- 1Oil Crops Research Institute of the Chinese Academy of Agricultural Sciences, Key Laboratory of Biology and Genetic Improvement of Oil Crops, Ministry of Agriculture, Wuhan, China
- 2Key Laboratory of Molecular Biology and Gene Engineering of Jiangxi Province, College of Life Science, Nanchang University, Nanchang, China
The trihelix family genes have important functions in light-relevant and other developmental processes, but their roles in response to adverse environment are largely unclear. In this study, we identified a new gene, BnSIP1-1, which fell in the SIP1 (6b INTERACTING PROTEIN1) clade of the trihelix family with two trihelix DNA binding domains and a fourth amphipathic α-helix. BnSIP1-1 protein specifically targeted to the nucleus, and its expression can be induced by abscisic acid (ABA) and different stresses. Overexpression of BnSIP1-1 improved seed germination under osmotic pressure, salt, and ABA treatments. Moreover, BnSIP1-1 decreased the susceptibility of transgenic seedlings to osmotic pressure and ABA treatments, whereas there was no difference under salt stress between the transgenic and wild-type seedlings. ABA level in the transgenic seedlings leaves was higher than those in the control plants under normal condition. Under exogenous ABA treatment and mannitol stress, the accumulation of ABA in the transgenic plants was higher than that in the control plants; while under salt stress, the difference of ABA content before treatment was gradually smaller with the prolongation of salt treatment time, then after 24 h of treatment the ABA level was similar in transgenic and wild-type plants. The transcription levels of several general stress marker genes (BnRD29A, BnERD15, and BnLEA1) were higher in the transgenic plants than the wild-type plants, whereas salt-responsive genes (BnSOS1, BnNHX1, and BnHKT) were not significantly different or even reduced compared with the wild-type plants, which indicated that BnSIP1-1 specifically exerted different regulatory mechanisms on the osmotic- and salt-response pathways in seedling period. Overall, these findings suggested that BnSIP1-1 played roles in ABA synthesis and signaling, salt and osmotic stress response. To date, information about the involvement of the Brassica napus trihelix gene in abiotic response is scarce. Here, we firstly reported abiotic stress response and possible function mechanisms of a new trihelix gene in B. napus.
Introduction
The trihelix transcription factor family is named after its conserved DNA binding domain which contains three tandem helices (helix-loop-helix-loop-helix). Phylogenetic analysis has identified several classes of trihelix transcription factors based on these features, including the GT-1, GT-2, SH4, GTγ and SIP1 subfamilies (Kaplan-Levy et al., 2012). The trihelix transcription factor family has 28 members in the Arabidopsis genome (Riechmann et al., 2000) and 22 members in the rice genome (Riano-Pachon et al., 2007). To date, there is no report about members of the trihelix family in rapeseeds (Brassica napus L.).
Early studies on the trihelix family focused only on their functions in the regulation of light-responsive genes (Gilmartin et al., 1992; Perisic and Lam, 1992; Kaplan-Levy et al., 2012). However, recent studies indicated that the trihelix family also played important roles in growth and developmental processes and in response to abiotic and biotic stresses (Li et al., 2008, 2015; Kaplan-Levy et al., 2012, 2014; Song et al., 2016; Wang et al., 2016; Zheng et al., 2016). Abiotic stresses affects plant growth and development and limits the productivity of crops (Boyer, 1982). To cope with various environmental stresses, plants have evolved various adjustment mechanisms to defend and adapt to stressful conditions, including the accumulation of osmoprotectants and the activation of stress-responsive pathways (Xiong et al., 2002). Several trihelix transcription factors have been shown to participate in the abiotic stress response (Kaplan-Levy et al., 2012; Qin et al., 2014). For example, Xie et al. (2009) showed that the constitutive expression of either GmGT-2A or GmGT-2B improved plant tolerance to salt, freezing and osmotic stress in transgenic Arabidopsis plants. Furthermore, other reports showed that the heterologous expression of GT-3b (from soybean) or GTγ-1-3-like1 (from rice) improved osmotic stress tolerance in Arabidopsis (Park et al., 2004; Fang et al., 2010), while the heterologous expression of PtaGTL1 (from Poplar) in Arabidopsis also enhanced the plants’ tolerance to drought (Weng et al., 2012). One member GT-4 was identified to improve salt tolerance of Arabidopsis by interacting with TEM2 and then co-regulates the salt responsive gene Cor15A (Wang et al., 2014). More recently, Zheng et al. (2016) reported the wheat GT Factor TaGT2L1D negatively regulated osmotic stress tolerance and plant development. Song et al. (2016) and Wang et al. (2016) characterized 20 and 56 full-length trihelix genes in Chrysanthemum and Populus trichocarpa, respectively. Expression profiling analysis of these genes indicated most of them were significantly induced by abiotic and biotic stress (Song et al., 2016; Wang et al., 2016).
Brassica napus is one of the most important oilseed crops in the production of animal feed and vegetable oil. Among the major food crops, Brassica crops are easily affected by drought or osmotic stress and salinity, since they are mainly grown in arid and semiarid areas. Therefore, rapeseeds growth and seed yield have greatly decreased owing to drought and salinity. It’s urgent to develop tolerant B. napus cultivars to ensure yield under such adverse conditions. Although great progress has been made in the understanding of the genetic mechanisms of osmotic and salt stress tolerance in the model plants Arabidopsis and rice (Nakashima et al., 2009; Fukao and Xiong, 2013), studies on the molecular mechanisms of stress tolerance in Brassica crops have made few advances. In recent years, the major advancements in next-generation sequencing technology have accelerated the batch identification of abiotic response genes. Several studies have identified hundreds of genes that are differentially expressed in response to osmotic or salt stress treatment using RNA-seq and proteomic methods, however, only a very few candidate genes were confirmed to function in salt or osmotic stress tolerance by forward and reverse genetic approaches (Zhang et al., 2014).
In this study, we characterized a new gene, BnSIP1-1, which belongs to the SIP1 subfamily of the trihelix transcription factor family. SIP1 clade was named after the first member of this subfamily which was identified through being bound to the Agrobacterium tumefaciens 6b oncogenic protein, and was named SIP1 (Kitakura et al., 2002; Kaplan-Levy et al., 2012). The new gene in our study is the first SIP1 clade gene identified in B. napus, so we named it as BnSIP1-1. The expression of BnSIP1-1 was induced by osmotic stress, salt and ABA. Interesting, overexpression of BnSIP1-1 enhanced the plants tolerance to osmotic and salt stress during the germination period, by contrast, during the seedling period, the transgenic plants exhibited only osmotic stress tolerance but not salt tolerance. In addition, our data also revealed that BnSIP1-1 functions in ABA synthesis and signaling. To date, information about the involvement of the B. napus trihelix gene in abiotic response is scarce. Here, we firstly investigate abiotic stress response and possible function mechanisms of a new trihelix gene in B. napus.
Materials and Methods
Plant Materials
Brassica napus cv. ZhongShuang 6 (ZS6), which is an elite Chinese cultivar, and the B. napus transgenic plants were grown in Pindstrup soil mix (Denmark) or 1/2 MS medium in a greenhouse at 22°C with 16 h light/8 h dark cycle, 40–65% humidity, and light intensity of 8,000 lux. Mature seeds of these plants were collected for experimental use.
Cloning and Sequence Analysis of BnSIP1-1
The full-length BnSIP1-1 cDNA was isolated by PCR amplification of cDNA prepared from the mRNA of ZS6 leaves. The primers were listed in Supplementary Table S1. The resulting sequences and the predicted BnSIP1-1 protein sequence were analyzed using BLAST software1. The protein structure regions were predicted by PSIPRED 3.32 (Sadowski and Jones, 2009) and the multiple protein alignment was performed using CLUSTALW23 (Li et al., 2013).
Transient Transformation and Fluorescence Microscopy Observation
The coding sequence of BnSIP1-1 was amplified using primers BnSIP1-1(3; Supplementary Table S1) for transient expression in onion epidermal cells. The PCR products were subcloned into PUC-35S-GFP expression vector under the control of the CaMV 35S promoter. The PUC-35S-GFP vector was transiently expressed in onion epidermal cells using a gene gun (PDS-1000, Bio-Rad, USA). Fluorescence was observed using a fluorescence microscopy (DM2500, Leica, Germany) after incubation at 25°C for 18 h on MS medium. Fluorophores were excited using an argon laser at 488 nm (GFP), and bright-field images were collected using a transmitted light detector.
Stable Transformation and Generation of Transgenic Plants
To generate the transgenic plants, the CDS of BnSIP1-1 was amplified from cDNA isolated from ZS6 leaves using BnSIP1-1(2) primers (Supplementary Table S1) by reverse transcription PCR, and then subcloned into the PBI121S vector under the CaMV 35S promoter and a terminal poly A sequence (Liu et al., 2014). The 35S:BnSIP1-1 recombinant vector was introduced into Agrobacterium tumefaciens GV3101 by electroporation, and positive clones were selected on LB agar plates at 28°C, supplemented with appropriate concentrations of antibiotics (gentamicin 50 mg L-1, rifampicin 50 mg L-1 and kanamycin 50 mg L-1) and verified using PCR. A single positive colony was used to transform B. napus (ZS6). The transgenic B. napus plants were generated by agrobacterium-mediated method as previously reported (Liu et al., 2014). In total, there were 22 independent T0 generation of transgenic lines generated, and 77% were positive transformants as confirmed by PCR with a forward primer designed from the CaMV 35S sequence and a reverse primer designed from the BnSIP1-1 sequence. Positive T3 generation transgenic plants were grown for phenotypic identification.
Phenotype Identification
For the salt, osmotic or ABA stress tolerance assay of the transgenic B. napus during germination period, the wild-type and T3 transgenic B. napus seeds were planted on plates filled with three-layer filter paper containing 8 ml of mannitol (400 and 600 mM), NaCl (150 and 200 mM), ABA (50 μM ABA and 100 μM ABA) or distilled water without any additions for 4 days. The germination rate was calculated every 12 h. Observations were made and images were acquired using a camera.
For the salt and osmotic stress assay of the transgenic B. napus during seedling period, the wild-type and transgenic seeds were germinated on wet filter paper for 7 days, and then transferred to liquid 1/2 MS medium under a 16 h light/8 h dark cycle at 25°C for 8 days. Finally, the 15-day-old seedlings were transferred to liquid 1/2 MS supplemented medium with NaCl solution (150 or 200 mM) or mannitol solution (300 or 600 mM). The control seedlings were transferred to liquid 1/2 MS medium supplemented without any additions. Photographs were taken after 6 h of treatment with 600 mM mannitol and 9 days of treatment with 300 mM mannitol. The chlorophyll contents of the wild-type and transgenic seedlings were detected after 9 days of treatment with 300 mM mannitol. Total chlorophyll was isolated using an extraction solution (2:1 acetone:95% alcohol), and the chlorophyll a and b contents were measured at 646.6, 663.6, and 750 nm using a spectrophotometer (Lambda, PerkinElmer, USA) according to previously described methods (Porra et al., 1989; Mishra et al., 2008).
For the ABA sensitivity assay during seedling period, the wild-type and transgenic B. napus seeds were sown in liquid 1/2 MS medium or soil with or without 1 μM ABA or 10 μM ABA. Photographs were taken after 12 days of plating on liquid 1/2 MS medium or 6 days of sowing in soil (Travaglia et al., 2010). The length of stem and root, and fresh weight of aboveground part and root of the wild-type and transgenic plants were measured after 12 days of treatment.
Real-Time Pcr Assay
RNA extraction and the first-strand cDNA synthesis were performed using an RNAprep Pure Plant Kit (TIANGEN BIOTECH, Beijing, China) and FastQuant RT Kit (with gDNase; TIANGEN BIOTECH, Beijing, China) according to the manufacturers’ instructions. Real-time PCR was performed using fivefold diluted cDNA and a SYBR Green I kit (Bio-Rad, Hercules, CA, USA) on the CFX96 real-time PCR platform (Bio-Rad, Hercules, CA, USA). Three independent biological replicates and three technical replicates for each biological replicate were run and the significance was determined through t-test of SPSS statistical software (p < 0.05). The B. napus β-actin gene (accession No. AF111812.1) was used as an internal reference control. Quantitative analysis was performed using the ΔΔCt comparative method (Livak and Schmittgen, 2001).
For the tissue specific analysis, the roots, leaves, and stems were harvested from randomly selected 2-month-old plants; and the pollens, stigmas, petals and immature seeds were collected from plants during reproduction period. For exploring the response of BnSIP1-1 to different abiotic stresses, the leaves and roots of 15-day-old seedlings (under 50 μM ABA, 200 mM NaCl, or 300 mM mannitol for 0, 1, 2, 4, 8, 12, and 24 h of treatments) were harvested for QPCR analysis. For detection of BnSIP1-1 expression of transgenic B. napus, the leaves of different independent transgenic lines were harvested. The leaves of 15-day-old transgenic and wild-type seedlings (under normal condition or treated with 50 μM ABA, 200 mM NaCl, or 300 mM mannitol for 0, 1, 6, 48, and 72h) were harvested for detection of the expression of stress-related genes (BnRD29A, BnERD15, BnLEA1, BnNAC485, BnCIPK6, BnSOS1, BnNHX1, BnKIN1, BnHKT, and BnABI5). The corresponding specific primers were listed in Supplementary Table S1.
Determination of ABA Content
For ABA content measurement, the leaves of 15-day-old transgenic and wild-type seedlings (under normal condition or treated with 50 μM ABA, 200 mM NaCl, or 300 mM mannitol for 0, 1, 6, and 24 h) were harvested. Quantification of endogenous ABA was performed as described (Chen M. et al., 2012).
Statistical Analysis
All of the expression, phenotypic and quantitative experiments have been replicated three times and data have been presented as mean ± SD (standard deviation). Significant variations between genotypes or treatments were evaluated statistically by Student’s t-test or one-way ANOVA (Sokal and Rohlf, 1995). Mean values that were significantly different (p < 0.05) from each other are marked with asterisks or different lowercase letters inside the figures.
Results
Sequence Analysis of BnSIP1-1
The full-length gene sequence of BnSIP1-1 was amplified from B. napus to analyze the structural properties. This analysis suggested that BnSIP1-1 has only one exon. The ORF of BnSIP1-1 is 924 bp long and encodes a predicted protein of 307 amino acids. Its predicted molecular mass and isoelectric point were 34.6 kDa and 9.30, respectively. Similarity searches revealed that BnSIP1-1 has 100% overall amino acid identity with a predicted gene product having an unknown function (accession No. CDX73485) in B. napus, 96% identity with an uncharacterized protein (accession No. XP_009116124) from B. rapa, 83% identity with an unknown protein (accession No. XP_006403581.1) from Eutrema salsugineum, and 26 and 19% identity with Arabidopsis ASIL1 (accession No. NP_564648.1) and ASIL2 (accession No. OAP02717.1), respectively. Analyses of the helix structure of the predicted protein suggested BnSIP1-1 protein had the classical conserved secondary structure of the SIP1 clade of the trihelix family, including the N-terminal trihelix domain, the fourth amphipathic α-helix, and the long, uninterrupted α-helical (Figure 1). Moreover, the three conserved amino acids in the three individual amphipathic α-helices of the N-terminal trihelix were also observed in BnSIP1-1, including two tryptophans (W) and one isoleucine (I). Overall, these results suggested that the BnSIP1-1 gene isolated in this study was a member of the SIP1 clade of the trihelix family.
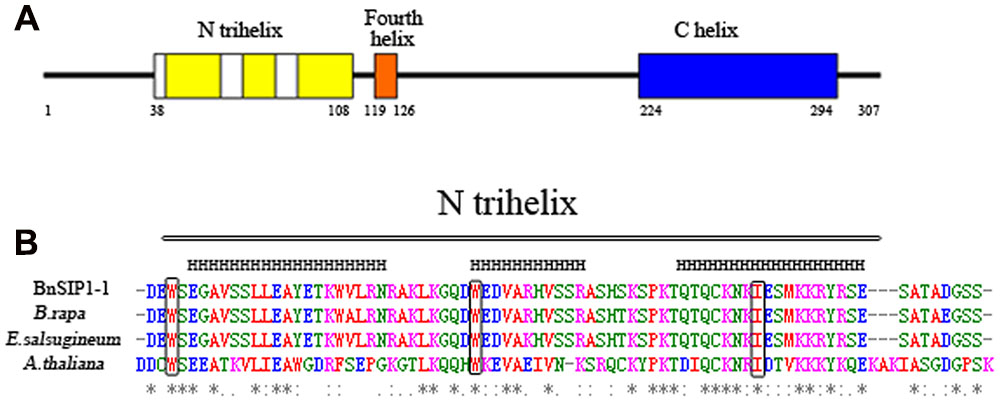
FIGURE 1. Conserved domain in the deduced BnSIP1-1 protein. (A) Structure of the deduced BnSIP1-1 protein of Brassica napus. The trihelix DNA binding domains are shown in yellow. The fourth amphipathic a-helix is shown in orange. The long, uninterrupted α-helical sequence in the C terminus is shown in blue. (B) Amino acid alignments of the conserved domain trihelix in BnSIP1-1 with orthologous sequences from B. rapa (accession No. XP_009116124), Eutrema salsugineum (accession No. XP_006403581.1) and Arabidopsis thaliana ASIL1 (accession No. NP_564648.1). The conserved tryptophan (or isoleucine) in each helix is indicated by the boxes. The asterisks denote the conserved amino acids.
Subcellular Localization of the BnSIP1-1 Protein
To further confirm the localization of the BnSIP1-1 gene product, the BnSIP1-1 gene was cloned the downstream of the CaMV (constitutive Cauliflower mosaic virus) 35S promoter of pCAMBIA1301-GFP vector and upstream of the GFP gene to create the 35S:BnSIP1-1-GFP fusion construct. The positive colonies were identified by PCR (Supplementary Figure S1). The BnSIP1-1-GFP fusion protein was transiently expressed in onion epidermal cells. Confocal microscopy analysis revealed that the BnSIP1-1 gene product was localized exclusively in the nucleus of cells, whereas the GFP control was found throughout the entire cell (Figure 2).
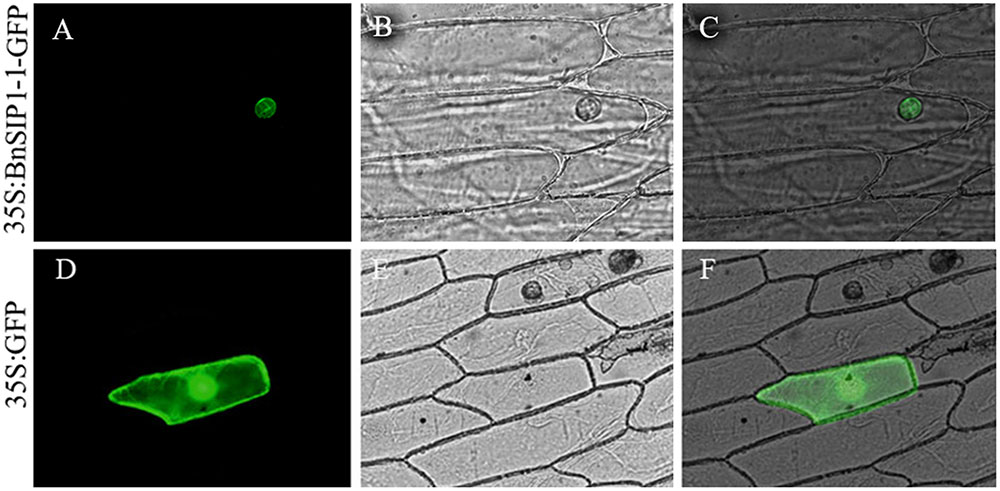
FIGURE 2. Subcellular localization of the BnSIP1-1 protein. Onion cells were transformed with the 35S:BnSIP1-1-GFP (A–C) and 35S:GFP (D–F) constructs. (A,D) Nuclear localization of BnSIP1-1-GFP and GFP photographed in the dark for green fluorescence. (B,E) The same cells as in (A,D) with bright light. (C,F) The merged images of (A,B), and (D,E), respectively. GFP and BnSIP1-1-GFP fusion proteins were under the control of the CaMV 35S promoter.
BnSIP1-1 Is Ubiquitously Expressed in B. napus Tissues
The trihelix family genes play important roles in different development processes and growth conditions (Kaplan-Levy et al., 2012). To determine the expression pattern of BnSIP1-1 in different B. napus tissues, QPCR was conducted using mRNA which was isolated from different tissues of the wild-type. The results showed that BnSIP1-1 was expressed in all of the examined tissues, including the roots, stems, leaves, pollens, stigmas, petals and immature seeds, with high expression levels in the roots, leaves, pollen and petals (Figure 3).
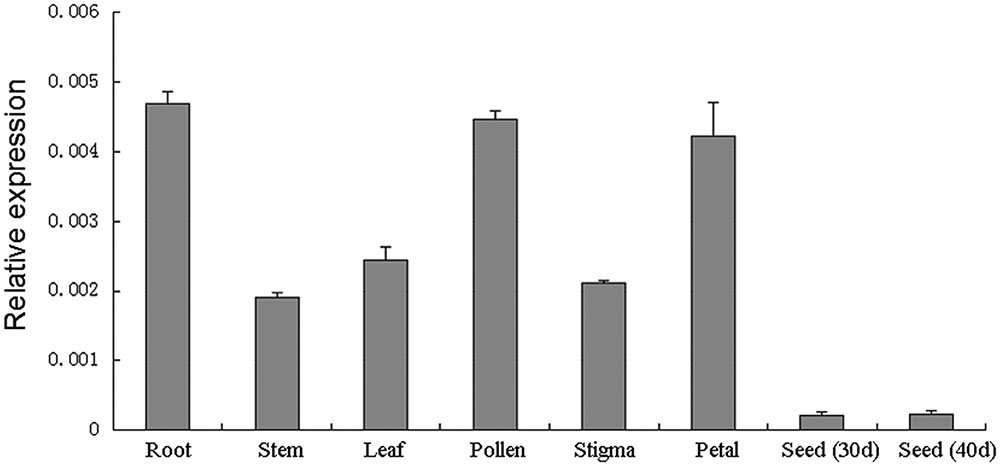
FIGURE 3. Expression patterns of BnSIP1-1 in different tissues in B. napus using QPCR analysis. The y-axis represents the relative fold difference in mRNA level and was calculated using the ΔΔCt method with BnACTIN as the internal control. The vertical bars indicate ± SD of four replicates on one sample. Three biological experiments were performed, which produced similar results.
ABA, Salt and Osmotic Stress Induce the Expression of BnSIP1-1
In order to explore the response of the BnSIP1-1 gene to various abiotic stresses, QPCR was used to analyze the BnSIP1-1 gene expression pattern in leaves and roots of 15-day-old seedlings under ABA, NaCl, and mannitol treatments (Figure 4). As shown in Figure 4A, the BnSIP1-1 transcript was remarkably enhanced in the leaves by ABA treatment, which amounted to an approximate threefold increase at the 1 h time point relative to the untreated control plants and reached the highest expression (an approximately fourfold increase) after 12 h of ABA treatment. Under NaCl treatment, BnSIP1-1 gene expression in the leaves increased gradually and also showed the highest level of expression (an approximately threefold increase) at the 12 h time point. By contrast, BnSIP1-1 gene expression in the leaves under mannitol treatment showed a rapid and transient approximately fourfold increase at the 1 h time point and then declined over time. The BnSIP1-1 gene expression eventually was 40% lower than the control plants after 24 h of osmotic stress treatment. The expression pattern of BnSIP1-1 was similar in the roots as in the leaves under salt stress. However, the expression pattern of BnSIP1-1 varied in roots under ABA and mannitol stress. BnSIP1-1 expression in the roots was up-regulated and reached its highest level (an approximately threefold increase) after 2 h of ABA treatment. Under mannitol treatment, the expression of BnSIP1-1 in the roots was comparatively higher in the first 12 h of treatment and then decreased to a similar level as the control plants after 24 h of treatment.
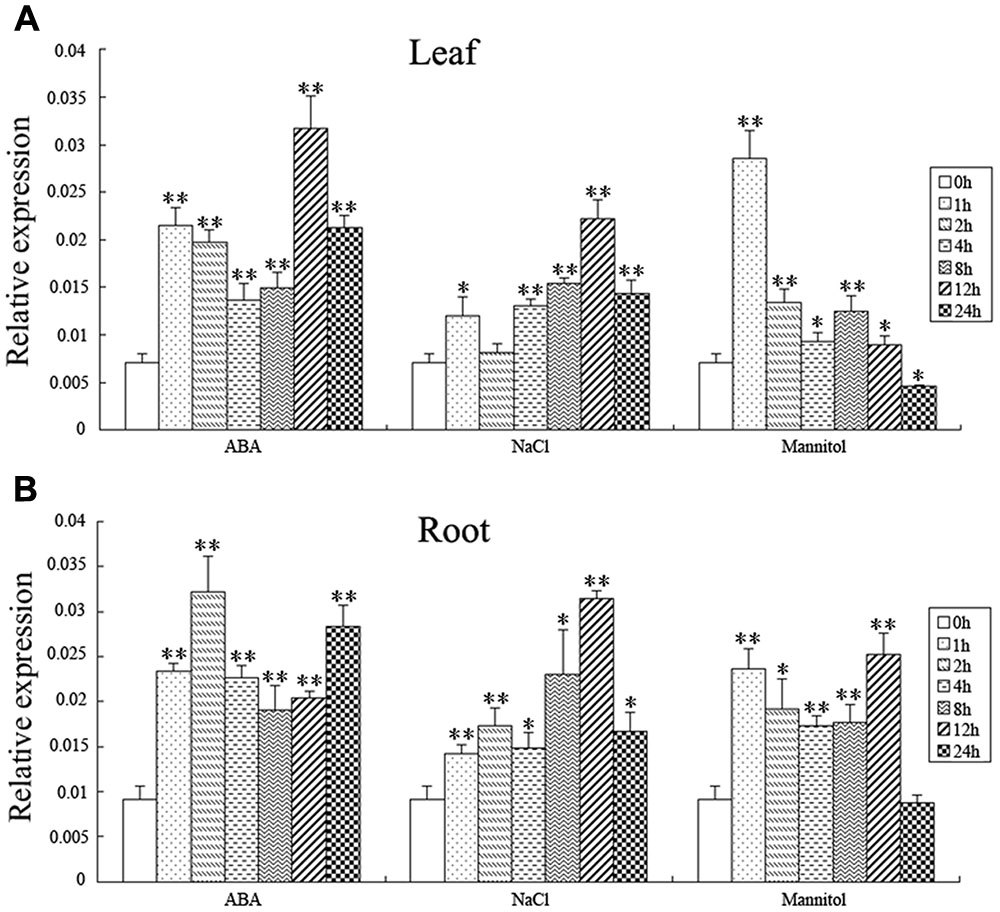
FIGURE 4. BnSIP1-1 gene expression pattern in the leaves (A) and roots (B) in the 15-day-old B. napus seedlings. B. napus plants were treated with 50 μM ABA, 200 mM NaCl, or 300 mM mannitol for 0, 1, 2, 4, 8, 12, and 24 h. The asterisks ∗ and ∗∗ indicate that the value of a t-test was P < 0.05 and P < 0.01, respectively, and a significant difference between the different abiotic treatments and the control.
Overexpression of BnSIP1-1 Conferred Osmotic Stress Tolerance in Transgenic B. napus Plants
To determine whether BnSIP1-1 played a role in the abiotic stress response, 17 independent transformants of the B. napus that OE BnSIP1-1 under the control of the CaMV 35S promoter were generated. Total RNA from young leaves of independent transgenic lines was extracted for QPCR. The results showed that the BnSIP1-1 gene was highly expressed in all of the positive lines (Figure 5A), and two lines (OE-7, OE-27) were chosen for further analysis. Fifteen-day-old seedlings were treated with salt (150 mM or 200 mM NaCl) or osmotic (300 mM or 600 mM mannitol) stress to analyze their tolerance to abiotic stresses. Compared to the wild-type seedlings, the transgenic lines showed enhanced tolerance to 600 mM mannitol after 6 h of treatment (Figure 5B), but no obvious differences were observed after salinity stress (data not shown). The leaves of 15-day-old transgenic seedlings treated with 300 mM mannitol for 9 days looked greener than the wild-type seedlings of the same age (Figure 5C). Therefore, we further determined the chlorophyll content of the leaves of the mannitol-treated seedlings. The chlorophyll content of the OE-7 and OE-27 transgenic B. napus leaves were 49.6 and 55.6% higher than that of the wild-type leaves, respectively (Figure 5D), which indicated that chlorophyll was reduced at a slower rate in the transgenic lines than in leaves of the wild-type seedlings after osmotic stress.
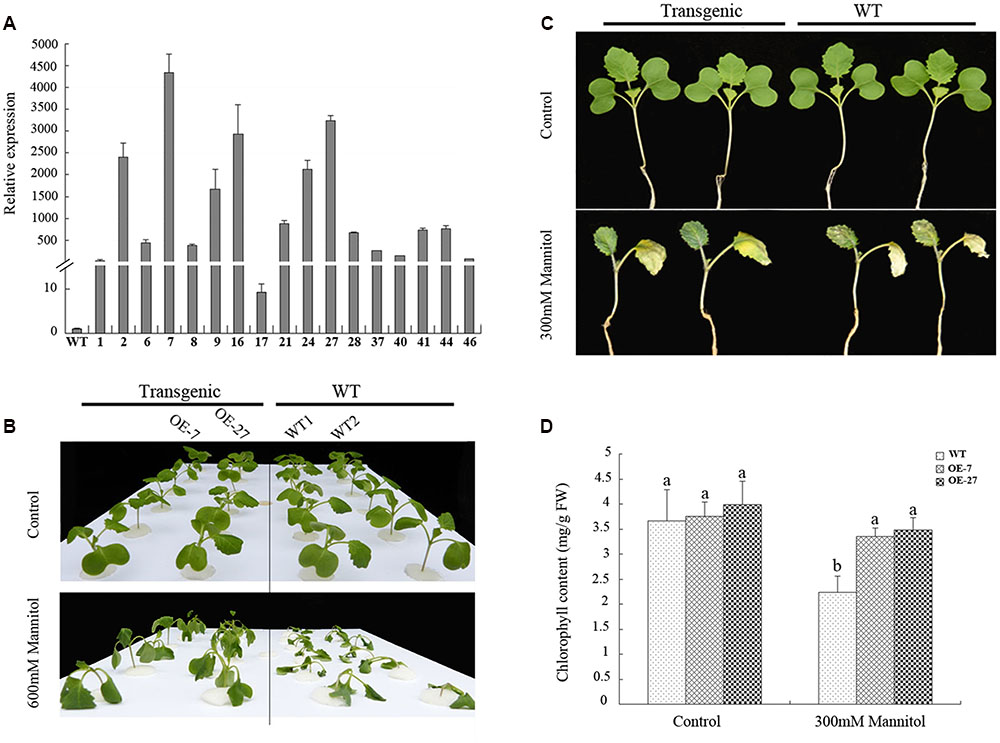
FIGURE 5. Overexpression of BnSIP1-1 in transgenic B. napus plants enhanced resistance to osmotic stress. (A) Relative gene expression of BnSIP1-1 in the leaves of 2-month-old wild-type and T0 35S:BnSIP1-1 transgenic plants by QPCR. Standard errors were derived from three repeated experiments for the expression levels of each transgenic line. (B) Fifteen-day-old seedlings were transferred to 1/2 MS medium supplemented with or without 600 mM mannitol for 6 h before the images shown were taken. (C) Fifteen-day-old seedlings were transferred to 1/2 MS medium supplemented with or without 300 mM mannitol for 9 days before the images shown were taken. (D) Total chlorophyll contents of the 300 mM mannitol-treated or untreated leaves from (C). FW, fresh weight. Values are mean ± SD (n = 3). Means denoted by the same letter did not differ significantly at P < 0.05.
Overexpression of BnSIP1-1 Leads to ABA Insensitivity in B. napus Seedlings
The expression pattern of BnSIP1-1 under ABA and abiotic stresses suggested that it had a significant role in ABA signaling, which prompted us to determine its in planta functional characterization. When plated and grown on MS medium, the BnSIP1-1 transgenic plants grew almost as same as the wild-type plants (Figures 6A,B). However, when cultured in MS medium or soil with 1 or 10 μM ABA for several days, the BnSIP1-1 OE seeds were less sensitive to ABA than the wild-type seeds (Figures 6A,B). In the absence of ABA, the root length and weight were similar in the wild-type and transgenic plants. However, under 10 μM ABA treatment, the stems of the transgenic plants were 35–45% longer than those of the wild-type plants (Figure 6C). There was no significant difference in the stem length between the wild-type and transgenic seedlings when under 1 μM ABA treatment. The root of the transgenic plants was 22–38% and 160–224% longer than the wild-type plants under 1 and 10 μM ABA, respectively (Figure 6C). At the same time, the fresh weight of the transgenic roots was 21–37% and 116–163% higher than the wild-type plants under 1 and 10 μM ABA treatments, respectively (Figure 6D). The fresh weight of the aboveground part of the transgenic plants was 24–28% and 142–185% higher than the wild-type plants under 1 and 10 μM ABA, respectively (Figure 6D). These data suggested that overexpression of the BnSIP1-1 gene caused reduced sensitivity to ABA in B. napus seedlings.
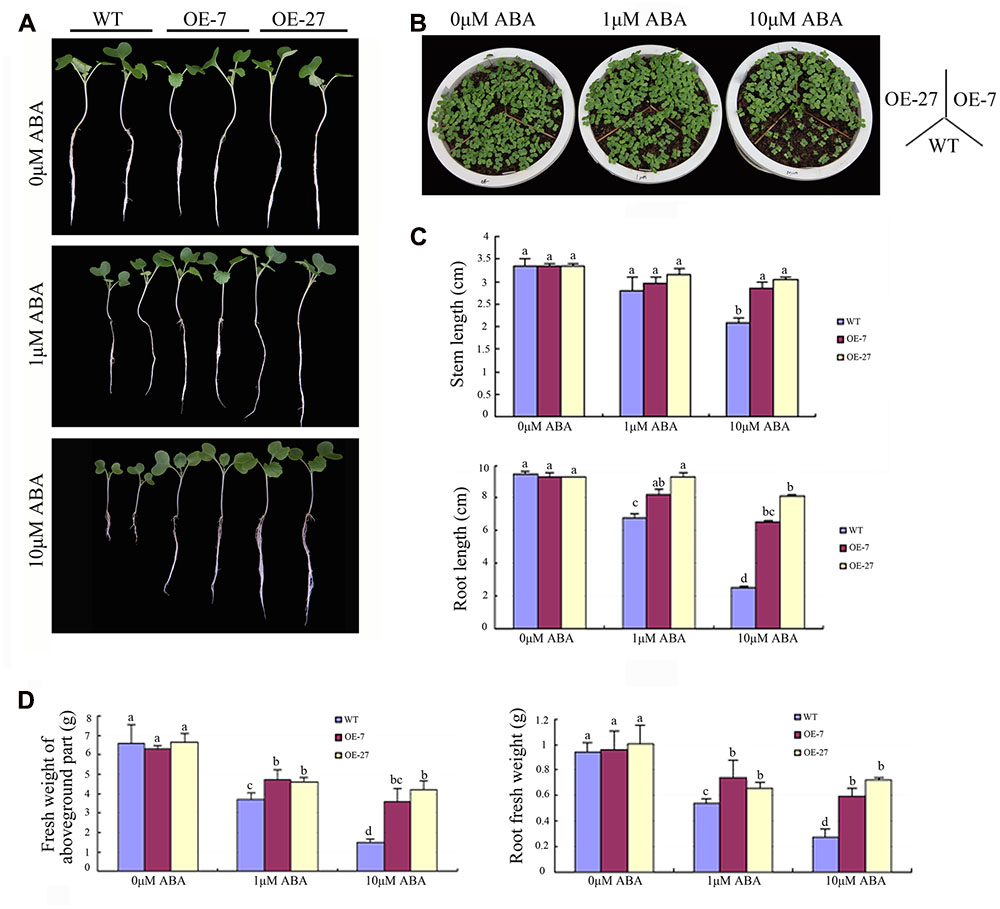
FIGURE 6. Reduced sensitivity of the BnSIP1-1-OE transgenic B. napus lines to ABA. (A) Growth of the wild-type and BnSIP1-1 transgenic seedlings on liquid 1/2 MS medium containing 0, 1, and 10 μM ABA. Representative photographs were taken after 12 days of plating. (B) Growth of the wild-type and BnSIP1-1 transgenic seedlings grown in pots containing 0, 1, and 10 μM ABA. Representative photographs were taken after 6 days plating. (C) The stem and root lengths of each plant were measured after 12 days plating. (D) The fresh weight of the aboveground part and root of each plant was measured after 12 days of plating. Values are mean ± SD (n = 3). Means denoted by the same letter did not differ significantly at P < 0.05.
BnSIP1-1 Overexpression Caused Enhanced Germination Rate of B. napus under Osmotic, Salt and ABA Stresses
The soil culture experiments of above mentioned dropped a hint that the germination rate of transgenic B. napus seeds was higher than that of the wild-type seeds under ABA treatment. To systematically study the effect of BnSIP1-1 on the germination process, we sowed the wild-type and transgenic T3 seeds on plates containing distilled water, mannitol, NaCl, and ABA for 4 days. Without any addition, there were no differences between the wild-type and transgenic seeds. However, when mannitol, NaCl or ABA were applied, the transgenic seeds germinated much better than the wild-type seeds (Figure 7). Under lower concentrations of mannitol (400 mM) or NaCl (150 mM), the germination rate of the transgenic seeds could reach higher than 80%, while the germination rate of the wild-type seeds was lower than 40% (Figures 7A-b/c,B-b/c) after 96 h of treatment. Under higher concentrations of mannitol (600 mM) or NaCl (200 mM), the germination rate of the transgenic seeds was approximately 30%, while the germination rate of the wild-type seeds was only about 10% (Figures 7A-e/f,B-e/f) after 96 h of treatment. In term of the ABA treatment, the transgenic seeds germinated faster than wild-type under both 50 μM ABA and 100 μM ABA treatment (Figures 7A-d/g,B-d/g). The increase of ABA concentration had a greater impact on the germination of the wild-type seeds than the transgenic seeds.
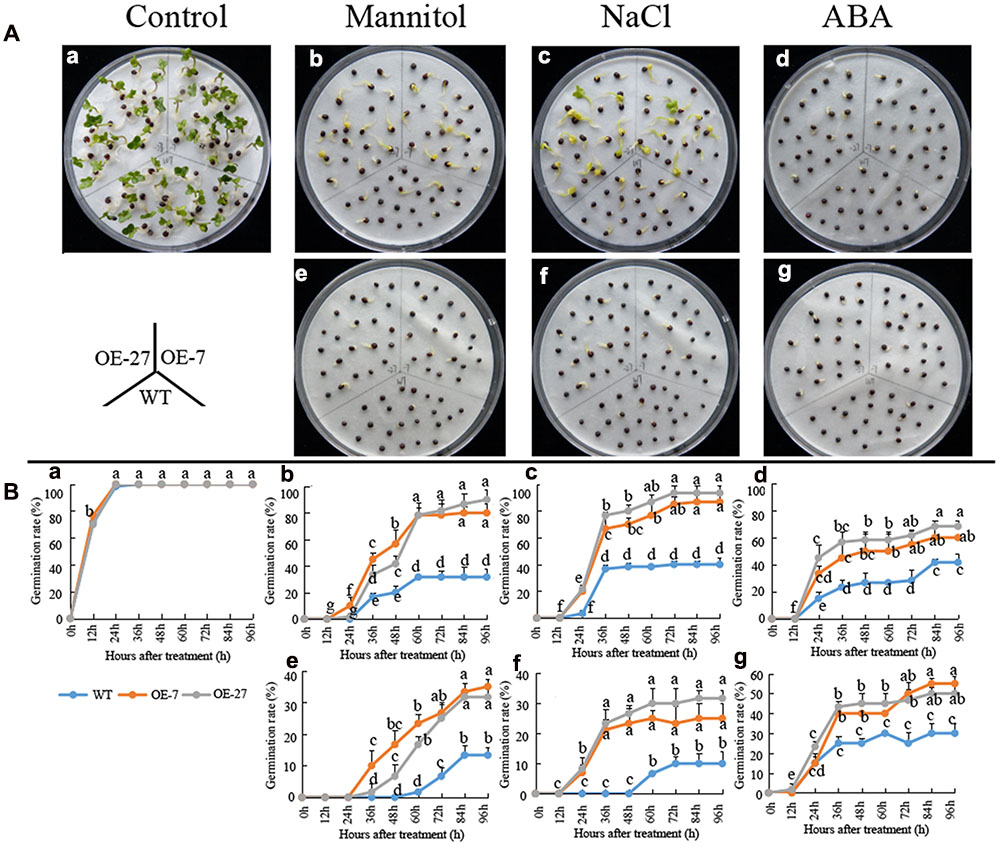
FIGURE 7. Germination rates of the wild-type and BnSIP1-1 OE transgenic seeds under mannitol, NaCl or ABA treatments. (A) Photographs were taken after 60 h of growth on liquid MS medium containing no addition (a), 400 mM mannitol (b), 150 mM NaCl (c), 50 μM ABA (d), 600 mM mannitol (e), 200 mM NaCl (f), or 100 μM ABA (g). (B) The time course of seed germination rates of the wild-type and BnSIP1-1 overexpression transgenic seeds in liquid MS medium containing no addition (a), 400 mM mannitol (b), 150 mM NaCl (c), 50 μM ABA (d), 600 mM mannitol (e), 200 mM NaCl (f), or 100 μM ABA (g). The data represent the mean ± SD of three independent experiments (germination percentage: 100 seeds for each line). Values are mean ± SD (n = 3). Means denoted by the same letter did not differ significantly at P < 0.05.
BnSIP1-1 Overexpression Changed ABA Accumulation
ABA is an extensively studied hormone that plays essential roles in stress responses of plants. Many kinds of abiotic stress promote ABA synthesis, which further induce stomatal closure and up-regulation of the expression of ABA-responsive genes, thereby contributing to plant stress resistance (Finkelstein et al., 2002).
To study whether OE BnSIP1-1 affect ABA accumulation, we detected endogenous ABA content in plants (Figure 8). All of the stress treatments, including ABA, mannitol and NaCl, can induce significant accumulation of ABA both in the wild-type and the transgenic plants. In addition, endogenous ABA level was 51% higher in transgenic plants than the wild-type during normal growth condition. Under exogenous ABA and mannitol treatment, the accumulation of ABA in the transgenic plants was higher than that in the control plants (Figures 8A,B). Under salt stress, the difference of ABA content before treatment was gradually decreased with the prolongation of salt treatment time, then after 24 h of treatment the ABA level in the transgenic plants became similar to the wild-type plants, which showing opposite change trend with ABA or mannitol treatments (Figure 8C).
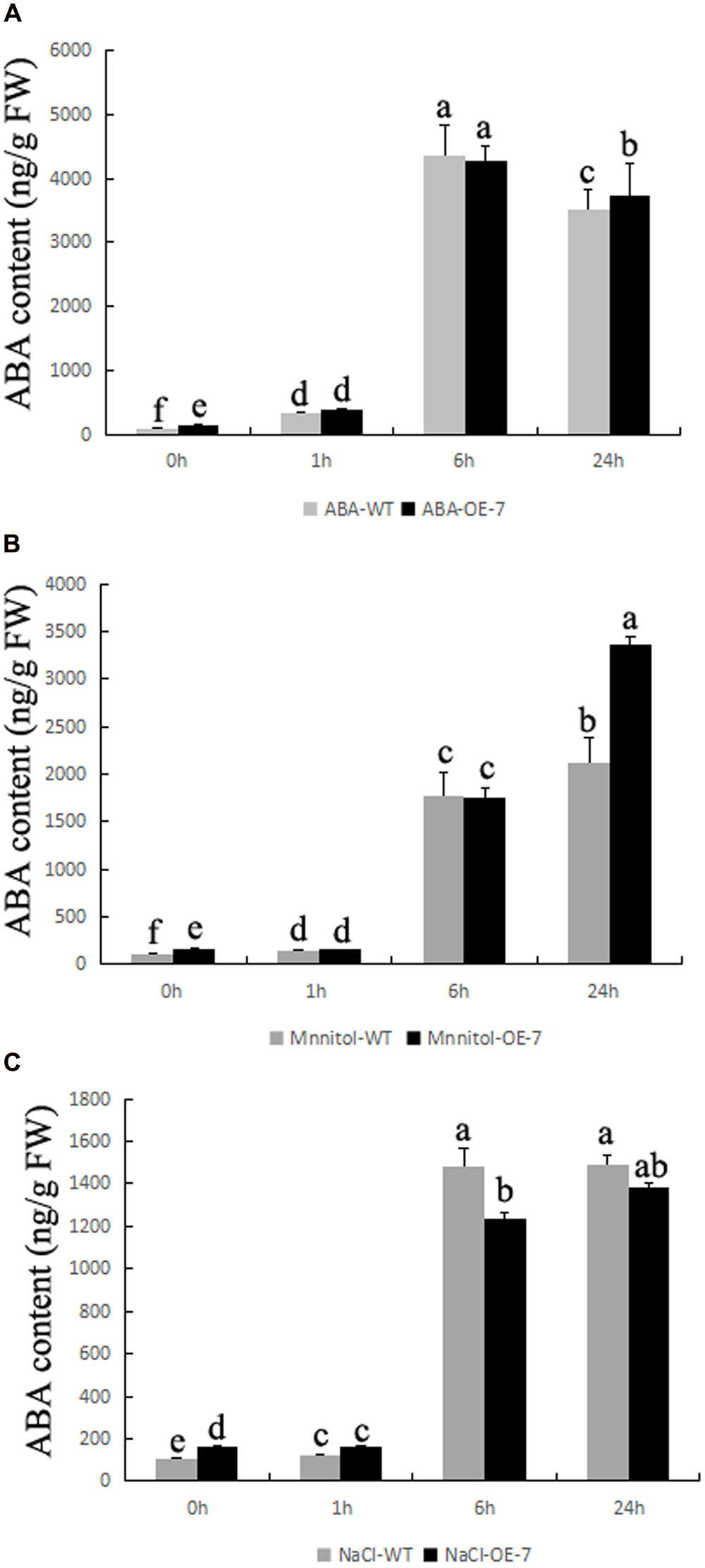
FIGURE 8. Changes of ABA content in transgenic plants during stress conditions. Fifteen-day-old seedlings were transferred to 1/2 MS medium supplemented with 50 μM ABA, 200 mM NaCl, or 300 mM mannitol for 0, 1, 6, and 24 h. (A) ABA content of the wild-type and transgenic leaves after ABA treatment for 0, 1, 6, and 24 h; (B) ABA content of the wild-type and transgenic leaves after mannitol treatment for 0, 1, 6, and 24 h; (C) ABA content of the wild-type and transgenic leaves after NaCl treatment for 0, 1, 6, and 24 h. Values are mean ± SD (n = 3). Means denoted by the same letter did not differ significantly at P < 0.05.
Altered Expression of Stress-Related and ABA Signaling Genes in BnSIP1-1 Transgenic B. napus
The BnSIP1-1 may function in response to stress and ABA through the regulation of downstream genes. To broaden our knowledge about the molecular mechanism of ABA-insensitivity and stress-tolerance in BnSIP1-1-OE plants, we first analyzed the expression level of related genes, including BnRD29A, BnERD15, BnLEA1, BnNAC485, BnCIPK6, BnSOS1, BnNHX1, BnKIN1, and BnHKT by QPCR in the wild-type and transgenic plants under normal conditions (Figures 9A,B). These genes were categorized into two groups: the expression-increased group (Figure 9A) and expression-unchanged/decreased group (Figure 9B). As shown in Figure 9A, the expression of the transcription factor BnNAC485 had the most significant increase in BnSIP1-1 transgenic plants, being approximately 14-fold higher than that in the wild-type plants. Similarly, the expression of BnRD29A was increased about 11-fold higher level in the transgenic lines than in the wild-type plants. Furthermore, the expression levels of BnERD15, BnLEA1 and BnCIPK6 were also up-regulated 3- to 5-fold in the BnSIP1-1-OE plants. However, there was no significant difference in the expression levels of BnSOS1, BnNHX1, BnABI5 and BnKIN1 between the transgenic and wild-type plants, as shown in Figure 9B. In addition, the expression level of BnHKT declined by 68% in the transgenic plants compared with the wild-type under normal growth conditions.
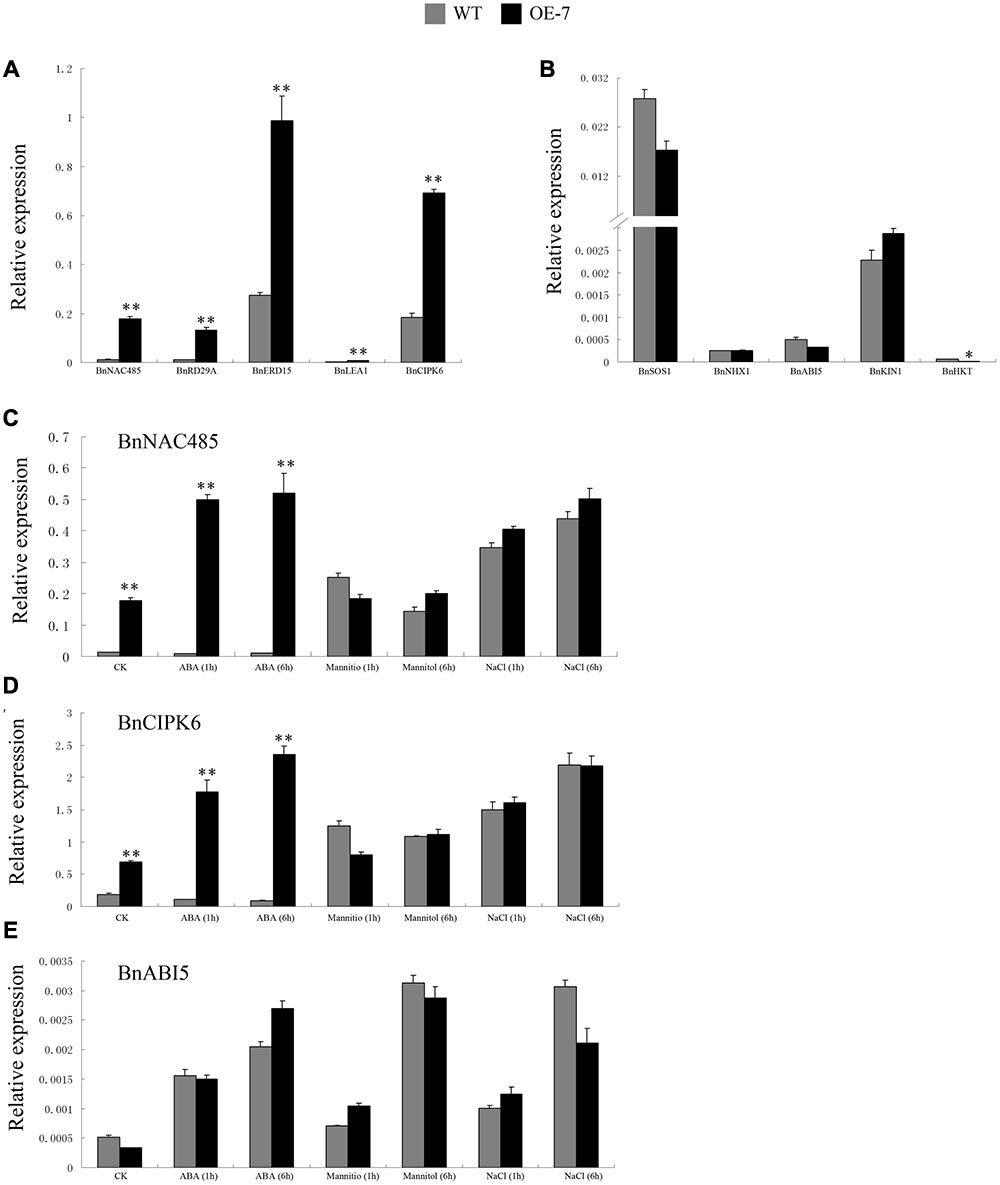
FIGURE 9. Expression pattern of stress- and ABA-responsive genes in the transgenic and wild-type plants under normal condition, ABA, mannitol or NaCl treatments. (A) Transcript abundance of BnNAC485, BnRD29A, BnERD15, BnLEA1, and BnCIPK6 in the leaves of 15-day-old B. napus seedlings under normal growth condition. (B) Transcript abundance of BnSOS1, BnNHX1, BnABI5, BnKIN1, and BnHKT in the leaves of 15-day-old B. napus seedlings under normal growth conditions. (C) Transcript abundance of BnNAC485 in the leaves of 15-day-old B. napus seedlings under control, ABA, mannitol and NaCl treatment for 1 and 6 h. (D) Transcript abundance of BnCIPK6 in the leaves of 15-day-old B. napus seedlings under control, ABA, mannitol and NaCl treatment for 1 and 6 h. (E) Transcript abundance of BnABI5 in the leaves of 15-day-old B. napus seedlings under control, ABA, mannitol and NaCl treatment for 1 and 6 h. Values are mean ± SD (n = 3). The asterisks indicate significant differences (Student’s t-test, ∗ indicates P < 0.05, ∗∗ indicates P < 0.01).
BnNAC485 and BnCIPK6 are two rare rapeseed genes which were experimentally identified to play significant roles in ABA signaling and stress responses (Chen L. et al., 2012; Ying et al., 2014). ABI5 is a basic leucine zipper protein which has already been identified to have important function in the ABA signaling pathway (Piskurewicz et al., 2008). Therefore, to further expand our understanding of regulation mechanism of BnSIP1-1, we compared the expression level of BnNAC485, BnCIPK6 and BnABI5 in the wild-type and transgenic plants treated by ABA, mannitol and NaCl stresses for different time periods. After 1 h of ABA treatment, the expression levels of BnNAC485 and BnCIPK6 were about 40-fold and 16-fold higher in the transgenic plants than in the wild-type plants, respectively. As the ABA-treatment time increased to 6 h, the high-expression level of BnNAC485 remained steady, while the expression level of BnCIPK6 was further increased to 27-fold in the transgenic plants compared to the wild-type plants. There were no significant differences in the expression levels of BnNAC485 and BnCIPK6 under osmotic or salt stresses between the transgenic and wild-type plants. Notably, the expression level of BnABI5 did not present any difference between the transgenic and wild-type plants under all three of the stress conditions.
Discussion
Although the trihelix family genes have been shown to function in the developmental processes of plants, their roles in the response to abiotic stress and ABA are largely unknown. Previous studies have shown that some of the gene members in the GT-1, GT-2 and GTγ trihelix subfamilies are involved in the abiotic-stress response, such as AtGT-3b, GmGT-2A, GmGT-2B, and OsGTγ-1 (Park et al., 2004; Xie et al., 2009; Fang et al., 2010). These genes work as positive or negative factors to regulate the adaptability of plants to stresses. In addition, only four genes, AtGT2L and OsGTγ-1/2/3 (Fang et al., 2010; Xi et al., 2012), were reported to function in the ABA response. No gene members in the other two subfamilies SH4 and SIP1 have been found to play roles in the abiotic-stress response or ABA response. In the present study, a new SIP1 subfamily gene designated as BnSIP1-1 from B. napus was first identified to be involved in stress responsive and ABA signaling, which could confer stress-tolerance and ABA insensitivity in transgenic B. napus plants through the regulation of downstream genes. These studies improve our knowledge about the abiotic stresses and ABA response-related functions of the trihelix family members in plants.
The expression level of BnSIP1-1 was up-regulated by osmotic and salt stress treatments, indicating a role for the BnSIP1-1 protein in response to abiotic stress. Detailed phenotypic analyses demonstrated that BnSIP1-1-OE B. napus plants showed a dramatically enhanced germination rate under osmotic or salt stress treatments. In addition, overexpression of BnSIP1-1 increased the osmotic stress tolerance of the transgenic B. napus plants by alleviating seedling wilting and decelerating chlorophyll degradation. Chlorophyll a and b are important photosynthetic pigments in the chloroplast of plant and participate in essential processes of harvesting light energy in the antenna systems by driving electron transfer in the reaction centers (Rissler et al., 2002). The chlorophyll content is generally taken as the primary index of plant stress tolerance (Parihar et al., 2015; Zhou et al., 2016). Overexpressing SbNHX1 gene could improve the salt tolerance of transgenic jatropha and castor by depressing deterioration of chlorophyll under different level of salinity stress (Joshi et al., 2013; Patel et al., 2015). Similar result was confirmed by a nuclear-localized histone-gene binding protein from rice (OsHBP1b), playing roles in salinity and osmotic stress tolerance by maintaining chlorophyll content and improving the antioxidant machinery (Lakra et al., 2015). These reports coincided with our findings that the BnSIP1-1 overexpression transgenic plants had more chlorophyll content and increased tolerance by compared with the wild-type plants under osmotic stress. However, we also detected the response of the transgenic B. napus seedlings to salt stress, and no obvious different growth status or chlorophyll content was observed compared to the wild-type seedlings. Therefore, we speculated that BnSIP1-1 functions differently in response to osmotic and salt stresses through differential regulatory mechanisms during the seedling period.
The plant hormone ABA regulates many key processes in seed dormancy and germination, growth regulation, and stress responses (Ton et al., 2009). Given that the expression of BnSIP1-1 was induced by ABA (Figure 4), we further explored whether BnSIP1-1 was involved in the ABA-mediated pathway. In terms of seed germination, the transgenic B. napus plants showed varying degrees of insensitivity to ABA compared to the wild-type seeds. Furthermore, during the seedling stage, over-expression of BnSIP1-1 resulted in enhanced growth in the transgenic plant than in the wild-type plants. The phenotype was more obvious under high concentrations of ABA than low concentrations. On this basis, we believe that BnSIP1-1 negatively participates in the ABA-response pathway.
In addition to the findings noted above, we also drew the conclusion that BnSIP1-1 can regulate the synthesis of ABA (Figure 8). ABA levels in the transgenic leaves were higher than those of the control plants under normal condition. Both of the ABA content and synthesis rate in the transgenic leaves were different between different stress conditions. This indicated that BnSIP1-1 involved in the fine-tune regulation of ABA synthesis. Previous studies have indicated that the induction of the ABA synthesis was conducive to the reduction of water loss, activating stress-related gene expression to improve plant’s osmotic stress tolerance (Sirichandra et al., 2009; Bhaskara et al., 2012). As expected, the higher endogenous ABA content in exogenous ABA-treated and mannitol-treated transgenic plants, and unchanged ABA content in salt-treated transgenic plants were consistent with ABA and osmotic stress tolerance and salt-sensitive phenotype of transgenic plants. Thus, we considered BnSIP1-1 not only response to exogenous ABA but also regulate endogenous ABA synthesis.
In general, osmotic stress response genes, such as BnNAC485 and BnCIPK6, also can increase the tolerance to salt stress (Chen L. et al., 2012; Ying et al., 2014). However, in the transgenic seedlings, BnSIP1-1 increased tolerance to ABA and osmotic but not salt stress in our study. We questioned why the BnSIP1-1 transgenic seedlings had different responses to salt, osmotic and ABA stress. Therefore, we examined the expression of several well-known stress responsive genes, such as BnRD29A, BnERD15, BnLEA1, and BnKIN1 (Kasuga et al., 1999; Msanne et al., 2011; Dubrovina et al., 2015); ABA-responsive genes, such as BnNAC485, BnCIPK6, and BnABI5 (Lopez-Molina et al., 2001; Chen L. et al., 2012; Ying et al., 2014); and salt-responsive genes, such as BnSOS1, BnNHX1 and BnHKT (Chakraborty et al., 2012; Dubrovina et al., 2015). Under normal condition, the expressions of BnRD29A, BnERD15, and BnLEA1 in the BnSIP1-1 OE transgenic plants were higher than in the wild-type plants, suggesting BnSIP1-1 positively regulated the stress response in plants (Figure 9A). However, the expressions of salt stress-responsive genes such as BnSOS1 and BnNHX, were unchanged (Figure 9B). Moreover, the expression of BnHKT1 was even decreased in the transgenic plants (Figure 9B). SOS1, NHX1 and HKT gene are three essential salt-specific response genes, which control Na+ exit (SOS1) and Na+ entry (HKT) from the cell, or help the compartmentalization of excess Na+ ions in the vacuole (NHX1; Zhu, 2003; Joshi et al., 2013; Hamamoto et al., 2014). The expression pattern of the above-mentioned genes in our study illustrated that BnSIP1-1 perhaps not regulate salt-specific stress-responsive pathway during the seedling period in plants (Figure 9). In order to verify our speculation, we further detected the expression of all stress-marker genes at 48 and 72 h of salt treatment to study the long term effect of stress on plants, avoiding the interference of 1/2 MS medium to Na+. As shown in Figure 10, we noticed that BnLEA1, BnKIN1, BnNHX1, BnSOS1, BnNAC485, and BnCIPK6 were clearly down-regulated in response to 48 h NaCl treatment. SOS1 and NHX1 help cells to remove Na+ in cytoplasm to extracellular environment or vacuole under salt stress. The down-regulation of these two genes indicated more toxic Na+ accumulation in the transgenic cells cytoplasm after 48 h NaCl treatment, which was consistent with the down-regulation of effector gene, e.g., BnLEA1 and BnKIN1. However, when the processing time was extended to 72 h, the situation was quite different. The effector genes at downstream of stress response pathway BnRD29A and BnKIN1, salt-specific regulators BnSOS1 and BnNHX1, and ABA response genes BnABI5, BnNAC485 and BnCIPK6 showed no difference between the transgenic and wild-type seedlings after 72 h of NaCl treatment. We speculated BnSIP1-1 had a dynamic response to salt stress in the early stage of salt treatment in B. napus seedlings. Yang et al. (2014) reported a similar gene, Cm-BBX24, which regulated flowering time and drought tolerance, but not salt. In their global transcriptome data, the categories of functional proteins included LEA and dehydration response proteins, members of WRKY, MYB and Zinc finger proteins, and some protein kinases were up-regulated in Cm-BBX24 overexpression plants. There were also numerous genes related to abiotic stress responses down-regulated, indicating complex function mechanism involved. Therefore, we considered that BnSIP1-1, similar to Cm-BBX24, did not improve, or at least not strongly improve plant salt tolerance, although they can respond to salt stress. Dynamic measurement of Na+ accumulation in the wild-type and transgenic seedlings need to be conducted to further explain why BnSIP1-1 did not enhance salt tolerance. In addition, Lu Ying et al. reported that overexpression of BnNAC485 improved the tolerance to osmotic and salt stress and increased the ABA-sensitivity by raising the expression of ABI5, which plays an important negative role in the ABA pathway (Piskurewicz et al., 2008; Ying et al., 2014). In our study, the expression level of BnNAC485 was increased in the BnSIP1-1 transgenic plants, but ABA hypersensitivity phenotype was not consistent with the BnNAC485 OE plants. We speculated that ABI5 played key role for this discrepancy, because the expression of ABI5 was not significantly changed in the BnSIP1-1 transgenic plants (Figure 9E), while ABI5 was highly induced in the BnNAC485 OE plants. Therefore, we considered that the high expression of BnSIP1-1 inhibited the increase of BnABI5, maintaining its expression at a normal level under the control condition and stress treatments and preventing the transgenic plants from responding to the ABA treatment. The above combined analysis indicated that BnSIP1-1 regulates different pathway genes in response to different stresses through a complex network.
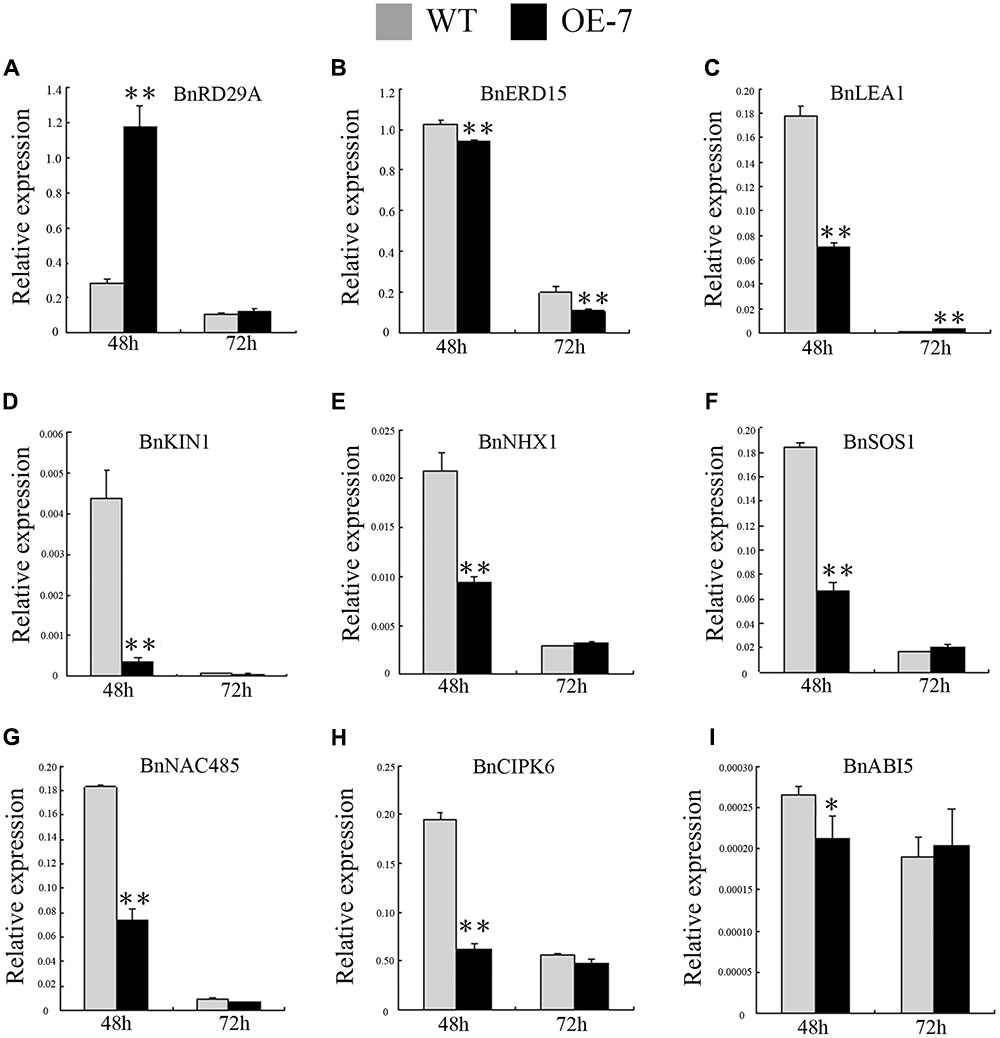
FIGURE 10. Expression pattern of stress- and ABA-responsive genes in the transgenic and wild-type plants under 48 and 72 h of NaCl treatments. Fifteen-day-old seedlings were transferred to 1/2 MS medium supplemented with 200 mM NaCl for 48 and 72 h. Leaves of the transgenic and wild-type plants were harvested for RT-PCR analysis of transcript abundance of (A) BnRD29A, (B) BnERD15, (C) BnLEA1, (D) BnKIN1, (E) BnNHX1, (F) BnSOS1, (G) BnNAC485, (H) BnCIPK6 and (I) BnABI5. Values are mean ± SD (n = 3). The asterisks indicate significant differences (Student’s t-test, ∗ indicates P < 0.05, ∗∗ indicates P < 0.01).
Overall, BnSIP1-1 can improve seed germination under osmotic, salt or ABA stress treatments, and increase osmotic stress tolerance and reduce ABA sensitivity during the seedling period. The manner in which BnSIP1-1 regulates downstream genes to respond to different stresses in different developmental processes in plants remains unclear, however, our data clearly illustrated that BnSIP1-1 played roles in ABA synthesis and signaling, salt and osmotic stress response by regulating BnABI5, BnNAC485 or other stress-related genes. The relationship between the molecular mechanisms of BnSIP1-1 in response to osmotic, salt and ABA stresses should be further investigated.
Author Contributions
Jl-L and GW designed the research. ST, FM, JL, XL, XY, XZ, FL, and YW performed the experiments and conducted data analyses. XP edited the manuscript. Jl-L and GW wrote the manuscript. All authors read and approved the final manuscript.
Funding
This work was supported by grants from the National Natural Science Foundation of China (grant numbers 31201152 and 31401038).
Conflict of Interest Statement
The authors declare that the research was conducted in the absence of any commercial or financial relationships that could be construed as a potential conflict of interest.
Supplementary Material
The Supplementary Material for this article can be found online at: http://journal.frontiersin.org/article/10.3389/fpls.2017.00044/full#supplementary-material
FIGURE S1 | Molecular confirmation of transgenic lines. PCR amplification of BnSIP1-1 gene from the wild-type and potential transgenic plants. Lane NC, negative control, lane WT, wild type plant, lanes L, putative transgenic lines and Lane M, marker ladder.
Abbreviation
ABA, abscisic acid; ANOVA, analysis of variance; CaMV, Cauliflower mosaic virus; GFP, green fluorescent protein; MS, Murashige and Skoog; OE, overexpressing; ORF, open reading frame; QPCR, quantitative reverse transcription–PCR; SIP1, 6b INTERACTING PROTEIN1; ZS6, Brassica napus lines cv. ZhongShuang 6.
Footnotes
- ^https://blast.ncbi.nlm.nih.gov/Blast.cgi
- ^http://bioinf.cs.ucl.ac.uk/psipred/
- ^http://www.ebi.ac.uk/Tools/msa/clustalw2
References
Bhaskara, G. B., Nguyen, T. T., and Verslues, P. E. (2012). Unique drought resistance functions of the highly ABA-induced clade A protein phosphatase 2Cs. Plant Physiol. 160, 379–395. doi: 10.1104/pp.112.202408
Boyer, J. S. (1982). Plant productivity and environment. Science 218, 443–448. doi: 10.1126/science.218.4571.443
Chakraborty, K., Sairam, R. K., and Bhattacharya, R. C. (2012). Differential expression of salt overly sensitive pathway genes determines salinity stress tolerance in Brassica genotypes. Plant Physiol. Biochem. 51, 90–101. doi: 10.1016/j.plaphy.2011.10.001
Chen, L., Ren, F., Zhou, L., Wang, Q. Q., Zhong, H., and Li, X. B. (2012). The Brassica napus calcineurin B-Like 1/CBL-interacting protein kinase 6 (CBL1/CIPK6) component is involved in the plant response to abiotic stress and ABA signalling. J. Exp. Bot. 63, 6211–6222. doi: 10.1093/jxb/ers273
Chen, M., Fu, X., Liu, J., Ye, T., Hou, S., Huang, Y., et al. (2012). Highly sensitive and quantitative profiling of acidic phytohormones using derivatization approach coupled with nano-LC–ESI-Q-TOF-MS analysis. J. Chromatogr. B Analyt. Technol. Biomed. Life Sci. 905, 67–74. doi: 10.1016/j.jchromb.2012.08.005
Dubrovina, A. S., Kiselev, K. V., Khristenko, V. S., and Aleynova, O. A. (2015). VaCPK20, a calcium-dependent protein kinase gene of wild grapevine Vitis amurensis Rupr., mediates cold and drought stress tolerance. J. Plant Physiol. 185, 1–12. doi: 10.1016/j.jplph.2015.05.020
Fang, Y., Xie, K., Hou, X., Hu, H., and Xiong, L. (2010). Systematic analysis of GT factor family of rice reveals a novel subfamily involved in stress responses. Mol. Genet. Genomics 283, 157–169. doi: 10.1007/s00438-009-0507-x
Finkelstein, R. R., Gampala, S. S., and Rock, C. D. (2002). Abscisic acid signaling in seeds and seedlings. Plant Cell 14, S15–S45.
Fukao, T., and Xiong, L. (2013). Genetic mechanisms conferring adaptation to submergence and drought in rice: simple or complex? Curr. Opin. Plant Biol. 16, 196–204. doi: 10.1016/j.pbi.2013.02.003
Gilmartin, P. M., Memelink, J., Hiratsuka, K., Kay, S. A., and Chua, N. H. (1992). Characterization of a gene encoding a DNA binding protein with specificity for a light-responsive element. Plant Cell 4, 839–849. doi: 10.1105/tpc.4.7.839
Hamamoto, S., Horie, T., Hauser, F., Deinlein, U., Schroeder, J. I., and Uozumi, N. (2014). HKT transporters mediate salt stress resistance in plants: from structure and function to the field. Curr. Opin. Biotechnol. 32, 113–120. doi: 10.1016/j.copbio.2014.11.025
Joshi, M., Jha, A., Mishra, A., and Jha, B. (2013). Developing transgenic Jatropha using the SbNHX1 gene from an extreme halophyte for cultivation in saline wasteland. PLoS ONE 8:e71136. doi: 10.1371/journal.pone.0071136
Kaplan-Levy, R. N., Brewer, P. B., Quon, T., and Smyth, D. R. (2012). The trihelix family of transcription factors–light, stress and development. Trends Plant Sci. 17, 163–171. doi: 10.1016/j.tplants.2011.12.002
Kaplan-Levy, R. N., Quon, T., O’Brien, M., Sappl, P. G., and Smyth, D. R. (2014). Functional domains of the PETAL LOSS protein, a trihelix transcription factor that represses regional growth in Arabidopsis thaliana. Plant J. 79, 477–491. doi: 10.1111/tpj.12574
Kasuga, M., Liu, Q., Miura, S., Yamaguchi-Shinozaki, K., and Shinozaki, K. (1999). Improving plant drought, salt, and freezing tolerance by gene transfer of a single stress-inducible transcription factor. Nat. Biotechnol. 17, 287–291. doi: 10.1038/7036
Kitakura, S., Fujita, T., Ueno, Y., Terakura, S., Wabiko, H., and Machida, Y. (2002). The protein encoded by oncogene 6b from Agrobacterium tumefaciens interacts with a nuclear protein of tobacco. Plant Cell 14, 451–463. doi: 10.1105/tpc.010360
Lakra, N., Nutan, K. K., Das, P., Anwar, K., Singla-Pareek, S. L., and Pareek, A. (2015). Functional biotechnology A nuclear-localized histone-gene binding protein from rice (OsHBP1b) functions in salinity and drought stress tolerance by maintaining chlorophyll content and improving the antioxidant machinery. J. Plant Physiol. 176, 36–46. doi: 10.1016/j.jplph.2014.11.005
Li, A., Zhou, Y., Jin, C., Song, W., Chen, C., and Wang, C. (2013). LaAP2L1, a heterosis-associated AP2/EREBP transcription factor of larix, increases organ size and final biomass by affecting cell proliferation in Arabidopsis. Plant Cell Physiol. 54, 1822–1836. doi: 10.1093/pcp/pct124
Li, B., Jiang, S., Yu, X., Cheng, C., Chen, S., Cheng, Y., et al. (2015). Phosphorylation of trihelix transcriptional repressor ASR3 by MAP KINASE4 negatively regulates Arabidopsis immunity. Plant Cell 27, 839–856. doi: 10.1105/tpc.114.134809
Li, X., Qin, G., Chen, Z., Gu, H., and Qu, L. J. (2008). A gain-of-function mutation of transcriptional factor PTL results in curly leaves, dwarfism and male sterility by affecting auxin homeostasis. Plant Mol. Biol. 66, 315–327. doi: 10.1007/s11103-007-9272-6
Liu, F., Xiong, X., Wu, L., Fu, D., Hayward, A., Zeng, X., et al. (2014). BraLTP1, a lipid transfer protein gene involved in epicuticular wax deposition, cell proliferation and flower development in Brassica napus. PLoS ONE 9:e110272. doi: 10.1371/journal.pone.0110272
Livak, K. J., and Schmittgen, T. D. (2001). Analysis of relative gene expression data using real-time quantitative PCR and the 2(-Delta Delta C(T)) Method. Methods 25, 402–408. doi: 10.1006/meth.2001.1262
Lopez-Molina, L., Mongrand, S., and Chua, N. H. (2001). A postgermination developmental arrest checkpoint is mediated by abscisic acid and requires the ABI5 transcription factor in Arabidopsis. Proc. Natl. Acad. Sci. U.S.A. 98, 4782–4787. doi: 10.1073/pnas.081594298
Mishra, A., Mandoli, A., and Jha, B. (2008). Physiological characterization and stress-induced metabolic responses of Dunaliella salina isolated from salt pan. J. Ind. Microbiol. Biotechnol. 35, 1093–1101. doi: 10.1007/s10295-008-0387-9
Msanne, J., Lin, J., Stone, J. M., and Awada, T. (2011). Characterization of abiotic stress-responsive Arabidopsis thaliana RD29A and RD29B genes and evaluation of transgenes. Planta 234, 97–107. doi: 10.1007/s00425-011-1387-y
Nakashima, K., Ito, Y., and Yamaguchi-Shinozaki, K. (2009). Transcriptional regulatory networks in response to abiotic stresses in Arabidopsis and grasses. Plant Physiol. 149, 88–95. doi: 10.1104/pp.108.129791
Parihar, P., Singh, S., Singh, R., Singh, V. P., and Prasad, S. M. (2015). Effect of salinity stress on plants and its tolerance strategies: a review. Environ. Sci. Pollut. Res. 22, 4056–4075. doi: 10.1007/s11356-014-3739-1
Park, H. C., Kim, M. L., Kang, Y. H., Jeon, J. M., Yoo, J. H., Kim, M. C., et al. (2004). Pathogen- and NaCl-induced expression of the SCaM-4 promoter is mediated in part by a GT-1 box that interacts with a GT-1-like transcription factor. Plant Physiol. 135, 2150–2161. doi: 10.1104/pp.104.041442
Patel, M. K., Joshi, M., Mishra, A., and Jha, B. (2015). Ectopic expression of SbNHX1 gene in transgenic castor (Ricinus communis L.) enhances salt stress by modulating physiological process. Plant Cell Tissue Organ Cult. 122, 477–490. doi: 10.1007/s11240-015-0785-4
Perisic, O., and Lam, E. (1992). A tobacco DNA binding protein that interacts with a light-responsive box II element. Plant Cell 4, 831–838. doi: 10.1105/tpc.4.7.831
Piskurewicz, U., Jikumaru, Y., Kinoshita, N., Nambara, E., Kamiya, Y., and Lopez-Molina, L. (2008). The gibberellic acid signaling repressor RGL2 inhibits Arabidopsis seed germination by stimulating abscisic acid synthesis and ABI5 activity. Plant Cell 20, 2729–2745. doi: 10.1105/tpc.108.061515
Porra, R. J., Thompson, W. A., and Kriedemann, P. E. (1989). Determination of accurate extinction coefficients and simultaneous equations for assaying chlorophylls a and b extracted with four different solvents: verification of the concentration of chlorophyll standards by atomic absorption spectroscopy. Biochim. Biophys. Acta Bioenerg. 975, 384–394. doi: 10.1016/S0005-2728(89)80347-0
Qin, Y., Ma, X., Yu, G., Wang, Q., Wang, L., Kong, L., et al. (2014). Evolutionary history of trihelix family and their functional diversification. DNA Res. 21, 499–510. doi: 10.1093/dnares/dsu016
Riano-Pachon, D. M., Ruzicic, S., Dreyer, I., and Mueller-Roeber, B. (2007). PlnTFDB: an integrative plant transcription factor database. BMC Bioinformatics 8:42. doi: 10.1186/1471-2105-8-42
Riechmann, J. L., Heard, J., Martin, G., Reuber, L., Jiang, C., Keddie, J., et al. (2000). Arabidopsis transcription factors: genome-wide comparative analysis among eukaryotes. Science 290, 2105–2110. doi: 10.1126/science.290.5499.2105
Rissler, H. M., Collakova, E., DellaPenna, D., Whelan, J., and Pogson, B. J. (2002). Chlorophyll biosynthesis. Expression of a second chl I gene of magnesium chelatase in Arabidopsis supports only limited chlorophyll synthesis. Plant Physiol. 128, 770–779. doi: 10.1104/pp.010625
Sadowski, M. I., and Jones, D. T. (2009). The sequence-structure relationship and protein function prediction. Curr. Opin. Struct. Biol. 19, 357–362. doi: 10.1016/j.sbi.2009.03.008
Sirichandra, C., Wasilewska, A., Vlad, F., Valon, C., and Leung, J. (2009). The guard cell as a single-cell model towards understanding drought tolerance and abscisic acid action. J. Exp. Bot. 60, 1439–1463. doi: 10.1093/jxb/ern340
Sokal, R. R., and Rohlf, F. J. (1995). Biometry, the Principles and Practice of Statistics in Biological Research, 3rd Edn. New York, NY: WH Freeman and Company, 321–356.
Song, A., Wu, D., Fan, Q., Tian, C., Chen, S., Guan, Z., et al. (2016). Transcriptome-wide identification and expression profiling analysis of Chrysanthemum trihelix transcription factors. Int. J. Mol. Sci. 17:198. doi: 10.3390/ijms17020198
Ton, J., Flors, V., and Mauch-Mani, B. (2009). The multifaceted role of ABA in disease resistance. Trends Plant Sci. 14, 310–317. doi: 10.1016/j.tplants.2009.03.006
Travaglia, C., Reinoso, H., Cohen, A., Luna, C., Tommasino, E., Castillo, C., et al. (2010). Exogenous ABA increases yield in field-grown wheat with moderate water restriction. J. Plant Growth Regul. 29, 366–374. doi: 10.1007/s00344-010-9147-y
Wang, X., Li, Q. T., Chen, H. W., Zhang, W. K., Ma, B., Chen, S. Y., et al. (2014). Trihelix transcription factor GT-4 mediates salt tolerance via interaction with TEM2 in Arabidopsis. BMC Plant Biol. 14:339. doi: 10.1186/s12870-014-0339-7
Wang, Z., Liu, Q., Wang, H., Zhang, H., Xu, X., Li, C., et al. (2016). Comprehensive analysis of trihelix genes and their expression under biotic and abiotic stresses in Populus trichocarpa. Sci. Rep. 6:36274. doi: 10.1038/srep36274
Weng, H., Yoo, C. Y., Gosney, M. J., Hasegawa, P. M., and Mickelbart, M. V. (2012). Poplar GTL1 is a Ca2+/calmodulin-binding transcription factor that functions in plant water use efficiency and drought tolerance. PLoS ONE 7:e32925. doi: 10.1371/journal.pone.0032925
Xi, J., Qiu, Y., Du, L., and Poovaiah, B. W. (2012). Plant-specific trihelix transcription factor AtGT2L interacts with calcium/calmodulin and responds to cold and salt stresses. Plant Sci. 18, 274–280. doi: 10.1016/j.plantsci.2011.11.013
Xie, Z. M., Zou, H. F., Lei, G., Wei, W., Zhou, Q. Y., Niu, C. F., et al. (2009). Soybean trihelix transcription factors GmGT-2A and GmGT-2B improve plant tolerance to abiotic stresses in transgenic Arabidopsis. PLoS ONE 4:e6898. doi: 10.1371/journal.pone.0006898
Xiong, L., Schumaker, K. S., and Zhu, J. K. (2002). Cell signaling during cold, drought, and salt stress. Plant Cell 14(Suppl.), S165–S183.
Yang, Y., Ma, C., Xu, Y., Wei, Q., Imtiaz, M., Lan, H., et al. (2014). A zinc finger protein regulates flowering time and abiotic stress tolerance in Chrysanthemum by modulating gibberellin biosynthesis. Plant Cell 26, 2038–2054. doi: 10.1105/tpc.114.124867
Ying, L., Chen, H., and Cai, W. (2014). BnNAC485 is involved in abiotic stress responses and flowering time in Brassica napus. Plant Physiol. Biochem. 79, 77–87. doi: 10.1016/j.plaphy.2014.03.004
Zhang, X., Lu, G., Long, W., Zou, X., Li, F., and Nishio, T. (2014). Recent progress in drought and salt tolerance studies in Brassica crops. Breed. Sci. 64, 60–73. doi: 10.1270/jsbbs.64.60
Zheng, X., Liu, H., Ji, H., Wang, Y., Dong, B., Qiao, Y., et al. (2016). The wheat GT factor TaGT2L1D negatively regulates drought tolerance and plant development. Sci. Rep. 6:27042. doi: 10.1038/srep27042
Zhou, C., Ma, Z., Zhu, L., Xiao, X., Xie, Y., Zhu, J., et al. (2016). Rhizobacterial strain Bacillus megaterium BOFC15 induces cellular polyamine changes that improve plant growth and drought resistance. Int. J. Mol. Sci. 17:976. doi: 10.3390/ijms17060976
Keywords: Brassica napus, ABA, osmotic stress, salt, BnSIP1-1, trihelix gene family
Citation: Luo J, Tang S, Mei F, Peng X, Li J, Li X, Yan X, Zeng X, Liu F, Wu Y and Wu G (2017) BnSIP1-1, a Trihelix Family Gene, Mediates Abiotic Stress Tolerance and ABA Signaling in Brassica napus. Front. Plant Sci. 8:44. doi: 10.3389/fpls.2017.00044
Received: 01 November 2016; Accepted: 09 January 2017;
Published: 26 January 2017.
Edited by:
Avinash Mishra, Central Salt & Marine Chemicals Research Institute (CSIR), IndiaReviewed by:
Mohamed Yousof Hazman, Agricultural Genetic Engineering Research Institute, EgyptRohit Joshi, Jawaharlal Nehru University, Delhi, India
Sudisha Jogaiah, Karnatak University, India
Pengcheng Wang, Purdue University, USA
Copyright © 2017 Luo, Tang, Mei, Peng, Li, Li, Yan, Zeng, Liu, Wu and Wu. This is an open-access article distributed under the terms of the Creative Commons Attribution License (CC BY). The use, distribution or reproduction in other forums is permitted, provided the original author(s) or licensor are credited and that the original publication in this journal is cited, in accordance with accepted academic practice. No use, distribution or reproduction is permitted which does not comply with these terms.
*Correspondence: Gang Wu, d3VnYW5nQGNhYXMuY24=