- 1Institute of Plant Science and Resources, Okayama University, Okayama, Japan
- 2Graduate School of Science, Hiroshima University, Hiroshima, Japan
- 3Institute of Low Temperature Science, Hokkaido University, Hokkaido, Japan
Chlorophylls (Chl) in photosynthetic apparatuses, along with other macromolecules in chloroplasts, are known to undergo degradation during leaf senescence. Several enzymes involved in Chl degradation, by which detoxification of Chl is safely implemented, have been identified. Chl degradation also occurs during embryogenesis and seedling development. Some genes encoding Chl degradation enzymes such as Chl b reductase (CBR) function during these developmental stages. Arabidopsis mutants lacking CBR (NYC1 and NOL) have been reported to exhibit reduced seed storability, compromised germination, and cotyledon development. In this study, we examined aberrant cotyledon development and found that NYC1 is solely responsible for this phenotype. We inferred that oxidative damage of chloroplast membranes caused the aberrant cotyledon. To test the inference, we attempted to trans-complement nyc1 mutant with overexpressing VIPP1 protein that is unrelated to Chl degradation but which supports chloroplast membrane integrity. VIPP1 expression actually complemented the aberrant cotyledon of nyc1, whereas stay-green phenotype during leaf senescence remained. The swollen chloroplasts observed in unfixed cotyledons of nyc1, which are characteristics of chloroplasts receiving envelope membrane damage, were recovered by overexpressing VIPP1. These results suggest that chloroplast membranes are a target for oxidative damage caused by the impairment in Chl degradation. Trans-complementation of nyc1 with VIPP1 also suggests that VIPP1 is useful for protecting chloroplasts against oxidative stress.
Introduction
At the onset of leaf senescence, an important physiological process at the final stage of leaf development, a leaf is reallocated from a sink to a source organ, from which macromolecules synthesized during the sink phase are degraded and are subsequently redistributed into the upper tissues or reproductive organs. Various factors such as transcriptional regulations, phytohormones, reactive oxygen species (ROS), and environmental factors influence leaf senescence progression (Lim et al., 2007; Woo et al., 2013). The major targets of the degradation processes have been demonstrated to reside in chloroplasts: macromolecules in chlo roplasts, such as protein, lipid, nucleic acid and pigment, undergo degradation (Krupinska, 2006; Hörtensteiner, 2013; Kato and Sakamoto, 2013; Sakamoto and Takami, 2014). Furthermore, autophagy has been demonstrated to incorporate chloroplasts during senescence (Ishida and Yoshimoto, 2008; Wada et al., 2009). These degradation processes contribute to the efficient recycling and redistribution of nitrogen compounds.
Among the degraded compounds in senescing leaves, chlorophyll (Chl) is the most abundant pigment. Higher plants contain both Chl a and Chl b in the light harvesting antennae and only Chl a in the reaction center of Photosystem I (PSI) and PSII. Chl is necessary for photosynthesis because the excitation energy received by Chl drives the water splitting reaction and subsequent electron transport. In contrast, an excited state of free Chl is toxic because it generates ROS ultimately leading to cell death. Chl degradation is therefore implicated as a safe means of detoxification (Tanaka and Tanaka, 2011; Hörtensteiner, 2013). Conversion of Chl b to Chl a mediated by Chl b reductase (CBR) is an initial step for Chl degradation (Ito et al., 1996; Rüdiger, 2002; Kusaba et al., 2007) because only Chl a undergoes the subsequent degradation pathway that involves (i) de-chelation of Chl a (pheophytin), (ii) removal of phyto-tail (pheophorbide a), and (iii) oxygenation of rings, leading to the production of non-toxic primary fluorescent catabolites. Mutations that impair any of the reactions above give rise to a ‘stay-green’ phenotype in which leaves implement senescence but retain Chl (Kusaba et al., 2013). This phenotype, designated as cosmetic stay-green, is apparent in many plant species such as Arabidopsis (Oh et al., 2004; Ren et al., 2007), rice (Kusaba et al., 2007; Park et al., 2007), pea (Armstead et al., 2007; Sato et al., 2007), tomato (Cheung et al., 1993; Akhtar et al., 1999), and soybean (Luquez and Guiamét, 2002; Fang et al., 2014).
Reportedly, Chl degradation takes place not only in senescing leaves but also during embryogenesis and cotyledon development. De-greening of developing embryos is usually observed in Arabidopsis and other species (Chao et al., 1995; Nakajima et al., 2012). Some enzymes for Chl degradation pathway appear to be up-regulated at these stages. Although the physiological implications of Chl degradation during embryogenesis and cotyledon development remain unclear, it is likely that Chl are catabolized properly during embryo maturation. Supporting the importance of Chl degradation in embryo, Nakajima et al. (2012) reported that the Arabidopsis mutant lacking CBR, Non-Yellow Coloring 1 (NYC1) and “NYC1-Like” (NOL1) caused the impairment of seed maturation and storability. This observation strongly implies that Chl accumulated in seeds are liable to cause oxidative damage to photosystems or peroxidation of chloroplast membranes, by which seeds do not show lethality but show reduced fitness.
In this study, we hypothesize that one target for the damage caused by impaired Chl degradation is chloroplast membranes. Previously, we demonstrated that VIPP1 plays a crucially important role in protecting photosynthetic membranes (Zhang et al., 2012; Zhang and Sakamoto, 2013, 2015). VIPP1 was originally identified as being abundant in envelopes (Li et al., 1994). Based on the seedling-lethal phenotype in vipp1 mutant lacking vesicle formation, VIPP1 was suggested to play a role in thylakoid formation through vesicle fusion (Kroll et al., 2001; Westphal et al., 2001). However, its precise function remains elusive. VIPP1 forms an extremely large homo-complex (>2 MDa) that is associated with inner envelope and thylakoid membranes. The disassembly/reassembly of the VIPP1 complex, often designated as VIPP1 functional particle, takes place according to the membrane damage (Aseeva et al., 2004; Liu et al., 2005, 2007). It is particularly interesting that we recently demonstrated that overexpression of VIPP1 prevents chloroplast membranes from being damaged against heat shock and hypotonic stress. Therefore, it is possible to assume that VIPP1 overexpression improves seed defects in Arabidopsis nyc1 mutant. Our results demonstrate that this is indeed the case, corroborating our hypothesis that the membrane integrity can be disturbed by Chl remaining in seeds. Our results also corroborate that VIPP1 functions in preventing chloroplast membranes from oxidative damage.
Materials and Methods
Material Preparation and Growth Conditions
Arabidopsis thaliana ecotype Columbia (Col) was used as the wild type in this study. The stay-green mutants, nyc1, nol, and BCG, were described previously. Briefly, both nyc1 (corresponding to AT4G13250) and nol (corresponding to AT5G04900) are T-DNA insertion mutants that were identified by Horie et al. (2009). BCG refers to Arabidopsis transformant with a transgene containing BC domain of CAO fused with GFP at its C-terminus (Sakuraba et al., 2010). VIPP1-GFP/nyc1, VIPP1-GFP/nol, and VIPP1-GFP/BCG were generated, respectively, by crossing VIPP1-GFP/Col (Zhang et al., 2012) with nyc1, nol or BCG. Homozygous transformants were selected from the third generation. In the case of VIPP1-GFP/nyc1 and VIPP1-GFP/nol, the corresponding T-DNA insertions at nyc1 and nol loci were verified using PCR (primers 5′-TCAGTAGCACAGTCTTTCGCTC-3′ and 5′-GCGTTATATGCAGCAGAAGC-3′ for nyc1; primers 5′-TGTTGGTCTCCCATCTGAAC-3′ and 5′-GGCTACTTGGAGTGGTTTCA-3′ for nol). Expression of VIPP1-GFP/BCG was confirmed by Western blotting with antibody against VIPP1 and GFP. Seeds of these lines were surface-sterilized and were kept at 4°C. After 2 days, the seeds were sown onto 0.7% MS agar plates supplemented with 1.5% (w/v) sucrose. Seedlings were growing under light (low light of 35 μmol photon m–2 s–1 or normal light of 70 μmol photon m–2 s–1) with 12 h/12 h light/dark cycle at a constant temperature of 22°C. After a 2-weeks growth period on MS medium, the seedlings were transferred to soil.
Darkness Treatment and Re-greening of Cotyledons
Sterilized seeds of Col, nyc1, and VIPP1-GFP/nyc1 were kept at 4°C for 2 days; then they were sown on MS medium and kept in darkness by coverage with aluminum foil for 5 days at 22°C. Subsequently the foil was removed and seedlings on the MS plates were exposed to the light with intensity of 70 μmol photon m–2 s–1. The seedling phenotypes of different lines were recorded after 0, 1, 5, or 9-days re-greening. Darkness treatment was also used to induce the senescence of detached mature leaves of Col, nyc1, and VIPP1-GFP/nyc1. The protocol of dark-induced leaf senescence was fundamentally similar to that described by Sato et al. (2009). The mature leaves detached from 4-weeks-old plants were incubated at 22°C in darkness for 5 days.
Chlorophyll Measurement
Chlorophyll (Chl) was extracted with 80% (v/v) acetone. Chl a and Chl b were determined, respectively, by absorbance at 663 and 645 nm with a spectrophotometer (Amersham Biosciences, Sweden). Quantification of Chl were calculated following the equations of Arnon’s (1949) method: total Chl (μg ml–1) = 20.2 (A645) + 8.02 (A663), Chl a (μg ml–1) = 12.7 (A663) – 2.69 (A645), Chl b (μg ml–1) = 22.9 (A645) – 4.68 (A663).
Microscopy Observation
The cotyledons of 12-days-old seedlings of different lines were used for microscopic observation, as described in a previous report (Zhang et al., 2012). The chloroplasts/plastids in living leaf tissues were examined using a fluorescence microscope (DSU-BX51; Olympus Corp.) under a 100× objective lens with oil. For observation of Arabidopsis seeds, autofluorescence from the intact seeds was monitored directly under a microscope (DSU-BX51; Olympus Corp.) with a filter set (U-MWIB2). Corresponding bright-field photographs were recorded simultaneously.
Protein Exaction, SDS-PAGE, and Western Blotting
For immunobloting, total proteins of 12-days-old seedlings were extracted through grinding with loading buffer (125 mM Tris-Cl, pH 6.8, 2% [w/v] SDS, 5% [v/v] glycerol, 5% [v/v] 2-mercaptoethanol, and 0.05% [w/v] bromophenol blue) directly. The extract was denatured continuously at 95°C for 5 min. Samples were loaded on SDS-PAGE in the equal fresh weight. One SDS-PAGE was stained with Coomassie Brilliant Blue R 250 as loading control. The other gel was electroblotted onto polyvinylidene difluoride membrane (Atto Corp.). The membrane was blocked with 5% (w/v) milk in PBST buffer (50 mM sodium phosphate buffer, pH 7.5, 155 mM NaCl, and 0.05% [v/v] Tween 20) for 1 h. After three washes with PBST buffer, the membranes were incubated with anti-VIPP1 (Aseeva et al., 2007) for 2 h. After washing three times again with PBST buffer, the membranes were incubated with second antibodies for 2 h. Proteins were detected using an ECL chemiluminescence detection system (Amersham Biosciences Corp.) as described in a previous report (Sakamoto et al., 2003).
Histochemical Detection of H2O2
In situ detection of hydrogen peroxide was performed by staining with 3,3′-diaminobenzidine (DAB, Sigma-Aldrich) according to the previous method (Daudi et al., 2012). Briefly, the detached cotyledons of different lines were infiltrated for 5 min under gentle vacuum with 1 mg/mL DAB containing 0.05% (v/v) Tween 20 and 10 mM sodium phosphate buffer (pH 7.0). At least five independent plants were used as biological replicates, and three cotyledons were sampled from each plant. Staining reaction was terminated 5 h after DAB infiltration. The pigments in the DAB-stained seedlings were removed with ethanol: acetic acid: glycerol (3:1:1) in a water bath at 95°C for 15 min. DAB staining was observed under white light and photographed.
Results
Aberrant Seedling Development in nyc1
In the course of characterizing Arabidopsis stay-green mutants or transgenic lines that are impaired in Chl degradation, we found that some showed defective growth at the seedling stage. We particularly examined the mutants lacking NYC1 and NOL: the enzymes involved in the first step of Chl degradation by conversion of Chl b to Chl a. It has been demonstrated that NYC1 and NOL act redundantly or differentially in Arabidopsis because only nyc1 mutant exhibits the stay-green leaf phenotype (Horie et al., 2009). In rice, in contrast, both NYC1 and NOL act synergistically by forming a heterocomplex in thylakoid membranes: a mutant lacking either NYC1 or NOL shows stay-green (Kusaba et al., 2007; Sato et al., 2009).
When the seeds from Arabidopsis nyc1 and nol were sown on MS agar plates supplemented with sucrose, we observed that nyc1 developed chlorotic cotyledons (Figures 1A,B). In contrast, nol developed normal-appearing cotyledons. No defects in seedling were detected. Aberrant seedlings in nyc1 were small and visible soon after cotyledon development (at least 4 days after sowing), showing small white or pale-green cotyledons occasionally leading to wilting. We have used at least three batches of seed stocks and reproducibly observed this aberrant seedling development only in nyc1 (81% of seedling exhibited white or pale cotyledons). Despite the effect in cotyledons, first and subsequent true leaves emerged normally and were green (Figure 1B). When transplanted to soil and further grown for 2 weeks, nyc1 seedlings appeared to grow normally, showing no visible phenotypes in our growth condition. At this stage, Chl a/b ratio of these plants grown in soil showed no marked difference between wild type Columbia and nyc1. Nakajima et al. (2012) reported that nyc1nol double mutant showed drastic reduction of germination rate along with prolonged storage of the seeds. That impaired germination was possibly induced by toxic Chl breakdown products. For this study, we sowed the 3-months-old seeds of nyc1 and nol mutant on MS medium, which revealed that the germination rate of both mutants is similar with that of wild type (Figure 1A). These results suggest that nyc1 has a defect in cotyledon formation, but it has no profound effect on the lateral leaf development.
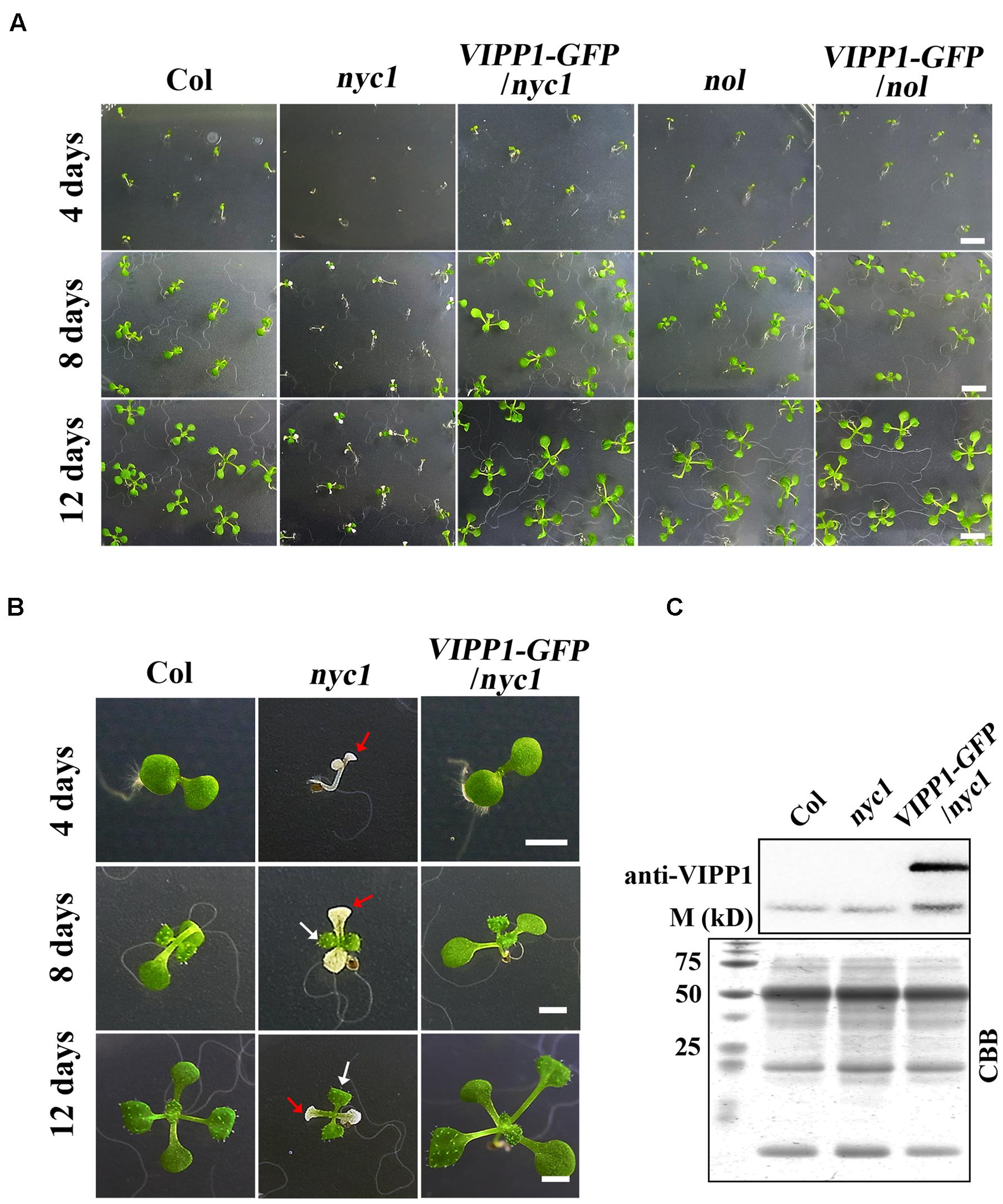
FIGURE 1. Defective seedling growth in nyc1 and its recovery in VIPP1-GFP/nyc1. (A) Germination and seedling development of Col, nyc1, VIPP1-GFP/nyc1, nol, and VIPP1-GFP/nol on MS-agar plate supplemented with 1.5% sucrose under normal light with 12 h/12 h light/dark cycle at 22°C. Photographs of the seedlings were taken 4, 8, or 12 days after sowing, respectively, as shown at the left. Bars = 5 mm. (B) Close-up view of representative seedlings of Col, nyc1, and VIPP1-GFP/nyc1 during seedling development at 4, 8, and 12 days after sowing. In nyc1, the cotyledon was defective and white (red arrows), whereas the first true leaf was green (white arrows). Bars = 2 mm. (C) Immunoblots of total protein extract from Col, nyc1, and VIPP1-GFP/nyc1 (12-days-old individuals as shown in (B) were probed with anti-VIPP1. Loading was based on the equal total protein. Proteins stained with Coomassie Brilliant Blue (CBB) after SDS-PAGE are shown as loading control at the bottom. M denotes the molecular marker shown along with size.
Cotyledon Re-greening of nyc1 Was Affected by Light Intensity
Given that nyc1/nol seeds accumulate chlorophyll-derived compounds as detected by excess fluorescence in Nakajima et al. (2012), the aberrant seedling development is probably caused by oxidative stress and is therefore light-dependent. To test this possibility, we grew Col, nyc1, and nol under three light conditions (darkness, low light of 35 μmol photon m–2 s–1, and normal light of 70 μmol photon m–2 s–1) and observed germination and cotyledon greening. Again, no great difference in germination rates was found between wild type and two mutants, irrespective of light conditions (Figures 2A,B). In contrast, cotyledon re-greening of nyc1 mutant differs with that of Col and nol under different light irradiation (Figures 2A,B). In nyc1, cotyledons presented various colors: green, pale-green, or white. The ratio of green cotyledons over germinated seedlings was 11.8%, which in turn increased to 28.7% when the light intensity was decreased to 35 from 70 μmol photon m–2 s–1. Therefore, re-greening of the nyc1 cotyledon appeared to result from photo-oxidative damage.
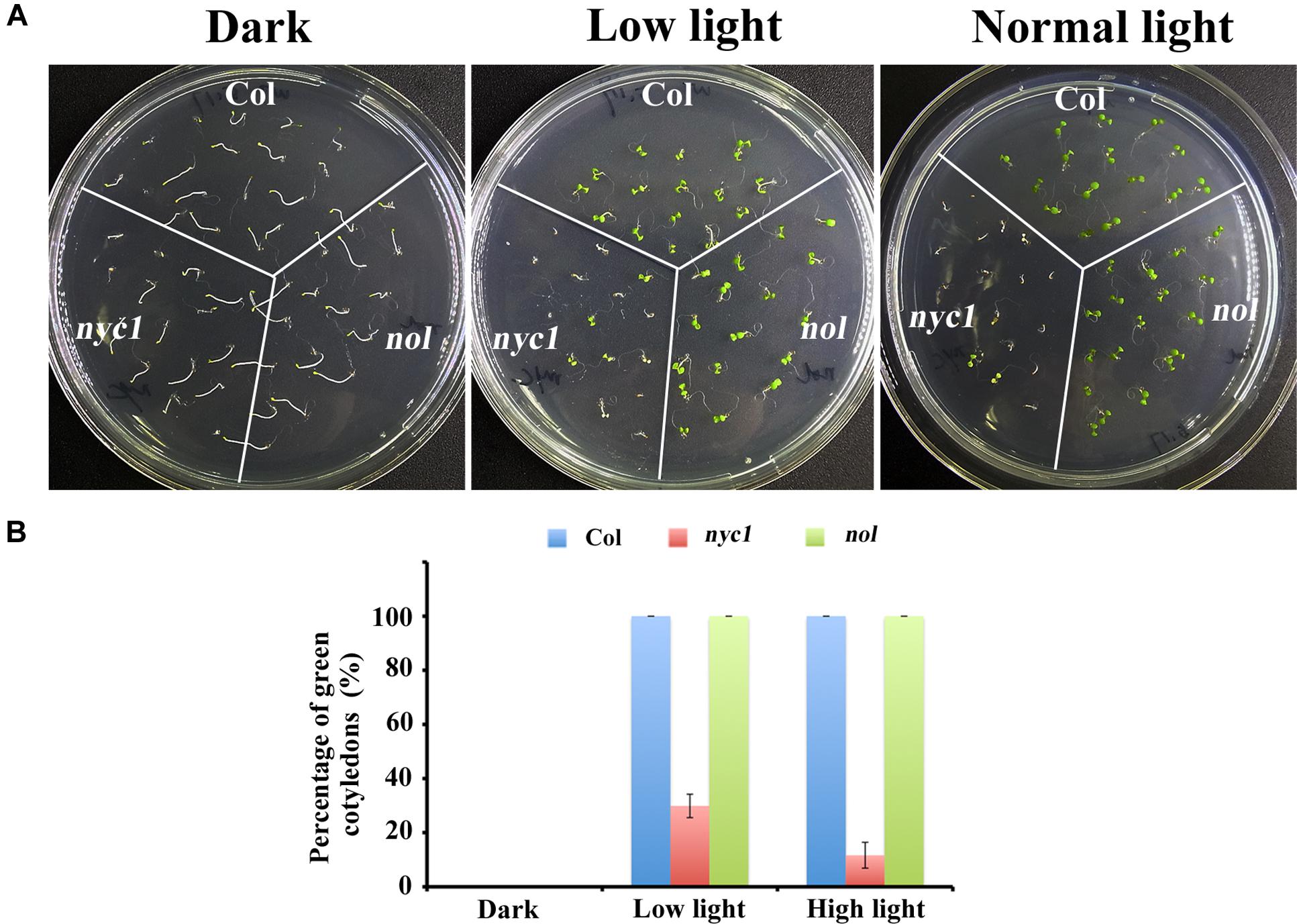
FIGURE 2. Effect of light on germination and seedling development of Col, nyc1, and nol. (A) Photographs of 5-days-old seedlings from Col, nyc1, and nol. Sterilized seeds were sown on MS-agar plate and were kept under three light conditions: darkness (plate was covered with aluminum), low light (30 μmol photon m–2 s–1), and normal light (70 μmol photon m–2 s–1). (B) Rate of green cotyledons over all seedling in Col (Blue), nyc1 (red), and nol (green) measured under three light conditions as noted at the bottom. Data shown in (B) are means of three independent experiments with error bars representing the SD.
Expression of VIPP1-GFP Recovers Aberrant Seedling Growth in nyc1
In this study, we attempted to overexpress VIPP1 in nyc1 to test if the aberrant cotyledon development is mitigated. We reported previously that VIPP1 which is C-terminally tagged with GFP (VIPP1-GFP) rescues seedling lethality of vipp1 knock-down (vipp1-kd) and knock-out (vipp1-ko) mutants (Zhang et al., 2012). Moreover, the transgenic line overexpressing VIPP1-GFP (termed VIPP1-GFP/Col) were shown to increase tolerance to recovery from heat shock when detached mature leaves were examined. To this end, we crossed VIPP1-GFP/Col with nyc1. The resulting progeny were further characterized to obtain VIPP1-GFP/nyc1 that harbored both nyc1 allele and VIPP1-GFP transgene as homozygous. As expected, our Western blotting confirmed substantial accumulation of VIPP1-GFP in VIPP1-GFP/nyc1 (Figure 1C), which appeared to be comparable to that described in a previous report (Zhang et al., 2012). Visual inspection of VIPP1-GFP/nyc1 revealed that it grew normally at the post-germination stage, representing normal cotyledons with size equivalent to that of wild type Columbia (Col; Figures 1A,B). Moreover, chlorotic cotyledons were never detected in the progeny of VIPP1-GFP/nyc1. These results demonstrated that overexpression of VIPP1 can trans-complement the aberrant growth of nyc1 at the seedling stage.
VIPP1 Overexpression Promote the Greening of Yellow nyc1 Cotyledon
To address the protection effect of VIPP1 on nyc1 cotyledons further, we next examined re-greening processes during transition from etioplast to chloroplast. Seeds of Col, nyc1, and VIPP1-GFP/nyc1 were germinated on MS-agar plates. Prolonged incubation in darkness for 5 days led these seedlings to develop long hypocotyl and etiolated cotyledon (Figure 3A, top panel). Subsequently, these etiolated seedlings were exposed to normal light (70 μmol proton m–2 s–1). Cotyledons of Col and VIPP1-GFP/nyc1 turned green from yellow (Figure 3A), implying that etioplasts of these yellow cotyledons were properly converted into chloroplasts upon light irradiation. However, cotyledons of nyc1 exhibited a range of colors from white to green (Figure 3A), indicating that some etioplasts cannot form chloroplasts. Even after prolonged light exposure of up to 12 days, some cotyledons of nyc1 mutant did not turn green, but true leaves developed normally (Figure 3B). We conclude that the aberrant cotyledon in nyc1 is caused partly by photo-oxidative damage in chloroplast membranes, which impairs the etioplast–chloroplast transition.
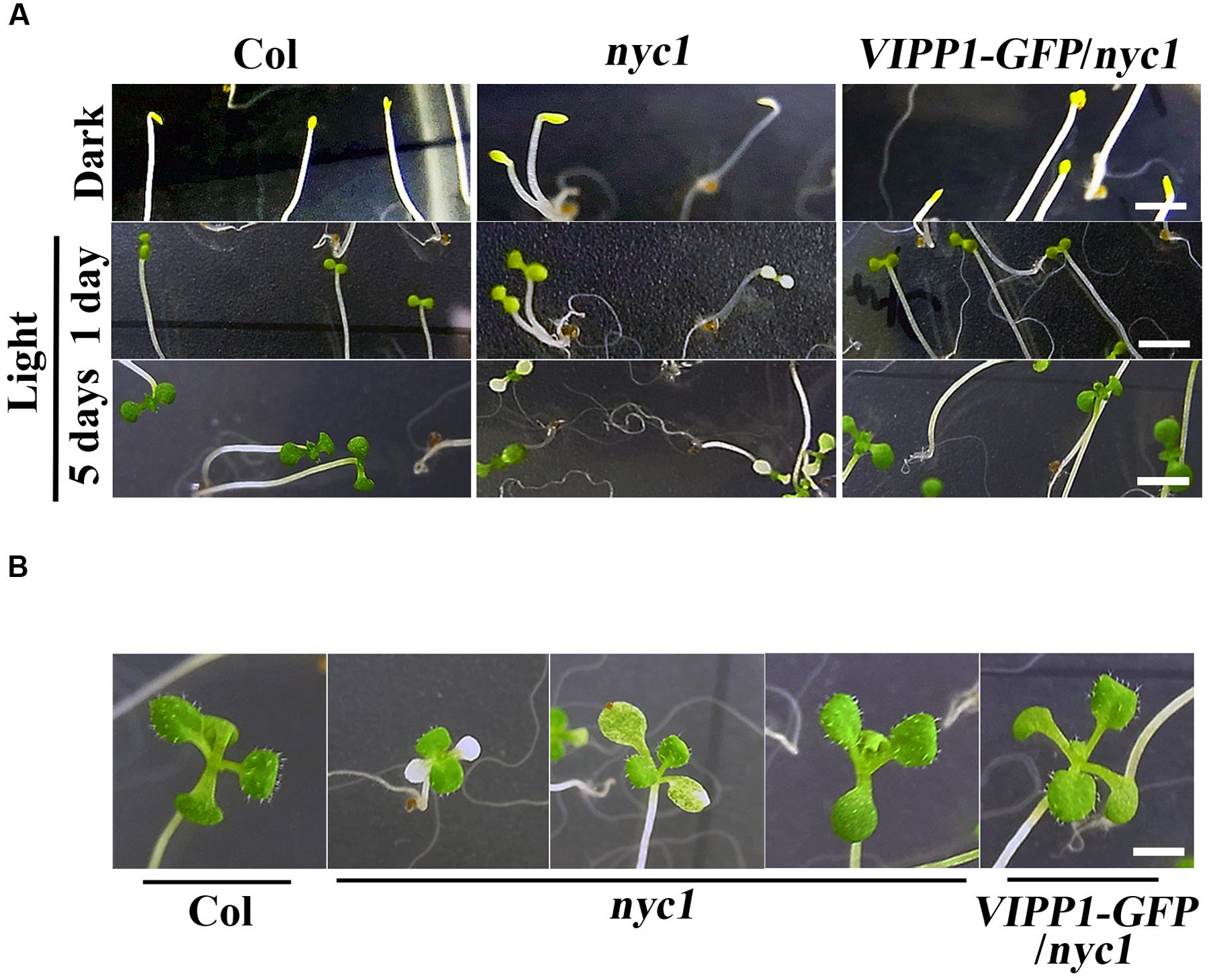
FIGURE 3. Re-greening of yellow cotyledons of Col, nyc1, and VIPP1-GFP/nyc1. (A) Seedling phenotypes of Col, nyc1, and VIPP1-GFP/nyc1. Seeds of Col, nyc1, and VIPP1-GFP/nyc1 were sown on MS-agar plate and grown further for 5 days in darkness (top panel) with subsequent exposure to normal light (70 μmol photon m–2 s–1) for 1 day (middle panel) or for 5 days (bottom panel). Bars = 5 mm. (B) Photographs of seedlings from Col, nyc1, and VIPP1-GFP/nyc1. These were taken 9 days after 5-days growth in darkness. Bars = 5 mm.
VIPP1-GFP/nyc1 Is Stay-Green Similarly to nyc1
A possibility that explains trans-complementation of nyc1 with VIPP1-GFP is that VIPP1 assists in degrading chlorophylls for unknown reasons, although it is unlikely. This possibility can be excluded by observing the stay-green phenotype of nyc1 and VIPP1-GFP/nyc1: these plants are expected to contain higher levels of Chl during leaf senescence. To examine this conjecture, we followed a previous report (Sato et al., 2009) and adopted dark-induced senescence of detached mature leaves. After incubation in darkness for 5 days, leaves of Col turned yellow and Chl were degraded, whereas VIPP1-GFP/nyc1 leaves, as well as those of nyc1, remained green because of Chl retention (Figures 4A,B). Moreover, reduced Chl a/b ratios in nyc1 and VIPP1-GFP/nyc1 indicated that both were deficient in CBR activity and that they indeed accumulated more Chl b (Figure 4B, right panel). In an earlier study, retention of Chl in nyc1 was likely to vary among the seeds, some exhibiting high fluorescence and others showing lower fluorescence (Nakajima et al., 2012). Although the exact compounds emitting fluorescence remained unclear, high fluorescence was regarded as derived from Chl degradation products. Similarly to nyc1, seeds of VIPP1-GFP/nyc1 also showed varied degrees of fluorescence (Figure 4C). Most of the wild-type seeds exhibited low fluorescence, indicating that a low level of Chl was retained in the wild-type seeds. These results demonstrate that overexpression of VIPP1 did not affect Chl degradation of the nyc1 mutant.
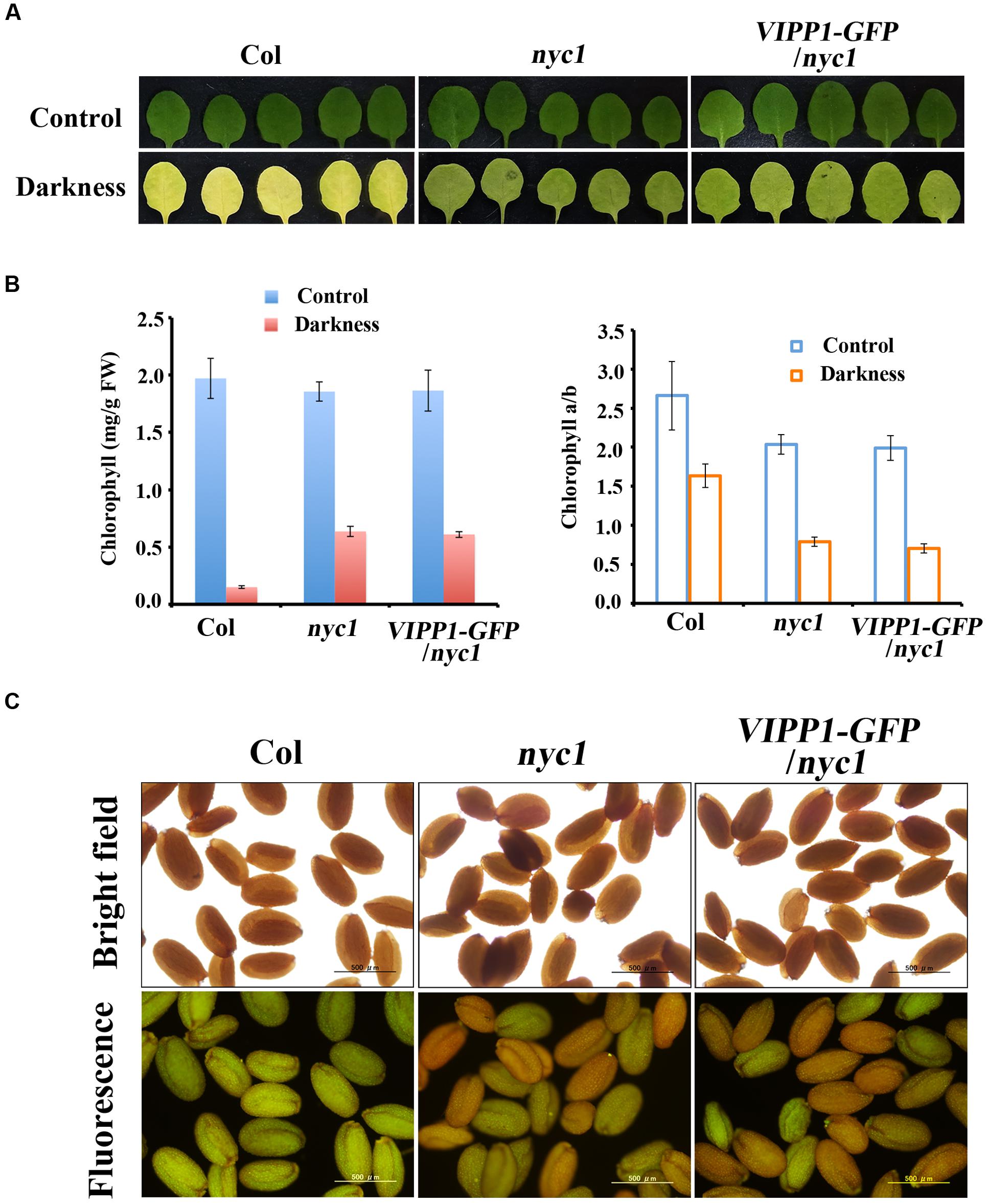
FIGURE 4. Stay-green phenotype and retention of Chl and fluorescence in nyc1 and VIPP1-GFP/nyc1. (A) Dark-induced senescence of mature leaves from Col, nyc1, and VIPP1-GFP/nyc1. Detached leaves of 3-weeks-old plants were incubated in darkness for 5 days at 22°C. Photographs of representative leaves before and after dark treatment are shown, respectively, as control (top panel) and darkness (bottom panel). (B) Total Chl content (left) and Chl a/b ratio (right) were calculated from the leaves as shown in (A). Three independent experiments were performed and error bars (SD) are represented as SD. Values before and after dark treatment are shown, respectively, as control (blue) and darkness (red). (C) Fluorescence emission of seeds from Col, nyc1, and VIPP1-GFP/nyc1 detected by microscopy (bottom panel). Photographs of the same seeds taken by bright field are shown at the top.
Envelope Damage Assessed by Chloroplast Swelling in nyc1 Cotyledons
As confirmed by previously described data, trans-complementation with VIPP1 strongly suggests that the defective re-greening of nyc1 is correlated with membrane damage in chloroplasts. Therefore, membrane integrity in chloroplasts, particularly that of envelopes, was assessed by observing chloroplasts in cotyledons. Our previous work demonstrated that the lack or decreased level of VIPP1 in vipp1-ko and vipp1-kd causes chloroplasts to form swelling of envelopes when chloroplasts in leaf tissues are observed directly: an enlarged stroma area within the chloroplast engenders ‘balloon-like’ chloroplasts, which are only slightly detectable in wild type (Figure 5). It is interesting that we were able to observe chloroplasts/plastids in white cotyledons of nyc1 that showed the swelling phenotype under microscopy (see Materials and Methods). Although, the appearance of such chloroplasts in these lines was rare (approximately 1% or less), it was detected consistently. However, we never detected them in Col. More importantly, the appearance of balloon-like chloroplasts in nyc1 were recovered by expressing VIPP1-GFP (Figure 5). These results strongly support our supposition that the recovery of the aberrant cotyledon development results from the improved tolerance of chloroplast membranes against oxidative damage.
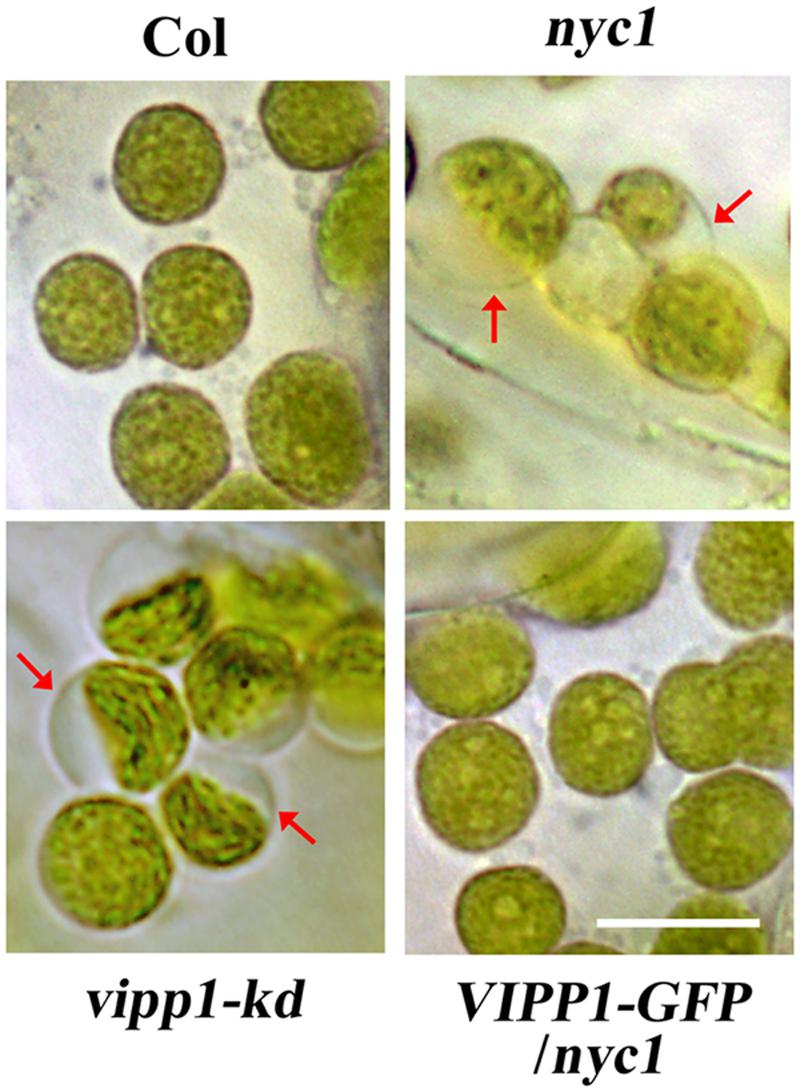
FIGURE 5. Chloroplasts of unfixed cotyledons from nyc1 and BCG show balloon-like structure characteristic to defective envelope. Representative photographs of chloroplasts in unfixed cotyledons from Col, nyc1, vipp1-kd, and VIPP1-GFP/nyc1 were taken by microscopic observation under bright field. Balloon-like chloroplasts that are characteristic of weakened envelope integrity (red arrows) were detected only in vipp1-kd and nyc1 but recovered in the lines overexpressing VIPP1-GFP. Bars = 10 μm.
Discussion
The aberrant cotyledon phenotype described in this study (Figure 1) has been briefly reported by Nakajima et al. (2012), in which the authors rather focused on the effect of impaired Chl degradation on seed storability. They noted that nyc1/nol double mutant displays the aberrant cotyledon that looked similar to what is reported in this study, showing plant-to-plant variability from white to pale yellow colors. However, this phenotype has not been investigated further. Here, we demonstrated that it is nyc1, but not nol, from which the aberrant phenotype derives: it is also the case in which in Arabidopsis, nyc1 shows a cosmetic stay-green phenotype during leaf senescence, whereas nol does not (Horie et al., 2009). These findings together suggest that unlike rice, NYC1 plays a major role in Chl degradation not only of senescing leaves but also of seed maturation. Furthermore, we demonstrate that light intensity affects the appearance of aberrant cotyledons (Figure 2), which partly explains why the penetrance of the cotyledon color phenotype varied. Chl acts as a potential photosensitizer when not integrated into the photosystems (Hörtensteiner, 2004). It is therefore likely that Chl degradation in seed tissues must be tightly regulated to maximize fitness. Although, no difference in germination rate was detected in our study (Figure 2), retention of Chl in the mature seeds has been implicated as correlated with the lower seed germination rate (Jalink et al., 1998). We detected only the aberrant cotyledon, not lowered germination rate, probably because we used seeds that had been stored only for a short period (3 months).
We reasoned that our result of trans-complementation should be confirmed by some other means because we used only one allele of nyc1. A stay-green transgenic line (termed BCG) showing the phenotype similar to nyc1 was reported by Sakuraba et al. (2012). Instead of knockout in NYC1, BCG overproduces Chl b oxygenase (CAO) that engenders increased Chl b levels. As a consequence, BCG was shown to exhibit a prolonged stay-green in senescing leaves. CAO consists of three domains (respectively designated as A, B, and C), and A domain negatively regulates CAO accumulation in vivo. To circumvent this negative regulation, BCG expressed CAO that contains only B and C domains: because BC-CAO is tagged with GFP, the resulting transgene (and transgenic line) was designated as BCG (Sakuraba et al., 2010). We found that under our growth condition (70 μmol photon m–2 s–1), BCG cotyledons show white or pale yellow as seen in nyc1 (Figure 6). Interestingly, introduction of VIPP1-GFP into BCG recovers the aberrant cotyledon phenotype (Figure 6). VIPP1-GFP/BCG apparently retained the stay-green phenotype in senescing leaves. Moreover, the envelope damage assessed by the balloon-like chloroplasts in cotyledons was also recovered by VIPP1-GFP (Figure 6). Results from VIPP1-GFP/BCG were consistent with VIPP1-GFP/nyc1, leading us to confirm that VIPP1 trans-complemented aberrant cotyledon development because of the disturbance in Chl degradation.
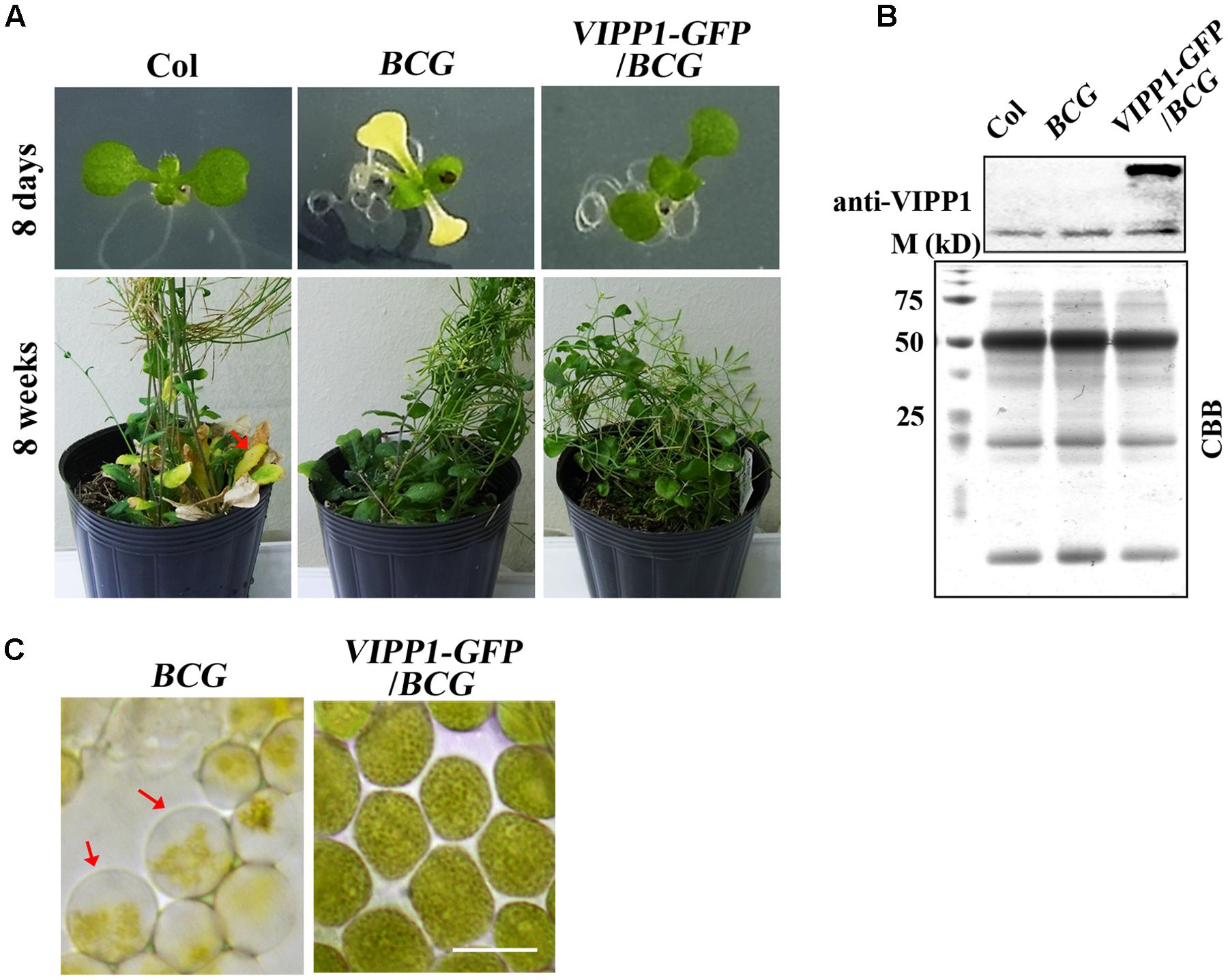
FIGURE 6. Defective seedling growth in BCG and its recovery in VIPP1-GFP/BCG. (A) Photographs of representative seedlings (8-days-old, top panel) and mature plants (8-weeks-old, bottom panel) of Col, BCG, and VIPP1-GFP/BCG. Aberrant cotyledon development of BCG shown in yellow is rescued by VIPP1 overexpression in VIPP1-GFP/BCG (top panel), whereas late senescence phenotype is retained in both lines (bottom panel). (B) Immunoblot of total protein extract from Col, BCG, and VIPP1-GFP/BCG was probed with anti-VIPP1 as presented in Figure 1C. (C) Recovery of the balloon-like chloroplasts in BCG (indicated by red arrows) by expressing VIPP1-GFP. Bars = 10 μm.
The similar phenotype between nyc1 and BCG implies tight regulation of Chl levels during cotyledon development. In contrast to the cotyledon, however, true leaves of nyc1 and BCG develop normally because of their distinct differentiation paths in these two organs. Chloroplasts in true leaves originate from proplastids of apical meristem cells, where robust thylakoid membranes are formed in a light-dependent manner. Chloroplasts in cotyledons are differentiated upon germination from pre-existing plastids, which contain thylakoid membranes developed during embryogenesis. Together, our data enable us to conclude that Chl degradation is necessary for the initial stage of plant life: seedling development.
Given the light-dependent chlorotic phenotype, we inferred that overproduction of Chl (and perhaps its degradation compounds detected as high fluorescence, Figure 4) tend to accumulate excitation energy that is not transferred to photosystems and ultimately engender production of toxic molecules such as ROS. Under this scenario, it was speculated that ROS accumulates more in nyc1 than Col cotyledons. However, histochemical detection of hydrogen peroxide showed no significant difference (Supplementary Figure S1). Perhaps subtle and transient difference in ROS accumulation is sufficient to result in aberrant cotyledons. Sakuraba et al. (2010) has shown that in BGC plants, true leaves accumulate high ROS in a light-dependent manner, which suggests that excess Chl may lead to photo-oxidative stress. Reportedly, oxidative stress is one of the factors that affect the seed storability (Sattler et al., 2004). Another remaining question is whether excess Chl can associate with envelopes or in its vicinity, which subsequently affects envelope membrane integrity. We infer that it is unlikely, because Nakajima et al. (2012) reports that not only Chl but also LHCII exist in nyc1 cotyledons. Although the physiological status of plastids in aberrant cotyledons is unclear, excess Chl appeared to form LHC, similarly to those in senescing leaves. A possibility that VIPP1 scavenges ROS directly is also unlikely: no such implication has been suggested.
Numerous mutants showing white cotyledons have been reported in Arabidopsis (e.g., sco1, cyo1, sco2, sig2). For example, cyo1 exhibits white cotyledons; CYO1 has been shown to have disulfide reductase activity (Shimada et al., 2007). Preferential expression of CYO1 in young seedlings implicates that, although the precise substrate of CYO1 remains unclear, redox regulation is crucial in cotyledons. Unlike nyc1, however, the appearance of white cotyledons in cyo1 is independent of the light intensity and does not result from photobleaching. It has been suggested that ROS generation in chloroplasts renders membranes sensitive to oxidative stress damage (Mahajan and Tuteja, 2005). Chloroplast membrane stability has been compromised when membrane lipid peroxidation has resulted from ROS (Anjum et al., 2011; Lata et al., 2011). Therefore, it seems reasonable to assume that one target damaged by Chl over-accumulation in the cotyledons of nyc1 is the chloroplast membrane.
Supporting this assumption, results of this study show that chloroplast envelopes in green cotyledons of nyc1 sustained membrane damage, as evidenced by balloon-like chloroplasts (Figure 5). Direct observation of chloroplasts in unfixed leaf tissues enables us to infer that chloroplast swelling is indicative of hypotonic emphasize that disturbs envelope membrane potential. Originally, we used this method to ascertain the important role of VIPP1 in envelope integrity: vipp1-kd shows the typical balloon-like chloroplasts (Zhang et al., 2012). VIPP1 is found specifically in photosynthetic organisms and is a multifunctional protein originated from bacterial ancestral protein PspA. It has a C-terminal extension that does not exist in PspA, has no transmembrane helix, and associates with membranes by forming large complexes (Zhang and Sakamoto, 2015). The large homo complex consists of VIPP1 function particles that mutually associate, thereby forming various dot-like, rod-like, and lattice-like structures. Various functions such as vesicle formation, maintenance of photosystems, protein import across thylakoid membranes, envelope maintenance, and membrane fusion have been inferred. Along with the lethal phenotype of vipp1-ko, there observations suggest that VIPP1 plays a fundamental role in maintaining chloroplast membranes. Trans-complementation of nyc1 with VIPP1 overexpression also suggests an important role of VIPP1 in chloroplast maintenance.
Conclusion
We drew two conclusions from our current study. First, one target for photo-oxidative damage caused by impaired Chl degradation is chloroplast membranes, particularly during seedling formation. VIPP1 apparently has little effect on stay-green phenotype in nyc1 because membranes undergo extensive destruction during leaf senescence. In addition, the possibility that VIPP1 assists in degrading Chl is excluded because none of VIPP1-GFP/nyc1 or VIPP1-GFP/BCG recovered stay-green during leaf senescence. A second conclusion is that VIPP1 can prevent chloroplasts from receiving damage against oxidative stress. In this regard, trans-complementation of nyc1 by overexpressing VIPP1 is related to tolerance against heat shock in VIPP1-GFP/Col, as found from our earlier study. The present study therefore reinforces our notion that VIPP1 is important to protect chloroplast membranes against environmental stresses.
Stay-green is a key plant trait with wide usage for managing crop production. Functional stay-green can increase biomass and grain yield of crop through delayed senescence of the photosynthetic organs (Kusaba et al., 2013). Although cosmetic stay-green is unlikely to contribute to high yields, it is useful for high-quality food production (e.g., evergreen leaves, grasses). The shortcoming of Chl retention inside seeds appears to accompany a low germination rate (Parcy et al., 1997; Nakajima et al., 2012), which can be expected to hinder the practical application of stay-green trait. In this paper, we presented the possibility that VIPP1 overcomes such weakness when its overexpression is combined with stay-green.
Author Contributions
WS, MK, AT designed the work; LZ, WS, AT prepared the materials; LZ and WS performed the experiments; LZ, WS, MK, AT analyzed the data; WS and LZ wrote the manuscript.
Funding
This work was supported by the Japan Science and Technology Agency (Core Research for Evolutional Science and Technology) and the Ohara Foundation.
Conflict of Interest Statement
The authors declare that the research was conducted in the absence of any commercial or financial relationships that could be construed as a potential conflict of interest.
Acknowledgment
The authors thank Yusuke Kato, Rie Hijiya, and Tsuneaki Takami for their technical assistance.
Supplementary Material
The Supplementary Material for this article can be found online at: http://journal.frontiersin.org/article/10.3389/fpls.2016.00533
FIGURE S1 | Histochemical detection of H2O2 in cotyledons. Seedlings from Col, nyc1, and VIPP1-GFP/nyc1 were grown on MS plates for 2 weeks under light with intensity of 70 μmol photons m–2 s–1. Detached cotyledons of these lines were used for H2O2 detection with DAB staining as described in Materials and Methods. Bars = 0.5 mm.
References
Akhtar, M. S., Goldschmidt, E. E., John, I., Rodoni, S., Matile, P., and Grierson, D. (1999). Altered patterns of senescence and ripening in gf, a staygreen mutant of tomato (Lycopersicon esculentum Mill.). J. Exp. Bot. 336, 1115–1122. doi: 10.1093/jxb/50.336.1115
Anjum, S. A., Xie, X. Y., Wang, L. C., Saleem, M. F., Man, C., and Lei, W. (2011). Morphological, physiological and biochemical responses of plants to drought stress. Afr. J. Agric. Res. 6, 2026–2032.
Armstead, I., Donnison, I., Aubry, S., Harper, J., Hörtensteiner, S., James, C., et al. (2007). Cross-species identification of Mendel’s I locus. Science 315:73. doi: 10.1126/science.1132912
Arnon, D. I. (1949). Copper enzymes in isolated chloroplasts: phenol oxidase in Beta vulgaris. Plant Physiol. 24, 1–15. doi: 10.1104/pp.24.1.1
Aseeva, E., Ossenbühl, F., Eichacker, L. A., Wanner, G., Soll, J., and Vothknecht, U. C. (2004). Complex formation of Vipp1 depends on its alpha-helical PspA-like domain. J. Biol. Chem. 279, 35535–35541. doi: 10.1074/jbc.M401750200
Aseeva, E., Ossenbühl, F., Sippel, C., Cho, W. K., Stein, B., Eichacker, L. A., et al. (2007). Vipp1 is required for basic thylakoid membrane formation but not for the assembly of thylakoid protein complexes. Plant Physiol. Biochem. 45, 119–128. doi: 10.1016/j.plaphy.2007.01.005
Chao, W. S., Liu, V., Thomson, W. W., Platt, K., and Walling, L. L. (1995). The impact of chlorophyll-retention mutation, d1d2 and cyt-G1, during embryogeny in soybean. Plant Physiol. 107, 253–262.
Cheung, A. Y., McNellis, T., and Piekos, B. (1993). Maintenance of chloroplast components during chromoplast differentiation in the tomato mutant green flesh. Plant Physiol. 101, 1223–1229.
Daudi, A., Cheng, Z., O’Brien, J. A., Mammarella, N., Khan, S., Ausubel, F. M., et al. (2012). The apoplastic oxidative burst peroxidase in Arabidopsis is a major component of pattern-triggered immunity. Plant Cell 24, 275–287. doi: 10.1105/tpc.111.093039
Fang, C., Li, C., Li, W., Wang, Z., Zhou, Z., Shen, Y., et al. (2014). Concerted evolution of D1 and D2 to regulate chlorophyll degradation in soybean. Plant J. 77, 700–712. doi: 10.1111/tpj.12419
Horie, Y., Ito, H., Kusaba, M., Tanaka, R., and Tanaka, A. (2009). Participation of chlorophyll b reductase in the initial step of the degradation of light harvesting chlorophyll a/b-protein complexes in Arabidopsis. J. Biol. Chem. 284, 17449–17456. doi: 10.1074/jbc.M109.008912
Hörtensteiner, S. (2004). The loss of green color during chlorophyll degradation-a prerequisite to prevent cell death? Planta 219, 191–194. doi: 10.1007/s00425-004-1231-8
Hörtensteiner, S. (2013). Update on the biochemistry of chlorophyll breakdown. Plant Mol. Biol. 82, 505–517. doi: 10.1007/s11103-012-9940-z
Ishida, H., and Yoshimoto, K. (2008). Chloroplasts are partially mobilized to the vacuole by autophagy. Autophagy 4, 961–962. doi: 10.4161/auto.6804
Ito, H., Ohtsuka, T., and Tanaka, A. (1996). Conversion of chlorophyll b to chlorophyll a via 7-hydroxymethyl chlorophyll. J. Biol. Chem. 271, 1475–1479. doi: 10.1074/jbc.271.3.1475
Jalink, H., Frandas, A., van Der Schoor, R., and Bino, J. B. (1998). Chlorophyll fluorescence of the testa of Brassica oleracea seeds as an indicator of seed maturity and seed quality. Sci. Agric. 55, 88–93. doi: 10.1590/S0103-90161998000500016
Kato, Y., and Sakamoto, W. (2013). “Plastid protein degradation during leaf development and senescence: role of proteases and chaperones,” in Plastid Development in Leaves during Growth and Senescence, Advances in Photosynthesis and Respiration, Vol. 36, eds B. Biswal, K. Krupinska, and U. C. Biswal (Dordrecht: Springer), 453–477.
Kroll, D., Meierhoff, K., Bechtold, N., Kinoshita, M., Westphal, S., Vothknecht, U. C., et al. (2001). VIPP1, a nuclear gene of Arabidopsis thaliana essential for thylakoid membrane formation. Proc. Natl. Acad. Sci. U.S.A. 98, 4238–4242. doi: 10.1073/pnas.061500998
Krupinska, K. (2006). “Fate and activities of plastids during leaf senescence,” in The Structure and Function of Plastids, eds R. R. Wise and J. K. Hoober (Amsterdam: Springer) 433–449.
Kusaba, M., Ito, H., Morita, R., Iida, S., Sato, Y., Fujimoto, M., et al. (2007). Rice NON-YELLOW COLORING1 is involved in light-harvesting complex II and grana degradation during leaf senescence. Plant Cell 19, 1362–1375. doi: 10.1105/tpc.106.042911
Kusaba, M., Tanaka, A., and Tanaka, R. (2013). Stay-green plants: what do they tell us about the molecular mechanism of leaf senescence. Photosynth. Res. 117, 221–234. doi: 10.1007/s11120-013-9862-x
Lata, C., Bhutty, S., Bahadur, R. P., Majee, M., and Prasad, M. (2011). Association of a SNP in a novel DREB2-like gene SiDREB2 with stress tolerance in foxtail millet [Setaria italica(L.)]. J. Exp. Bot. 62, 3387–3401. doi: 10.1093/jxb/err016
Li, H. M., Kaneko, Y., and Keegstra, K. (1994). Molecular cloning of a chloroplastic protein associated with both the envelope and thylakoid membranes. Plant Mol. Biol. 25, 619–632. doi: 10.1007/BF00029601
Lim, P. O., Kim, H. J., and Nam, H. G. (2007). Leaf senescence. Annu. Rev. Plant Biol. 58, 115–136. doi: 10.1146/annurev.arplant.57.032905.105316
Liu, C. M., Willmund, F., Golecki, J. R., Cacace, S., Hess, B., Markert, C., et al. (2007). The chloroplast HSP70B-CDJ2-CGE1 chaperones catalyse assembly and disassembly of VIPP1 oligomers in Chlamydomonas. Plant J. 50, 265–277. doi: 10.1111/j.1365-313X.2007.03047.x
Liu, C. M., Willmund, F., Whitelegge, J. P., Hawat, S., Knapp, B., Lodha, M., et al. (2005). J-domain protein CDJ2 and HSP70B are a plastidic chaperone pair that interacts with vesicleinducing protein in plastids 1. Mol. Biol. Cell 16, 1165–1177. doi: 10.1091/mbc.E04-08-0736
Luquez, V. M., and Guiamét, J. J. (2002). The stay green mutations d1 and d2 increase water stress susceptibility in soybeans. J. Exp. Bot. 376, 1421–1428. doi: 10.1093/jexbot/53.373.1421
Mahajan, S., and Tuteja, N. (2005). Cold, salinity and drought stresses: an overview. Arch. Biochem. Biophys. 444, 139–158. doi: 10.1016/j.abb.2005.10.018
Nakajima, S., Ito, H., Tanaka, R., and Tanaka, A. (2012). Chlorophyll b reductase plays an essential role in maturation and storability of Arabidopsis seeds. Plant Physiol. 160, 261–273. doi: 10.1104/pp.112.196881
Oh, M. H., Kim, J. H., Moon, Y. H., and Lee, C. H. (2004). Defects in a proteolytic step of light-harvesting complex II in an Arabidopsis stay-green mutant, ore10, during dark-induced leaf senescence. J. Plant Biol. 47, 330–337. doi: 10.1007/BF03030548
Parcy, F., Valon, C., Kohara, A., Misera, S., and Giraudat, J. (1997). The ABSCISIC ACID-INSENSITIVE3, FUSCA3, and LEAFY COTYLEDON1 loci act in concert to control multiple aspects of Arabidopsis seed development. Plant Cell 9, 1265–1277. doi: 10.1105/tpc.9.8.1265
Park, S. Y., Yu, J. W., Park, J. S., Li, J., Yoo, S. C., Lee, N. Y., et al. (2007). The senescence-induced stay green protein regulates chlorophyll degradation. Plant Cell 19, 1649–1664. doi: 10.1105/tpc.106.044891
Ren, G. D., An, K., Liao, Y., Zhou, X., Cao, Y. J., Zhao, H. F., et al. (2007). Identification of a novel chloroplast protein AtNYE1 regulating chlorophyll degradation during leaf senescence in Arabidopsis. Plant Physiol. 144, 1429–1441. doi: 10.1104/pp.107.100172
Rüdiger, W. (2002). Biosynthesis of chlorophyll b and the chlorophyll cycle. Photosynth. Res. 74, 187–193. doi: 10.1023/A:1020959610952
Sakamoto, W., and Takami, T. (2014). Nucleases in higher plants and their possible involvement in DNA degradation during leaf senescence. J. Exp. Bot. 65, 3835–3843. doi: 10.1093/jxb/eru091
Sakamoto, W., Zaltsman, A., Adam, Z., and Takahashi, Y. (2003). Coordinated regulation and complex formation of yellow variegated1 and yellow variegated2, chloroplastic FtsH metalloproteases involved in the repair cycle of photosystem II in Arabidopsis thylakoid membranes. Plant Cell 15, 2843–2855. doi: 10.1105/tpc.017319
Sakuraba, Y., Schelbert, S., Park, S. Y., Han, S. H., Lee, B. D., Andrès, C. B., et al. (2012). STAY-GREEN and chlorophyll catabolic enzymes interact at light-harvesting complex II for chlorophyll detoxification during leaf senescence in Arabidopsis. Plant Cell 24, 507–518. doi: 10.1105/tpc.111.089474
Sakuraba, Y., Yokono, M., Akimoto, S., Tanaka, R., and Tanaka, A. (2010). Deregulated chlorophyll b synthesis reduces the energy transfer rate between photosynthetic pigments and induces photodamage in Arabidopsis thaliana. Plant Cell Physiol. 51, 1055–1065. doi: 10.1093/pcp/pcq050
Sato, Y., Morita, R., Katsuma, S., Nishimura, M., Tanaka, A., and Kusaba, M. (2009). Two short-chain dehydrogenase/reductases, NON-YELLOW COLORING 1 and NYC1-LIKE, are required for chlorophyll b and light-harvesting complex II degradation during senescence in rice. Plant J. 57, 120–131. doi: 10.1111/j.1365-313X.2008.03670.x
Sato, Y., Morita, R., Nishimura, M., Yamaguchi, H., and Kusaba, M. (2007). Mendel’s green cotyledon gene encodes a positive regulator of the chlorophyll-degrading pathway. Proc. Natl. Acad. Sci. U.S.A. 104, 14169–14174. doi: 10.1073/pnas.0705521104
Sattler, S. E., Gilliland, L. U., Magallanes-Lundback, M., Pollard, M., and DellaPenna, D. (2004). Vitamin E is essential for seed longevity and for preventing lipid peroxidation during germination. Plant Cell 16, 1419–1432. doi: 10.1105/tpc.021360
Shimada, H., Mochizuki, M., Ogura, K., Froehlich, J. E., Osteryoung, K. W., Shirano, Y., et al. (2007). Arabidopsis cotyledon-specific chloroplast biogenesis factor CYO1 is a protein disulfide isomerase. Plant Cell 19, 3157–3169. doi: 10.1105/tpc.107.051714
Tanaka, R., and Tanaka, A. (2011). Chlorophyll cycle regulates the construction and destruction of the light-harvesting complexes. Biochim. Biophys. Acta 1807, 968–976. doi: 10.1016/j.bbabio.2011.01.002
Wada, S., Ishida, H., Izumi, M., Yoshimoto, K., Ohsumi, Y., Mae, T., et al. (2009). Autophagy plays a role in chloroplast degradation during senescence in individually darkened leaves. Plant Physiol. 149, 885–893. doi: 10.1104/pp.108.130013
Westphal, S., Heins, L., Soll, J., and Vothknecht, U. C. (2001). Vipp1 deletion mutant of Synechocystis: A connection between bacterial phage shock and thylakoid biogenesis? Proc. Natl. Acad. Sci. U.S.A. 98, 4243–4248. doi: 10.1073/pnas.061501198
Woo, H. R., Kim, H. J., Nam, H. G., and Lim, P. O. (2013). Plant leaf senescence and death – regulation by multiple layers of control and implications for aging in general. J. Cell Sci. 126, 4823–4833. doi: 10.1242/jcs.109116
Zhang, L. G., Kato, Y., Otters, S., Vothknecht, U. C., and Sakamoto, W. (2012). Essential role of VIPP1 in chloroplast envelope maintenance in Arabidopsis. Plant Cell 24, 3695–3707. doi: 10.1105/tpc.112.103606
Zhang, L. G., and Sakamoto, W. (2013). Possible function of VIPP1 in thylakoids: protection but not formation? Plant Signal. Behav. 8:e22860. doi: 10.4161/psb.22860
Keywords: chlorophyll degradation, cotyledon development, NYC1, VIPP1, chloroplast membrane integrity, oxidative damage
Citation: Zhang L, Kusaba M, Tanaka A and Sakamoto W (2016) Protection of Chloroplast Membranes by VIPP1 Rescues Aberrant Seedling Development in Arabidopsis nyc1 Mutant. Front. Plant Sci. 7:533. doi: 10.3389/fpls.2016.00533
Received: 04 February 2016; Accepted: 04 April 2016;
Published: 28 April 2016.
Edited by:
Jirong Huang, Shanghai Institutes for Biological Sciences – Chinese Academy of Sciences, ChinaReviewed by:
Koichi Kobayashi, University of Tokyo, JapanLianwei Peng, Institute of Botany – Chinese Academy of Sciences, China
Copyright © 2016 Zhang, Kusaba, Tanaka and Sakamoto. This is an open-access article distributed under the terms of the Creative Commons Attribution License (CC BY). The use, distribution or reproduction in other forums is permitted, provided the original author(s) or licensor are credited and that the original publication in this journal is cited, in accordance with accepted academic practice. No use, distribution or reproduction is permitted which does not comply with these terms.
*Correspondence: Wataru Sakamoto, c2FrYUBva2F5YW1hLXUuYWMuanA=