- Chongqing Engineering Research Center for Rapeseed, College of Agronomy and Biotechnology, Southwest University, Chongqing, China
Drought and salinity are severe and wide-ranging abiotic stresses that substantially affect crop germination, development and productivity, and seed germination is the first critical step in plant growth and development. To comprehensively investigate small-RNA targets and improve our understanding of miRNA-mediated post-transcriptional regulation networks during Brassica napus seed imbibition under drought and salt stresses, we constructed three small-RNA libraries from B. napus variety ZS11 embryos exposed to salt (200 mM NaCl, denoted “S”), drought (200 g L−1 PEG-6000, denoted “D”), and distilled water (denoted “CK”) during imbibition and sequenced them using an Illumina Genome Analyzer. A total of 11,528,557, 12,080,081, and 12,315,608 raw reads were obtained from the CK, D, and S libraries, respectively. Further analysis identified 85 known miRNAs belonging to 31 miRNA families and 882 novel miRNAs among the three libraries. Comparison of the D and CK libraries revealed significant down-regulation of six miRNA families, miR156, miR169, miR860, miR399, miR171, and miR395, whereas only miR172 was significantly up-regulated. In contrast, comparison of the S library with the CK library showed significant down-regulation of only two miRNA families: miRNA393 and miRNA399. Putative targets for 336, 376, and 340 novel miRNAs were successfully predicted in the CK, D, and S libraries, respectively, and 271 miRNA families and 20 target gene families [including disease resistance protein (DIRP), drought-responsive family protein (DRRP), early responsive to dehydration stress protein (ERD), stress-responsive alpha-beta barrel domain protein (SRAP), and salt tolerance homolog2 (STH2)] were confirmed as being core miRNAs and genes involved in the seed imbibition response to salt and drought stresses. The sequencing results were partially validated by quantitative RT-PCR for both conserved and novel miRNAs as well as the predicted target genes. Our data suggest that diverse and complex miRNAs are involved in seed imbibition, indicating that miRNAs are involved in plant hormone regulation, and may play important roles during seed germination under salt- or drought-stress conditions.
Introduction
Drought and salinity are severe and wide-ranging abiotic stresses that substantially affect the germination, development, and productivity of crops. Unlike most animals, plants are sessile organisms that have evolved mechanisms to cope with a wide range of changes in the environment and climate through the regulation of gene expression (Sunkar, 2010). These mechanisms involve complicated biological processes that finely coordinate molecular signaling pathways. To prevent cellular damage and to aid in recovery, specific genes, or proteins are activated at the molecular level by various stresses (Chinnusamy et al., 2007; Shinozaki and Yamaguchi-Shinozaki, 2007). Although there are specific pathways for each stress, certain key genes, or proteins act as nodal components that are integral in stress responsive signal transduction pathways (Knight and Knight, 2001; Fujita et al., 2006). Much effort has been devoted to elucidating gene expression in plants exposed to dry and brackish conditions; however, the mechanisms underlying the regulation of gene expression under these conditions remain largely unknown.
Various types of short and long non-coding RNAs (ncRNAs) are of great interest to molecular biologists. Among ncRNA subclasses, microRNAs (miRNAs), which are endogenous, non-coding RNAs ~21 nucleotides in length that negatively regulate gene expression at the transcriptional, post-transcriptional, and translational levels in both plants and animals (Bartel, 2004; Brodersen et al., 2008; Yang X. Y. et al., 2013), are the most thoroughly characterized (Bartel, 2009). The first discovered miRNAs were lin-4 and let-7, which control developmental timing in Caenorhabditis elegans and were later identified in almost all multicellular eukaryotes (Lee et al., 1993). In plants, miRNAs are involved in controlling many biological and metabolic processes, including organ maturation (Juarez et al., 2004; Guo et al., 2005), hormone signaling (Liu et al., 2009), developmental timing (Achard et al., 2004), and responses to pathogens (Sullivan and Ganem, 2005; Navarro et al., 2006) as well as to environmental abiotic stresses such as drought (Zhao et al., 2007), salinity (Zhao et al., 2009), heavy metals (Huang et al., 2009), and cold (Zhou et al., 2008). For example, miR159 and miR160 have been shown to be involved in the regulation of seed germination through effects on the sensitivity of seeds to abscisic acid (ABA) and auxin, suggesting that these miRNAs may function in the seed germination process (Reyes and Chua, 2007; Nonogaki, 2010). Many other miRNAs have also been shown to act under conditions of environmental stress, such as miR169 with high salt (Zhao et al., 2009), miR395 with sulfate starvation (Jones-Rhoades and Bartel, 2004), and miR398 with heavy metal toxicity (Sunkar et al., 2006). To date, more than 40 miRNA families have been observed to be involved in responses to abiotic stresses in plants (Sunkar, 2010), many of which are involved in responses to salt and drought stresses (Xiong et al., 2006; Peng et al., 2014). In addition, some miRNAs have been identified in more than one plant species (Sunkar et al., 2007), suggesting that their function in response to stress might be conserved among species, whereas others, called non-conserved or novel microRNAs, are species or tissue specific.
Brassica napus L. (AACC, 2n = 38), commonly known as rapeseed, is an amphidiploid species that originated from interspecies crosses between Brassica rapa (AA, 2n = 20) and Brassica oleracea (CC, 2n = 18). B. napus is the third most economically important member of the genus Brassica, provides many useful products, and is grown worldwide (Dalton-Morgan et al., 2014). Similar to all crops, exposure to soil salinity and drought stresses at specific developmental stages in Brassica leads to compromised growth, development, and seed production. Although miRNAs and their targets have been widely studied in model plants, there is only limited knowledge to date on the small-RNA population of rapeseed. In the first report of miRNAs in Brassica, Xie et al. (2007) identified 21 miRNAs in B. napus using computational methods (Xie et al., 2007). Pant et al. (2009) performed the first deep sequencing of small-RNA libraries to identify phosphate deprivation-responsive miRNAs in B. rapa and Arabidopsis (Pant et al., 2009). A study of the global miRNA response to phosphate deficiency and cadmium stress in B. napus was conducted by Huang et al. (2010), validating BnAPS3, BnAPS4, and BnSultr2:1 as targets of miR395. Subsequently, both conserved and Brassica-specific miRNAs were reported in studies of B. rapa (Kim et al., 2012; Wang F. D. et al., 2012; Yu et al., 2012), B. napus (Korbes et al., 2012; Wang J. Y. et al., 2012; Xu et al., 2012; Zhao et al., 2012; Zhou et al., 2012), B. oleracea (Wang J. Y. et al., 2012), and Brassica juncea (Yang J. H. et al., 2013).
Seed germination is the first critical step in plant growth and development. Plant development is determined by a multitude of factors that include genetic makeup and both biological and non-biological challenges. As abiotic stresses, such as drought, salt, heat, cold, and heavy metals, are major factors affecting seed germination, it is important to study the regulatory molecules and associated gene networks of seed germination under drought and salt stresses to improve crop yields via biotechnology, particularly with respect to the possible small RNA-mediated regulation of seed germination under such stress (Martin et al., 2010). miRNA expression during seed germination has been characterized in maize (Wang et al., 2011) and Arabidopsis thaliana (Jung and Kang, 2007; Kim et al., 2010) by analyzing conserved miRNAs derived from miRBase (Griffiths-Jones et al., 2008) through high-throughput sequencing. Since the initial report on rapeseed miRNAs, many conserved and rapeseed-specific miRNAs in different tissues have been identified as being involved in different biological processes (Buhtz et al., 2008; Huang et al., 2010; Korbes et al., 2012; Xu et al., 2012; Zhao et al., 2012; Zhou et al., 2012). However, the roles of miRNAs during B. napus seed germination under drought and salt stresses remain largely unknown. Potential information about miRNA mediated regulation under salt and drought stresses in rapeseed which may help to explore genomics behind stress mediated response.
To investigate small-RNA targets comprehensively and to further understand the miRNA-mediated post-transcriptional regulation network during rapeseed germination under drought and salt stresses, we constructed three small-RNA libraries from embryos of B. napus variety ZS11 seeds undergoing 60 h of imbibition under salt (S) or drought (D) stress compared to distilled water (CK) and then performed miRNA sequencing. The miRNAs and targets identified in this study may facilitate an understanding of the molecular mechanisms of stress signaling.
Materials and Methods
Plant Material, RNA Isolation, and Small-RNA High-Throughput Sequencing
Healthy B. napus ZS11 seeds were surface sterilized and soaked using filter paper as the underlay in distilled water, 200 mM NaCl, or 200 g L−1 PEG-6000 solution, marked CK, S, and D, respectively; three replicates were performed. The seeds were incubated at 22°C in darkness for 60 h until the hypocotyl emerged but the length was < 2 mm. The embryo and hypocotyl together were frozen in liquid nitrogen immediately after collection and then stored at −80°C. Total RNA was isolated using TRIzol H (Invitrogen, USA) according to the manufacturer's instructions. The quality of the RNA samples was evaluated as described previously (Han et al., 2014). Three RNA samples were sent to BGI (Shenzhen, China) for sRNA library construction and Solexa sequencing using the Illumina HiSeq 2000 platform according to the manufacturer's protocols (Liang et al., 2010).
Small-RNA Data Analysis
Small-RNA reads of each sample were filtered with the SOAPnuke software (http://soap.genomics.org.cn/) (Li et al., 2009), developed by the Beijing Genome Institute (BGI), to remove low-quality reads, reads smaller than 18 nt, adaptor sequences, and contamination formed by adaptor–adaptor ligation according to the software's default settings (http://soap.genomics.org.cn). The Rfam database (http://www.sanger.ac.uk/software/Rfam) (Griffiths-Jones et al., 2005) and the GenBank noncoding RNA database (http://www.ncbi.nlm.nih.gov/) were used to annotate these perfectly matched sequences, which might include noncoding RNAs (e.g., tRNA, rRNA, snRNA, and snoRNA) or mRNA degradation fragments. Subsequently, small RNAs were aligned to miRNA precursors of rapeseed in miRBase 21.0 (Kozomara and Griffiths-Jones, 2014) to obtain known miRNAs with the following two criteria: (1) the small RNA tags align to the miRNA precursors in miRBase with no mismatches; (2) after fulfilling the first criterion, the tags align to mature miRNAs in miRBase with at least 16 nt of overlap, allowing for offsets. Those miRNAs that satisfied both the above criteria were counted to determine the expression of known miRNAs. In addition, sequencing data of three samples were uploaded to NIH Short Read Archive with accession number SRP073343.
Prediction of Novel miRNAs
To identify novel miRNAs in rapeseed, small-RNA sequences were aligned to the B. napus genome sequence to obtain precursor sequences. Novel miRNA candidates were identified with the Mireap program developed by the BGI. The sequences were regarded as novel miRNA candidates only if their structures met the previously reported criteria (Allen et al., 2005; Wang et al., 2011). Briefly, the secondary structures of filtered pre-miRNA sequences were checked using Mfold (Wiese and Hendriks, 2006). In each case, only the structure exhibiting the minimum free energy was selected for manual inspection.
Target Gene Prediction
The psRobot software (Wu et al., 2012) was used to predict the potential targets of newly identified miRNAs responsive to salt and drought stresses. All predicted target genes were assessed using the scoring system and according to previously described criteria (Srivastava et al., 2014). Genes with a score < 2.5 were considered miRNA targets.
Expression Analysis of Rapeseed miRNAs Using qRT-PCR
Samples of embryos were collected from the CK, D, and S treatments via the same method used for miRNA library construction. Total RNA from each sample was extracted as described above. miRNA abundance was checked by qPCR according to previous reports (Shi and Chiang, 2005). The addition of poly(A) tails to sRNAs by poly(A) polymerase and cDNA synthesis was carried out using miRcute miRNA First-Strand cDNA Synthesis Kit (TIANGEN, Beijing, China), and qPCR was performed with miRcute miRNA qPCR Detection Kit according to the manufacturer's instructions. qPCR was performed using a CFX96 Real-time System (BIO-RAD, USA) with SYBR® Premix (TIANGEN, Beijing, China). The miRNA-specific forward primer for each miRNA was designed based on the entire miRNA sequence with the Tm adjusted to 60°C (Table S1). The universal reverse primer was designed based on the adapter sequence, which was provided with the miRNA cDNA synthesis kit (TIANGEN, Beijing, China). The B. napus U6 gene was used as the internal control to normalize the qPCR data, as previously described (Huang et al., 2010). The PCR conditions were as follows: 95°C for 2 min, followed by 40 cycles of 95°C for 20 s and 60°C for 30 s. A final ramping stage of 65–95°C was performed to confirm the absence of multiple products and primer dimers. The fold-changes in miRNA expression level were calculated using the comparative Ct (2−ΔΔCt) method and normalized against the expression level in the CK sample. Triplicate biological and technological replicates were performed for the three samples.
Validation of Predicted miRNA Target Gene Expression Profiles by Quantitative RT-PCR
Predicted target genes were obtained from the B. napus Sequence database (http://www.genoscope.cns.fr/brassicanapus/). The software Primer Premier 5.0 (PREMIER Biosoft Int., Palo Alto, CA, USA) was used to design specific primers for quantitative RT-PCR (Table S2), and qPCR was performed using a CFX96 Real-time System (BIO-RAD, USA) with SYBR® Premix (TIANGEN, Beijing, China). Briefly, each 20 μL PCR reaction contained ~100 ng cDNA, 8 μL 2.5 × RealMasterMix/20 × SYBR solution, and 200 nM each primer. The PCR conditions were as follows: 94°C for 2 min, followed by 40 cycles of 94°C for 15 s and 60 °C for 30 s. A final ramping stage of 65–95°C was performed to confirm the absence of multiple products and primer dimers. Actin was used for each sample as an endogenous control. All samples were subjected to at least three technical replicates. Data were analyzed using the Ct (2−ΔΔCt) method described above. Moreover, the software SPSS19.0 (SPSS Inc., Chicago, IL, USA) was used for statistical analysis in this study.
Results
Sequence Analysis of Short RNAs
To identify and characterize conserved and novel miRNAs involved in B. napus seed germination in response to salt and drought stresses, we constructed three small-RNA libraries and obtained sequence reads by Illumina high-throughput sequencing. A total of 11,528,557, 12,080,081, and 12,315,608 raw reads were obtained from the control, drought, and salt stress treatments, respectively. After the removal of low-quality reads, 3′ adapters and 5′ adapters, reads shorter than 18 nt, and other contaminating sequences, 11,317,915 (98.68%), 11,917,020 (99.17%), and 12,087,933 (98.66%) clean reads representing 3,754,707, 4,246,335, and 3,658,598 unique sequences were obtained, respectively (Table S3). Additionally, we annotated all 18–28 nt reads from the CK, D, and S libraries and obtained 4,534,861, 5,095,273, and 4,454,112 unique reads, respectively (Table 1). Approximately 2.74, 1.59, and 2.24% of the unique reads matched noncoding RNAs (rRNAs, tRNAs, snRNAs, snoRNAs), accounting for 11.06, 10.84, and 16.05% of the total reads in the CK, D, and S libraries, respectively (Table 1). Subsequently, ~15.38, 14.99, and 15.54% of the reads in CK, D, and S libraries, respectively, were mapped to coding sequences, which may constitute RNA degradation products, whereas a very small percentage of reads were mapped to intron sequences, which may be able to produce pre-miRNAs.
The distribution of various sizes of the small RNAs was not homogeneous. The most abundant were small RNAs 20–24 nt in length (Figure 1), among which sequences 24 nt long were in the greatest abundance, accounting for 39.42, 47.82, and 38.69% of the sequences in the control, drought, and salt libraries, respectively. These results are similar to those in other plant species, including A. thaliana (Rajagopalan et al., 2006), Arachis hypogaea (Zhao et al., 2010), Oryza sativa (Morin et al., 2008), Populus spp. (Lu et al., 2005), and Zea mays (Wang et al., 2011). The second most abundant sequences were 23 nt in length, accounting for 13.81% of the sequences in the drought stress library, whereas sequences with a length of 20 nt were the second most abundant in the control and salt stress libraries, accounting for 19.50 and 18.16%, respectively.
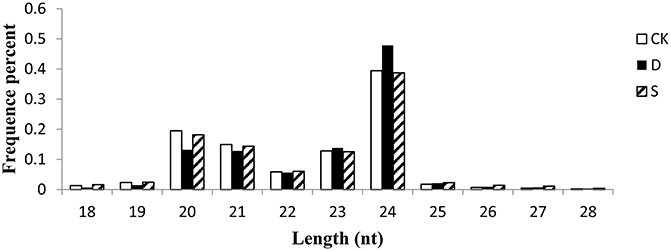
Figure 1. Size distribution of small RNAs in CK, D, and S libraries from rapeseed. CK, control; D, drought stress; S, salt stress.
Identification of Conserved miRNAs in the Three Libraries
Conserved miRNA families found in many species are responsive to many changes in the plant life cycle. To identify such conserved miRNAs in the three libraries, unique reads (minus reads mapping to snRNAs, snoRNAs, tRNAs, intron sequences, and coding sequences) from the libraries were subjected to a homolog search against miRBase21.
In the CK, D, and S libraries, 85, 81, and 81 known miRNAs, respectively, were found, belonging to 29, 29, and 28 different miRNA families, respectively, with an average of 3 miRNA members per family among the three libraries (Table S4). The miRNA members of known families were highly divergent. Briefly, the miR169 family was the most abundant, with 14 members distinguished by internal nucleotide differences in the CK, S, and D small-RNA libraries, and 6–7 members of the miR156, miR166, miR171, and miR395 families were also found. Of the remaining miRNA families, 7 families had 2–4 members and 15 families had a single member in at least one library (Figure 2).
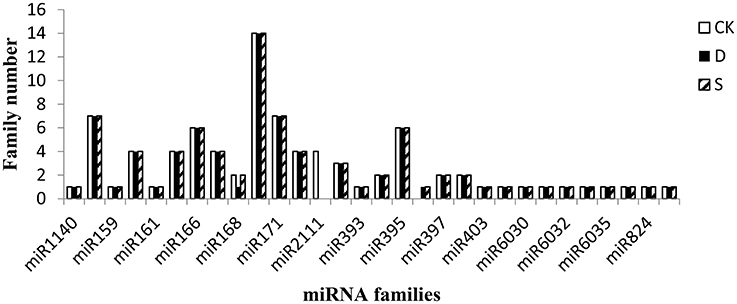
Figure 2. Number of family members per miRNA family detected in the three libraries. Candidate miRNA families were considered together and grouped by their miRBase numerical identifiers. CK, control; D, drought stress; S, salt stress.
The relative abundance of miRNAs can be estimated by the number of reads revealed by high-throughput sequencing. Intriguingly, the rapeseed miRNA abundance exhibited substantial variation. For example, miR156, with 6,955,462, 4,784,853, and 6,920,959 redundancies in the CK, D, and S libraries, respectively, was the miRNA most frequently sequenced in the three libraries; in contrast, many miRNAs (e.g., miR6035, miR393, miR6034, miR2111, miR6036, and miR396) were sequenced <20 times (Figure 3, Table 2). Our sequence analysis indicated that the abundance of certain members of miRNA families can vary dramatically among the three libraries, suggesting functional variation within each family. As an example, the abundance of the miR168 family in the CK library varied from 1 read (miR168b) to 12,878 reads (miR168a) based on deep sequencing. Similar instances were found in other miRNA families, such as the miR156 (62,938–1,698,631 reads), miR167 (2785–240,853 reads), miR169 (8–2565 reads), and miR171 (6–592 reads) families. Such variation was similar in the other two libraries (Table S5), suggesting that distinct members in the same miRNA family have dramatically different levels of expression, likely due to tissue-specific, developmental stage-specific, or environment-specific expression.
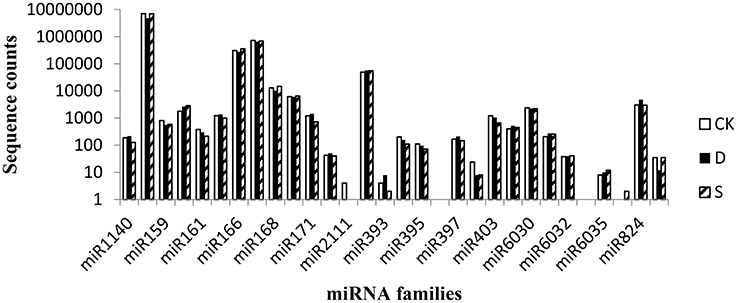
Figure 3. Abundance of conserved miRNAs in the CK, D, and S libraries in rapeseed. CK, control; D, drought stress; S, salt stress.
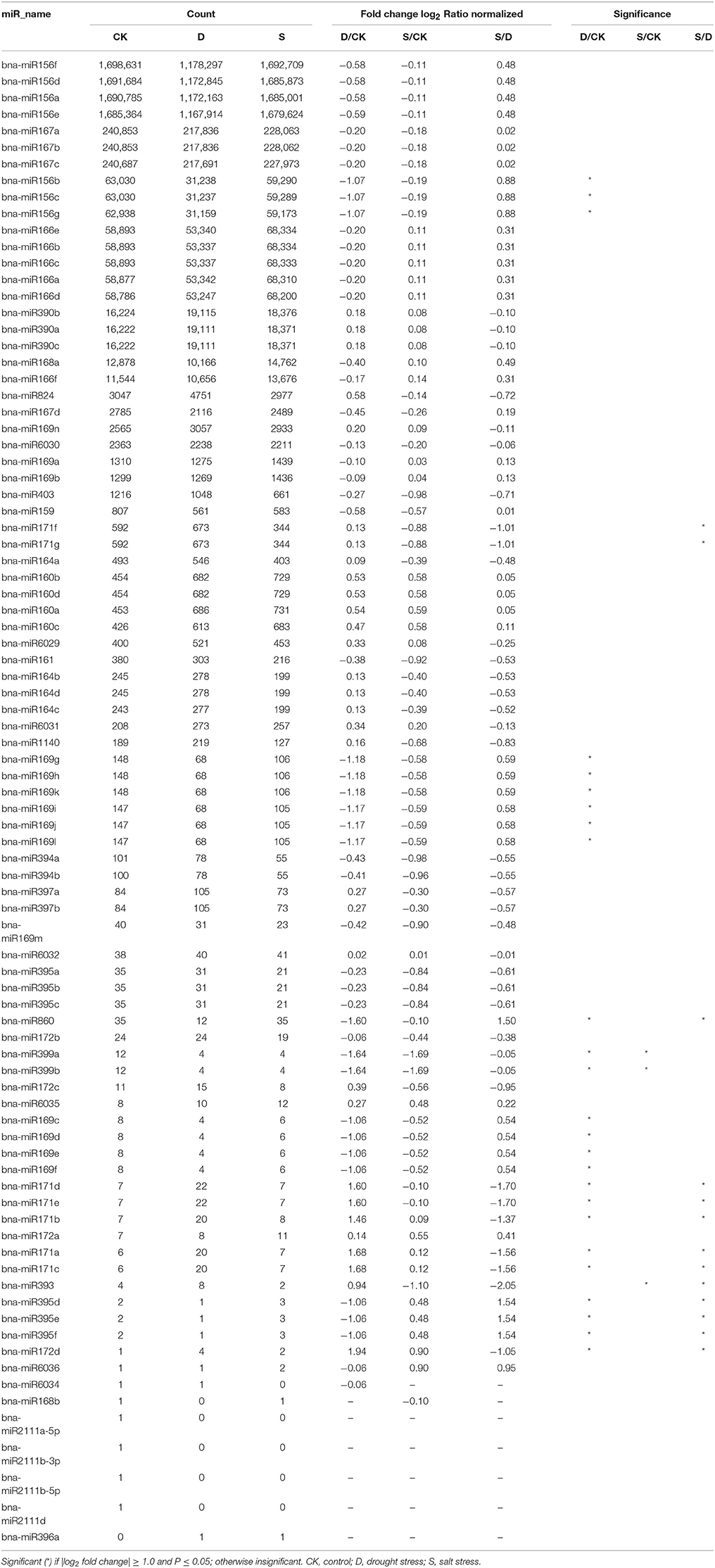
Table 2. The miRNA abundance of conserved miRNA families among control (CK), salt (S), and drought (D) treatments.
Based on the criterion, |log2 fold change| ≥ 1.0 and P ≤ 0.05, the expression of miR166, miR167, and miR390, which were among the top 10 most expressed miRNAs, did not show significant differential expression. Indeed, only six miRNAs (miR156, miR169, miR171, miR395, miR399, and miR860), which were sequenced <50 times, were significantly differentially expressed between the drought stress and control treatments. Compared with salt stress, four miRNAs (miR171, miR393, miR395, and miR860) were markedly differentially expressed in the D library, and miR393 and miR399 were significantly differentially expressed in the CK library (Table 2). The miRNA family groups with similar expression patterns were then classified using Heatmap clustering. For instance, miR161, miR394, miR395, and miR403 were down-regulated by salt stress, whereas miR171, miR393, and miR824 were up-regulated by drought stress (Figure S1).
To explore the roles of these conserved miRNAs in evolution, further analyses focusing on broader comparisons against known conserved miRNAs in Viridiplantae, including Brassicaceae, Malvaceae, Rutaceae, Solanaceae, Salicaceae, Rhizophoraceae, and Lamiales, and even monocotyledons, Embryophyta, Coniferophyta, and Chlorophyta, were performed. Based on BLAST analysis, some miRNA families, such as miR156/157, miR159, miR160, miR165/166, miR171, and miR396, were found to be highly conserved (Table S6). For example, miR156/157 is highly expressed during the seedling stage in different species.
Identification of Novel miRNAs in Rapeseed
Each species possesses species-specific miRNAs (Zhao et al., 2010; Chi et al., 2011; Li et al., 2011; Schreiber et al., 2011; Song et al., 2011; Gao et al., 2012; Guo et al., 2012; Lertpanyasampatha et al., 2012; Lv et al., 2012), and the characteristic hairpin structures of miRNA precursors, the Dicer cleavage site, and the minimum free energy are important factors for predicting novel miRNAs. Many papers have reported the prediction of novel miRNAs using Mireap according to strict criteria (Li et al., 2013), and based on these criteria, 882 novel miRNAs were predicted from the three libraries (Table S7). The lengths of the novel miRNAs were 20–23 nt, with 23 nt being the most common length in the three libraries (Table S8). More than half of the novel predicted miRNAs begin with a 5′ uridine, and these miRNAs accounted for more than eighty percent of small RNAs 20 and 21 nt in length (Table S7) (Bonnet et al., 2004; Zhang et al., 2006). According to Mfold3.2, the average negative minimal folding free energy of these miRNA precursors is approximately −245 to −18 kcal mol−l, which was similar to miRNA precursors in other species (Wang et al., 2011). Compared with known miRNA families, the abundance of novel miRNAs was very low, and the majority of these miRNAs were present <100 times. Nonetheless, these miRNAs comprised 87.79% (367/418), 88.83% (382/430), and 88.92% (369/415) of the CK, D, and S libraries, respectively. The most abundant novel miRNA was novel_mir_34, which was sequenced 58,924, 70,723, and 60,320 times in the CK, D, and S libraries, respectively. Unlike conserved miRNAs, different types of novel miRNAs were expressed in the three independent libraries. Of the novel miRNAs identified, 199, 216, and 204 were specific to the CK, D, and S libraries, respectively, and only 118 were shared among the libraries. In the CK and D libraries, 170 novel miRNAs were shared, whereas 167 and 162 miRNAs were shared between the CK and S and D and S libraries, respectively (Figure 4).
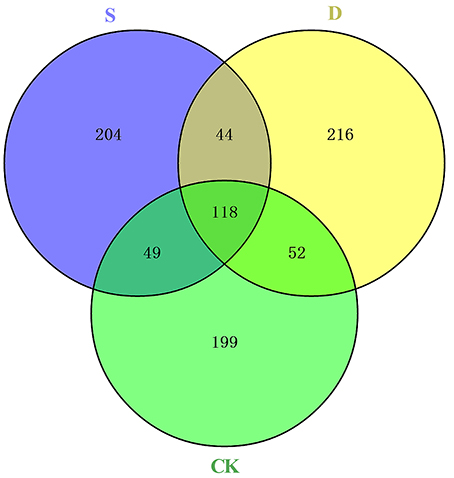
Figure 4. Diagram of novel miRNAs identified in three libraries. CK, control; D, drought stress; S, salt stress.
Using the criteria of |log2 fold change| ≥ 1.0 and P ≤ 0.05, 268, 242, and 273 miRNAs exhibited significantly different expression between the CK and D, CK and S, and S and D libraries, respectively. Two novel miRNAs—bna-miR25 and bna-miR385—were significantly up-regulated under drought stress and down-regulated under salt stress, respectively (Figure 5).
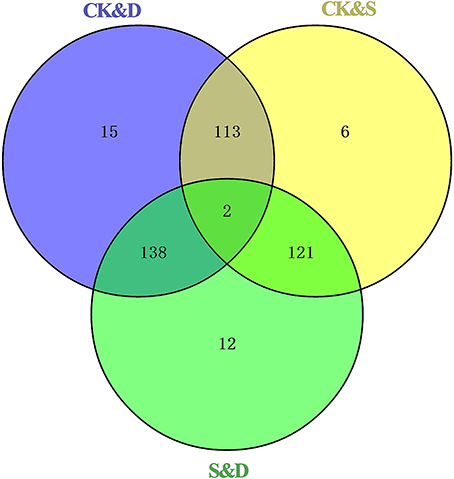
Figure 5. Diagram of significantly different expression novel miRNAs between CK&D, CK&S, and S&D. CK, control; D, drought stress; S, salt stress.
Target Prediction of Rapeseed miRNAs
As the identification of mRNA targets is necessary for an understanding of the biological roles of B. napus miRNAs (Korbes et al., 2012), psRobot software was used to predict the targets of conserved and novel miRNAs. The cut-off threshold was set at 2.5, and 610 and 4133 putative targets were found for the conserved and 656 novel miRNA families, respectively (Tables S9, S10). However, no target genes were found for 226 of the novel miRNA families (Table S11). Various transcription factors, including SBP, MYB, ARF, NAM, NAC, CBF, TCP, NF-YA, and GRF, were among the majority of the conserved miRNA targets identified in different species, including Arabidopsis (Adai et al., 2005), grape (Carra et al., 2009), mustard (Xie et al., 2007), poplar (Lu et al., 2008), rice (Sunkar et al., 2005), soybean (Subramanian et al., 2008), and wheat (Jin et al., 2008). F-box protein, ATP sulfurylase, CCHC-type zinc finger protein, NAD(P)-binding protein, and Poly(ADP-ribose) polymerase, proteins involved in the regulation of metabolic processes, were also identified as conserved miRNA targets. In our datasets, miRNA156 showed the highest abundance, followed by miRNA167, miRNA166, and miRNA390, during the very early stage of seed germination under salt and drought stresses. miRNA156 is involved in floral development and phase change through down-regulation of the SQUAMOSA promoter binding protein-like (SPL) family of transcription factor genes, which are up-regulated in the juvenile phase and down-regulated in the adult phase (Wu et al., 2009; Zhang et al., 2009; Yang et al., 2011). Many studies have emphasized that the transcription factor HD-ZIP participates in plant leaf morphogenesis, and ATHB15, a member of the HD-ZIP family targeted by miRNA166, may play a role in plant vascular development because it is predominantly expressed in vascular tissues (Ohashi-Ito and Fukuda, 2003). The miRNA156 and miRNA166 families are very important in seed germination not only due to their high abundance but also because of the large size of those miRNA families compared to other miRNA families. Studies have demonstrated cross-talk regulation between ABA and auxin during seed germination (Weitbrecht et al., 2011), and miRNA167 and miRNA160 have been shown to be involved in inhibiting auxin response factors ARF6 and ARF8 (Wu et al., 2006) and ARF10, ARF16, and ARF17 (Mallory et al., 2005; Wang et al., 2005), respectively. Additionally, miRNA159 has been shown to be involved in the regulation of seed dormancy and germination by inhibiting MYB33 and MYB101, two positive regulators of ABA response during germination.
To gain further insight into the functions of novel miRNAs in rapeseed during seed germination under salt and drought stresses, putative targets of the 656 newly identified miRNAs were predicted, revealing various functions, including transcription, metabolism, transport, kinases, oxidative reduction and isomerase and helicase activities. According to miRNA regulatory network analysis using Cytoscape (http://www.cytoscape.org/), 271 miRNA families and 20 target gene families in rapeseed were confirmed to be core miRNAs and genes involved in response to salt and drought stresses. Direct stress-responsive gene families, such as disease resistance protein (DIRP), drought-responsive family protein (DRRP), early responsive to dehydration stress protein (ERD), stress-responsive alpha-beta barrel domain protein (SRAP), and salt tolerance homolog2 (STH2), were also predicted in our analysis. Overall, miRNA regulatory mechanisms responsive to salt and drought stresses are highly sophisticated in rapeseed, and these miRNAs and their targets related to stress response add to our knowledge of stress resistance and the stress tolerance mechanism in this plant.
Expression Profiles of miRNAs and Their Targets in Response to Salt and Drought Stresses
To confirm the expression patterns of miRNAs in response to salt and drought stresses, quantitative RT-PCR (qRT-PCR) was performed for 6 conserved miRNAs (miR1140, miR403, miR824, miR164a, miR166e, and miR169n) and 9 novel ones (bna-miR17, bna-miR37, bna-miR77, bna-miR122, bna-miR144, bna-miR290, bna-miR485, bna-miR516, and bna-miR700). As expected, the qRT-PCR results were consistent with the expression profiles obtained by RNA-seq (Figure 6). qRT-PCR was also performed on target genes of 17 randomly selected miRNAs, of which 8 are from known miRNA families (miR156b, miR403, miR160a, miR164a, miR166e miR171f, miR6030, and miR169n) and 9 from novel miRNA families (bna-miR17, bna-miR37, bna-miR77, bna-miR122, bna-miR144, bna-miR290, bna-miR485, bna-miR700, and bna-miR814). However, as illustrated in Figure 7, the expression profiles of 19 target genes showed an inverse relationship with the expression profiles of their corresponding miRNAs, suggesting that the miRNAs indeed target those genes.
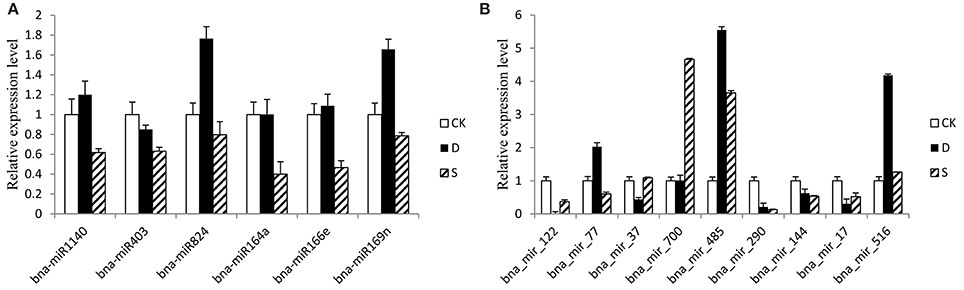
Figure 6. Quantitative RT-PCR validation of mature miRNAs. The expression profiles of mature miRNAs were consistent with the results obtained by small-RNA deep sequencing. The expression values presented are the means of three technical replicates. U6 was used for each sample as an endogenous control. (A,B) Represent the expression pattern of mature conserved and novel miRNAs, respectively.
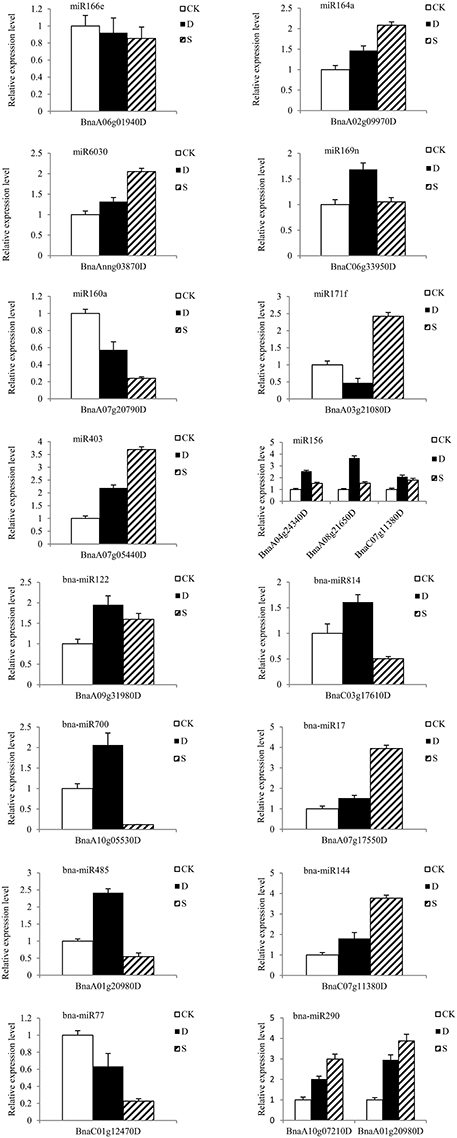
Figure 7. Quantitative RT-PCR analyses of relative expression levels of various predicted target genes. The rapeseed housekeeping gene actin7 was used as the internal control. The values presented are the means of three technical replicates.
Discussion
Seed germination is a critical step in plant growth and development. However, abiotic stresses such as drought and salt are major factors affecting the seed germination rate. Thus, understanding this process as a whole—from early seed germination to the establishment of the seedling—can help us to engineer and select more robust crop species and increase crop yield and quality. In this study, three sRNA libraries from imbibed seed embryos under control, salt, and drought treatments were constructed, and miRNAs were sequenced using high-throughput sequencing.
Many miRNAs Participate in Rapeseed Germination
High-throughput sequencing has been applied to study miRNAs at the whole-genome level in many plant species. However, the means by which miRNA families function in plant development, stress responses, and other processes in B. napus, one of the most important oil crops in the world, are not very clear. In our study, the three libraries contained 35,322,868 clean reads and 14,084,059 unique small-RNA reads. Two of the three libraries shared from 65.27 to 67.88% of the total clean reads; however, they shared only 14.34–14.68% of the unique small-RNA reads, a phenomenon also observed in maize (Li et al., 2013). These findings suggest that the shared reads have high expression levels and the library-specific small RNAs low expression levels. The low expression levels of these specific unique small RNAs may indicate that they function in specific and unique regulation pathways.
Successful seed germination and the establishment of a normal seedling are essential. Although the evidence is fragmentary, there are several sources that emphasize miRNAs as a very important component of seed germination (Li et al., 2013; Zhang et al., 2013). For instance, Weitbrecht et al. (2011) highlighted that ABA and gibberellin acid (GA) play vital roles in seed germination. Briefly, ABA levels generally decrease during imbibition, whereas GA levels increase during the transition to germination. In Arabidopsis, two positive ABA regulators, MYB33 and MYB101, which are negatively regulated by miR159, play key roles in seed germination. In the present study, BnaA04g18810D and BnaC04g43020D, homologs of AtMYB101, were predicted to be target genes of miR159. In the absence of miR159, MYB33, and MYB101 up-regulated genes are deregulated during seed germination (Alonso-Peral et al., 2010); thus, MYB-like genes participate in GA-induced pathways via miR159-mediated regulation during seed germination. AUXIN RESPONSIVE FACTORs (ARFs), which are pivotal for translating auxin signals into transcriptional responses, comprise a class of targets for the miRNA160/167 families. Indeed, studies have shown that the negative regulation of ARF10 by miR160 plays an important role in seed germination (Liu et al., 2007), and miR167 regulates ARF6 and ARF8 in adventitious rooting in Arabidopsis (Gutierrez et al., 2009) and in cultured rice cells (Yang et al., 2006). The crosstalk between ABA and auxin in imbibed mature seeds has been discussed by Liu et al. (2007), and studies have indicated that ABA sensitivity would decrease in mature seeds via the down-regulation of auxin signal transduction. Regardless, the mechanisms of ABA-auxin crosstalk during seed germination remain enigmatic. Laccases, which are involved in the lignification and thickening of the cell wall during secondary cell growth (Constabel et al., 2000), were found to be down-regulated by miR397, suggesting that miR397 plays a vital role in decreasing the thickness of the cell wall during the transition from a dormant embryo to a germinated embryo by causing laccase mRNA cleavage. Other miRNA families have been reported to be involved in seed germination in other species, such as miR166 (HD-ZIP), miR164 (NAC1), and miR396 (GRF) (Tahir et al., 2006; Li et al., 2013; Zhang et al., 2013). These findings indicate the existence of complex mechanisms of gene regulation involving miRNAs during seed germination.
Potential Roles of miRNAs in Seed Germination Response to Salt and Drought Stresses
Many conserved and novel miRNAs are aberrantly expressed in drought stress in different crops, such as cowpea (Barrera-Figueroa et al., 2011), tobacco (Frazier et al., 2011), wheat (Kantar et al., 2011), soybean (Kulcheski et al., 2011), and bean (Arenas-Huertero et al., 2009). miR169, one of the most important miRNAs involved in drought stress, was down-regulated in our study. Similarly, the expression of miR169a and miR169c in Arabidopsis decreased under drought stress, and the miR169-target gene NFYA5, a member of the Arabidopsis family of CCAAT-box nuclear transcription factors functioning in drought resistance, was strongly induced (Li et al., 2008). However, miR169 was found to be up-regulated in rice and tomato under drought conditions (Zhao et al., 2007; Zhang et al., 2011). The three nuclear factor Y subunit genes (SlNF-YA1/2/3) targeted by miR169 were also down-regulated. Moreover, overexpressing miR169c in tomato plants resulted in better tolerance to drought. In Arabidopsis, miR157, miR167, miR168, miR171, miR408, miR393, and miR396 were reported to be up-regulated in response to drought stress (Liu et al., 2008). In our study, miR156b/c/g, miR171a/d/e, and miR172d were significantly up-regulated, whereas miR860, miR399a/b, and miR395d/e/f were down-regulated.
Although plants invoke similar cellular and metabolic responses to salt and drought stresses (Munns, 2005), the genes and pathways involved vary (Bartels and Sunkar, 2005; Golldack et al., 2011). In our study, miR393 and miR399, both are involved in phosphate homeostasis, were significantly down-regulated. However, miR399 was up-regulated under low phosphate in Arabidopsis (Fujii et al., 2005) and rice (Wang et al., 2009). When plants suffer from phosphate starvation, mature miR399 activated by the PHR1 transcription factor cleaves target PHO2 mRNA (Pant et al., 2008). In addition, mRNAs of TIR1/AFB2 auxin receptors (TAARs), regulators of auxin signaling homeostasis, are targeted and cleaved by up-regulated miR393 during salt and drought stresses in O. sativa, A. thaliana, M. truncatula, and Phaseolus vulgaris (Dharmasiri et al., 2005; Chen et al., 2011; Si-Ammour et al., 2011; Windels and Vazquez, 2011). miR393 also functions in the root development response to nitrate treatment by cleaving TAAR transcripts, which are positive regulators of auxin signaling (Vidal et al., 2010). It is easy to understand the crucial rule of miR393 in response to different stresses because auxin is involved in almost every aspect of plant life (Windels and Vazquez, 2011). These miRNAs may increase drought or salt tolerance through unknown mechanisms associated with crosstalk regulation in plants, and future studies in rapeseed involving miRNA over-expression for functional annotation are warranted.
Author Contributions
LL conceived and designed the experiments. HJ and JW performed the experiments. HJ, JW, and TW analyzed the data. LW, JL, and LL contributed reagents/materials/analysis tools. HJ wrote the paper.
Funding
This study was supported by National Natural Science Foundation of China (31371655), 973 Program (2015CB150201), and Chongqing graduate student research innovation project (CYB2015063).
Conflict of Interest Statement
The authors declare that the research was conducted in the absence of any commercial or financial relationships that could be construed as a potential conflict of interest.
The reviewer AVCR and handling Editor declared their shared affiliation, and the handling Editor states that the process nevertheless met the standards of a fair and objective review.
Supplementary Material
The Supplementary Material for this article can be found online at: http://journal.frontiersin.org/article/10.3389/fpls.2016.00658
Table S1. The primers used for mature miRNAs qRT-PCR.
Table S2. The primers used for predicted miRNAs targets qRT-PCR.
Table S3. Raw data analysis for the three libraries.
Table S4. The number of conserved miRNA family members.
Table S5. The miRNA abundance of conserved miRNA families among the control (CK), salt (S), and drought (D) treatments.
Table S6. Conserved miRNAs from B. napus and their homologs in selected other species. +, detected in this species; −, not detected in this species.
Table S7. The miRNA abundance of novel miRNA families among the control (CK), salt (S), and drought (D) treatments.
Table S8. Size distribution of novel miRNAs in the rapeseed CK, D, and S libraries.
Table S9. Predicted targets of conserved miRNAs in the CK, D, and S libraries.
Table S10. Predicted targets of novel miRNAs in the CK, D, and S libraries.
Table S11. Novel miRNAs with no targets predicted in the CK, D, and S libraries.
Figure S1. Heat map of conserved miRNA read abundance in the B. napus control, salt, and drought libraries.
References
Achard, P., Herr, A., Baulcombe, D. C., and Harberd, N. P. (2004). Modulation of floral development by a gibberellin-regulated microRNA. Development 131, 3357–3365. doi: 10.1242/dev.01206
Adai, A., Johnson, C., Mlotshwa, S., Archer-Evans, S., Manocha, V., Vance, V., et al. (2005). Computational prediction of miRNAs in Arabidopsis thaliana. Genome Res. 15, 78–91. doi: 10.1101/gr.2908205
Allen, E., Xie, Z. X., Gustafson, A. M., and Carrington, J. C. (2005). microRNA-directed phasing during trans-acting siRNA biogenesis in plants. Cell 121, 207–221. doi: 10.1016/j.cell.2005.04.004
Alonso-Peral, M. M., Li, J., Li, Y., Allen, R. S., Schnippenkoetter, W., Ohms, S., et al. (2010). The microRNA159-regulated GAMYB-like genes inhibit growth and promote programmed cell death in Arabidopsis. Plant Physiol. 154, 757–771. doi: 10.1104/pp.110.160630
Arenas-Huertero, C., Perez, B., Rabanal, F., Blanco-Melo, D., De la Rosa, C., Estrada-Navarrete, G., et al. (2009). Conserved and novel miRNAs in the legume Phaseolus vulgaris in response to stress. Plant Mol. Biol. 70, 385–401. doi: 10.1007/s11103-009-9480-3
Barrera-Figueroa, B. E., Gao, L., Diop, N. N., Wu, Z. G., Ehlers, J. D., Roberts, P. A., et al. (2011). Identification and comparative analysis of drought-associated microRNAs in two cowpea genotypes. BMC Plant Biol. 11:127. doi: 10.1186/1471-2229-11-127
Bartel, D. P. (2004). MicroRNAs: genomics, biogenesis, mechanism, and function. Cell 116, 281–297. doi: 10.1016/S0092-8674(04)00045-5
Bartel, D. P. (2009). MicroRNAs: target recognition and regulatory functions. Cell 136, 215–233. doi: 10.1016/j.cell.2009.01.002
Bartels, D., and Sunkar, R. (2005). Drought and salt tolerance in plants. CRC. Crit. Rev. Plant Sci. 24, 23–58. doi: 10.1080/07352680590910410
Bonnet, E., Wuyts, J., Rouze, P., and Van de Peer, Y. (2004). Evidence that microRNA precursors, unlike other non-coding RNAs, have lower folding free energies than random sequences. Bioinformatics 20, 2911–2917. doi: 10.1093/bioinformatics/bth374
Brodersen, P., Sakvarelidze-Achard, L., Bruun-Rasmussen, M., Dunoyer, P., Yamamoto, Y. Y., Sieburth, L., et al. (2008). Widespread translational inhibition by plant miRNAs and siRNAs. Science 320, 1185–1190. doi: 10.1126/science.1159151
Buhtz, A., Springer, F., Chappell, L., Baulcombe, D. C., and Kehr, J. (2008). Identification and characterization of small RNAs from the phloem of Brassica napus. Plant J. 53, 739–749. doi: 10.1111/j.1365-313X.2007.03368.x
Carra, A., Mica, E., Gambino, G., Pindo, M., Moser, C., Pe, M. E., et al. (2009). Cloning and characterization of small non-coding RNAs from grape. Plant J. 59, 750–763. doi: 10.1111/j.1365-313X.2009.03906.x
Chen, Z. H., Bao, M. L., Sun, Y. Z., Yang, Y. J., Xu, X. H., Wang, J. H., et al. (2011). Regulation of auxin response by miR393-targeted transport inhibitor response protein 1 is involved in normal development in Arabidopsis. Plant Mol. Biol. 77, 619–629. doi: 10.1007/s11103-011-9838-1
Chi, X. Y., Yang, Q. L., Chen, X. P., Wang, J. Y., Pan, L. J., Chen, M. N., et al. (2011). Identification and characterization of microRNAs from peanut (Arachis hypogaea L.) by high-throughput sequencing. PLoS ONE 6:e27530. doi: 10.1371/journal.pone.0027530
Chinnusamy, V., Zhu, J., and Zhu, J. K. (2007). Cold stress regulation of gene expression in plants. Trends Plant Sci. 12, 444–451. doi: 10.1016/j.tplants.2007.07.002
Constabel, C. P., Yip, L., Patton, J. J., and Christopher, M. E. (2000). Polyphenol oxidase from hybrid poplar. Cloning and expression in response to wounding and herbivory. Plant Physiol. 124, 285–295. doi: 10.1104/pp.124.1.285
Dalton-Morgan, J., Hayward, A., Alamery, S., Tollenaere, R., Mason, A. S., Campbell, E., et al. (2014). A high-throughput SNP array in the amphidiploid species Brassica napus shows diversity in resistance genes. Funct. Integr. Genomics 14, 643–655. doi: 10.1007/s10142-014-0391-2
Dharmasiri, N., Dharmasiri, S., and Estelle, M. (2005). The F-box protein TIR1 is an auxin receptor. Nature 435, 441–445. doi: 10.1038/nature03543
Frazier, T. P., Sun, G. L., Burklew, C. E., and Zhang, B. H. (2011). Salt and drought stresses induce the aberrant expression of microRNA genes in tobacco. Mol. Biotechnol. 49, 159–165. doi: 10.1007/s12033-011-9387-5
Fujii, H., Chiou, T. J., Lin, S. I., Aung, K., and Zhu, J. K. (2005). A miRNA involved in phosphate-starvation response in Arabidopsis. Curr. Biol. 15, 2038–2043. doi: 10.1016/j.cub.2005.10.016
Fujita, M., Fujita, Y., Noutoshi, Y., Takahashi, F., Narusaka, Y., Yamaguchi-Shinozaki, K., et al. (2006). Crosstalk between abiotic and biotic stress responses: a current view from the points of convergence in the stress signaling networks. Curr. Opin. Plant Biol. 9, 436–442. doi: 10.1016/j.pbi.2006.05.014
Gao, Z. H., Shi, T., Luo, X. Y., Zhang, Z., Zhuang, W. B., and Wang, L. J. (2012). High-throughput sequencing of small RNAs and analysis of differentially expressed microRNAs associated with pistil development in Japanese apricot. BMC Genomics 13:371. doi: 10.1186/1471-2164-13-371
Golldack, D., Luking, I., and Yang, O. (2011). Plant tolerance to drought and salinity: stress regulating transcription factors and their functional significance in the cellular transcriptional network. Plant Cell Rep. 30, 1383–1391. doi: 10.1007/s00299-011-1068-0
Griffiths-Jones, S., Moxon, S., Marshall, M., Khanna, A., Eddy, S. R., and Bateman, A. (2005). Rfam: annotating non-coding RNAs in complete genomes. Nucleic Acids Res. 33, D121–D124. doi: 10.1093/nar/gki081
Griffiths-Jones, S., Saini, H. K., van Dongen, S., and Enright, A. J. (2008). miRBase: tools for microRNA genomics. Nucleic Acids Res. 36, D154–D158. doi: 10.1093/nar/gkm952
Guo, H. S., Xie, Q., Fei, J. F., and Chua, N. H. (2005). MicroRNA directs mRNA cleavage of the transcription factor NAC1 to downregulate auxin signals for Arabidopsis lateral root development. Plant Cell 17, 1376–1386. doi: 10.1105/tpc.105.030841
Guo, W. X., Wu, G. T., Yan, F., Lu, Y. W., Zheng, H. Y., Lin, L., et al. (2012). Identification of novel Oryza sativa miRNAs in deep sequencing-based small RNA libraries of rice infected with rice stripe virus. PLoS ONE 7:e46443. doi: 10.1371/journal.pone.0046443
Gutierrez, L., Bussell, J. D., Pacurar, D. I., Schwambach, J., Pacurar, M., and Bellini, C. (2009). Phenotypic plasticity of adventitious rooting in arabidopsis is controlled by complex regulation of AUXIN RESPONSE FACTOR transcripts and MicroRNA abundance. Plant Cell 21, 3119–3132. doi: 10.1105/tpc.108.064758
Han, R., Jian, C., Lv, J. Y., Yan, Y., Chi, Q., Li, Z. J., et al. (2014). Identification and characterization of microRNAs in the flag leaf and developing seed of wheat (Triticum aestivum L.). BMC Genomics 15:289. doi: 10.1186/1471-2164-15-289
Huang, S. Q., Peng, J., Qiu, C. X., and Yang, Z. M. (2009). Heavy metal-regulated new microRNAs from rice. J. Inorg. Biochem. 103, 282–287. doi: 10.1016/j.jinorgbio.2008.10.019
Huang, S. Q., Xiang, A. L., Che, L. L., Chen, S., Li, H., Song, J. B., et al. (2010). A set of miRNAs from Brassica napus in response to sulphate deficiency and cadmium stress. Plant Biotechnol. J. 8, 887–899. doi: 10.1111/j.1467-7652.2010.00517.x
Jin, W. B., Li, N. N., Zhang, B., Wu, F. L., Li, W. J., Guo, A. G., et al. (2008). Identification and verification of microRNA in wheat (Triticum aestivum). J. Plant Res. 121, 351–355. doi: 10.1007/s10265-007-0139-3
Jones-Rhoades, M. W., and Bartel, D. P. (2004). Computational identification of plant MicroRNAs and their targets, including a stress-induced miRNA. Mol. Cell 14, 787–799. doi: 10.1016/j.molcel.2004.05.027
Juarez, M. T., Kui, J. S., Thomas, J., Heller, B. A., and Timmermans, M. C. P. (2004). microRNA-mediated repression of rolled leaf1 specifies maize leaf polarity. Nature 428, 84–88. doi: 10.1038/nature02363
Jung, H. J., and Kang, H. (2007). Expression and functional analyses of microRNA417 in Arabidopsis thaliana under stress conditions. Plant Physiol. Biochem. 45, 805–811. doi: 10.1016/j.plaphy.2007.07.015
Kantar, M., Lucas, S. J., and Budak, H. (2011). miRNA expression patterns of Triticum dicoccoides in response to shock drought stress. Planta 233, 471–484. doi: 10.1007/s00425-010-1309-4
Kim, B., Yu, H. J., Park, S. G., Shin, J. Y., Oh, M., Kim, N., et al. (2012). Identification and profiling of novel microRNAs in the Brassica rapa genome based on small RNA deep sequencing. BMC Plant Biol. 12:218. doi: 10.1186/1471-2229-12-218
Kim, J. Y., Kwak, K. J., Jung, H. J., Lee, H. J., and Kang, H. (2010). MicroRNA402 affects seed germination of Arabidopsis thaliana under stress conditions via targeting DEMETER-LIKE Protein3 mRNA. Plant Cell Physiol. 51, 1079–1083. doi: 10.1093/pcp/pcq072
Knight, H., and Knight, M. R. (2001). Abiotic stress signalling pathways: specificity and cross-talk. Trends Plant Sci. 6, 262–267. doi: 10.1016/S1360-1385(01)01946-X
Korbes, A. P., Machado, R. D., Guzman, F., Almerao, M. P., de Oliveira, L. F. V., Loss-Morais, G., et al. (2012). Identifying conserved and novel MicroRNAs in developing seeds of Brassica napus using deep sequencing. PLoS ONE 7:e50663. doi: 10.1371/journal.pone.0050663
Kozomara, A., and Griffiths-Jones, S. (2014). miRBase: annotating high confidence microRNAs using deep sequencing data. Nucleic Acids Res. 42, D68–D73. doi: 10.1093/nar/gkt1181
Kulcheski, F. R., de Oliveira, L. F. V., Molina, L. G., Almerao, M. P., Rodrigues, F. A., Marcolino, J., et al. (2011). Identification of novel soybean microRNAs involved in abiotic and biotic stresses. BMC Genomics 12:307. doi: 10.1186/1471-2164-12-307
Lee, R. C., Feinbaum, R. L., and Ambros, V. (1993). The C. elegans heterochronic gene lin-4 encodes small RNAs with antisense complementarity to lin-14. Cell 75, 843–854. doi: 10.1016/0092-8674(93)90529-Y
Lertpanyasampatha, M., Gao, L., Kongsawadworakul, P., Viboonjun, U., Chrestin, H., Liu, R. Y., et al. (2012). Genome-wide analysis of microRNAs in rubber tree (Hevea brasiliensis L.) using high-throughput sequencing. Planta 236, 437–445. doi: 10.1007/s00425-012-1622-1
Li, D. T., Wang, L. W., Liu, X., Cui, D. Z., Chen, T. T., Zhang, H., et al. (2013). Deep sequencing of maize small RNAs reveals a diverse set of microRNA in dry and imbibed seeds. PLoS ONE 8:e55107. doi: 10.1371/journal.pone.0055107
Li, H. Y., Dong, Y. Y., Yin, H. L., Wang, N., Yang, J., Liu, X. M., et al. (2011). Characterization of the stress associated microRNAs in Glycine max by deep sequencing. BMC Plant Biol. 11:170. doi: 10.1186/1471-2229-11-170
Li, R. Q., Yu, C., Li, Y. R., Lam, T. W., Yiu, S. M., Kristiansen, K., et al. (2009). SOAP2: an improved ultrafast tool for short read alignment. Bioinformatics 25, 1966–1967. doi: 10.1093/bioinformatics/btp336
Li, W. X., Oono, Y., Zhu, J. H., He, X. J., Wu, J. M., Iida, K., et al. (2008). The Arabidopsis NFYA5 transcription factor is regulated transcriptionally and posttranscriptionally to promote drought resistance. Plant Cell 20, 2238–2251. doi: 10.1105/tpc.108.059444
Liang, C. W., Zhang, X. W., Zou, J., Xu, D., Su, F., and Ye, N. H. (2010). Identification of miRNA from Porphyra yezoensis by high-throughput sequencing and bioinformatics analysis. PLoS ONE 5:e10698. doi: 10.1371/journal.pone.0010698
Liu, H. H., Tian, X., Li, Y. J., Wu, C. A., and Zheng, C. C. (2008). Microarray-based analysis of stress-regulated microRNAs in Arabidopsis thaliana. RNA 14, 836–843. doi: 10.1261/rna.895308
Liu, P. P., Montgomery, T. A., Fahlgren, N., Kasschau, K. D., Nonogaki, H., and Carrington, J. C. (2007). Repression of AUXIN RESPONSE FACTOR10 by microRNA160 is critical for seed germination and post-germination stages. Plant J. 52, 133–146. doi: 10.1111/j.1365-313X.2007.03218.x
Liu, Q., Zhang, Y. C., Wang, C. Y., Luo, Y. C., Huang, Q. J., Chen, S. Y., et al. (2009). Expression analysis of phytohormone-regulated microRNAs in rice, implying their regulation roles in plant hormone signaling. FEBS Lett. 583, 723–728. doi: 10.1016/j.febslet.2009.01.020
Lu, S. F., Sun, Y. H., and Chiang, V. L. (2008). Stress-responsive microRNAs in Populus. Plant J. 55, 131–151. doi: 10.1111/j.1365-313X.2008.03497.x
Lu, S. F., Sun, Y. H., Shi, R., Clark, C., Li, L. G., and Chiang, V. L. (2005). Novel and mechanical stress-responsive microRNAs in Populus trichocarpa that are absent from Arabidopsis. Plant Cell 17, 2186–2203. doi: 10.1105/tpc.105.033456
Lv, S. Z., Nie, X. J., Wang, L., Du, X. H., Biradar, S. S., Jia, X. O., et al. (2012). Identification and characterization of microRNAs from Barley (Hordeum vulgare L.) by high-throughput sequencing. Int. J. Mol. Sci. 13, 2973–2984. doi: 10.3390/ijms13032973
Mallory, A. C., Bartel, D. P., and Bartel, B. (2005). MicroRNA-directed regulation of Arabidopsis AUXIN RESPONSE FACTOR17 is essential for proper development and modulates expression of early auxin response genes. Plant Cell 17, 1360–1375. doi: 10.1105/tpc.105.031716
Martin, R. C., Liu, P. P., Goloviznina, N. A., and Nonogaki, H. (2010). microRNA, seeds, and Darwin?: diverse function of miRNA in seed biology and plant responses to stress. J. Exp. Bot. 61, 2229–2234. doi: 10.1093/jxb/erq063
Morin, R. D., Aksay, G., Dolgosheina, E., Ebhardt, H. A., Magrini, V., Mardis, E. R., et al. (2008). Comparative analysis of the small RNA transcriptomes of Pinus contorta and Oryza sativa. Genome Res. 18, 571–584. doi: 10.1101/gr.6897308
Munns, R. (2005). Genes and salt tolerance: bringing them together. New Phytol. 167, 645–663. doi: 10.1111/j.1469-8137.2005.01487.x
Navarro, L., Dunoyer, P., Jay, F., Arnold, B., Dharmasiri, N., Estelle, M., et al. (2006). A plant miRNA contributes to antibacterial resistance by repressing auxin signaling. Science 312, 436–439. doi: 10.1126/science.1126088
Nonogaki, H. (2010). MicroRNA gene regulation cascades during early stages of plant development. Plant Cell Physiol. 51, 1840–1846. doi: 10.1093/pcp/pcq154
Ohashi-Ito, K., and Fukuda, H. (2003). HD-Zip III homeobox genes that include a novel member, ZeHB-13 (Zinnia)/ATHB-15 (Arabidopsis), are involved in procambium and xylem cell differentiation. Plant Cell Physiol. 44, 1350–1358. doi: 10.1093/pcp/pcg164
Pant, B. D., Buhtz, A., Kehr, J., and Scheible, W. R. (2008). MicroRNA399 is a long-distance signal for the regulation of plant phosphate homeostasis. Plant J. 53, 731–738. doi: 10.1111/j.1365-313X.2007.03363.x
Pant, B. D., Musialak-Lange, M., Nuc, P., May, P., Buhtz, A., Kehr, J., et al. (2009). Identification of nutrient-responsive Arabidopsis and rapeseed microRNAs by comprehensive real-time polymerase chain reaction profiling and small RNA sequencing. Plant Physiol. 150, 1541–1555. doi: 10.1104/pp.109.139139
Peng, Z., He, S. P., Gong, W. F., Sun, J. L., Pan, Z. E., Xu, F. F., et al. (2014). Comprehensive analysis of differentially expressed genes and transcriptional regulation induced by salt stress in two contrasting cotton genotypes. BMC Genomics 15:760. doi: 10.1186/1471-2164-15-760
Rajagopalan, R., Vaucheret, H., Trejo, J., and Bartel, D. P. (2006). A diverse and evolutionarily fluid set of microRNAs in Arabidopsis thaliana. Genes Dev. 20, 3407–3425. doi: 10.1101/gad.1476406
Reyes, J. L., and Chua, N. H. (2007). ABA induction of miR159 controls transcript levels of two MYB factors during Arabidopsis seed germination. Plant J. 49, 592–606. doi: 10.1111/j.1365-313X.2006.02980.x
Schreiber, A. W., Shi, B. J., Huang, C. Y., Langridge, P., and Baumann, U. (2011). Discovery of barley miRNAs through deep sequencing of short reads. BMC Genomics 12:129. doi: 10.1186/1471-2164-12-129
Shi, R., and Chiang, V. L. (2005). Facile means for quantifying microRNA expression by real-time PCR. Biotechniques 39, 519–525. doi: 10.2144/000112010
Shinozaki, K., and Yamaguchi-Shinozaki, K. (2007). Gene networks involved in drought stress response and tolerance. J. Exp. Bot. 58, 221–227. doi: 10.1093/jxb/erl164
Si-Ammour, A., Windels, D., Arn-Bouldoires, E., Kutter, C., Ailhas, J., Meins, F., et al. (2011). miR393 and secondary siRNAs regulate expression of the TIR1/AFB2 auxin receptor clade and auxin-related development of Arabidopsis leaves. Plant Physiol. 157, 683–691. doi: 10.1104/pp.111.180083
Song, Q. X., Liu, Y. F., Hu, X. Y., Zhang, W. K., Ma, B. A., Chen, S. Y., et al. (2011). Identification of miRNAs and their target genes in developing soybean seeds by deep sequencing. BMC Plant Biol. 11:5. doi: 10.1186/1471-2229-11-5
Srivastava, P. K., Moturu, T. R., Pandey, P., Baldwin, I. T., and Pandey, S. P. (2014). A comparison of performance of plant miRNA target prediction tools and the characterization of features for genome-wide target prediction. BMC Genomics 15:348. doi: 10.1186/1471-2164-15-348
Subramanian, S., Fu, Y., Sunkar, R., Barbazuk, W. B., Zhu, J. K., and Yu, O. (2008). Novel and nodulation-regulated microRNAs in soybean roots. BMC Genomics 9:160. doi: 10.1186/1471-2164-9-160
Sullivan, C. S., and Ganem, D. (2005). MicroRNAs and viral infection. Mol. Cell 20, 3–7. doi: 10.1016/j.molcel.2005.09.012
Sunkar, R. (2010). MicroRNAs with macro-effects on plant stress responses. Semin. Cell Dev. Biol. 21, 805–811. doi: 10.1016/j.semcdb.2010.04.001
Sunkar, R., Chinnusamy, V., Zhu, J. H., and Zhu, J. K. (2007). Small RNAs as big players in plant abiotic stress responses and nutrient deprivation. Trends Plant Sci. 12, 301–309. doi: 10.1016/j.tplants.2007.05.001
Sunkar, R., Girke, T., and Zhu, J. K. (2005). Identification and characterization of endogenous small interfering RNAs from rice. Nucleic Acids Res. 33, 4443–4454. doi: 10.1093/nar/gki758
Sunkar, R., Kapoor, A., and Zhu, J. K. (2006). Posttranscriptional induction of two Cu/Zn superoxide dismutase genes in Arabidopsis is mediated by downregulation of miR398 and important for oxidative stress tolerance. Plant Cell 18, 2415. doi: 10.1105/tpc.106.041673
Tahir, M., Law, D. A., and Stasolla, C. (2006). Molecular characterization of PgAGO, a novel conifer gene of the ARGONAUTE family expressed in apical cells and required for somatic embryo development in spruce. Tree Physiol. 26, 1257–1270. doi: 10.1093/treephys/26.10.1257
Vidal, E. A., Araus, V., Lu, C., Parry, G., Green, P. J., Coruzzi, G. M., et al. (2010). Nitrate-responsive miR393/AFB3 regulatory module controls root system architecture in Arabidopsis thaliana. Proc. Natl. Acad. Sci. U.S.A. 107, 4477–4482. doi: 10.1073/pnas.0909571107
Wang, C., Ying, S., Huang, H. J., Li, K., Wu, P., and Shou, H. X. (2009). Involvement of OsSPX1 in phosphate homeostasis in rice. Plant J. 57, 895–904. doi: 10.1111/j.1365-313X.2008.03734.x
Wang, F. D., Li, L. B., Liu, L. F., Li, H. Y., Zhang, Y. H., Yao, Y. Y., et al. (2012). High-throughput sequencing discovery of conserved and novel microRNAs in Chinese cabbage (Brassica rapa L. ssp pekinensis). Mol. Genet. Genomics 287, 555–563. doi: 10.1007/s00438-012-0699-3
Wang, J. W., Wang, L. J., Mao, Y. B., Cai, W. J., Xue, H. W., and Chen, X. Y. (2005). Control of root cap formation by microRNA-targeted auxin response factors in Arabidopsis. Plant Cell 17, 2204–2216. doi: 10.1105/tpc.105.033076
Wang, J. Y., Yang, X. D., Xu, H. B., Chi, X. Y., Zhang, M., and Hou, X. L. (2012). Identification and characterization of microRNAs and their target genes in Brassica oleracea. Gene 505, 300–308. doi: 10.1016/j.gene.2012.06.002
Wang, L. W., Liu, H. H., Li, D. T., and Chen, H. B. (2011). Identification and characterization of maize microRNAs involved in the very early stage of seed germination. BMC Genomics 12:154. doi: 10.1186/1471-2164-12-154
Weitbrecht, K., Muller, K., and Leubner-Metzger, G. (2011). First off the mark: early seed germination. J. Exp. Bot. 62, 3289–3309. doi: 10.1093/jxb/err030
Wiese, K. C., and Hendriks, A. (2006). Comparison of P-RnaPredict and mfold - algorithms for RNA secondary structure prediction. Bioinformatics 22, 934–942. doi: 10.1093/bioinformatics/btl043
Windels, D., and Vazquez, F. (2011). miR393: integrator of environmental cues in auxin signaling? Plant Signal. Behav. 6, 1672–1675. doi: 10.4161/psb.6.11.17900
Wu, G., Park, M. Y., Conway, S. R., Wang, J. W., Weigel, D., and Poethig, R. S. (2009). The sequential action of miR156 and miR172 regulates developmental timing in Arabidopsis. Cell 138, 750–759. doi: 10.1016/j.cell.2009.06.031
Wu, H. J., Ma, Y. K., Chen, T., Wang, M., and Wang, X. J. (2012). PsRobot: a web-based plant small RNA meta-analysis toolbox. Nucleic Acids Res. 40, W22–W28. doi: 10.1093/nar/gks554
Wu, M. F., Tian, Q., and Reed, J. W. (2006). Arabidopsis microRNA167 controls patterns of ARF6 and ARF8 expression, and regulates both female and male reproduction. Development 133, 4211–4218. doi: 10.1242/dev.02602
Xie, F. L., Huang, S. Q., Guo, K., Xiang, A. L., Zhu, Y. Y., Nie, L., et al. (2007). Computational identification of novel microRNAs and targets in Brassica napus. FEBS Lett. 581, 1464–1474. doi: 10.1016/j.febslet.2007.02.074
Xiong, L. M., Wang, R. G., Mao, G. H., and Koczan, J. M. (2006). Identification of drought tolerance determinants by genetic analysis of root response to drought stress and abscisic acid. Plant Physiol. 142, 1065–1074. doi: 10.1104/pp.106.084632
Xu, M. Y., Dong, Y., Zhang, Q. X., Zhang, L., Luo, Y. Z., Sun, J., et al. (2012). Identification of miRNAs and their targets from Brassica napus by high-throughput sequencing and degradome analysis. BMC Genomics 13:421. doi: 10.1186/1471-2164-13-421
Yang, J. H., Han, S. J., Yoon, E. K., and Lee, W. S. (2006). 'Evidence of an auxin signal pathway, microRNA167-ARF8-GH3, and its response to exogenous auxin in cultured rice cells'. Nucleic Acids Res. 34, 1892–1899. doi: 10.1093/nar/gkl118
Yang, J. H., Liu, X. Y., Xu, B. C., Zhao, N., Yang, X. D., and Zhang, M. F. (2013). Identification of miRNAs and their targets using high-throughput sequencing and degradome analysis in cytoplasmic male-sterile and its maintainer fertile lines of Brassica juncea. BMC Genomics 14:9. doi: 10.1186/1471-2164-14-9
Yang, L., Conway, S. R., and Poethig, R. S. (2011). Vegetative phase change is mediated by a leaf-derived signal that represses the transcription of miR156. Development 138, 245–249. doi: 10.1242/dev.058578
Yang, X. Y., Wang, L. C., Yuan, D. J., Lindsey, K., and Zhang, X. L. (2013). Small RNA and degradome sequencing reveal complex miRNA regulation during cotton somatic embryogenesis. J. Exp. Bot. 64, 1521–1536. doi: 10.1093/jxb/ert013
Yu, X., Wang, H., Lu, Y. Z., de Ruiter, M., Cariaso, M., Prins, M., et al. (2012). Identification of conserved and novel microRNAs that are responsive to heat stress in Brassica rapa. J. Exp. Bot. 63, 1025–1038. doi: 10.1093/jxb/err337
Zhang, B. H., Pan, X. P., Cox, S. B., Cobb, G. P., and Anderson, T. A. (2006). Evidence that miRNAs are different from other RNAs. Cell. Mol. Life Sci. 63, 246–254. doi: 10.1007/s00018-005-5467-7
Zhang, J. H., Zhang, S. G., Han, S. Y., Li, X. M., Tong, Z. K., and Qi, L. W. (2013). Deciphering small noncoding RNAs during the transition from dormant embryo to germinated embryo in larches (Larix leptolepis). PLoS ONE 8:e81452. doi: 10.1371/journal.pone.0081452
Zhang, L. F., Chia, J. M., Kumari, S., Stein, J. C., Liu, Z. J., Narechania, A., et al. (2009). A genome-wide characterization of microRNA genes in maize. PLoS Genetics 5:e1000716. doi: 10.1371/journal.pgen.1000716
Zhang, X. H., Zou, Z., Gong, P. J., Zhang, J. H., Ziaf, K., Li, H. X., et al. (2011). Over-expression of microRNA169 confers enhanced drought tolerance to tomato. Biotechnol. Lett. 33, 403–409. doi: 10.1007/s10529-010-0436-0
Zhao, B. T., Ge, L. F., Liang, R. Q., Li, W., Ruan, K. C., Lin, H. X., et al. (2009). Members of miR-169 family are induced by high salinity and transiently inhibit the NF-YA transcription factor. BMC Mol. Biol. 10:29. doi: 10.1186/1471-2199-10-29
Zhao, B. T., Liang, R. Q., Ge, L. F., Li, W., Xiao, H. S., Lin, H. X., et al. (2007). Identification of drought-induced microRNAs in rice. Biochem. Biophys. Res. Commun. 354, 585–590. doi: 10.1016/j.bbrc.2007.01.022
Zhao, C. Z., Xia, H., Frazier, T. P., Yao, Y. Y., Bi, Y. P., Li, A. Q., et al. (2010). Deep sequencing identifies novel and conserved microRNAs in peanuts (Arachis hypogaea L.). BMC Plant Biol. 10:3. doi: 10.1186/1471-2229-10-3
Zhao, Y. T., Wang, M., Fu, S. X., Yang, W. C., Qi, C. K., and Wang, X. J. (2012). Small RNA profiling in Two Brassica napus cultivars identifies microRNAs with oil production- and development-correlated expression and new small RNA classes. Plant Physiol. 158, 813–823. doi: 10.1104/pp.111.187666
Zhou, X. F., Wang, G. D., Sutoh, K., Zhu, J. K., and Zhang, W. X. (2008). Identification of cold-inducible microRNAs in plants by transcriptome analysis. Biochim. Biophys. Acta 1779, 780–788. doi: 10.1016/j.bbagrm.2008.04.005
Keywords: drought stress, salt stress, microRNA, Brassica napus, seed germination
Citation: Jian H, Wang J, Wang T, Wei L, Li J and Liu L (2016) Identification of Rapeseed MicroRNAs Involved in Early Stage Seed Germination under Salt and Drought Stresses. Front. Plant Sci. 7:658. doi: 10.3389/fpls.2016.00658
Received: 14 October 2015; Accepted: 29 April 2016;
Published: 13 May 2016.
Edited by:
Alison Kingston-Smith, Aberystwyth University, UKReviewed by:
Shirish Anand Ranade, CSIR-National Botanical Research Institute, IndiaAnyela Valentina Camargo Rodriguez, Aberystwyth University, UK
Copyright © 2016 Jian, Wang, Wang, Wei, Li and Liu. This is an open-access article distributed under the terms of the Creative Commons Attribution License (CC BY). The use, distribution or reproduction in other forums is permitted, provided the original author(s) or licensor are credited and that the original publication in this journal is cited, in accordance with accepted academic practice. No use, distribution or reproduction is permitted which does not comply with these terms.
*Correspondence: Liezhao Liu, bGllemhhbzIwMDNAMTI2LmNvbQ==
†These authors have contributed equally to this work.