- 1Forestry Bureau of Dongchuan County, Kunming, China
- 2Kunming Forest Resources Administration, Kunming, China
- 3Kunming Institute of Botany, Chinese Academy of Sciences, Kunming, China
The gall wasp, Leptocybe invasa (Hymenoptera; Eulophidae), is a devastating pest of eucalypt plantations in the Middle East, the Mediterranean basin, Africa, India, South-East Asia, and China. Heavy galling causes the leaves to warp and in extreme cases it may stunt the growth of the trees of Eucalyptus camaldulensis. However, the physiological mechanisms underlying how L. invasa inhibits the growth of plants of E. camaldulensis are unclear. Because the growth rate of plants is mainly dependent on photosynthesis that is largely correlated with hydraulic architecture, we speculate that galling of L. invasa depresses hydraulic conductance of stem and leaf. In the present study, we examined the effects of L. invasa galling on hydraulic architecture and photosynthetic parameters in E. camaldulensis plants. We found that galling of L. invasa significantly decreased stem hydraulic conductance (Kstem), midday leaf water potential (Ψmd), minor vein density, and stomatal density (SD). Furthermore, the stomatal conductance (gs), chlorophyll content, CO2 assimilation rate (An) and photosynthetic electron flow were reduced in infected plants. Therefore, the galling of L. invasa not only declined the water supply from stem to leaves, but also restricted water transport within leaf. As a result, galled plants of E. camaldulensis reduced leaf number, leaf area, SD and gs to balance water supply and transpirational demand. Furthermore, galled plants had lower leaf nitrogen content, leading to decreases in chlorophyll content, CO2 assimilation rate and photosynthetic electron flow. These results indicate that the change in hydraulic architecture is responsible for the inhibition of growth rate in galled plants.
Introduction
In China, Eucalyptus sp. has been introduced for plantation in many tropical and subtropical areas for economic and social development because they can tolerate and grow well on degraded, unfertile soils, where it is very difficult to establish other tree species. The wood of Eucalyptus species can be used for production of paper, which providing income for local people and government. The genus Eucalyptus in its native range sustains a rich fauna of gall-inducing insects (Blanche and Westoby, 1995; Blanche, 2000). Eucalyptus is also a unique genus in hosting several Eulophidae wasps (Hymenoptera: Chalcidoidea) such as Leptocybe invasa Fisher & LaSalle. Eucalyptus camaldulensis Dehnh. is one of the most susceptible species to this gall wasp L. invasa (Thu et al., 2009). Recently, the massive presence of galls induced by L. invasa cause severe damage and consequent economic impact in nurseries and eucalyptus plantations with E. camaldulensis (Thu et al., 2009).
Leptocybe invasa, a gall-inducing wasp on several Eucalyptus sp., was first reported in 2000 from the Middle East and was subsequently found in many countries in the Mediterranean basin, Africa, and Asia (Mendel et al., 2004). The adult of L. invasa is very small (1.0–1.4 mm long), and it lays the eggs in the cortex of shoots or the midribs of leaves. The eggs hatch and the larvae feed and then pupate. The adults emerge from the gall within a period of 4–5 months (Mendel et al., 2004). The gall size is correlated to the number of wasps developing in the gall. The developing larvae form typical galls in the form of distinct swellings on the leaf midribs, petioles, and stems on new foliage on trees of all ages (Kim et al., 2008). Seedlings in the nursery and young plantations are particularly susceptible (Thu et al., 2009). Heavy galling causes the leaves to warp and in extreme cases it may stunt the growth of the tree (Mendel et al., 2004). However, the physiological mechanisms underlying why L. invasa inhibits the growth of E. camaldulensis in unclear.
Field observations show that the presence of L. invasa largely decreases the leaf number and leaf area. It has been indicated that leaf water status is one of the most important factor determining the leaf area (Anyia and Herzog, 2004; Koch et al., 2004). Under drought stress, plants decrease leaf area and leaf number to diminish the water transpiration (Anyia and Herzog, 2004; Koch et al., 2004). Therefore, we speculate that the infection of L. invasa decreases the leaf water potential. In addition, the growth of plants is dependent on leaf primary productivity (photosynthesis), which in turn relies on the function of hydraulic architecture such as stomatal density (SD) and minor vein density (MVD; Brodribb et al., 2007; Tanaka et al., 2013; Zhu et al., 2013; Hu et al., 2014). Therefore, the severe damage to E. camaldulensis plants caused by galling of L. invasa is probably lined to change in the function of leaf hydraulic architecture.
Water transport from stem to leaf is a prerequisite for the maintenance of optimum leaf water potential (Chone et al., 2001; Sperry et al., 2002; Zhang and Cao, 2009). The stem hydraulic conductance (Kstem) is determined by the number and radius of functional conduit (Hellemans et al., 1980). Under drought stress, the number of functional conduit decreases due to cavitation, causing the decline in Kstem (Sperry et al., 2002). Once Kstem decreases, plants regulate the development of leaf hydraulic architecture to decrease transpirational demand. As mentioned above, the leaf development in the galled plants of E. camaldulensis is similar to the phenomenon under drought stress. Therefore, we speculate that the presence of galls in the cortex of shoots maybe leaf to cavitation of stem conduit, which decreases the number of functional conduit and thus Kstem.
To explain, why E. camaldulensis plants galled by L. invasa display lower growth rate, we determined the difference in hydraulic architecture between galled and non-galled plants. To our knowledge, the effect of L. invasa infection on the function of hydraulic architecture in E. camaldulensis has never been reported before. The present study aims to test the hypothesis that galling of L. invasa decreases the water supply to leaf and then affect the development of leaf hydraulic architecture. Furthermore, the effects of galling of L. invasa on stomatal conductance and photosynthetic rate have been studied.
Materials and Methods
Plant Materials and Study Site
This study was conducted at Dongchuan County, Yunnan Province, China. E. camaldulensis Dehnh. is one of the most susceptible species to the gall wasp Leptocybe invasa Fisher & LaSalle. In the present study, galled and non-galled plants of E. camaldulensis grown in the same open-field place with sufficient water were used for measurements of Kstem, leaf anatomy, and gas exchange. All measurements were conducted in August 2014 (summer). Three-years-old plants of galled and non-galled plants were used for hydraulic and photosynthetic measurements. The galled-plants were naturally got infected by L. invasa. Six independent stems from galled or non-galled plants were used for measurements of Kstem. Nine independent leaves of galled or non-galled plants were used for measurements of leaf anatomy. Four independent leaves of galled or non-galled plants were used for photosynthetic measurements. Immediately after the measurements of gas exchange, leaf samples were taken for measurements of leaf anatomy. Some leaves taken from other plants were also used for measurements of leaf anatomy.
Hydraulics
Stem hydraulic conductance was determined using a high-pressure flow meter (HPFM; Dynamax Inc., Houston, TX, USA). The high-pressure flow meter measured resistance (the inverse of conductance) as the force required to push water through a sample for a given flow rate. Samples were cut from the trees and wrapped with wet tissue in plastic black bags for transport to the laboratory. Briefly, the stem was recut under water and then connected to the flow meter. Water flowed through the stem and the necessary applied pressure were recorded every 3 s while pressure was ramped. Here, Kstem was computed as the water flow rate per unit cross-sectional area of the stem divided by the pressure drop. During this measurement period, the laboratory temperature was about 25°C. Leaf water potential was measured on six fully expanded and mature leaves per treatment (galled and non-galled plants). After measuring photosynthesis, leaves were taken and wrapped with wet tissue in plastic black bags for transport to the laboratory. In our present study, leaf water potential was measured with WP4 Dewpoint Potentiometer (Decagon Devices, Inc., Pullman, WA, USA).
Leaf Anatomy
Leaves were boiled in 7% NaOH for 3 min and then stained with safranin. The sections were then photographed under a light microscope at 4× magnification (Nikon Optiphot; Nikon, Tokyo, Japan). Values for MVD were expressed as the sum of the lengths of third- and higher-order veins per unit area (Zhu et al., 2012). SD was measured on both adaxial and abaxial cuticles. SD was measured from digital photomicrographs of the cuticle preparation at 20× magnification. The data of MVD and SD include six section per leaf, for a total of six leaves per treatment (galled and non-galled plants).
Gas Exchange Measurement
Parameters for gas exchange and chlorophyll fluorescence were monitored with an open gas exchange system that incorporated infrared CO2 and water vapor analyzers (Li-6400XT; Li-Cor Biosciences, Lincoln, NE, USA) and a 2-cm2 measuring head (6400-40 Leaf Chamber Fluorometer; Li-Cor Biosciences). The atmospheric CO2 concentration was maintained at 390 μmol mol–1 by the Li-6400XT with a relative humidity of approximately 50%. To generate a light response curve, we initially exposed the mature leaves to strong irradiance (1500 μmol photons m–2 s–1) for 15 min to obtain steady, high levels of gs and CO2 assimilation. Afterward, photosynthetic parameters were evaluated at 2-min intervals at photosynthetic photon flux densities (PPFDs) of 2000, 1600, 1200, 1000, 800, 500, 300, 150, 100, 50, 20, and 0 μmol photons m–2 s–1. Both photosynthetic rate and stomatal conductance were recorded automatically by Licor-6400XT. The fluorescence parameters Fm′, and Fs were evaluated as previously described in Baker and Rosenqvist (2004). Fm′ represents the minimum and maximum fluorescence after light-adaption. Fs is the light-adapted steady-state fluorescence. Effective quantum yield of PSII was calculated as ΦPSII = (Fm′ – Fs)/Fm′ (Genty et al., 1989). Total photosynthetic electron flow through PSII was calculated as ETRII = ΦPSII × PPFD × 0.85 × 0.5 (Krall and Edwards, 1992).
Chlorophyll Content
The chlorophyll content was measured according to the method of Lichtenthaler and Wellburn (1983).
Statistical Analysis
The results were displayed as mean values of independent measurements (n = 4–9). We used one-way ANOVA and SPSS 16.0 software (SPSS Inc., Chicago, IL, USA) to examine differences between the galled and non-galled plants. Those differences were considered significant at P < 0.05.
Results
Values for the stem hydraulic conductance (Kstem) were 0.36 and 0.92 Kg m–1 s–1 MPa–1 in galled and non-galled plants, respectively (Figure 1). The value of Kstem in galled plants was 60% lower than that in non-galled plants. Therefore, galling in the stems largely decreased Kstem, probably as a result of jam and cavitation of conduit. Meanwhile, the midday leaf water potential (Ψmd) was affected by galling of L. invasa. The value of Ψmd was significantly lower in galled plants (–1.93 MPa) than non-galled plants (–1.55 MPa; Figure 2A). In leaves of galled plants, the MVD largely decreased compared with non-galled plants. The value of MVD was 8.5 mm mm–2 in galled plants versus 11.3 mm mm–2 in non-galled plants (Figure 2B). Because MVD is significantly and positively correlated with leaf hydraulic conductance (Kleaf), the decrease in MVD in galled plants can cause a decline in Kleaf. The lower Ψmd in leaves of galled plants is probably a result of decreases in both Kleaf and Kstem.
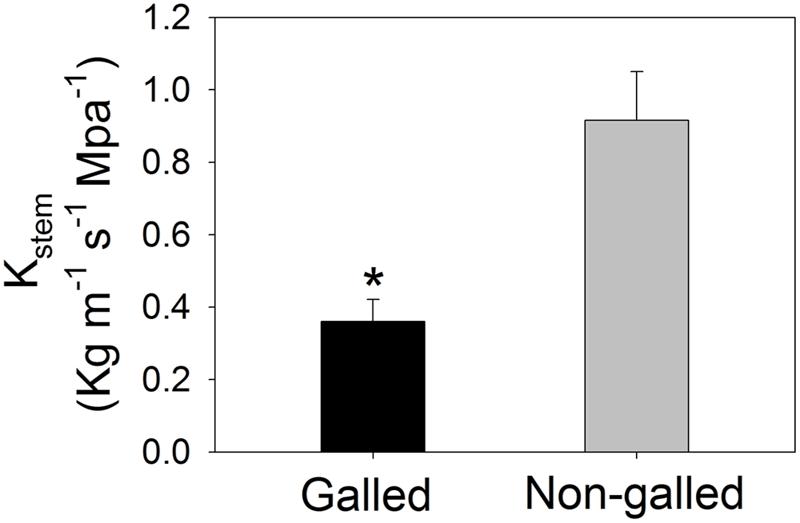
FIGURE 1. Stem hydraulic conductance (Kstem) in galled and non-galled plants of Eucalyptus camaldulensis. An asterisk indicates a significant difference between galled and non-galled plants.
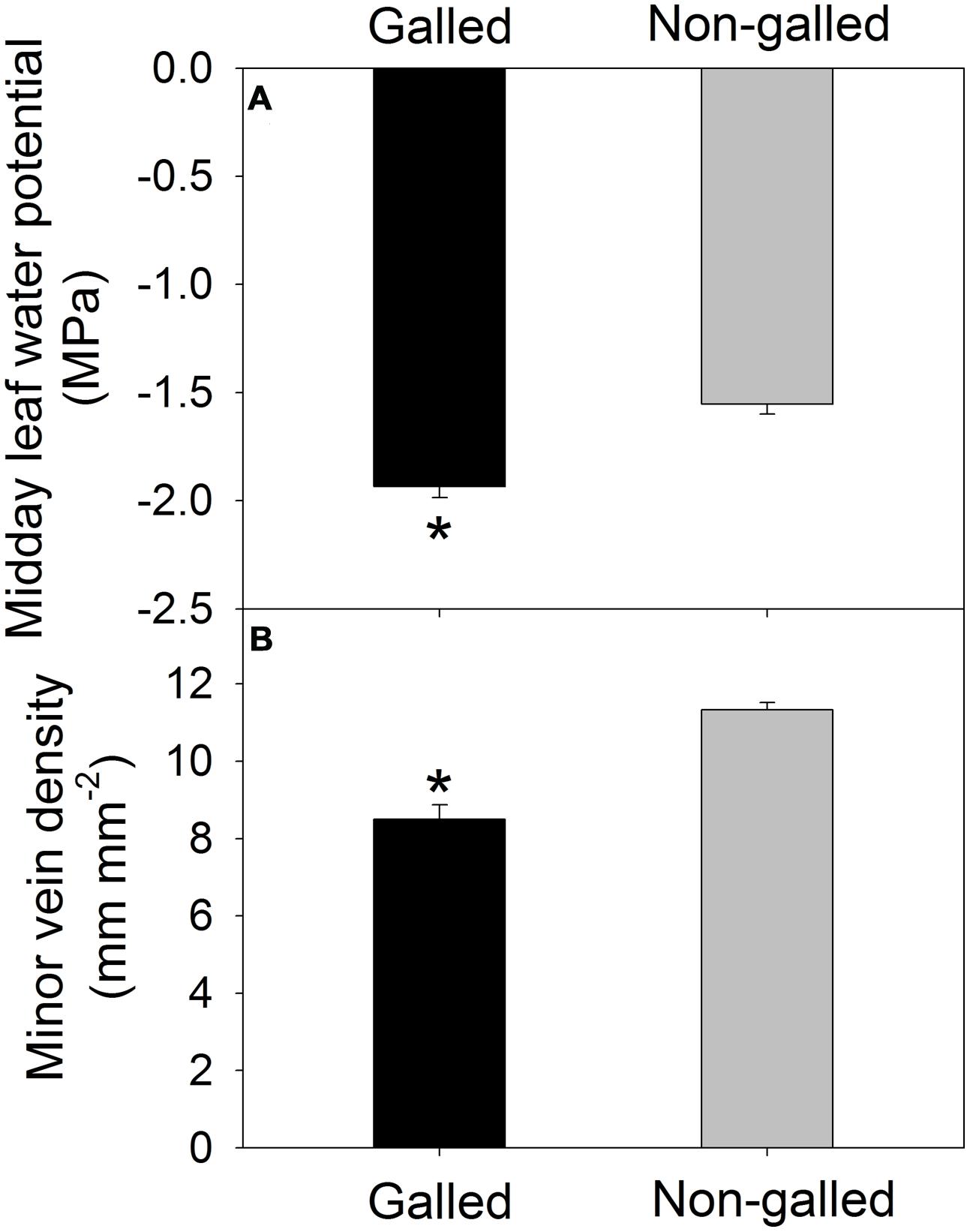
FIGURE 2. Midday leaf water potential (A) and minor vein density (B) in galled and non-galled plants of Eucalyptus camaldulensis. An asterisk indicates a significant difference between galled and non-galled plants.
Stomatal density is another important functional trait that reflects leaf water status and determines stomatal conductance (gs) and photosynthetic rate (An). Stomata exist in both adaxial and abaxial sides in leaves of E. camaldulensis, contributing to its high level of photosynthetic rate. Interestingly, galling of L. invasa significantly affected the development of stomata. In galled and non-galled plants, SD in adaxial side numbered 278.3 and 351.3 per square millimeter, respectively, and SD in abaxial side numbered 378.2 and 460.3 per square millimeter, respectively, (Figure 3). The values of SD in adaxial and abaxial sides in galled plants were approximately 80% of non-galled plants (Figure 3 and Supplementary Figure S1).
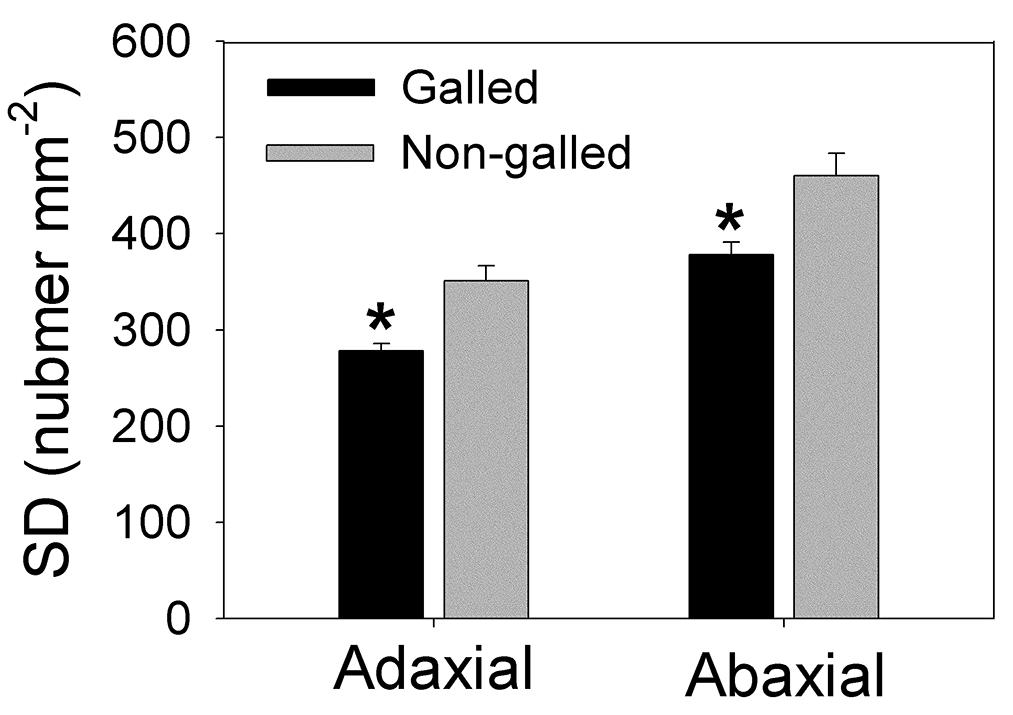
FIGURE 3. Stomatal density (SD) in adaxial and abaxial sides in galled and non-galled plants of Eucalyptus camaldulensis. An asterisk indicates a significant difference between galled and non-galled plants.
To determine the effect of galling of L. invasa on photosynthetic characteristics in E. camaldulensis, we measured chlorophyll content and gas change in galled and non-galled plants. The galled plants showed significantly lower chlorophyll content than the non-galled plants (Figure 4). Because chlorophyll content is mainly determined by leaf N content, this result suggested that galling of L. invasa decreased leaf N content. Light response curves indicated that galling of L. invasa significantly affected the values of gs (Figure 5A). Furthermore, as indicated by light response curves, the maximum rate of CO2 assimilation was depressed by the galling of L. invasa (Figure 5B). As a result, the primary productivity was affected by galling of L. invasa. Under light intensities above 1200 μmol photons m–2 s–1, values for effective quantum yield of PSII (ΦPSII) and electron flow through PSII (ETRII) were significantly lower in the galled-plants than non-galled plants (Figure 6), further indicating the depression of photosynthesis by the galling of L. invasa.
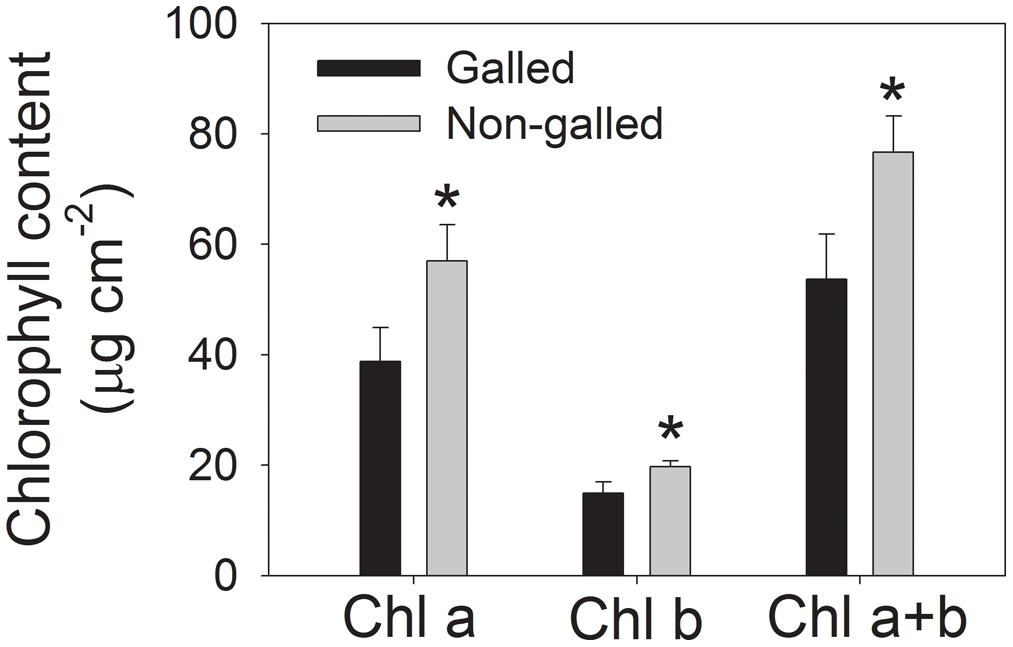
FIGURE 4. Chlorophyll content in galled and non-galled plants of Eucalyptus camaldulensis. An asterisk indicates a significant difference between galled and non-galled plants.
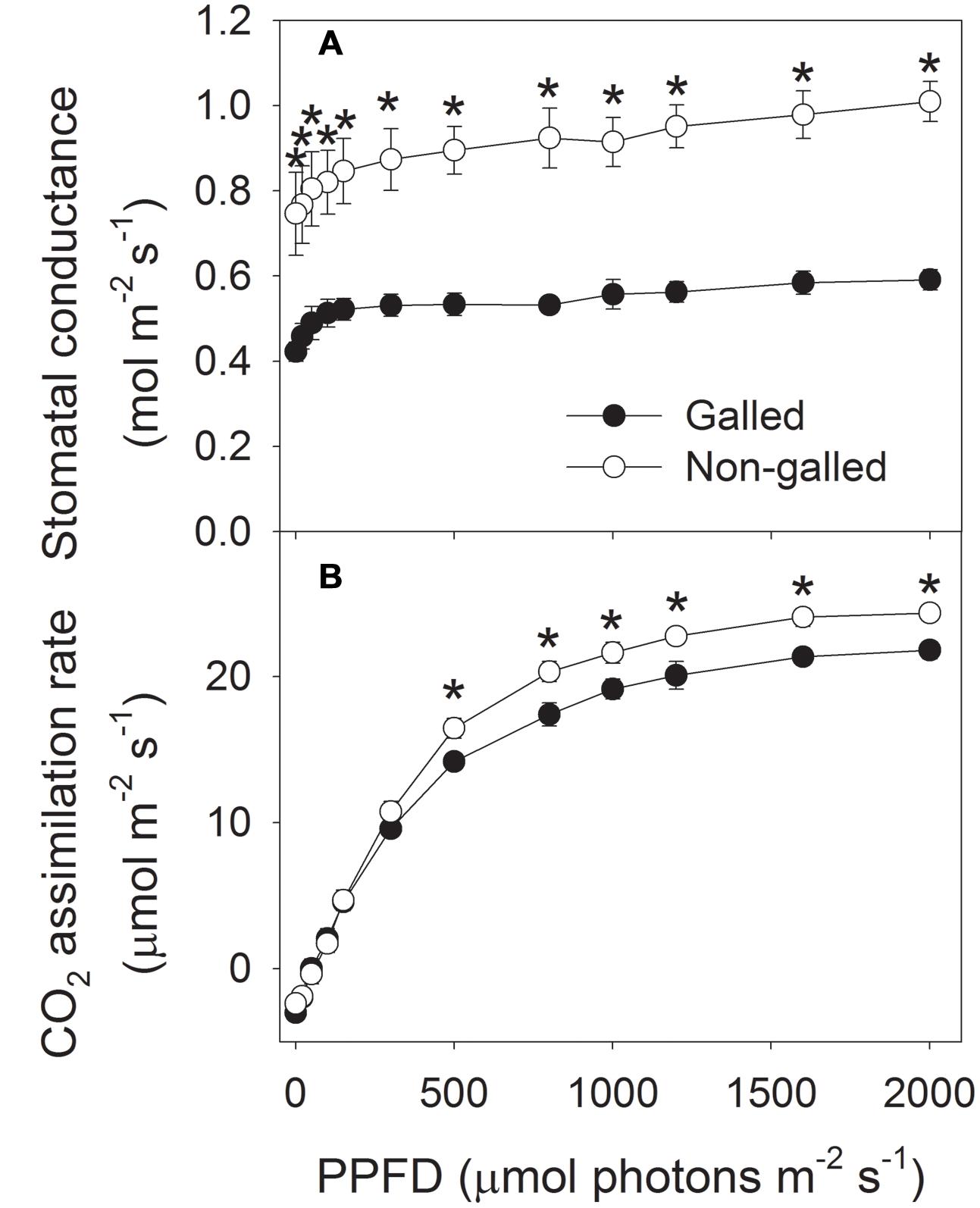
FIGURE 5. Responses of stomatal conductance (A) and CO2 assimilation rate (B) to incident light intensity in galled and non-galled plants of Eucalyptus camaldulensis. An asterisk indicates a significant difference between galled and non-galled plants.
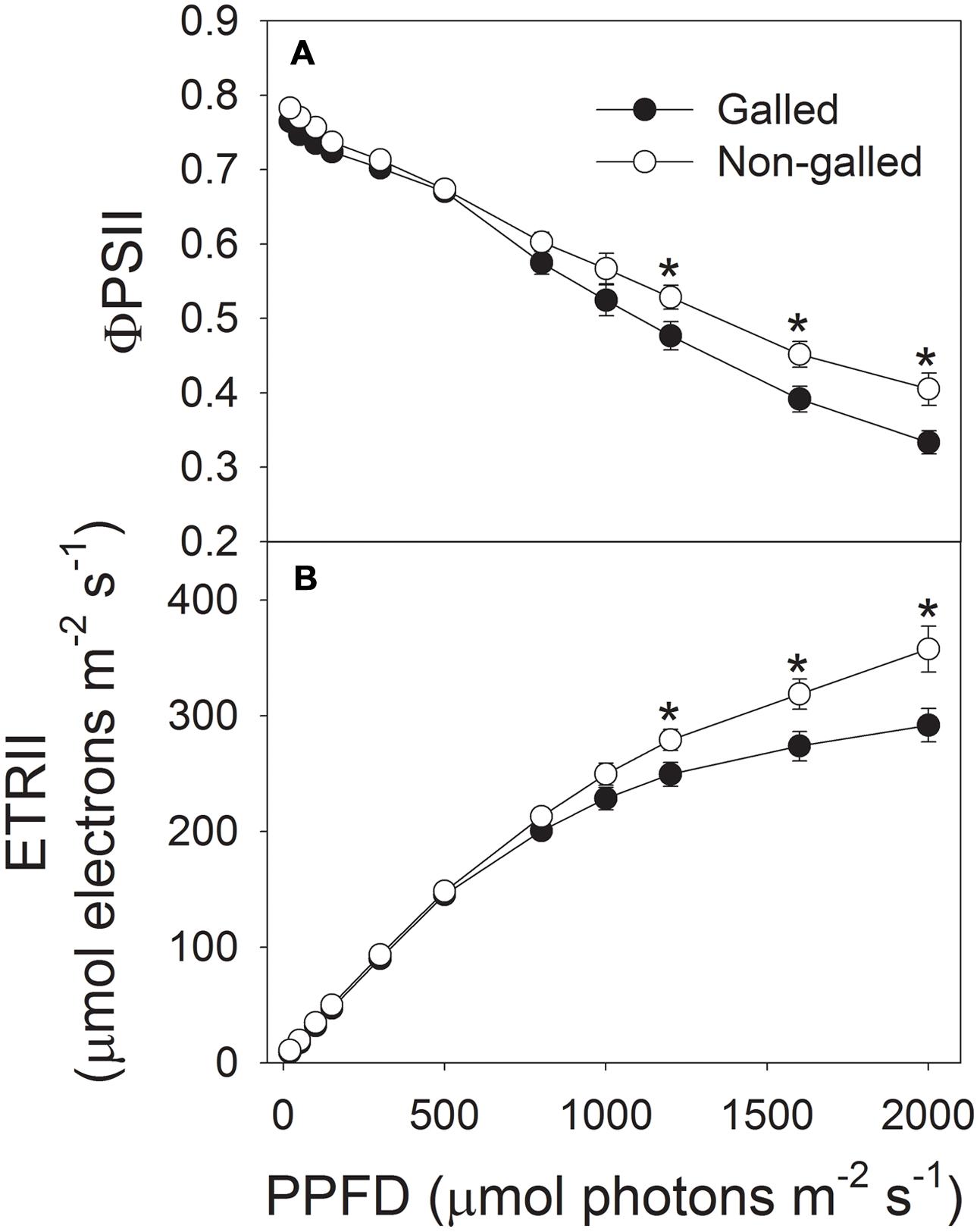
FIGURE 6. Responses of effective quantum yield of PSII (ΦPSII) (A) and electron flow through PSII (ETRII) (B) to incident light intensity in leaves of galled and non-galled plants of Eucalyptus camaldulensis. An asterisk indicates a significant difference between galled and non-galled plants.
Discussion
Gall density decreased with maturity of the host plant and was three times higher on juvenile plants compared with mature plants (Pires and Price, 2000). The distribution of attack in relation to plant age was not related to changes in shoot size with the age of the plants (Pires and Price, 2000). An infestation of L. invasa can cause stun of growth of the young trees of E. camaldulensis. Moreover, severe infestation may result in die-back and death of galled trees (Nyeko et al., 2009; Thu et al., 2009). However, the underlying physiological mechanisms are unclear. Here, we focused on the question whether galling of L. invasa affect the change in hydraulic function in E. camaldulensis. Particularly, we examined whether gall induction restricts stem hydraulic conductance and subsequently affects leaf functional traits.
We found that galling of L. invasa largely decreased the stem water transport in plants of E. camaldulensis. Theoretically, Kstem is mainly determined by conduit radius according to Hagen–Poiseuille equation (Hellemans et al., 1980). Conductance through an ideal pipe is proportional to the fourth power of its radius. As a result, a small increase in conduit radius leads to a large increase in vascular hydraulic conductance. The developing larvae of L. invasa form typical galls in stems, which hardly changes the conduit radius but can cause the jam of conduit. When the jam of conduit occurred, water transport through conduit would be restricted, leading to the decrease in Kstem. Furthermore, the galls caused by L. invasa increase the risk of cavitations of conduit that decline stem hydraulic conductance. Whatever which mechanism, the developing larvae of L. invasa largely decreased Kstem in E. camaldulensis. It is documented that the gall formation by L. invasa on shoots and leaves of E. camaldulensis results in quicker abscission of leaves and drying up of shoots and even death of trees (Nyeko et al., 2009; Thu et al., 2009). The data of Kstem presented here provided new evidence that infection of L. invasa caused hydraulic limitation in the galled plants of E. camaldulensis.
It has been indicated that Kstem plays an important role in determining leaf water status and development of leaf hydraulics (Sack and Frole, 2006; Zhu et al., 2013). Our results indicated that the infected plants of E. camaldulensis showed lower midday leaf potential. What is more, the MVD also decreased in the infected plants of E. camaldulensis. Because MVD is an important determining factor for leaf hydraulic conductance (Kleaf; Hu et al., 2014), the decrease in MVD can cause a decline Kleaf, which further restricts the water supply to leaf. Therefore, decreases in both Kstem and Kleaf induced the significant decline in midday leaf water potential in plants galled by L. invasa. Meanwhile, comparing with uninfected plants, SD decreased significantly in the infected plants. The parameter SD is corresponding to the potential transpirational demands in leaves. The coordination between MVD and SD allowed the leaves to maintain an efficient balance between water supply and transpiration. The MVD and SD usually develop coordinately in plants. For example, tobacco leaves grown at high temperature had higher values of MVD and SD than plants grown at lower temperature (Hu et al., 2014). Genotypes of Arabidopsis thaliana in which mature leaves have lower MVD tend to have reduced SD (Pérez-Pérez et al., 2011). In Paphiopedilum (Orchidaceae), evolutionary association of stomatal traits with leaf vein density is found (Zhang et al., 2012). Taking together, the declined SD in the galled plants diminished transpirational rate, thereby balancing water supply with transpirational demand.
In the infected plants of E. camaldulensis, mature leaves showed much lower gs, as indicated by light response curves. Stomatal conductance is a complex process that can be affected by several factors, such as leaf ABA content (Tardieu et al., 1991, 1992, 1996), SD (Tanaka et al., 2013), leaf water potential (Tardieu et al., 1996; Comstock and Mencuccini, 1998; Zhu et al., 2009; Choat et al., 2012), stem hydraulic conductance (Brodribb et al., 2007; Zhu et al., 2013), and leaf hydraulic conductance (Brodribb et al., 2007; Zhu et al., 2013). Because ABA content changed slightly between galled and non-galled plants (data not shown), the lower gs in the infected plants was independent on ABA content. In the galled plants, the decline in Kstem and Kleaf restricted water supply to leaves and caused a decrease in leaf water potential. Under such conditions, leaves of the galled plants closed stomata to reserve water and avoid severe water deficit. Furthermore, the decrease in gs can avoid over-cavitation of stem conduit (Zhang et al., 2013). Therefore, for the galled plants, the lower gs is an adaptive strategy to control transpiration in response to the changes in stem and leaf hydraulic conductance.
Gall formation has been found to significantly modify foliar gas exchange processes (Fay et al., 1993; Larson, 1998; Dorchin et al., 2006; Patankar et al., 2011). Cynipid wasp galls formed by Antistrophus silphii on Silphium integrifolium increased photosynthesis, stomatal conductance, and xylem water potential (Fay et al., 1993). In Acacia pycnantha, photosynthetic rates in phyllodes subtending clusters of galls by Trichilogaster signiventris were greater than rates in control phyllodes. However, a gall-inducing arthropod Vasates aceriscrumena caused declines in canopy photosynthetic rate and stomatal conductance in plants of Acer saccharum (Patankar et al., 2011). We found that the infection of L. invasa led to declines in leaf chlorophyll content, CO2 assimilation rate and photosynthetic electron flow. For a given plants grown at the same environmental conditions, leaf chlorophyll content is positively correlated with leaf nitrogen content (Yamori et al., 2011). For example, tobacco plant grown with high nitrogen concentration had higher leaf N content and chlorophyll content (Yamori et al., 2011). Therefore, the lower chlorophyll content in leaves of galled plants of E. camaldulensis indicated that gall wasp significantly decreased leaf N content. Nitrogen supply to leaf is dependent on stem and leaf hydraulic conductance. In galled plants, Kstem and Kleaf were restricted by the larvae of L. invasa. Furthermore, the development of larvae in the cortex of shoots or the midribs of leaves needs lots of organic matters including N, which further decreases the N supply in leaves. The decline in leaf nitrogen content induces a decrease in RuBisCO content and thus declines in CO2 assimilation rate and photosynthetic electron flow. For the infected plants, the rate of CO2 assimilation under high light was significantly lower than the non-galled plants. What is more, the galled plants have much lower leaf number, which largely decreases the primary productivity of whole plants, thereby slowed the growth of plants.
In summary, we found that the stem and leaf hydraulic conductance significantly decreased in the galled plants due to development of larvae in the cortex of shoots or the midribs of leaves. Subsequently, the development of stomata and MVD were affected. To balance water supply and transpiration, leaves of galled plants had lower SD and gs. In addition, transpiration in the galled plants was further controlled due to decreased leaf number and total leaf area. The decreased photosynthetic rate and lower leaf number restricted the primary productivity in galled plants. The present study strongly indicated that L. invasa largely declined the hydraulic conductance and then depressed the growth of E. camaldulensis.
Author Contributions
Conceived and designed the experiments: WH, Y-GT, and X-XD. Performed the experiments: WH, K-CZ, and XY. Analyzed the data: WH and YGT. Wrote the paper: WH, Y-GT, and X-XD.
Conflict of Interest Statement
The authors declare that the research was conducted in the absence of any commercial or financial relationships that could be construed as a potential conflict of interest.
The reviewer ZN and handling Editor declared their shared affiliation, and the handling Editor states that the process nevertheless met the standards of a fair and objective review.
Acknowledgments
This work was supported by National Natural Science Foundation of China (grant 31300332) and a fund from Forestry Bureau of Dongchuan County.
Supplementary Material
The Supplementary Material for this article can be found online at: http://journal.frontiersin.org/article/10.3389/fpls.2016.00130
FIGURE S1 | Representative paradermal sections from leaves of galled and non-galled plants. (A) abaxial side of non-galled plants; (B) adaxial side of non-galled plants; (C), abaxial of galled plants; (D) adaxial side of galled plants.
References
Anyia, A. O., and Herzog, H. (2004). Water-use efficiency, leaf area and leaf gas exchange of cowpeas under mid-season drought. Eur. J. Agron. 20, 327–339. doi: 10.1016/S1161-0301(03)00038-8
Baker, N. R., and Rosenqvist, E. (2004). Applications of chlorophyll fluorescence can improve crop production strategies: an examination of future possibilities. J. Exp. Bot. 55, 1607–1621. doi: 10.1093/jxb/erh196
Blanche, K. R. (2000). Diversity of insect-induced galls along a temperature-rainfall gradient in the tropical savannah region of the Northern Territory, Australia. Austral Ecol. 25, 311–318. doi: 10.1046/j.1442-9993.2000.01040.x
Blanche, K. R., and Westoby, M. (1995). Gall-forming insect diversity is linked to soil fertility via host plant taxon. Ecology 76, 2334–2337. doi: 10.2307/1941706
Brodribb, T. J., Feild, T. S., and Jordan, G. J. (2007). Leaf maximum photosynthetic rate and venation are linked by hydraulics. Plant Physiol. 144, 1890–1898. doi: 10.1104/pp.107.101352
Choat, B., Jansen, S., Brodribb, T. J., Cochard, H., Delzon, S., Bhaskar, R., et al. (2012). Global convergence in the vulnerability of forests to drought. Nature 491, 752–755. doi: 10.1038/nature11688
Chone, X., Leeuwen, C. V., Dubourdieu, D., and Gaudillere, J. P. (2001). Stem water potential is a sensitive indicator of grapevine water status. Ann. Bot. 87, 477–483. doi: 10.1006/anbo.2000.1361
Comstock, J., and Mencuccini, M. (1998). Control of stomatal conductance by leaf water potential in Hymenoclea salsola (T. & G.), a desert subshrub. Plant Cell Environ. 21, 1029–1038. doi: 10.1046/j.1365-3040.1998.00353.x
Dorchin, N., Cramer, M. D., and Hoffmann, J. H. (2006). Photosynthesis and sink activity of wasp-induced galls in Acacia pycnantha. Ecology 87, 1781–1791. doi: 10.1890/0012-9658(2006)87[1781:PASAOW]2.0.CO;2
Fay, P. A., Hartnett, D. C., and Knapp, A. K. (1993). Increased photosynthesis and water potentials in Silphium integrifolium galled by cynipid wasps. Oecologia 93, 114–120. doi: 10.1007/BF00321200
Genty, B., Briantais, J. M., and Baker, N. R. (1989). The relationship between the quantum yield of photosynthetic electron transport and quenching of chlorophyll fluorescence. Biochim. Biophys. Acta 99, 87–92. doi: 10.1016/S0304-4165(89)80016-9
Hellemans, J., Forrez, P., and Wilde, R. D. (1980). Experiment illustrating Bernoulli’s equation and Hagen–Poiseuille’s law. Am. J. Physiol. 48, 254–255. doi: 10.1119/1.12154
Hu, J., Yang, Q.-Y., Wei, H., Zhang, S.-B., and Hu, H. (2014). Effects of temperature on leaf hydraulic architecture of tobacco plants. Planta 240, 489–496. doi: 10.1007/s00425-014-2097-z
Kim, I., Mendel, Z., Protasov, A., Blumberg, D., and La Salle, J. (2008). Taxonomy, biology, and efficacy of two Australian parasitoids of the eucalyptus gall wasp, Leptocybe invasa Fisher & La Salle (Hymenoptera: Eulophidae: Tetrastichinae). Zootaxa 1910, 1–20.
Koch, G. W., Sillett, S. C., Jennings, G. M., and Davis, S. D. (2004). The limits to tree height. Nature 428, 851–854. doi: 10.1038/nature02417
Krall, J. P., and Edwards, G. E. (1992). Relationship between photosystem II activity and CO2 fixation in leaves. Physiol. Plant. 86, 180–187. doi: 10.1007/BF00016557
Larson, K. C. (1998). The impact of two gall-forming arthropods on the photosynthetic rates of their hosts. Oecologia 115, 161–166. doi: 10.1007/s004420050503
Lichtenthaler, H. K., and Wellburn, A. R. (1983). Determinations of total carotenoids and chlorophylls a and b in leaf extracts in different solvents. Biochem. Soc. Trans. 11, 591–592. doi: 10.1042/bst0110591
Mendel, Z., Protasov, A., Fisher, N., and La Salle, J. (2004). Taxonomy and biology of Leptocybe invasa gen. & sp.n. (Hymenoptera: Eulophidae), an invasive gall inducer on Eucalyptus. Aust. J. Entomol. 43, 101–113. doi: 10.1111/j.1440-6055.2003.00393.x
Nyeko, P., Mutitu, E. K., and Day, R. K. (2009). Eucalyptus infestation by Leptocybe invasa in Uganda. Afr. J. Ecol. 47, 299–307. doi: 10.1111/j.1365-2028.2008.01004.x
Patankar, R., Thomas, S. C., and Smith, S. M. (2011). A gall-inducing arthropod drives declines in canopy tree photosynthesis. Oecologia 167, 701–709. doi: 10.1007/s00442-011-2019-8
Pérez-Pérez, J. M., Rubio-Díaz, S., Dhondt, S., Hernández-Romero, D., Sánchez-Soriano, J., Beemster, G. T. S., et al. (2011). Whole organ, venation and epidermal cell morphological variations are correlated in the leaves of Arabidopsis mutants. Plant Cell Environ. 34, 2200–2211. doi: 10.1111/j.1365-3040.2011.02415.x
Pires, C. S. S., and Price, P. W. (2000). Patterns of host plant growth and attack and establishment of gall-inducing wasp (Hymenoptera: Cynipidae). Environ. Entomol. 29, 49–54. doi: 10.1603/0046-225X-29.1.49
Sack, L., and Frole, K. (2006). Leaf structural diversity is related to hydraulic capacity in tropical rain forest trees. Ecology 87, 483–491. doi: 10.1890/05-0710
Sperry, J. S., Hacke, U. G., Oren, R., and Comstock, J. P. (2002). Water deficits and hydraulic limits to leaf water supply. Plant Cell Environ. 25, 251–263. doi: 10.1046/j.0016-8025.2001.00799.x
Tanaka, Y., Sugano, S. S., Shimada, T., and Nishimura, I. H. (2013). Enhancement of leaf photosynthetic capacity through increased stomatal density in Arabidopsis. New Phytol. 198, 757–764. doi: 10.1111/nph.12186
Tardieu, F., Katerji, N., Bethenod, O., Zhang, J., and Davies, W. J. (1991). Maize stomatal conductance in the field: its relationship with soil and plant water potentials, mechanical constraints and ABA concentration in the xylem sap. Plant Cell Environ. 14, 121–126. doi: 10.1111/j.1365-3040.1991.tb01378.x
Tardieu, F., Lafarge, T., and Simonneau, T. (1996). Stomatal control by fed or endogenous xylem ABA in sunflower: interpretation of correlations between leaf water potential and stomatal conductance in anisohydric species. Plant Cell Environ. 19, 75–84. doi: 10.1111/j.1365-3040.1996.tb00228.x
Tardieu, F., Zhang, J., Katerji, N., Bethenod, O., Palmer, S., and Davies, W. J. (1992). Xylem ABA controls the stomatal conductance of field-grown maize subjected to soil compaction or soil drying. Plant Cell Environ. 15, 193–197. doi: 10.1111/j.1365-3040.1992.tb01473.x
Thu, P. Q., Dell, B., and Burgess, T. I. (2009). Susceptibility of 18 eucalypt species to the gall wasp Leptocybe invasa in the nursery and young plantations in Vietnam. Sci. Asia 35, 113–117. doi: 10.2306/scienceasia1513-1874.2009.35.113
Yamori, W., Nagai, T., and Makino, A. (2011). The rate-limiting step for CO2 assimilation at different temperatures is influenced by the leaf nitrogen content in several C3 crop species. Plant Cell Environ. 34, 764–777. doi: 10.1111/j.1365-3040.2011.02280.x
Zhang, J.-L., and Cao, K.-F. (2009). Stem hydraulics mediates leaf water status, carbon gain, nutrient use efficiencies and plant growth rates across dipterocarp species. Funct. Ecol. 23, 658–667. doi: 10.1111/j.1365-2435.2009.01552.x
Zhang, S.-B., Guan, Z.-J., Sun, M., Zhang, J.-J., Cao, K.-F., and Hu, H. (2012). Evolutionary association of stomatal traits with leaf vein density in Paphiopedilum, Orchidaceae. PLoS ONE 7:e40080. doi: 10.1371/journal.pone.0040080
Zhang, Y.-J., Meinzer, F. C., Qi, J.-H., Goldstein, G., and Cao, K.-F. (2013). Midday stomatal conductance is more related to stem rather than leaf water status in subtropical deciduous and evergreen broadleaf trees. Plant Cell Environ. 36, 149–158. doi: 10.1111/j.1365-3040.2012.02563.x
Zhu, J.-J., Zhang, J.-L., Liu, H.-C., and Cao, K.-F. (2009). Photosynthesis, non-photochemical pathways and activities of antioxidant enzymes in a resilient evergreen oak under different climatic conditions from a valley-savanna in Southwest China. Physiol. Plant. 135, 62–72. doi: 10.1111/j.1399-3054.2008.01171.x
Zhu, S.-D., Song, J.-J., Li, R.-H., and Ye, Q. (2013). Plant hydraulics and photosynthesis of 34 woody species from different successional stages of subtropical forests. Plant Cell Environ. 36, 879–891. doi: 10.1111/pce.12024
Keywords: Eucalyptus camaldulensis, gall wasp, hydraulic function, Leptocybe invasa, photosynthesis
Citation: Tong Y-G, Ding X-X, Zhang K-C, Yang X and Huang W (2016) Effect of the Gall Wasp Leptocybe invasa on Hydraulic Architecture in Eucalyptus camaldulensis Plants. Front. Plant Sci. 7:130. doi: 10.3389/fpls.2016.00130
Received: 15 October 2015; Accepted: 23 January 2016;
Published: 15 February 2016.
Edited by:
Abdul Latif Khan, University of Nizwa, OmanCopyright © 2016 Tong, Ding, Zhang, Yang and Huang. This is an open-access article distributed under the terms of the Creative Commons Attribution License (CC BY). The use, distribution or reproduction in other forums is permitted, provided the original author(s) or licensor are credited and that the original publication in this journal is cited, in accordance with accepted academic practice. No use, distribution or reproduction is permitted which does not comply with these terms.
*Correspondence: Wei Huang, aHVhbmd3ZWlAbWFpbC5raWIuYWMuY24=
†These authors have contributed equally to this work.