- 1State Key Laboratory Breeding Base for Zhejiang Sustainable Pest and Disease Control, Institute of Vegetables, Zhejiang Academy of Agricultural Sciences, Hangzhou, China
- 2College of Chemistry and Life Science, Zhejiang Normal University, Jinhua, China
The Hsp20 genes are involved in the response of plants to environment stresses including heat shock and also play a vital role in plant growth and development. They represent the most abundant small heat shock proteins (sHsps) in plants, but little is known about this family in tomato (Solanum lycopersicum), an important vegetable crop in the world. Here, we characterized heat shock protein 20 (SlHsp20) gene family in tomato through integration of gene structure, chromosome location, phylogenetic relationship, and expression profile. Using bioinformatics-based methods, we identified at least 42 putative SlHsp20 genes in tomato. Sequence analysis revealed that most of SlHsp20 genes possessed no intron or a relatively short intron in length. Chromosome mapping indicated that inter-arm and intra-chromosome duplication events contributed remarkably to the expansion of SlHsp20 genes. Phylogentic tree of Hsp20 genes from tomato and other plant species revealed that SlHsp20 genes were grouped into 13 subfamilies, indicating that these genes may have a common ancestor that generated diverse subfamilies prior to the mono-dicot split. In addition, expression analysis using RNA-seq in various tissues and developmental stages of cultivated tomato and the wild relative Solanum pimpinellifolium revealed that most of these genes (83%) were expressed in at least one stage from at least one genotype. Out of 42 genes, 4 genes were expressed constitutively in almost all the tissues analyzed, implying that these genes might have specific housekeeping function in tomato cell under normal growth conditions. Two SlHsp20 genes displayed differential expression levels between cultivated tomato and S. pimpinellifolium in vegetative (leaf and root) and reproductive organs (floral bud and flower), suggesting inter-species diversification for functional specialization during the process of domestication. Based on genome-wide microarray analysis, we showed that the transcript levels of SlHsp20 genes could be induced profusely by abiotic and biotic stresses such as heat, drought, salt, Botrytis cinerea, and Tomato Spotted Wilt Virus (TSWV), indicating their potential roles in mediating the response of tomato plants to environment stresses. In conclusion, these results provide valuable information for elucidating the evolutionary relationship of Hsp20 gene family and functional characterization of the SlHsp20 gene family in the future.
Introduction
Plants live in a complex environment, where multiple biotic and abiotic stresses may seriously restrict their growth and development (Cramer et al., 2011). In the recent years, due to unprecedented global warming caused by various factors, high temperature has appeared as one of the most severe abiotic stresses around the world. To survive and acclimatize under the adverse environment conditions, plants have established self-defense mechanisms during the course of long-term evolution. Heat shock proteins (Hsps), acknowledged as evolutionarily conserved ubiquitous proteins in all organisms, were first discovered in Drosophila melanogaster in response to the elevated temperature stress (Ritossa, 1962; Neta-Sharir et al., 2005; Cashikar et al., 2006). Previous studies have shown that high temperature as well as other environmental cues (cold, salinity, drought, heavy metals, anoxia, pathogens, etc.) could induce the occurrence of Hsps (Lindquist and Craig, 1988; Wang et al., 2003). In addition, the Hsps were also found to be associated with plant growth and development, such as embryogenesis, seed germination, and fruit maturation (Neta-Sharir et al., 2005).
According to sequence homology and molecular weight, Hsps in eukaryotes can be grouped into six families such as Hsp110, Hsp90, Hsp70, Hsp60, small heat shock protein (sHsp) of 15–42 kDa (or Hsp20) and ubiquitin (Carper et al., 1987; Sarkar et al., 2009). Out of these six groups of Hsps, sHsps are the most primary and abundant proteins under thermal stimulation in many species (Vierling et al., 1989; Vierling, 1991). Notably, among eukaryotes, the higher plants possessed more quantities of Hsp20s (Vierling, 1991). As Hsp20 is encoded by a multigene family, it is considered as the most ample and complicated member in Hsps (Vierling, 1991). The characteristic feature of Hsp20 is the presence of a carboxyl-terminal conserved domain of 80–100 amino acid residues, which can be defined as the α-crystallin domain (ACD). This highly conserved ACD, which is flanked by a short carboxyl-terminal extension and a variable amino-terminal domain, is believed to comprise two hydrophobic β-sheet motifs that are separated by a hydrophilic α-helical region of variable length (de Jong et al., 1998). Moreover, the Hsp20 gene family also exhibits extensive sequence variability and evolutionary divergence, which is remarkably different from other families of Hsps (Basha et al., 2012).
An earlier study showed that Hsp20 proteins in eukaryotes, which are collectively known as molecular chaperones, function as multimeric complexes ranging from 8 to 24 or more subunits (Van Montfort et al., 2002). These chaperons can selectively bind to partially folded or denatured proteins in an ATP-independent manner, which can prevent proteins from the irreversible aggregation and facilitate them folding properly (Lee and Vierling, 2000; Sun et al., 2002; Van Montfort et al., 2002). Recent studies revealed that these chaperones were important for disease resistance triggered by resistance (R) proteins and played a fundamental role in plant immunity (Botër et al., 2007; Shirasu, 2009).
To date, the Hsp20 gene families have been investigated in several plant species, including Arabidopsis, rice, soybean, pepper, and Populus trchocarpa (Scharf et al., 2001; Waters et al., 2008; Ouyang et al., 2009; Sarkar et al., 2009; Lopes-Caitar et al., 2013; Guo et al., 2015). In addition, some key features of Hsp20 and biologic function of several Hsp20 genes had been identified (Nautiyal and Shono, 2010; Goyal et al., 2012; Huther et al., 2013; Mahesh et al., 2013; Arce et al., 2015; Zhang et al., 2016). Although the availability of the tomato whole-genome sequence provides valuable resources for getting into an in-depth understanding of Hsp20s (Sato et al., 2012), little information is available on the integrated Hsp20 family at whole genomic level in tomato.
In the current paper, the members of SlHsp20 gene family in tomato were identified using a bioinformatics method and characterized by integration of sequence features, chromosome location, phylogenetic relationship, evolutionary origin, and expression patterns. These results provide valuable information that can be implicated in elucidating the evolutionary relationship of Hsp20 gene family in higher plants and functional characterization of the SlHsp20 gene family in the future.
Materials and Methods
Retrieval and Identification of Hsp20 Genes in Tomato
In this paper, the predicted SlHsp20 genes were identified as follows: firstly, the tomato genome sequences were downloaded from the database Sol Genomics Network (SGN, Release 2. 5, http://solgenomics.net/) and used to set up a local database by the software “DNATOOLs.” Secondly, the Hidden Markov Model (HMM) profile of Hsp20 domain (PF00011) from PFam (http://pfam.sanger.ac.uk/) was employed to search against the local database using BlastP method (e < 1e-5). Furthermore, Hsp20 candidates with incomplete Hsp20 domain might be missed using HMM profile. Name search using the word “hsp20” as a keyword also applied to retrieve in SGN database. The redundant sequences were manually removed. Finally, all these predicted genes were examined for the Hsp20 domain in SMART (http://smart.embl-heidelberg.de/smart/batch.pl), Pfam and InterProScan (http://www.ebi.ac.uk/interpro/), and those without the common Hsp20 domain were excluded.
Sequence Analysis and Structural Characterization
Information of candidate SlHsp20 genes was obtained via searching the SGN database (http://solgenomics.net/search/locus), including chromosome locations, intron numbers, genomic sequences, coding sequences (CDS), and amino acid sequences. Intron-exon structure was determined by alignment of genome DNA and full-length cDNA sequence using Gene Structure Display Server 2.0 (http://gsds.cbi.pku.edu.cn/) (Hu et al., 2015). Molecular weight, theoretical isoelectric point (theoretical pI), and instability index (II; with the value >40 classified as unstable) of SlHsp20 proteins were analyzed by using ProtParam tool (http://web.expasy.org/protparam/).
The putative protein sequences were subjected to MEME program (http://meme-suite.org/tools/meme) to investigate conserved motifs with the following parameters: site distribution—any number of repetitions, number of motifs—10, the motif width between 6 and 200.
Phylogenetic Analysis
To illuminate evolutionary relationship of Hsp20 gene family, the representative Hsp20 genes from Arabidopsis, rice, soybean, bluebunch wheatgrass, barley, common wheat, Eurasian aspen, together with SlHsp20 genes from tomato, were selected for constructing phylogenetic tree (Ouyang et al., 2009; Sarkar et al., 2009; Lopes-Caitar et al., 2013). Multiple sequence alignment of Hsp20 proteins was conducted using ClustalX 1.83. An un-rooted Neighbor-joining phylogenetic tree was constructed using MEGA 7.0 software with default settings (Kumar et al., 2016). The bootstrap test was performed by 1000 replications.
Four Online tools were employed to predict subcellular localization, including, Predotar (https://urgi.versailles.inra.fr/Tools/Predotar), Wolf Psort (http://www.genscript.com/psort/wolf_psort.html), TargetP (http://www.cbs.dtu.dk/services/TargetP/), and MultiLoc (http://abi.inf.uni-tuebingen.de/Services/MultiLoc2). The prediction of signal peptide and transmembrane domain was performed with SMART program (http://smart.embl-heidelberg.de/smart/batch.pl).
Chromosome Localization and Gene Duplication
Chromosome mapping of the candidate SlHsp20 genes was viewed using the software MapDraw V2.1 (Liu and Meng, 2003). Tandem duplication and segmental duplication were also further investigated. The former was confirmed with the following criteria: (1) an array of two or more SlHsp20 genes within a range of 100 kb distance; (2) the alignment had a coverage rate more than 70% of the longer gene; (3) and the identity of the aligned region was no less than 70% (Li et al., 2010; Huang et al., 2012; Wei et al., 2016). The latter was identified based on Plant Genome Duplication Database (PGDD, http://chibba.agtec.uga.edu/duplication/index/locus).
Tissue-Specific Expression Analysis
In this study, RNA-seq data from Tomato Functional Genomics Database (TFGD, http://ted.bti.cornell.edu/cgi-bin/TFGD/digital/home.cgi) were used to investigate expression patterns of putative SlHsp20 genes in different tissues of cultivated tomato (Solanum lycopersicum) and the wild relative (Solanum pimpinellifolium). Different tissues in cultivated tomato included leaves, roots, flower buds, fully opened flowers, 1 cm, 2 cm, 3 cm, mature green, breaker, and breaker+10 fruits. In the wild species (S. pimpinellifolium), nine tissues and organs were selected for analysis, including leaves, whole root, hypocotyl, cotyledons, flower buds 10 days before anthesis or younger, flowers at anthesis, 10 days post anthesis (DPA) fruit, 20 DPA fruit and breaker stage ripening fruit. Digital gene expression analysis of the putative SlHsp20 gene family was performed using software MultiExperiment Viewer (MeV) (Howe et al., 2010).
Expression Profile of SlHsp20 Genes under Different Stress Conditions
To get insight into the expression profiles of the SlHsp20 gene family under different environmental stresses, microarray analysis was performed. Whole genome microarray data for diverse environment stresses such as heat, drought, salt, Botrytis cinerea, and Tomato Spotted Wilt Virus (TSWV) was downloaded from database TFGD (http://ted.bti.cornell.edu/). The array platforms for microarray data included TOM2 oligo array and Affymetrix genome array. For TOM2 oligo array, the probe sets of the SlHsp20 genes were identified through BlastN analysis in the database “TOM2 oligo sequences.” For Affymetrix genome array, Probe Match tool in NetAffx Analysis Center (http://www.affymetrix.com) was used to obtain probe sequences. Average value was considered for SlHsp20 genes that had more than one probe set. The expression values of SlHsp20 genes that were up- or down-regulated more than two-fold with P < 0.05 were considered as differently expressed.
Results
Identification of Hsp20 Family Members in Tomato
Name search and HMM analysis showed a total of 42 candidate SlHsp20 genes, four of which were identified to contain incomplete Hsp20 domains. For convenience, the SlHsp20 genes were named according to their molecular weight in our study. Details on gene name, locus name, chromosome location, open reading frame (ORF) length, intron number, protein length, molecular weight, isoelectric point (pI), and instability index were listed in Table 1.
The four SlHsp20 genes with incomplete Hsp20 domain encoded truncated proteins (67–129 aa) and could be non- functional or pseudogenes. Therefore, these SlHsp20 genes were excluded in phylogenetic tree construction. Molecular weight of the remaining predicted SlHsp20 genes ranged from 15.2 to 49.3 kDa, except for SlHsp11.9 and SlHsp14.5 that were less than 15 kDa. Molecular weights of these SlHsp20 proteins had a large variation. Isoelectric points ranged from 4.53 (SlHsp9.0) to 9.8 (SlHsp23.8A), and protein length ranged from 67 (SlHsp7.8) to 441 aa (SlHsp49.3). The instability index indicates that 18 of the predicted SlHsp20 proteins were deemed to be stable proteins, while others were unstable.
Gene Structure of the SlHsp20 Genes
Structure and phases of introns/exons were determined by alignment of genomic DNA and full-length cDNA sequences of SlHsp20 genes (Supplementary Figure S1). This information was available on Sol Genomics Network (Supplementary Table S1). It was found that among the total 42 SlHsp20 genes, 13(30.95%) were noted to be intronless, while 22 genes (52.38%) had one intron, and only 7(16.67%) had two or more introns. Based on the number of introns, we divided the genes into three patterns (pattern one has no intron, pattern two has one intron, and pattern three has more than one) (Ouyang et al., 2009). It was evident that most SlHsp20 genes belonged to pattern 1 and 2. Additionally, a relatively short intron length was found in SlHsp20 proteins in tomato, which was similar to the results reported on sHsp20 proteins in rice (Sarkar et al., 2009).
Using the MEME tool, 10 putative conserved motifs (motif 1 to motif 10) in SlHsp20 gene family were identified (Supplementary Table S2). The lengths of these conserved motifs varied from 15 to 57 aa (P < 0.0001). Details of all the putative motifs are outlined in Table 2. Among the 10 motifs, motif 2 appeared in all putative SlHsp20 genes, except for SlHsp15.5. Based on the analysis of Pfam, the full sequences of motif 1, 2, 3, 4, 5, and 8 were corresponding to the region of conserved ACD. We also found that motif 1 was in the C-terminal regions, while motif 6 appeared in the N-terminal regions generally. In addition, motif 9 was also distributed in the C-terminal regions of several genes. Notably, four SlHsp20 genes (SlHsp11.9, SlHsp17.3A, SlHsp14.5, and SlHsp15.8) present similar patterns of motif distribution. Also, the same scenario was found in other four SlHsp20 genes (SlHsp17.6C, SlHsp17.7A, SlHsp17.6B, and SlHsp17.6A) on chromosome 6. These results suggest that these genes may have relatively high conservation. Nevertheless, the functions of these highly conserved amino acid motifs still remain elusive.
It was reported that highly conserved ACD domain might be associated with the formation of multimeric complexes that are crucial for the chaperone activity of Hsp20 genes (Waters et al., 1996; Sun et al., 2002). In the current paper, in line with a previous report (Waters et al., 1996), the highly conserved ACD in SlHsp20 proteins was divided into two parts (consensus I and consensus II) based on multiple sequence alignment (Figure 1). These two conserved regions were separated by a hydrophilic domain with variable length and characterized by residue Pro-X (14)-Gly-Val-Leu and Pro-X (14)-X-Val/Leu/Ile-Val/Leu/Ile, respectively (Caspers et al., 1995). Intriguingly, consensus I matched well with the motif 1 located on carboxyl terminal presumed by MEME.
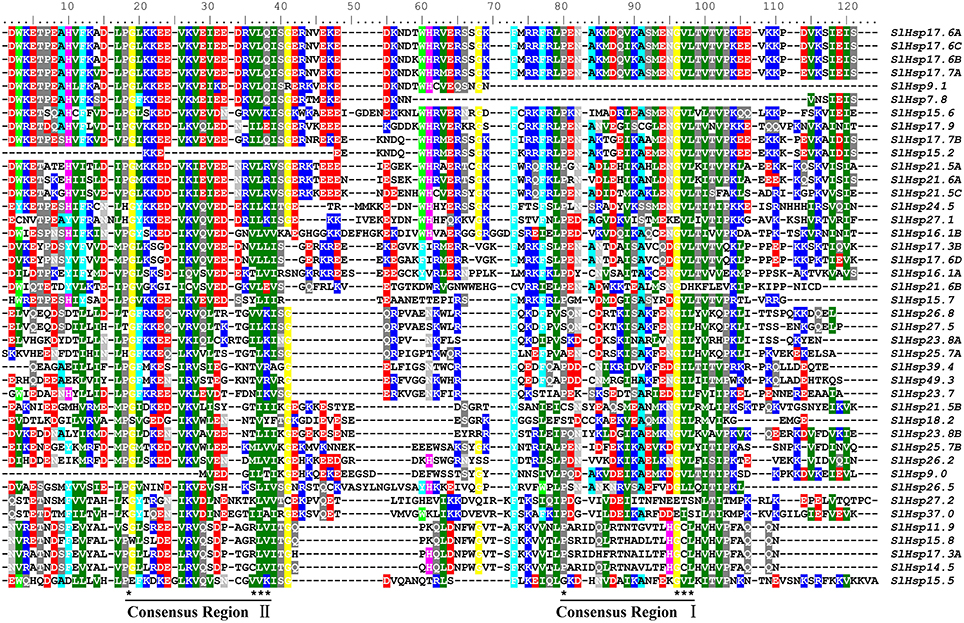
Figure 1. Multiple sequence alignment of crystallin domain of SlHsp20 proteins. Names of all the 42 members are listed on the right side of the figure. Conserved amino acid residues are indicated by color shading. Two consensus regions (consensus I and consensus II) are underlined at the bottom and the typical amino acid residues within these regions are indicated by asterisks.
Phylogenetic Analysis of SlHsp20 Gene Family
An unrooted N-J phylogenetic tree was constructed from a complete alignment of amino acid sequences of Hsp20 proteins in tomato and other seven plant species (Figure 2). All these Hsp20 proteins were grouped into 17 distinct subfamilies. However, the SlHsp20 proteins were distributed into 13 out of 17 subfamilies, including previously identified subfamilies like CI, CII, CIII, P, Px, ER, and MI (Waters et al., 1996), recently defined CV, CVI, CVII, CIX, and CXI subfamilies (Siddique et al., 2008; Sarkar et al., 2009) and a new subfamily, CXII, identified by in silico prediction of subcellular localization (Supplementary Table S3). Besides, we also found two orphan SlHsp20 genes in tomato that lack homologs genes in all seven organisms. The SlHsp20 genes from 13 subfamilies were distributed to a variety of cellular organelles: 29 were nucleo-cytoplasmic (C) SlHsp20 genes (9 subfamilies), 3 were endoplasmic reticulum (ER) genes, 2 were plastidial (P) genes, and 1 each for mitochondrial (M) and peroxisomal (Px) genes.
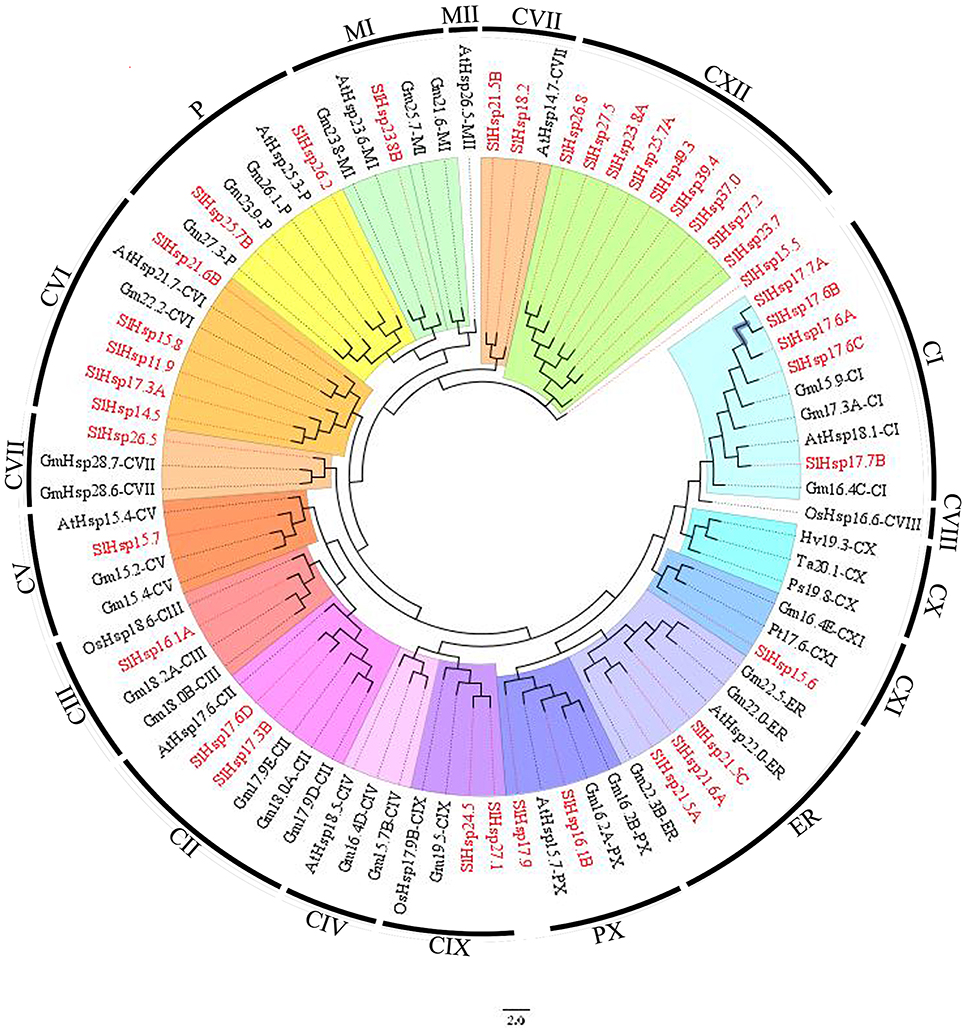
Figure 2. Phylogenetic relationship of Hsp20s of tomato with diverse plant species. The phylogenetic tree of Hsp20 proteins was constructed by Neighbor-Joining method using MEGA 7.0 software from the following species: Sl, Solanum lycopersicum; At, Arabidopsis thaliana; Gm, Glycine max; Os, Oryza sativa; Ps, Pseudoroegneria spicata; Hv, Hordeum vulgare; Ta, Triticum aestivum; Pt, Populus tremula. The putative Hsp20 genes were divided into 17 subfamilies based on their in silico prediction of subcellular localization. The SlHsp20 genes were highlighted in red. C, cytoplasmic/nuclear; ER, endoplasmic reticulum; P, plastid; Px, peroxisome; M, mitochondria.
Notably, all three SlHsp20 genes belonging to ER subfamily have a signal peptide in the N-terminal region, which was consistent with the result showed by Lopes-Caitar et al. (2013) (Table 1). The signal peptides were reported to play a positive role in facilitating the process of protein synthesis via guiding the proteins into rough endoplasmic reticulum (Bauvois, 2012). Moreover, eight SlHsp20 genes, one from CVI subfamily and seven from CXII, had a transmembrane domain in the C-terminal region (Table 1).
We also found a close relationship between intron pattern and phylogenetic classification (Supplementary Figure S1). The result showed that the CI, CII, and ER subfamilies lacked introns. All the CVII members had more than one intron, indicating a particular phylogenetic status (Ouyang et al., 2009). The CIII, CV, CVI, CXI, MI, and Px subfamilies, together with most members of the CXII subfamily, had one intron, which may indicate a close phylogenetic relationship. In addition, gene structure may provide clues for evolutionary relationship of SlHsp20 family.
Chromosomal Localization and Gene Duplication
Out of the 42 predicted SlHsp20 genes, 41 are randomly distributed across the 12 tomato chromosomes, except for the SlHsp11.9 (Figure 3). Majority of the SlHsp20 genes were located on the distal ends of the chromosomes and mainly on the lower arms. A maximum number of eight predicted SlHsp20 genes scattered in three clusters, were present on chromosome 1.
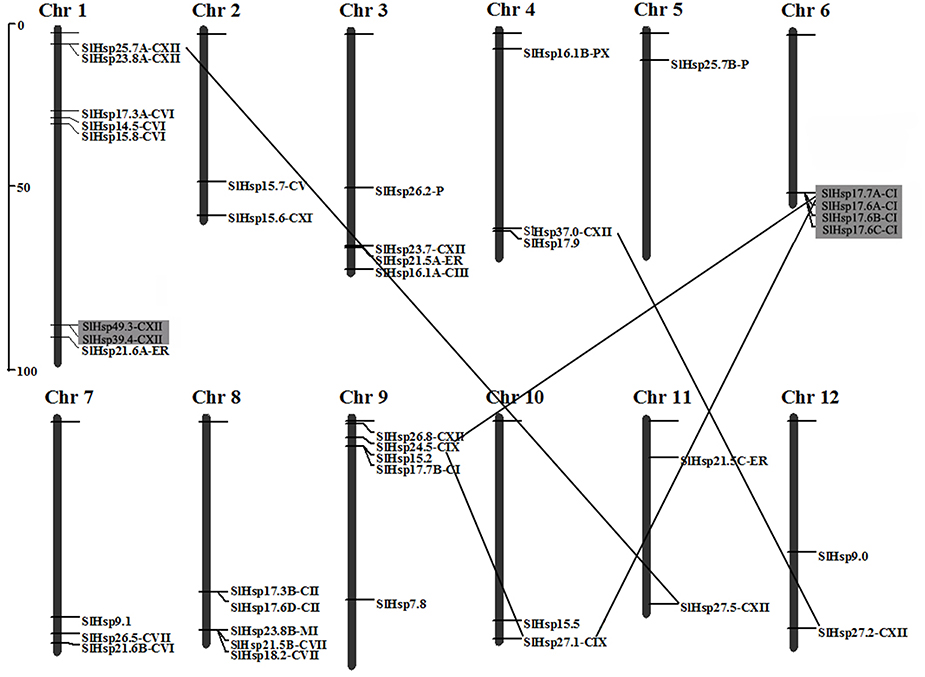
Figure 3. Location and duplications of paralogous SlHsp20 gene candidates on tomato chromosomes. Chromosome numbers are shown at the top of each bar. Predicted tandem duplicated genes are indicated by gray rectangles. The SlHsp20 genes present on duplicated chromosomal segments are connected by black lines. The scale presented on the left indicates chromosome sizes in megabases (Mb).
We further performed chromosome mapping to determine the gene duplication of SlHsp20 genes on the 12 tomato chromosomes. As shown in Figure 3, two groups of SlHsp20 genes (SlHsp49.3/SlHsp39.4 and SlHsp17.7A/SlHsp17.6A/SlHsp17.6B/SlHsp17.6C) can be identified as tandem duplication genes (Supplementary Table S4). One group (SlHsp49.3 and SlHsp39.4) was from the CXII subfamily and located on chromosome 1. The other (SlHsp17.7A, SlHsp17.6A, SlHsp17.6B, and SlHsp17.6C) was from one branch of CI subfamily and juxtaposed compactly on chromosome 6, implying that the high density of SlHsp20 genes on this chromosome was mainly due to the tandem duplication events. The chromosome location of tandemed genes showed that these four pairs of SlHsp20 genes (SlHsp49.3/SlHsp39.4, SlHsp17.7A/SlHsp17.6A, SlHsp17.6A/SlHsp17.6B, SlHsp17.6B/ SlHsp17.6C) was intervened by no more than one gene and was close in distance on the chromosomes (approximately 4.6, 3.8, 8.2, and 3.9 kb) (Supplementary Table S5).
On the other hand, three segmental duplication groups were found to scatter in seven chromosomes (Figure 3; Supplementary Table S6). A duplicated segment of the SlHsp37.0 region on the distal part of chromosome 4 was present on the same location of chromosome 12, where SlHsp27.2 was located. SlHsp25.7A on chromosome 1 also showed synteny to SlHsp27.5 localized on a duplicated segment of chromosome 11. In addition, three SlHsp20 genes (SlHsp17.7A, SlHsp24.5, and SlHsp27.1) regions showed segmental duplication and were present on chromosome 6, 9, and 10, respectively.
Genome-Wide Expression Analysis of SlHsp20 Genes
In silico expression patterns of the putative SlHsp20 genes at different tissues and development stages of tomato cultivar Heinz and the wild species S. pimpinellifolium were analyzed (Figure 4). It showed that most of the genes (83.3%) were expressed in at least one stage (tissue) from at least one genotype. Fourteen genes (SlHsp17.7A, SlHsp17.6B, SlHsp17.6C, SlHsp24.5, SlHsp18.2, SlHsp16.1A, SlHsp17.7B, SlHsp17.6A, SlHsp25.7B, SlHsp15.2, SlHsp21.6A, SlHsp17.6D, SlHsp16.1B, SlHsp23.8B) were expressed constitutively in all the stages analyzed, whereas the transcripts of 12 genes (SlHsp23.8A, SlHsp17.3A, SlHsp17.9, SlHsp9.1, SlHsp39.4, SlHsp37.0, SlHsp21.5B, SlHsp11.9, SlHsp14.5, SlHsp15.8, SlHsp7.8, and SlHsp15.5) were at almost undetectable levels. Among these genes, SlHsp17.6B had the highest expression level in the 30 DPA fruit.
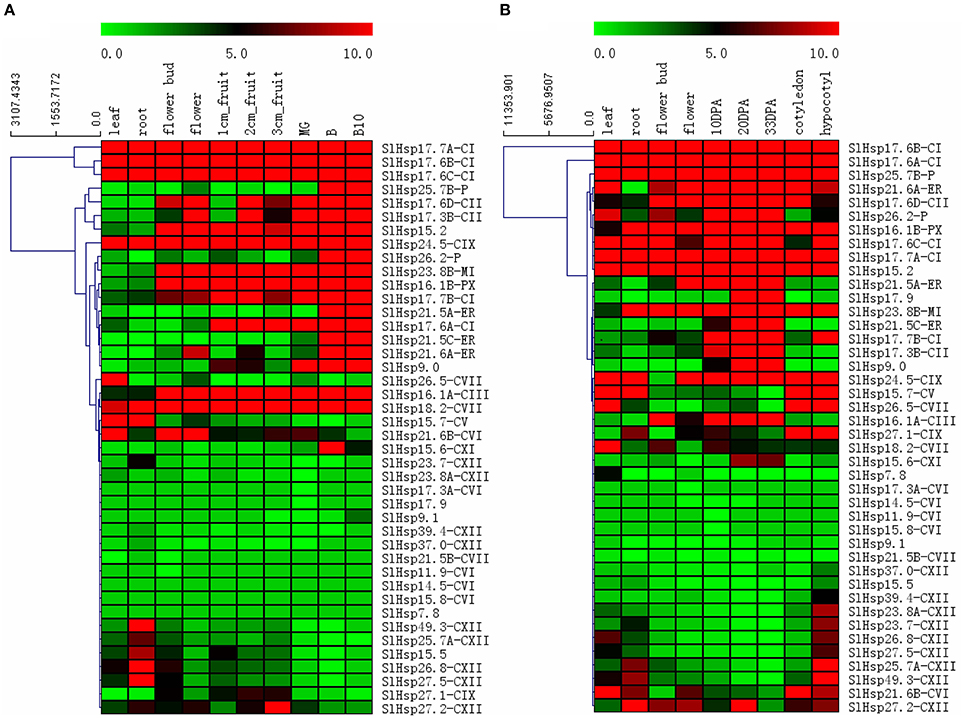
Figure 4. Heat map of the expression profiles of SlHsp20 genes in cultivated tomato cultivar Heniz (A) and the wild species S. pimpinellifolium (B). (A) MG-mature green fruit; B-breaker fruit; B10-breaker+10 fruit. (B) DPA-days post anthesis. Cluster dendrogram is shown on the left side of heat map. Heat maps are presented in green/black/red colors that represent low/medium/high expression, respectively.
When the expression patterns of SlHsp20 genes in vegetative organs (leaf and root) and reproductive organs (flower bud and flower) were compared between the 2 tomato genotype, 17 genes were either highly-induced (3) or barely expressed (14) (Figure 4). Conversely, two genes (SlHsp25.7B and SlHsp17.6A) displayed significantly differential expression in the various genotypes. Further, seven genes exhibited varied expression in vegetative and reproductive organs of tomato cultivar Heinz, while only two of them expressed differentially in S. pimpinellifolium. Notably, expression of seven genes was restricted to the leaf (SlHsp26.5) and root (SlHsp23.7, SlHsp49.3, SlHsp25.7, SlHsp26.8, SlHsp27.5, and SlHsp15.5) in Heniz, and only one gene was noted to root (SlHsp7.8) in S. pimpinellifolium. It indicates that these genes are regulated in a tissue-specific manner.
Expression levels of SlHsp20 genes at breaker stage fruits were higher than that in other development stages in both genotypes. In tomato cultivar Heniz, expression of several SlHsp20 genes (SlHsp25.7B, SlHsp26.2, SlHsp21.5A, SlHsp21.5C, and SlHsp15.6) was not almost detected in young tomato fruits (1 cm-, 2 cm-, 3 cm-, and MG fruit), but a distinct expression was observed in the breaker fruits (Figure 4A). In S. pimpinellifolium, two SlHsp20 genes, SlHsp17.9, and SlHsp15.6, were only expressed in 20 DPA and 33 DPA fruits (Figure 4B). Furthermore, expression of three SlHsp20 genes (SlHsp23.8A, SlHsp23.7, and SlHsp39.4) derived from CXII subfamily showed tissue specificity in hypocotyl. Besides, by analyzing the expression profiles of tandem and segmental duplication of SlHsp20 genes in two genotypes, we found that two groups of tandemly SlHsp20 genes (SlHsp49.3/ SlHsp39.4; SlHsp17.7A/SlHsp17.6A/SlHsp17.6B/SlHsp17.6C) displayed a more similar expression pattern, while difference in expression was observed for SlHsp20 genes (SlHsp27.1/SlHsp17.7A/SlHsp24.5; SlHsp37.0/SlHsp27.2) in segmental duplication regions.
Expression Profiles of SlHsp20 Genes Induced by Different Biotic and Abiotic Stresses
To further explore the expression profiles of SlHsp20 genes under various abiotic and biotic stresses, microarray analysis were performed (Figure 5). In the present paper, five tomato microarray data sets, belonging to two array platforms (TOM2 oligo array and Affymetrix genome array), were obtained from Tomato Functional Genomics Database (TFGD, http://ted.bti.cornell.edu/). A total of 24 probes (57%) corresponding to SlHsp20 genes were identified, while five probes (LE17D07, LE26P10, LE26F10, LE13N06, Les.4004.1.S1_a_at) showed cross-reactivity with 12 SlHsp20 genes (Supplementary Table S7).
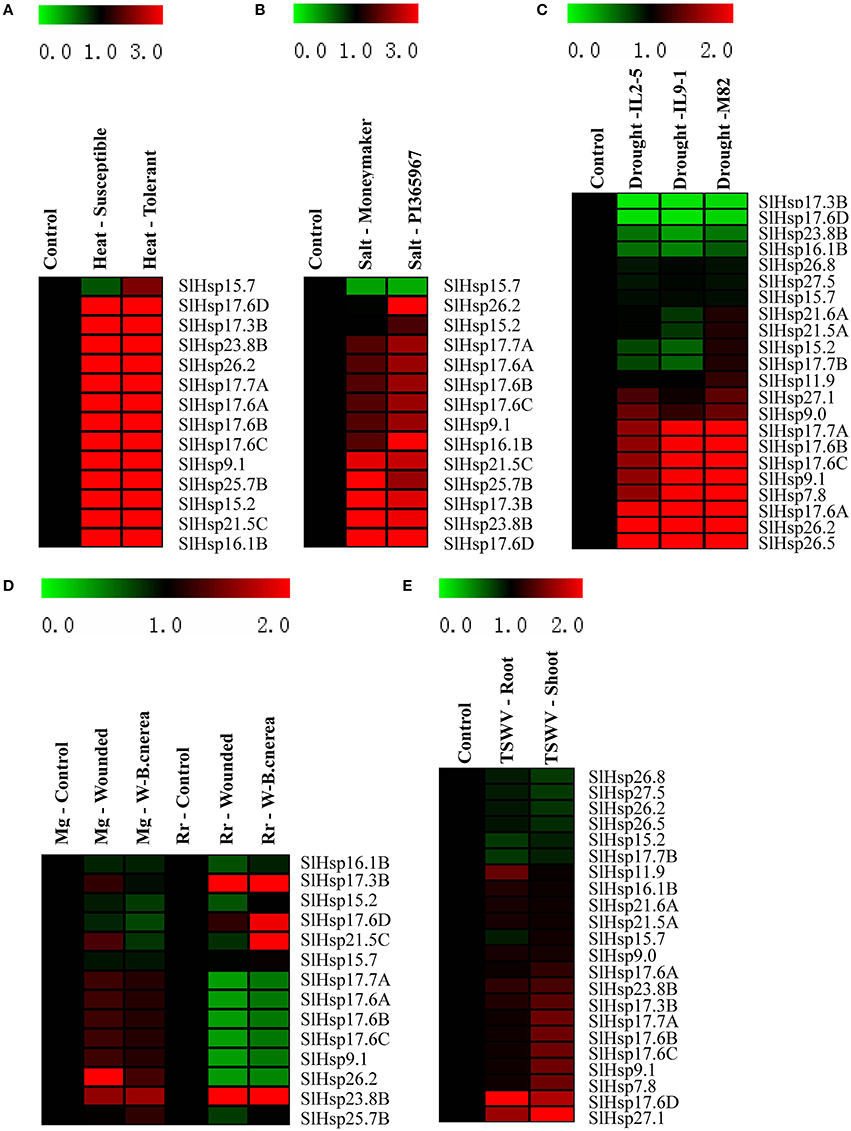
Figure 5. Expression profiles of SlHsp20 genes under various biotic and abiotic stresses conditions. Blocks with colors represent decreased (green) or increased (red) transcript levels relative to the respective control. (A) Expression profiles of SlHsp20 genes in tolerant and susceptible tomatoes under heat stress condition. (B) Expression profiles of SlHsp20 genes under salt stress in a wild tomato genotype “PI365967” (salt-tolerant) and cultivated tomato var. moneymaker (salt-sensitive). (C) Expression profiles of SlHsp20 genes under drought stress condition in two drought-tolerant lines (IL2-5 and IL9-1) and a drought-sensitive cultivar (M82). (D) Expression profiles of SlHsp20 genes infected by wound and wound-inoculated with Botrytis cinerea in mature green (Mg) and red fruits (Rr). (E) Expression profiles of SlHsp20 genes in tomato leaves and roots infected by tomato spotted wilt virus (TSWV).
Microarray-based expression analysis of tomato under various abiotic stresses revealed that expression of most of the SlHsp20 genes were highly variable (Figures 5A–C). Expression of 13 of all tested SlHsp20 genes, especially SlHsp25.7B, SlHsp15.2, SlHsp21.5C, and SlHsp16.1B, was drastically enhanced in resistant and susceptible tomato plants under high temperature condition, except for SlHsp15.7 that was down-regulated in susceptible plants in response to heat stress (Figure 5A). Half of the analyzed SlHsp20 genes in susceptible plants showed a higher expression level than those in tolerant plants. Under salt treatment condition, 12 SlHsp20 genes displayed highly elevated expression, whereas SlHsp15.7 was shown to be significantly down-regulated (Figure 5B). PI365967, a more salt-tolerant tomato genotype, showed relatively more responsive genes compared to tomato cultivar Moneymaker. Under drought stress condition, transcript levels of 10 and 4 SlHsp20 genes were up-regulated and down-regulated in drought-tolerant tomato lines (IL2-5 and IL9-1) and drought-sensitive cultivar M82, respectively (Figure 5C). Among these genes, SlHsp26.5 showed a drastic enhancement of the transcript level (more than 16 fold) in three tested tomato genotypes. The expression of SlHsp26.2 was dramatically up-regulated in M82 (a drought-sensitive cultivar), which was much higher (four times) than that in IL2-5 and IL9-1.
Invoked by wound and the invasion of B. cinerea, transcript levels of three genes (SlHsp15.7, SlHsp23.8B, and SlHsp16.1B) were remained unaltered, up- and down-regulated levels, respectively, in all tested samples (Figure 5D). In mature green fruit, most of the SlHsp20 genes displayed a stronger expression in wounded fruits than that in fruits of wound-inoculated with B. cinerea. In red ripe fruit, however, the expression patterns of SlHsp20 genes showed a reverse pattern between the wounded and wound-inoculated with B. cinerea fruits. Interestingly, seven genes (SlHsp17.6D, SlHsp17.7A, SlHsp17.6A, SlHsp17.6B, SlHsp17.6C, SlHsp9.1, and SlHsp26.2) displayed differential expression between mature green- and red ripe fruits. In addition, under TSWV infection condition, three SlHsp20 genes (SlHsp11.9, SlHsp17.6D, and SlHsp27.1) were up-regulated in tomato roots, three genes (SlHsp15.2, SlHsp17.7B, and SlHsp15.7) were down-regulated, and expression of the remaining genes remained unchanged (Figure 5E). In shoots of tomato, almost half SlHsp20 genes were enhanced, six genes were reduced and expression levels of the remaining six genes were unaltered.
Discussion
Identification and Phylogentic Relationship of SlHsp20 Gene Family
Using in silico methods to search for Hsp20 genes in the S. lycopersicum genome, at least 42 putative SlHsp20 genes were identified (Table 1). Among them, four predicted SlHsp20 genes were excluded in the phylogenetic tree construction due to incomplete Hsp20 domain. Previously, Hsp20 gene family in Arabidopsis was categorized into 12 subfamilies (CI, CII, CIII, CIV, CV, CVI, CVII, MI, MII, P, ER, and Px) (Scharf et al., 2001; Siddique et al., 2008). Likewise, four novel nucleocytoplasmic subfamilies (CVIII, CIX, CX, and CXI) were also reported on OsHsp20 genes in rice (Sarkar et al., 2009). In the present paper, to reveal phylogenetic relationship of Hsp20 genes, Hsp20 genes from seven plant species, together with Hsp20 genes from tomato, were used to construct phylogenetic tree (Figure 2). The results showed that Hsp20 genes from the tested plant species could be grouped into 17 subfamilies, according to the method described previously (Scharf et al., 2001; Siddique et al., 2008; Sarkar et al., 2009). The Hsp20 genes from tomato were grouped into 13 out of 17 subfamilies. A novel subfamily, CXII, was identified using in silico localization prediction, which was composed of 9 Hsp20 genes from tomato (Supplementary Table S3). No SlHsp20 genes were grouped into the remaining four subfamilies (CIV, CVIII, CX, and MII subfamilies). Previous study showed that CIV and MII subfamilies may play an important role in response to various stress conditions and were developmentally regulated (Siddique et al., 2008). Members of CVIII subfamily could be heat-induced and CX subfamily might be involved in specific housekeeping functions under normal growth conditions (Sarkar et al., 2009). Similarly, several subfamilies of the Hsp20 genes in pepper also lacks, including CIV, CV, CVIII, CIX, CX, and CXI subfamilies. (Guo et al., 2015). Furthermore, the CIV and CVII subfamilies in rice Hsp20 family were absent (Sarkar et al., 2009). Thus, we were tempting to speculate that gene gain and gene loss events were occurred widely in plant species. The lack of subfamilies exists not only in tomato, but also in other species. It also revealed a wide range of genetic diversity in dicotyledons and monocotyledons.
Furthermore, we also found that most of Hsp20 genes (69.0%) were clustered into nine nucleocytoplasmic subfamilies, which also had been described in Arabidopsis and rice (Scharf et al., 2001; Sarkar et al., 2009). Among these subfamilies, CXII was the largest subfamily with nine members. Therefore, we speculated that cytoplasm, as a site mainly for proteins to be synthesized, could be the primary place for Hsp20 interacting with denatured proteins to avoid them from inappropriate aggregation and degradation (Lopes-Caitar et al., 2013). In addition, most subfamilies included Hsp20 genes from multiple plant species and formed a mixture of groups, suggested that diversification of SlHsp20 subfamilies predated the divergence of these species from the ancestral species.
Organization of SlHsp20 Genes
It was reported that gene organization played a vital role in the evolution of multigene family (Xu et al., 2012). Our results showed that SlHsp20 genes of CI, CII, and ER subfamilies had no intron (pattern one) (Supplementary Figure S1). Members of CIII, CV, CIX, MI, and PX subfamilies as well as most members of CXII subfamily had one intron (pattern two). Furthermore, these SlHsp20 genes within the same subfamily shared a similar motif arrangement (Supplementary Table S2). This correlation between motif arrangement, intron numbers and phylogeny can be served as an additional support to their classification.
The results also showed that most of SlHsp20 genes had no intron or one intron and the length of introns of SlHsp20 genes with one intron is relatively short. This is concurrent with the result reported previously by the researcher that plants were prone to retain more genes with no intron or a short intron (Mattick and Gagen, 2001). In addition, the instability index of most proteins was equal or greater than 40, signifying that SlHsp20 proteins can be identified as unstable proteins (Table 1). The instability is also considered as a common trait of stress-activated proteins and thus sheds a brilliant light on the rapid induction of the Hsp20 genes (Rao et al., 2008).
Evolution of the SlHsp20 Gene Family
Previous research reported that Hsp20 proteins were ubiquitous from single-celled creatures including bacteria to higher organisms like human (Kim et al., 1998; Waters et al., 2008; Li et al., 2009). It implied that Hsp20 proteins may have evolved in early stages of the life's history which predated to the divergence of the three domains of life (Eukarya, Bacteria, and Archaea). However, the formation of individual Hsp20 subfamilies was various. There were no Hsp20 subfamilies but only cytosolic sHsps in the green algae and then CI, CII, and P subfamilies appeared in mosses (Waters and Vierling, 1999a,b). The process generated gene families attribute to gene duplication, gene loss, and recombination (including gene conversion) (Nei and Rooney, 2005; Flagel and Wendel, 2009). The sHsps might had undergone duplication events early in the history of land plant (Waters, 2013).
Gene duplication was reported as one of the primary forces that drive the evolution processes of genetic systems and genomes (Moore and Purugganan, 2003). In this paper, chromosome mapping showed that 41 SlHsp20 genes were located unevenly on 12 tomato chromosomes (Figure 3). The localization of most of the SlHsp20 genes was on the terminal regions of the chromosomes, which might contribute to the occurrence of duplication events in tomato Hsp20 gene family.
In our study, a total of 12 SlHsp20 genes were demonstrated to be involved in gene duplication, including tandem duplication and segmental duplication (Figure 3). Two tandem duplication events (SlHsp49.3/SlHsp39.4 and SlHsp17.7A/SlHsp17.6A/SlHsp17.6B/ SlHsp17.6C) and three segmental duplication events (SlHsp25.7A/SlHsp27.5, SlHsp17.7A/ SlHsp24.5/SlHsp27.1, and SlHsp37.0/SlHsp27.2) were observed in CI, CIX, and CXII subfamilies. Intriguingly, SlHsp17.7A on chromosome 6 was shown to participate in both tandem and segmental events. It shared a duplicated region with SlHsp27.1 at the similar position on chromosome 10 and SlHsp24.5 on the upper arm of chromosome 9, while SlHsp27.1 and SlHsp24.5 had no tandem duplicated genes in their surrounding region. Comparative analysis revealed that sequence similarity between SlHsp17.7A and SlHsp27.1 was lower than that between SlHsp17.7A and SlHsp17.6A, suggesting that segmental duplication event predated the tandem duplication event in the SlHsp17.7A cluster, and the tandemly organized genes close to SlHsp27.1 might be lost after the segmental duplication event (Supplementary Tables S5, S6). Similar scenario was observed for SlHsp17.7A and SlHsp24.5. Together, these results indicated that both tandem duplication and segmental duplication made significant contributions to the expansion of the SlHsp20 gene family in tomato.
Expression Patterns of SlHsp20 Genes in Different Tissues
Based on RNA-Seq atlas, a spatio-temporal regulation of SlHsp20 gene family was observed in various tissues and development stages. Under normal growth conditions, a high or preferential expression of 11 SlHsp20 genes was found, which showed tissue- and development-specific expression in leaf, root, hypocotyl, and breaker fruit (Figure 4). All these tissue- and development-preferential expressed genes may play a critical role in growth and development of tomato and their functions still deserve further investigation. In addition, the expression behavior of some SlHsp20 genes differed in various tissues and development stages, indicating that the SlHsp20 proteins may play diverse functional roles. We also found that four genes (SlHsp17.7A, SlHsp17.6B, SlHsp17.6C, and SlHsp24.5) were highly expressed in all the investigated tissues, implying that they might be implicated in specific housekeeping activity of tomato cell under normal growth conditions. In vegetative and reproductive organs, two SlHsp20 genes (SlHsp25.7B and SlHsp17.6A) displayed differential expression levels between cultivated tomato and the wild relative S. pimpinellifolium, which indicated that inter-species divergence of gene expression was occurred and it might lead to functional specialization.
Expression Patterns of SlHsp20 Genes under Abiotic and Biotic Stresses
Under various stress conditions, it's evident that the SlHsp20 genes were induced to a larger extent when tomato suffered from abiotic stresses, including heat, salt, and drought treatments (Figure 5). Furthermore, we found that the heat stress inducibility of SlHsp20 genes in susceptible plants was stronger than that in tolerant plants, which also had been demonstrated in CaHsp20 genes (Guo et al., 2015). This finding indicated that a more efficient mechanism might have been built in the tolerant plants so that fewer Hsp20 genes were sufficient to reduce the damage from heat shock.
Previous research had reported that the duplicated genes were easier for increasing the diversity of gene expression than single-copy genes (Gu et al., 2004). Here, we found that expression patterns of two groups of the tandemly duplicated genes were highly similar, which reflected that these SlHsp20 genes might share similar induction mechanisms and network (Ouyang et al., 2009). Actually, compared with tandem duplications, SlHsp20 genes in segmental duplicated group showed a more differential expression behavior. For example, the different expression between SlHsp37.0-CXII and SlHsp27.2-CXII suggested that segmentally duplicated genes may also exhibit divergent expression patterns (Waters, 2013). Earlier study revealed that distantly related duplicate genes may exist functional redundancy and have more chances to acclimatize than the single-copy genes (Gu et al., 2003). Thus, the duplicated SlHsp20 genes might go through crucial diversification after duplication events, which eventually caused neo-functionalization (Ouyang et al., 2009). It was also reported as a means for the retention of those duplicated genes in a genome (Force et al., 1999).
Conclusions
In the current study, 42 putative SlHsp20 genes were identified in tomato. Subsequently, characterization of SlHsp20 genes was performed through integration of comprehensive sequence, genome organization and expression profile analysis among different tissues and under different stresses (heat, drought, salt, TSWV, and B. cinerea) by using RNA-seq and microarray atlas. This study provided a comprehensive understanding of the SlHsp20 gene family in tomato and made a basis for working out the functional roles of the Hsp20 genes in the Solanaceae family in the future.
Author Contributions
Conceived and designed the experiments: HW and YY. Performed the experiments: JY, YC, MR, QY, RW, ZL, GZ, and ZY. Analyzed the data: JY, YC, and KF. Wrote the paper: JY and YC. All authors have read and approved the manuscript.
Conflict of Interest Statement
The authors declare that the research was conducted in the absence of any commercial or financial relationships that could be construed as a potential conflict of interest.
The reviewer CY and handling Editor declared their shared affiliation, and the handling Editor states that the process nevertheless met the standards of a fair and objective review.
Acknowledgments
Research is supported by the National Natural Science Foundation of China (31301774, 31272156, and 31071800), Zhejiang Provincial Natural Science Foundation of China (Q15C150010) and Young Talent Cultivation Project of Zhejiang Academy of Agricultural Sciences (2015R23R08E07, 2015R23R08E09), State Key Laboratory Breeding Base for Zhejiang Sustainable Pest and Disease Control (No. 2010DS700124-KF1518), Public Agricultural Technology Research in Zhejiang (2016C32101, 2015C32049), and Technological System of Ordinary Vegetable Industry (CARS-25-G-16).
Supplementary Material
The Supplementary Material for this article can be found online at: http://journal.frontiersin.org/article/10.3389/fpls.2016.01215
Supplementary Figure S1. Intron/exon configurations of SlHsp20 genes. The numbers (0, 1, 2) represented introns phases, which indicated three different splicing patterns.
Supplementary Table S1. Genomic sequences, coding sequences and amino acid sequences of SlHsp20 genes.
Supplementary Table S2. Organization of putative motifs in SlHsp20 proteins. Ten putative motifs are represented by numbered color boxes. Names of all the proteins were indicated on the left side.
Supplementary Table S3. Predicted subcellular location of SlHsp20 genes.
Supplementary Table S4. Homology matrixes of predicted tandem SlHsp20 gene sequences.
Supplementary Table S5. Tandemly duplicated SlHsp20 genes.
Supplementary Table S6. SlHsp20 genes localized on duplicated segments of the tomato genome.
Supplementary Table S7. Information of probe sets used for microarray expression analysis.
References
Arce, D. P., Krsticevic, F. J., Bertolaccini, M. R., Ezpeleta, J., Ponce, S. D., and Tapia, E. (2015). “Analysis of small heat shock protein gene family expression (RNA-Seq) during the tomato fruit maturation,” in IFMBE Proceedings, ed A. Braidot (Berlin: Springer International Publishing), 679–682.
Basha, E., O'Neill, H., and Vierling, E. (2012). Small heat shock proteins and α-crystallins: dynamic proteins with flexible functions. Trends Biochem. Sci. 37, 106–117. doi: 10.1016/j.tibs.2011.11.005
Bauvois, B. (2012). New facets of matrix metalloproteinases MMP-2 and MMP-9 as cell surface transducers: outside-in signaling and relationship to tumor progression. Biochim. Biophys. Acta Rev. Cancer 1825, 29–36. doi: 10.1016/j.bbcan.2011.10.001
Botër, M., Amigues, B., Peart, J., Breuer, C., Kadota, Y., Casais, C., et al. (2007). Structural and functional analysis of SGT1 reveals that its interaction with HSP90 is required for the accumulation of Rx, an R protein involved in plant immunity. Plant Cell 19, 3791–3804. doi: 10.1105/tpc.107.050427
Carper, S. W., Duffy, J. J., and Gerner, E. W. (1987). Heat shock proteins in thermotolerance and other cellular processes. Cancer Res. 47, 5249–5255.
Cashikar, A. G., Duennwald, M., and Lindquist, S. L. (2006). A chaperone pathway in protein disaggregation. J. Biol. Chem. 281, 8996. doi: 10.1074/jbc.M502854200
Caspers, G. J., Leunissen, J. A. M., and de Jong, W. W. (1995). The expanding small heat-shock protein family, and structure predictions of the conserved “α-crystallin domain.” J. Mol. Evol. 40, 238–248. doi: 10.1007/BF00163229
Cramer, G. R., Urano, K., Delrot, S., Pezzotti, M., and Shinozaki, K. (2011). Effects of abiotic stress on plants: a systems biology perspective. BMC Plant Biol. 11:163. doi: 10.1186/1471-2229-11-163
de Jong, W. W., Caspers, G. J., and Leunissen, J. A. M. (1998). Genealogy of the α-crystallin-small heat-shock protein superfamily. Int. J. Biol. Macromol. 22, 151–162. doi: 10.1016/S0141-8130(98)00013-0
Flagel, L. E., and Wendel, J. F. (2009). Gene duplication and evolutionary novelty in plants. New Phytol. 183, 557–564. doi: 10.1111/j.1469-8137.2009.02923.x
Force, A., Lynch, M., Pickett, F. B., Amores, A., Yan, Y. L., and Postlethwait, J. (1999). Preservation of duplicate genes by complementary, degenerative mutations. Genetics 151, 1531–1545.
Goyal, R. K., Kumar, V., Shukla, V., Mattoo, R., Liu, Y., Chung, S. H., et al. (2012). Features of a unique intronless cluster of class I small heat shock protein genes in tandem with box C/D snoRNA genes on chromosome 6 in tomato (Solanum lycopersicum). Planta 235, 453–471. doi: 10.1007/s00425-011-1518-5
Gu, Z., Rifkin, S. A., White, K. P., and Li, W. H. (2004). Duplicate genes increase gene expression diversity within and between species. Nat. Genet. 36, 577–579. doi: 10.1038/ng1355
Gu, Z., Steinmetz, L. M., Gu, X., Scharfe, C., Davis, R. W., and Li, W. H. (2003). Role of duplicate genes in genetic robustness against null mutations. Nature 421, 63–66. doi: 10.1038/nature01198
Guo, M., Liu, J. H., Lu, J. P., Zhai, Y. F., Wang, H., Gong, Z. H., et al. (2015). Genome-wide analysis of the CaHsp20 gene family in pepper: comprehensive sequence and expression profile analysis under heat stress. Front. Plant Sci. 6:806. doi: 10.3389/fpls.2015.00806
Howe, E., Holton, K., Nair, S., Schlauch, D., Sinha, R., and Quackenbush, J. (2010). “Mev: multiexperiment viewer,” in Biomedical Informatics for Cancer Research, ed M. F. Ochs (New York, NY: Springer US), 267–277.
Hu, B., Jin, J., Guo, A. Y., Zhang, H., Luo, J., and Gao, G. (2015). GSDS 2.0: an upgraded gene feature visualization server. Bioinformatics 31, 1296–1297. doi: 10.1093/bioinformatics/btu817
Huang, S., Gao, Y., Liu, J., Peng, X., Niu, X., Fei, Z., et al. (2012). Genome-wide analysis of WRKY transcription factors in Solanum lycopersicum. Mol. Genet. Genomics 287, 495–513. doi: 10.1007/s00438-012-0696-6
Huther, C. M., Ramm, A., Rombaldi, C. V., and Bacarin, M. A. (2013). Physiological response to heat stress of tomato ‘Micro-Tom’ plants expressing high and low levels of mitochondrial sHSP23. 6 protein. Plant Growth Regul. 70, 175–185. doi: 10.1007/s10725-013-9790-y
Kim, K. K., Kim, R., and Kim, S. H. (1998). Crystal structure of a small heat-shock protein. Nature 394, 595–599. doi: 10.1038/29106
Kumar, S., Stecher, G., and Tamura, K. (2016). MEGA7: molecular evolutionary genetics analysis version 7.0 for bigger datasets. Mol. Biol. Evol. 33, 1870–1874. doi: 10.1093/molbev/msw054
Lee, G. J., and Vierling, E. (2000). A small heat shock protein cooperates with heat shock protein 70 systems to reactivate a heat-denatured protein. Plant Physiol. 122, 189–198. doi: 10.1104/pp.122.1.189
Li, X., Cheng, Y., Ma, W., Zhao, Y., Jiang, H., and Zhang, M. (2010). Identification and characterization of NBS-encoding disease resistance genes in Lotus japonicus. Plant Syst. Evol. 289, 101–110. doi: 10.1007/s00606-010-0331-0
Li, Z. W., Li, X., Yu, Q. Y., Xiang, Z. H., Kishino, H., and Zhang, Z. (2009). The small heat shock protein (sHSP) genes in the silkworm, Bombyx mori, and comparative analysis with other insect sHSP genes. BMC Evol. Biol. 9:215. doi: 10.1186/1471-2148-9-215
Lindquist, S., and Craig, E. A. (1988). The heat-shock proteins. Annu. Rev. Genet. 22, 631–677. doi: 10.1146/annurev.ge.22.120188.003215
Liu, R. H., and Meng, J. L. (2003). MapDraw: a microsoft excel macro for drawing genetic linkage maps based on given genetic linkage data. Hereditas (Beijing) 25, 317–321. doi: 10.3321/j.issn:0253-9772.2003.03.019
Lopes-Caitar, V. S., de Carvalho, M. C., Darben, L. M., Kuwahara, M. K., Nepomuceno, A. L., Dias, W. P., et al. (2013). Genome-wide analysis of the Hsp20 gene family in soybean: comprehensive sequence, genomic organization and expression profile analysis under abiotic and biotic stresses. BMC genomics 14:577. doi: 10.1186/1471-2164-14-577
Mahesh, U., Mamidala, P., Rapolu, S., Aragao, F. J., Souza, M. T., Rao, P. J. M., et al. (2013). Constitutive overexpression of small HSP24.4 gene in transgenic tomato conferring tolerance to high-temperature stress. Mol. Breeding 32, 687–697. doi: 10.1007/s11032-013-9901-5
Mattick, J. S., and Gagen, M. J. (2001). The evolution of controlled multitasked gene networks: the role of introns and other noncoding RNAs in the development of complex organisms. Mol. Biol. Evol. 18, 1611–1630. doi: 10.1093/oxfordjournals.molbev.a003951
Moore, R. C., and Purugganan, M. D. (2003). The early stages of duplicate gene evolution. Proc. Natl. Acad. Sci. U.S.A. 100, 15682–15687. doi: 10.1073/pnas.2535513100
Nautiyal, P. C., and Shono, M. (2010). Analysis of the role of mitochondrial and endoplasmic reticulum localized small heat shock proteins in tomato. Biol. Plant 54, 715–719. doi: 10.1007/s10535-010-0127-7
Nei, M., and Rooney, A. P. (2005). Concerted and birth-and-death evolution of multigene families. Annu. Rev. Genet. 39, 121–152. doi: 10.1146/annurev.genet.39.073003.112240
Neta-Sharir, I., Isaacson, T., Lurie, S., and Weiss, D. (2005). Dual role for tomato heat shock protein 21: protecting photosystem II from oxidative stress and promoting color changes during fruit maturation. Plant Cell 17, 1829–1838. doi: 10.1105/tpc.105.031914
Ouyang, Y., Chen, J., Xie, W., Wang, L., and Zhang, Q. (2009). Comprehensive sequence and expression profile analysis of Hsp20 gene family in rice. Plant Mol. Biol. 70, 341–357. doi: 10.1007/s11103-009-9477-y
Rao, P. K., Roxas, B. A., and Li, Q. (2008). Determination of global protein turnover in stressed mycobacterium cells using hybrid-linear ion trap-fourier transform mass spectrometry. Anal. Chem. 80, 396–406. doi: 10.1021/ac701690d
Ritossa, F. (1962). A new puffing pattern induced by temperature shock and DNP in Drosophila. Experientia 18, 571–573. doi: 10.1007/BF02172188
Sarkar, N. K., Kim, Y. K., and Grover, A. (2009). Rice sHsp genes: genomic organization and expression profiling under stress and development. BMC Genomics 10:393. doi: 10.1186/1471-2164-10-393
Sato, S., Tabata, S., Hirakawa, H., Asamizu, E., Shirasawa, K., Isobe, S., et al. (2012). The tomato genome sequence provides insights into fleshy fruit evolution. Nature 485, 635–641. doi: 10.1038/nature11119.
Scharf, K. D., Siddique, M., and Vierling, E. (2001). The expanding family of Arabidopsis thaliana small heat stress proteins and a new family of proteins containing α-crystallin domains (Acd proteins). Cell Stress Chaperones 6, 225–237.
Shirasu, K. (2009). The HSP90-SGT1 chaperone complex for NLR immune sensors. Annu. Rev. Plant Biol. 60, 139–164. doi: 10.1146/annurev.arplant.59.032607.092906
Siddique, M., Gernhard, S., von Koskull-Döring, P., Vierling, E., and Scharf, K. D. (2008). The plant sHSP superfamily: five new members in Arabidopsis thaliana with unexpected properties. Cell Stress Chaperones 13, 183–197. doi: 10.1007/s12192-008-0032-6
Sun, W., Van Montagu, M., and Verbruggen, N. (2002). Small heat shock proteins and stress tolerance in plants. Biochim. Biophys. Acta 1577, 1–9. doi: 10.1016/S0167-4781(02)00417-7
Van Montfort, R., Slingsby, C., and Vierling, E. (2002). Structure and function of the small heat shock protein/alpha-crystallin family of molecular chaperones. Adv. Protein Chem. 59, 105–156. doi: 10.1016/S0065-3233(01)59004-X
Vierling, E. (1991). The roles of heat shock proteins in plants. Annu. Rev. Plant Biol. 42, 579–620. doi: 10.1146/annurev.pp.42.060191.003051
Vierling, E., Harris, L. M., and Chen, Q. (1989). The major low-molecular-weight heat shock protein in chloroplasts shows antigenic conservation among diverse higher plant species. Mol. Cell. Biol. 9, 461–468. doi: 10.1128/MCB.9.2.461
Wang, W., Vinocur, B., and Altman, A. (2003). Plant responses to drought, salinity and extreme temperatures: towards genetic engineering for stress tolerance. Planta 218, 1–14. doi: 10.1007/s00425-003-1105-5
Waters, E. R. (2013). The evolution, function, structure, and expression of the plant sHSPs. J. Exp. Bot. 64, 391–403. doi: 10.1093/jxb/ers355
Waters, E. R., Aevermann, B. D., and Sanders-Reed, Z. (2008). Comparative analysis of the small heat shock proteins in three angiosperm genomes identifies new subfamilies and reveals diverse evolutionary patterns. Cell Stress Chaperones 13, 127–142. doi: 10.1007/s12192-008-0023-7
Waters, E. R., Lee, G. J., and Vierling, E. (1996). Evolution, structure and function of the small heat shock proteins in plants. J. Exp. Bot. 47, 325–338. doi: 10.1093/jxb/47.3.325
Waters, E. R., and Vierling, E. (1999a). The diversification of plant cytosolic small heat shock proteins preceded the divergence of mosses. Mol. Biol. Evol. 16, 127–139. doi: 10.1093/oxfordjournals.molbev.a026033
Waters, E. R., and Vierling, E. (1999b). Chloroplast small heat shock proteins: evidence for atypical evolution of an organelle-localized protein. Proc. Natl. Acad. Sci. U.S.A. 96, 14394–14399. doi: 10.1073/pnas.96.25.14394
Wei, Y., Wan, H., Wu, Z., Wang, R., Ruan, M., Ye, Q., et al. (2016). A comprehensive analysis of carotenoid cleavage dioxygenases genes in Solanum lycopersicum. Plant Mol. Biol. Rep. 34, 512–523. doi: 10.1007/s11105-015-0943-1
Xu, G., Guo, C., Shan, H., and Kong, H. (2012). Divergence of duplicate genes in exon-intron structure. Proc. Natl. Acad. Sci. U.S.A. 109, 1187–1192. doi: 10.1073/pnas.1109047109
Zhang, J., Chen, H., Wang, H., Li, B., Yi, Y., Kong, F., et al. (2016). Constitutive expression of a tomato small heat shock protein gene LeHSP21 improves tolerance to high-temperature stress by enhancing antioxidation capacity in tobacco. Plant Mol. Biol. Rep. 34, 399–409. doi: 10.1007/s11105-015-0925-3
Keywords: heat shock protein 20, gene organization, phylogenetic relationship, expression profile, abiotic and biotic stresses
Citation: Yu J, Cheng Y, Feng K, Ruan M, Ye Q, Wang R, Li Z, Zhou G, Yao Z, Yang Y and Wan H (2016) Genome-Wide Identification and Expression Profiling of Tomato Hsp20 Gene Family in Response to Biotic and Abiotic Stresses. Front. Plant Sci. 7:1215. doi: 10.3389/fpls.2016.01215
Received: 12 June 2016; Accepted: 02 August 2016;
Published: 17 August 2016.
Edited by:
Jie Zhou, Zhejiang University, ChinaReviewed by:
Chu-Yu Ye, Zhejiang University, ChinaJianfei Kuang, South China Agricultural University, China
Copyright © 2016 Yu, Cheng, Feng, Ruan, Ye, Wang, Li, Zhou, Yao, Yang and Wan. This is an open-access article distributed under the terms of the Creative Commons Attribution License (CC BY). The use, distribution or reproduction in other forums is permitted, provided the original author(s) or licensor are credited and that the original publication in this journal is cited, in accordance with accepted academic practice. No use, distribution or reproduction is permitted which does not comply with these terms.
*Correspondence: Hongjian Wan, d2FuaG9uZ2ppYW5Ac2luYS5jb20=
†These authors have contributed equally to this work.