- 1Metallurgical and Materials Engineering Department, The University of Texas at El Paso, El Paso, TX, USA
- 2Chemistry Department, The University of Texas at El Paso, El Paso, TX, USA
- 3University of California Center for Environmental Implications of Nanotechnology – The University of Texas at El Paso, El Paso, TX, USA
Reports indicate that silver nanoparticles (nAg) are toxic to vegetation, but little is known about their effects in crop plants. This study examines the impacts of nAg on the physiology and nutritional quality of radish (Raphanus sativus) sprouts. Seeds were germinated and grown for 5 days in nAg suspensions at 0, 125, 250, and 500 mg/L. Seed germination and seedling growth were evaluated with traditional methodologies; the uptake of Ag and nutrients was quantified by inductively coupled plasma-optical emission spectroscopy (ICP-OES) and changes in macromolecules were analyzed by infrared (IR) spectroscopy. None of the nAg concentrations reduced seed germination. However, the water content (% of the total weight) was reduced by 1.62, 1.65, and 2.54% with exposure to 125, 250, and 500 mg/L, respectively, compared with the control. At 500 mg/L, the root and shoot lengths were reduced by 47.7 and 40%, with respect to the control. The seedlings exposed to 500 mg/L had 901 ± 150 mg Ag/kg dry wt and significantly less Ca, Mg, B, Cu, Mn, and Zn, compared with the control. The infrared spectroscopy analysis showed changes in the bands corresponding to lipids (3000–2800 cm-1), proteins (1550–1530 cm-1), and structural components of plant cells such as lignin, pectin, and cellulose. These results suggest that nAg could significantly affect the growth, nutrient content and macromolecule conformation in radish sprouts, with unknown consequences for human health.
Introduction
The “Nano-Era” started in the late 1990’s propelled by the worldwide increase in government investments into nanomaterials (NMs) and their applications. In the United States, the National Nanotechnology Initiative, created at the beginning of 2000, had coordinated the research and development of nanotechnology (Roco, 2003). Since then, a number of carbon-based and metal-based NMs have been produced and are currently being used in many fields. NMs are commonly referred to as small objects with one or more external dimensions in the size range 1–100 nm. At these dimensions, materials exhibit a distinctive behavior in comparison to larger particles of the same composition. Silver is an important component in the area of NMs, with over 450 metric tons of silver nanoparticles (nAg) produced in 2010 (Keller et al., 2013). In November 2014, fifty percent of the nano-enabled products on the “Project on Emerging Nanotechnologies” inventory contained nAg1. Among other goods, listed products included wound dressings with bactericidal effects that enhance healing, antibacterial door knobs and anti-odor socks; all articles containing nAg that provide antimicrobial properties (Kim et al., 2007). At some point, it is assumed that all the nanoparticles (NPs) in different items will end up in the soil, air or water (Keller et al., 2013). Even though some studies have reported the effects of nAg in the environment (Daniel et al., 2010; Beer et al., 2012), their toxicity on crop plants is not yet well understood. Previous investigations indicate that the effects of nAg on seed germination vary with NP characteristics and plant species. Kumari et al. (2009) reported that nAg decrease the mitotic index and cause multiple chromosomal breaks and cell disintegration in onion (Allium cepa). Stampoulis et al. (2009) found that nAg at 500 and 100 mg/L reduced plant biomass and transpiration in zucchini (Cucurbita pepo) by 57 and 41%, respectively. In another experiment, C. pepo sp. ovifera was exposed in hydroponics to 0, 100, and 500 mg nAg/L and corresponding bulk Ag (Ag powder). Results showed more negative effects in plants exposed to nAg than bulk Ag (Musante and White, 2012). It has also been observed that nAg reduced growth in mung bean (Phaseolus radiatus) and sorghum (Sorghum bicolor) cultivated in soil or agar-based medium (Lee et al., 2012). In addition, Pokhrel and Dubey (2013) found that coated nAg promoted histological changes in maize (Zea mays L.) by inducing elongation of root cells, and reduced root growth in cabbage (Brassica oleracea var. capitata L.) by 24%. In tomato (Solanum lycopersicum), nAg did not affect germination even at 5000 mg/L (Song et al., 2013). Thuesombat et al. (2014) reported that the toxicity of nAg in rice (Oryza sativa cv. KDML 105) increased directly with the particle size and concentration. A more recent report indicates that nAg at a concentration of 100 mg/L reduced germination in Brassica nigra (Amooaghaie et al., 2015). However, the effects of nAg on radish have not been reported yet. Radish (Raphanus sativus L.) sprouts are widely consumed worldwide due to their nutritional content and antioxidant properties (Xiao et al., 2014; Baenas et al., 2015). More than two decades ago, radish was proposed as a model plant for the study of environmental stresses, mainly atmospheric contaminants (Kostka-Rick and Manning, 1993). More recently, due to its short growing period, this plant has been considered as a model of edible roots for the study of the interaction of plants with soil contaminants (Létondor et al., 2015). A few reports have shown different responses of radish seedlings exposed to NMs. Ma et al. (2010) reported that nLa2O3, nGd2O3, and nYb2O3 at 2000 mg/L inhibited root elongation. However, Trujillo-Reyes et al. (2013) found that citric acid coated nCeO2, at 200 mg/L, increased root biomass and seedlings’ water content. In addition, Corral-Diaz et al. (2014) reported that nCeO2 at 250 mg/kg soil increased radish tubers’ antioxidant capacity. There is concern about the trophic transfer of NPs from edible plants into the food chain (Gardea-Torresdey et al., 2014). The present investigation addresses the effects of a nAg suspension, intended for human ingestion, in a terrestrial plant. In this study, radish seeds were exposed to different nAg concentrations from a commercially available nAg suspension to test its effects on radish sprouts. The marketed nAg product, at 500 mg/L per serving, is indicated as a dietary supplement for immune support2. Even though the environmental concentrations of nAg are lower than the amounts used for this study (Gottschalk et al., 2013), we chose 500 mg/L as the highest concentration for the experiment, assuming the worst case scenario for this product. The effects on seedlings’ development, nutrient uptake and changes of macromolecules were studied by using spectrophotometric analytical techniques.
Materials and Methods
Silver Nanoparticles and Radish Seeds
Silver nanoparticles (nAg) from Natural Path/Silver Wings (Nashville, TN, USA) came suspended in deionized water at 500 mg/L. According to the manufacturer, the majority of the nAg are 2 nm in size forming colloids in the range of 1-10 nm. The hydrodynamic size of the suspended particles in water and the zeta potential (ζ, the electrostatic charge between particles) was analyzed by dynamic light scattering (DLS) using a Malvern Zetasizer (Nano-ZS90, Malvern Instruments, UK). Radish (Champion variety) seeds were obtained from Del Norte Seeds and Feed (Vinton, TX, USA).
Seed Germination
Thirty seeds were directly incubated without previous treatment in sterilized standard Petri dishes (10 cm diameter) over germination paper, modified from López-Moreno et al. (2010). Treatments consisted of nAg suspensions at 0 (control), 125, 250, and 500 mg/L; four replicates per treatment. The concentrations for the study were selected considering that the worst case scenario at which plants could be exposed is the commercially available product of nAg at 500 mg/L. Suspensions were prepared by diluting the stock suspension of 500 mg/L (as supplied by the vendor) with Millipore water (18 MΩ cm). We utilized Millipore water for the experiments because it has similar resistivity than deionized water (Yin et al., 2012). Aliquots of five milliliters of nAg suspension were administered to each Petri dish, except for control seeds that received five milliliters of Millipore water. The dishes containing the seeds were covered with aluminum foil for 3 days. Then, they were set into a growth chamber (Environmental Growth Chamber, Chagrin Falls, OH, USA), where seedlings grew for a total of 5 days before analysis. Environmental conditions inside the chamber were 25/20°C day/night temperature, 14/10 h light/dark photoperiod, 60 ± 3% relative humidity, and 340 μmol/m2s light intensity. The percent germination (%G), relative germination (%RG), and germination change (%GC) were calculated as per de la Rosa et al. (2011). The length of the roots and shoots was measured on 15 plants per replicate. Water content, fresh and dry weights (dry wt) were also determined on 15 plants per replicate.
Elemental Analysis
At harvest, seedlings were washed with 0.01 M HNO3 and rinsed with Millipore water to remove the nAg adhered to tissues. After washing, seedlings were oven dried at 70°C for 72 h (Corral-Diaz et al., 2014). Dried samples were prepared for analysis, according to the EPA method 3051. Briefly, the seedlings were powdered using mortar and pestle and acid digested (0.1 g per sample) with a 1:4 ratio of HNO3:H2O2 in a microwave oven (MarsX, CEM Mathews, NC, USA). Digested samples were placed in 15 mL polypropylene centrifuge tubes and the final volume was adjusted to 15 mL. The digests were analyzed for macronutrients, micronutrients, and Ag content by using inductively coupled plasma-optical emission spectroscopy (ICP-OES, Perkin Elmer Optima 4300 DV, Shelton, CT, USA). For quality assurance/quality control, a standard of 0.5 mg/L from the calibration curve that contained Ag, micro and macro elements was analyzed every five samples.
FT-IR Analysis
Changes in lipids, proteins, carbohydrates and other organic polymers (e.g., lignin) were studied by using Fourier transform infrared (FT-IR) spectroscopy. The sprouts were washed with 0.01 M HNO3 and rinsed with Millipore water, separated into roots, stems and leaves and oven dried at 70°C for at least 72 h. Samples of roots, stems, and leaves were analyzed by using a Perkin-Elmer, Spectrum 100 with a Universal Attenuated Total Reflectance (ATR) sampling accessory. Background correction was performed by acquiring a spectrum without sample. Powdered samples were placed on the spectrometer stand and spectra were recorded. The data was collected in a frequency range from 4000 to 600 cm-1 at a resolution of 1 cm-1 and three scans per reading. Results are averages of triplicate determinations, as similarly reported by Servin et al. (2013). The amide I peak is commonly taken as an internal standard to normalize biological samples’ spectra (Yu and Irudayaraj, 2005). Data was normalized at wavenumber 1650 cm-1 for roots, stems, and leaves. This allowed us to compare the spectra within the same plant tissue at different nAg concentrations.
Statistical Analysis
Data was analyzed using the Statistical Package for the Social Sciences 20.0 (SPSS, Chicago, IL, USA). Variance was evaluated by one-way analysis of variance (one-way ANOVA) and the difference between treatment means was compared by Tukey’s honest significant difference (Tukey’s HSD) test at a p-value of 0.05, unless otherwise stated. Regression analysis was performed on growth data.
Results
Characterization and Effects of nAg on Seed Germination
The nAg suspended in Millipore water had (at the highest concentration) a hydrodynamic size of 77 ± 2.44 nm and a zeta potential (ζ) of -24.4 ± 12.6 mV. Results suggest that nAg may be aggregating given that the manufacturer specifications indicate a colloid size of 1–10 nm. Note that our recorded dimensions (77 ± 2.44 nm) include any layer that forms around the nAg due to interactions with the aqueous media, where the inorganic complex is suspended (Supplementary Figure S1).
Supplementary Figure S2 shows an overall view of the experimental setup, while Supplementary Figure S3 shows enlarged views of one Petri dish/treatment. The germination percent and changes in germination of radish seeds exposed to nAg are shown in Table 1. As seen in this table, at 125 mg/L nAg increased the germination by 3%, while at 250 and 500 mg/L reduced the germination by 3 and 6%, respectively. However, none of the treatments reached a statistical significant difference in comparison to the control.
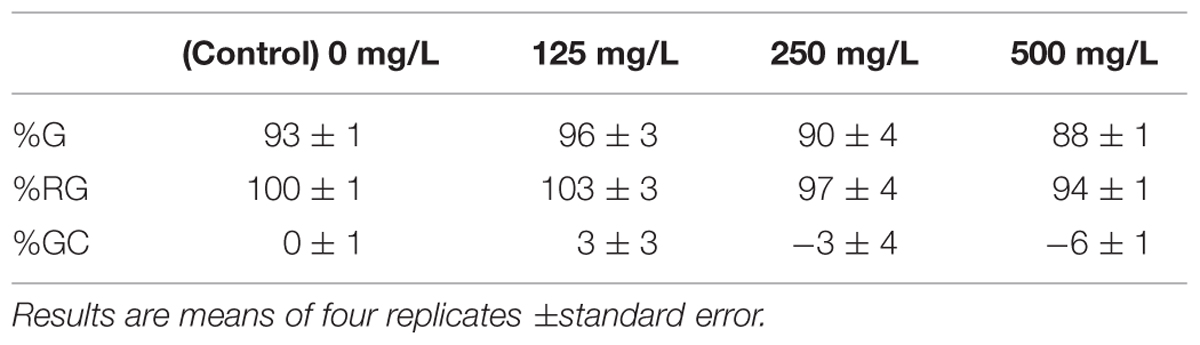
TABLE 1. Germination percent (%G), relative germination percent (%RG) and germination change (GC%) of radish seeds after 5 days of incubation in nAg suspension at 0, 125, 250, and 500 mg/L.
Seedling Growth, Silver Uptake, Biomass Production, and Water Content
Figure 1 shows the root and shoot elongation, dry biomass production, water content, and Ag concentration in seedlings exposed to nAg at 0 (control), 125, 250, and 500 mg/L. Individual images of seedlings from the different treatments are shown in Supplementary Figure S4. As seen in Figure 1A, accumulation of Ag was concentration dependent, but there was no difference between 125 and 250 mg/L treatments (114 and 204 mg Ag/kg dry tissue, respectively). However, at 500 mg/L, Ag accumulation was significantly higher (900 mg Ag/kg dry tissue) compared to the other treatments (p ≤ 0.05).
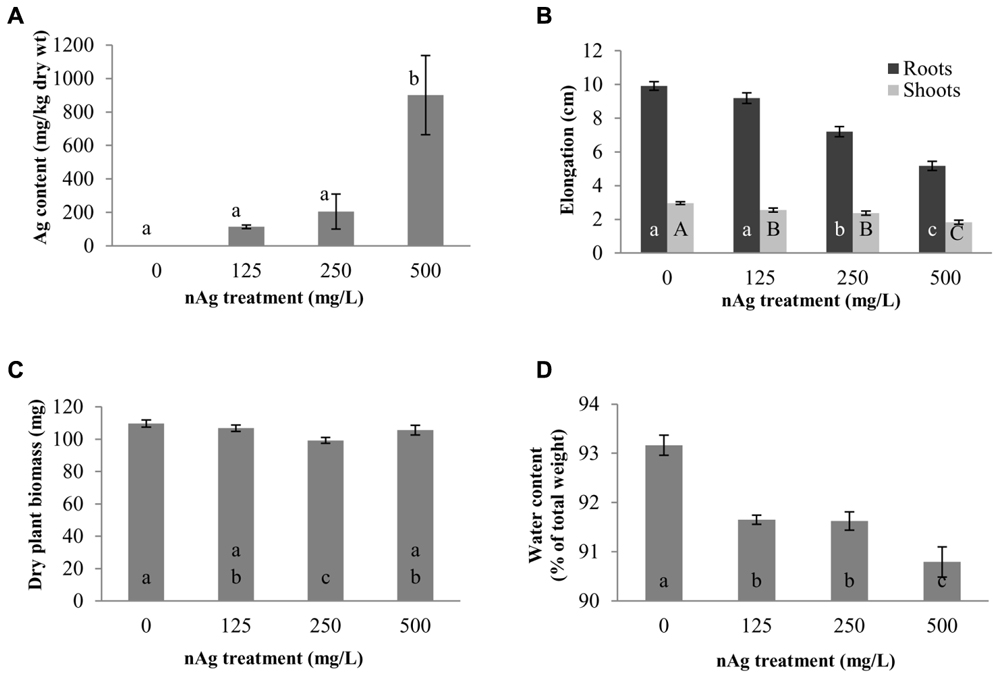
FIGURE 1. Concentration of Ag, mg per kg of dry plant tissue (A), root and shoot length (B), dry biomass (C), and water content (D) in radish seedlings exposed for 5 days to nAg at 0 (control), 125, 250, and 500 mg/L. Values are means of four replicates per treatment (15 plants each replicate) ± standard error. Different letters denote statistically significant differences according to the Tukey’s HSD test (p < 0.05). In (B), small case letters are for roots and upper case letters for shoots.
The elongation of roots and shoots is shown in Figure 1B. As shown in this figure, there was a concentration-dependent reduction in root elongation (r2 = 0.9626) that reached statistical significance in seedlings exposed to 250 and 500 mg/L with respect to control. The percent reductions in the two treatments were 27.3 and 47.7%, respectively. In addition, the root length of seedlings exposed to 500 mg/L (5.2 cm) was statistically lower compared to the length of roots exposed to 250 mg/L (7.2 cm). Figure 1B also shows the shoot length of radish seedlings. Similar to root length, there was a concentration-dependent significant reduction (r2 = 0.9677) in shoot elongation. However, in the case of shoots, all nAg concentrations significantly reduced shoot elongation, although the reduction at 125 and 250 mg/L was statistically similar. At 500 mg/L nAg exposure, the reduction in shoot length reached 38%.
The effects of nAg on dry biomass are shown in Figure 1C. Plants exposed to 250 mg/L had less biomass, compared with control and the other treatments. The reduction was about 10%, compared with control, and about 7% compared with the other treatments.
Water content of the whole seedlings is shown in Figure 1D. As shown in this figure, the water content (expressed as % of the total seedlings’ weight) was reduced by 1.62, 1.65, and 2.54% with exposure to 125, 250, and 500 mg/L, respectively, compared with the control. In all cases the differences were statistically significant, compared with the control; however, there were no differences between the 125 and 250 mg/L treatments (p ≤ 0.05).
Effects of nAg on Macro and Micronutrient Accumulation
Figure 2 shows the concentration of elements that were affected by nAg in the whole seedling. Amongst macroelements, only Ca and Mg were significantly reduced by the highest concentrations of nAg (Figure 2A). Calcium was reduced by 20 and 33% and Mg by 10 and 19% at 250 and 500 mg/L, respectively, compared with control.
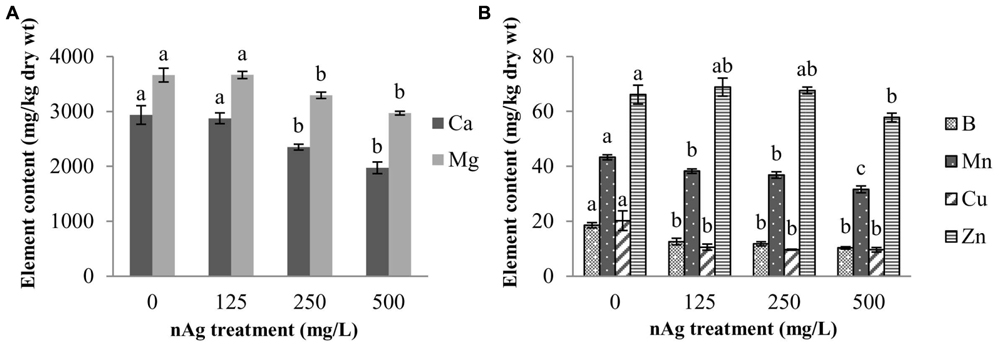
FIGURE 2. Concentration of macroelements (A) and microelements (B) in radish seedlings germinated and grown for 5 days in nAg suspensions at 0 (control), 125, 250, and 500 mg/L. Values are means of four replicates per treatment (15 plants each) ± standard error. Different letters denote statistically significant differences according to the Tukey’s HSD test (p < 0.05).
With regard to micronutrient absorption, none of the nAg concentrations impaired the absorption of Fe, Ni, and Mo. However, all treatments significantly reduced (p ≤ 0.05) absorption of B, Mn, and Cu, while Zn was only reduced at 500 mg/L, compared with control (Figure 2B). Percent reductions at 125, 250, and 500 mg/L were 32, 36.8, and 44.6% for B; 11.6, 14.8, and 26.9% for Mn, and 47.5, 52.5, and 52.5% for Cu, respectively. Zinc was reduced by 12.6% at the highest concentration treatment.
FT-IR Analysis of Roots, Stems, and Leaves
Fourier transform infrared spectroscopy has been used to identify conformational changes in the macromolecules of plants exposed to contaminants, including NPs. Table 2 provides a summary of previously compiled FT-IR data from plant samples that relates the functional groups identified and the macromolecules involved (Dokken and Davis, 2007; Lammers et al., 2009; Rico et al., 2015). For comparison purposes, we chose specific spectral regions where lipids (3000–2800 cm-1), proteins and lignin (1700–1500 cm-1), lipids and pectin (1790–1720 cm-1) cellulose, and hemicellulose (1300–1180 cm-1) and other carbohydrates (fingerprint region 1200–900 cm-1) are presumably found. Changes in the FT-IR spectra of radish seedlings (Figures 3–5) were compared with the data shown in Table 2. There are no apparent band shifts in the FT-IR spectra of the roots, stems or leaves treated with nAg at different concentrations as seen in Figures 3–5. However, changes in band intensities were found in all studied regions.
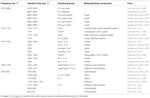
TABLE 2. Summary of FT-IR band frequencies found in plants exposed to NPs and other contaminants (Dokken and Davis, 2007; Lammers et al., 2009; Rico et al., 2015).
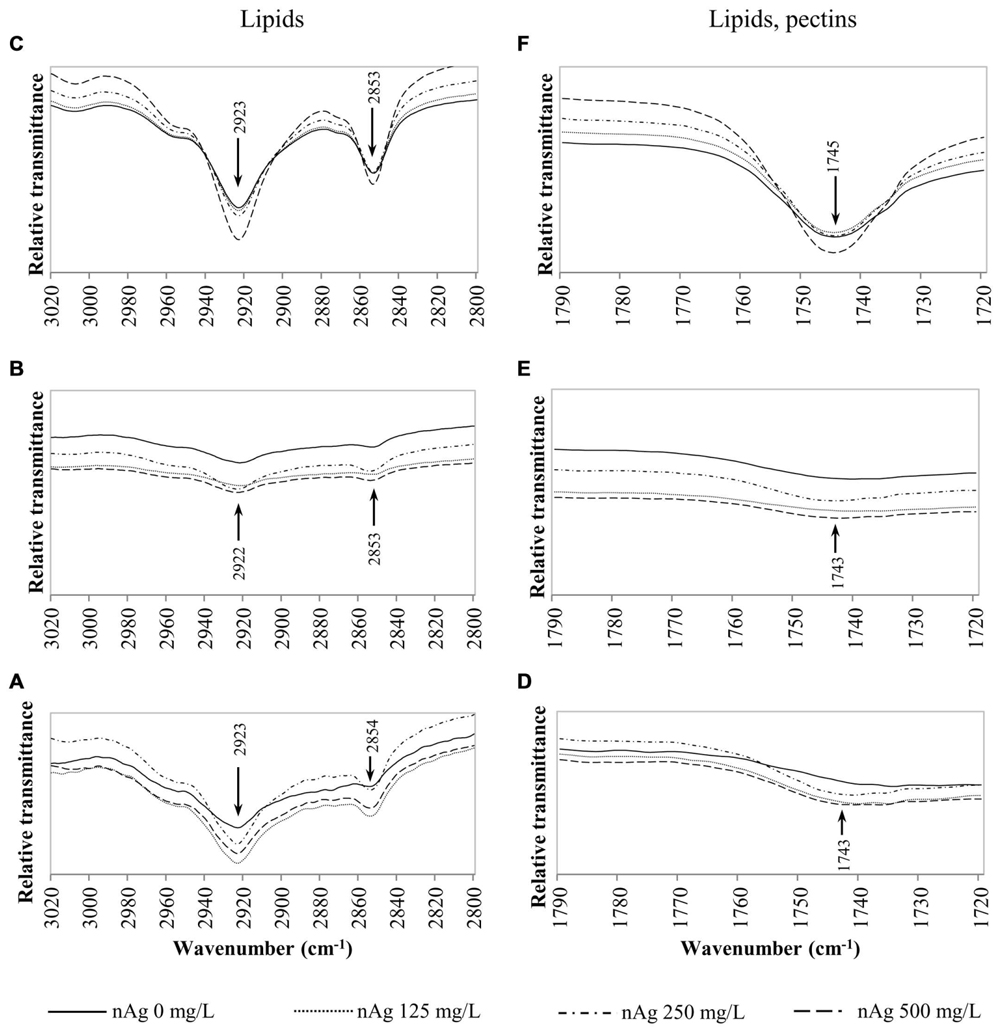
FIGURE 3. Overlap ATR-FTIR spectra of radish sprouts exposed to nAg at 0, 125, 250, and 500 mg/L. Spectral region associated with lipids in seedlings’ roots (A), stems (B) and leaves (C); and regions related to lipids and pectins in sprouts’ roots (D), stems (E), and leaves (F).
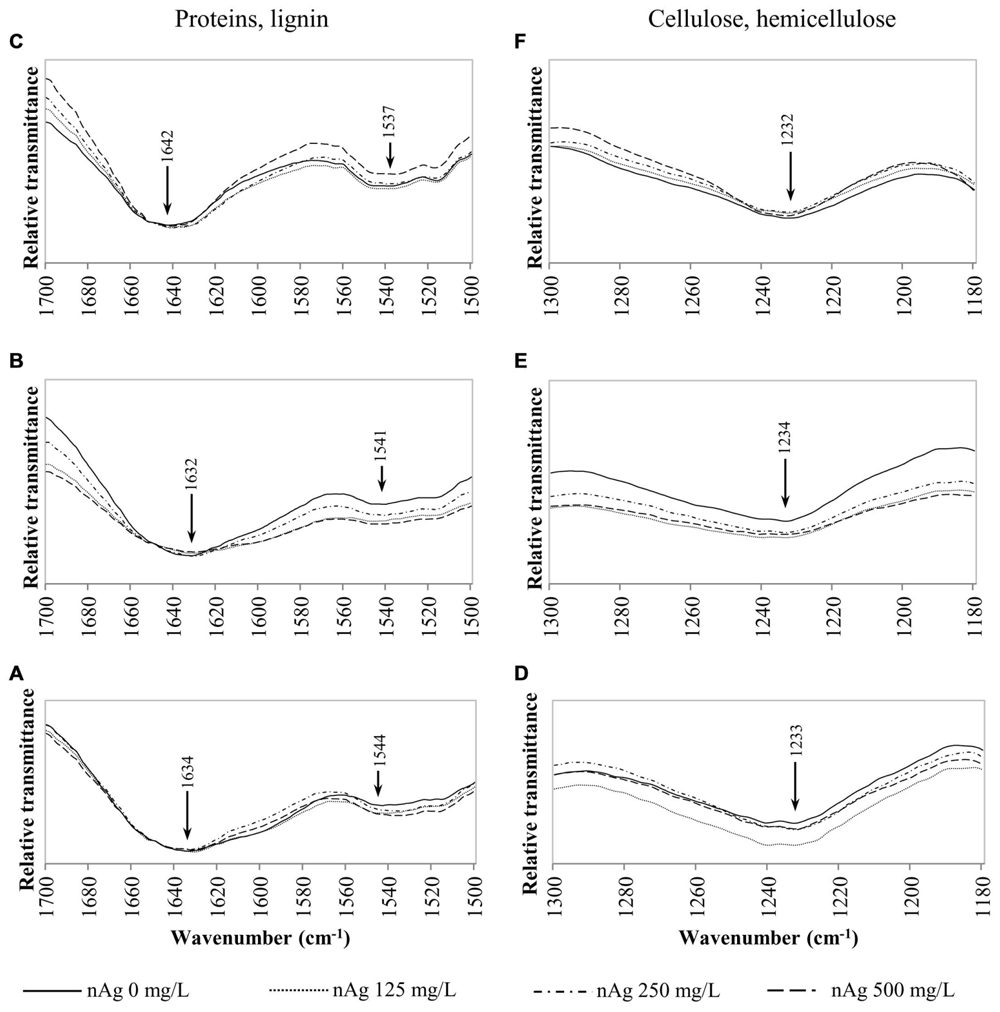
FIGURE 4. Overlap ATR-FTIR spectra of radish sprouts exposed to nAg at 0, 125, 250, and 500 mg/L. Spectral region associated with proteins and lignin in seedlings’ roots (A), stems (B), and leaves (C); and regions related to cellulose and hemicellulose in sprouts’ roots (D), stems (E) and leaves (F).
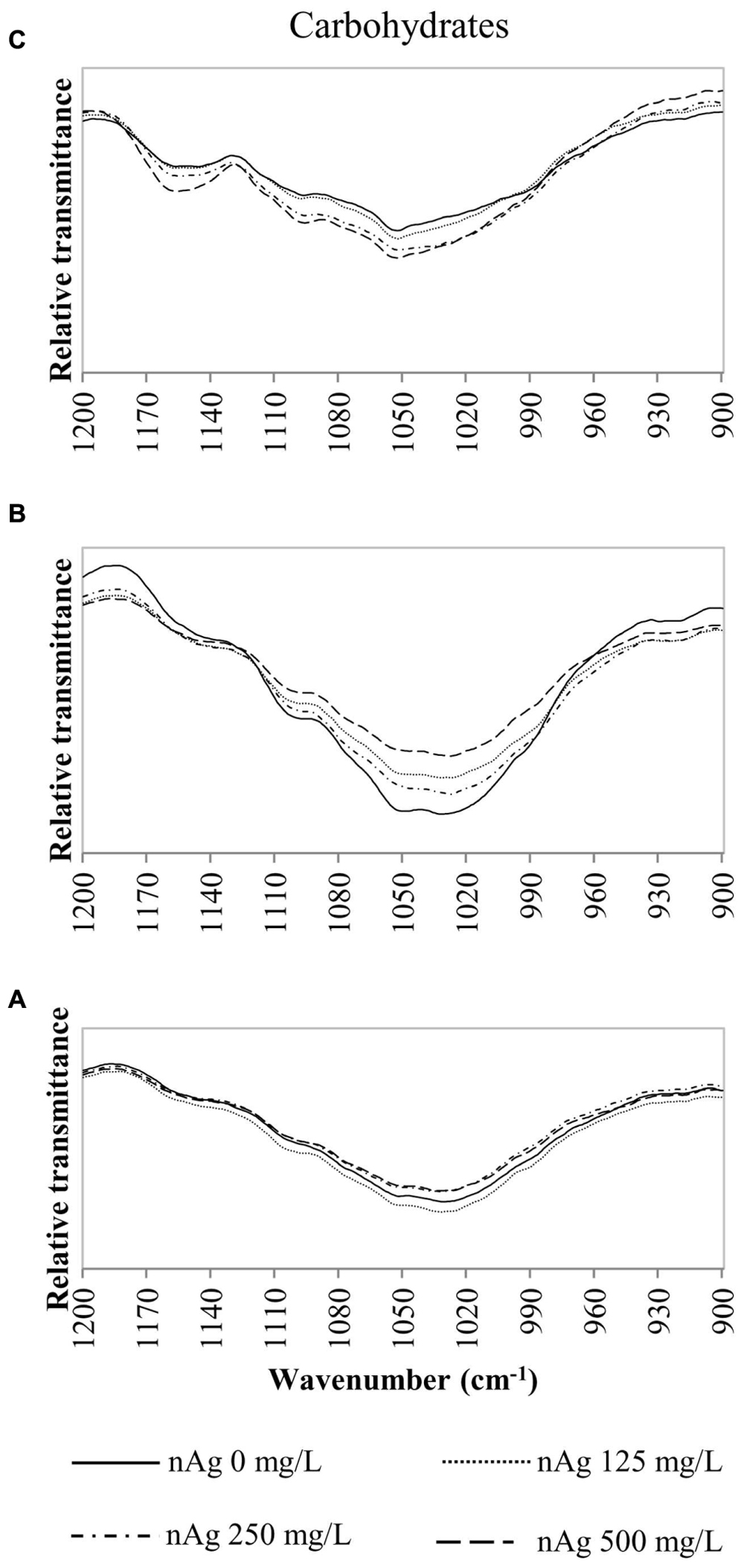
FIGURE 5. Overlap ATR-FTIR spectra of radish sprouts exposed to nAg at 0, 125, 250, and 500 mg/L. Spectral region associated with carbohydrates in seedlings’ roots (A), stems (B), and leaves (C).
Discussion
Seed Germination
Nanoparticles tend to agglomerate in suspension due to their size, composition of the medium and ionic strength, among other variables (Murdock et al., 2008). nAg showed moderate agglomeration (77 ± 2.44 nm) and negative surface charge when suspended in Millipore water (Murdock et al., 2008). However, these characteristics did not appear to interact with the cellulosic component of the radish seed coat (Esau, 1977). A previous study has shown that radish is a robust plant, in terms of germination under environmental stresses, due to the hard coat of it seeds that may prevent the entrance of contaminants, like heavy metals and nanoparticles (Koul et al., 2000). Previous studies have also shown no effects of heavy metal solutions or NP suspensions on radish germination. Lane and Martin (1977) reported no penetration of Pb within radish seeds exposed to 95 mg/L of “Analar,” a lead nitrate solution. Lin and Xing (2007) reported that radish germination was not altered by nAl2O3, nAl, nZn, and nZnO, even at the very high concentration of 2000 mg/L. Wu et al. (2012) found that the EC50 for radish germination exposed to nNiO and nCuO was 401 and 398 mg/L, respectively. Trujillo-Reyes et al. (2013) reported no effects on radish seed germination exposed up to 200 mg/L of citric acid coated and uncoated nCeO2. Corral-Diaz et al. (2014) reported that germination of radish seeds sown in soil amended with nCeO2 at 0–500 mg/kg was retarded, but not reduced. Thus, it is not surprising that nAg, even at 500 mg/L, did not affect radish seed germination.
Silver Uptake, Seedling Growth, Biomass Production, and Water Content
The measurement of heavy metals or NPs’ uptake by plant roots through ICP includes particles/elements adsorbed/ absorbed by the root system (Larue et al., 2012). Hong et al. (2015) showed that washing the tissues with CaCl2 and HNO3 removed about 80% of the nCeO2 sprayed to the leaves of cucumber. Thus, although radish seedlings were washed with HNO3 to remove the nAg adhered to the seedlings surface, some particles that could have remained were absorbed by the epidermis. Consequently, the reported data points include both the nAg adsorbed, plus the Ag taken up by the roots. The data reported in the present study, mainly at the highest concentration treatment (500 mg/L), differs from previously reported data. For instance, Song et al. (2013) reported no differences in silver uptake by tomato seedlings developed under exposure to 100 and 1000 mg/L of colloidal silver. These researchers determined that nAg hardly penetrated the hard coat of tomato seeds, and an analogous result could be expected with the hard coat of radish seeds (Koul et al., 2000). In specimens not protected by a hard coat, like rice seeds, penetration of the nAg has been associated with particle size and concentration. Thuesombat et al. (2014) soaked rice seedlings for 24 h in 1000 mg/L of either 20 or 150 nm nAg and germinated the seeds in a sand bed. Seven days after germination, concentrations of Ag in roots were 22 and 12 mg/kg, respectively. In our study, at 500 mg/L the uptake was higher than that reported by Thuesombat et al. (2014) at 1000 mg/L; however, the particles used in the present study were of a smaller size, which could explain the difference. In addition, radish seedlings were in contact with the nAg suspension during the entire experimental period. Results from our study and reports from the literature indicate that the uptake of Ag from nAg depends on a series of factors such as treatment concentration, particle size, plant species, and exposure media.
There is no consistency in the reports of the effects of nAg on seedlings growth. For instance, Lee et al. (2012) reported a reduction of about 60% in mung bean and 75% in sorghum seedlings exposed in agar for 2 days to nAg at 10 mg/L. These reduction rates are considerable larger than those observed in radish, although their support (agar) was different than the suspension used. However, the reduction in radish growth was similar to the reduction in sorghum, but different from that found with mung bean exposed in soil to 800 mg/kg of nAg (Lee et al., 2012), which does not support a comparison between liquid medium or agar. On the other hand, Nair and Chung (2014) exposed rice seedlings for 1 week to nAg in hydroponics (similar conditions of the present study), but at lower concentrations (0.2–1.0 mg/L) and did not report changes in root elongation. This corroborates that the effects of nAg on root growth depend on a series of factors. In addition, differences in treatment concentrations and exposure media made it difficult to compare the results.
Our results on the effects of nAg in biomass production concurs with the reports found in 4-week old rice seedlings exposed to nAg at 0.1–1000 mg/L (Thuesombat et al., 2014). However, they differ from the results with tomato exposed for 15 days to a similar nAg concentration, where the reduction was about 75% (Song et al., 2013). The mechanisms for reduction in biomass production by nAg are not known; however, Nair and Chung (2014) found differential transcription of genes associated with stress tolerance, which could explain the reduction in biomass production.
The reduction in seedlings’ water content under NP exposure has been previously reported. Trujillo-Reyes et al. (2014) found that nCu at 10 and 20 mg/L significantly reduced water content in lettuce. According to the literature, both Cu and Ag block water permeability in roots cells (Niemietz and Tyerman, 2002; Chang et al., 2012), which in turn reduce water absorption. In addition, Qian et al. (2013) proposed that nAg have the potential to change the transcription of antioxidant and aquaporin genes, affecting the balance of water in Arabidopsis. It is possible that nAg block aquaporins, reducing the water uptake in radish seedlings. This raises a question which requires more studies for a complete understanding of the aquaporins blockage by nAg.
Effects of nAg on Macro and Micronutrient Accumulation
Previous studies have shown that the uptake of nutrient elements is affected by both the NM and the species of plant. For instance, Servin et al. (2013) reported increases in Ca, K, and Mg in cucumber exposed to nTiO2. Trujillo-Reyes et al. (2014) reported changes on the accumulation of Mn and Zn in lettuce leaves exposed to core-shell Fe/Fe3O4 and Cu/CuO NPs. Hong et al. (2015) exposed alfalfa and lettuce to several Cu-based NPs/compounds in hydroponics and found changes in the absorption of some macroelements such as K, Mg, and Cu. In addition, Trujillo-Reyes et al. (2013) reported tha CeO2 NPs modified the content of Mn and Ni in hydroponically grown radish. In the present study, it was found that nAg reduced the uptake of macroelements Ca and Mg and microelements like B, Mn, and Zn. There is the possibility that nAg decrease the expression of the Ca channel protein, reducing Ca uptake. Magnesium is absorbed in a similar way as bacterial transporters CorA Mg2+ (Maathuis, 2009). It is very likely that at high concentrations, nAg are physically blocking the channels, reducing the absorption of Mg.
Boron uptake mechanisms include passive transport through the uncharged boric acid molecule, active transport through boron transporter 1 (BOR1) that uploads B into the xylem, and facilitated diffusion through channels belonging to intrinsic proteins (Dordas et al., 2000; Miwa and Fujiwara, 2010; Wimmer and Eichert, 2013). Trujillo-Reyes et al. (2013) reported that citric acid coated nCeO2 reduced the uptake of B in radish; however, to the best of the authors’ knowledge, there is no explanation about the interference of NPs on B uptake. The uptake of Mn and Zn by roots is mediated by putative transporters, Nramp and ZIP family (Guerinot, 2000). The current information is not sufficient enough to get a clear idea of how nAg could affect the uptake of these microelements. As explained above, it is possible that nAg physically block the diffusion pathway or the channels for active absorption. In addition, Magesky and Pelletier (2015) mentioned that silver is a membrane disruptor that breaks down cellular homeostasis. Very likely, this disruption affected the uptake of essential elements. It is also possible that nAg down regulate the genes encoding for metal transporters. Further investigation is needed in order to unravel the mechanism of nAg interference with the uptake of micronutrients.
FT-IR Functional Groups/Macromolecules Analysis
According to Table 2, the C–H bond in CH2–CH3 groups associated with lipids is found in the region of 3000–2800 cm-1. These macromolecules are constituents of the lipid bilayer found in cell membranes. Figures 3A–C shows the relative transmittance of bands in the spectra obtained for roots, stems and leaves assigned to lipids in radish sprouts. Bands in the region between 1790 and 1720 cm-1 (Figures 3D–F) are associated to C=O and COOH groups assigned also to lipids and pectins, these last are made of polysaccharides and are accountable for the structure of the primary cell wall.
Figures 4A–C shows the peaks found at 1650–1630 cm-1 for lignin and bonds identified for proteins at 1650 cm-1, 1549–1530 cm-1 corresponding to C=O, N–H and C–N (Dokken and Davis, 2007; Rico et al., 2015). Changes in cellulose and hemicellulose are observed at 1242–1230 and 1054–1051 cm-1 in Figures 4D–F and 5 respectively. Also, carbohydrates not attributed to a specific biopolymer are shown from 1200–900 cm-1 in Figure 5.
Alterations in lipids and carbohydrates were similar to those reported for cilantro exposed to nCeO2 (Morales et al., 2013). While hemicellulose, cellulose and pectin are structural components of primary cell walls, lignin provides rigidity to terrestrial plants (Cabane et al., 2012). Disruption in these macromolecules may lead to changes in morphology that could impair the normal development of the plants. The fact that no band shifts were observed suggests that there are no chemical changes in the macromolecules studied, only shape alterations in the plant tissue components (Morales et al., 2013).
In summary, concentrations of nAg used in this study did not affect radish seed germination. However, there was a concentration-dependent reduction in seedling elongation and water content. In addition, at 250 mg/L the biomass was reduced by 10%, compared with control (p ≤ 0.05). Silver NPs also impaired the absorption of nutritional elements in radish seedlings. Important macroelements such as Ca and Mg and microelements B, Cu, Mn, and Zn were reduced by the highest concentration of nAg. Moreover, nAg induced conformational changes in carbohydrates, lignin, and lipids. The impacts of such changes in the nutritional value of radish sprouts are not known yet. In addition, as per Holden et al. (2014), “it may be premature for manufactured NMs risk research to sanction information on the basis of concentration ‘environmental relevance’.”
Author Contributions
All authors listed, have made substantial, direct and intellectual contribution to the work, and approved it for publication.
Conflict of Interest Statement
The authors declare that the research was conducted in the absence of any commercial or financial relationships that could be construed as a potential conflict of interest.
Acknowledgments
This material is based upon work supported by the National Science Foundation and the Environmental Protection Agency under Cooperative Agreement Number DBI-0830117. Any opinions, findings, and conclusions or recommendations expressed in this material are those of the author(s) and do not necessarily reflect the views of the National Science Foundation or the Environmental Protection Agency. This work has not been subjected to EPA review and no official endorsement should be inferred. The authors also acknowledge the USDA Grant number 2011-38422-30835 and the NSF Grant # CHE-0840525. Partial funding was provided by the NSF ERC on Nanotechnology-Enabled Water Treatment (EEC-1449500). This work was also supported by Grant 2G12MD007592 from the National Institutes on Minority Health and Health Disparities (NIMHD), a component of the National Institutes of Health (NIH). JG-T acknowledges the Dudley family for the Endowed Research Professorship and the Academy of Applied Science/US Army Research Office, Research and Engineering Apprenticeship program (REAP) at UTEP, grant #W11NF-10-2-0076, sub-grant 13-7. NZ-M acknowledges the “Consejo Nacional de Ciencia y Tecnología (CONACyT)” from Mexico and Dr. Jessica Trujillo-Reyes for her contribution in the experimental design.
Supplementary Material
The Supplementary Material for this article can be found online at: http://journal.frontiersin.org/article/10.3389/fpls.2016.00090
Footnotes
References
Amooaghaie, R., Tabatabaei, F., and Ahadi, A. M. (2015). Role of hematin and sodium nitroprusside in regulating Brassica nigra seed germination under nanosilver and silver nitrate stresses. Ecotoxicol. Environ. Saf. 113, 259–270. doi: 10.1016/j.ecoenv.2014.12.017
Baenas, N., Ferreres, F., García-Viguera, C., and Moreno, D. A. (2015). Radish sprouts—characterization and elicitation of novel varieties rich in anthocyanins. Food Res. Int. 69, 305–312. doi: 10.1016/j.foodres.2015.01.009
Beer, C., Foldbjerg, R., Hayashi, Y., Sutherland, D. S., and Autrup, H. (2012). Toxicity of silver nanoparticles—nanoparticle or silver ion? Toxicol. Lett. 208, 286–292. doi: 10.1016/j.toxlet.2011.11.002
Cabane, M., Afif, D., and Hawkins, S. (2012). “Chapter 7: Lignins and abiotic stresses,” in Advances in Botanical Research, eds J. Lise and L. Catherine (Cambridge, MA: Academic Press), 219–262.
Chang, Y.-N., Zhang, M., Xia, L., Zhang, J., and Xing, G. (2012). The toxic effects and mechanisms of CuO and ZnO nanoparticles. Materials 5, 2850–2871. doi: 10.3390/ma5122850
Corral-Diaz, B., Peralta-Videa, J. R., Alvarez-Parrilla, E., Rodrigo-García, J., Morales, M. I., Osuna-Avila, P., et al. (2014). Cerium oxide nanoparticles alter the antioxidant capacity but do not impact tuber ionome in Raphanus sativus (L). Plant Physiol. Biochem. 84, 277–285. doi: 10.1016/j.plaphy.2014.09.018
Daniel, S. C. G. K., Tharmaraj, V., Sironmani, T. A., and Pitchumani, K. (2010). Toxicity and immunological activity of silver nanoparticles. Appl. Clay Sci. 48, 547–551. doi: 10.1016/j.clay.2010.03.001
de la Rosa, G., López-Moreno, M. L., Hernandez-Viezcas, J. A., Montes, M. O., Peralta-Videa, J., and Gardea-Torresdey, J. (2011). Toxicity and biotransformation of ZnO nanoparticles in the desert plants Prosopis juliflora-velutina, Salsola tragus and Parkinsonia florida. Int. J. Nanotechnol. 8, 492–506. doi: 10.1504/IJNT.2011.040190
Dokken, K. M., and Davis, L. C. (2007). Infrared imaging of sunflower and maize root anatomy. J. Agric. Food Chem. 55, 10517–10530. doi: 10.1021/jf072052e
Dordas, C., Chrispeels, M. J., and Brown, P. H. (2000). Permeability and channel-mediated transport of boric acid across membrane vesicles isolated from squash roots. Plant Physiol. 124, 1349–1362. doi: 10.1104/pp.124.3.1349
Gardea-Torresdey, J. L., Rico, C. M., and White, J. C. (2014). Trophic transfer, transformation, and impact of engineered nanomaterials in terrestrial environments. Environ. Sci. Technol. 48, 2526–2540. doi: 10.1021/es4050665
Gottschalk, F., Sun, T. Y., and Nowack, B. (2013). Environmental concentrations of engineered nanomaterials: review of modeling and analytical studies. Environ. Pollut. 181, 287–300. doi: 10.1016/j.envpol.2013.06.003
Guerinot, M. L. (2000). The ZIP family of metal transporters. Biochim. Biophys. Acta 1465, 190–198. doi: 10.1016/S0005-2736(00)00138-3
Holden, P., Klaessig, F., Turco, R., Priester, J. H., Rico, C., Arias, H., et al. (2014). Evaluation of exposure concentrations used in assessing manufactured nanomaterial nnvironmental hazards: are they relevant? Environ. Sci. Technol. 48, 10541–10551. doi: 10.1021/es502440s
Hong, J., Rico, C. M., Zhao, L., Adeleye, A. S., Keller, A. A., Peralta-Videa, J. R., et al. (2015). Toxic effects of copper-based nanoparticles or compounds to lettuce (Lactuca sativa) and alfalfa (Medicago sativa). Environ. Sci. Process. Impacts 17, 177–185. doi: 10.1039/c4em00551a
Keller, A. A., Mcferran, S., Lazareva, A., and Suh, S. (2013). Global life cycle releases of engineered nanomaterials. J. Nanopart. Res. 15, 1–17. doi: 10.1007/s11051-013-1692-4
Kim, J. S., Kuk, E., Yu, K. N., Kim, J. H., Park, S. J., Lee, H. J., et al. (2007). Antimicrobial effects of silver nanoparticles. Nanomedicine 3, 95–101. doi: 10.1016/j.nano.2006.12.001
Kostka-Rick, R., and Manning, W. J. (1993). Radish (Raphanus sativus L.): a model for studying plant responses to air pollutants and other environmental stresses. Environ. Pollut. 82, 107–138. doi: 10.1016/0269-7491(93)90109-2
Koul, K., Nagpal, R., and Raina, S. (2000). Seed coat microsculpturing in Brassica and allied genera (subtribes Brassicinae, Raphaninae, Moricandiinae). Ann. Bot. 86, 385–397. doi: 10.1006/anbo.2000.1197
Kumari, M., Mukherjee, A., and Chandrasekaran, N. (2009). Genotoxicity of silver nanoparticles in Allium cepa. Sci. Total Environ. 407, 5243–5246. doi: 10.1016/j.scitotenv.2009.06.024
Lammers, K., Arbuckle-Keil, G., and Dighton, J. (2009). FT-IR study of the changes in carbohydrate chemistry of three New Jersey pine barrens leaf litters during simulated control burning. Soil Biol. Biochem. 41, 340–347. doi: 10.1016/j.soilbio.2008.11.005
Lane, S., and Martin, E. (1977). A histochemical investigation of lead uptake in Raphanus sativus. New Phytol. 79, 281–286. doi: 10.1111/j.1469-8137.1977.tb02206.x
Larue, C., Laurette, J., Herlin-Boime, N., Khodja, H., Fayard, B., Flank, A. M., et al. (2012). Accumulation, translocation and impact of TiO2 nanoparticles in wheat (Triticum aestivum spp.): influence of diameter and crystal phase. Sci. Total Environ. 431, 197–208. doi: 10.1016/j.scitotenv.2012.04.073
Lee, W. M., Kwak, J. I., and An, Y. J. (2012). Effect of silver nanoparticles in crop plants Phaseolus radiatus and Sorghum bicolor: media effect on phytotoxicity. Chemosphere 86, 491–499. doi: 10.1016/j.chemosphere.2011.10.013
Létondor, C., Pascal-Lorber, S., and Laurent, F. (2015). Uptake and distribution of chlordecone in radish: different contamination routes in edible roots. Chemosphere 118, 20–28. doi: 10.1016/j.chemosphere.2014.03.102
Lin, D., and Xing, B. (2007). Phytotoxicity of nanoparticles: inhibition of seed germination and root growth. Environ. Pollut. 150, 243–250. doi: 10.1016/j.envpol.2007.01.016
López-Moreno, M. L., De La Rosa, G., Hernández-Viezcas, J. A., Peralta-Videa, J. R., and Gardea-Torresdey, J. L. (2010). X-ray absorption spectroscopy (XAS) corroboration of the uptake and storage of CeO2 nanoparticles and assessment of their differential toxicity in four edible plant species. J. Agric. Food Chem. 58, 3689–3693. doi: 10.1021/jf904472e
Ma, Y., Kuang, L., He, X., Bai, W., Ding, Y., Zhang, Z., et al. (2010). Effects of rare earth oxide nanoparticles on root elongation of plants. Chemosphere 78, 273–279. doi: 10.1016/j.chemosphere.2009.10.050
Maathuis, F. J. M. (2009). Physiological functions of mineral macronutrients. Curr. Opin. Plant Biol. 12, 250–258. doi: 10.1016/j.pbi.2009.04.003
Magesky, A., and Pelletier, E. (2015). Toxicity mechanisms of ionic silver and polymer-coated silver nanoparticles with interactions of functionalized carbon nanotubes on early development stages of sea urchin. Aquat. Toxicol. 167, 106–123. doi: 10.1016/j.aquatox.2015.07.011
Miwa, K., and Fujiwara, T. (2010). Boron transport in plants: co-ordinated regulation of transporters. Ann. Bot. 105, 1103–1108. doi: 10.1093/aob/mcq044
Morales, M. I., Rico, C. M., Hernandez-Viezcas, J. A., Nunez, J. E., Barrios, A. C., Tafoya, A., et al. (2013). Toxicity assessment of cerium oxide nanoparticles in cilantro (Coriandrum sativum L.) plants grown in organic soil. J. Agric. Food Chem. 61, 6224–6230. doi: 10.1021/jf401628v
Murdock, R. C., Bradich-Stolle, L., Schrand, A. M., Schlanger, J. J., and Hussain, S. M. (2008). Characterization of nanomaterial dispersion in solution prior to in vitro exposure using dynamic light scaterring technique. Toxicol. Sci. 101, 239–253. doi: 10.1093/toxsci/kfm240
Musante, C., and White, J. C. (2012). Toxicity of silver and copper to Cucurbita pepo: differential effects of nano and bulk-size particles. Environ. Toxicol. 27, 510–517. doi: 10.1002/tox.20667
Nair, P. M. G., and Chung, I. M. (2014). Physiological and molecular level effects of silver nanoparticles exposure in rice (Oryza sativa L.) seedlings. Chemosphere 112, 105–113. doi: 10.1016/j.chemosphere.2014.03.056
Niemietz, C. M., and Tyerman, S. D. (2002). New potent inhibitors of aquaporins: silver and gold compounds inhibit aquaporins of plant and human origin. FEBS Lett. 531, 443–447. doi: 10.1016/S0014-5793(02)03581-0
Pokhrel, L. R., and Dubey, B. (2013). Evaluation of developmental responses of two crop plants exposed to silver and zinc oxide nanoparticles. Sci. Total Environ. 45, 321–332. doi: 10.1016/j.scitotenv.2013.02.059
Qian, H., Peng, X., Han, X., Ren, J., Sun, L., and Fu, Z. (2013). Comparison of the toxicity of silver nanoparticles and silver ions on the growth of terrestrial plant model Arabidopsis thaliana. J. Environ. Sci. 25, 1947–1956. doi: 10.1016/S1001-0742(12)60301-5
Rico, C. M., Peralta-Videa, J. R., and Gardea-Torresdey, J. L. (2015). Differential effects of cerium oxide nanoparticles on rice, wheat, and barley roots: a fourier transform infrared (FT-IR) microspectroscopy study. Appl. Spectrosc. 69, 287–295. doi: 10.1366/14-07495
Roco, M. C. (2003). Nanotechnology: convergence with modern biology and medicine. Curr. Opin. Biotechnol. 14, 337–346. doi: 10.1016/S0958-1669(03)00068-5
Servin, A. D., Morales, M. I., Castillo-Michel, H., Hernandez-Viezcas, J. A., Munoz, B., Zhao, L., et al. (2013). Synchrotron verification of TiO2 accumulation in cucumber fruit: a possible pathway of TiO2 nanoparticle transfer from soil into the food chain. Environ. Sci. Technol. 47, 11592–11598. doi: 10.1021/es403368j
Song, U., Jun, H., Waldman, B., Roh, J., Kim, Y., Yi, J., et al. (2013). Functional analyses of nanoparticle toxicity: a comparative study of the effects of TiO2 and Ag on tomatoes (Lycopersicon esculentum). Ecotoxicol. Environ. Saf. 93, 60–67. doi: 10.1016/j.ecoenv.2013.03.033
Stampoulis, D., Sinha, S. K., and White, J. C. (2009). Assay-dependent phytotoxicity of nanoparticles to plants. Environ. Sci. Technol. 43, 9473–9479. doi: 10.1021/es901695c
Thuesombat, P., Hannongbua, S., Akasit, S., and Chadchawan, S. (2014). Effect of silver nanoparticles on rice (Oryza sativa L. cv. KDML 105) seed germination and seedling growth. Ecotoxicol. Environ. Saf. 104, 302–309. doi: 10.1016/j.ecoenv.2014.03.022
Trujillo-Reyes, J., Majumdar, S., Botez, C. E., Peralta-Videa, J. R., and Gardea-Torresdey, J. L. (2014). Exposure studies of core–shell Fe/Fe3O4 and Cu/CuO NPs to lettuce (Lactuca sativa) plants: are they a potential physiological and nutritional hazard? J. Hazard. Mater. 267, 255–263. doi: 10.1016/j.jhazmat.2013.11.067
Trujillo-Reyes, J., Vilchis-Nestor, A., Majumdar, S., Peralta-Videa, J., and Gardea-Torresdey, J. (2013). Citric acid modifies surface properties of commercial CeO2 nanoparticles reducing their toxicity and cerium uptake in radish (Raphanus sativus) seedlings. J. Hazard. Mater. 263, 677–684. doi: 10.1016/j.jhazmat.2013.10.030
Wimmer, M. A., and Eichert, T. (2013). Review: mechanisms for boron deficiency-mediated changes in plant water relations. Plant Sci. 20, 25–32. doi: 10.1016/j.plantsci.2012.12.012
Wu, S. G., Huang, L., Head, J., Chen, D. R., Kong, I. C., and Tang, Y. J. (2012). Phytotoxicity of metal oxide nanoparticles is related to both dissolved metals ions and adsorption of particles on seed surfaces. J. Pet. Environ. Biotechnol. 3, 1–5. doi: 10.4172/2157-7463.1000126
Xiao, Z., Luo, Y., Lester, G. E., Kou, L., Yang, T., and Wang, Q. (2014). Postharvest quality and shelf life of radish microgreens as impacted by storage temperature, packaging film, and chlorine wash treatment. LWT-Food Sci. Technol. 55, 551–558. doi: 10.1016/j.lwt.2013.09.009
Yin, L., Colman, B. P., McGill, B. M., Wright, J. P., and Bernhardt, E. S. (2012). Exposure of silver nanoaprticloe exposure on germination and early growth of eleven wetland plants. PLoS ONE 7:e47674. doi: 10.1371/journal.pone.0047674
Keywords: silver nanoparticles, radish, FTIR, elemental analysis, macromolecules
Citation: Zuverza-Mena N, Armendariz R, Peralta-Videa JR and Gardea-Torresdey JL (2016) Effects of Silver Nanoparticles on Radish Sprouts: Root Growth Reduction and Modifications in the Nutritional Value. Front. Plant Sci. 7:90. doi: 10.3389/fpls.2016.00090
Received: 28 August 2015; Accepted: 18 January 2016;
Published: 16 February 2016.
Edited by:
Nelson Marmiroli, University of Parma, ItalyReviewed by:
Jürgen Kreuzwieser, University of Freiburg, GermanySibia Ranjbar, Temple University, USA
Copyright © 2016 Zuverza-Mena, Armendariz, Peralta-Videa and Gardea-Torresdey. This is an open-access article distributed under the terms of the Creative Commons Attribution License (CC BY). The use, distribution or reproduction in other forums is permitted, provided the original author(s) or licensor are credited and that the original publication in this journal is cited, in accordance with accepted academic practice. No use, distribution or reproduction is permitted which does not comply with these terms.
*Correspondence: Jorge L. Gardea-Torresdey, amdhcmRlYUB1dGVwLmVkdQ==