamic balance of daumone production in Caenorhabditis elegans. J. Biol. Chem. 285, 29319–29325. doi: 10.1074/jbc.M110.122663
Lager, I., Yilmaz, J. L., Zhou, X. R., Jasieniecka, K., Kazachkov, M., Wang, P., et al. (2013). Plant acyl-CoA: lysophosphatidylcholine acyltransferases (LPCATs) have different specificities in their forward and reverse reactions. J. Biol. Chem. 288, 36902–36914. doi: 10.1074/jbc.M113.521815
Lee, B., Henderson, D. A., and Zhu, J. K. (2005). The Arabidopsis cold-responsive transcriptome and its regulation by ICE1. Plant Cell 17, 3155–3175. doi: 10.1105/tpc.105.035568
Li, D., Zhao, Y., Liu, C., Chen, X., Qi, Y., Jiang, Y., et al. (2011). Analysis of MiR-195 and MiR-497 expression, regulation and role in breast cancer. Clin. Cancer Res. 17, 1722–1730. doi: 10.1158/1078-0432.CCR-10-1800
Liu, Y., Wang, J., Wei, Y., Zhang, H., Xu, M., and Dai, J. (2008). Induction of time-dependent oxidative stress and related transcriptional effects of perfluorododecanoic acid in zebrafish liver. Aquat. Toxicol. 89, 242–250. doi: 10.1016/j.aquatox.2008.07.009
Marsh, A. G., and Pasqualone, A. A. (2014). DNA methylation and temperature stress in an Antarctic polychaete, Spiophanes tcherniai. Front. Physiol. 5:173. doi: 10.3389/fphys.2014.00173
Nie, Q., Gao, G. L., Fan, Q. J., Qiao, G., Wen, X. P., Liu, T., et al. (2015). Isolation and characterization of a catalase gene “HuCAT3” from pitaya (Hylocereus undatus) and its expression under abiotic stress. Gene 563, 63–71. doi: 10.1016/j.gene.2015.03.007
Peredo, E. L., Revilla, M. A., and Arroyo-Garcyá, R. (2006). Assessment of genetic and epigenetic variation in hop plants regenerated from sequential subcultures of organogenic calli. J. Plant Physiol. 163, 1071–1079. doi: 10.1016/j.jplph.2005.09.010
Rakei, A., Maali-Amiri, R., Zeinali, H., and Ranjbar, M. (2015). DNA methylation and physio-biochemical analysis of chickpea in response to cold stress. Protoplasma [Epub ahead of print].
Roach, T., Colville, L., Beckett, R. P., Minibayeva, F. V., Havaux, M., and Kranner, I. (2015). A proposed interplay between peroxidase, amine oxidase and lipoxygenase in the wounding-induced oxidative burst in Pisum sativum seedlings. Phytochemistry 112, 130–138. doi: 10.1016/j.phytochem.2014.06.003
Rykov, S. V., Khodyrev, D. S., Pronina, I. V., Kazubskaya, T. P., Loginov, V. I., and Braga, E. A. (2013). Novel miRNA genes methylated in lung tumors. Russ. J. Gen. 49, 782–786. doi: 10.1134/S1022795413070119
Rzezniczak, T. Z., and Merritt, T. J. (2012). Interactions of NADP-reducing enzymes across varying environmental conditions: a model of biological complexity. G3 (Bethesda). 2, 1613–1623. doi: 10.1534/g3.112.003715
Sha, A. H., Lin, X. H., Huang, J. B., and Zhang, D. P. (2005). Analysis of DNA methylation related to rice adult plant resistance to bacterial blight based on methylation-sensitive AFLP (MSAP) analysis. Mol. Genet. Genomics 273, 484–490. doi: 10.1007/s00438-005-1148-3
Song, Y. P., Ci, D., Tian, M., and Zhang, D. Q. (2014). Comparison of the physiological effects and transcriptome responses of Populus simonii under different abiotic stresses. Plant Mol. Biol. 86, 139–156. doi: 10.1007/s11103-014-0218-5
Song, Y. P., Ma, K. F., Ci, D., Chen, Q. Q., Tian, J. X., and Zhang, D. Q. (2013). Sexual dimorphic floral development in dioecious plants revealed by transcriptome, phytohormone, and DNA methylation analysis in Populus tomentosa. Plant Mol. Biol. 83, 559–576. doi: 10.1007/s11103-013-0108-2
Sunkar, R., and Zhu, J. K. (2004). Novel and stress-regulated microRNAs and other small RNAs from Arabidopsis. Plant Cell 16, 2001–2019. doi: 10.1105/tpc.104.022830
Tang, X. M., Tao, X., Wang, Y., Ma, D. W., Li, D., Yang, H., et al. (2014). Analysis of DNA methylation of perennial ryegrass under drought using the methylation-sensitive amplification polymorphism (MSAP) technique. Mol. Genet. Genomics 289, 1075–1084. doi: 10.1007/s00438-014-0869-6
Tixier, M. H., Sourdille, P., Röder, M., Leroy, P., and Bernard, M. (1997). Detection of wheat microsatellites using a non radioactive silver-nitrate staining method. J. Gen. Breed. 51, 175–178.
Wang, L., Wang, B. L., Wei, Z. Z., Du, Q. Z., Zhang, D. Q., and Li, B. L. (2012). Development of 35 microsatellite markers from heat stress transcription factors in Populus simonii (Salicaceae). Am. J. Bot. 99, e357–e361. doi: 10.3732/ajb.1200056
You, J., Zong, W., Hu, H. H., Li, X. H., Xiao, J. H., and Xiong, L. Z. (2014). A STRESS-RESPONSIVE NAC1-regulated protein phosphatase gene rice protein phosphatase18 modulates drought and oxidative stress tolerance through abscisic acid-independent reactive oxygen species scavenging in rice. Plant Physiol. 166, 2100–2114. doi: 10.1104/pp.114.251116
Zhang, D., Ren, L., Chen, G. Q., Zhang, J., Reed, B. M., and Shen, X. H. (2015). ROS-induced oxidative stress and apoptosis-like event directly affect the cell viability of cryopreserved embryogenic callus in Agapanthus praecox. Plant Cell Rep. 34, 1499–1513. doi: 10.1007/s00299-015-1802-0
Keywords: DNA methylation, lipid metabolism, miRNA, Populus simonii, temperature stress
Citation: Ci D, Song Y, Tian M and Zhang D (2015) Methylation of miRNA genes in the response to temperature stress in Populus simonii. Front. Plant Sci. 6:921. doi: 10.3389/fpls.2015.00921
Received: 29 July 2015; Accepted: 12 October 2015;
Published: 30 October 2015.
Edited by:
Mahmoud W. Yaish, Sultan Qaboos University, OmanReviewed by:
Qing Liu, Commonwealth Scientific and Industrial Research Organisation, AustraliaMayumi Iwasaki, University of Cambridge, UK
Copyright © 2015 Ci, Song, Tian and Zhang. This is an open-access article distributed under the terms of the Creative Commons Attribution License (CC BY). The use, distribution or reproduction in other forums is permitted, provided the original author(s) or licensor are credited and that the original publication in this journal is cited, in accordance with accepted academic practice. No use, distribution or reproduction is permitted which does not comply with these terms.
*Correspondence: Deqiang Zhang, ZGVxaWFuZ3poYW5nQGJqZnUuZWR1LmNu