- 1Laboratoire de Recherche en Sciences Végétales, Centre National de la Recherche Scientifique, Université de Toulouse III, Paul Sabatier, Toulouse, France
- 2Department of Plant Physiology, Umeå University, Umeå, Sweden
- 3“Agronomie et Environnement” Nancy-Colmar, Institut National de la Recherche Agronomique, Université de Lorraine UMR1121, Vandœuvre-lès-Nancy, France
Comparative phylogenetic analyses of the R2R3-MYB transcription factor family revealed that five subgroups were preferentially found in woody species and were totally absent from Brassicaceae and monocots (Soler et al., 2015). Here, we analyzed one of these subgroups (WPS-I) for which no gene had been yet characterized. Most Eucalyptus members of WPS-I are preferentially expressed in the vascular cambium, the secondary meristem responsible for tree radial growth. We focused on EgMYB88, which is the most specifically and highly expressed in vascular tissues, and showed that it behaves as a transcriptional activator in yeast. Then, we functionally characterized EgMYB88 in both transgenic Arabidopsis and poplar plants overexpressing either the native or the dominant repression form (fused to the Ethylene-responsive element binding factor-associated Amphiphilic Repression motif, EAR). The transgenic Arabidopsis lines had no phenotype whereas the poplar lines overexpressing EgMYB88 exhibited a substantial increase in the levels of the flavonoid catechin and of some salicinoid phenolic glycosides (salicortin, salireposide, and tremulacin), in agreement with the increase of the transcript levels of landmark biosynthetic genes. A change in the lignin structure (increase in the syringyl vs. guaiacyl, S/G ratio) was also observed. Poplar lines overexpressing the EgMYB88 dominant repression form did not show a strict opposite phenotype. The level of catechin was reduced, but the levels of the salicinoid phenolic glycosides and the S/G ratio remained unchanged. In addition, they showed a reduction in soluble oligolignols containing sinapyl p-hydroxybenzoate accompanied by a mild reduction of the insoluble lignin content. Altogether, these results suggest that EgMYB88, and more largely members of the WPS-I group, could control in cambium and in the first layers of differentiating xylem the biosynthesis of some phenylpropanoid-derived secondary metabolites including lignin.
Introduction
Eucalypts have extremely high adaptive potential and a considerable capacity to produce lignocellulosic biomass, explaining why this genus is the most planted hardwood worldwide for many industrial uses (Myburg et al., 2014). As tree species, eucalypts are long-living organisms characterized by a massive secondary growth produced by the activity of a secondary meristem, called the vascular cambium. Although essential for secondary growth, the vascular cambium is difficult to study because of its internal location and has received much less attention that the other plant meristems. During tree radial growth, the cambial cells divide and differentiate centripetally into xylem cells, characterized by thick secondary cell walls made of cellulose (40–50%), hemicelluloses (25%), and lignin (25–35%) (Plomion et al., 2001).
Lignin is a complex phenolic polymer which ensures essential functions for terrestrial plants providing mechanical support and facilitating the water transport. It is composed of mainly two units, syringyl (S) and guaiacyl (G), with also minor amounts of p-hydroxyphenyl (H) units, all produced by the monolignol-specific branch of the general phenylpropanoid metabolism. In addition to lignin monomers, the phenylpropanoid metabolism serves as a starting point for a vast array of other important compounds, such as flavonoids (flavonols, anthocyans, and proanthocyanidins), coumarins (Vogt, 2010), or phenolic glycosides (Babst et al., 2010). All these phenylpropanoid-derived secondary metabolites are crucial for plant survival, contributing to all aspects of plant responses toward biotic and abiotic stimuli (Vogt, 2010).
R2R3-MYB genes constitute one of the largest families of transcription factors in plants. They regulate many aspects of plant biology, such as primary and secondary metabolism, cell fate, developmental processes, and responses to biotic and abiotic stresses. Noteworthy, more than 30% of these genes characterized in Arabidopsis regulate different aspects of the phenylpropanoid metabolism, including the biosynthesis of lignin and flavonoids (Dubos et al., 2010). R2R3-MYB proteins are characterized by a highly conserved N-terminal DNA-binding domain (R2R3-MYB domain) and a highly variable C-terminal activation or repression domain. The combination of phylogenetic studies with the detection of conserved motives in the C-terminal region enabled to define 22 subgroups in Arabidopsis (Stracke et al., 2001) found to be quite well conserved in other species. Interestingly, genes within the same subgroup are thought to realize similar functions (Dubos et al., 2010).
Recently, the phylogenetic analysis of the R2R3-MYB members from Eucalyptus grandis, Arabidopsis thaliana, Populus trichocarpa, Vitis vinifera, and Oryza sativa allowed to identify five subgroups of R2R3-MYB proteins preferentially found in woody species, named as Woody Preferential Subgroups (WPS-I, II, III, IV, and V), which are totally absent in the basal lineages of the Bryophytes and Lycophytes, as well as in the more modern Monocot and Brassicaceae lineages (Soler et al., 2015). E. grandis genes from WPS-I, II, and III, are preferentially expressed in the cambial region, and given the close phylogenetic relationship with genes from subgroups involved in the regulation of the phenylpropanoid metabolism such as S4, S5, S6, S7, and SAtMYB5 (Dubos et al., 2010), it could be hypothesized that genes belonging to WPS-I, II, and III regulate the biosynthesis of some phenylpropanoid-derived compounds (Soler et al., 2015). Indeed, some genes belonging to WPS-II and III, but attributed earlier to subdivisions of S4 or S5, have already been shown to regulate the biosynthesis of flavonoids. For example, the two genes characterized from WPS-II (VvMYBPA1 from grapevine and DkMYB2 from persimmon) act as activators of the biosynthesis of proanthocyanidins and other phenylpropanoid-derived compounds (Bogs et al., 2007; Akagi et al., 2010), whereas the genes characterized from WPS-III (FaMYB1 from Fragaria x ananasa, FcMYB1 from Fragaria chiloensis, VvMYBC2-L1, and VvMYBC2-L3 from grapevine, PhMYB27 from petunia and PtMYB182 in poplar) act as repressors of the biosynthesis of these compounds (Aharoni et al., 2001; Salvatierra et al., 2013; Albert et al., 2014; Huang et al., 2014; Cavallini et al., 2015; Yoshida et al., 2015). These studies focused mostly on leaves, fruits, flowers, or in vitro cultivated hairy roots. None has investigated the function of these genes in cambium and/or in differentiating xylem, nor their effects over lignin content and composition. Moreover, no gene belonging to WPS-I has been characterized to date in any plant species.
With the aim to better understand the role of WPS-I R2R3 MYBs, we functionally characterized a representative member, EgMYB88, shown to be highly and preferentially expressed in the cambial region. We showed that it behaves as an autoactivator of Gal4 in yeast. Given the considerable difficulty to obtain transgenic eucalypts, we decided to overexpress EgMYB88 in both Arabidopsis and poplar, either as a native form or fused to an active repressor motif [Ethylene-responsive element binding factor-associated Amphiphilic Repression (EAR)] to transform it into a dominant repressor (Hiratsu et al., 2003). As could be expected from a gene absent in Brassicaceae, the Arabidopsis transgenic lines showed no phenotypic differences compared to controls. In contrast, poplar transgenic lines overexpressing EgMYB88 showed a substantial increase in phenolic glycosides and flavonoids concomitant with a modification of the lignin structure (increase in the S/G ratio). Poplar lines overexpressing EgMYB88-EAR showed a significant reduction of flavonoids and soluble oligolignols accompanied by a reduction of the lignin content. Altogether, these results suggest that the main role of EgMYB88 in the cambial region, and likely of its orthologs in poplar, is to control specific branches of the phenylpropanoid metabolism.
Materials and Methods
Phylogenetic Analysis
Sequences of MYB proteins from E. grandis, P. trichocarpa, V. vinifera, and A. thaliana from a phenylpropanoid metabolism-related super clade were obtained from Soler et al. (2015). It includes members of S4 (with AtMYB6 and AtMYB8), S5, S6, S7, S15, SAtMYB5, SAtMYB82, WPS-I, WPS-II, and WPS-III. For P. trichocarpa, we updated the protein sequences from the last genome version (3.0). Two gene models from version 2.2 were not included in the analysis: POPTR_0019s05200 was absent in version 3.0, whereas the new amino acid sequence prediction of POPTR_0018s08500 (Potri.018G049000) lacked part of the R3 MYB domain.
Amino acid sequences were aligned using MAFFT with the FFT-NS-i algorithm (Katoh et al., 2002) and used to construct a Neighbor-joining phylogenetic tree using Mega5 (Tamura et al., 2011) with 1000 bootstrap replicates. Sequences were compared using the complete deletion method and the evolutionary distances were computed with the Jones–Taylor–Thornton substitution model using the rate variation among sites with a gamma distribution of 1, as done by Soler et al. (2015). A distant R2R3-MYB gene involved in the lignin biosynthesis, AtMYB52 (Cassan-Wang et al., 2013) was also used to root the phylogenetic reconstruction.
Gene Cloning and Vector Construction
EgMYB88 coding sequence was obtained from Eucalyptus gunnii (Eg) xylem cDNA using the primers CACCCATATGGAGAAATCATCAGCTGCAA and GAGCTCTCCTGATCTCTCATCACA with the Phusion taq (Finnzymes). EgMYB88 sequence from E. gunnii (accession number KX470407) was highly similar to EgrMYB88 (Egr indicates E. grandis) sequence (Eucgr.F04423.1), with just one nucleotide change at the R3 MYB domain which do not modify the amino acid sequence. Amplicon was then inserted in the pENTR D-TOPO vector (Invitrogen) and subsequently transferred into several destination vectors using LR clonase II (Invitrogen) following manufacturer's instructions. Destination vectors were pGBD-GTW (kindly provide by Dr Laurent Deslandes, LIPM, INRA, France) for yeast auto activation assays, pFAST-G02 (Shimada et al., 2010) for overexpression in Arabidopsis, pJCV53 (obtained from the Gateway Vectors facility from Ghent University, Belgium) for overexpression in poplar, and pH35SGEAR (kindly provided by Dr Taku Demura, NAIST, Nara, Japan) for dominant repression in Arabidopsis and poplar. Coding sequences of EgMYB1 and EgMYB2 (Goicoechea et al., 2005; Legay et al., 2007) were cloned into the pDONR207 vector (Invitrogen) and were transferred into the pGBD-GTW vector also using the LR clonase II.
Plant Material
EgMYB88 cloned into the pFAST-G02, the pJCV53, or the pH35SGEAR vectors was inserted into Agrobacterium tumefaciens GV3101 (pMP90) using freeze and thaw method. Wild type Col-0 Arabidopsis plants were transformed using the floral dip method (Clough and Bent, 1998) with A. tumefaciens harboring EgMYB88 into the pFAST-G02 and the pH35SGEAR vectors. As controls, we used wild type plants and plants transformed with the respective empty vectors. Transformed seeds from pFAST-G02 vector were screened using a fluorescent stereomicroscope with GFP filters as described in Shimada et al. (2010), whereas seedlings transformed with the pH35SGEAR vector were screened using MS ½ media supplemented with hygromcycin (20 μg/ml). More than 10 transformed independent lines without any visible phenotype as compared to controls were obtained for each construct and screened by conventional RetroTranscriptase-quantitative Polymerase Chain Reaction (RT-qPCR) to assess the level of transgene expression. For detailed characterization of their phenotypes, three lines were selected for each construct based on high transgene expression levels (Figure S1). Plants were grown in a growth chamber in short day conditions (9 h light and 15 h dark) to promote secondary growth. Sampling was done in plants from at least 8 weeks old, when the inflorescence stems were fully developed and the first siliques were clearly observed. The base of the inflorescence stem was kept in ethanol 80% for histochemistry analysis. The rest of the inflorescence stem without leaves and siliques (except the first 5 cm, which were discarded because the proportion of xylem tissue was very low) was immediately frozen in liquid nitrogen. Five plants samples were pooled for each independent line. They were subsequently milled to powder using a ball-mill (MM400, Retsch) with liquid nitrogen to keep them protected from degradation, and kept at −80°C for lignin quantification. Similarly, hypocotyls were harvested, pooled, frozen in liquid nitrogen, milled to powder and kept at −80°C for Pyrolysis-Gas Chromatography/Mass Spectrometry (Py-GC/MS) phenolic profiling.
Hybrid Populus tremula x P. alba (INRA clone 717-1-B4) was maintained on MS-1B media (Duchefa) supplemented with 2% sucrose, 0.5 mg/L of IAA (indole-3-acetic acid, Duchefa), and 0.5 mg/L of IBA (indole-3-butyric acid, Sigma). Hybrid poplar was transformed with A. tumefaciens harboring EgMYB88 into the pJCV53 and the pH35SGEAR vectors following the protocol described by Gallardo et al. (1999). As controls we used both wild type plants and plants transformed with the empty vectors. Selection of transformants was performed using kanamycin (50 μg/ml) for the pJCV53 and hygromycin (20 μg/ml) for pH35GEAR transformed plantlets. Several transformed plantlets were obtained for each construct without any visible phenotype as compared to controls, and screened by conventional RT-qPCR to assess the level of transgene expression. For detailed characterization of their phenotypes, three lines were selected for each construct according to transgene expression levels (Figure S2); however, for plants overexpressing EgMYB88 into the pJCV53 vector we did not chose the lines with the highest transgene expression as they did not multiply well in vitro. Selected plants were then acclimated for 3 weeks in a growth chamber in long day conditions (16 h light and 8 h dark) and then transferred into a greenhouse for 2 months. Sampling was done taking a 5 cm portion of the base of the stem and kept it in ethanol 80% for histochemistry analysis. The subsequent 5 cm portion of the base of the stem, taken for transcriptomics, as well as the rest of the stem (except the first 12 internodes, discarded because the proportion of xylem was low), taken for biochemistry, were immediately debarked, frozen in liquid nitrogen, milled to powder and kept at −80 °C for transcriptomic and biochemical analyses.
Yeast Autoactivation Tests
Yeast cells, strain Y2HGold (Clontech), were transformed with the plasmid pGBD-GTW using the Yeast Transformation System 2 (Clontech) following manufacturer's instructions and plating them on a Synthetically Defined medium without Tryptophan (SD-Trp). Plasmid pGBD-GTW contains a gene which confers the ability to grow on media without Tryptophan. Transformed yeast cells were resuspended in NaCl 0.9% solution until OD600 of 2 and then plated in different selective media. After 3 days incubation at 30°C, growing of yeast cells was visible.
Transcriptomic Analysis
Total RNA was extracted from Arabidopsis leaves using the protocol for “vegetative tissues” from Oñate-Sánchez and Vicente-Carbajosa (2008) and from poplar leaves and debarked stems using the protocol from Southerton et al. (1998). RNA was further digested using TurboDNase (Ambion) to remove residual traces of contaminating DNA. One Microgram of RNA was retrotranscribed into cDNA using the High Capacity RNA to cDNA kit (Applied Biosystems) and diluted 5 times before use as template in conventional or microfluidic RT-qPCR.
Conventional RT-qPCR was performed on cDNA from Arabidopsis and poplar leaves to assess the level of transgene expression in technical triplicates using ABI 7900HT real-time PCR system (Applied Biosystems) with the Power SYBR Green PCR Master Mix (Applied Biosystems). PCR conditions were 95 °C for 10 min, followed by 40 cycles of 95°C for 15 s and 60°C for 1 min. After amplification, a dissociation step was performed to confirm the presence of a simple amplicon. Transcript abundance was calculated using the 2−ΔΔCt method, with the housekeeping gene actin2 (ACT2, Legay et al., 2010) for Arabidopsis and the housekeeping gene cell division cycle2 (CDC2, Legay et al., 2010) for poplar to normalize data. Control samples were used to standardize results.
Microfluidic RT-qPCR was performed on cDNA from poplar debarked stems to assess the levels of phenylpropanoid metabolism genes using the Biomark® 96.96 Dynamic Array platform (Fluidigm) as described in Cassan-Wang et al. (2012). After amplification, a dissociation step was performed to confirm the presence of a simple amplicon. Transcript abundance was calculated using the 2−ΔΔCt method, with the geometric mean of five validated housekeeping genes to normalize the results [Ubiquitin (UBQ), CDC2 (Legay et al., 2010), HK1, HK3, and HK11 (Sixto et al., 2016)]. Control samples were used to standardize the results. Finally, data was used in MeV (Saeed et al., 2003) to generate a heatmap.
Genes analyzed by microfluidic RT-qPCR were selected according to their importance in the phenylpropanoid metabolism or in cambium activity and differentiation, based on literature search (Ranocha et al., 2002; Shi et al., 2010; Berthet et al., 2011; Sun et al., 2011; Huang et al., 2012; Vanholme et al., 2013; Zhu et al., 2013; Liu et al., 2014a,b; Chedgy et al., 2015; Etchells et al., 2015; Yoshida et al., 2015). Primer sequences were obtained from literature whenever possible or designed either using Quantprime (Arvidsson et al., 2008) or Primer3 (Rozen and Skaletsky, 2000). Primer sequences for RT-qPCR are detailed in Table S1.
Histological Analysis
Arabidopsis and poplar stems were cut into 90 μm-thick sections using a vibratome (VT 100S, Leica). Due to the strong lignification of secondary xylem of poplar stems, vibratome was equipped with a Sapphire blade (Delaware Diamond Knives). Lignified secondary cell walls were stained with phloroglucinol-HCl reagent (VWR) and observed immediately under a bright-field inverted microscope (DM IRBE, Leica) equipped with a CDD color camera (DFC300 FX, Leica).
Biochemical Analysis
Milled samples from Arabidopsis and poplar stems were lyophilized and then subjected to exhaustive extraction with water, then ethanol, ethanol/toluene (50/50) and acetone in a Soxhlet apparatus. Recovered extractive-free samples were dried at 60°C overnight. Lignin content was evaluated in Arabidopsis using the acetyl bromide lignin method on 10 mg samples as described by Soler et al. (2016). Klason procedure was performed as previously described (Méchin et al., 2014) to quantify the acid-insoluble lignin on 50 mg of poplar samples.
The poplar lignin structure was analyzed using the thioacidolysis method on 10 mg of extractive-free sample as described by Méchin et al. (2014). Briefly, we used 1 ml of thioacidolysis reagent per mg of sample (for 100 ml of solution: 2.5 ml of boron trifluoride, 10 ml of ethanethiol and 0.2 ml of tetracosane 1.25 mg/ml, up to 100 ml dioxane). An aliquot of this solution (5 μl) was trimethylsilylated with 100 μl of N,O-bis(trimethylsilyl)trifluoroacetamide and 10 μl of pyridine for 30 min at 60°C. The trimethylsilylated samples were injected in a Gas Chromatography/Mass Spectrometry (GC-MS) (TSQ Quantu, Thermo Scientific) fitted with an autosampler, a splitless injector (280°C), and a iontrap mass spectrometer operating in the electronic impact mode with a source at 220°C, an interface at 280°C, and a 50–650 m/z scanning range. The column was a ZB5-MSi (Phénomenex) operated in the temperature program mode (from 45 to 180°C at +30°C/min, then 180 to 260°C at +3°C/min) with helium carrier gas at 1.5 ml/min flow rate.
Pyrolysis–Gas Chromatography/Mass Spectrometry (Py-GC/MS) analysis were performed with 80 μg of lyophilized powder per injection using non-extracted Arabidopsis hypocotyls. Powder was applied to a pyrolyzer equipped with an auto sampler (PY-2020iD and AS-1020E, Frontier Lab) connected to a GC/MS (7890A/5975C, Agilent). Pyrolysate separation and analysis, including peak detection, integration, normalization, and identification was done according to Gerber et al. (2012).
Metabolite Profiling
A total of 30 mg of lyophilized powder from poplar stems was extracted with 1 mL methanol 70% blended for 1 min using an Ultra-thurrax and then centrifuged at 10,000 g for 10 min. The supernatant was transferred to a new tube and one additional mL was added to the pellet, vortexed and let for 2 h at room temperature. The mixture was centrifuged again at 10,000 g for 10 min and the recovered supernatant was mixed with the first one. The 2 mL solution was evaporated in a speed-vacuum until dryness. Then, dried pellet was dissolved in 500 μL methanol 70% and passed through 0.22 μm filter.
Extracts (1 μL) were analyzed on Ultra-High Performance Liquid Chromatography (U-HPLC) system (Shimadzu) equipped with a photoDiode Array Detector (DAD) and a mass spectrometer. Sample was separated on a C18 kinetex (100 × 2.1 mm) column (Phenomenex). The mobile phase consisted in 0.1% formic acid in ultra-pure water (solvent A) and 0.1% formic acid in methanol (solvent B).
The molecules were eluted through a gradient elution from 1 to 99% B for 13 min with a flow rate of 400 μL/min and then 3 min in 99% B. The column was then re-equilibrated to 1% B prior to the next run. Mass spectrometry analysis was carried out in ESI negative mode. Quantification was performed by measuring the area under each peak at 280, 320, or 350 nm, depending on the lambda max of each molecule. Total metabolic signal was performed at 280 nm. Due to co-eluting signal at 280 nm, catechin quantification was realized by measuring the area under peak at m/z 289, corresponding to the [M-H]− of the molecule. Experimental exact masses and MS fragments were compared to metabolomics data banks (Respect: http://spectra.psc.riken.jp/; Mass Bank: http://massbank.jp/, DNP: http://dnp.chemnetbase.com/) and data available in the literature in order to identify the nature of the metabolites.
Results
The WPS-I Belonging Gene EgrMYB88 Is Highly and Preferentially Expressed in the Cambial Region
Based on the phylogeny of the whole R2R3-MYB family in E. grandis (Soler et al., 2015), we selected the genes from the WPS-I and from closely related subgroups (S4, S5, S6, S7, SAtMYB5, SAtMYB82, WPS-II, and III) that constitute a phenylpropanoid metabolism-related super-clade. We reconstructed a phylogenetic sub-tree using sequences of E. grandis, A. thaliana, P. trichocarpa, and V. vinifera (Figure 1). WPS-I is supported by a high bootstrap value and is clearly separated from the other subgroups. It contains six genes from E. grandis, four from poplar, three from grapevine, but none from Arabidopsis.
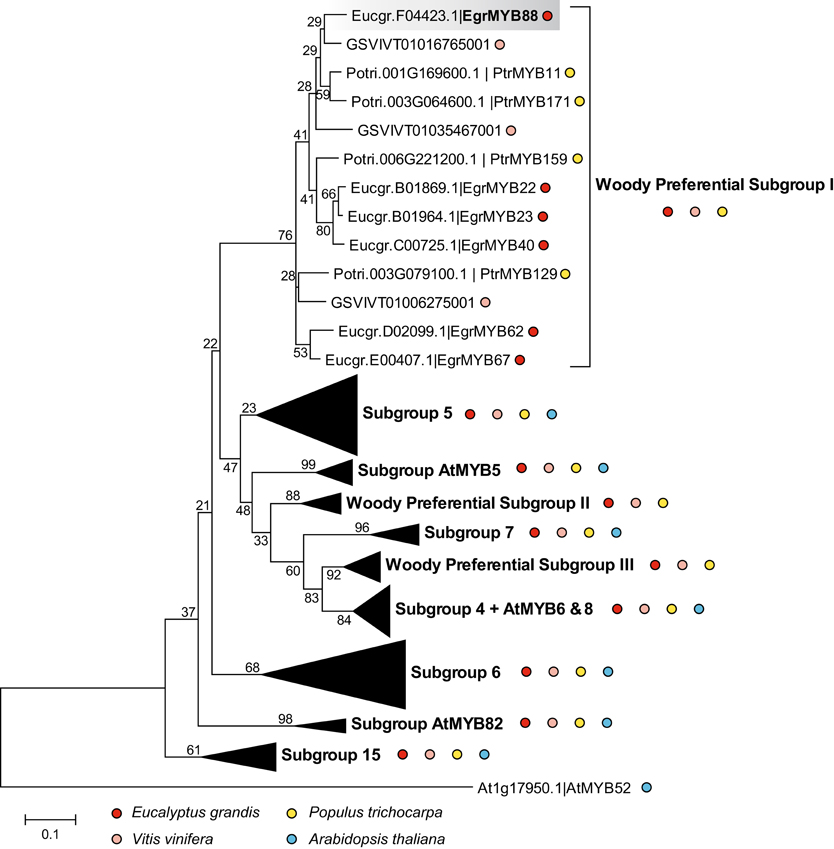
Figure 1. Neighbor-Joining phylogenetic tree constructed with R2R3-MYB members of phenylpropanoid metabolism-related super clade. The proteins included belong to the subgroups S4, S5, S6, S7, S15, SAtMYB5, SAtMYB82, WPS-I, WPS-II, and WPS-III from E. grandis (red dots), V. vinifera (pink dots), P. trichocarpa (yellow dots), and A. thaliana (blue dots). The distant protein AtMYB52 (Cassan-Wang et al., 2013), involved in the lignin biosynthesis, was used to root the tree. The WPS-I is expanded to show the different members from E. grandis, P. trichocarpa and V. vinifera, and the absence of genes from Arabidopsis. EgrMYB88 is highlighted with a gray background. Bootstrap values are shown on the internodes.
No segmental, whole genome or tandem duplications were previously detected for any of the WPS-I genes in the E. grandis genome (Soler et al., 2015). However, giving the extremely high sequence conservation (98% similarity at the protein level) between EgrMYB22 and EgrMYB23, the corresponding genes likely derived from a relatively recent duplication event further supported by their close location on the same chromosome (Figure S3, Table S2). All the other members of the WPS-I subgroup are located on different chromosomes. The EgrMYB88 protein sequence is the most divergent from the other Eucalyptus WPS-I members (Table S2). It has two putative orthologs in P. trichocarpa, PrtMYB11 and PtrMYB171 (Figure 1, Table S2).
The six E. grandis and the four P. trichocarpa proteins exhibit high sequence homology in the R2R3-MYB domain, and also a conserved motif in the C-terminal region (Figure 2). This motif specific of the subgroup suggests similar function of the WPS-I genes. Moreover, as found for many other R2R3-MYB genes, a predicted motif for interaction with basic Helix-Loop-Helix (bHLH) proteins was also detected [(DE)Lx2(RK)x3Lx6Lx3R; Figure 2] (Zimmermann et al., 2004), which indicates that the function of these genes may be modulated by protein-protein interactions.
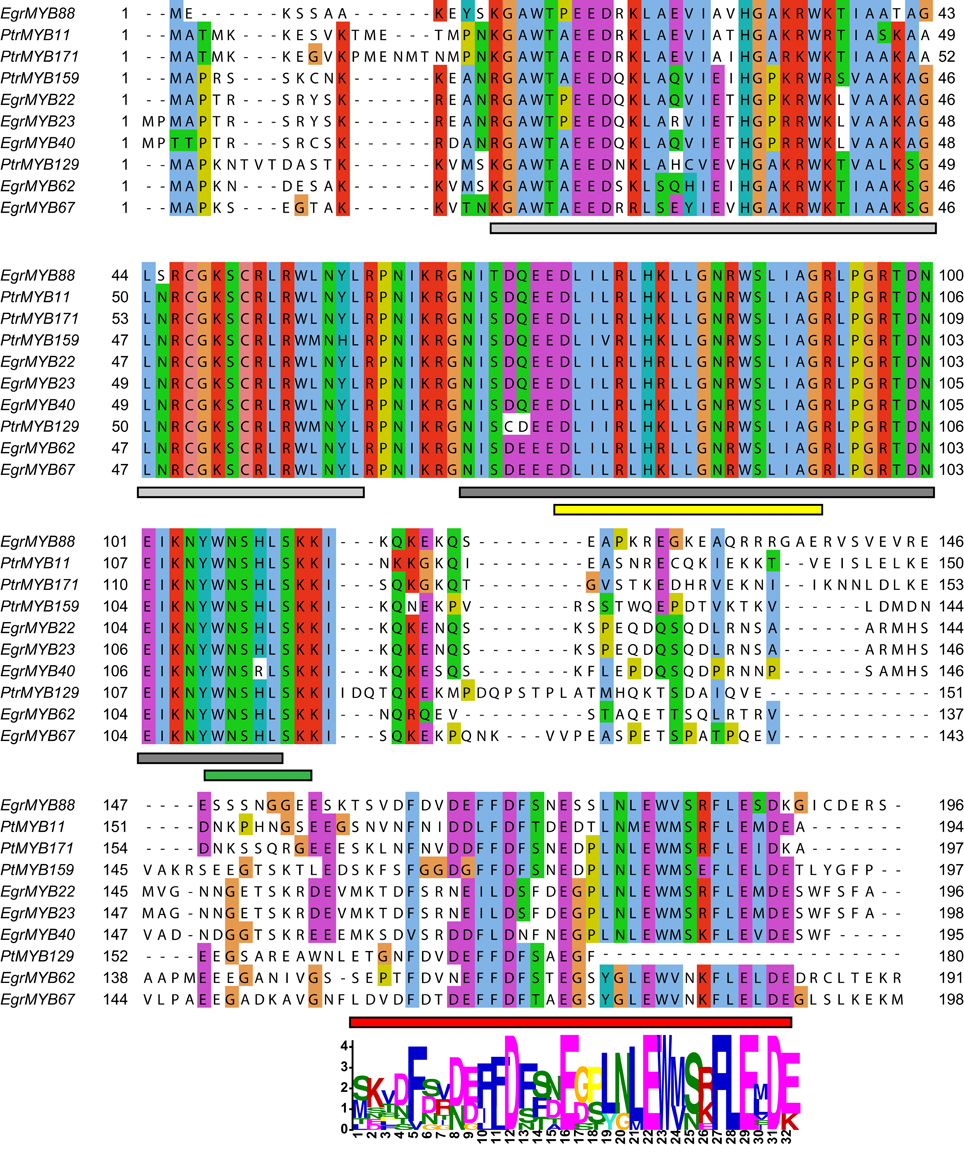
Figure 2. Amino acid sequence alignment of the E. grandis and P. trichocarpa genes belonging to the WPS-I. Amino acid are colored according to their similarity degree using the ClustalX option of Jalview (Waterhouse et al., 2009; http://www.jalview.org/help/html/colourSchemes/clustal.html). Light gray and dark gray rectangles at the bottom of the alignment indicate the R2 and the R3 MYB domains, respectively. Yellow rectangle indicates the bHLH interaction motif described by Zimmermann et al. (2004). Green rectangle indicates the region that, at the mRNA level, is targeted by the miR828 (Xia et al., 2012). Red rectangle indicates the position of the conserved C-terminal motif, whose sequence obtained using the MEME Suite (Bailey et al., 2009) is indicated below.
Because WPS-I closely related subgroups such as S6 and S7 involved in flavonoids biosynthesis were shown to be post-transcriptionally repressed by microRNAs, (mostly miR828 and/or miR858; Xia et al., 2012), we examined if the genes of WPS-I could also be targets of these microRNAs. By performing an in silico search, we found that the miRNA828 recognition sequence located at the end of the R2R3-MYB domain was highly conserved (Figure 3) in all the WPS-I genes from Eucalyptus and poplar with the exception of EgrMYB40. No cleavage site for miR858 was found in any of these genes (data not shown). These results are in agreement with a Eucalyptus degradome analysis showing that EgrMYB22, 23, 62, 67, and 88, but no EgrMYB40, were cleaved by miR828 (Carocha and Paiva, personal communication).
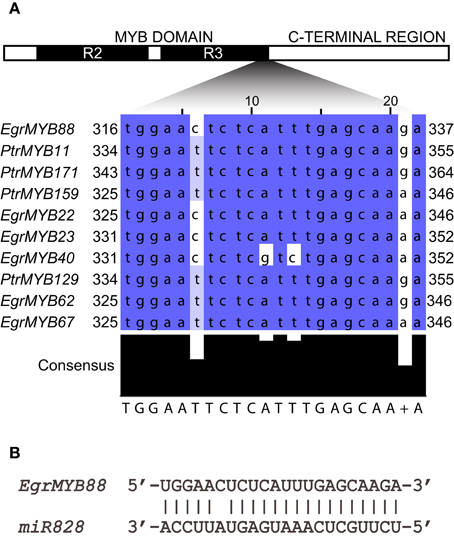
Figure 3. Sequence region targeted by miR828 among the E. grandis and P. trichocarpa members of WPS-I. (A) Gene nucleotide sequence alignment colored according to their degree of identity percentage using Jalview (Waterhouse et al., 2009; http://www.jalview.org/help/html/colourSchemes/pid.html). (B) Alignment between the EgMYB88 mRNA and the miR828 showing one single mismatch among 22 nucleotides. Sequence targeted by miR828 is based on Xia et al. (2012).
We then analyzed the transcript abundance of the genes from WPS-I in different Eucalyptus organs and tissues previously described in Soler et al. (2015). All of them showed the highest expression in the cambial region although some were also expressed in other tissues/organs (Figure 4). EgrMYB22, for instance was also highly expressed in leaves. EgrMYB88 was preferentially expressed in the vascular tissues (xylem and phloem) and exhibited the highest expression in the cambial region. The expression of its orthologs in P. tremula was obtained from Popgenie database (Sjödin et al., 2009) based on the RNAseq survey performed by Sundell et al. (2015). Similarly to EgMYB88, PtMYB11, and PtMYB171 were highly expressed in wood, but exhibited distinct expression profiles in other non-woody tissues (Figure S4). Because of the importance of the vascular cambium and differentiating xylem in the secondary growth characteristic of woody plants, we decided to investigate the function of EgrMYB88 by cloning its coding sequence from E. gunnii growing in Southwest France (EgMYB88). Nucleotide coding sequence is nearly identical between EgrMYB88 and EgMYB88, with just a base change at the R3 MYB domain that do not modify the amino acid sequence.
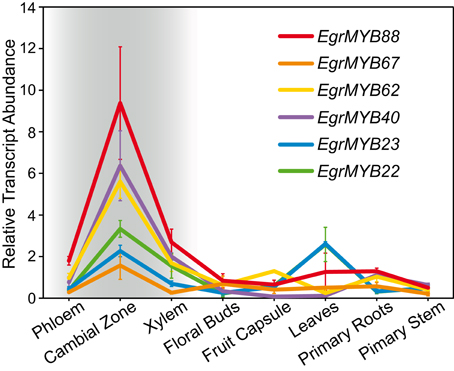
Figure 4. Transcript abundance data of the different Eucalyptus genes from WPS-I. Expression data (means ± standard deviation) in different organs and tissues from Eucalyptus was extracted from Soler et al. (2015). Vascular tissues are highlighted with a gray background. EgrMYB88 is the gene with the highest expression in vascular tissues.
EgMYB88 Behaves as a Transcriptional Activator in Yeast
To determine if EgMYB88 is a transcriptional activator, we fused it to the Gal4 binding domain and investigated its capacity to activate the transcription of four reporter genes in yeast (Figure 5A). EgMYB88 was able to activate the reporter gene HIS3 and MEL1, as revealed by the ability of the yeast transformed with EgMYB88 to grow on selective media lacking Histidine and to metabolize X-α-Gal (5-bromo-4-chloro-3-indolyl alpha-D-galactopyranoside) producing blue colonies (Figure 5B). EgMYB88 was, however, unable to induce the reporter gene ADE2 and AUR1C, since the yeast transformed with EgMYB88 did not grow on media lacking both Adenine and Histidine, or containing the antibiotic Aureobasidin A in addition to X-α-Gal. Thus, EgMYB88 behaves as an activator in yeast, but its activity seems weaker than that of EgMYB2, a known transcriptional activator (Goicoechea et al., 2005), which was able to activate the four reporter genes (Figure 5B). In contrast, EgMYB1, a known transcriptional repressor (Legay et al., 2007; Soler et al., 2016), as well as the effector empty vector, were unable to activate any of the reporter genes.
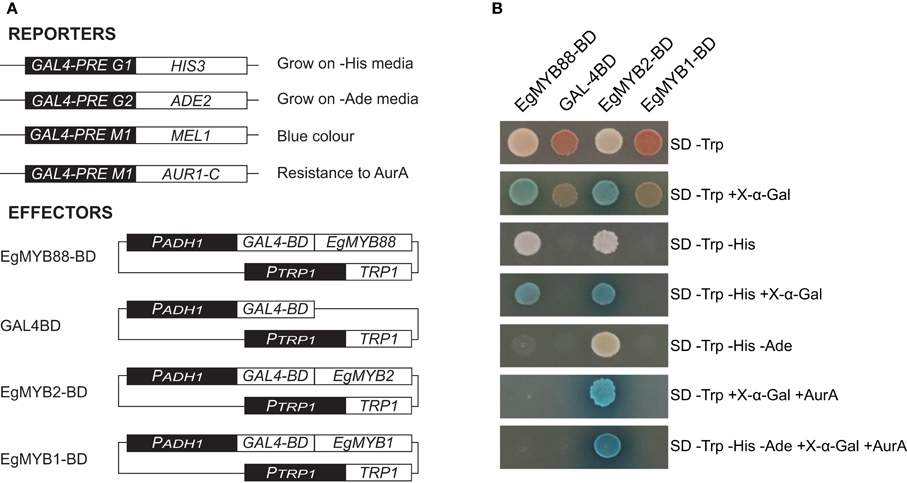
Figure 5. Yeast auto-activation tests of EgMYB88 fused to the DNA binding domain of Gal4. (A) Reporter and effector constructs used in the assay. The reporter constructs consists of the cis-regulatory elements to which Gal4 binds before the four reporter genes tested. The effector constructs consist of fusion proteins with the Gal4 binding domain (BD) at the N-terminal end. As controls, we tested the empty vector as well as two well-known Eucalyptus genes, one acting as an activator (EgMYB2, Goicoechea et al., 2005) and the other as a repressor (EgMYB1, Legay et al., 2007). (B) Growth of yeast cells in different selective media. Growth in auxotrophic media (SD) without Tryptophan (Trp) indicates the presence of the effector plasmid. Growth in the absence of Histidine (His) or Adenine (Ade) are indicative of activation of HIS3 and ADE2 reporter genes. Growth in presence of the antibiotic Aureobasidin A (AurA) indicates the activation of AUR1-C, which confers resistance to this antibiotic. Blue color represent the activation of MEL1 reporter gene, able to produce a blue precipitate in presence of 5-bromo-4-chloro-3-indolyl alpha-D-galactopyranoside (X-α-Gal).
Overexpression of EgMYB88 or EgMYB88-EAR Does Not Show Effects in Arabidopsis
As a first step to functionally characterize EgMYB88 in planta, we used the 35S Cauliflower Mosaic Virus promoter promoter (Pro35S) to overexpress in Arabidopsis either its native form (Pro35S:EgMYB88) or a dominant repression form (Pro35S:EgMYB88-EAR). The fusion with the EAR motif was previously shown to efficiently convert transcriptional activators into strong repressors (Hiratsu et al., 2003). Several independent transgenic plants were obtained for each of the two constructs, which were phenotypically similar to the controls (wild-type plants and lines containing empty vectors) after visual inspection. We selected three lines for each construct (Figure S5) in order to further characterize their phenotypes by performing histochemical and biochemical analyses. Sections at the basis of the inflorescence stems were stained in red with phloroglucinol-HCl, a common stain for lignin. No differences in the staining intensity nor in the xylem structure were observed in the Pro35S:EgMYB88 and/or Pro35S:EgMYB88-EAR lines compared to controls (Figure 6). Lignin contents assessed using the acetyl bromide method on extractive-free cell walls of Arabidopsis inflorescence stems were similar in Pro35S:EgMYB88, Pro35S:EgMYB88-EAR lines and controls (Table 1), in agreement with the histological observations. In order to have a wider overview of cell wall composition, we performed Py-GC/MS analysis of non-extracted Arabidopsis hypocotyls. The comparison between the transgenic lines and the controls did not reveal any differences (Table S3).
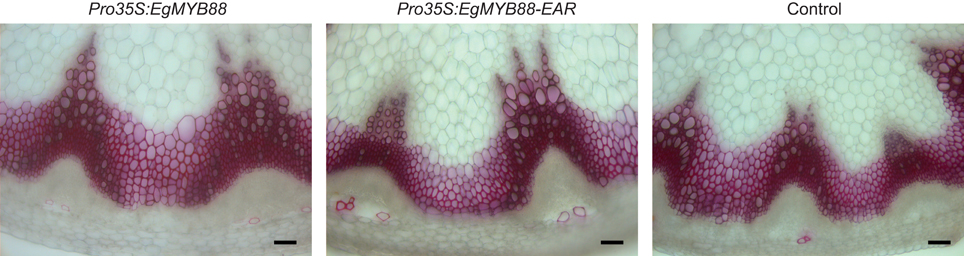
Figure 6. Transverse sections of inflorescence stems of transgenic Arabidopsis plants overexpressing EgMYB88 either as a native form or fused to an EAR motif. Detail of Arabidopsis inflorescence stems where lignin are stained in red by phloroglucinol-HCl. Scale bars: 50 μm. Images shown are representative of the three independent lines analyzed for each construction (Pro35S:EgMYB88 and Pro35S:EgMYB88-EAR, specified in Figure S1).
Lignin Structure is Altered in Poplars Overexpressing EgMYB88 While Lignin Content Is Reduced When Overexpressing EgMYB88-EAR
Poplar plants were transformed with the same kind of constructs, i.e., Pro35S:EgMYB88 and Pro35S:EgMYB88-EAR. Transgenic lines generated in vitro did not show any visible differences as compared to control lines. Three independent lines per construct (Figure S6) were acclimated and transferred into the greenhouse for a deeper characterization by histochemical and biochemical analyses. The Pro35S:EgMYB88 and Pro35S:EgMYB88-EAR plants were grown in two separate batches together with their appropriate controls. None of the lines showed differences in their overall xylem structure, including cell wall thickness (observed in mature xylem cells as well as in differentiating xylem close to the vascular cambium), vessel frequency and size (Figure 7). The intensity of the phloroglucinol-HCl staining of Pro35S:EgMYB88 xylem was similar to that of their controls, whereas a weaker red color was observed in the xylem of Pro35S:EgMYB88-EAR lines, suggesting a reduction of the lignin content (Figure 7).
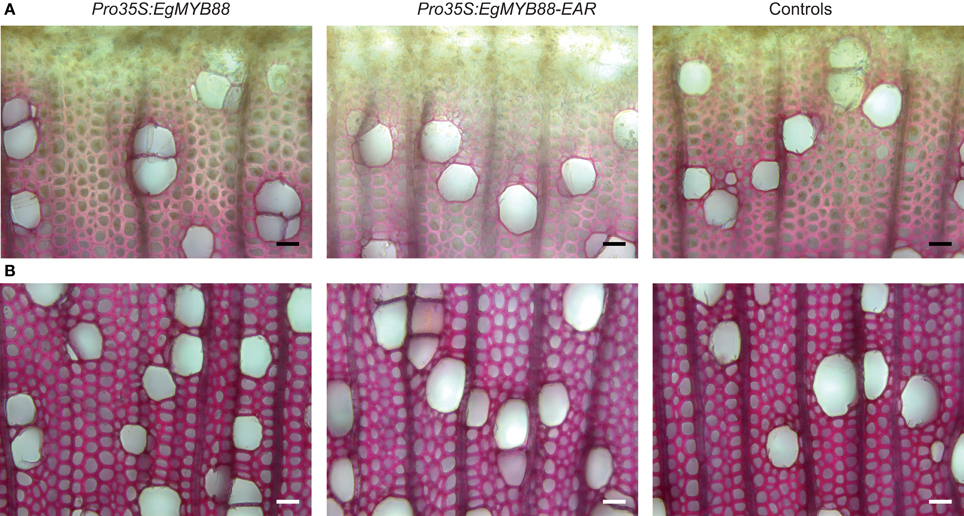
Figure 7. Transverse sections of stems from transgenic poplar plants overexpressing EgMYB88 either as a native form or fused to an EAR motif. (A) Detail of the cambial region and of the first layers of differentiating xylem cells. (B) Detail of mature xylem cells. Sections were stained using phloroglucinol-HCl which stains the lignin in red. Scale bars: 25 μm. Images shown are representative of the three independent lines analyzed for each construction (Pro35S:EgMYB88 and Pro35S:EgMYB88-EAR, specified in Figure S2). One single control representative of the two poplar batches is shown.
These histochemical observations were confirmed by Klason lignin quantification (Table 2). The insoluble lignin content of Pro35S:EgMYB88 poplar plants was unchanged as compared to their corresponding controls (Table 2) while the Pro35S:EgMYB88-EAR poplar plants exhibited a small but significant reduction (6%) of the Klason lignin content (Table 3). Lignin structure was then analyzed by thioacidolysis, which specifically targets the lignin units (S and G) involved only in labile β-O-4 linkages (Méchin et al., 2014). When normalized relative to the Klason lignin content, the thioacidolysis yield was not significantly different between the Pro35S:EgMYB88 lines and their controls, meaning that the total amount of G and S lignin units involved only in β-O-4 linkages was unaffected (Table 2). However, Pro35S:EgMYB88 plants showed a significant different proportion of lignin units, with an increase in S units and a decrease in G units, resulting in a higher S/G ratio (13% higher than controls, Table 2). The thioacidolysis yield was not affected in the Pro35S:EgMYB88-EAR poplar xylem, but the proportion of S and G units were slightly affected resulting in a tendency of lower S/G ratio, although not statistically significant (Table 3).

Table 2. Lignin content and structure in stems of Pro35S:EgMYB88 poplar transgenic lines evaluated by Klason (KL) and thioacidolysis.
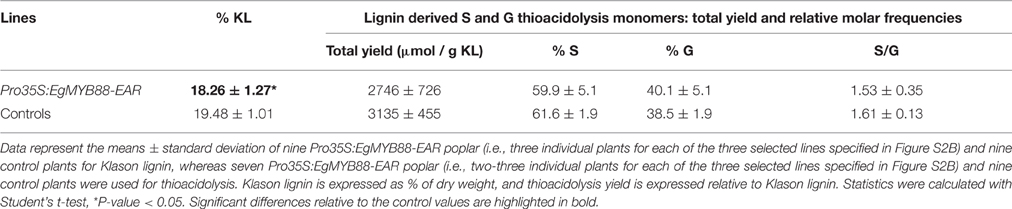
Table 3. Lignin content and structure in stems of Pro35S:EgMYB88-EAR poplar transgenic lines evaluated by Klason (KL) and thioacidolysis.
Overexpression of EgMYB88 and EgMYB88-EAR Have Distinct but Not Strictly Opposite Effects on Poplar Secondary Metabolism
We also analyzed the effects of EgMYB88 and EgMYB88-EAR on the profiles of soluble secondary metabolites in debarked stems of transgenic poplar lines. The Liquid Chromatography with photoDiode Array Detection–Mass Spectrometry (LC-DAD-MS) analysis of the water-methanol extracts revealed a complex composition of molecules absorbing at 280 nm. Among them, the 21 main different peaks were quantified, of which 14 could be attributed to a compound name or family (Figure S7).
The total content of phenolic secondary metabolites was increased by 12% in Pro35S:EgMYB88 plants compared to controls. This increase was mostly due to salicinoid phenolic glycosides and to the flavonoid catechin, although some unidentified compounds were also increased (Table 4, Table S4). The three salicinoid phenolic glycosides detected were tremulacin, salicortin, and salireposide, which increased by 87, 70, and 56%, respectively as compared to controls. The level of the flavonoid catechin was also strongly increased (about 2.6 fold compared to controls). In the Pro35S:EgMYB88-EAR poplar plants, the total content of secondary metabolites was not significantly different from controls, neither were the contents of phenolic glycosides (Table 5, Table S5). However, the contents of catechin and oligolignols were significantly reduced by about 40% and 18%, respectively. The oligolignols G(8-O-4)SP(8-5)G [coniferyl alcohol (8-O-4) sinapyl p-hydroxybenzoate (8-5) coniferyl alcohol], G(8-O-4)SP(8-5)G′ [coniferyl alcohol (8-O-4) sinapyl p-hydroxybenzoate (8-5) coniferaldehyde] and a putative oligolignol not yet identified, were reduced by 35, 36, and 24%, respectively.
The Transcript Levels of Phenylpropanoid Metabolism Genes Show Opposite Tendencies in EgMYB88 and EgMYB88-EAR Transgenic Poplars
To investigate the main modifications in gene expression induced by the overexpression of EgMYB88 and EgMYB88-EAR in transgenic poplars, we analyzed the transcript levels of key genes involved in the phenylpropanoid metabolism and in cambium activity and patterning by microfluidic qPCR. In general, as can be seen in the overall picture of Figure 8, Pro35S:EgMYB88 and Pro35S:EgMYB88-EAR showed opposed tendencies, meaning that genes induced in one transgenic type tend to be repressed in the other, and vice versa. However, whereas transcript abundance levels were clearly altered in Pro35S:EgMYB88, they were only moderately altered in Pro35S:EgMYB88-EAR plants (Tables 6, 7).
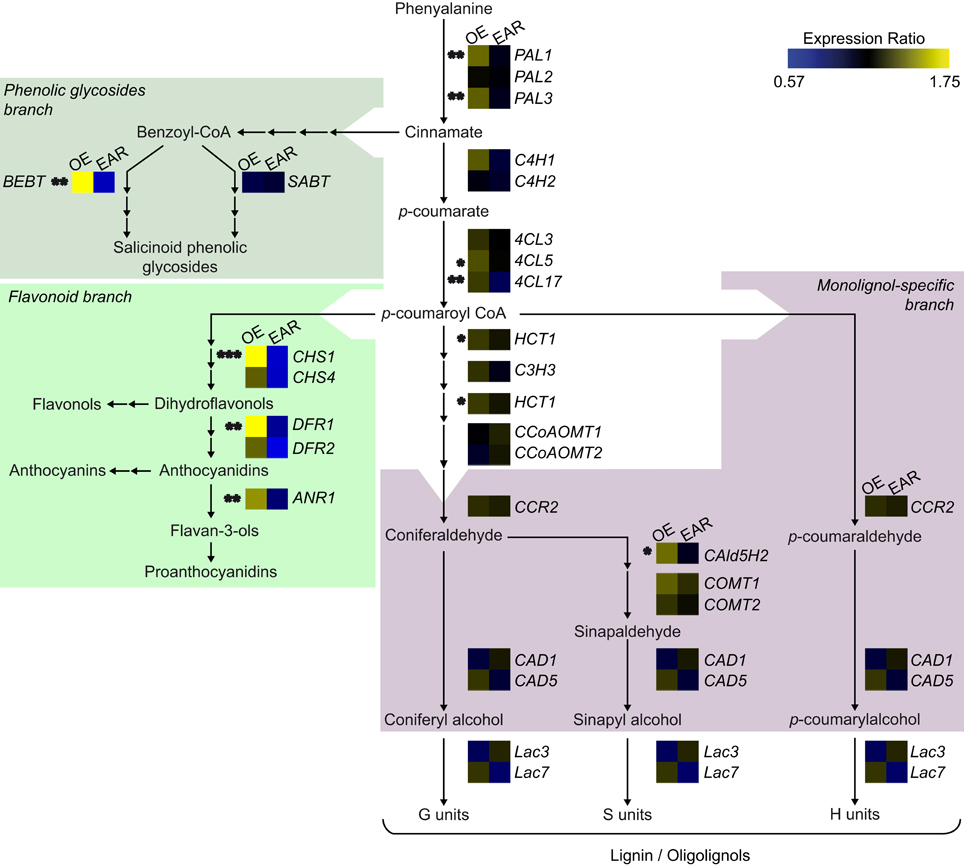
Figure 8. Transcript abundance of genes involved in the phenylpropanoid metabolism in transgenic poplars overexpressing EgMYB88 either as a native form or fused to an EAR motif. The genes are placed in a schematic representation of the pathways leading to lignin, flavonoids, and salicinoid phenolic glycosides, where relevant intermediates are shown (adapted from Takos et al., 2006; Peng et al., 2008; Jaakola, 2013; Carocha et al., 2015; Chedgy et al., 2015; Wang et al., 2015). Transcript abundance was expressed as a ratio of the abundance of a given gene in transgenic poplar plants, either overexpressing EgMYB88 (OE, shown at the left of the heatmap) or EgMYB88-EAR (EAR, shown at the right of the heat map), respective to its abundance in the corresponding controls. Values were calculated as the means of nine Pro35S:EgMYB88 (i.e., three plants for each of the three selected independent transgenic lines, specified in Figure S2A) and nine respective control poplar plants, or eight Pro35S:EgMYB88-EAR (i.e., two-three plants for each of the three selected independent transgenic lines, specified in Figure S2B) and seven respective control poplar plants. Statistical significance was calculated with Student's t-test, ***P-value < 0.001, **P-value < 0.01, *P-value < 0.05. Gene names, expression data, and orthologs in other species are detailed in Tables 6, 7, Table S1.
Most of the genes involved in the biosynthesis of phenylpropanoids, including those of lignin, flavonoids, and phenolic glycosides branches, were induced either significantly or at least showed a tendency to be up-regulated in Pro35S:EgMYB88 poplar plants (Figure 8, Table 6). In contrast, they were repressed in Pro35S:EgMYB88-EAR poplars although not significantly because of the high variation found among plants (Figure 8, Table 7). Interestingly, genes involved in the biosynthesis of flavonoids and salicinoid phenolic glycosides were the most induced ones in Pro35S:EgMYB88 poplars (Figure 8, Table 6). The gene encoding benzoyl-CoA:benzyl alcohol O-benzoyltransferase (BEBT), an enzyme involved in the biosynthesis of salicortin and other salicinoid phenolic glycosides (Babst et al., 2010; Chedgy et al., 2015) was the most induced (2.4 fold induction), in agreement with the strong accumulation of phenolic glycosides found in these lines. The genes encoding chalcone synthase (CHS), dihydroflavonol-4-reductase (DFR), and anthocyanidin reductase (ANR), involved in the biosynthesis of flavonoids, were also strongly induced (Figure 8, Table 6), in agreement with the increase in catechin levels. The genes from the general phenylpropanoid pathway (phenylalanine ammonia-lyase, PAL and 4-coumarate:CoA ligase, 4CL) were moderately induced in Pro35S:EgMYB88 poplars as was ferulate 5-hydroxylase (CAld5H), involved in the biosynthesis of sinapyl alcohol which generate S lignin units, in agreement with the increase in the S/G ratio.
Most of the genes regulating the cambial activity tested in this study were repressed or showed a tendency to be repressed in Pro35S:EgMYB88 poplars (Table 6), whereas they seemed to be induced in Pro35S:EgMYB88-EAR lines (Table 7). HB2/PopREV and HB4, respectively orthologs of Arabidopsis REVOLUTA and PHABULOSA/PHAVOLUTA (Zhu et al., 2013), and PIN1b, ortholog of Arabidopsis PIN1 (Liu et al., 2014b), were significantly repressed in EgMYB88 overexpressing poplars (22, 22, and 23% of repression, respectively). These genes are related to cambium activity and to the differentiation of vascular tissues in Arabidopsis (Gälweiler et al., 1998; Zhong and Ye, 1999) and poplar (Robischon et al., 2011).
Finally, we analyzed the transcript levels of the two poplar orthologs of EgMYB88: MYB11 and MYB171. Unexpectedly, the two genes exhibited opposite behaviors depending on the transgenic lines type. For example, in Pro35S:EgMYB88 plants, MYB171 was clearly repressed (35% repression), whereas MYB11 was not significantly affected (Table 6). In contrast, MYB171 was not significantly altered in Pro35S:EgMYB88-EAR plants whereas MYB11 was clearly induced (40% induction) (Table 7).
Discussion
Our study focused on the R2R3 MYB genes from subgroup WPS-I which are present preferentially in woody perennial plants exhibiting secondary growth (Soler et al., 2015). To the best of our knowledge, none of the genes belonging to this subgroup has been functionally characterized so far in any species. In Eucalyptus, the six genes of this subgroup have not been tandem-duplicated in contrast to most of the genes belonging to the woody-preferential and woody-expanded subgroups (Soler et al., 2015). Five of the six WPS-I Eucalyptus genes and the four poplar genes are targets of miR828, the same miR which targets R2R3 MYBs from several subgroups, many being involved in the regulation of the anthocyanin and the lignin pathways (Xia et al., 2012). Moreover, miR828 was also shown to target GhMYB2, which is involved in cotton fiber development (Guan et al., 2014). In Eucalyptus, miR828 belong to a cluster of miRs regulated during reaction wood formation (Carocha and Paiva, personal communication) suggesting that WPS-I genes can be controlled post-transcriptionally in response to a mechanical stress. In addition, the transcriptional activities of the WPS-I genes could be modulated through interactions with other proteins, as suggested by the presence of a well conserved bHLH interacting motif among all the genes from the subgroup. Indeed, many R2R3-MYBs interact with bHLH and WD40 proteins, thereby specifying their functions (Ramsay and Glover, 2005). Such interactions were shown to contribute to the tight regulation of the flavonoid biosynthetic genes (Xu et al., 2015).
The transcript profiles of all Eucalyptus MYBs from WPS-I revealed a high expression in the cambial region, reinforcing the idea that they may be involved in some aspects of wood formation. We selected EgrMYB88, which had the highest expression and was also preferentially expressed in this tissue. We showed that EgMYB88 acts as an autoactivator in yeast, suggesting that it is also a transcriptional activator in plants. We functionally characterized EgMYB88 in Arabidopsis and poplar, two model plants previously shown to be useful to decipher the function of Eucalyptus MYB genes (Legay et al., 2010). When overexpressed in Arabidopsis, either in its native form or fused to an EAR motif, EgMYB88 did not produce any phenotype evaluated either at the macroscopic, histologic or biochemical levels. This lack of phenotype could be expected since this gene is absent from the Brassicaceae, suggesting that EgMYB88 is not active in the herbaceous model Arabidopsis due to the lack of either appropriate DNA-binding elements in target genes and/or absence of protein partners necessary for the proper function of the gene. It is also possible that miR828 modulates EgMYB88 expression in the transgenic plants resulting in moderate phenotypes in poplar and no phenotypes in Arabidopsis.
In poplar plants, EgMYB88 is able to directly or indirectly activate the phenylpropanoid pathway, as shown by the accumulation of catechin and salicinoid phenolic glycosides in the wood-forming tissues of EgMYB88-overexpressing plants, and also by the induction of many phenylpropanoid biosynthetic genes. The regulation of the phenylpropanoid pathway is also supported by the phenotype of poplars overexpressing EgMYB88-EAR, showing a decrease of catechin levels and lowered transcript levels of many phenylpropanoid biosynthetic genes. However, the phenotype of poplars overexpressing EgMYB88-EAR is not exactly the opposite of that of poplars overexpressing EgMYB88, as the former did not show changes in the levels of salicinoid phenolic glycosides, but a reduction in the level of soluble oligolignols concomitant with a reduction of lignin level. Oligolignols are soluble oligomers constituted of monolignols that polymerize similarly to lignin (Morreel et al., 2010; Vanholme et al., 2010) and, in general, plants that produce less lignin show a concomitant reduction of their content in oligolignols (Morreel et al., 2004; Damiani et al., 2005). Interestingly, the oligolignols that were repressed in EgMYB88-EAR overexpressing poplars were those containing sinapyl p-hydroxybenzoate (SP) compounds, formed by the acylation of a sinapyl alcohol with a p-hydroxybenzoic acid prior to their incorporation to the growing lignin polymer. SP compounds are present in lignin from poplar as well as other plants (Morreel et al., 2004). The fact that EgMYB88-EAR overexpressing poplars contain a significant reduction of oligolignols deriving from benzoic acid, whereas EgMYB88 overexpressing plants show an important accumulation of salicinoid phenolic glycosides also deriving from benzoic acid suggests an unexpected link between these two phenylpropanoid branch pathways possibly mediated by EgMYB88.
Salicortin, tremulacin and salireposide are three salicinoid phenolic glycosides abundant in leaves and bark of Salicaceae, where they play a role as anti-herbivore defenses (Boeckler et al., 2011). They were shown to accumulate in the xylem ray cells of transgenic poplar silenced for laccase3, which was interpreted as a consequence of a defect in the polymerization of some phenolic compounds in the cell walls of neighboring xylem fibers without altering lignin levels (Ranocha et al., 2002). It is worth noting that recent studies have shown the incorporation of non-monolignol units in the lignin polymer. It is the case for instance of the flavonoid tricin in monocots (Lan et al., 2015). In poplar plants overexpressing EgMYB88, laccase3 showed a tendency to be down-regulated in contrast to many of the phenylpropanoid genes tested which were in general induced. The lignin content of these plants was not affected but lignin structure was altered with an increased S/G ratio.
The biosynthesis of the salicinoids is poorly understood but their phenylpropanoid origin has been confirmed (Tsai et al., 2011). Two BADH-type acyltransferases have recently been proposed to be involved in the biosynthesis of the salicinoids: The benzoyl-CoA:salicyl alcohol O-benzoyltransferase (SABT) and the benzoyl-CoA:benzyl alcohol O-benzoyltransferase (BEBT) (Chedgy et al., 2015). The transcript encoding BEBT was the most induced in the xylem of poplar overexpressing EgMYB88, while that encoding SABT was not affected. This result strongly supports the hypothesis that benzyl benzoate could be the preferred intermediate in the synthesis of salicortin, tremulacin, and salireposide in poplar as proposed by Chedgy et al. (2015). Since salicinoid phenolic glycosides are taxonomically limited to the Salicaceae, EgMYB88 is likely regulating the biosynthesis of other benzoic-acid derivative compounds within the phenylpropanoid metabolism in the cambial region of Eucalyptus.
Poplar lines overexpressing EgMYB88 showed a notable induction of genes encoding enzymes involved in the biosynthesis of flavonoids, CHS1, DFR1, and ANR1, in agreement with the high levels of catechin. Catechin is a flavonoid of the flavan-3-ol subfamily found in many vascular plants, and also widely found as part of proanthocyanidins. Flavonoids have many roles in plants, like conferring protection against UV-B and oxidative stress, protection against pathogens and herbivores, facilitating plant reproduction and fertility, improving the resistance to toxic metals (Falcone Ferreyra et al., 2012), and also interfering with the polar auxin transport (Peer and Murphy, 2007; Kuhn et al., 2011). Flavan-3-ols are considered as markers of undifferentiated callus-like cells and their accumulation have been correlated to a lack of cambium cell differentiation (reviewed by Treutter, 2010). Taking into account that polar auxin transport is thought to be necessary for cambial activity and vascular tissue formation (Schuetz et al., 2013), it is tempting to hypothesize that some R2R3-MYB genes like EgMYB88 could regulate the biosynthesis of some flavonoids to modify auxin transport in cambium and thus locally modify cambium activity in response to environmental factors (Soler et al., 2015). Supporting this hypothesis, genes presumably involved in polar auxin transport necessary for the cambium proliferation and differentiation of vascular tissues like PIN1b (Liu et al., 2014b), or in the cambium initiation and patterning of secondary vascular tissues like HB2/PopREV (Robischon et al., 2011), are repressed in EgMYB88 overexpressing poplars. It is also worth noting that Eucalyptus supplemented with flavonoids show an increase of the lignin S/G ratio (Lepikson-Neto et al., 2013), similarly found in EgMYB88 overexpressing poplar lines containing higher levels of catechin.
The two poplar orthologs of EgMYB88, which to the best of our knowledge have not been yet functionally characterized, showed unexpected opposite behaviors in the transgenic poplar lines. The transcript of MYB171 was clearly repressed when EgMYB88 was overexpressed, whereas that of MYB11 was induced when the dominant repressive version of EgMYB88 was overexpressed. It is possible that MYB11 and MYB171 have only partially overlapping targets in poplar, explaining why poplar plants overexpressing a dominant repression form of EgMYB88 (potentially strong repressor), did not show the opposite phenotypes of the ones overexpressing the native form (activator). One could hypothesize that MYB171 is involved in the regulation of the phenolic glycosides and flavonoids pathways, and its transcription could be regulated by a negative regulatory loop when the production of these compounds is too high or to counterbalance the induction of its target genes, as it happens in EgMYB88 overexpressing poplars. On the other hand, when EgMYB88-EAR is acting as a repressor, the transcript of MYB11 is induced possibly to counterbalance the repressive effects on its target genes. These compensatory mechanisms could explain why the transcripts levels of genes significantly induced in Pro35S:EgMYB88 exhibited only a tendency to be repressed in Pro35S:EgMYB88-EAR.
The regulation of the biosynthesis of phenylpropanoid-derived secondary metabolites and their relationship with lignin has not been yet properly studied in tissues like cambium and differentiating xylem. EgMYB88 is a R2R3-MYB gene belonging to WPS-I, a group of genes preferentially found in woody perennials exhibiting secondary growth (Soler et al., 2015). The highly preferential expression of EgMYB88 in vascular cambium makes it an outstanding candidate to study the regulation of wood formation. EgMYB88 behaves as a transcriptional activator in yeast, but when overexpressed in Arabidopsis plants, no phenotype was observed. In contrast, the analyses of the transgenic poplar plants overexpressing EgMYB88 indicate that this gene regulates the phenylpropanoid metabolism by controlling, either directly or indirectly, the biosynthesis of lignin, but also of flavonoids and salicinoid phenolic glycosides, mostly known for their protective roles. As genes involved in cambium proliferation and differentiation of vascular tissues are also altered in these transgenic poplar plants, it seems plausible that EgMYB88 contributes to adapt wood formation depending on environmental conditions. This is particularly important because woody plants need to face a myriad of complex stresses over their long lifespans. The characterization of this gene using a homologous transformation system, such as the Eucalyptus transgenic hairy roots recently set up in our lab (Plasencia et al., 2016), together with the identification of its direct target genes, will enable to more accurately specify its role in the cambial region.
Author Contributions
MS, AP, RL, and JG designed the research. MS, AP, JL, EC, AD, NL, FM, and RL performed research. MS, AP, JL, EP, RL, and JG analyzed data. MS, AP, RL, and JG wrote the article. All authors read, corrected, and approved the article.
Funding
This work was supported by the Centre National pour la Recherche Scientifique (CNRS), the University Paul Sabatier Toulouse III (UPS), the French Laboratory of Excellence project “TULIP” (ANR-10-LABX-41; ANR-11-IDEX-0002-02), and the TREEFORJOULES project (ANR-2010-KBBE-007-01). MS was supported by the postdoctoral fellowship “Beatriu de Pinós” (2009 BP-A 00185) from the Departament d'Universitats, Recerca i Societat de la Informació de la Generalitat de Catalunya and by grants from TREEFORJOULES and TULIP. AP by a PhD grant from the Ministère de l'Education Nationale, de l'Enseignement Supérieur et de la Recherche. JL by a postdoctoral fellowship from the Sao Paulo Research Foundation (FAPESP, 2013/17846-0). EC by a postdoctoral fellowship from CNPQ-Brazil (202228/2015-0).
Conflict of Interest Statement
The authors declare that the research was conducted in the absence of any commercial or financial relationships that could be construed as a potential conflict of interest.
Acknowledgments
The authors are grateful to A. Moreno and I. Allona (UPM/INIA, Madrid, Spain) for their help with poplar transformation, to H. Yu, A.-L Fonseca, A. Desplat, and M. Liu (LRSV, Castanet-Tolosan, France) for her help with sampling and transformant analysis, to Y. Martinez (FR3450, Castanet-Tolosan, France) for assistance with imaging, to A. Fauvet (INRA/UL, Vandoeuvre, France) for her precious help in the preparation of poplar methanolic extractions, to J. J. Molina-Rueda (UMA, Málaga, Spain) for his precious advice, and to J. Takahashi-Schmidt (UPSC, Umea, Sweden) for her guidance in Py-GC/MS.
Supplementary Material
The Supplementary Material for this article can be found online at: http://journal.frontiersin.org/article/10.3389/fpls.2016.01422
Abbreviations
4CL, 4-coumarate:CoA ligase; ACT2, actin2; Ade, Adenine; ANR, anthocyanidin reductase; AurA, Aureobasidin A; BD, Gal4 DNA binding domain; BEBT, benzoyl-CoA, benzyl alcohol O-benzoyltransferase; bHLH, basic Helix-Loop-Helix proteins; Cald5H, ferulate 5-hydroxylase; CDC2, cell division cycle2; CHS, chalcone synthase; DFR, dihydroflavonol-4-reductase; EAR, Ethylene-responsive element binding factor-associated Amphiphilic Repression motif; G units, guaiacyl lignin units; G(8-O-4)SP(8-5)G, coniferyl alcohol (8-O-4) sinapyl p-hydroxybenzoate (8-5) coniferyl alcohol; G(8-O-4)SP(8-5)G′, coniferyl alcohol (8-O-4) sinapyl p-hydroxybenzoate (8-5) coniferaldehyde; GC/MS, Gas Chromatography/Mass Spectrometry; H units, p-hydroxyphenyl lignin units; His, Histidine; HK1, HK3, and HK11, Housekeeping genes 1, 3, and 11; IBA, indole-3-butyric acid; KL, Klason lignin; LC-DAD-MS, Liquid Chromatography with photoDiode Array Detection-Mass Spectrometry; PAL, phenylalanine ammonia-lyase; Pro35S, 35S Cauliflower Mosaic Virus promoter; Py-GC/MS, Pyrolysis–Gas Chromatography/Mass Spectrometry; RT-qPCR, RetroTranscriptase-quantitative Polymerase Chain Reaction; S units, syringyl lignin units; SABT: benzoyl-CoA, salicyl alcohol O-benzoyltransferase; SD, synthetically defined medium used in the yeast autoactivation tests; SP, sinapyl p-hydroxybenzoate; Trp, Tryptophan; U-HPLC, Ultra-High Performance Liquid Chromatography; UBQ, Ubiquitin; WPS, Woody Preferential Subgroups; X-α-Gal, 5-bromo-4-chloro-3-indolyl alpha-D-galactopyranoside.
References
Aharoni, A., De Vos, C. H., Wein, M., Sun, Z., Greco, R., Kroon, A., et al. (2001). The strawberry FaMYB1 transcription factor suppresses anthocyanin and flavonol accumulation in transgenic tobacco. Plant J. 28, 319–332. doi: 10.1046/j.1365-313X.2001.01154.x
Akagi, T., Ikegami, A., and Yonemori, K. (2010). DkMyb2 wound-induced transcription factor of persimmon (Diospyros kaki Thunb.), contributes to proanthocyanidin regulation. Planta 232, 1045–1059. doi: 10.1007/s00425-010-1241-7
Albert, N. W., Davies, K. M., Lewis, D. H., Zhang, H., Montefiori, M., Brendolise, C., et al. (2014). A conserved network of transcriptional activators and repressors regulates anthocyanin pigmentation in eudicots. Plant Cell 26, 962–980. doi: 10.1105/tpc.113.122069
Arvidsson, S., Kwasniewski, M., Riaño-Pachón, D. M., and Mueller-Roeber, B. (2008). QuantPrime - a flexible tool for reliable high-throughput primer design for quantitative PCR. BMC Bioinformatics 9:465. doi: 10.1186/1471-2105-9-465
Babst, B. A., Harding, S. A., and Tsai, C. J. (2010). Biosynthesis of phenolic glycosides from phenylpropanoid and benzenoid precursors in populus. J. Chem. Ecol. 36, 286–297. doi: 10.1007/s10886-010-9757-7
Bailey, T. L., Boden, M., Buske, F. A., Frith, M., Grant, C. E., Clementi, L., et al. (2009). MEME SUITE: tools for motif discovery and searching. Nucleic Acids Res. 37(Web Server issue), W202–W208. doi: 10.1093/nar/gkp335
Berthet, S., Demont-Caulet, N., Pollet, B., Bidzinski, P., Cézard, L., Le Bris, P., et al. (2011). Disruption of LACCASE4 and 17 results in tissue-specific alterations to lignification of Arabidopsis thaliana stems. Plant Cell 23, 1124–1137. doi: 10.1105/tpc.110.082792
Boeckler, G. A., Gershenzon, J., and Unsicker, S. B. (2011). Phenolic glycosides of the Salicaceae and their role as anti-herbivore defenses. Phytochemistry 72, 1497–1509. doi: 10.1016/j.phytochem.2011.01.038
Bogs, J., Jaffé, F. W., Takos, A. M., Walker, A. R., and Robinson, S. P. (2007). The grapevine transcription factor VvMYBPA1 regulates proanthocyanidin synthesis during fruit development. Plant Physiol. 143, 1347–1361. doi: 10.1104/pp.106.093203
Carocha, V., Soler, M., Hefer, C., Cassan-Wang, H., Fevereiro, P., Myburg, A. A., et al. (2015). Genome-wide analysis of the lignin toolbox of Eucalyptus grandis. New Phytol. 206, 1297–1313. doi: 10.1111/nph.13313
Cassan-Wang, H., Goué, N., Saidi, M. N., Legay, S., Sivadon, P., Goffner, D., et al. (2013). Identification of novel transcription factors regulating secondary cell wall formation in Arabidopsis. Front. Plant Sci. 4:189. doi: 10.3389/fpls.2013.00189
Cassan-Wang, H., Soler, M., Yu, H., Camargo, E. L. O., Carocha, V., Ladouce, N., et al. (2012). Reference genes for high-throughput quantitative reverse transcription-PCR analysis of gene expression in organs and tissues of Eucalyptus grown in various environmental conditions. Plant Cell Physiol. 53, 2101–2116. doi: 10.1093/pcp/pcs152
Cavallini, E., Matus, J. T., Finezzo, L., Zenoni, S., Loyola, R., Guzzo, F., et al. (2015). The phenylpropanoid pathway is controlled at different branches by a set of R2R3-MYB C2 repressors in grapevine. Plant Physiol. 167, 1448–1470. doi: 10.1104/pp.114.256172
Chedgy, R. J., Köllner, T. G., and Constabel, C. P. (2015). Functional characterization of two acyltransferases from Populus trichocarpa capable of synthesizing benzyl benzoate and salicyl benzoate, potential intermediates in salicinoid phenolic glycoside biosynthesis. Phytochemistry 113, 149–159. doi: 10.1016/j.phytochem.2014.10.018
Clough, S. J., and Bent, A. F. (1998). Floral dip: a simplified method for Agrobacterium-mediated transformation of Arabidopsis thaliana. Plant J. 16, 735–743.
Damiani, I., Morreel, K., Danoun, S., Goeminne, G., Yahiaoui, N., Marque, C., et al. (2005). Metabolite profiling reveals a role for atypical cinnamyl alcohol dehydrogenase CAD1 in the synthesis of coniferyl alcohol in tobacco xylem. Plant Mol. Biol. 59, 753–769. doi: 10.1007/s11103-005-0947-6
Dubos, C., Stracke, R., Grotewold, E., Weisshaar, B., Martin, C., and Lepiniec, L. (2010). MYB transcription factors in Arabidopsis. Trends Plant Sci. 15, 573–581. doi: 10.1016/j.tplants.2010.06.005
Etchells, J. P., Mishra, L. S., Kumar, M., Campbell, L., and Turner, S. R. (2015). Wood formation in trees is increased by manipulating PXY-regulatedc division. Curr. Biol. 25, 1050–1055. doi: 10.1016/j.cub.2015.02.023
Falcone Ferreyra, M. L., Rius, S. P., and Casati, P. (2012). Flavonoids: biosynthesis, biological functions, and biotechnological applications. Front. Plant Sci. 3:222. doi: 10.3389/fpls.2012.00222
Gallardo, F., Fu, J., Canton, F. R., Garcia-Gutierrez, A., Canovas, F. M., and Kirby, E. G. (1999). Expression of a conifer glutamine synthetase gene in transgenic poplar. Planta 210, 19–26.
Gälweiler, L., Guan, C., Muller, A., Wisman, E., Mendgen, K., Yephremov, A., et al. (1998). Regulation of polar auxin transport by AtPIN1 in Arabidopsis vascular tissue. Science 282, 2226–2230.
Gerber, L., Eliasson, M., Trygg, J., Moritz, T., and Sundberg, B. (2012). Multivariate curve resolution provides a high-throughput data processing pipeline for pyrolysis-gas chromatography/mass spectrometry. J. Anal. Appl. Pyrol. 95, 95–100. doi: 10.1016/j.jaap.2012.01.011
Goicoechea, M., Lacombe, E., Legay, S., Mihaljevic, S., Rech, P., Jauneau, A., et al. (2005). EgMYB2, a new transcriptional activator from Eucalyptus xylem, regulates secondary cell wall formation and lignin biosynthesis. Plant J. 43, 553–567. doi: 10.1111/j.1365-313X.2005.02480.x
Guan, X., Pang, M., Nah, G., Shi, X., Ye, W., Stelly, D. M., et al. (2014). miR828 and miR858 regulate homoeologous MYB2 gene functions in Arabidopsis trichome and cotton fibre development. Nat. Commun. 5, 3050. doi: 10.1038/ncomms4050
Hiratsu, K., Matsui, K., Koyama, T., and Ohme-Takagi, M. (2003). Dominant repression of target genes by chimeric repressors that include the EAR motif, a repression domain, in Arabidopsis. Plant J. 34, 733–739. doi: 10.1046/j.1365-313X.2003.01759.x
Huang, Y. F., Vialet, S., Guiraud, J. L., Torregrosa, L., Bertrand, Y., Cheynier, V., et al. (2014). A negative MYB regulator of proanthocyanidin accumulation, identified through expression quantitative locus mapping in the grape berry. New Phytol. 201, 795–809. doi: 10.1111/nph.12557
Huang, Y., Gou, J., Jia, Z., Yang, L., Sun, Y., Xiao, X., et al. (2012). Molecular cloning and characterization of two genes encoding dihydroflavonol-4-reductase from Populus trichocarpa. PLoS ONE 7:e30364. doi: 10.1371/journal.pone.0030364
Jaakola, L. (2013). New insights into the regulation of anthocyanin biosynthesis in fruits. Trends Plant Sci. 18, 477–483. doi: 10.1016/j.tplants.2013.06.003
Katoh, K., Misawa, K., Kuma, K., and Miyata, T. (2002). MAFFT: a novel method for rapid multiple sequence alignment based on fast Fourier transform. Nucleic Acids Res. 30, 3059–3066. doi: 10.1093/nar/gkf436
Kuhn, B. M., Geisler, M., Bigler, L., and Ringli, C. (2011). Flavonols accumulate asymmetrically and affect auxin transport in Arabidopsis. Plant Physiol. 156, 585–595. doi: 10.1104/pp.111.175976
Lan, W., Lu, F., Regner, M., Zhu, Y., Rencoret, J., Ralph, S. A., et al. (2015). Tricin, a flavonoid monomer in monocot lignification. Plant Physiol. 167, 1284–1295. doi: 10.1104/pp.114.253757
Legay, S., Lacombe, E., Goicoechea, M., Brière, C., Séguin, A., Mackay, J., et al. (2007). Molecular characterization of EgMYB1, a putative transcriptional repressor of the lignin biosynthetic pathway. Plant Sci. 173, 542–549. doi: 10.1016/j.plantsci.2007.08.007
Legay, S., Sivadon, P., Blervacq, A. S., Pavy, N., Baghdady, A., Tremblay, L., et al. (2010). EgMYB1, an R2R3 MYB transcription factor from eucalyptus negatively regulates secondary cell wall formation in Arabidopsis and poplar. New Phytol. 188, 774–786. doi: 10.1111/j.1469-8137.2010.03432.x
Lepikson-Neto, J., Alves, A., Deckmann, A. C., Camargo, E. L. O., Salazar, M. M., Scatollin Rio, M. C., et al. (2013). Flavonoid supplementation reduces extractives content and increases S/G ratio on Eucalyptus grandis x Eucalyptus urophylla hybrid trees. BioResources 8, 1–11. doi: 10.1186/s12870-014-0301-8
Liu, B., Wang, L., Zhang, J., Li, J., Zheng, H., Chen, J., et al. (2014a). WUSCHEL-related Homeobox genes in Populus tomentosa: diversified expression patterns and a functional similarity in adventitious root formation. BMC Genomics 15:296. doi: 10.1186/1471-2164-15-296
Liu, B., Zhang, J., Wang, L., Li, J., Zheng, H., Chen, J., et al. (2014b). A survey of Populus PIN-FORMED family genes reveals their diversified expression patterns. J. Exp. Bot. 65, 2437–2448. doi: 10.1093/jxb/eru129
Méchin, V., Laluc, A., Legée, F., Cézard, L., Denoue, D., Barrière, Y., et al. (2014). Impact of the brown-midrib bm5 mutation on maize lignins. J. Agric. Food Chem. 62, 5102–5107. doi: 10.1021/jf5019998
Morreel, K., Dima, O., Kim, H., Lu, F., Niculaes, C., Vanholme, R., et al. (2010). Mass spectrometry-based sequencing of lignin oligomers. Plant Physiol. 153, 1464–1478. doi: 10.1104/pp.110.156489
Morreel, K., Ralph, J., Kim, H., Lu, F., Goeminne, G., Ralph, S., et al. (2004). Profiling of oligolignols reveals monolignol coupling conditions in lignifying poplar xylem. Plant Physiol. 136, 3537–3549. doi: 10.1104/pp.104.049304
Myburg, A. A., Grattapaglia, D., Tuskan, G. A., Hellsten, U., Hayes, R. D., Grimwood, J., et al. (2014). The genome of Eucalyptus grandis. Nature 510, 356–362. doi: 10.1038/nature13308
Oñate-Sánchez, L., and Vicente-Carbajosa, J. (2008). DNA-free RNA isolation protocols for Arabidopsis thaliana, including seeds and siliques. BMC Res. Notes 1:93. doi: 10.1186/1756-0500-1-93
Peer, W. A., and Murphy, A. S. (2007). Flavonoids and auxin transport: modulators or regulators? Trends Plant Sci. 12, 556–563. doi: 10.1016/j.tplants.2007.10.003
Peng, M., Hudson, D., Schofield, A., Tsao, R., Yang, R., Gu, H., et al. (2008). Adaptation of Arabidopsis to nitrogen limitation involves induction of anthocyanin synthesis which is controlled by the NLA gene. J. Exp. Bot. 59, 2933–2944. doi: 10.1093/jxb/ern148
Plasencia, A., Soler, M., Dupas, A., Ladouce, N., Silva-Martins, G., Martinez, Y., et al. (2016). Eucalyptus hairy roots, a fast, efficient and versatile tool to explore function and expression of genes involved in wood formation. Plant Biotechnol. J. 14, 1381–1393. doi: 10.1111/pbi.12502
Plomion, C., Leprovost, G., and Stokes, A. (2001). Wood formation in trees. Plant Physiol. 127, 1513–1523. doi: 10.1104/pp.010816
Ramsay, N. A., and Glover, B. J. (2005). MYB-bHLH-WD40 protein complex and the evolution of cellular diversity. Trends Plant Sci. 10, 63–70. doi: 10.1016/j.tplants.2004.12.011
Ranocha, P., Chabannes, M., Chamayou, S., Danoun, S., Jauneau, A., Boudet, A. M., et al. (2002). Laccase down-regulation causes alterations in phenolic metabolism and cell wall structure in poplar. Plant Physiol. 129, 145–155. doi: 10.1104/pp.010988
Robischon, M., Du, J., Miura, E., and Groover, A. (2011). The Populus class III HD ZIP, popREVOLUTA, influences cambium initiation and patterning of woody stems. Plant Physiol. 155, 1214–1225. doi: 10.1104/pp.110.167007
Rozen, S., and Skaletsky, H. (2000). Primer3 on the WWW for general users and for biologist programmers. Methods Mol. Biol. 132, 365–386. doi: 10.1385/1-59259-192-2:365
Saeed, A. I., Sharov, V., White, J., Li, J., Liang, W., Bhagabati, N., et al. (2003). TM4: a free, open-source system for microarray data management and analysis. BioTechniques 34, 374–378.
Salvatierra, A., Pimentel, P., Moya-León, M. A., and Herrera, R. (2013). Increased accumulation of anthocyanins in Fragaria chiloensis fruits by transient suppression of FcMYB1 gene. Phytochemistry 90, 25–36. doi: 10.1016/j.phytochem.2013.02.016
Schuetz, M., Smith, R., and Ellis, B. (2013). Xylem tissue specification, patterning, and differentiation mechanisms. J. Exp. Bot. 64, 11–31. doi: 10.1093/jxb/ers287
Shimada, T. L., Shimada, T., and Hara-Nishimura, I. (2010). A rapid and non-destructive screenable marker, FAST, for identifying transformed seeds of Arabidopsis thaliana. Plant J. 61, 519–528. doi: 10.1111/j.1365-313X.2009.04060.x
Shi, R., Sun, Y. H., Li, Q., Heber, S., Sederoff, R., and Chiang, V. L. (2010). Towards a systems approach for lignin biosynthesis in Populus trichocarpa: transcript abundance and specificity of the monolignol biosynthetic genes. Plant Cell Physiol. 51, 144–163. doi: 10.1093/pcp/pcp175
Sixto, H., González-González, B. D., Molina-Rueda, J. J., Garrido-Aranda, A., Sanchez, M. M., López, G., et al. (2016). Eucalyptus spp. and Populus spp. coping with salinity stress: an approach on growth, physiological and molecular features in the context of short rotation coppice (SRC). Trees. doi: 10.1007/s00468-016-1420-7
Sjödin, A., Street, N. R., Sandberg, G., Gustafsson, P., and Jansson, S. (2009). The Populus Genome Integrative Explorer (PopGenIE): a new resource for exploring the Populus genome. New Phytol. 182, 1013–1025. doi: 10.1111/j.1469-8137.2009.02807.x
Soler, M., Camargo, E. L., Carocha, V., Cassan-Wang, H., San Clemente, H., Savelli, B., et al. (2015). The Eucalyptus grandis R2R3-MYB transcription factor family: evidence for woody growth-related evolution and function. New Phytol. 206, 1364–1377. doi: 10.1111/nph.13039
Soler, M., Plasencia, A., Larbat, R., Pouzet, C., Jauneau, A., Rivas, S., et al. (2016). The Eucalyptus linker histone variant EgH1.3 cooperates with the transcription factor EgMYB1 to control wood formation. New phytol. doi: 10.1111/nph.14129. [Epub ahead of print].
Southerton, S. G., Marshall, H., Mouradov, A., and Teasdale, R. D. (1998). Eucalypt MADS-box genes expressed in developing flowers. Plant Physiol. 118, 365–372. doi: 10.1104/pp.118.2.365
Stracke, R., Werber, M., and Weisshaar, B. (2001). The R2R3-MYB gene family in Arabidopsis thaliana. Curr. Opin. Plant. Biol. 4, 447–456. doi: 10.1016/S1369-5266(00)00199-0
Sundell, D., Mannapperuma, C., Netotea, S., Delhomme, N., Lin, Y. C., Sjödin, A., et al. (2015). The plant genome integrative explorer resource: plantGenIE.org. New Phytol. 208, 1149–1156. doi: 10.1111/nph.13557
Sun, Y., Tian, Q., Yuan, L., Jiang, Y., Huang, Y., Sun, M., et al. (2011). Isolation and promoter analysis of a chalcone synthase gene PtrCHS4 from Populus trichocarpa. Plant Cell Rep. 30, 1661–1671. doi: 10.1007/s00299-011-1075-1
Takos, A. M., Jaffé, F. W., Jacob, S. R., Bogs, J., Robinson, S. P., and Walker, A. R. (2006). Light-induced expression of a MYB gene regulates anthocyanin biosynthesis in red apples. Plant Physiol. 142, 1216–1232. doi: 10.1104/pp.106.088104
Tamura, K., Peterson, D., Peterson, N., Stecher, G., Nei, M., and Kumar, S. (2011). MEGA5: molecular evolutionary genetics analysis using maximum likelihood, evolutionary distance, and maximum parsimony methods. Mol. Biol. Evol. 28, 2731–2739. doi: 10.1093/molbev/msr121
Treutter, D. (2010). Managing phenol contents in crop plants by phytochemical farming and breeding—visions and constraints. Int. J. Mol. Sci. 11, 807–857. doi: 10.3390/ijms11030807
Tsai, C. J., Guo, W., Babst, B., Nyamdari, B., Yuan, Y., Payyavula, R., et al. (2011). Salicylate metabolism in Populus. BMC Proc. 5(Suppl. 7):I9. doi: 10.1186/1753-6561-5-S7-I9
Vanholme, R., Cesarino, I., Rataj, K., Xiao, Y., Sundin, L., Goeminne, G., et al. (2013). Caffeoyl shikimate esterase (CSE) is an enzyme in the lignin biosynthetic pathway in Arabidopsis. Science 341, 1103–1106. doi: 10.1126/science.1241602
Vanholme, R., Demedts, B., Morreel, K., Ralph, J., and Boerjan, W. (2010). Lignin biosynthesis and structure. Plant Physiol. 153, 895–905. doi: 10.1104/pp.110.155119
Wang, P., Dudareva, N., Morgan, J. A., and Chapple, C. (2015). Genetic manipulation of lignocellulosic biomass for bioenergy. Curr. Opin. Chem. Biol. 29, 32–39. doi: 10.1016/j.cbpa.2015.08.006
Waterhouse, A. M., Procter, J. B., Martin, D. M. A., Clamp, M., and Barton, G. J. (2009). Jalview Version 2-a multiple sequence alignment editor and analysis workbench. Bioinformatics 25, 1189–1191. doi: 10.1093/bioinformatics/btp033
Xia, R., Zhu, H., An, Y., Beers, E. P., and Liu, Z. (2012). Apple miRNAs and tasiRNAs with novel regulatory networks. Genome Biol. 13:R47. doi: 10.1186/gb-2012-13-6-r47
Xu, W., Dubos, C., and Lepiniec, L. (2015). Transcriptional control of flavonoid biosynthesis by MYB-bHLH-WDR complexes. Trends Plant Sci. 20, 176–185. doi: 10.1016/j.tplants.2014.12.001
Yoshida, K., Ma, D., and Constabel, C. P. (2015). The MYB182 protein down-regulates proanthocyanidin and anthocyanin biosynthesis in poplar by repressing both structural and regulatory flavonoid genes. Plant Physiol. 167, 693–710. doi: 10.1104/pp.114.253674
Zhong, R., and Ye, Z. H. (1999). IFL1, a gene regulating interfascicular fiber differentiation in Arabidopsis, encodes a homeodomain-leucine zipper protein. Plant Cell 11, 2139–2152.
Zhu, Y., Song, D., Sun, J., Wang, X., and Li, L. (2013). PtrHB7, a class III HD-Zip gene, plays a critical role in regulation of vascular cambium differentiation in Populus. Mol. Plant 6, 1331–1343. doi: 10.1093/mp/sss164
Keywords: vascular cambium, MYB transcription factors, phenylpropanoid metabolism, lignin, oligolignols, flavonoids, salicinoid phenolic glycosides, Eucalyptus
Citation: Soler M, Plasencia A, Lepikson-Neto J, Camargo ELO, Dupas A, Ladouce N, Pesquet E, Mounet F, Larbat R and Grima-Pettenati J (2016) The Woody-Preferential Gene EgMYB88 Regulates the Biosynthesis of Phenylpropanoid-Derived Compounds in Wood. Front. Plant Sci. 7:1422. doi: 10.3389/fpls.2016.01422
Received: 04 July 2016; Accepted: 06 September 2016;
Published: 22 September 2016.
Edited by:
Thomas Vogt, Leibniz Institute of Plant Biochemistry, GermanyReviewed by:
Akiyoshi Kawaoka, Nippon Paper Industries Co., Ltd., JapanAnton R. Schäffner, Helmholtz Zentrum München, Germany
Christin Fellenberg, University of Victoria, Canada
Copyright © 2016 Soler, Plasencia, Lepikson-Neto, Camargo, Dupas, Ladouce, Pesquet, Mounet, Larbat and Grima-Pettenati. This is an open-access article distributed under the terms of the Creative Commons Attribution License (CC BY). The use, distribution or reproduction in other forums is permitted, provided the original author(s) or licensor are credited and that the original publication in this journal is cited, in accordance with accepted academic practice. No use, distribution or reproduction is permitted which does not comply with these terms.
*Correspondence: Jacqueline Grima-Pettenati, grima@lrsv.ups-tlse.fr
†Present Address: Jorge Lepikson-Neto, Laboratório de Genomica e Expressão, Universidade Estadual de Campinas, Campinas, Brazil
Edouard Pesquet, Arrhenius Laboratories, Department of Ecology, Environment and Plant Sciences, Stockholm, Sweden