- 1Department of Molecular Plant Physiology, Institute for Water and Wetland Research, Radboud University, Nijmegen, Netherlands
- 2Department of Horticulture, Forestry and Recreation Resources, Kansas State University, Manhattan, KS, USA
High temperature has become a global concern because it seriously affects the growth and reproduction of plants. Exposure of plant cells to high temperatures result in cellular damage and can even lead to cell death. Part of the damage can be ascribed to the action of reactive oxygen species (ROS), which accumulate during abiotic stresses such as heat stress. ROS are toxic and can modify other biomacromolecules including membrane lipids, DNA, and proteins. In order to protect the cells, ROS scavenging is essential. In contrast with their inherent harms, ROS also function as signaling molecules, inducing stress tolerance mechanisms. This review examines the evidence for crosstalk between the classical heat stress response, which consists of heat shock factors (HSFs) and heat shock proteins (HSPs), with the ROS network at multiple levels in the heat response process. Heat stimulates HSF activity directly, but also indirectly via ROS. HSFs in turn stimulate the expression of HSP chaperones and also affect ROS scavenger gene expression. In the short term, HSFs repress expression of superoxide dismutase scavenger genes via induction of miRNA398, while they also activate scavenger gene expression and stabilize scavenger protein activity via HSP induction. We propose that these contrasting effects allow for the boosting of the heat stress response at the very onset of the stress, while preventing subsequent oxidative damage. The described model on HSFs, HSPs, ROS, and ROS scavenger interactions seems applicable to responses to stresses other than heat and may explain the phenomenon of crossacclimation.
The Heat Response
Plants are continuously exposed to biotic and abiotic stress factors, such as herbivory, pathogen attack, drought, salinity and extreme temperatures. These challenges pose a serious threat to their growth and reproduction and as such affect agricultural yields. With considerable advances in pest and disease management, abiotic factors are now thought to be the primary cause for crop losses worldwide (Wang et al., 2003; Suzuki et al., 2014). In case plants cannot prevent an abiotic stress factor from affecting organismal homeostasis (i.e., escape or avoid internal stress), they may adapt their metabolism to acquire a certain level of tolerance (Larkindale and Knight, 2002; Valliyodan and Nguyen, 2006; Munns and Tester, 2008; Krasensky and Jonak, 2012).
Heat stress can be defined as a rise in temperature beyond a threshold level for a period of time, sufficient to cause irreversible damage to plant growth and development (Wahid et al., 2007). Sudden rises in temperature to high levels may lead to cell death within a few minutes as a consequence of extensive protein denaturation and aggregation and loss of membrane integrity (Schöffl et al., 1999; Wahid et al., 2007). Furthermore, prolonged exposure to moderately high temperatures can lead to reduced cellular function and overall plant fitness (Bokszczanin et al., 2013). An important process in this respect is the accumulation of reactive oxygen species (ROS), formed as a by-product in various aerobic metabolic pathways in different cellular compartments such as chloroplasts, mitochondria and peroxisomes (del Rio et al., 2006; Navrot et al., 2007) and probably also in the apoplast through the activation of NADPH oxidases (Gechev and Hille, 2005; Torres and Dangl, 2005; Miller et al., 2009; Wang et al., 2014a). Under steady state conditions, ROS molecules are formed as quickly as they are scavenged by anti-oxidative defense mechanisms, but this equilibrium is perturbed by abiotic stress factors such as heat (Foyer and Noctor, 2005). There is ample evidence that, when plants are exposed to heat, ROS production rapidly becomes excessive (Morgan et al., 1986; Dat et al., 1998; Vacca et al., 2004; Volkov et al., 2006; Bhattacharjee, 2012, 2013; Chou et al., 2012; Hasanuzzaman et al., 2012, 2013; Wu et al., 2012; Hossain et al., 2013; Das and Roychoudhury, 2014; Mostofa et al., 2014). This causes cellular damage to membranes, proteins, lipids, organelles, and DNA (Baker and Orlandi, 1995; O’Kane et al., 1996; Giardi et al., 1997; Larkindale and Knight, 2002; Volkov et al., 2006; Wu et al., 2012; Bokszczanin et al., 2013). In order to prevent cell damage and regain redox homeostasis, one of the responses to heat is the hyper-activation of the ROS scavenging machinery. The expression and protein level of genes responsible for ROS scavenging are induced under heat stress in many different plant species (Chao et al., 2009; Chou et al., 2012; Mittal et al., 2012; Suzuki et al., 2013) and has been associated to basal heat tolerance (Rui et al., 1990; Badiani et al., 1993; Gupta et al., 1993; Sairam et al., 2000; Almeselmani et al., 2006; Kang et al., 2009; Bhattacharjee, 2012; Wang et al., 2014c). Furthermore, the induction of scavenging genes was significantly stronger in heat tolerant genotypes than that of sensitive ones (Rainwater et al., 1996), and improvement of plant heat stress tolerance has been achieved by increasing antioxidant enzymes activities (Rui et al., 1990; Badiani et al., 1993; Gupta et al., 1993; Sairam et al., 2000; Almeselmani et al., 2006; Wu et al., 2012; Chen et al., 2013). Taken together, this shows the importance of ROS scavenging in the heat-stress response.
In contrast to their harmful character, however, ROS are also considered as important signal molecules. Cells are capable of rapid and dynamic production and control of several forms of ROS, enabling a tight local control in the cell as well as more holistic control of the entire plant (Vranová et al., 2002; Mittler et al., 2011; Petrov and Van Breusegem, 2012). Therefore, they are thought to be involved in the transduction of intracellular and intercellular signals controlling gene expression and activity of anti-stress systems (Desikan et al., 2001, 2004; Apel and Hirt, 2004; Foyer and Noctor, 2005; Torres and Dangl, 2005; Miller et al., 2009; Galvez-Valdivieso and Mullineaux, 2010; Mittler et al., 2011; Kreslavski et al., 2012). Indeed, NADPH oxidase activity is rapidly induced upon heat (Miller et al., 2009) and the mutation of RBOHB makes Arabidopsis seedlings more sensitive to heat (Larkindale et al., 2005; Wang et al., 2014a).
One of the best studied anti-stress mechanisms is the production of heat shock proteins (HSPs) upon exposure to high temperatures (Wang et al., 2004). By acting as molecular chaperones, HSPs prevent deleterious protein conformations and eliminate non-native aggregations, which are formed during stress (Vierling, 1991; Boston et al., 1996; Morimoto, 1998). The expression of HSPs and other heat-responsive genes is regulated by heat shock factors (HSFs; Kotak et al., 2007) through their association to a palindromic binding motif (5′-nAGAAnnTTCTn-3′) in the promoter region of the heat-responsive genes: the heat shock element (HSE; Pelham, 1982; Scharf et al., 2012). Activation of HSFs upon stress occurs via a multistep process involving homotrimer formation and acquisition of transcriptional competence for target gene induction (Liu et al., 2013).
Clearly, both the activation and production of HSFs/HSPs and the increase in ROS/scavenging activity belong to the major responses of plants to heat stress and play important roles in acclimation. A number of recent genetic and biochemical studies, however, indicate that there are complex interactions between these responses. This review describes the evidence for crosstalk between HSFs, HSPs, ROS, and ROS scavenging enzymes at various points in the heat stress response pathway and presents a model with a timing component.
Activation of HSFs by ROS
In non-stressed situations, the HSFs are located in the cytoplasm for most eukaryotes, in an inactive monomeric form due to association with HSP70, HSP90, and potentially other proteins (Morimoto, 1998; Schöffl et al., 1998). According to the chaperone titration model, heat results in a higher load of denatured proteins, which pulls HSPs away from HSF complexes through competitions to act as molecular chaperones. This then leads to the release of HSFs, which form trimers and relocate to the nucleus to activate expression of HSP and other heat-responsive genes (Zou et al., 1998; Volkov et al., 2006).
A number of studies, however, report that expression of heat-responsive genes is also increased upon application of the ROS H2O2 (Uchida et al., 2002; Wahid et al., 2007; Banti et al., 2008). For example, AtHSP17.6 and AtHSP18.6 achieved similar expression levels through heat treatment as they do through H2O2 application at room temperature (Volkov et al., 2006). Several hypotheses have been formulated that suggest that heat can indirectly activate HSFs via the action of ROS.
Firstly, damaging amounts of heat-induced ROS also induce protein denaturation. In this way ROS enhances dissociation of the HSP–HSF complex, as described by the titration model (Schöffl et al., 1998). Secondly, and similar to what was found for mammalian and Drosophila HSFs, it has been proposed that certain plant HSFs act as H2O2 sensors (Ahn and Thiele, 2003; Miller and Mittler, 2006). Among all the ROS molecules, H2O2 plays a key role in signaling due to its moderate reactivity and thus relatively long lifetime (Vranová et al., 2002). In addition, H2O2 can bypass membranes easily, making it a good candidate to function as a signaling molecule (Petrov and Van Breusegem, 2012). Miller and Mittler (2006) suggested that H2O2 might directly modify HSFs and induce HSF trimerization. Indeed, both heat and oxidative stresses result in the formation of high molecular weight HSE-binding complexes and the formation of these complexes has been shown to be a signature of early HSFA1a/A1b-dependent gene expression in heat-stressed leaves of Arabidopsis (Lohmann et al., 2004; Volkov et al., 2006). In vitro and in vivo studies confirmed activation of AtHSFA1a via trimerization in response to heat and H2O2 stress but also via pH alterations (Liu et al., 2013). HSFA1a, purified from E. coli, sensed the different stresses directly in a redox dependent fashion. In vitro stress treatments caused monomer-to-trimer transitions of HSFA1a, while the presence of the reducing agent dithiothreitol reversed this action. Although the study suggested a redox dependent fashion for HSF trimerization for all three stresses, the exact mechanism of action is still unclear. There is empirical evidence that the transcription factors may be sensitive to H2O2 via “single-Cys” or “two-Cys” redox sensory mechanisms (Mittler et al., 2011). These cysteine residues are typically responsive to oxidative stress. HSFA1a contains one Cys residue located at the N-terminal portion of the trimerization domain (Hübel and Schöffl, 1994). N-terminal deletions of HSFA1a negatively affected the sensing of H2O2 and pH changes, which suggests that trimerizations were induced by HSF conformational changes (Liu et al., 2013). In addition, Giesguth et al. (2015) recently showed that an HSFA8 Cys residue is responsible for translocation to the nucleus upon oxidative stress: H2O2 treated protoplasts showed cytosol-to-nucleus translocations of the wild-type HSFA8, but not of the HSFA8C24S mutant variant (Giesguth et al., 2015). Interestingly, however, the N-terminal deletion of HSFA1a did not inhibit heat sensing. This shows that activation of this particular transcription factor is stress-specifically regulated despite a common dependency on oxidative activity (Mittler et al., 2011). Notably, all stress treatments induced of HSFA1a binding to the HSP18.2 and HSP70 promoter, as detected by both formaldehyde cross-linking and chromatin immunoprecipitation, which paralleled the mRNA expression of these HSFA1a target genes (Volkov et al., 2006; Liu et al., 2013).
In addition to the above two processes, cellular communication between ROS and HSFs may involve mitogen-activated protein kinases (MAPK). HSF phosphorylation has been observed in yeasts and mammals (Chu et al., 1996; Knauf et al., 1996; Kim et al., 1999) and might thus occur in plants as well (Link et al., 2002). Indeed, Arabidopsis HSFA2 was found to be phosphorylated by MPK6 on T249 after heat treatment, and this was associated with subsequent intracellular localization changes (Evrard et al., 2013). Furthermore, MPK3- and MPK6-dependent phosphorylation of AtHSFA4A Ser309 and physical interaction between the proteins was reported recently (Pérez-Salamó et al., 2014). Activated HSFA4A in turn controlled the transcription of HSP17.6A (Pérez-Salamó et al., 2014). In tomato, heat-induced MAPKs were shown to transduce heat stress signals via HSFA3 (Link et al., 2002). In Arabidopsis, the same MAPKs that phosphorylate HSFs, namely MAPK3 and MAPK6, have been shown to be activated by H2O2 (Kovtun et al., 2000; Moon et al., 2003; Rentel et al., 2004). However, despite the presence of putative phosphorylation sites in tomato HSFA1, no heat-induced phosphorylation of this HSF was observed. Also, the phosphorylation site in AtHSFA4 was not conserved in HSFA4A proteins of citrus, grapevine and poplar (Pérez-Salamó et al., 2014). Taken together, this implies that both HSF oxidation and ROS-dependent phosphorylation can play a role in HSF activation, but that the latter is not a general signaling mechanism.
HSF–ROS Scavenging Gene Interactions
In addition to activation of HSFs by ROS signaling, evidence for interaction between HSFs and ROS scavenging genes has also been obtained. The expression of APX1 was found to be regulated by HSFA2: overexpression of HSFA2 resulted in increased expression of APX1, while AthsfA2 knock out mutants showed a reduced expression of APX1 (Li et al., 2005). In agreement with this, AtHSFA2 overexpression lines showed increased heat and oxidative stress tolerance (Li et al., 2005). Expression of a dominant-negative construct for AtHSFA4a prevented the accumulation of APX1 transcripts (Pnueli et al., 2003; Apel and Hirt, 2004; Mittler et al., 2004; Davletova et al., 2005). Interestingly, the AtHSFA4a dominant-negative construct also prevented accumulation of the H2O2-responsive zinc-finger protein ZAT12, which is required for APX1 expression during oxidative stress. The ZAT12 promoter contains HSE binding sites (Rizhsky et al., 2004) and therefore, HSFA4a might directly interact with the ZAT12 promoter (Davletova et al., 2005). However, HSEs are also present in the promoter region of the APX1 gene itself, suggesting that direct activation via HSFs is also possible (Storozhenko et al., 1998; Panchuk et al., 2002). Using Pennisetum glaucum APX1 and a PgHSFA, a specific binding interaction between the APX1 HSE and HSF was confirmed, via in vitro gel shift assays as well as their expression patterns over time (Reddy et al., 2009).
Although APX1 has been shown to be a central component of the Arabidopsis ROS network (Davletova et al., 2005), APX2, another isoform also localized in the cytosol, revealed a stronger induction by heat stress (Panchuk et al., 2002). AtHSFA2 has also been found to act as an APX2 activator (Schramm et al., 2006; Nishizawa et al., 2008). Transcription level comparison between wild-type and athsfa2 knock out plants revealed that transcripts of APX2 were absent in heat shock induced leaves of the knock out background, but present in the wild-type plants (Schramm et al., 2006). Deletion analyses of the promoter region of APX2 functionally mapped the HSFA2 binding sites to HSEs near the transcription start site (Schramm et al., 2006).
In addition, Nishizawa et al. (2006) and Banti et al. (2010) found strongly enhanced expression of galactinol synthase (GolS1 and GolS2) ROS scavenging genes in an HSFA2 overexpressing line.
Combining these results, HSFA2 seems to play a central role in ROS scavenger expression and thus constitute an important link between heat shock and oxidative stress responses.
HSP Chaperones Support ROS Scavenging Activity
Heat shock proteins function as molecular chaperones and play an important role in stress tolerance. In tomato, overexpression of the LeCDJ1 DnaJ protein coding gene (also known as J-protein or HSP40; Qiu et al., 2006) resulted in improved thermotolerance, accompanied by increased APX and superoxide dismutase (SOD) activity after heat stress and reduced accumulation of O2– and H2O2. Despite the higher APX and SOD activity, transcription of the corresponding genes was not enhanced in the transgenic plants. Therefore, the influence of DnaJ proteins on APX and SOD activity was proposed to be post-transcriptional, due to their functionality as chaperones. Other studies have found similar effects of HSPs on ROS scavenging proteins upon heat stress. In Arabidopsis, overexpression of RcHSP17.8 enhanced SOD activity (Jiang et al., 2009) whereas overexpression of ZmHSP16.9 in tobacco enhanced POD, CAT, and SOD activity (Sun et al., 2012). Altogether, it may be hypothesized that the HSP proteins positively affect thermotolerance by protecting ROS scavenging protein conformation and activity, resulting in a lower ROS concentration (Kong et al., 2014a).
An alternative link between DnaJ proteins and ROS scavenging was suggested by Zhou et al. (2012). They showed that Arabidopsis AtDjB1 knockout plants (atj1-1) were more sensitive to heat stress than wild-type plants. After heat shock, the knockout plants showed an increased concentration of H2O2 and other oxidative products as well as a decreased concentration of the antioxidant ascorbate (ASC; Mittler et al., 2004; Zhou et al., 2012). The viability of atj1-1 knockout seedlings after heat stress was rescued by exogenous ASC application. This suggests that lower concentrations of the antioxidant in atj1-1 knockout plants resulted in increased H2O2 concentrations leading to a decreased thermotolerance (Zhou et al., 2012). As the underlying cause, the authors hypothesize a link with the electron transport chain (ETC). AtDjB1 directly interacts with a mitochondrial HSP70 and stimulates ATPase activity (Zhou et al., 2012), a mechanism which is conserved among several kingdoms (Qiu et al., 2006). AtDjB1 knockout potentially leads to the accumulation of cellular ATP, which feedback inhibits ETC. Because the last step of ASC synthesis is linked to the ETC (Bartoli et al., 2000), decreased ETC results in decreased ASC concentration and, consequently, the accumulation of H2O2 (Zhou et al., 2012).
Although it is unclear whether there is a specific interaction between HSPs and the ROS scavenging machinery or that HSPs generally maintain protein functions, these results indicate that upon heat stress, accumulation of ROS is reduced via HSP supported ROS scavenger activity.
A Positive Feedback Loop Including HSFs, ROS Scavenging Genes, and miRNA398
In contrast to the positive effects of ROS reducing mechanisms on heat stress tolerance, an Arabidopsis study provided evidence linking enhanced ROS accumulation to higher stress tolerance (Guan et al., 2013). The research indicated the existence of a positive a feedback loop, whereby heat and ROS allow for further ROS accumulation, depending on the actions of microRNA398 (miRNA398). miRNA398 expression was found to be induced within 1 h and reach its peak 2 h after heat stress. The miRNA398 promoter region contains a putative HSE, and chromatin immune-precipitation assays revealed direct binding of HSFA1b and HSFA7b to the HSE promoter region under heat stress. Thus, association of these HSFs to the promoter region seems to be responsible for the induction of this miRNA upon heat stress (Guan et al., 2013). miRNA398 negatively regulates the expression of three target genes: CSD1, CSD2, and CCS (Guan et al., 2013). CSD1 and CSD2 genes are isoforms of copper/zinc-SOD scavenging genes which are located in the cytoplasm and chloroplasts, respectively (Bowler et al., 1992; Kliebenstein et al., 1998) and CCS is a copper chaperone encoding gene, which delivers copper to both CSD genes (Cohu et al., 2009). Consequently, CSD1, CSD2, and CCS are down-regulated during heat stress, allowing further ROS accumulation. This pathway acts in an autocatalytic manner, as H2O2 in turn promotes expression of various HSFs, including HSFA7b (Guan et al., 2013). Accumulation of ROS seems to be an unfavorable response for the plant to survive heat stress. However, comparison of wild-type and csd1, csd2, and ccs mutants plants revealed higher heat tolerance in mutant plants while transgenic plants over-expressing miR398-resistant versions of CSD1, CSD2, or CCS were hypersensitive to heat stress (Guan et al., 2013).
These unexpected outcomes may be explained by the increases in oxidative power for helping activate the primary set of HSFs at the start of the heat response. In contrast to Guan et al. (2013); Sunkar et al. (2006) found that lack of miRNA398 enhances tolerance to some other stress factors, high light and chemically induced ROS, via enhanced expression of CSD1 and CSD2 (Sunkar et al., 2006). Therefore, it seems that the benefit of reduced SOD activity is heat-specific, potentially due to the importance of high HSF activity in the first hours of the response to this stress.
A Multi-Level Interaction Model
A number of recent studies have provided evidence for connections between HSFs, HSPs, ROS, and ROS scavengers upon heat stress. Here, we propose a comprehensive model on the relations between the various components to explain a large proportion of the observations (Figure 1). Through contrasting effects on ROS scavenging activity, heat shock induces a short-term positive (roughly, within the first few hours of heat stress) and a long-term negative feedback loop (after the first few hours of heat stress) on the HSF signaling pathway. The proposed complexity of the heat-stress response network is mirrored in some of the counter-intuitive observations, such as enhanced heat tolerance in certain scavenger mutants (Rizhsky et al., 2002; Vanderauwera et al., 2011). However, analogous to the proposed miRNA398 mechanism, constitutively, slightly elevated ROS levels in such mutants may result in a primed state and, as a consequence, a stronger and/or faster response to a heat treatment. Guan et al. (2013) indeed showed that knockout mutations in CSD1 and CSD2 were accompanied by constitutively higher levels of HSF and HSP transcripts. In accordance, the importance of ROS at early heat response was shown by Volkov et al. (2006): a rapid oxidative burst of ROS during the first 15 min of the heat shock stimulates HSF DNA-binding and is essential for the induction of heat responsive gene expression, e.g., of HSPs and APX2 (Volkov et al., 2006). The typical “late” high mobility HSE-binding complexes, formed after 2 h, were shown to be ROS independent (Lohmann et al., 2004; Volkov et al., 2006), which is in accordance with the production of anti-oxidants and ROS scavengers reducing the ROS overload after the early onset of the HSR (Chaitanya et al., 2002; Wahid et al., 2007; Frank et al., 2009; Dong et al., 2015). Nevertheless, if plants are continuously exposed to heat stress, the activity of some antioxidants and scavengers, e.g., APX and CAT, decreased after 3 days of heat stress in tomato, alfalfa and tobacco cell cultures (Wu et al., 2012; Li et al., 2013; Sgobba et al., 2015). The changes of these components of the antioxidant system were ascribed to the impaired health and growth of plants under long term heat stress and are different from short term heat stress (de Pinto et al., 2015).
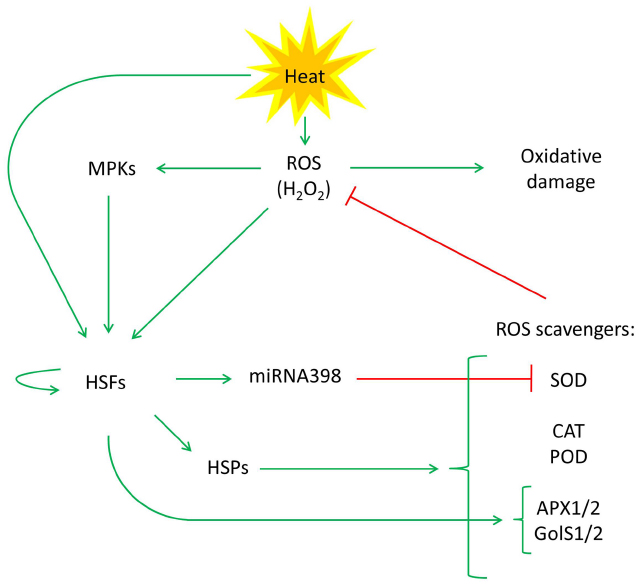
Figure 1. Model describing crosstalk between the HSF/HSP and ROS pathways in the heat stress response. In addition to directly activating HSFs, high temperature leads to the accumulation of ROS. This, in turn, leads to a further activation of HSFs, either directly or indirectly via activation of a MAPK pathway. The HSFs bind to HSE in the promoter region of HSF, HSP, miRNA398, and ROS scavenging genes. The miRNA398-dependent down-regulation of a subset of SOD scavengers might play a role in the rapid ROS accumulation upon exposure to heat. This would support the activation of HSFs and thereby boost the induction of the heat-stress response in the short term. In the longer term, the induction and stabilization of other scavengers would start to suppress ROS levels to avoid excessive cellular damage.
Importantly, the model described here refers specifically to the complexity of events after a short term heat shock; its applicability to other types of heat stress, e.g., mild levels of heat stress, which only affect plant physiology in the long term, is not evident and more research will be necessary in order to clarify how the HSF/HSP and ROS systems behave under those circumstances. Also, it should be noted that the proposed model is not stand-alone and will interact with other factors, such as phytohormones. Abscisic acid (ABA), salicylic acid (SA) and ethylene have all been implicated in the heat response and can induce the production of ROS (Kwak et al., 2006; Foyer and Noctor, 2009). While a number of phytohormone-related mutants show impaired tolerance to heat (Larkindale et al., 2005), application of these hormones may enhance thermotolerance via an effect of ROS. SA application, for example, enhanced SOD activity and HSP expression during heat stress (Clarke et al., 2004; He et al., 2005). Dedicated analysis of the role of hormones during the first hours of heat treatment should clarify their putative positions in the response model.
The model may well have broader applicability then to the heat response only (Jiang and Zhang, 2002; Jammes et al., 2009; Bartoli et al., 2013; Wang et al., 2014b; Hossain et al., 2015). Not only are ROS accumulation, signaling and scavenging thought to occur and play a role in myriad other stress responses (Mittler, 2002; Hossain et al., 2015), but so is HSP activity (Pastori and Foyer, 2002; Banti et al., 2008; Pucciariello et al., 2012). HSPs are also induced upon water stress, salinity and osmotic stress, cold, anoxia, UV-B light, and oxidative stress (Vierling, 1991; Waters et al., 1996; Wang et al., 2004; Loreti et al., 2005; Swindell et al., 2007). Furthermore, overexpression of various HSFs enhanced tolerance to abiotic stresses other than heat, including salt, drought, osmotic, and anoxic stress (Bechtold et al., 2013; Chauhan et al., 2013; Shen et al., 2013; Pérez-Salamó et al., 2014). Also, tomato plants overexpressing the DnaJ/HSP40 LeCDJ1 showed both higher heat and chilling tolerance (Kong et al., 2014a,b) and overexpression of BRZ-INSENSITIVE-LONG HYPOCOTYLS 2 (BIL2), a mitochondrial-localized DnaJ/HSP40 family member, enhanced resistance against salinity and high light stress (Bekh-Ochir et al., 2013). The role of both the oxidative stress and HSF/HSP systems in multiple stress responses might explain the phenomenon of cross-acclimation, where exposure to a certain stress factor improves tolerance to a subsequent different stress factor (Banti et al., 2008, 2010; Chou et al., 2012; Byth-Illing and Bornman, 2013; Hossain et al., 2015).
Conflict of Interest Statement
The authors declare that the research was conducted in the absence of any commercial or financial relationships that could be construed as a potential conflict of interest.
Acknowledgments
This work was supported by the Dutch Topsector Horticulture and Starting Materials (grant number 2013-H320), the China Scholarship Council (grant number 201207565002), the European Commission (Marie Curie Initial Training Network: Solanaceae Pollen Thermotolarance/SPOT-ITN, grant number 289220) and The Netherlands Organisation for Scientific Research (NWO-ALW, grant number 867.15.011).
References
Ahn, S.-G., and Thiele, D. J. (2003). Redox regulation of mammalian heat shock factor 1 is essential for Hsp gene activation and protection from stress. Genes Dev. 17, 516–528. doi: 10.1101/gad.1044503
Almeselmani, M., Deshmukh, P., Sairam, R., Kushwaha, S., and Singh, T. (2006). Protective role of antioxidant enzymes under high temperature stress. Plant Sci. 171, 382–388. doi: 10.1016/j.plantsci.2006.04.009
Apel, K., and Hirt, H. (2004). Reactive oxygen species: metabolism, oxidative stress, and signal transduction. Annu. Rev. Plant Biol. 55, 373–399. doi: 10.1146/annurev.arplant.55.031903.141701
Badiani, M., Schenone, G., Paolacci, A. R., and Fumagalli, I. (1993). Daily fluctuations of antioxidants in bean (Phaseolus vulgaris L.) leaves as affected by the presence of ambient air pollutants. Plant Cell Physiol. 34, 271–279.
Baker, C. J., and Orlandi, E. W. (1995). Active oxygen in plant pathogenesis. Annu. Rev. Phytopathol. 33, 299–321. doi: 10.1146/annurev.py.33.090195.001503
Banti, V., Loreti, E., Novi, G., Santaniello, A., Alpi, A., and Perata, P. (2008). Heat acclimation and cross-tolerance against anoxia in Arabidopsis. Plant Cell Environ. 31, 1029–1037. doi: 10.1111/j.1365-3040.2008.01816.x
Banti, V., Mafessoni, F., Loreti, E., Alpi, A., and Perata, P. (2010). The heat-inducible transcription factor HsfA2 enhances anoxia tolerance in Arabidopsis. Plant Physiol. 152, 1471–1483. doi: 10.1104/pp.109.149815
Bartoli, C. G., Casalongué, C. A., Simontacchi, M., Marquez-Garcia, B., and Foyer, C. H. (2013). Interactions between hormone and redox signalling pathways in the control of growth and cross tolerance to stress. Environ. Exp. Bot. 94, 73–88. doi: 10.1016/j.envexpbot.2012.05.003
Bartoli, C. G., Pastori, G. M., and Foyer, C. H. (2000). Ascorbate biosynthesis in mitochondria is linked to the electron transport chain between complexes III and IV. Plant Physiol. 123, 335–344.
Bechtold, U., Albihlal, W. S., Lawson, T., Fryer, M. J., Sparrow, P. A., Richard, F., et al. (2013). Arabidopsis HEAT SHOCK TRANSCRIPTION FACTORA1b overexpression enhances water productivity, resistance to drought, and infection. J. Exp. Bot. 64, 3467–3481. doi: 10.1093/jxb/ert185
Bekh-Ochir, D., Shimada, S., Yamagami, A., Kanda, S., Ogawa, K., Nakazawa, M., et al. (2013). A novel mitochondrial DnaJ/Hsp40 family protein BIL2 promotes plant growth and resistance against environmental stress in brassinosteroid signaling. Planta 237, 1509–1525. doi: 10.1007/s00425-013-1859-3
Bhattacharjee, S. (2012). An inductive pulse of hydrogen peroxide pretreatment restores redox-homeostasis and oxidative membrane damage under extremes of temperature in two rice cultivars. Plant Growth Regul. 68, 395–410. doi: 10.1007/s10725-012-9728-9
Bhattacharjee, S. (2013). Heat and chilling induced disruption of redox homeostasis and its regulation by hydrogen peroxide in germinating rice seeds (Oryza sativa L., Cultivar Ratna). Physiol. Mol. Biol. Plants 19, 199–207. doi: 10.1007/s12298-012-0159-x
Bokszczanin, K. L., Solanaceae PollenThermotolerance Initial Training Network (SPOT-ITN) Consortium, and Fragkostefanakis, S. (2013). Perspectives on deciphering mechanisms underlying plant heat stress response and thermotolerance. Front. Plant Sci. 4:315. doi: 10.3389/fpls.2013.00315
Boston, R. S., Viitanen, P. V., and Vierling, E. (1996). Molecular chaperones and protein folding in plants. Plant Mol. Biol. 32, 191–222.
Bowler, C., Montagu, M. V., and Inze, D. (1992). Superoxide dismutase and stress tolerance. Annu. Rev. Plant Biol. 43, 83–116. doi: 10.1146/annurev.pp.43.060192.000503
Byth-Illing, H.-A., and Bornman, L. (2013). Heat shock, with recovery, promotes protection of Nicotiana tabacum during subsequent exposure to Ralstonia solanacearum. Cell Stress Chaperones 19, 193–203. doi: 10.1007/s12192-013-0445-8
Chaitanya, K., Sundar, D., Masilamani, S., and Reddy, A. R. (2002). Variation in heat stress-induced antioxidant enzyme activities among three mulberry cultivars. Plant Growth Regul. 36, 175–180. doi: 10.1023/A:1015092628374
Chao, Y.-Y., Hsu, Y., and Kao, C. (2009). Involvement of glutathione in heat shock- and hydrogen peroxide-induced cadmium tolerance of rice (Oryza sativa L.) seedlings. Plant Soil 318, 37–45. doi: 10.1007/s11104-008-9815-x
Chauhan, H., Khurana, N., Agarwal, P., Khurana, J., and Khurana, P. (2013). A seed preferential heat shock transcription factor from wheat provides abiotic stress tolerance and yield enhancement in transgenic Arabidopsis under heat stress environment. PLoS ONE 8:e79577. doi: 10.1371/journal.pone.0079577
Chen, S., Liu, A., Zhang, S., Li, C., Chang, R., Liu, D., et al. (2013). Overexpression of mitochondrial uncoupling protein conferred resistance to heat stress and Botrytis cinerea infection in tomato. Plant Physiol. Biochem. 73, 245–253. doi: 10.1016/j.plaphy.2013.10.002
Chou, T.-S., Chao, Y.-Y., and Kao, C. H. (2012). Involvement of hydrogen peroxide in heat shock-and cadmium-induced expression of ascorbate peroxidase and glutathione reductase in leaves of rice seedlings. J. Plant Physiol. 169, 478–486. doi: 10.1016/j.jplph.2011.11.012
Chu, B., Soncin, F., Price, B. D., Stevenson, M. A., and Calderwood, S. K. (1996). Sequential phosphorylation by mitogen-activated protein kinase and glycogen synthase kinase 3 represses transcriptional activation by heat shock factor-1. J. Biol. Chem. 271, 30847–30857. doi: 10.1074/jbc.271.48.30847
Clarke, S. M., Mur, L. A., Wood, J. E., and Scott, I. M. (2004). Salicylic acid dependent signaling promotes basal thermotolerance but is not essential for acquired thermotolerance in Arabidopsis thaliana. Plant J. 38, 432–447. doi: 10.1111/j.1365-313X.2004.02054.x
Cohu, C. M., Abdel-Ghany, S. E., Reynolds, K. A. G., Onofrio, A. M., Bodecker, J. R., Kimbrel, J. A., et al. (2009). Copper delivery by the copper chaperone for chloroplast and cytosolic copper/zinc-superoxide dismutases: regulation and unexpected phenotypes in an Arabidopsis mutant. Mol. Plant 2, 1336–1350. doi: 10.1093/mp/ssp084
Das, K., and Roychoudhury, A. (2014). Reactive oxygen species (ROS) and response of antioxidants as ROS-scavengers during environmental stress in plants. Front. Environ. Sci. 2:53. doi: 10.3389/fenvs.2014.00053
Dat, J. F., Foyer, C. H., and Scott, I. M. (1998). Changes in salicylic acid and antioxidants during induced thermotolerance in mustard seedlings. Plant Physiol. 118, 1455–1461. doi: 10.1104/pp.118.4.1455
Davletova, S., Rizhsky, L., Liang, H., Shengqiang, Z., Oliver, D. J., Coutu, J., et al. (2005). Cytosolic ascorbate peroxidase 1 is a central component of the reactive oxygen gene network of Arabidopsis. Plant Cell 17, 268–281. doi: 10.1105/tpc.104.026971
del Rio, L. A., Sandalio, L. M., Corpas, F. J., Palma, J. M., and Barroso, J. B. (2006). Reactive oxygen species and reactive nitrogen species in peroxisomes. Production, scavenging, and role in cell signaling. Plant Physiol. 141, 330–335. doi: 10.1104/pp.106.078204
de Pinto, M. C., Locato, V., Paradiso, A., and De Gara, L. (2015). Role of redox homeostasis in thermo-tolerance under a climate change scenario. Ann. Bot. 116, 487–496. doi: 10.1093/aob/mcv071
Desikan, R., Hancock, J., and Neill, S. (2004). “Oxidative stress signalling,” in Plant Responses to Abiotic Stress, eds H. Hirt, and K. Shinozaki (Berlin: Springer), 121–149.
Desikan, R., Soheila, A.-H., Hancock, J. T., and Neill, S. J. (2001). Regulation of the Arabidopsis transcriptome by oxidative stress. Plant Physiol. 127, 159–172. doi: 10.1104/pp.127.1.159
Dong, X., Yi, H., Lee, J., Nou, I.-S., Han, C.-T., and Hur, Y. (2015). Global gene-expression analysis to identify differentially expressed genes critical for the heat stress response in Brassica rapa. PLoS ONE 10:e0130451. doi: 10.1371/journal.pone.0130451
Evrard, A., Kumar, M., Lecourieux, D., Lucks, J., von Koskull-Döring, P., and Hirt, H. (2013). Regulation of the heat stress response in Arabidopsis by MPK6-targeted phosphorylation of the heat stress factor HsfA2. PeerJ 1, e59. doi: 10.7717/peerj.59
Foyer, C., and Noctor, G. (2005). Redox homeostasis and antioxidant signaling: a metabolic interface between stress perception and physiological responses. Plant Cell 17, 1866–1875. doi: 10.1105/tpc.105.033589
Foyer, C. H., and Noctor, G. (2009). Redox regulation in photosynthetic organisms: signaling, acclimation, and practical implications. Antioxid. Redox Signal. 11, 861–905. doi: 10.1089/ars.2008.2177
Frank, G., Pressman, E., Ophir, R., Althan, L., Shaked, R., Freedman, M., et al. (2009). Transcriptional profiling of maturing tomato (Solanum lycopersicum L.) microspores reveals the involvement of heat shock proteins, ROS scavengers, hormones, and sugars in the heat stress response. J. Exp. Bot. 60, 3891–3908. doi: 10.1093/jxb/erp234
Galvez-Valdivieso, G., and Mullineaux, P. (2010). The role of reactive oxygen species in signalling from chloroplasts to the nucleus. Physiol. Plant. 138, 430–439. doi: 10.1111/j.1399-3054.2009.01331.x
Gechev, T. S., and Hille, J. (2005). Hydrogen peroxide as a signal controlling plant programmed cell death. J. Cell Biol. 168, 17–20. doi: 10.1083/jcb.200409170
Giardi, M. T., Masojidek, J., and Godde, D. (1997). Effects of abiotic stresses on the turnover of the D1 reaction centre II protein. Physiol. Plant. 101, 635–642. doi: 10.1111/j.1399-3054.1997.tb01048.x
Giesguth, M., Sahm, A., Simon, S., and Dietz, K.-J. (2015). Redox-dependent translocation of the heat shock transcription factor AtHSFA8 from the cytosol to the nucleus in Arabidopsis thaliana. FEBS Lett. 589, 718–725. doi: 10.1016/j.febslet.2015.01.039
Guan, Q., Lu, X., Zeng, H., Zhang, Y., and Zhu, J. (2013). Heat stress induction of miR398 triggers a regulatory loop that is critical for thermotolerance in Arabidopsis. Plant J. 74, 840–851. doi: 10.1111/tpj.12169
Gupta, A. S., Webb, R. P., Holaday, A. S., and Allen, R. D. (1993). Overexpression of superoxide dismutase protects plants from oxidative stress (induction of ascorbate peroxidase in superoxide dismutase-overexpressing plants). Plant Physiol. 103, 1067–1073.
Hasanuzzaman, M., Hossain, M. A., da Silva, J. A. T., and Fujita, M. (2012). “Plant response and tolerance to abiotic oxidative stress: antioxidant defense is a key factor,” in Crop Stress and its Management: Perspectives and Strategies, eds B. Venkateswarlu, A. K. Shanker, C. Shanker, and M. Maheswari (New York: Springer), 261–315.
Hasanuzzaman, M., Nahar, K., Alam, M. M., Roychowdhury, R., and Fujita, M. (2013). Physiological, biochemical, and molecular mechanisms of heat stress tolerance in plants. Int. J. Mol. Sci. 14, 9643–9684. doi: 10.3390/ijms14059643
He, Y., Liu, Y., Cao, W., Huai, M., Xu, B., and Huang, B. (2005). Effects of salicylic acid on heat tolerance associated with antioxidant metabolism in Kentucky bluegrass. Crop Sci. 45, 988–995. doi: 10.2135/cropsci2003.0678
Hossain, M. A., Bhattacharjee, S., Armin, S., Qian, P., Xin, W., Li, H., et al. (2015). Hydrogen peroxide priming modulates abiotic oxidative stress tolerance: insights from ROS detoxification and scavenging. Front. Plant Sci. 6:420. doi: 10.3389/fpls.2015.00420
Hossain, M. A., Mostofa, M. G., and Fujita, M. (2013). Cross protection by cold-shock to salinity and drought stress-induced oxidative stress in mustard (Brassica campestris L.) seedlings. Mol. Plant Breed. 4, 50–70. doi: 10.5376/mpb.2013.04.0007
Hübel, A., and Schöffl, F. (1994). Arabidopsis heat shock factor: isolation and characterization of the gene and the recombinant protein. Plant Mol. Biol. 26, 353–362. doi: 10.1007/BF00039545
Jammes, F., Song, C., Shin, D., Munemasa, S., Takeda, K., Gu, D., et al. (2009). MAP kinases MPK9 and MPK12 are preferentially expressed in guard cells and positively regulate ROS-mediated ABA signaling. Proc. Natl. Acad. Sci. U.S.A. 106, 20520–20525. doi: 10.1073/pnas.0907205106
Jiang, C., Xu, J., Zhang, H., Zhang, X., Shi, J., Li, M., et al. (2009). A cytosolic class I small heat shock protein, RcHSP17.8, of Rosa chinensis confers resistance to a variety of stresses to Escherichia coli, yeast and Arabidopsis thaliana. Plant Cell Environ. 32, 1046–1059. doi: 10.1111/j.1365-3040.2009.01987.x
Jiang, M., and Zhang, J. (2002). Involvement of plasma-membrane NADPH oxidase in abscisic acid-and water stress-induced antioxidant defense in leaves of maize seedlings. Planta 215, 1022–1030. doi: 10.1007/s00425-002-0829-y
Kang, N. J., Kang, Y. I., Kang, K. H., and Jeong, B. R. (2009). Induction of thermotolerance and activation of antioxidant enzymes in H2O2 pre-applied leaves of cucumber and tomato seedlings. J. Jpn. Soc. Hort. Sci. 78, 320–329. doi: 10.2503/jjshs1.78.320
Kim, D., Kim, S., and Li, G. (1999). Proteasome inhibitors MG132 and lactacystin hyperphosphorylate HSF1 and induce hsp70 and hsp27 expression. Biochem. Biophys. Res. Commun. 254, 264–268. doi: 10.1006/bbrc.1998.9840
Kliebenstein, D. J., Monde, R.-A., and Last, R. L. (1998). Superoxide dismutase in Arabidopsis: an eclectic enzyme family with disparate regulation and protein localization. Plant Physiol. 118, 637–650. doi: 10.1104/pp.118.2.637
Knauf, U., Newton, E. M., Kyriakis, J., and Kingston, R. E. (1996). Repression of human heat shock factor 1 activity at control temperature by phosphorylation. Genes Dev. 10, 2782–2793. doi: 10.1101/gad.10.21.2782
Kong, F., Deng, Y., Wang, G., Wang, J., Liang, X., and Meng, Q. (2014a). LeCDJ1, a chloroplast DnaJ protein, facilitates heat tolerance in transgenic tomatoes. J. Integr. Plant Biol. 56, 63–74. doi: 10.1111/jipb.12119
Kong, F., Deng, Y., Zhou, B., Wang, G., Wang, Y., and Meng, Q. (2014b). A chloroplast-targeted DnaJ protein contributes to maintenance of photosystem II under chilling stress. J. Exp. Bot. 65, 143–158. doi: 10.1093/jxb/ert357
Kotak, S., Larkindale, J., Lee, U., von Koskull-Döring, P., Vierling, E., and Scharf, K.-D. (2007). Complexity of the heat stress response in plants. Curr. Opin. Plant Biol. 10, 310–316. doi: 10.1016/j.pbi.2007.04.011
Kovtun, Y., Chiu, W.-L., Tena, G., and Sheen, J. (2000). Functional analysis of oxidative stress-activated mitogen-activated protein kinase cascade in plants. Proc. Natl. Acad. Sci. U.S.A. 97, 2940–2945. doi: 10.1073/pnas.97.6.2940
Krasensky, J., and Jonak, C. (2012). Drought, salt, and temperature stress-induced metabolic rearrangements and regulatory networks. J. Exp. Bot. 63, 1593–1608. doi: 10.1093/jxb/err460
Kreslavski, V. D., Los, D. A., Allakhverdiev, S. I., and Vl, V. K. (2012). Signaling role of reactive oxygen species in plants under stress. Russ. J. Plant Physiol. 59, 163–178. doi: 10.1134/S1021443712020057
Kwak, J. M., Nguyen, V., and Schroeder, J. I. (2006). The role of reactive oxygen species in hormonal responses. Plant Physiol. 141, 323–329. doi: 10.1104/pp.106.079004
Larkindale, J., Hall, J. D., Knight, M. R., and Vierling, E. (2005). Heat stress phenotypes of Arabidopsis mutants implicate multiple signaling pathways in the acquisition of thermotolerance. Plant Physiol. 138, 882–897. doi: 10.1104/pp.105.062257
Larkindale, J., and Knight, M. R. (2002). Protection against heat stress-induced oxidative damage in Arabidopsis involves calcium, abscisic acid, ethylene, and salicylic acid. Plant Physiol. 128, 682–695. doi: 10.1104/pp.010320
Li, C., Chen, Q., Gao, X., Qi, B., Chen, N., Xu, S., et al. (2005). AtHsfA2 modulates expression of stress responsive genes and enhances tolerance to heat and oxidative stress in Arabidopsis. Sci. China C Life Sci. 48, 540–550.
Li, W., Wei, Z., Qiao, Z., Wu, Z., Cheng, L., and Wang, Y. (2013). Proteomics analysis of alfalfa response to heat stress. PLoS ONE 8:e82725. doi: 10.1371/journal.pone.0082725
Link, V., Sinha, A., Vashista, P., Hofmann, M., Proels, R., Ehness, R., et al. (2002). A heat-activated MAP kinase in tomato: a possible regulator of the heat stress response. FEBS Lett. 531, 179–183. doi: 10.1016/S0014-5793(02)03498-1
Liu, Y., Zhang, C., Chen, J., Guo, L., Li, X., Li, W., et al. (2013). Arabidopsis heat shock factor HsfA1a directly senses heat stress, pH changes, and hydrogen peroxide via the engagement of redox state. Plant Physiol. Biochem. 64, 92–98. doi: 10.1016/j.plaphy.2012.12.013
Lohmann, C., Eggers-Schumacher, G., Wunderlich, M., and Schöffl, F. (2004). Two different heat shock transcription factors regulate immediate early expression of stress genes in Arabidopsis. Mol. Genet. Genomics 271, 11–21. doi: 10.1007/s00438-003-0954-8
Loreti, E., Poggi, A., Novi, G., Alpi, A., and Perata, P. (2005). A genome-wide analysis of the effects of sucrose on gene expression in Arabidopsis seedlings under anoxia. Plant Physiol. 137, 1130–1138. doi: 10.1104/pp.104.057299
Miller, G., and Mittler, R. (2006). Could heat shock transcription factors function as hydrogen peroxide sensors in plants? Ann. Bot. 98, 279–288. doi: 10.1093/aob/mcl107
Miller, G., Schlauch, K., Tam, R., Cortes, D., Torres, M. A., Shulaev, V., et al. (2009). The plant NADPH oxidase RBOHD mediates rapid systemic signaling in response to diverse stimuli. Sci. Signal. 2, ra45. doi: 10.1126/scisignal.2000448
Mittal, D., Madhyastha, D., and Grover, A. (2012). Genome-wide transcriptional profiles during temperature and oxidative stress reveal coordinated expression patterns and overlapping regulons in rice. PLoS ONE 7:e40899. doi: 10.1371/journal.pone.0040899
Mittler, R. (2002). Oxidative stress, antioxidants and stress tolerance. Trends Plant Sci. 7, 405–410. doi: 10.1016/S1360-1385(02)02312-9
Mittler, R., Vanderauwera, S., Gollery, M., and Van Breusegem, F. (2004). Reactive oxygen gene network of plants. Trends Plant Sci. 9, 490–498. doi: 10.1016/j.tplants.2004.08.009
Mittler, R., Vanderauwera, S., Suzuki, N., Miller, G., Tognetti, V. B., Vandepoele, K., et al. (2011). ROS signaling: the new wave? Trends Plant Sci. 16, 300–309. doi: 10.1016/j.tplants.2011.03.007
Moon, H., Lee, B., Choi, G., Shin, D., Prasad, D. T., Lee, O., et al. (2003). NDP kinase 2 interacts with two oxidative stress-activated MAPKs to regulate cellular redox state and enhances multiple stress tolerance in transgenic plants. Proc. Natl. Acad. Sci. U.S.A. 100, 358–363. doi: 10.1073/pnas.252641899
Morgan, R. W., Christman, M. F., Jacobson, F. S., Storz, G., and Ames, B. N. (1986). Hydrogen peroxide-inducible proteins in Salmonella typhimurium overlap with heat shock and other stress proteins. Proc. Natl. Acad. Sci. U.S.A. 83, 8059–8063. doi: 10.1073/pnas.83.21.8059
Morimoto, R. (1998). Regulation of the heat shock transcriptional response: cross talk between a family of heat shock factors, molecular chaperones, and negative regulators. Genes Dev. 12, 3788–3796. doi: 10.1101/gad.12.24.3788
Mostofa, M. G., Yoshida, N., and Fujita, M. (2014). Spermidine pretreatment enhances heat tolerance in rice seedlings through modulating antioxidative and glyoxalase systems. Plant Growth Regul. 73, 31–44. doi: 10.1007/s10725-013-9865-9
Munns, R., and Tester, M. (2008). Mechanisms of salinity tolerance. Annu. Rev. Plant Biol. 59, 651–681. doi: 10.1146/annurev.arplant.59.032607.092911
Navrot, N., Rouhier, N., Gelhaye, E., and Jacquot, J. (2007). Reactive oxygen species generation and antioxidant systems in plant mitochondria. Physiol. Plant. 129, 185–195. doi: 10.1111/j.1399-3054.2006.00777.x
Nishizawa, A., Yabuta, Y., Yoshida, E., Maruta, T., Yoshimura, K., and Shigeoka, S. (2006). Arabidopsis heat shock transcription factor A2 as a key regulator in response to several types of environmental stress. Plant J. 48, 535–547.
Nishizawa, A., Yabuta, Y., and Shigeoka, S. (2008). Galactinol and raffinose constitute a novel function to protect plants from oxidative damage. Plant Physiol. 147, 1251–1263. doi: 10.1104/pp.108.122465
O’Kane, D., Gill, V., Boyd, P., and Burdon, R. (1996). Chilling, oxidative stress and antioxidant responses in Arabidopsis thaliana callus. Planta 198, 371–377. doi: 10.1007/BF00620053
Panchuk, I. I., Volkov, R. A., and Schöffl, F. (2002). Heat stress-and heat shock transcription factor-dependent expression and activity of ascorbate peroxidase in Arabidopsis. Plant Physiol. 129, 838–853. doi: 10.1104/pp.001362
Pastori, G., and Foyer, C. (2002). Common components, networks, and pathways of cross-tolerance to stress. The central role of “redox” and abscisic acid-mediated controls. Plant Physiol. 129, 460–468. doi: 10.1104/pp.011021
Pelham, H. R. (1982). A regulatory upstream promoter element in the Drosophila hsp 70 heat-shock gene. Cell 30, 517–528. doi: 10.1016/0092-8674(82)90249-5
Pérez-Salamó, I., Papdi, C., Gábor, R., Zsigmond, L., Vilela, B., Lumbreras, V., et al. (2014). The Heat Shock Factor HSFA4A confers salt tolerance and is regulated by oxidative stress and the MAP kinases, MPK3 and MPK6. Plant Physiol. 165, 319–334. doi: 10.1104/pp.114.237891
Petrov, V. D., and Van Breusegem, F. (2012). Hydrogen peroxide—a central hub for information flow in plant cells. AoB Plants 2012, pls014. doi: 10.1093/aobpla/pls014
Pnueli, L., Liang, H., Rozenberg, M., and Mittler, R. (2003). Growth suppression, altered stomatal responses, and augmented induction of heat shock proteins in cytosolic ascorbate peroxidase (Apx1)-deficient Arabidopsis plants. Plant J. 34, 187–203. doi: 10.1046/j.1365-313X.2003.01715.x
Pucciariello, C., Banti, V., and Perata, P. (2012). ROS signaling as common element in low oxygen and heat stresses. Plant Physiol. Biochem. 59, 3–10. doi: 10.1016/j.plaphy.2012.02.016
Qiu, X.-B., Shao, Y.-M., Miao, S., and Wang, L. (2006). The diversity of the DnaJ/Hsp40 family, the crucial partners for Hsp70 chaperones. Cell. Mol. Life Sci. 63, 2560–2570. doi: 10.1007/s00018-006-6192-6
Rainwater, D., Gossett, D., Millhollon, E., Hanna, H., Banks, S., and Lucas, M. (1996). The relationship between yield and the antioxidant defense system in tomatoes grown under heat stress. Free Radic. Res. 25, 421–435. doi: 10.3109/10715769609149065
Reddy, R., Kumar, B., Reddy, P., Mishra, R., Mahanty, S., Kaul, T., et al. (2009). Molecular cloning and characterization of genes encoding Pennisetum glaucum ascorbate peroxidase and heat-shock factor: interlinking oxidative and heat-stress responses. J. Plant Physiol. 166, 1646–1659. doi: 10.1016/j.jplph.2009.04.007
Rentel, M. C., Lecourieux, D., Ouaked, F., Usher, S. L., Petersen, L., Okamoto, H., et al. (2004). OXI1 kinase is necessary for oxidative burst-mediated signalling in Arabidopsis. Nature 427, 858–861. doi: 10.1038/nature02353
Rizhsky, L., Davletova, S., Liang, H., and Mittler, R. (2004). The zinc finger protein Zat12 is required for cytosolic ascorbate peroxidase 1 expression during oxidative stress in Arabidopsis. J. Biol. Chem. 279, 11736–11743. doi: 10.1074/jbc.M313350200
Rizhsky, L., Hallak-Herr, E., Van Breusegem, F., Rachmilevitch, S., Barr, J. E., Rodermel, S., et al. (2002). Double antisense plants lacking ascorbate peroxidase and catalase are less sensitive to oxidative stress than single antisense plants lacking ascorbate peroxidase or catalase. Plant J. 32, 329–342. doi: 10.1046/j.1365-313X.2002.01427.x
Rui, R., Nie, Y., and Tong, H. (1990). SOD activity as a parameter for screening stress-tolerant germplasm resources in sweet potato (Ipomoea batatas L.). Jiangsu J. Agric. Sci. 6, 52–56.
Sairam, R., Srivastava, G., and Saxena, D. (2000). Increased antioxidant activity under elevated temperatures: a mechanism of heat stress tolerance in wheat genotypes. Biol. Plant. 43, 245–251. doi: 10.1023/A:1002756311146
Scharf, K.-D., Berberich, T., Ebersberger, I., and Nover, L. (2012). The plant heat stress transcription factor (Hsf) family: structure, function and evolution. Biochim. Biophys. Acta 1819, 104–119. doi: 10.1016/j.bbagrm.2011.10.002
Schöffl, F., Prändl, R., and Reindl, A. (1998). Regulation of the heat-shock response. Plant Physiol. 117, 1135–1141. doi: 10.1104/pp.117.4.1135
Schöffl, F., Prändl, R., and Reindl, A. (1999). “Molecular responses to heat stress,” in Molecular Responses to Cold, Drought, Heat and Salt Stress in Higher Plants, K. Yamaguchi-Shinozai and K. Shinozaki (Austin, TX: Landes Co.), 81–98.
Schramm, F., Ganguli, A., Kiehlmann, E., Englich, G., Walch, D., and von Koskull-Döring, P. (2006). The heat stress transcription factor HsfA2 serves as a regulatory amplifier of a subset of genes in the heat stress response in Arabidopsis. Plant Mol. Biol. 60, 759–772. doi: 10.1007/s11103-005-5750-x
Sgobba, A., Paradiso, A., Dipierro, S., De Gara, L., and de Pinto, M. C. (2015). Changes in antioxidants are critical in determining cell responses to short- and long-term heat stress. Physiol. Plant. 153, 68–78. doi: 10.1111/ppl.12220
Shen, Z., Ding, M., Sun, J., Deng, S., Zhao, R., Wang, M., et al. (2013). Overexpression of PeHSF mediates leaf ROS homeostasis in transgenic tobacco lines grown under salt stress conditions. Plant Cell Tissue Organ Cult. 115, 299–308. doi: 10.1007/s11240-013-0362-7
Storozhenko, S., De Pauw, P., Van Montagu, M., Inzé, D., and Kushnir, S. (1998). The heat-shock element is a functional component of the Arabidopsis APX1 gene promoter. Plant Physiol. 118, 1005–1014. doi: 10.1104/pp.118.3.1005
Sun, L., Liu, Y., Kong, X., Zhang, D., Pan, J., Zhou, Y., et al. (2012). ZmHSP16.9, a cytosolic class I small heat shock protein in maize (Zea mays), confers heat tolerance in transgenic tobacco. Plant Cell Rep. 31, 1473–1484. doi: 10.1007/s00299-012-1262-8
Sunkar, R., Kapoor, A., and Zhu, J.-K. (2006). Posttranscriptional induction of two Cu/Zn superoxide dismutase genes in Arabidopsis is mediated by downregulation of miR398 and important for oxidative stress tolerance. Plant Cell 18, 2051–2065. doi: 10.1105/tpc.106.041673
Suzuki, N., Miller, G., Sejima, H., Harper, J., and Mittler, R. (2013). Enhanced seed production under prolonged heat stress conditions in Arabidopsis thaliana plants deficient in cytosolic ascorbate peroxidase 2. J. Exp. Bot. 64, 253–263. doi: 10.1093/jxb/ers335
Suzuki, N., Rivero, R. M., Shulaev, V., Blumwald, E., and Mittler, R. (2014). Abiotic and biotic stress combinations. New Phytol. 203, 32–43. doi: 10.1111/nph.12797
Swindell, W., Huebner, M., and Weber, A. (2007). Transcriptional profiling of Arabidopsis heat shock proteins and transcription factors reveals extensive overlap between heat and non-heat stress response pathways. BMC Genomics 8:125. doi: 10.1186/1471-2164-8-125
Torres, M. A., and Dangl, J. L. (2005). Functions of the respiratory burst oxidase in biotic interactions, abiotic stress and development. Curr. Opin. Plant Biol. 8, 397–403. doi: 10.1016/j.pbi.2005.05.014
Uchida, A., Jagendorf, A. T., Hibino, T., Takabe, T., and Takabe, T. (2002). Effects of hydrogen peroxide and nitric oxide on both salt and heat stress tolerance in rice. Plant Sci. 163, 515–523. doi: 10.1016/S0168-9452(02)00159-0
Vacca, R., de Pinto, M., Valenti, D., Passarella, S., Marra, E., and De Gara, L. (2004). Production of reactive oxygen species, alteration of cytosolic ascorbate peroxidase, and impairment of mitochondrial metabolism are early events in heat shock-induced programmed cell death in tobacco Bright-Yellow 2 cells. Plant Physiol. 134, 1100–1112. doi: 10.1104/pp.103.035956
Valliyodan, B., and Nguyen, H. T. (2006). Understanding regulatory networks and engineering for enhanced drought tolerance in plants. Curr. Opin. Plant Biol. 9, 189–195. doi: 10.1016/j.pbi.2006.01.019
Vanderauwera, S., Suzuki, N., Miller, G., van de Cotte, B., Morsa, S., Ravanat, J.-L., et al. (2011). Extranuclear protection of chromosomal DNA from oxidative stress. Proc. Natl. Acad. Sci. U.S.A. 108, 1711–1716. doi: 10.1073/pnas.1018359108
Vierling, E. (1991). The roles of heat shock proteins in plants. Annu. Rev. Plant Biol. 42, 579–620. doi: 10.1146/annurev.pp.42.060191.003051
Volkov, R. A., Panchuk, I. I., Mullineaux, P. M., and Schöffl, F. (2006). Heat stress-induced H2O2 is required for effective expression of heat shock genes in Arabidopsis. Plant Mol. Biol. 61, 733–746. doi: 10.1007/s11103-006-0045-4
Vranová, E., Inzé, D., and Van Breusegem, F. (2002). Signal transduction during oxidative stress. J. Exp. Bot. 53, 1227–1236. doi: 10.1093/jexbot/53.372.1227
Wahid, A., Gelani, S., Ashraf, M., and Foolad, M. (2007). Heat tolerance in plants: an overview. Environ. Exp. Bot. 61, 199–223. doi: 10.1016/j.envexpbot.2007.05.011
Wang, L., Guo, Y., Jia, L., Chu, H., Zhou, S., Chen, K., et al. (2014a). Hydrogen peroxide acts upstream of nitric oxide in the heat shock pathway in Arabidopsis seedlings. Plant Physiol. 164, 2184–2196. doi: 10.1104/pp.113.229369
Wang, X., Jia, N., Zhao, C., Fang, Y., Lv, T., Zhou, W., et al. (2014b). Knockout of AtDjB1, a J-domain protein from Arabidopsis thaliana, alters plant responses to osmotic stress and abscisic acid. Physiol. Plant. 152, 286–300. doi: 10.1111/ppl.12169
Wang, Y., Zhang, J., Li, J.-L., and Ma, X.-R. (2014c). Exogenous hydrogen peroxide enhanced the thermotolerance of Festuca arundinacea and Lolium perenne by increasing the antioxidative capacity. Acta Physiol. Plant. 36, 2915–2924. doi: 10.1007/s11738-014-1661-2
Wang, W., Vinocur, B., and Altman, A. (2003). Plant responses to drought, salinity and extreme temperatures: towards genetic engineering for stress tolerance. Planta 218, 1–14. doi: 10.1007/s00425-003-1105-5
Wang, W., Vinocur, B., Shoseyov, O., and Altman, A. (2004). Role of plant heat-shock proteins and molecular chaperones in the abiotic stress response. Trends Plant Sci. 9, 244–252. doi: 10.1016/j.tplants.2004.03.006
Waters, E. R., Lee, G. J., and Vierling, E. (1996). Evolution, structure and function of the small heat shock proteins in plants. J. Exp. Bot. 47, 325–338. doi: 10.1093/jxb/47.3.325
Wu, Q., Lin, J., Liu, J.-Z., Wang, X., Lim, W., Oh, M., et al. (2012). Ectopic expression of Arabidopsis glutaredoxin AtGRXS17 enhances thermotolerance in tomato. Plant Biotechnol. J. 10, 945–955. doi: 10.1111/j.1467-7652.2012.00723.x
Zhou, W., Zhou, T., Li, M.-X., Zhao, C.-L., Jia, N., Wang, X.-X., et al. (2012). The Arabidopsis J-protein AtDjB1 facilitates thermotolerance by protecting cells against heat-induced oxidative damage. New Phytol. 194, 364–378. doi: 10.1111/j.1469-8137.2012.04070.x
Keywords: heat response, heat shock factor, heat shock protein, reactive oxygen species, ROS scavenging, signaling, interaction, cross-talk
Citation: Driedonks N, Xu J, Peters JL, Park S and Rieu I (2015) Multi-Level Interactions Between Heat Shock Factors, Heat Shock Proteins, and the Redox System Regulate Acclimation to Heat. Front. Plant Sci. 6:999. doi: 10.3389/fpls.2015.00999
Received: 05 August 2015; Accepted: 30 October 2015;
Published: 17 November 2015.
Edited by:
Girdhar K. Pandey, University of Delhi, IndiaReviewed by:
Serge Delrot, University of Bordeaux, FranceRamamurthy Mahalingam, USDA Agricultural Research Service, USA
Copyright © 2015 Driedonks, Xu, Peters, Park and Rieu. This is an open-access article distributed under the terms of the Creative Commons Attribution License (CC BY). The use, distribution or reproduction in other forums is permitted, provided the original author(s) or licensor are credited and that the original publication in this journal is cited, in accordance with accepted academic practice. No use, distribution or reproduction is permitted which does not comply with these terms.
*Correspondence: Ivo Rieu, aS5yaWV1QHNjaWVuY2UucnUubmw=