- Plant Biology Unit, Department of Agricultural and Environmental Sciences, University of Udine, Udine, Italy
The synthesis of ATP in mitochondria is dependent on a low permeability of the inner membrane. Nevertheless, mitochondria can undergo an increased permeability to solutes, named permeability transition (PT) that is mediated by a permeability transition pore (PTP). PTP opening requires matrix Ca2+ and leads to mitochondrial swelling and release of intramembrane space proteins (e.g., cytochrome c). This feature has been initially observed in mammalian mitochondria and tentatively attributed to some components present either in the outer or inner membrane. Recent works on mammalian mitochondria point to mitochondrial ATP synthase dimers as physical basis for PT, a finding that has been substantiated in yeast and Drosophila mitochondria. In plant mitochondria, swelling and release of proteins have been linked to programmed cell death, but in isolated mitochondria PT has been observed in only a few cases and in plant cell cultures only indirect evidence is available. The possibility that mitochondrial ATP synthase dimers could function as PTP also in plants is discussed here on the basis of the current evidence. Finally, a hypothetical explanation for the origin of PTP is provided in the framework of molecular exaptation.
The Permeability Transition
ATP synthesis in mitochondria occurs by a chemiosmotic coupling of substrate oxidation and phosphorylation (Mitchell, 1961). This explanation is based on the highly selective permeability of the inner mitochondrial membrane (IMM) and on utilization of protonmotive force by the F1FO ATP synthase (F-ATPase) for the synthesis of ATP. Nevertheless, a sudden increase in permeability of the IMM has been described in the 1950s (Raaflaub, 1953a,b) and characterized in the late 1970s (Haworth and Hunter, 1979; Hunter and Haworth, 1979a,b). Initially considered an artifact, later it has been named Permeability Transition (PT) and associated to a pore, the Permeability Transition Pore (PTP). The appreciation of its relevance has increased since it has been related to many diseases in mammals, including reperfusion injury of the heart and muscular dystrophy (Bernardi, 2013a). This mitochondrial PT requires matrix Ca2+ and is favored by matrix Pi, as well as benzodiazepine Bz-423 and thiol oxidants, while it can be inhibited by Mg2+, thiol reductants, ADP and ATP (Bernardi, 2013b). Cyclosporin A (CsA) acts as inhibitor of PT (Crompton et al., 1988) by binding with the peptidyl-prolyl isomerase Cyclophilin D (CyPD) (Halestrap and Davidson, 1990). The features of PTP (e.g., pore diameter of ∼2.8 nm and size exclusion of about 1500 Da) are consistent with those described for the Mitochondrial Mega-Channel (MMC), a high-conductance channel, which is considered to be its electrophysiological equivalent (Szabó and Zoratti, 1992).
The PT in Plants
The first evidence of a Ca2+-induced and CsA-delayed collapse of transmembrane electrical potential difference (ΔΨ) in pea stem mitochondria dates back to 1995 (Vianello et al., 1995). PT has been then observed in different plant species, although the features of this phenomenon cannot be summarized in a straightforward model (Table 1). Potato tuber mitochondria exhibit a typical Ca2+/Pi-induced PT, inhibited (Arpagaus et al., 2002) or not (Fortes et al., 2001) by CsA. These mitochondria do not show any Ca2+ uptake, suggesting an external effect of Ca2+ on PT (Fortes et al., 2001), which is not consistent with the observations in mammals (Bernardi et al., 2015). The PT described in oat leaves (Curtis and Wolpert, 2002) and wheat roots (Virolainen et al., 2002) shows a Ca2+/Pi -induced ΔΨ collapse and matrix swelling, which are CsA-insensitive. Calcium uptake by isolated plant mitochondria occurs spontaneously in wheat, but requires the addition of the Ca2+/H+ ionophore A23187 in oat.
Indirect evidence of PT in plants has been also based on the CsA-induced inhibition of programmed cell death (PCD), reviewed by Vianello et al. (2007, 2012). However, the prevention of PCD might depend on CsA binding to cytosolic Cyclophilin A (a ubiquitous enzyme) that drives enzymatic cascades (Lu et al., 2007), linked to oxidative stress (Nigro et al., 2013).
The Mitochondrial Ca2+ Accumulation in Plants
The PT requires Ca2+ accumulation into the mitochondrial matrix (i.e., matrix Ca2+ is a permissive factor, although it may not be sufficient per se). Calcium transport in isolated plant mitochondria exhibits distinct features. The uptake could be mediated by a low-affinity electrophoretic Pi-dependent symport, with low or no sensitivity to ruthenium red and lanthanides (Dieter and Marme, 1980; Akerman and Moore, 1983; Silva et al., 1992), but also by a uniport mechanism (Zottini and Zannoni, 1993). CsA inhibits mitochondrial Ca2+ transport in Citrus (de Oliveira et al., 2007), suggesting its synergic effect with PT. A low concentration of matrix free Ca2+ (∼100 nM) is maintained under steady state, where influx is balanced by an efflux through a yet speculative Na+-independent Ca2+/H+ antiport mechanism (Nomura and Shiina, 2014). The influx of Ca2+ in plant mitochondria is highly variable, depending on species and tissues, or might be even completely absent (Martins and Vercesi, 1985). In vivo Ca2+ dynamics have been monitored by fluorescent probes targeted to plant mitochondria (Manzoor et al., 2012; Loro and Costa, 2013). Matrix Ca2+ uptake can be induced by abiotic stresses such as heat, oxidative stress, or anoxia, and follows the cytosolic Ca2+ pattern (Subbaiah et al., 1998; Logan and Knight, 2003; Schwarzländer et al., 2012; Rikhvanov et al., 2014).
Homologue genes of mammalian mitochondrial Ca2+ uniporter (MCU) and its regulatory protein MICU1 have been found in plants (Bick et al., 2012; Stael et al., 2012; Rikhvanov et al., 2014). The MICU1 homologue in Arabidopsis (AtMICU) is a negative regulator of mitochondrial Ca2+ uptake in root tips, providing strong evidence for the operation of a mitochondrial Ca2+ uniporter in plants (Wagner et al., 2015).
The Involvement of PT/PCD in Plant Development and Stress Responses
The physiological role of mitochondrial PT in plants is often related to developmental processes (Reape et al., 2015) and mild environmental stresses, which involve also PCD in many cases. However, the mechanistic link between PT and PCD remains still speculative.
Permeability transition/programmed cell death are fundamental in the selection of damaged cells and in sculpturing new anatomical and morphological structures (Van Hautegem et al., 2015). Morphological modifications are also needed for adaptive responses to environment (e.g., climate changes) and, more in general, for fitness increase. In particular, Aponogeton madagascariensis forms lacunae on its leaves by executing PCD, which is inhibited by CsA, suggesting the involvement of PT (Lord et al., 2013). In aerenchyma formation, lack of oxygen induces stress characterized by mitochondrial PT, ATP depletion, and PCD induction (Yamauchi et al., 2013). Consistently, stressed pea plants show cytochrome c release, followed by DNA fragmentation (Sarkar and Gladish, 2012).
Programmed cell death is a common response in plants subjected to abiotic and biotic stresses, which may be linked to the sessile lifestyle, providing a survival strategy for the whole organism. Excess of UV-C stimulates reactive oxygen species (ROS) formation and collapse of ΔΨ in Arabidopsis mitochondria (Gao et al., 2008). The role of PT has also been described in case of extreme temperatures. In Arabidopsis protoplasts, heat stress induces mitochondrial swelling, and ΔΨ loss, but these damages are counteracted by a heat shock transcription factor (Zhang et al., 2009). Similarly, ROS and mild heat shock induce mitochondrial PT and the subsequent induction of cell death in Arabidopsis protoplasts, which are prevented by the superoxide dismutase analog TEMPOL, by the Ca2+ channel-blocker lanthanum chloride, and by CsA (Scott and Logan, 2008). The role of mitochondria in PCD is confirmed in heat-stressed rice protoplasts, where mHSP70 overexpression maintains mitochondrial ΔΨ, partially inhibits cytochrome c release and suppresses PCD by lowering ROS formation (Qi et al., 2011). In wheat cells subjected to freezing, ROS-dependent PCD is associated to ΔΨ collapse and cytochrome c release (Lyubushkina et al., 2014). In salt-stressed tobacco protoplasts, PCD is triggered by ROS produced by mitochondria, through a process controlled by a CsA-sensitive PT (Lin et al., 2006).
The response to heavy metals requires the participation of mitochondrial PT. In particular, aluminum triggers a high ROS production in peanut, by plasmalemma NADPH oxidases, which induce mitochondrial mediated-PCD (Huang et al., 2014). Consistently, metal phytotoxicity appears to be also mediated by PT in aluminum- treated Arabidopsis protoplasts (Li and Xing, 2011) and in cadmium-treated rice roots (Yeh et al., 2007).
Biotic stress, such as pathogen attack, may lead to protoplast shrinkage, mitochondria swelling and cytochrome c release. These responses appear to be associated to PCD involvement during the hypersensitive response, a strategy to counteract biotrophic pathogens. The generation of a defensive layer, promoted by PT-induced PCD, has been shown in Arabidopsis. In particular, PCD is mediated by a rapid decrease in mitochondrial ΔΨ, which is partially counteracted by CsA (Yao et al., 2004). Finally, there is evidence on the release of cytochrome c induced by elicitors such as harpin or victorin (Curtis and Wolpert, 2002; Krause and Durner, 2004).
The Molecular Structure of PTP
The components involved in PTP formation initially included the voltage-dependent anion channel, the benzodiazepine receptor, the adenine nucleotide translocase and the phosphate carrier. This model has been questioned, since isolated mitochondria from organisms where the expression of each of these proteins has been suppressed still exhibit a PT (Kokoszka et al., 2004; Krauskopf et al., 2006; Baines et al., 2007; Gutiérrez-Aguilar et al., 2014; Šileikytė et al., 2014).
Recent evidence shows that F-ATPase is involved in PTP formation in different species and taxa (Bernardi, 2013b; Bonora et al., 2013; Alavian et al., 2014). This enzyme is highly conserved in both prokaryotes and eukaryotes (Hamasur and Glaser, 1992; Heazlewood et al., 2003), consisting in the hydrophilic F1 and the hydrophobic FO sectors, which operate in concert to carry out distinct functions (Antoniel et al., 2014).
The F1 contains five subunits: α and β forming the catalytic region, while γ, δ, and ε are organized in the central stalk. In all eukaryotes these subunits show a high degree of similarity in the sequences (Hamasur and Glaser, 1992; Antoniel et al., 2014; Jiko et al., 2015), while the subunit composition of the FO varies among different taxa and species (Hamasur and Glaser, 1992). For details about F-ATPase components in mammals, fungi and algae, see Vázquez-Acevedo et al. (2006), van Lis et al. (2007), Dabbeni-Sala et al. (2012), Antoniel et al. (2014), Lee et al. (2015) and Liu et al. (2015). Specific subunits have been characterized in plants such as sweet potato (Morikami et al., 1992), potato (Dell’Orto et al., 1993; Polgreen et al., 1995) and soybean (Smith et al., 1994).
Plant F1 includes the classical five-subunit structure (Hamasur and Glaser, 1990, 1992), and also a 24 kDa protein (Li et al., 2012), but the picture of FO components remains still incomplete. Several proteins belonging to FO have been identified in spinach (Hamasur and Glaser, 1992), potato (Jänsch et al., 1996), rice (Heazlewood et al., 2003), and Arabidopsis (Heazlewood et al., 2003; Meyer et al., 2008; Klodmann et al., 2011). As shown by Klodmann et al. (2011) and by Li et al. (2012), FO includes subunits a, c, d, 4 (corresponding to subunit b or orf25, Heazlewood et al., 2003), a 6 kDa protein (plant specific), subunit 8 (also called AL6 or orfB, Heazlewood et al., 2003), ATP17 (plant specific) and Oligomycin Sensitivity-Conferring Protein (OSCP), sometimes referred to as δ’ in plants (Morikami et al., 1992), for some authors belonging to F1 (Jänsch et al., 1996). Subunit g was found detached from F-ATPase monomer, suggesting that it could represent a dimer-specific protein (Meyer et al., 2008; Klodmann et al., 2011). Plant subunit e sequences have been identified so far only in protein databases for few species (e.g., rice and Medicago truncatula).
Multimeric structures of F-ATPase are present in animal, fungi (Davies et al., 2011; Seelert and Dencher, 2011; Liu et al., 2015) and plant mitochondria (Eubel et al., 2003, 2004; Krause et al., 2004; Bultema et al., 2009). Eubel et al. (2003) highlighted the presence of F-ATPase dimers in Arabidopsis, potato, bean, and barley. The relative abundance of dimers in plants is low, with respect to the total F-ATPase, and even lower when comparing different organisms (Eubel et al., 2003, 2004).
Rows of F-ATPase dimers in cristae seem to be a universal feature of all mitochondria (Davies et al., 2011) that enable the formation of highly curved ridges in cristae (Davies et al., 2012). The Inhibitory factor 1 (IF1) that binds F-ATPase at low pH (Campanella et al., 2008) could favor dimer formation even if it is not clear how it improves dimer stability. The arrangement of F-ATPase in mammals and fungi is different from that of potato, being the angle between monomers in the latter larger (∼115°) than in the former (∼80°) (Davies et al., 2011). Interestingly, this correlates with cristae morphology observed for many plant mitochondria, where irregular saccular structures with a less convex curvature appear particularly prevalent (Douce, 1985). In aging Podospora anserina (Ascomycetes) mitochondria, the IMM is progressively vesiculated, the cristae collapse and the F-ATPase dimers are disassembled (Daum et al., 2013). The impairment of ATP synthesis, and the outer membrane rupture by swelling, lead to the release of pro-apoptotic factors and, finally, to cell death.
Animal mitochondria F-ATPase dimers have been shown to act as pores with properties of the PTP (Giorgio et al., 2013). CyPD modulates F-ATPase activity by binding OSCP (Giorgio et al., 2009) and this interaction is favored by Pi, while CsA displaces CyPD from the enzyme. F-ATPase is inhibited by Bz-423, which binds to OSCP (Cleary et al., 2007). These features are consistent with those observed for PT regulation. Magnesium, Ca2+, adenine nucleotides, membrane potential and matrix pH are also key modulators of both F-ATPase activity and PTP. Electrophysiological experiments, after isolation and insertion of F-ATPase dimers in artificial phospholipid bilayers, showed that the pore activity matches that of PTP-MMC (Giorgio et al., 2013).
The involvement of F-ATPase dimers in PTP formation has been extended and confirmed in yeast and Drosophila, even if these organisms show specific differences. In yeast mitochondria the ionophore ETH129 is needed for Ca2+ uptake in the matrix and the PT displays a low conductance (around 300 pS). Phosphate acts as an inhibitor of PT, while CsA does not interfere with PTP. Yeast mutants lacking of subunits e and g, which are involved in dimerization, display a striking resistance to PTP opening (Carraro et al., 2014). In Drosophila (von Stockum et al., 2015), PTP has been initially identified as mitochondrial Ca2+-induced Ca2+ release channel (mCrC). The main differences between mCrC and mammalian PTP are: (i) absence of swelling; (ii) absence of CsA effect, since no CyPD is present in this species; (iii) sensitivity to rotenone, an inhibitor of Complex I; (iv) inhibition of mCrC by Pi; (v) low conductance (around 53 pS) of the F-ATPase dimers in artificial bilayer.
Other research groups have also suggested that F-ATPase is involved in pore formation by the channel activity within the c-ring formed by c subunits of FO (Bonora et al., 2013; Alavian et al., 2014). Nevertheless, this hypothesis is still under debate, since it does not justify the different pore size observed in bovine, yeast, and Drosophila, where similar c-rings are present (Bernardi et al., 2015). Finally, the possible involvement of IF1 in modulation of PTP through F-ATPase dimerization needs further investigations (Faccenda et al., 2013; Bernardi et al., 2015).
The presence in plants of many common components and features of F-ATPase lead us to speculate that, similarly to mammals, yeast, and Drosophila, PT function could be exerted by F-ATPase dimers also in such organisms.
The Emergence of PT During Evolution
Evolution does not always proceed by adaptations. It may also develop a non-adaptive exaptation/cooptation (pre-adaptation), where the term exaptation/cooptation means a trait evolved to accomplish a specific function (or even no function), which may be then exapted/coopted to perform a novel function (or to acquire a function) (Gould and Vrba, 1982).
It has been suggested that the structure of PTP (as a multicomponent complex, Bernardi, 2013a) may have arisen by a mechanism of molecular exaptation, a phenomenon largely recognized at different levels of complexity (genes, proteins, organs), during evolution (Vianello et al., 2012; Barve and Wagner, 2013). The new model, involving F-ATPase dimer in PTP formation, does not contradict our previous interpretation on its origin, but rather appears to support it further. The dimer appears to be the result of a molecular exaptation/cooptation, where two monomers are assembled to perform an additional function (Figure 1A). In other words, F-ATPase seems to have a “Janus double face”, catalyzing the synthesis of ATP, but in some circumstances preventing such a synthesis (Bernardi et al., 2015). This dimer could even possess a “triple face”, because the dimerization induces also the curvature of the IMM.
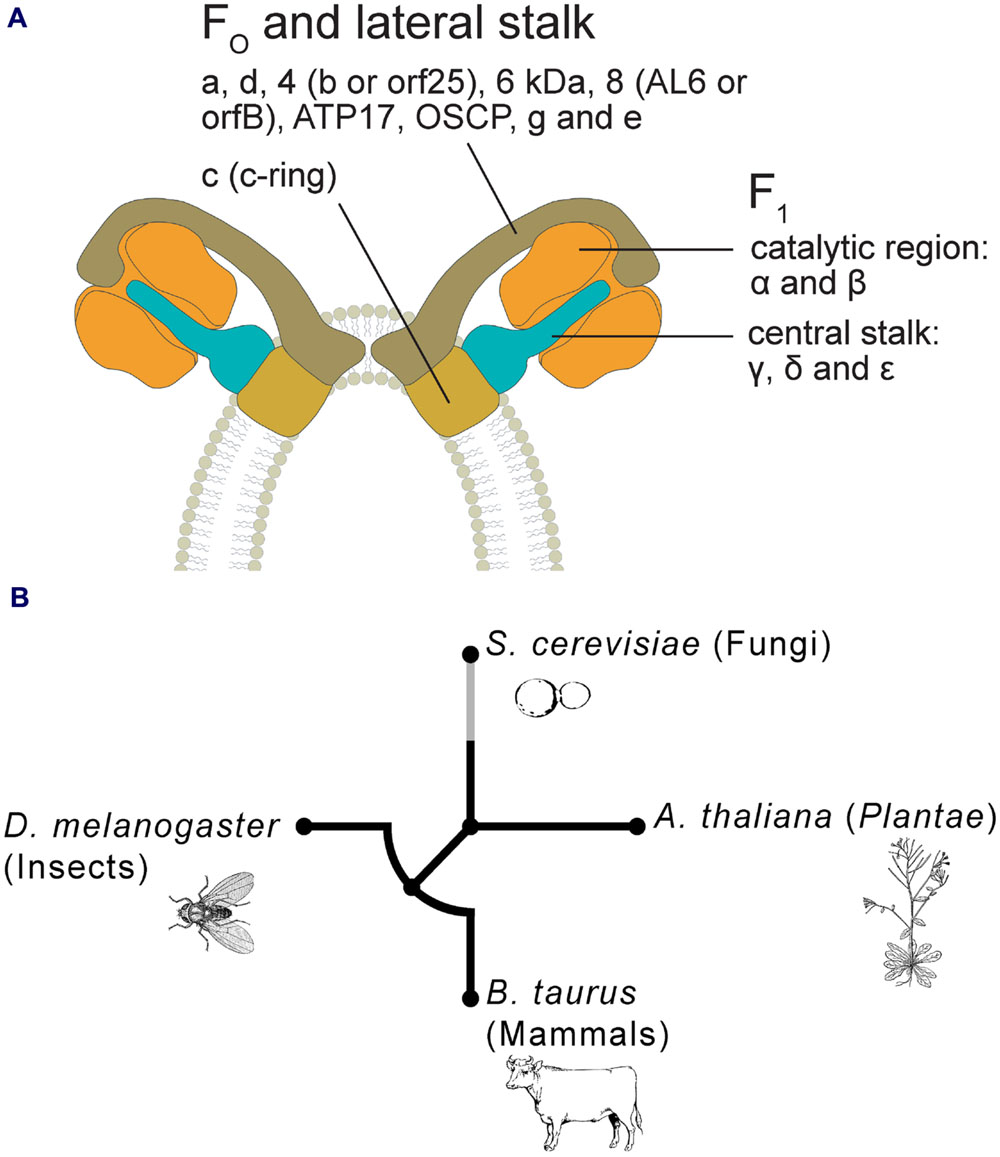
FIGURE 1. (A) Hypothetical model of PTP in plants, based on F-ATPase dimer formation, as proposed by Bernardi (2013b), Bonora et al. (2013), and Alavian et al. (2014). Plant F-ATPase subunits are organized on the basis of their putative correspondence to the mammalian ones. (B) Circular phylogenetic tree of peptide sequences of homologous subunit g of mitochondrial ATP synthase in four representative taxa (i.e., Bos taurus, Drosophila melanogaster, Saccharomyces cerevisiae, and Arabidopsis thaliana). Alignments of multiple amino acid sequences were performed using MUSCLE software (Edgar, 2004). Phylogenetic trees were obtained using phyML version 3.0 with the maximum-likelihood (ML) method (Guindon et al., 2010). The NCBI Reference Sequence accession codes for the g subunit are: B. taurus = NP_001019721; D. melanogaster = NP_609142; S. cerevisiae = NP_015345; A. thaliana = NP_179558. Where more isoforms were found in NCBI databases, we randomly selected only one of these sequences.
The F-ATPase dimer is present in eukaryotes, but not in prokaryotes, because the F-ATPase of the latter is lacking of some crucial subunits (e and g) involved in dimer formation (Antoniel et al., 2014). It is thus reasonable to assume that the dimer/PTP may be arisen after the endosymbiosis between an alpha-proteobacterium and an archaeon (Martin and Müller, 1998). At the beginning, these dimers could have transferred ATP from the endosymbiont to the cytoplasm of the host cell, because the former presumably did not have ATP/ADP transporters. PTP was then maintained to dissipate the protonmotive force, thus regulating both ATP synthesis and exchanges of solutes between the cytoplasm and the mitochondrial matrix.
The presence of F-ATPase dimer has been assessed in different evolutionary divergent eukaryotes, some of which exhibit mitochondrial PT, such as ‘Unikonts’ (Opisthokonts) and Plantae (Arpagaus et al., 2002; Giorgio et al., 2013; Carraro et al., 2014; von Stockum et al., 2015). To understand the phylogenesis of this structure/function, a cladogram has been generated by comparing the ancestral sequences of FO subunit g from bovine and Drosophila (animals), yeast (fungi, Ascomycetes), and Arabidopsis (Plantae) (Figure 1B). The tree suggests an early differentiation at higher taxonomical levels (supergroups): Plantae show the highest phylogenetic distance and within the Opisthokonts, mammals, and insects exhibit similar distances, whereas yeast shows a higher distance. These phylogenetic patterns are consistent with the main evolutionary life tree (e.g., Keeling et al., 2005).
It has been suggested that F-ATPase shows a progressive differentiation along the main steps of evolution. In turn, some features of PTP seem to be occurred independently from changes in ATP synthase. As an example, swelling of mitochondria occurs only in bovine (Bernardi et al., 2015) and in some plants (see Table 1), suggesting that PTP has been differently shaped by exaptation during the evolution. Hence, exaptation leading to PT seems to have occurred in diverse contexts during life history, depending on the molecular characteristics of F-ATPase structure and the specific requirements of the respective taxa.
Future Directions
The molecular nature of PTP in plants is still elusive. Further structural and functional studies are required to verify if F-ATPase dimers represent the channel associated to PT also in plants. This is needed to understand better the relationship between mitochondrial PT and PCD in plants.
Author Contributions
MZ and AV co-supervised the manuscript and co-wrote the article. VC, EP, CP, SP, AB, and EB co-wrote the article. VDC and FB performed the phylogenetic analyses and co-wrote the article.
Funding
This work was supported by the Italian Ministry of Education, University and Research (National Research Program PRIN2010CSJX4F) and the European Social Fund (Program 2007/2013).
Conflict of Interest Statement
The authors declare that the research was conducted in the absence of any commercial or financial relationships that could be construed as a potential conflict of interest.
Acknowledgments
We thank Paolo Bernardi (University of Padova), Markus Schwarzländer (University of Bonn), and Giovanna Lippe (University of Udine) for their critical reading of the manuscript and Manuela Antoniel (University of Padova) for help in drawing F-ATPase.
References
Akerman, K. E., and Moore, A. L. (1983). Phosphate dependent, ruthenium red insensitive Ca2+ uptake in mung bean mitochondria. Biochem. Biophys. Res. Commun. 114, 1176–1181. doi: 10.1016/0006-291X(83)90686-1
Alavian, K. N., Beutner, G., Lazrove, E., Sacchetti, S., Park, H.-A., Licznerski, P., et al. (2014). An uncoupling channel within the c-subunit ring of the F1FO ATP synthase is the mitochondrial permeability transition pore. Proc. Natl. Acad. Sci. U.S.A. 111, 10580–10585. doi: 10.1073/pnas.1401591111
Antoniel, M., Giorgio, V., Fogolari, F., Glick, G. D., Bernardi, P., and Lippe, G. (2014). The oligomycin-sensitivity conferring protein of mitochondrial ATP synthase: emerging new roles in mitochondrial pathophysiology. Int. J. Mol. Sci. 15, 7513–7536. doi: 10.3390/ijms15057513
Arpagaus, S., Rawyler, A., and Braendle, R. (2002). Occurrence and characteristics of the mitochondrial permeability transition in plants. J. Biol. Chem. 277, 1780–1787. doi: 10.1074/jbc.M109416200
Baines, C. P., Kaiser, R. A., Shelko, T., Craigen, W. J., and Molkentin, D. (2007). Voltage-dependent anion channels are dispensable for mitochondrial-dependent cell death. Nat. Cell Biol. 9, 550–555. doi: 10.1038/ncb1575
Barve, A., and Wagner, A. (2013). A latent capacity for evolutionary innovation through exaptation in metabolic systems. Nature 500, 203–206. doi: 10.1038/nature12301
Bernardi, P. (2013a). “Mitochondrial permeability transition pore,” in Encyclopedia of Biological Chemistry, ed. W. J. L. D. Lane (Waltham: Academic Press), 162–167.
Bernardi, P. (2013b). The mitochondrial permeability transition pore: a mystery solved? Front. Physiol. 4:95. doi: 10.3389/fphys.2013.00095
Bernardi, P., Lisa, F. D., Fogolari, F., and Lippe, G. (2015). From ATP to PTP and back: a dual function for the mitochondrial ATP synthase. Circ. Res. 116, 1850–1862. doi: 10.1161/CIRCRESAHA.115.306557
Bick, A. G., Calvo, S. E., and Mootha, V. K. (2012). Evolutionary diversity of the mitochondrial calcium uniporter. Science 336, 886–886. doi: 10.1126/science.1214977
Bonora, M., Bononi, A., De Marchi, E., Giorgi, C., Lebiedzinska, M., Marchi, S., et al. (2013). Role of the c subunit of the FO ATP synthase in mitochondrial permeability transition. Cell Cycle 12, 674–683. doi: 10.4161/cc.23599
Bultema, J. B., Braun, H.-P., Boekema, E. J., and Kouřil, R. (2009). Megacomplex organization of the oxidative phosphorylation system by structural analysis of respiratory supercomplexes from potato. Biochim. Biophys. Acta 1787, 60–67. doi: 10.1016/j.bbabio.2008.10.010
Campanella, M., Casswell, E., Chong, S., Farah, Z., Wieckowski, M. R., Abramov, A. Y., et al. (2008). Regulation of mitochondrial structure and function by the F1FO-ATPase inhibitor prorein, IF1. Cell Metab. 8, 13–25. doi: 10.1016/j.cmet.2008.06.001
Carraro, M., Giorgio, V., Šileikytė, J., Sartori, G., Forte, M., Lippe, G., et al. (2014). Channel formation by yeast F-ATP synthase and the role of dimerization in the mitochondrial permeability transition. J. Biol. Chem. 289, 15980–15985. doi: 10.1074/jbc.C114.559633
Cleary, J., Johnson, K. M., Opipari, A. W. Jr., and Glick, G. D. (2007). Inhibition of the mitochondrial F1FO-ATPase by ligands of the peripheral benzodiazepine receptor. Bioorg. Med. Chem. Lett. 17, 1667–1670. doi: 10.1016/j.bmcl.2006.12.102
Crompton, M., Ellinger, H., and Costi, A. (1988). Inhibition by cyclosporin A of a Ca2+-dependent pore in heart mitochondria activated by inorganic phosphate and oxidative stress. Biochem. J. 255, 357–360.
Curtis, M. J., and Wolpert, T. J. (2002). The oat mitochondrial permeability transition and its implication in victorin binding and induced cell death. Plant J. 29, 295–312. doi: 10.1046/j.0960-7412.2001.01213.x
Dabbeni-Sala, F., Rai, A. K., and Lippe, G. (2012). “F1FO ATP Synthase: a fascinating challenge for proteomics,” in Proteomics: Human Diseases and Protein Functions, eds T.-K. Man and R. J. Flores (Rijeka: InTech), 161–188.
Daum, B., Walter, A., Horst, A., Osiewacz, H. D., and Kühlbrandt, W. (2013). Age-dependent dissociation of ATP synthase dimers and loss of inner-membrane cristae in mitochondria. Proc. Natl. Acad. Sci. U.S.A. 110, 15301–15306. doi: 10.1073/pnas.1305462110
Davies, K. M., Anselmi, C., Wittig, I., Faraldo-Gómez, J. D., and Kühlbrandt, W. (2012). Structure of the yeast F1FO-ATP synthase dimer and its role in shaping the mitochondrial cristae. Proc. Natl. Acad. Sci. U.S.A. 109, 13602–13607. doi: 10.1073/pnas.1204593109
Davies, K. M., Strauss, M., Daum, B., Kief, J. H., Osiewacz, H. D., Rycovska, A., et al. (2011). Macromolecular organization of ATP synthase and complex I in whole mitochondria. Proc. Natl. Acad. Sci. U.S.A. 108, 14121–14126. doi: 10.1073/pnas.1103621108
Dell’Orto, P., Moenne, A., Vincent Graves, P., and Jordana, X. (1993). The potato mitochondrial ATP synthase subunit 9: gene structure, RNA editing and partial protein sequence. Plant Sci. 88, 45–53. doi: 10.1016/0168-9452(93)90108-C
de Oliveira, H. C., Saviani, E. E., de Oliveira, J. F. P., and Salgado, I. (2007). Cyclosporin A inhibits calcium uptake by Citrus sinensis mitochondria. Plant Sci. 172, 665–670. doi: 10.1016/j.plantsci.2006.12.002
Dieter, P., and Marme, D. (1980). Ca2+ transport in mitochondrial and microsomal fractions from higher plants. Planta 150, 1–8. doi: 10.1007/BF00385606
Douce, R. (1985). Mitochondria in Higher Plants: Structure, Function, and Biogenesis. New York, NY: Elsevier.
Edgar, R. C. (2004). MUSCLE: a multiple sequence alignment method with reduced time and space complexity. BMC Bioinformatics 5:113. doi: 10.1186/1471-2105-5-113
Eubel, H., Heinemeyer, J., Sunderhaus, S., and Braun, H.-P. (2004). Respiratory chain supercomplexes in plant mitochondria. Plant Physiol. Biochem. 42, 937–942. doi: 10.1016/j.plaphy.2004.09.010
Eubel, H., Jänsch, L., and Braun, H.-P. (2003). New insights into the respiratory chain of plant mitochondria. Supercomplexes and a unique composition of complex II. Plant Physiol. 133, 274–286. doi: 10.1104/pp.103.024620
Faccenda, D., Tan, C. H., Seraphim, A., Duchen, M. R., and Campanella, M. (2013). IF1 limits the apoptotic-signalling cascade by preventing mitochondrial remodeling. Cell Death Differ. 20, 686–697. doi: 10.1038/cdd.2012.163
Fortes, F., Castilho, R. F., Catisti, R., Carnieri, E. G. S., and Vercesi, A. E. (2001). Ca2+ induces a cyclosporin A-insensitive permeability transition pore in isolated potato tuber mitochondria mediated by reactive oxygen species. J. Bioenerg. Biomembr. 33, 43–51. doi: 10.1023/A:1005672623709
Gao, C., Xing, D., Li, L., and Zhang, L. (2008). Implication of reactive oxygen species and mitochondrial dysfunction in the early stages of plant programmed cell death induced by ultraviolet-C overexposure. Planta 227, 755–767. doi: 10.1007/s00425-007-0654-4
Giorgio, V., Bisetto, E., Soriano, M. E., Dabbeni-Sala, F., Basso, E., Petronilli, V., et al. (2009). Cyclophilin D modulates mitochondrial FOF1-ATP synthase by interacting with the lateral stalk of the complex. J. Biol. Chem. 284, 33982–33988. doi: 10.1074/jbc.M109.020115
Giorgio, V., von Stockum, S., Antoniel, M., Fabbro, A., Fogolari, F., Forte, M., et al. (2013). Dimers of mitochondrial ATP synthase form the permeability transition pore. Proc. Natl. Acad. Sci. U.S.A. 110, 5887–5892. doi: 10.1073/pnas.1217823110
Gould, S. J., and Vrba, E. S. (1982). Exaptation – A missing term in the science of form. Paleobiology 8, 4–15.
Guindon, S., Dufayard, J. F., Lefort, V., Anisimova, M., Hordijk, W., and Gascuel, O. (2010). New algorithms and methods to estimate maximum-likelihood phylogenies: assessing the performance of PhyML 3.0. Syst. Biol. 59, 307–321. doi: 10.1093/sysbio/syq010
Gutiérrez-Aguilar, M., Douglas, D. L., Gibson, A. K., Domeier, T. L., Molkentin, J. D., and Baines, C. P. (2014). Genetic manipulation of the cardiac mitochondrial phosphate carrier does not affect permeability transition. J. Mol. Cell. Cardiol. 72, 316–325. doi: 10.1016/j.yjmcc.2014.04.008
Halestrap, A. P., and Davidson, A. M. (1990). Inhibition of Ca2+-induced large-amplitude swelling of liver and heart mitochondria by cyclosporin is probably caused by the inhibitor binding to mitochondrial-matrix peptidyl-prolyl cis-trans isomerase and preventing it interacting with the adenine nucleotide translocase. Biochem. J. 268, 153–160. doi: 10.1042/bj2680153
Hamasur, B., and Glaser, E. (1990). FOF1-ATPase of plant mitochondria: isolation and polypeptide composition. Biochem. Biophys. Res. Commun. 170, 1352–1358. doi: 10.1016/0006-291X(90)90543-V
Hamasur, B., and Glaser, E. (1992). Plant mitochondrial FOF1 ATP synthase. Identification of the individual subunits and properties of the purified spinach leaf mitochondrial ATP synthase. Eur. J. Biochem. 205, 409–416. doi: 10.1111/j.1432-1033.1992.tb16794.x
Haworth, R. A., and Hunter, D. R. (1979). The Ca2+-induced membrane transition in mitochondria: II. Nature of the Ca2+ trigger site. Arch. Biochem. Biophys. 195, 460–467. doi: 10.1016/0003-9861(79)90372-2
Heazlewood, J. L., Whelan, J., and Millar, A. H. (2003). The products of the mitochondrial orf25 and orfB genes are FO components in the plant F1FO ATP synthase. FEBS Lett. 540, 201–205. doi: 10.1016/S0014-5793(03)00264-3
Huang, W., Yang, X., Yao, S., LwinOo, T., He, H., Wang, A., et al. (2014). Reactive oxygen species burst induced by aluminum stress triggers mitochondria-dependent programmed cell death in peanut root tip cells. Plant Physiol. Biochem. 82, 76–84. doi: 10.1016/j.plaphy.2014.03.037
Hunter, D. R., and Haworth, R. A. (1979a). The Ca2+-induced membrane transition in mitochondria: I. The protective mechanisms. Arch. Biochem. Biophys. 195, 453–459. doi: 10.1016/0003-9861(79)90371-0
Hunter, D. R., and Haworth, R. A. (1979b). The Ca2+-induced membrane transition in mitochondria: III. Transitional Ca2+ release. Arch. Biochem. Biophys. 195, 468–477. doi: 10.1016/0003-9861(79)90373-4
Jänsch, L., Kruft, V., Schmitz, U. K., and Braun, H.-P. (1996). New insights into the composition, molecular mass and stoichiometry of the protein complexes of plant mitochondria. Plant J. 9, 357–368. doi: 10.1046/j.1365-313X.1996.09030357.x
Jiko, C., Davies, K. M., Shinzawa-Itoh, K., Tani, K., Maeda, S., Mills, D. J., et al. (2015). Bovine F1FO ATP synthase monomers bend the lipid bilayer in 2D membrane crystals. Elife 4, e06119. doi: 10.7554/eLife.06119
Keeling, P. J., Burger, G., Durnford, D. G., Lang, B. F., Lee, R. W., Pearlman, R. E., et al. (2005). The tree of eukaryotes. Trends Ecol. Evol. 20, 670–676. doi: 10.1016/j.tree.2005.09.005
Klodmann, J., Senkler, M., Rode, C., and Braun, H.-P. (2011). Defining the protein complex proteome of plant mitochondria. Plant Physiol. 157, 587–598. doi: 10.1104/pp.111.182352
Kokoszka, J. E., Waymire, K. G., Levy, S. E., Sligh, J. E., Cai, J., Jones, D. P., et al. (2004). The ADP/ATP translocator is not essential for the mitochondrial permeability transition pore. Nature 427, 461–465. doi: 10.1038/nature02229
Krause, F., Reifschneider, N. H., Vocke, D., Seelert, H., Rexroth, S., and Dencher, N. A. (2004). “Respirasome”-like supercomplexes in green leaf mitochondria of spinach. J. Biol. Chem. 279, 48369–48375. doi: 10.1074/jbc.M406085200
Krause, M., and Durner, J. (2004). Harpin inactivates mitochondria in Arabidopsis suspension cells. Mol. Plant Microbe Interact. 17, 131–139. doi: 10.1094/MPMI.2004.17.2.131
Krauskopf, A., Eriksson, O., Craigen, W. J., Forte, M. A., and Bernardi, P. (2006). Properties of the permeability transition in VDAC1-/- mitochondria. Biochim. Biophys. Acta 1757, 590–595. doi: 10.1016/j.bbabio.2006.02.007
Lee, J., Ding, S., Walpole, T. B., Holding, A. N., Montgomery, M. G., Fearnley, I. M., et al. (2015). Organization of subunits in the membrane domain of the bovine F-ATPase revealed by covalent cross-linking. J. Biol. Chem. 290, 13308–13320. doi: 10.1074/jbc.M115.645283
Li, L., Carrie, C., Nelson, C., Whelan, J., and Millar, A. H. (2012). Accumulation of newly synthesized F1 in vivo in Arabidopsis mitochondria provides evidence for modular assembly of the plant F1FO ATP synthase. J. Biol. Chem. 287, 25749–25757. doi: 10.1074/jbc.M112.373506
Li, Z., and Xing, D. (2011). Mechanistic study of mitochondria-dependent programmed cell death induced by aluminium phytotoxicity using fluorescence techniques. J. Exp. Bot. 62, 331–343. doi: 10.1093/Jxb/Erq279
Lin, J., Wang, Y., and Wang, G. (2006). Salt stress-induced programmed cell death in tobacco protoplasts is mediated by reactive oxygen species and mitochondrial permeability transition pore status. J. Plant Physiol. 163, 731–739. doi: 10.1016/j.jplph.2005.06.016
Liu, S., Charlesworth, T. J., Bason, J. V., Montgomery, M. G., Harbour, M. E., Fearnley, I. M., et al. (2015). The purification and characterization of ATP synthase complexes from the mitochondria of four fungal species. Biochem. J. 468, 167–175. doi: 10.1042/BJ20150197
Logan, D. C., and Knight, M. R. (2003). Mitochondrial and cytosolic calcium dynamics are differentially regulated in plants. Plant Physiol. 133, 21–24. doi: 10.1104/pp.103.026047
Lord, C. E. N., Dauphinee, A. N., Watts, R. L., and Gunawardena, A. H. L. A. N. (2013). Unveiling interactions among mitochondria, caspase-like proteases, and the actin cytoskeleton during plant programmed cell death (PCD). PLoS ONE 8:e57110. doi: 10.1371/journal.pone.0057110
Loro, G., and Costa, A. (2013). Imaging of mitochondrial and nuclear Ca2+ dynamics in Arabidopsis roots. Cold Spring Harb. Protoc. 8, 781–785. doi: 10.1101/pdb.prot073049
Lu, K. P., Finn, G., Lee, T. H., and Nicholson, L. K. (2007). Prolyl cis-trans isomerization as a molecular timer. Nat. Chem. Biol. 3, 619–629. doi: 10.1038/nchembio.2007.35
Lyubushkina, I. V., Grabelnych, O. I., Pobezhimova, T. P., Stepanov, A. V., Fedyaeva, A. V., Fedoseeva, I. V., et al. (2014). Winter wheat cells subjected to freezing temperature undergo death process with features of programmed cell death. Protoplasma 251, 615–623. doi: 10.1007/s00709-013-0562-3
Manzoor, H., Chiltz, A., Madani, S., Vatsa, P., Schoefs, B., Pugin, A., et al. (2012). Calcium signatures and signaling in cytosol and organelles of tobacco cells induced by plant defense elicitors. Cell Calcium 51, 434–444. doi: 10.1016/j.ceca.2012.02.006
Martin, W., and Müller, M. (1998). The hydrogen hypothesis for the first eukaryote. Nature 392, 37–41. doi: 10.1038/32096
Martins, I. S., and Vercesi, A. E. (1985). Some characteristics of Ca2+ transport in plant mitochondria. Biochem. Biophys. Res. Commun. 129, 943–948. doi: 10.1016/0006-291x(85)91982-5
Meyer, E. H., Taylor, N. L., and Millar, A. H. (2008). Resolving and identifying protein components of plant mitochondrial respiratory complexes using three dimensions of gel electrophoresis. J. Proteome Res. 7, 786–794. doi: 10.1021/pr700595p
Mitchell, P. (1961). Coupling of phosphorylation to electron and hydrogen transfer by a chemi-osmotic type of mechanism. Nature 191, 144–148. doi: 10.1038/191144a0
Morikami, A., Aiso, K., Asahi, T., and Nakamura, K. (1992). The delta’-subunit of higher plant six-subunit mitochondrial F1-ATPase is homologous to the delta-subunit of animal mitochondrial F1-ATPase. J. Biol. Chem. 267, 72–76.
Nigro, P., Pompilio, G., and Capogrossi, M. C. (2013). Cyclophilin A: a key player for human disease. Cell Death Dis. 4, e888. doi: 10.1038/cddis.2013.410
Nomura, H., and Shiina, T. (2014). Calcium signaling in plant endosymbiotic organelles: mechanism and role in physiology. Mol. Plant 7, 1094–1104. doi: 10.1093/Mp/Ssu020
Polgreen, K. E., Featherstone, J., Willis, A. C., and Harris, D. A. (1995). Primary structure and properties of the inhibitory protein of the mitochondrial ATPase (H+-ATP synthase) from potato. Biochim. Biophys. Acta 1229, 175–180. doi: 10.1016/0005-2728(94)00193-9
Qi, Y., Wang, H., Zou, Y., Liu, C., Liu, Y., Wang, Y., et al. (2011). Over-expression of mitochondrial heat shock protein 70 suppresses programmed cell death in rice. FEBS Lett. 585, 231–239. doi: 10.1016/j.febslet.2010.11.051
Raaflaub, J. (1953a). Mechanism of adenosinetriphosphate as cofactor of isolated mitochondria. Helv. Physiol. Pharmacol. Acta 11, 157–165.
Raaflaub, J. (1953b). Swelling of isolated mitochondria of the liver and their susceptibility to physicochemical influences. Helv. Physiol. Pharmacol. Acta 11, 142–156.
Reape, T. J., Kacprzyk, J., Brogan, N., Sweetlove, L., and McCabe, P. F. (2015). Mitochondrial markers of programmed cell death in Arabidopsis thaliana. Methods Mol. Biol. 1305, 211–221. doi: 10.1007/978-1-4939-2639-8_15
Rikhvanov, E. G., Fedoseeva, I. V., Pyatrikas, D. V., Borovskii, G. B., and Voinikov, V. K. (2014). Role of mitochondria in the operation of calcium signaling system in heat-stressed plants. Russ. J. Plant Physiol. 61, 141–153. doi: 10.1134/S1021443714020125
Sarkar, P., and Gladish, D. K. (2012). Hypoxic stress triggers a programmed cell death pathway to induce vascular cavity formation in Pisum sativum roots. Physiol. Plant. 146, 413–426. doi: 10.1111/j.1399-3054.2012.01632.x
Schwarzländer, M., Logan, D. C., Johnston, I. G., Jones, N. S., Meyer, A. J., Fricker, M. D., et al. (2012). Pulsing of membrane potential in individual mitochondria: a stress-induced mechanism to regulate respiratory bioenergetics in Arabidopsis. Plant Cell 24, 1188–1201. doi: 10.1105/tpc.112.096438
Scott, I., and Logan, D. C. (2008). Mitochondrial morphology transition is an early indicator of subsequent cell death in Arabidopsis. New Phytol. 177, 90–101. doi: 10.1111/j.1469-8137.2007.02255.x
Seelert, H., and Dencher, N. A. (2011). ATP synthase superassemblies in animals and plants: two or more are better. Biochim. Biophys. Acta 1807, 1185–1197. doi: 10.1016/j.bbabio.2011.05.023
Šileikytė, J., Blachly-Dyson, E., Sewell, R., Carpi, A., Menabò, R., Lisa, F. D., et al. (2014). Regulation of the mitochondrial permeability transition pore by the outer membrane does not involve the peripheral benzodiazepine receptor (Translocator Protein of 18 kDa (TSPO)). J. Biol. Chem. 289, 13769–13781. doi: 10.1074/jbc.M114.549634
Silva, M. A. P., Carnieri, E. G. S., and Vercesi, A. E. (1992). Calcium-transport by corn mitochondria – evaluation of the role of phosphate. Plant Physiol. 98, 452–457. doi: 10.1104/Pp.98.2.452
Smith, M. K., Day, D. A., and Whelan, J. (1994). Isolation of a novel soybean gene encoding a mitochondrial ATP synthase subunit. Arch. Biochem. Biophys. 313, 235–240. doi: 10.1006/abbi.1994.1382
Stael, S., Wurzinger, B., Mair, A., Mehlmer, N., Vothknecht, U. C., and Teige, M. (2012). Plant organellar calcium signalling: an emerging field. J. Exp. Bot. 63, 1525–1542. doi: 10.1093/jxb/err394
Subbaiah, C. C., Bush, D. S., and Sachs, M. M. (1998). Mitochondrial contribution to the anoxic Ca2+ signal in maize suspension-cultured cells. Plant Physiol. 118, 759–771. doi: 10.1104/Pp.118.3.759
Szabó, I., and Zoratti, M. (1992). The mitochondrial megachannel is the permeability transition pore. J. Bioenerg. Biomembr. 24, 111–117. doi: 10.1007/BF00769537
Van Hautegem, T., Waters, A. J., Goodrich, J., and Nowack, M. K. (2015). Only in dying, life: programmed cell death during plant development. Trends Plant Sci. 20, 102–113. doi: 10.1016/j.tplants.2014.10.003
van Lis, R., Mendoza-Hernández, G., Groth, G., and Atteia, A. (2007). New insights into the unique structure of the FOF1-ATP synthase from the chlamydomonad algae Polytomella sp. and Chlamydomonas reinhardtii. Plant Physiol. 144, 1190–1199. doi: 10.1104/pp.106.094060
Vázquez-Acevedo, M., Cardol, P., Cano-Estrada, A., Lapaille, M., Remacle, C., and González-Halphen, D. (2006). The mitochondrial ATP synthase of chlorophycean algae contains eight subunits of unknown origin involved in the formation of an atypical stator-stalk and in the dimerization of the complex. J. Bioenerg. Biomembr. 38, 271–282. doi: 10.1007/s10863-006-9046-x
Vianello, A., Casolo, V., Petrussa, E., Peresson, C., Patui, S., Bertolini, A., et al. (2012). The mitochondrial permeability transition pore (PTP) — An example of multiple molecular exaptation? Biochim. Biophys. Acta 1817, 2072–2086. doi: 10.1016/j.bbabio.2012.06.620
Vianello, A., Macrì, F., Braidot, E., and Mokhova, E. (1995). Effect of cyclosporin A on energy coupling in pea stem mitochondria. FEBS Lett. 371, 258–260. doi: 10.1016/0014-5793(95)00897-I
Vianello, A., Zancani, M., Peresson, C., Petrussa, E., Casolo, V., Krajnakova, J., et al. (2007). Plant mitochondrial pathway leading to programmed cell death. Physiol. Plant. 129, 242–252. doi: 10.1111/j.1399-3054.2006.00767.x
Virolainen, E., Blokhina, O., and Fagerstedt, K. (2002). Ca2+-induced high amplitude swelling and cytochrome c release from wheat (Triticum aestivum L.) mitochondria under anoxic stress. Ann. Bot. 90, 509–516. doi: 10.1093/Aob/Mcf221
von Stockum, S., Giorgio, V., Trevisan, E., Lippe, G., Glick, G. D., Forte, M. A., et al. (2015). F-ATPase of Drosophila melanogaster forms 53-pS channels responsible for mitochondrial Ca2+-induced Ca2+ release. J. Biol. Chem. 290, 4537–4544. doi: 10.1074/jbc.C114.629766
Wagner, S., Behera, S., De Bortoli, S., Logan, D. C., Fuchs, P., Carraretto, L., et al. (2015). The EF-hand Ca2+ binding protein MICU choreographs mitochondrial Ca2+ dynamics in Arabidopsis. Plant Cell (in press). doi: 10.1105/tpc.15.00509
Yamauchi, T., Shimamura, S., Nakazono, M., and Mochizuki, T. (2013). Aerenchyma formation in crop species: a review. Field Crops Res. 152, 8–16. doi: 10.1016/j.fcr.2012.12.008
Yao, N., Eisfelder, B. J., Marvin, J., and Greenberg, J. T. (2004). The mitochondrion – an organelle commonly involved in programmed cell death in Arabidopsis thaliana. Plant J. 40, 596–610. doi: 10.1111/j.1365-313X.2004.02239.x
Yeh, C.-M., Chien, P.-S., and Huang, H.-J. (2007). Distinct signalling pathways for induction of MAP kinase activities by cadmium and copper in rice roots. J. Exp. Bot. 58, 659–671. doi: 10.1093/jxb/erl240
Zhang, L., Li, Y., Xing, D., and Gao, C. (2009). Characterization of mitochondrial dynamics and subcellular localization of ROS reveal that HsfA2 alleviates oxidative damage caused by heat stress in Arabidopsis. J. Exp. Bot. 60, 2073–2091. doi: 10.1093/jxb/erp078
Keywords: permeability transition, plant mitochondria, ATP synthase, exaptation, environmental stress
Citation: Zancani M, Casolo V, Petrussa E, Peresson C, Patui S, Bertolini A, De Col V, Braidot E, Boscutti F and Vianello A (2015) The Permeability Transition in Plant Mitochondria: The Missing Link. Front. Plant Sci. 6:1120. doi: 10.3389/fpls.2015.01120
Received: 30 September 2015; Accepted: 26 November 2015;
Published: 15 December 2015.
Edited by:
Ildikò Szabò, University of Padova, ItalyCopyright © 2015 Zancani, Casolo, Petrussa, Peresson, Patui, Bertolini, De Col, Braidot, Boscutti and Vianello. This is an open-access article distributed under the terms of the Creative Commons Attribution License (CC BY). The use, distribution or reproduction in other forums is permitted, provided the original author(s) or licensor are credited and that the original publication in this journal is cited, in accordance with accepted academic practice. No use, distribution or reproduction is permitted which does not comply with these terms.
*Correspondence: Marco Zancani, bWFyY28uemFuY2FuaUB1bml1ZC5pdA==