- 1Key Laboratory of Plant Resources Conservation and Sustainable Utilization, South China Botanical Garden, Chinese Academy of Sciences, Guangzhou, China
- 2Guangdong Provincial Key Laboratory of Applied Botany, South China Botanical Garden, Chinese Academy of Sciences, Guangzhou, China
- 3College of Life Science, University of Chinese Academy of Sciences, Beijing, China
- 4Zhangzhou Xiangcheng District Agricultural Bureau, Fujian, China
Mandarin (Citrus reticulata), a non-climacteric fruit, is an economically important fruit worldwide. The mechanism underlying senescence of non-climacteric fruit is poorly understood. In this study, a gel-based proteomic study followed by LC-ESI-MS/MS analysis was carried out to investigate the proteomic changes involved in peel senescence in harvested mandarin “Shatangju” fruit stored for 18 days. Over the course of the storage period, the fruit gradually senesced, accompanied by a decreased respiration rate and increased chlorophyll degradation and disruption of membrane integrity. Sixty-three proteins spots that showed significant differences in abundance were identified. The up-regulated proteins were mainly associated with cell wall degradation, lipid degradation, protein degradation, senescence-related transcription factors, and transcription-related proteins. In contrast, most proteins associated with ATP synthesis and scavenging of reactive oxygen species were significantly down-regulated during peel senescence. Three thioredoxin proteins and three Ca2+ signaling-related proteins were significantly up-regulated during peel senescence. It is suggested that mandarin peel senescence is associated with energy supply efficiency, decreased antioxidant capability, and increased protein and lipid degradation. In addition, activation of Ca2+ signaling and transcription factors might be involved in cell wall degradation and primary or secondary metabolism.
Introduction
Fruit ripening is a complex and genetically programmed process, which involves a series of organoleptic, physiological, and biochemical changes, resulting in the development of edible quality (Prasanna et al., 2007). However, ripening-associated changes also increase the susceptibility to physical injury and decrease resistance to microbial infection (Giovannoni, 2001), which cause enormous economic losses in some fruit crops. Therefore, an improved understanding of fruit ripening attributes may help to develop strategies to improve the nutritional and sensorial quality and reduce postharvest losses of fruit.
On the basis of ripening attributes, fruit are classified as climacteric or non-climacteric. Ethylene synthesis is essential for climacteric fruit ripening (Lin et al., 2009). Two systems (system-1 and system-2) are involved in ethylene biosynthesis. System-1 is responsible for the basal level of ethylene production in all plant tissues, whereas system-2 functions during climacteric fruit ripening and petal senescence (Lelievre et al., 2007). The transition from system-1 to system-2 results in the sharp increase in climacteric fruit ethylene biosynthesis, which initiates the ripening-related changes by a signal transduction cascade (Alexander and Grierson, 2002). In addition, a large group of transcription factors (TFs), such as NAC, WRKY, C2H2-type zinc finger, AP2/EREBP, and MYB, play important roles in fruit ripening and senescence (Lim et al., 2007; Asif et al., 2014). For non-climacteric fruit, no marked changes in respiration are observed and ethylene biosynthesis remains at very low levels (Alexander and Grierson, 2002). Some studies have linked abscisic acid to the regulation of non-climacteric fruit ripening and senescence (Ji et al., 2012; Wu J. et al., 2014). However, the ripening or senescence of non-climacteric fruit is still inadequately understood.
Proteomics provides an important approach to reveal the complexity of the post-harvest physiology of fruits at the protein level. Recently, a proteomics approach has been widely used to explore the molecular mechanism involved in ripening or senescence of fruits, including tomato (Wang et al., 2014), apple (Zheng et al., 2013), peach (Jiang et al., 2014), banana (Toledo et al., 2012), papaya (Huerta-Ocampo et al., 2012), mango (Wu H. X. et al., 2014), strawberry (Song et al., 2015), and bell pepper (Aizat et al., 2013). The elucidation of important physiological regulatory networks by such studies will allow optimization of storage and processing conditions to minimize product losses. In addition, the information obtained from proteomic studies will help to support the breeding and selection of novel cultivars to prolong shelf-life and improve nutrition and flavor quality (Pedreschi et al., 2013).
Citrus is one of the most economically important edible fruits worldwide. Citrus fruit are non-climacteric and can be classified into two classes, i.e., tight-skin citrus (such as sweet orange and pummelo) and loose-skin citrus (mainly mandarin) (Ding et al., 2015). In contrast to climacteric fruit, citrus fruit gradually senesce after harvest with dysfunction or malfunction of physiological processes in the fruit tissues, which finally leads to loss of storability (Ding et al., 2015). The recent publication of draft genomes for mandarin and sweet orange makes it possible to explore the mechanisms involved in citrus fruit senescence at the molecular level (Xu et al., 2013; Wu G.A. et al., 2014;). Several studies have analyzed the proteome of citrus fruit. Ma et al. (2013) reported that 2,4-D retarded Olinda Valencia orange senescence in postharvest citrus fruit by regulating the expression of stress-responsive and calcium-binding EF proteins. Yun et al. (2013) showed that the up-regulation of chloroplast stromal ascorbate peroxidase, ATP synthase beta subunit, and cytoplasmic malate dehydrogenase might be involved in heat treatment-induced disease resistance of citrus fruit. However, the ripening and senescence characteristics differed markedly among different cultivars. Therefore, it will be necessary to perform proteomic analyses of diverse citrus cultivars to unravel the ripening and senescence mechanism of citrus fruit.
To get more information on the senescence of harvested citrus fruit, the proteome of “Shatangju” mandarin peel at four stages of senescence were compared using a gel-based proteomic study (two-dimensional gel electrophoresis; 2-DE). The proteins that showed significant differences in abundance were sequenced using coupled mass spectrometry (liquid chromatography-electrospray ionization-tandem mass spectrometry; LC-ESI-MS/MS). In addition, selected postharvest physiological attributes were evaluated. The newly identified proteins enhance our understanding of the molecular basis of senescence of harvested non-climacteric fruit.
Materials and Methods
Plant Material
Mandarin (Citrus reticulata Blanco “Shatangju”) fruit were harvested at approximately 240 days after blooming from a commercial orchard in Zhaoqing, Guangdong Province, China. Fruit were selected for uniformity of shape, color, and size, with a total soluble solid of approximately 11.0%. The selected fruit were dipped for 3 min in 0.1% Sportak® (a.i. prochloraz; Bayer) fungicide solution to control postharvest diseases and allowed to air dry. The fruit were placed in polyethylene bags (0.03-mm film thickness) and stored at 25°C (80–85% relative humidity) for 18 days. Physiological parameters were evaluated and peel samples were taken at initiation of the experiment (day 0) and at three successive 6-day intervals during storage. The samples were immediately frozen in liquid nitrogen and stored at −80°C for protein extraction and analysis. Three independent biological replicates were conducted.
Measurement of Physiological Parameters
Eight fruit were sealed inside a 4.2-L plastic container for 2 h at 25°C. Aliquots (1 mL) of headspace gas were withdrawn from each container and injected into a gas chromatograph (GC-9A; Shimadzu, Kyoto, Japan). Carbon dioxide (CO2) concentrations were determined using a thermal conductivity detector and a Poropak N column (Shimadzu). Ethylene concentrations were measured using a flame ionization detector and an HP-PLOT Q capillary column (Agilent Technologies, Palo Alto, CA, USA). Rates of ethylene production and respiration were expressed on a fresh weight basis.
Fruit color was measured with a Konica Minolta CR-400 colorimeter (Konica Minolta Co. Ltd., Tokyo, Japan) in the CIE-L*a*b mode. Color changes were quantified as the hue angle with the formula h = 180° + tan−1 (b*/a*) in accordance with Li et al. (2012). The contents of chlorophyll and carotenoids were determined spectrophotometrically following the method of Lichtenthaler (1987).
Membrane permeability was expressed as relative electrolyte leakage and measured in accordance with the method of Sun et al. (2012). Malondialdehyde (MDA) content was measured following the method described by Huang et al. (2013).
Protein Extraction from Mandarin Peel
Five grams of mandarin peel tissue from each subgroup was used for protein extraction following the method of Li et al. (2015a). The concentration of total proteins was determined using the Bio-Rad Protein Assay kit (Bio-Rad, Hercules, CA, USA).
Two-Dimensional Electrophoresis and Image Analysis
Two-dimensional electrophoresis (2-DE) was performed in accordance with the method of Li et al. (2015a) with 17-cm IPGstrips (pH 5–8; BioRad). At least three independent gels for each biological replicate were run. After staining with Coomassie blue, PDQuest 2-DE software (version 8.0; Bio-Rad) was used to analyze the images. In brief, automated and manual matching functions were used to obtain the highest gel matching. The data were normalized using the total quantity of valid spots on the corresponding gel to account for quantitative variations in intensity of protein spots between samples. The normalized intensity of spots on three independent biological-replicate 2-DE gels was averaged and subjected to Student's t-test analysis. The spots that showed more than two-fold differences between two samples were considered to show significant changes in abundance and were excised for protein identification.
In-Gel Protein Digestion and Protein Identification by LC-ESI-MS/MS
In-gel protein digestion was performed in accordance with the method of Li et al. (2015b). LC-ESI-MS/MS was analyzed based on Orbitrap. Briefly, following protein digestion, peptide samples were desalted using a Strata X column (Phenomenex), vacuum-dried and then resuspended in a 200 μL volume of buffer A (2% ACN, 0.1% FA). After centrifugation, the supernatant was recovered to obtain a peptide solution with a final concentration of approximately 0.5 μg/μL. 10 μL supernatant was loaded on a LC-20AD nanoHPLC (Shimadzu, Kyoto, Japan) by the autosampler onto a 2 cm C18 trap column. Then, the peptides were eluted onto a 10 cm analytical C18 column (inner diameter 75 μm) packed in-house. The samples were loaded at 15 μL/min for 4 min, then the 91 min gradient was run at 400 nL/min starting from 2 to 35% B (98%ACN, 0.1%FA), followed by 5 min linear gradient to 80%, and maintenance at 80% B for 8 min, and finally return to 2% in 2 min.
The peptides were subjected to nanoelectrospray ionization followed by tandem mass spectrometry (MS/MS) in a LTQ Orbitrap Velos (Thermo) coupled online to the HPLC. Intact peptides were detected in the Orbitrap at a resolution of 60,000. Peptides were selected for MS/MS using collision induced dissociation (CID) operating mode with a normalized collision energy setting of 35%. Ion fragments were detected in the LTQ. A data-dependent procedure that alternated between one MS scan followed by ten MS/MS scans was applied for the ten most abundant precursor ions above a threshold ion count of 5000 in the MS survey scan with a following Dynamic Exclusion settings: repeat counts, 2; repeat duration, 30 s; and exclusion duration, 120 s. The applied electrospray voltage was 1.5 kV. Automatic gain control (AGC) was used to prevent overfilling of the ion trap; 1 × 104 ions were accumulated in the ion trap to generate CID spectra. For MS scans, the m/z scan range was 350–2000 Da.
Proteins were identified by using Mascot search engine (Matrix Science, London, UK version 2.3.02) against JGI_Citrus_clementina genome database (30796 sequences). A mass tolerance of 20 ppm for intact peptide and a 0.1 Da for fragmented ions were permitted, with allowance for one missed cleavages in the trypsin digests. Glu ->pro-Glu (N-term Q), Oxidation (M), Deamidated (NQ) as the potential variable modifications, and Carbamidomethyl (C), as fixed modifications were applied. The charge states of peptides were set to +2 and +3. Specifically, an automatic decoy database search was performed in Mascot by choosing the decoy checkbox in which a random sequence of database is generated and tested for raw spectra as well as the real database.
To reduce the probability of false peptide identification, peptides with ion scores greater than the “identity” score were counted as identified. Each confident protein identification involved at least one unique peptide. All matched peptides are shown in Table S1.
Protein–Protein Interaction Analysis
Protein–protein interaction (PPI) analysis of differentially expressed proteins during mandarin peel senescence was conducted following the method described by Dong et al. (2015) with minor modifications. Briefly, all proteins were subjected to a BLAST search against Arabidopsis thaliana data lodged in the STRING database (version 10.0; http://string-db.org). The matching proteins with a confidence score of at least 0.400 were used for PPI analysis. The PPI network was constructed and displayed using Cytoscape (version 3.0.2) software.
Statistical Analysis
Experiments were conducted with three biological replicates. The significance of differences in physiological parameters was tested by analysis of variance using SPSS (version 13.0; SPSS Inc., Chicago, IL, USA). Least significant differences were calculated to compare significant effects at the 5% level. Principal component analysis (PCA) of differentially expressed proteins at different stages of peel senescence was conducted using the “vegan” package in R version 3.1.0.
Results
Physiological Characteristics of Harvested Fruit during Storage
In contrast to climacteric fruit, the rates of ethylene production and respiration in harvested “Shatangju” mandarin fruit decreased with increasing duration of storage (Figures 1A,B). After storage for 18 days, the hue angle value (a measure of the change in peel color during senescence) decreased from the initial value of 70.58–63.07 (Figure 1C), and the percentage water loss increased significantly (Figure 1D). Consistent with the change in color, the chlorophyll content of the fruit peel decreased gradually during storage, accompanied by an increase in carotenoid content (Figures 1E,F). Relative electrolyte leakage is an index of cellular membrane integrity and fruit senescence. The initial (day 0) relative electrolyte leakage was 24.9%. After 18 days of storage, relative electrolyte leakage increased to 36.4% and MDA content showed a similar pattern (Figures 1G,H). Thus, “Shatangju” mandarin fruit exhibited typical non-climacteric senescence characteristics after harvest.
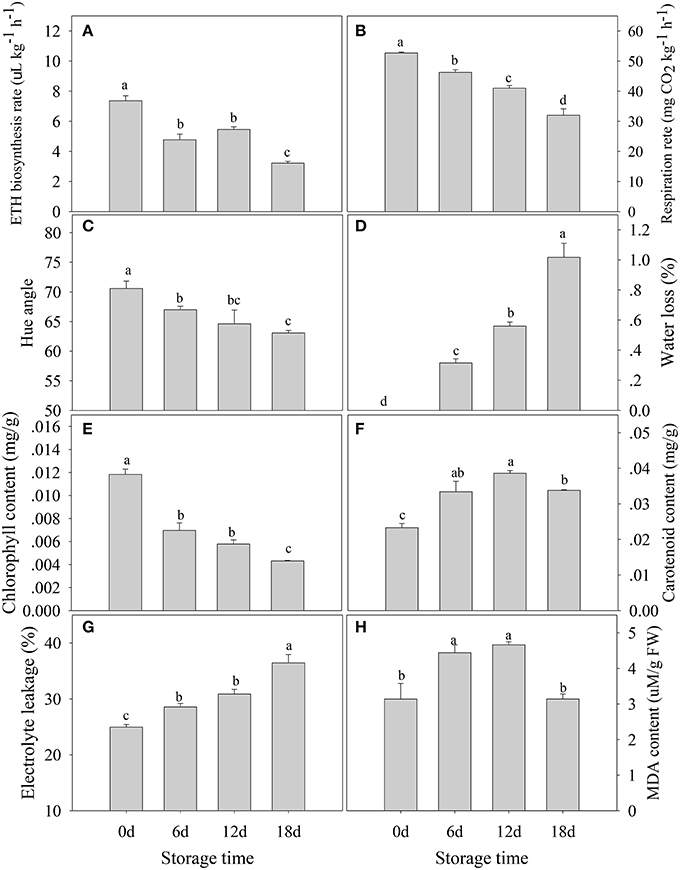
Figure 1. Physiological characteristics of mandarin “Shatangju” fruit during storage. (A) Ethylene biosynthesis rate; (B) respiration rate; (C) hue angle; (D) water loss; (E) chlorophyll content; (F) carotenoids content; (G) relative electrolyte leakage rate; (H) malondialdehyde (MDA) content. Ethylene biosynthesis rate, respiration rate, and water loss were measured for the whole fruit. Hue angle, chlorophyll content, relative electrolyte leakage rate, and MDA content were measured for the peel tissues only. Data presented are means ± standard errors (n = 3). Different letters above bars represent a significant difference (p < 0.05).
Proteomic Analysis of Peel from Harvested Fruit during Storage
A 2-DE analysis was performed to investigate changes in the proteome of mandarin peel during senescence. Over 700 reproducible protein spots were detected by PDQuest 2-D analysis software (Figure 2). Representative 2-DE gels showing proteins isolated from the peel at different stages of senescence are shown in Figure S1. After normalization against the total density, 64 spots were differentially expressed (P < 0.05) with more than two-fold differences in abundance. Of these spots, 63 spots were identified successfully by LC-ESI-MS/MS analysis with ion scores greater than the “identity” scores for each peptide (Figure 2). Compared with the day 0 sample, 41 protein spots were up-regulated significantly and 22 were down-regulated in the day 6 sample. Thirty-eight protein spots were up-regulated significantly and 25 were down-regulated in the day 12 sample. After 18 days of storage, 40 protein spots were up-regulated significantly and 23 were down-regulated. Of the protein spots, 33 were up-regulated during the entire senescence process and 18 were down-regulated in all of the 6, 12, and 18 days samples (Figure 3). High-magnification views of some of the typical differentially expressed proteins are shown in Figure 4.
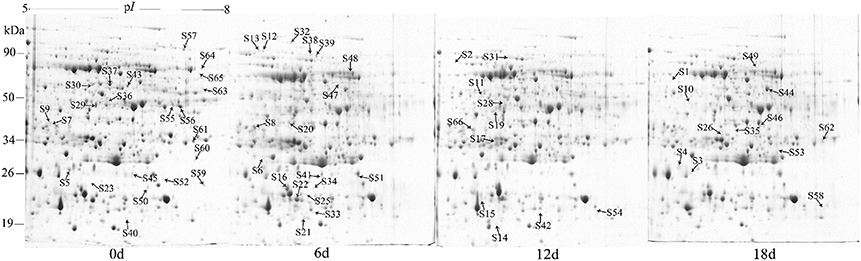
Figure 2. Representative two-dimensional electrophoresis maps for the peel at different stages of senescence in mandarin “Shatangju” fruit. The 2-DE maps show the total proteins isolated from the peel of harvested fruit after storage for 0, 6, 12, and 18 days. The labeled protein spots are those showing significant differences in abundance that were identified successfully.
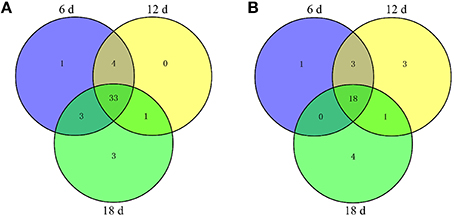
Figure 3. Number of differentially expressed proteins in the peel at different stages of senescence in mandarin “Shatangju” fruit. The number of (A) up-regulated proteins and (B) down-regulated proteins at different stages of senescence are indicated.
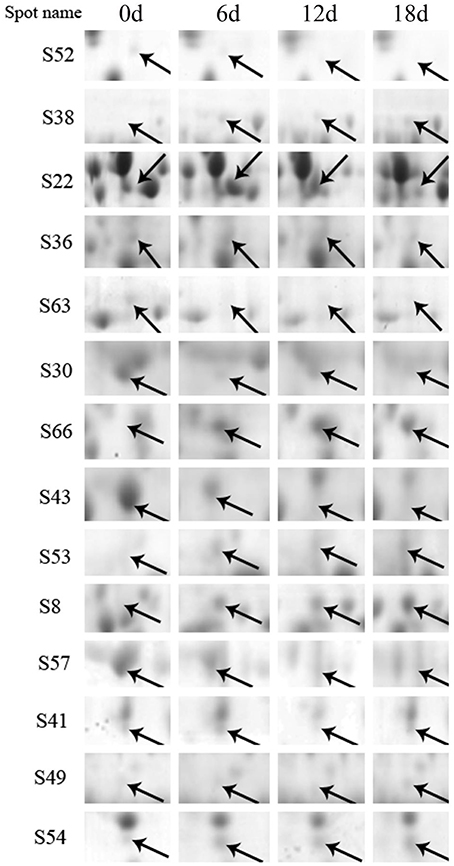
Figure 4. High-magnification images of selected differentially expressed proteins. Typical protein spots that showed significantly different abundances are indicated by arrows. Their sample names followed the manuscript annotation.
Functional Classification and Expression Patterns of Identified Proteins
The 63 differentially expressed proteins were classified into eight functional classes using Blast2Go software (Figure 5, Table 1). The major functional classes were metabolism, protein destination, energy, and signal transduction, which accounted for 25.4, 15.9, 12.7, and 12.7% of the differentially expressed proteins, respectively.
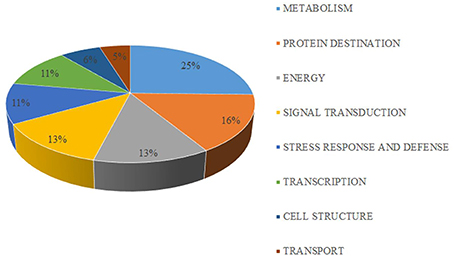
Figure 5. Functional classification of differentially expressed proteins identified from mandarin “Shatangju” peel at different stages of senescence. A total of 63 proteins were classified into biological process categories using the Blast2Go software.
Metabolism
Eleven proteins (S1, S3, S5, S14, S39, S41, S42, S48, S56, S58, and S60) classified in the metabolism category are involved in primary metabolic processes (Table 1). Of these proteins, phospholipase A 2A (S1), lactoylglutathionelyase family protein/glyoxalase I family protein (S3), S-adenosylmethionine synthetase 2 (S39), phospholipase D P2 (S41), nucleoside diphosphate kinase 2 (S42), and serine hydroxymethyl transferase 3 (S48) were significantly up-regulated during the senescence of mandarin peel.
With regard to secondary metabolic processes, the five proteins identified (S15, S17, S33, S34, and S54) comprised two UDP-glucosyltransferases, one thiazole biosynthetic enzyme, one S-adenosyl-L-methionine-dependent methyltransferase superfamily protein, and one chorismate synthase. All of the proteins were up-regulated during peel senescence.
Protein Destination
Ten proteins were involved in protein metabolic processes, of which three thioredoxins (TRXs; S10, S28, and S31), one rotamase CYP 4 (S26), one heat shock protein 21 (S4), and one ATP-dependent Clp protease (S11) were up-regulated after fruit harvest.
Energy
Of the eight proteins involved in energy metabolism, six proteins (ATP synthase alpha/beta family protein, malate dehydrogenase, phosphoglycerate kinase, Mog1/PsbP/DUF1795-like photosystem II reaction center PsbP family protein, photosystem II subunit P-1, and isocitrate dehydrogenase 1) were down-regulated, and one protein (triosephosphate isomerase) was up-regulated after fruit harvest.
Signal Transduction
Eight proteins were classified in the signal transduction category. Interestingly, except for plasma-membrane associated cation-binding protein 1 (S7) and regulatory component of ABA receptor 1 (S52), all of the other proteins in this group, such as calreticulin 3 and calcium-binding EF-hand family protein, were significantly induced during fruit storage, especially early in the storage period.
Stress Response and Defense
In this category, six proteins involved in the scavenging of reactive oxygen species (ROS), comprising iron/manganese superoxide dismutase (S6), monodehydroascorbate reductase 1 (S43), ascorbate peroxidase (S9 and S61), and catalase 2 (S55 and S64), were down-regulated during senescence (Table 1). The accelerated cell death 2 (ACD2, S19) protein was significantly up-regulated.
Transcription
Seven transcription-associated proteins were identified. Of these proteins, three TFs, namely Jumonji C (JmjC) domain-containing protein (S22), NAC domain protein (S44), and NmrA-like negative transcriptional regulator (S46), were up-regulated (Table 1). In addition, three RNA-binding (RRM/RBD/RNP motifs) family proteins (S20, S21, and S51) were up-regulated early in the storage period.
Cell Structure
Three cell wall modification-related proteins (pectin methylesterase 3, xyloglucan endotransglucosylase/hydrolase (XTH) 16 and XTH 24) were up-regulated during peel senescence. However, plant invertase/pectin methylesterase inhibitor (S57) was down-regulated.
Transport
Three proteins were classified in the transport category, consisting of ATP binding cassette protein 1 (S2), AAA-type ATPase family protein (S65), and ADP/ATP carrier 2 (S66).
Principal Component Analysis (PCA) of Differentially Expressed Proteins
The PCA indicated that the different fruit storage durations could be discriminated, based on the differentially expressed proteins. The first principal component (PC1) explained 60.29% of the total variation and discriminated the biological samples on the basis of the storage duration, whereas PC2 explained 24.17% of the variation (Figure 6A). According to the score plot for PC1 and PC2, the 6 and 12 days samples could not be discriminated, whereas the 0 and 18 days samples were easily discriminated (Figure 6A). Overall, the PCA demonstrated senescence stage-specific differences in protein expression in the peel of stored mandarin fruit. To clarify which of the proteins contributed to the discrimination, variable importance plots were produced, based on the loading scores for PC1 and PC2 (Figure 6B). The variable importance plots indicated that only a small number of proteins contributed highly to the observed variability between the samples collected at different storage time-points (Figures 7A,B).
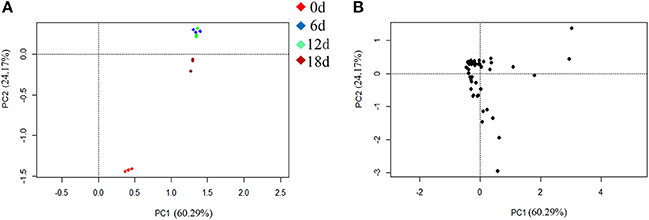
Figure 6. PCA of differentially expressed proteins identified from peel of mandarin “Shatangju” fruit at different senescence stages. (A) Score plot; (B) loading plot.
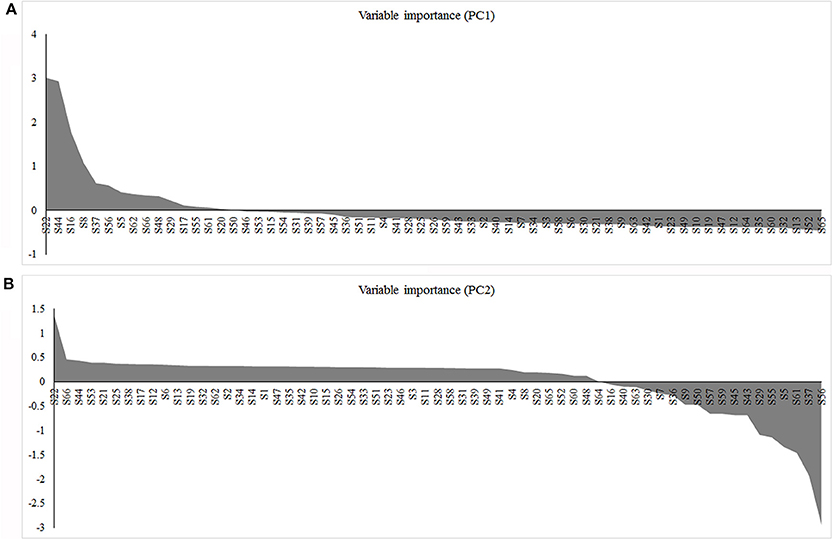
Figure 7. Variable importance plots for the PCA of differentially expressed proteins. (A) Loading scores for each identified protein on the first principal component axis (PC1). Proteins with a high positive PC1 loading score showed high abundance in samples from fruit stored for 6, 12, and 18 days. (B) Loading scores for each identified protein on the second principal component axis (PC2). Proteins with a high negative PC2 loading score showed high abundance in day 0 samples.
Protein–Protein Interaction (PPI) Network Analysis of Key Differentially Expressed Proteins Involved in Mandarin Peel Senescence
Mandarin peel senescence is a complex biological process that involves the interaction of numerous proteins. To further investigate the role of the differentially expressed proteins in the senescence process, we conducted a PPI analysis. The results indicated seven important functional categories with a total of 33 nodes (Table S2) mainly involved in signaling and transcription, protein processing, energy metabolism, stress response/defense, primary and secondary metabolism, cell wall modification, and transport (Figure 8). With the onset of peel senescence, the senescence signal was transduced by the Ca2+ signal pathway. Subsequently, protein processing became active, which might affect energy metabolism and response to stress. In addition, primary and secondary metabolism, and cell wall modification were also regulated by signaling and transcription pathways. Overall, the PPI analysis indicated that the differentially accumulated proteins involved in diverse physiological processes might interact and act synergistically during mandarin peel senescence.
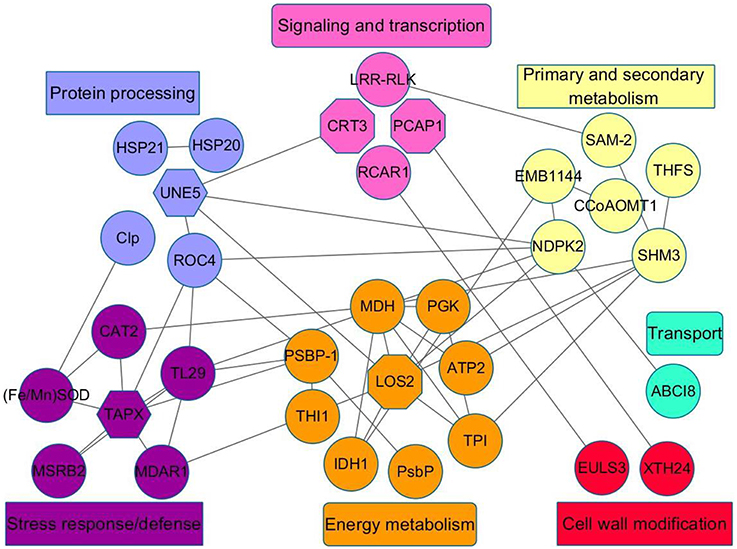
Figure 8. Regulatory network of differentially expressed proteins identified from peel of mandarin “Shatangju” fruit at different stages of senescence. Interactions among the differentially expressed proteins were analyzed using the STRING database with a confidence cutoff of 0.400. The interaction network was reconstructed using the Cytoscape software. The details of all nodes in the figure are listed in Table S2.
Discussion
Proteins differentially expressed in the peel tissues during mandarin fruit senescence were identified using a 2-DE approach. Comprehensive analysis of these proteins will help to elucidate the molecular mechanism involved in senescence of non-climacteric fruit.
Proteins Related to Energy Metabolism
Energy metabolism involves the release, transfer, storage, and use of energy in organisms. Glycolysis, the tricarboxylic acid (TCA) cycle, and oxidative phosphorylation are important energy-generating pathways that are active, at least in part, in almost all living organisms. In this study, four energy production-related enzymes showed significant differential expression in mandarin peel with increasing storage duration, namely phosphoglycerate kinase (PGK) (S37), enolase (ENO) (S29), isocitrate dehydrogenase (IDH) (S63), and malate dehydrogenase (MDH) (S36).
Phosphoglycerate kinase catalyzes the first ATP-generating step in the glycolytic pathway, whereas ENO catalyzes the last step of the glycolytic pathway, which generates phosphoenolpyruvate. We observed that PGK and ENO were significantly down-regulated during mandarin peel senescence, except for ENO at 18 days, which was inconsistent with the results reported for the ripening of some climacteric fruits. In these studies, increased carbon flux through glycolysis and increased glycolytic enzyme activities are reported at the transcript and protein levels (Borsani et al., 2009; D'Ambrosio et al., 2013). In a previous study we showed that PGK is involved in ethylene-induced chilling tolerance in harvested banana fruit (Li et al., 2015a).
Malate dehydrogenase reversibly catalyzes the conversion of malate to oxaloacetate in the citric acid cycle, whereas IDH catalyzes the oxidative decarboxylation of isocitrate in the TCA cycle. Both MDH and IDH are implicated in the generation of energy stored in the form of NADH in the TCA cycle. In this study, MDH and IDH were significantly down-regulated as mandarin peel senescence proceeded. Proteomic analyses suggest that up-regulated MDH or IDH are involved in ripening of the climacteric fruits tomato, apricot, and papaya (Rocco et al., 2006; Nogueira et al., 2012; D'Ambrosio et al., 2013). Thus, a discrepancy between climacteric fruit ripening and non-climacteric fruit senescence is suggested in terms of the TCA cycle.
In addition, one energy synthesis-related protein and one energy transport-related protein exhibited the opposite trend. ATP synthase alpha/beta subunits, an important component of the F1 ATP synthase complex, are vital for the final step of ATP synthesis from ADP and inorganic phosphate (Pedersen et al., 2000). The ADP/ATP carrier protein is a transport-related protein. The present results showed that one ATP synthase alpha/beta family protein (S30) was significantly down-regulated, whereas one ADP/ATP carrier protein (S66) was substantially up-regulated at the onset of senescence (Figure 4). These changes might be indicative of decreased ATP synthesis and increased energy consumption.
Energy supply is important for the maintenance of the homeostasis of proteins and membrane lipids (Liu et al., 2007; Sanchez-Bel et al., 2012). Mounting evidence suggests that senescence or physiological disorders of postharvest horticultural crops may be associated with inadequate energy supply and reduced efficiency of energy generation (Song et al., 2006; Wang H. et al., 2013; Li et al., 2016). Exogenous ATP treatment inhibits ROS accumulation, and maintains unsaturated fatty acid levels and membrane integrity, thus delaying senescence and deterioration of horticultural products (Yi et al., 2009, 2010). The down-regulation of PGK, MDH, IDH, and ATP synthase alpha/beta subunits inevitably would result in decreased efficiency of energy generation. Interestingly, both PGK (S37) and ADP/ATP carrier protein (S66) contributed highly to the variability in the proteome at different storage time-points, as indicated by the PCA (Figure 7). Therefore, it is suggested that reduced efficiency of energy generation and increased energy consumption led to energy depletion or ATP deficiency, which accelerated the senescence of mandarin peel.
Proteins Related to Stress Response and Defense
Reactive oxygen species (ROS) are mainly generated by photosynthesis in chloroplasts, respiration in mitochondria, and other physiological processes involving electron transfer in plants (Tovar-Mendez et al., 2011). Most fruits after harvest undergo vigorous aerobic respiration, accompanied by accumulation of ROS. Previous research documented that fruit deterioration and senescence may be induced by imbalance between the production and scavenging of ROS (Macarisin et al., 2010). Damage caused by ROS includes protein oxidation, lipid oxidation, DNA strand breakages, and base modification, which all contribute to senescence. Malondialdehyde accumulation, a product of lipid peroxidation, and relative electrolyte leakage rate, which is an index of cellular membrane integrity, may reflect the extent of oxidative damage or senescence (Marangoni et al., 1996). The present results showed that the relative electrolyte leakage rate and MDA content significantly increased after fruit storage for 6 days (Figure 1), indicating that peel senescence had been initiated.
Redox regulation has been proposed as a response to oxidative stress during fruit ripening and senescence (Zheng et al., 2013). Antioxidant enzymes are important redox regulation systems and mainly consist of ascorbate peroxidase (APX), superoxide dismutase, catalase (CAT), glutathione peroxidase, and glutathione reductase (Moller, 2001). Ascorbate peroxidase is the main enzyme responsible for H2O2 removal in plant subcellular compartments. In this study, two APXs (S9 and S61) were identified and down-regulated significantly with the onset of peel senescence, which was inconsistent with results observed in tomato (Rocco et al., 2006), apricot (D'Ambrosio et al., 2013), and apple (Zheng et al., 2013). Catalase is also an important enzyme catalyzing the hydrolysis of H2O2. The abundance of two CATS (S55 and S64) decreased during the storage period, consistent with previous findings for the non-climacteric grape berries during ripening (Giribaldi et al., 2007). The down-regulation of APXs and CATs suggests that the capability to scavenge peroxide decreased in mandarin peel, which, in turn, accelerated senescence.
Monodehydroascorbate reductase (MDHAR) is an important component of the glutathione–ascorbatecycle, which is a crucial antioxidant system in plant cells to protect against damage induced by ROS. Li et al. (2010) reported that overexpression of chloroplastic MDHAR enhanced tolerance to methyl viologen-mediated oxidative stresses in tomato. Nahar et al. (2015) observed that exogenous spermidine alleviates low-temperature injury in mung bean seedlings by increasing the activities of glutathione-ascorbate cycle enzymes such as APX, MDHAR, dehydroascorbate reductase, and glutathione reductase. We observed that MDHAR (S43) was down-regulated during mandarin fruit storage (Figure 4), which consequently would influence the non-enzymatic components of the glutathione-ascorbate cycle.
The PCA indicated that S43 (MDHAR), S55 (CAT), and S61 (APX), which showed low abundances with increasing storage duration, contributed highly to discrimination between the different storage periods (Figure 7). Considering all of the above-mentioned results, we propose that down-regulation of these antioxidant enzymes played a role in peel senescence during fruit storage, which resulted in excessive accumulation of ROS and oxidative damage.
Proteins Related to Signal Transduction and Transcription
Calcium, as a second messenger, plays an important role in plant growth, development and stress response. In the present study, three Ca2+ signaling-related proteins, consisting of two calcium-binding EF-hand family proteins (S16 and S35) and one calreticulin (S47), were differentially expressed during senescence. Calcium-binding EF-hand family proteins are Ca2+ sensors that recognize Ca2+signals. Calreticulin is a Ca2+-binding chaperone and modifies Ca2+ homeostasis in plants (Xiang et al., 2015). Overexpression of CBP gene decrease salt and osmotic tolerance in Arabidopsis (Chen et al., 2015). Therefore, we speculate that Ca2+ signaling initiates the senescence of harvested mandarin fruit.
Transcriptional regulation plays a vital role in fruit development and ripening as well as abiotic stresses. However, because of the limitations for protein identification and qualitative accuracy of 2-DE analysis, only a few differentially expressed TFs were detected in the current study. These TFs included a NAC domain-containing protein 50 (S44), JmjC domain-containing protein (S22), NmrA-like negative transcriptional regulator (S46), and bZIP (S32). NACs are plant-specific TFs that function in plant growth, development, and stress response (Zhao et al., 2010). In fruit, NACs are involved in the regulation of ripening via ethylene-signaling components (Shan et al., 2012) and low-temperature tolerance through ICE1-CBF (Shan et al., 2014). The present results showed that one NAC protein was substantially up-regulated as fruit senescence progressed. It is well known that non-climacteric ripening is ethylene independent. The involvement of NAC in mandarin fruit senescence possibly acts by regulating other genes rather than through ethylene signaling. The JmjC domain-containing protein shows histone demethylase activity (Tsukada et al., 2005). Histone modifications, such as methylation, acetylation, and phosphorylation, play an important role in epigenetic control of gene expression. Histone deacetylation is implicated in longan fruit senescence by interaction with ERF1 (Kuang et al., 2011). Up-regulation of the JmjC domain-containing protein may be beneficial for demethylation of certain TFs, which control the expression of senescence-related genes. The PCA also confirmed the important role of the JmjC domain-containing protein during mandarin peel senescence (Figure 7). Overall, the present proteomic data hinted that TFs may play important roles in regulating citrus peel senescence during fruit storage.
In addition, five transcription-related proteins, consisting of three RNA-binding proteins (RBPs) (S20, S21, and S51) and two cyclin-dependent kinase-activating kinase assembly factors (MAT1) (S12 and S25), were significantly up-regulated during mandarin fruit senescence. RBPs are associated with transcription, RNA processing, localization and stability, and translation (Shi et al., 2015). MAT1 mediates the assembly of cyclin-dependent kinase-activating kinase, which is functionally associated with the general TF IIH and is involved in transcription initiation and DNA repair. A role for RNA binding-proteins in the control of leaf senescence is implicated (Kim et al., 2008). There is little information available on the involvement of RBPs and MAT1 in fruit senescence. It is suggested that the up-regulated RBP and MAT1 proteins may be associated with the transcription or translation of certain senescence-responsive genes during mandarin peel senescence. The present PPI analysis also suggested these transcription-related proteins have important roles in citrus fruit senescence (Figure 8).
Proteins Related to Protein Metabolism
Fruit ripening involves protein modification, including biosynthesis, degradation, and folding. In this study, the differentially expressed proteins involved in protein metabolism mainly belonged to the TRX protein family, protease, and small heat shock protein. Moreover, these proteins may interact with other proteins to affect the mandarin peel senescence process (Figure 8).
Thioredoxins are small proteins with a conserved redox active-site WCG(P)PC, which regulate the redox status of target proteins (Holmgren et al., 2005). Thioredoxins play an important role in plant tolerance to oxidative stress by reducing the disulfide bond formed from the oxidation of cysteine residues by ROS (Dos Santos and Rey, 2006). It is well documented that oxidative stress may induce TRX expression (Shahpiri et al., 2009). We observed that three TRXs were significantly up-regulated in mandarin peel after 6 days of storage, indicating that the oxidation of protein cysteine residues occurred on a large scale. The increased expression of TRX proteins therefore would be beneficial for decreasing the extent of protein oxidation.
Caseinolytic protease (ClpP) is an energy-dependent serine protease, which unfolds protein substrates and transport them through a central pore and into the degradation chamber of ClpP. Up-regulation of ClpP is associated with ethylene-induced chilling tolerance in harvested banana fruit (Li et al., 2015a). An additional protein degradation-related protein, ubiquitin-associated (UBA)/TS-N domain-containing protein, was up-regulated in mandarin fruit, especially at the late storage stage. The ubiquitin–proteasome system is an important mechanism of protein degradation. Ubiquitin is covalently attached to a diverse array of target proteins and then degraded by proteasomes (Ramadan et al., 2015). Wang et al. (2014) reported that proteins involved in the ubiquitin–proteasome system were markedly induced during tomato fruit ripening. Given the significant up-regulation of ClpP (S41) and Ubac (S49) observed in the present study (Figure 4), it is suggested that ClpP and the UBA/TS-N domain-containing protein might be involved in protein degradation during the senescence of mandarin peel.
Proteins Related to Cell Wall Degradation
The plant primary cell wall is a complex matrix of polysaccharides, mainly consisting of pectic and hemicellulosic polysaccharides and cellulose. Modification of the cell wall is important to attain commercial quality in some fruits (Brummell and Harpster, 2001). However, over-softening results in loss of marketability and decreased disease resistance, which in turn accelerates fruit senescence. Depolymerization of pectic and hemicellulosic polysaccharides plays an important role in fruit ripening, leading to disassembly of the cellulose-hemicellulose network and a decrease in fruit firmness (Wang D. et al., 2013). Xyloglucans are the main component of hemicellulosic polysaccharides. Thus, depolymerization of hemicelluosic polysaccharides is associated with the degradation of xyloglucans. The XTH family is believed to play a crucial role in this process (Miedes et al., 2013). Increasing evidence indicates that XTHs are involved in postharvest softening of many fruits (Han et al., 2015; Legay et al., 2015). In the present study, two XTH proteins (S53 and S62) were significantly up-regulated after 6 days of storage, which may have accelerated xyloglucan depolymerization and disassembly of the cellulose-hemicellulose network.
Proteins Related to Primary Metabolism
In senescence anabolic metabolism gives way to catabolic metabolism, resulting in aging and finally death of the tissue. In this study, a number of proteins associated with primary metabolite degradation were up-regulated, while a number of synthesis-related proteins were down-regulated.
Phospholipids are major components of all cell membranes. The degradation of phospholipids is mediated by a variety of phospholipases, including phospholipase A, C, and D. Phospholipase D cleaves phospholipids into phosphatidic acid and alcohol. Phospholipase D is reported to be involved in leaf senescence. Suppression of phospholipase D retards abscisic acid- and ethylene-promotedleaf senescence in Arabidopsis by attenuating lipid degradation (Jia et al., 2013; Jia and Li, 2015). Natalini et al. (2013) reported that cutting stimulated phospholipase D activities in tomato, in parallel with rapid softening and membrane degradation (as indicated by increased electrolyteleakage). In the current study, one phospholipase D and one phospholipase A2 were significantly up-regulated during mandarin fruit senescence, consistent with the implicated increase in membrane degradation (Table 1, Figures 1G,H). These results suggested that phospholipase may play an important role in mandarin peel senescence by degrading phospholipid in cell membranes.
Hydroxyproline-rich glycoproteins are important cell wall proteins, which form a continuous glycol network with non-cellulosic polysaccharides via covalent bonds or non-covalent interactions, thereby strongly contributing to cell wall architecture (Hijazi et al., 2014). The present results showed that one hydroxyproline-rich glycoprotein (S56) was dramatically down-regulated as mandarin peel senesced, which indicated that cell wall biosynthesis decreased. Considering the above-mentioned results and the up-regulation of cell-wall-degrading enzymes, the cell wall network was likely to be disrupted, which, in turn, accelerated peel senescence and resulted in loss of resistance to biotic or abiotic stresses.
Overview of Important Biological Processes Involved in Mandarin Peel Senescence
With consideration of the differentially expressed proteins identified in this study and previous reports, we propose a preliminary overview of important processes involved in mandarin peel senescence (Figure 9). After 6 days of storage, senescence had been initiated in the peel. With the onset of the senescence, the capability for ROS scavenging and the efficiency of energy generation was notably decreased, which resulted in accumulation of ROS and deficiency in energy supply, respectively. Excessive ROS accumulation causes oxidative damage to macromolecules, including proteins, lipids, and DNA. To cope with oxidative stress, proteins associated with protein modification were up-regulated to repair or degrade the damaged proteins. Some proteins involved in protein degradation or lipid repair require adequate energy supply; however, the deficiency in energy supply, in turn, accelerated protein damage and lipid degradation. During mandarin peel senescence, senescence-related signaling was likely activated by Ca2+signaling and TFs might be involved in the regulation of cell wall degradation, primary or secondary metabolism, and protein processing.
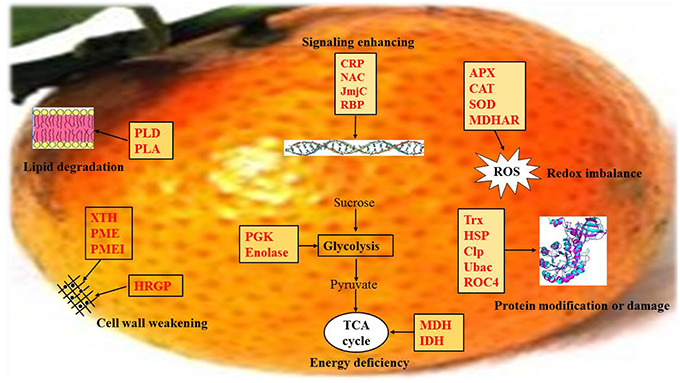
Figure 9. Schematic of the important differentially expressed proteins involved in mandarin “Shatangju” peel senescence. The proteins differentially expressed during peel senescence are indicated in red. CRP, Calcium related protein; NAC, NAM, ATAF1/2, CUC1/2; JmjC, Jumonji transcription factor; RBP, RNA-binding proteins; APX, Ascorbate peroxidase; CAT, Catalase; SOD, Superoxide dismutase; MDHAR, monodehydroascorbate reductase; PLD, Phospholipase D; PLA, Phospholipase A; XTH, Xyloglucan endotransglucosylase/hydrolase; PME, Pectin methylesterase; PMEI, Pectin methylesterase inhibitor; HRGP, Hydroxyproline-rich glycoprotein proteins; PGK, Phosphoglycerate kinase; Trx, Thioredoxins; HSP, Heat shock protein; Clp, Caseinolytic protease; Ubac, ubiquitin-associated (UBA)/TS-N domain-containing protein; ROC4, rotamase CYP 4; MDH, Malate dehydrogenase; IDH, Isocitrate dehydrogenase.
Conclusion
In this study, proteins differentially expressed during mandarin fruit senescence were mainly associated with primary and secondary metabolism, signaling and transcription, protein modification, energy metabolism, and stress response and defense. The PPI analysis indicated that these proteins might contribute cooperatively to the senescence of the mandarin peel. It is suggested that Ca2+ signaling initiates senescence via transcription regulators. Energy deficiency and weakening of the antioxidant system accelerated senescence. Degradation of cell structural and functional components, including lipids, proteins, and cell wall polysaccharides, contributed directly to the progression of senescence. This study enhances our understanding of senescence in non-climacteric fruit and will contribute to the development of novel technologies to reduce postharvest losses of citrus fruit.
Author Contributions
TL and XD conceived and designed the study. TL, JZ, HZ, and SY performed the experiments and analyzed the data. TL and XD drafted the manuscript. All authors participated in the interpretation of data, the revision of the manuscript. All authors approved the submission and agreed to be accountable for all aspects of the work.
Conflict of Interest Statement
The authors declare that the research was conducted in the absence of any commercial or financial relationships that could be construed as a potential conflict of interest.
Acknowledgments
This work was supported by National Basic Research Program of China (No. 2013CB127104), National Natural Science Foundation of China (Nos. 31171778; 31301821; 31271971; 31229004; 31301822), Science and Technology Planning Project of Guangdong Province (No. 2015B090901058; 2013B091500092), Science and Technology Service Network Initiative of Chinese Academy of Sciences (No.KFJ-EW-STS-118), and Key Project Program of Chinese Academy of Sciences (No. KSZD-EW-Z-021-3-3), Talent Program of Guangdong Province (No. 2014TX01N049), The Transformation Project of National Agricultural Science and Technology Achievements (No. 2013GB24910681), Talent Program of Wuzhong (WC201324) and Science and Technology Planning Project of Jiangsu Province (No. BZ2013004).
Supplementary Material
The Supplementary Material for this article can be found online at: http://journal.frontiersin.org/article/10.3389/fpls.2016.00725
References
Aizat, W. M., Able, J. A., Stangoulis, J. C. R., and Able, A. J. (2013). Proteomic analysis during capsicum ripening reveals differential expression of ACC oxidase isoform 4 and other candidates. Funct. Plant Biol. 40, 1115–1128. doi: 10.1071/FP12330
Alexander, L., and Grierson, D. (2002). Ethylene biosynthesis and action in tomato: a model for climacteric fruit ripeing. J. Exp. Bot. 53, 2039–2055. doi: 10.1093/jxb/erf072
Asif, M. H., Lakhwani, D., Pathak, S., Gupta, P., Bag, S. K., Nath, P., et al. (2014). Transcriptome analysis of ripe and unripe fruit tissue of banana identifies major metabolic networks involved in fruit ripening process. BMC Plant Biol. 14:316. doi: 10.1186/s12870-014-0316-1
Borsani, J., Budde, C. O., Porrini, L., Lauxmann, M. A., Lombardo, V., Murray, R., et al. (2009). Carbon metabolism of peach fruit harvest: changes in enzymes involved in organic acid and sugar level modifications. J. Exp. Bot. 60, 1823–1837. doi: 10.1093/jxb/erp055
Brummell, D. A., and Harpster, M. H. (2001). Cell wall metabolism in fruit softening and quality and its manipulation in transgenic plants. Plant Mol. Biol. 47, 311–340. doi: 10.1023/A:1010656104304
Chen, C., Sun, X., Duanmu, H., Zhu, D., Yu, Y., Cao, L., et al. (2015). GsCML27, a gene encoding a calcium-binding EF-hand protein from Glycine soja, plays differential roles in plant responses to bicarbonate, salt and osmotic stresses. PLoS ONE 10:e0141888. doi: 10.1371/journal.pone.0141888
D'Ambrosio, C., Arena, S., Rocco, M., Verrillo, F., Novi, G., Viscosi, V., et al. (2013). Proteomic analysis of apricot fruit during ripening. J. Proteomics 78, 39–57. doi: 10.1016/j.jprot.2012.11.008
Ding, Y., Chang, J., Ma, Q., Chen, L., Liu, S., Jin, S., et al. (2015). Network analysis of postharvest senescence process in citrus fruits revealed by transcriptomic and metabolomic profiling. Plant Physiol. 168, 357–376. doi: 10.1104/pp.114.255711
Dong, K., Zhen, S., Cheng, Z., Cao, H., Ge, P., and Yan, Y. (2015). Proteomic analysis reveals key proteins and phosphoproteins upon seed germination of wheat (Triticum aestivum L.). Front. Plant Sci. 6:1017. doi: 10.3389/fpls.2015.01017
Dos Santos, C. V., and Rey, P. (2006). Plant thioredoxins are key actors in the oxidative stress response. Trends Plant Sci. 11, 329–334. doi: 10.1016/j.tplants.2006.05.005
Giovannoni, J. (2001). Molecular biology of fruit maturation and ripening. Annu. Rev. Plant Physiol. Plant Mol. Biol. 52, 725–749. doi: 10.1146/annurev.arplant.52.1.725
Giribaldi, M., Perugini, I., Sauvage, F. X., and Schubert, A. (2007). Analysis of protein changes during grape berry ripening by 2-DE and MALDI-TOF. Proteomics 7, 3154–3170. doi: 10.1002/pmic.200600974
Han, Y., Zhu, Q., Zhang, Z., Meng, K., Hou, Y., Ban, Q., et al. (2015). Analysis of xyloglucan endotransglycosylase/hydrolase (XTH) genes and diverse roles of isoenzymes during persimmon fruit development and postharvest softening. PLoS ONE 10:e0123668. doi: 10.1371/journal.pone.0123668
Hijazi, M., Velasquez, S. M., Jamet, E., Estevez, J. M., and Albenne, C. (2014). An update on post-translational modifications of hydroxyproline-rich glycoproteins: toward a model highlighting their contribution to plant cell wall architecture. Front. Plant Sci. 5:395. doi: 10.3389/fpls.2014.00395
Holmgren, A., Johansson, C., Berndt, C., Lonn, M. E., Hudemann, C., and Lillig, C. H. (2005). Thiol redox control via thioredoxin and glutaredoxin systems. Biochem. Soc. Trans. 33, 1375–1377. doi: 10.1042/BST0331375
Huang, H., Jing, G., Guo, L., Zhang, D., Yang, B., Duan, X., et al. (2013). Effect of oxalic acid on ripening attributes of banana fruit during storage. Postharvest. Biol. Technol. 84, 22–27. doi: 10.1016/j.postharvbio.2013.04.002
Huerta-Ocampo, J. Á., Osuna-Castro, J. A., Lino-López, G. J., Barrera-Pacheco, A., Mendoza-Hernández, G., De León-Rodríguez, A., et al. (2012). Proteomic analysis of differentially accumulated proteins during ripening and in response to 1-MCP in papaya fruit. J. Proteomics 75, 2160–2169. doi: 10.1016/j.jprot.2012.01.015
Ji, K., Chen, P., Sun, L., Wang, Y., Dai, S., Li, Q., et al. (2012). Non-climacteric ripening in strawberry fruit is linked to ABA, FaNCED2 and FaCYP707A1. Funct. Plant Biol. 39, 351–357. doi: 10.1071/FP11293
Jia, Y., and Li, W. (2015). Characterisation of lipid changes in ethylene-promoted senescence and its retardation by suppression of phospholipase D deta in Arabidopsis leaves. Front. Plant Sci. 6:1045. doi: 10.3389/fpls.2015.01045
Jia, Y., Tao, F., and Li, W. (2013). Lipid profiling demonstrates that suppressing Arabidopsis hospholipase Dδ retards ABA-promoted leaf senescence by attenuating lipid degradation. PLoS ONE 8:e65687. doi: 10.1371/journal.pone.0065687
Jiang, L., Zhang, L., Shi, Y., Lu, Z., and Yu, Z. (2014). Proteomic analysis of peach fruit during ripening upon post-harvest heat combined with 1-MCP treatment. J. Proteomics 98, 31–43. doi: 10.1016/j.jprot.2013.11.019
Kim, C. Y., Bove, J., and Assmann, S. M. (2008). Overexpression of wound-responsive RNA-binding proteins induces leaf senescence and hypersensitive-like cell death. New Phytol. 180, 57–70. doi: 10.1111/j.1469-8137.2008.02557.x
Kuang, J. F., Chen, J. Y., Luo, M., Wu, K. Q., Sun, W., Jiang, Y. M., et al. (2011). Histone deacetylase HD2 interacts with ERF1 and is involved in longan fruit senescence. J. Exp. Bot. 63, 441–454. doi: 10.1093/jxb/err290
Legay, S., Guerriero, G., Deleruelle, A., Lateur, M., Evers, D., André, C. M., et al. (2015). Apple russeting as seen through the RNA-seq lens: strong alterations in the exocarp cell wall. Plant Mol. Biol. 88, 21–40. doi: 10.1007/s11103-015-0303-4
Lelievre, J. M., Latche, A., Jones, B., Bouzayen, M., and Pech, J. C. (2007). Ethylene and fruit ripening. Physiol. Plant. 101, 727–739. doi: 10.1111/j.1399-3054.1997.tb01057.x
Li, F., Wu, Q. Y., Sun, Y. L., Wang, L. Y., Yang, X. H., and Meng, Q. W. (2010). Overexpression of chloroplastic monodehydroascorbate reductase enhanced tolerance to temperature and methyl viologen-mediated oxidative stresses. Physiol. Plant. 139, 421–434. doi: 10.1111/j.1399-3054.2010.01369.x
Li, L., Lv, F., Guo, Y. Y., and Wang, Z. Q. (2016). Respiratory pathway metabolism and energy metabolism associated with senescence in postharvest Broccoli (Brassica oleracea L. var. italica) florets in response to O-2/CO2 controlled atmospheres. Postharvest Biol Tec. 111, 330–336. doi: 10.1016/j.postharvbio.2015.09.032
Li, Q., Wu, F., Li, T., Su, X., Jiang, G., Qu, H., et al. (2012). 1-Methylcyclopropene extends the shelf-life of ‘Shatangju’ mandarin (Citrus reticulate Blanco) fruit with attached leaves. Postharvest Biol. Technol. 67, 92–95. doi: 10.1016/j.postharvbio.2012.01.001
Li, T., Yun, Z., Zhang, D., Yang, C., Zhu, H., Jiang, Y., et al. (2015a). Proteomic analysis of differentially expressed proteins involved in ethylene-induced chilling tolerance in harvested banana fruit. Front. Plant Sci. 6:845. doi: 10.3389/fpls.2015.00845
Li, T., Zhu, H., Wu, Q., Yang, C., Duan, X., Qu, H., et al. (2015b). Comparative proteomic approaches to analysis of litchi pulp senescence after harvest. Food Res. Inter. 78, 274–285. doi: 10.1016/j.foodres.2015.09.033
Lichtenthaler, H. K. (1987). Chlorophylls and carotenoids: pigments of photosynthetic biomembranes. Meth. Enzymol. 148c, 350–382. doi: 10.1016/0076-6879(87)48036-1
Lim, P. O., Kim, H. J., and Nam, H. G. (2007). Leaf senescence. Annu. Rev. Plant Biol. 58, 115–136. doi: 10.1146/annurev.arplant.57.032905.105316
Lin, Z., Zhong, S., and Grierson, D. (2009). Recent advances in ethylene research. J. Exp. Bot. 60, 3311–3336. doi: 10.1093/jxb/erp204
Liu, H., Song, L., Jiang, Y., Joyce, D. C., Zhao, M., You, Y., et al. (2007). Short-term anoxia treatment maintains tissue energy levels and membrane integrity and inhibits browning of harvested litchi fruit. J. Sci. Food Agric. 87, 1767–1771. doi: 10.1002/jsfa.2920
Ma, Q., Ding, Y., Chang, J., Sun, X., Zhang, L., Wei, Q., et al. (2013). Comprehensive insights on how 2,4-dichlorophenoxyacetic acid retards senescence in post-harvest citrus fruits using transcriptomic and proteomic approaches. J. Exp. Bot. 65, 61–74. doi: 10.1093/jxb/ert344
Macarisin, D., Droby, S., Bauchan, G., and Wisniewski, M. (2010). Superoxide anion and hydrogen peroxide in the yeast antagonist-fruit interaction: a new role for reactive oxygen species in postharvest biocontrol? Postharvest Biol. Technol. 58, 194–202. doi: 10.1016/j.postharvbio.2010.07.008
Marangoni, A. G., Paima, T., and Stanley, D. W. (1996). Membrane effects in postharvest physiology. Postharvest Biol. Technol. 7, 193–217. doi: 10.1016/0925-5214(95)00042-9
Miedes, E., Suslov, D., Vandenbussche, F., Kenobi, K., Ivakov, A., Van Der Straeten, D., et al. (2013). Xyloglucan endotransglucosylase/hydrolase (XTH) overexpression affects growth and cell wall mechanics in etiolated Arabidopsis hypocotyls. J. Exp. Bot. 64, 2481–2497. doi: 10.1093/jxb/ert107
Moller, I. M. (2001). Plant mitochondria and oxidative stress: electron transport, NADPH turnover, and metabolism of reactive oxygen species. Annu. Rev. Plant Physiol. Plant Mol. Biol. 52, 561–591. doi: 10.1146/annurev.arplant.52.1.561
Nahar, K., Hasanuzzaman, M., Alam, M. M., and Fujita, M. (2015). Exogenous spermidine alleviates low temperature injury in mung bean (Vigna radiata L.) seedlings by modulating ascorbate-glutathione and glyoxalase pathway. Int. J. Mol. Sci. 16, 30117–30132. doi: 10.3390/ijms161226220
Natalini, A., Vanesa, M. D., Ferrante, A., and Pardossi, A. (2013). Effect of temperature and ripening stages on membrane integrity of fresh-cut tomatoes. Acta Physiol. Plant. 36, 191–198. doi: 10.1007/s11738-013-1399-2
Nogueira, S. B., Labate, C. A., Gozzo, F. C., Pilau, E. J., Lajolo, F. M., and do Nascimento, J. R. O. (2012). Proteomic analysis of papaya fruit ripening using 2DE-DIGE. J. Proteomics 75, 1428–1439. doi: 10.1016/j.jprot.2011.11.015
Pedersen, P. L., Ko, Y. H., and Hong, S. J. (2000). ATP syntheses in the year 2000: evolving views about the structures of these remarkable enzyme complexes. J. Bioenerg. Biomembr. 32, 325–332. doi: 10.1023/A:1005594800983
Pedreschi, R., Lurie, S., Hertog, M., Nicolai, B., Mes, J., and Woltering, E. (2013). Post-harvest proteomics and food security. Proteomics 13, 1772–1783. doi: 10.1002/pmic.201200387
Prasanna, V., Prabha, T. N., and Tharanathan, R. N. (2007). Fruit ripening phenomena - An overview. Crit. Rev. Food Sci. Nutr. 47, 1–19. doi: 10.1080/10408390600976841
Ramadan, A., Nemoto, K., Seki, M., Shinozaki, K., Takeda, H., Takahashi, H., et al. (2015). Wheat germ-based protein libraries for the functional characterisation of the Arabidopsis E2 ubiquitin conjugating enzymes and the RING-type E3 ubiquitin ligase enzymes. BMC Plant Biol. 15:275. doi: 10.1186/s12870-015-0660-9
Rocco, M., D'Ambrosio, C., Arena, S., Faurobert, M., Scaloni, A., and Marra, M. (2006). Proteomic analysis of tomato fruits from two ecotypes during ripening. Proteomics 6, 3781–3791. doi: 10.1002/pmic.200600128
Sanchez-Bel, P., Egea, I., Sanchez-Ballesta, M. T., Sevillano, L., Del Carmen Bolarin, M., and Flores, F. B. (2012). Proteome changes in tomato fruits prior to visible symptoms of chilling injury are linked to defensive mechanisms, uncoupling of photosynthetic processes and protein degradation machinery. Plant Cell Physiol. 53, 470–484. doi: 10.1093/pcp/pcr191
Shahpiri, A., Svensson, B., and Finnie, C. (2009). From proteomics to structural studies of cytosolic/mitochondrial-type thioredoxin systems in barley seeds. Mol. Plant 2, 378–389. doi: 10.1093/mp/ssn096
Shan, W., Kuang, J. F., Chen, L., Xie, H., Peng, H. H., Xiao, Y. Y., et al. (2012). Molecular characterization of banana NAC transcription factors and their interactions with ethylene signalling component EIL during fruit ripening. J. Exp. Bot. 63, 5171–5187. doi: 10.1093/jxb/ers178
Shan, W., Kuang, J. F., Lu, W. J., and Chen, J. Y. (2014). Banana fruit NAC transcription factor MaNAC1 is a direct target of MaICE1 and involved in cold stress through interacting with MaCBF1. Plant Cell Environ. 37, 2116–2127. doi: 10.1111/pce.12303
Shi, X., Hanson, M. R., and Bentolila, S. (2015). Two RNA recognition motif-containing proteins are plant mitochondrial editing factors. Nucleic Acids Res. 43, 3814–3825. doi: 10.1093/nar/gkv245
Song, J., Du, L., Li, L., Palmer, L. C., Forney, C. F., Fillmore, S., et al. (2015). Targeted quantitative proteomic investigation employing multiple reaction monitoring on quantitative changes in proteins that regulate volatile biosynthesis of strawberry fruit at different ripening stages. J. Proteomics 126, 288–295. doi: 10.1016/j.jprot.2015.06.004
Song, L. L., Liu, H., Su, X. G., You, Y. L., and Jiang, Y. M. (2006). Effects of adenosine triphosphate on the vase life of cut carnation flowers. Aust. J. Exp. Agr. 46, 137–139. doi: 10.1071/EA05011
Sun, J., Li, C., Prasad, K. N., You, X., Li, L., Liao, F., et al. (2012). Membrane deterioration, enzymatic browning and oxidative stress in fresh fruits of three litchi cultivars during six-day storage. Sci. Hortic. 148, 97–103. doi: 10.1016/j.scienta.2012.09.023
Toledo, T. T., Nogueira, S. B., Cordenunsi, B. R., Gozzo, F. C., Pilau, E. J., Lajolo, F. M., et al. (2012). Proteomic analysis of banana fruit reveals proteins that are differentially accumulated during ripening. Postharvest Biol. Technol. 70, 51–58. doi: 10.1016/j.postharvbio.2012.04.005
Tovar-Mendez, A., Matamoros, M. A., Bustos-Sanmamed, P., Dietz, K. J., Cejudo, F. J., Rouhier, N., et al. (2011). Peroxiredoxins and NADPH-dependent thioredoxin systems in the model legume Lotus japonicus. Plant Physiol. 156, 1535–1547. doi: 10.1104/pp.111.177196
Tsukada, Y. I., Fang, J., Erdjument-Bromage, H., Warren, M. E., Borchers, C. H., Tempst, P., et al. (2005). Histone demethylation by a family of JmjC domain-containing proteins. Nature 439, 811–816. doi: 10.1038/nature04433
Wang, D., Zhang, H., Wu, F., Li, T., Liang, Y., and Duan, X. (2013). Modification of pectin and hemicellulose polysaccharides in relation to aril breakdown of harvested longan fruit. Int. J. Mol. Sci. 14, 23356–23368. doi: 10.3390/ijms141223356
Wang, H., Qian, Z., Ma, S., Zhou, Y., Patrick, J. W., Duan, X. W., et al. (2013). Energy status of ripening and postharvest senescent fruit of litchi (Litchi chinensis Sonn.). BMC Plant Biol. 13:55. doi: 10.1186/1471-2229-13-55
Wang, Y., Wang, W., Cai, J., Zhang, Y., Qin, G., and Tian, S. (2014). Tomato nuclear proteome reveals the involvement of specific E2 ubiquitin-conjugating enzymes in fruit ripening. Genome Biol. 15:548. doi: 10.1186/s13059-014-0548-2
Wu, G. A., Prochnik, S., Jenkins, J., Salse, J., Hellsten, U., Murat, F., et al. (2014). Sequencing of diverse mandarin, pummelo and orange genomes reveals complex history of admixture during citrus domestication. Nat. Biotechnol. 32, 656–662. doi: 10.1038/nbt.2906
Wu, H. X., Jia, H. M., Ma, X. W., Wang, S. B., Yao, Q. S., Xu, W. T., et al. (2014). Transcriptome and proteomic analysis of mango (Mangifera indica Linn) fruits. J. Proteomics 105, 19–30. doi: 10.1016/j.jprot.2014.03.030
Wu, J., Xu, Z., Zhang, Y., Chai, L., Yi, H., and Deng, X. (2014). An integrative analysis of the transcriptome and proteome of the pulp of a spontaneous late-ripening sweet orange mutant and its wild type improves our understanding of fruit ripening in citrus. J. Exp. Bot. 65, 1651–1671. doi: 10.1093/jxb/eru044
Xiang, Y., Lu, Y. H., Song, M., Wang, Y., Xu, W., Wu, L., et al. (2015). Overexpression of Triticum aestivum Calreticulin gene (TaCRT1) improves salinity tolerance in tobacco. PLoS ONE 10:e0140591. doi: 10.1371/journal.pone.0140591
Xu, Q., Chen, L. L., Ruan, X., Chen, D., Zhu, A., Chen, C., et al. (2013). The draft genome of sweet orange (Citrus sinensis). Nat. Genet. 45, U59–U92. doi: 10.1038/ng.2472
Yi, C., Jiang, Y. M., Shi, J., Qu, H. X., Duan, X. W., Yang, B., et al. (2009). Effect of adenosine triphosphate on changes of fatty acids in harvested litchi fruit infected by Peronophythora litchii. Postharvest Biol. Tec. 54, 159–164. doi: 10.1016/j.postharvbio.2009.06.008
Yi, C., Jiang, Y. M., Shi, J., Qu, H. X., Xue, S., Duan, X. W., et al. (2010). ATP regulation of antioxidant properties and phenolics in litchi fruit during browning and pathogen infection process. Food Chem. 118, 42–47. doi: 10.1016/j.foodchem.2009.04.074
Yun, Z., Gao, H., Liu, P., Liu, S., Luo, T., Jin, S., et al. (2013). Comparative proteomic and metabolomic profiling of citrus fruit with enhancement of disease resistance by postharvest heat treatment. BMC Plant Biol. 13:44. doi: 10.1186/1471-2229-13-44
Zhao, Q., Gallego-Giraldo, L., Wang, H., Zeng, Y., Ding, S. Y., Chen, F., et al. (2010). An NAC transcription factor orchestrates multiple features of cell wall development in Medicago truncatula. Plant J. 63, 100–114. doi: 10.1111/j.1365-313x.2010.04223.x
Keywords: mandarin, proteomics, peel, senescence, Ca2+ signaling
Citation: Li T, Zhang J, Zhu H, Qu H, You S, Duan X and Jiang Y (2016) Proteomic Analysis of Differentially Expressed Proteins Involved in Peel Senescence in Harvested Mandarin Fruit. Front. Plant Sci. 7:725. doi: 10.3389/fpls.2016.00725
Received: 30 March 2016; Accepted: 11 May 2016;
Published: 31 May 2016.
Edited by:
Pingfang Yang, Wuhan Botanical Garden, Chinese Academy of Sciences, ChinaReviewed by:
Abelmon Silva Gesteira, Embrapa, BrazilYunjiang Cheng, Huazhong Agricultural University, China
Copyright © 2016 Li, Zhang, Zhu, Qu, You, Duan and Jiang. This is an open-access article distributed under the terms of the Creative Commons Attribution License (CC BY). The use, distribution or reproduction in other forums is permitted, provided the original author(s) or licensor are credited and that the original publication in this journal is cited, in accordance with accepted academic practice. No use, distribution or reproduction is permitted which does not comply with these terms.
*Correspondence: Xuewu Duan, xwduan@scbg.ac.cn