- Division of Applied Life Science (BK21 Plus), Plant Molecular Biology and Biotechnology Research Center, Gyeongsang National University, Jinju, South Korea
Aphids are the most destructive insect pests. They suck the sap and transmit plant viruses, causing widespread yield loss of many crops. A multifunctional endophytic bacterial strain Bacillus velezensis YC7010 has been found to induce systemic resistance against bacterial and fungal pathogens of rice. However, its activity against insects attack and underlying cellular and molecular defense mechanisms are not elucidated yet. Here, we show that root drenching of Arabidopsis seedlings with B. velezensis YC7010 can induce systemic resistance against green peach aphid (GPA), Myzus persicae. Treatment of bacterial suspension of B. velezensis YC7010 at 2 × 107 CFU/ml to Arabidopsis rhizosphere induced higher accumulation of hydrogen peroxide, cell death, and callose deposition in leaves compared to untreated plants at 6 days after infestation of GPA. Salicylic acid, jasmonic acid, ethylene, and abscisic acid were not required to confer defense against GPA in Arabidopsis plants treated by B. velezensis YC7010. Bacterial treatment with B. velezensis YC7010 significantly reduced settling, feeding and reproduction of GPA on Arabidopsis leaves via strongly expressing senescence-promoting gene PHYTOALEXIN DEFICIENT4 (PAD4) while suppressing BOTRYTIS-INDUCED KINASE1 (BIK1). These results indicate that B. velezensis YC7010-induced systemic resistance to the GPA is a hypersensitive response mainly dependent on higher expression of PAD4 with suppression of BIK1, resulting in more accumulation of hydrogen peroxide, cell death, and callose deposition in Arabidopsis.
Introduction
Plants are usually challenged by various herbivorous insects in their natural environments. They have to develop diverse defense responses to protect themselves against attacks from different insects including aphids. Green peach aphid (GPA), Myzus persicae, a phloem sap feeding insect, has a wide range of hosts. It causes severe yield losses. It is also involved in the transmission of several plant viral diseases (Kennedy et al., 1962; Matthews, 1991; Blackman and Eastop, 2000). These aphids have highly modified stylets that enable them to enter sieve elements and secrete gelling and watery saliva to a certain extent during probing and feeding. Salivation of aphid plays a major role in successful colonization. It is also associated with its virulence (Tjallingii, 2006). During infestation of plants by aphids, the major defense related plant hormones are salicylic acid (SA), jasmonic acid (JA), ethylene (ET), and abscisic acid (ABA). They are reported to be involved in the induced systemic resistance (ISR) of many plants against aphids (Morkunas and Gabryś, 2011). The SA signaling pathway is activated to induce resistance in a number of plant species by aphid feeding (Moran et al., 2002; Zhu-Salzman et al., 2004; Coppola et al., 2013). On the contrary, Pegadaraju et al. (2005) have reported that SA signaling is not involved in the defense response of Arabidopsis against aphids. It has been reported that exogenous application of JA to a tomato plant can induce systemic defense against potato aphid (Cooper and Goggin, 2005). The population of GPA is increased in ET-insensitive Arabidopsis mutant ein2, indicating that ET can also confer resistance to aphid (Kettles et al., 2013). Resistance of Arabidopsis to aphid also depends on ABA biosynthesis and signaling (Kerchev et al., 2013).
Plant defense and cell death pathways induced by pathogens and insects are often regulated by certain plant hormones to elicit the accumulation of hydrogen peroxide (H2O2) and callose deposition (De Vos et al., 2005; Ahn et al., 2007; Zhou et al., 2009). In pathogen-infected plants, the production of reactive oxygen species (ROS, e.g., H2O2), and consequentially the onset of cell death referred to as ‘hypersensitive response’ (HR) can lead to systemic resistance (Durrant and Dong, 2004; Jones and Dangl, 2006; Singh et al., 2016). ROS and local cell death are also major defense mechanisms used by plants to protect themselves against phloem sap feeding GPA (Lei et al., 2014). Arabidopsis PHYTOALEXIN DEFICIENT4 (PAD4) is especially crucial for its defense against aphids. Elevated expression of PAD4 can deter GPA from settling on plants or feeding from the sieve elements (Louis et al., 2012; Lei et al., 2014). In addition, it has been reported that PAD4 can stimulate premature leaf senescence, resulting in elevated expression of a subset of SENESENCE ASSOCIATED GENES (SAG) characterized by chlorophyll loss and cell death in GPA infested plants (Pegadaraju et al., 2005, 2007). The expression of PAD4-dependent constitutive expression of SAG13 can confer hyper-resistance of Arabidopsis to GPA infestation (Louis et al., 2010). Recently, it has been reported that the molecular mechanism of PAD4 resistance against aphid is reliant on interaction with BOTRYTIS-INDUCED KINASE1 (BIK1). The mutant bik1 can induce resistance to aphids through ROS production, cell death and leaf senescence. Such bik1 induced resistance is dependent on the expression of PAD4. However, BIK1 overexpression can make Arabidopsis plants more susceptible to aphid infestation (Lei et al., 2014). It has been shown that BIK1 can control plant defense against aphids by negatively regulating PAD4 expression (Louis and Shah, 2014).
BOTRYTIS-INDUCED KINASE1, a receptor-like cytoplasmic kinase (RLCK), is directly phosphorylated by BRASSINOSTEROID INSENSITIVE1-ASSOCIATED RECEPTOR KINASE (BAK1) and associated with FLAGELLIN-SENSITIVE2 (FLS2)/BAK1 complex in modulating pathogen-associated molecular patterns (PAMPs) mediated signaling (Lu et al., 2010; Zhang et al., 2010; Liu et al., 2013). Detection of PAMPs or microbe-associated molecular patterns (MAMPs) by particular transmembrane pattern recognition receptors is responsible for basal plant defense response collectively referred to as MAMP-triggered immunity (MTI) (Boller and Felix, 2009; Monaghan and Zipfel, 2012). The most characterized PAMP/MAMP receptors are receptor like kinases (RLKs), among which FLS2 and EF-TU RECEPTOR (EFR) can recognize bacterial elongation factor EF-Tu (Gómez-Gómez and Boller, 2000; Zipfel et al., 2006). Upon binding to their cognate MAMPs, FLS2, or EFR is associated with BAK1 (another RLK) during bacterial interaction with host (Chinchilla et al., 2007). For successful colonization of rhizobacteria and beneficial interaction with host plants, it is necessary to suppress MTI (Gutjahr and Paszkowski, 2009; Zamioudis and Pieterse, 2012). Pseudomonas fluorescens WCS417, one of plant growth promoting rhizobacteria (PGPR), has been shown to be able to suppress flagellin-triggered MTI responses and induce callose depositions during colonization in Arabidopsis (Millet et al., 2010). Callose deposition on sieve plates of rice plants can affect phloem transportation. It plays an important role in preventing brown planthopper (BPH) from ingesting the phloem sap (Hao et al., 2008). Several PGPR have been reported to use ISR to protect plant against pathogens. However, few studies have reported on ISR used by PGPR against insects (Zehnder et al., 1997; Lugtenberg and Kamilova, 2009; Pieterse et al., 2012). The main mechanisms of these bacteria involved in ISR upon pathogen infection or insect infestation include HR-type reactions, elevated cell wall or apoplastic peroxidase activity, callose deposition, and H2O2 accumulation (Conrath, 2006; Valenzuela-Soto et al., 2010; Niu et al., 2011; Rahman et al., 2015). Recently, these PGPR have been used for plant growth promotion, stress tolerance and biocontrol agents for insects and plant pathogens (Phi et al., 2010; Van de Mortel et al., 2012; Chung et al., 2015; Hossain et al., 2016; Zebelo et al., 2016). Some endophytic PGPR inhabiting the interior of host plants have shown ISR activity against insects (de Oliveira Araujo, 2015).
Recently, we have reported that novel endophytic strain of B. oryzicola YC7010 isolated from rice roots can inhibit the growth of important fungal and bacterial pathogens of rice such as Fusarium fujikuroi and Burkholderia glumae via antibiotic production and ISR (Chung et al., 2015; Hossain et al., 2016). The novelty of this species is now on debate and the name for this species was suggested to be changed as B. velezensis (Dunlap et al., 2016). The objective of this study was to determine whether B. velezensis YC7010 could induce systemic resistance against GPA in Arabidopsis and elucidate its underlying mechanism in terms of enhancing the expression of PAD4 to activate cellular defense responses.
Materials and Methods
Plant Materials, Growth Conditions, and Aphid Rearing
Wild-type Arabidopsis ecotype Columbia-0 (Col-0), NahG and mutants sid2, jar1, ein2-1, abi2-2, pad4, bik1, and bik1pad4 were used in this experiment. Seeds were sterilized with 70% (v/v) ethanol for 5 min followed by treatment with 1.2% (v/v) sodium hypochlorite (NaOCl) for 5 min. They were then washed with sterile distilled water. After sterilization, seeds were kept at 4°C for 48 h and grown on 0.5x Murashige and Skoog (MS) agar media supplemented with 1% (w/v) sucrose. Agar plates were then kept horizontally in a plant growth chamber for growing seedlings. Seedlings at 10 days old were transferred into pots containing 100 g soils autoclaved twice for 20 min at 120°C. Phloem sap-feeding GPA were cultured on cabbage (Brassica oleracea) and maintained in an environmental chamber with a long day photoperiod (16 h of light and 8 h of darkness) at 22°C with a light intensity of 100 μmol m-2 s-1. All experiments were performed in the growth chamber.
Bioassay for Induced Systemic Resistance to Aphid by Bacteria
For bacterial inoculation of Arabidopsis plants, strain B. velezensis YC7010 (KACC18228, Jeonju, Korea) was cultivated in one-tenth strength tryptic soy broth (1/10 TSB, BactoTM, Sparks, MD, USA) at 28°C for 48 h on a rotary shaker (160 rpm). Cells were then harvested by centrifugation at 6,000 × g for 15 min and suspended in a buffer solution (10 mM MgSO4) to adjust to 2 × 107 colony forming unit (CFU) ml-1 for use. Seedlings of Arabidopsis at 4 weeks old were drenched with 10 ml bacterial suspension on the rhizosphere in each pot. Equal volume of 10 mM MgSO4 was drenched as control. No-choice and choice tests were performed to assess ISR to aphid after bacterial treatment. For the no-choice tests, five second-instar nymphs were placed at 5 days after bacterial inoculation. Seven days after infestation, total aphid population (adults and nymphs) on each plant was recorded. Each treatment had eight replicates. For the choice tests, 35 adult aphids were released at an equal distance between bacterial inoculated and uninoculated plants of different genotypes. At 24 h after releasing, the number of adult aphids settled on each plant was recorded. For each comparison, eight pairs of plants were used.
Determination of Aphid Feeding Activity
Aphid feeding activity can be determined by measuring the amount of produced honeydew. To measure honeydew production, line split Whatman filter papers were placed under Arabidopsis plants of both treated and untreated plants infested by 30 adult aphids. To avoid absorbance of water from soil, a plastic membrane was placed under the filter paper. The filter papers used to collect honeydew at 3, 4, and 5 days after infestation of aphids were soaked in 0.1% (w/v) ninhydrin solution in acetone and dried in a 65°C oven for 30 min. Purple spots were shown when honeydew was stained by ninhydrin (Kim and Jander, 2007). To quantify honeydew stains, the stained filter papers were cut into pieces and extracted with 1 ml of 90% (v/v) methanol for 1 h at 4°C with continuous agitation. The absorbance of the supernatant was measured at 500 nm after centrifugation at 6,000 × g for 1 min. Methanol (90%) was used as a blank (Nisbet et al., 1994).
Measurement of Hydrogen Peroxide Content
The concentrations of H2O2 in bacterial treated plants were measured at 0, 12, 24, 48, and 72 h after aphid infestation using a spectrophotometer. Treated leaf tissues (200 mg) were homogenized with 3 ml phosphate buffer (50 mM, pH 6.8) containing 1 mM hydroxylamine (catalase inhibitor). The mixture was centrifuged at 6,000 × g for 25 min and 3 ml of the supernatant was mixed with 1 ml 0.1% (v/v) titanium sulfate in 20% (v/v) H2SO4. The absorbance of the supernatant of the mixture was then determined at 410 nm after centrifugation at 6,000 × g for 15 min. Extinction co-efficient (0.28 μmol-1 cm-1) was used to calculate H2O2 content (Jana and Choudhuri, 1982).
Histochemical Analyses of Cellular Defense Responses
For histochemical analyses of cellular defense responses, H2O2 accumulation, cell death, and callose deposition in Arabidopsis leaves were observed. Four weeks old Arabidopsis plants were treated with the same bacterial strain used for bioassay of ISR. A total of 40 aphids were placed on both treated plants and untreated control plants at 5 days after bacterial treatment. To visualize H2O2 accumulation, collected leaves at 6 days after infestation of aphids were vacuum infiltrated with 3,3’-diaminobenzidine (DAB) solution (1 mg ml-1 of DAB in pH 3.5 water). Under dark condition, leaves were immersed in the same solution overnight followed by destaining with 95% (v/v) ethanol until clear. They were preserved in 50% (v/v) ethanol. A dissecting microscope was used to capture the image of accumulated H2O2 in the leaves.
To visualize cell death of treated leaves, trypan blue staining was performed. Trypan blue was dissolved at a concentration of 0.125 mg ml-1 in lactophenol solution (phenol:lactic acid:glycerol:water [1:1:1:1]). Leaves were boiled in this staining solution for 1 min and destained in 95% (v/v) ethanol after cooling. Cell death images of leaves were captured with an Olympus Provis AX70 microscope at 10× magnifications.
To detect callose deposition, aniline blue staining was performed using published protocol (Clay et al., 2009). Buffer solution containing 10% (v/v) formaldehyde, 5% (v/v) acetic acid, and 50% (v/v) ethanol was used for fixation of Arabidopsis leaves at 37°C overnight. Fixed leaves were washed in 95% ethanol several times until clear, rinsed twice in water and then stained for 4 h or longer in the dark with 0.01% (w/v) aniline blue in 150 mM K2HPO4 (pH 9.5). Callose deposition was visualized with an Olympus Provis AX70 microscope at 10 × magnifications under UV illumination equipped with a broad band DAPI filter set. To quantify callose accumulation in the Arabidopsis leaves, images were subjected to intensity analysis using the image processing software IMAGEJ.
Determination of Bacterial Population
In order to determine the bacterial population of B. velezensis YC7010 in Arabidopsis roots during plant growth, 10 ml of cell suspension (2.0 × 107 CFU/ml) was drenched into Arabidopsis seedlings in a pot containing 100 g soils. Roots pieces (100 mg) were collected from treated seedlings at 0, 1, 2, 4, and 8 days after inoculation. Collected samples were surface-sterilized with 1.2% (v/v) NaOCl for 5 min followed by 70% ethanol treatment for 5 min. Finally, they were washed with sterile distilled water several times. The sterilized samples were homogenized in a buffer solution (10 mM MgSO4) using a sterile pestle and mortal. The aliquots were appropriately diluted before plating onto 1/10 TSB agar media supplemented with chloramphenicol (40 μgml-1) (Hossain et al., 2016). Plates were incubated at 28°C for 48 h. The CFU/g of fresh roots was counted.
Measurement of Chlorophyll Content
To measure chlorophyll content, bacterial suspension of B. velezensis YC7010 and 40 aphids were used to treat 4 weeks old Arabidopsis plants using the protocols described earlier. Leaves were then collected at 6 days after aphid infestation of treated and untreated plants. Sample tissue (10 mg) was crushed with 1 ml of 80% (v/v) acetone in a glass grinder followed by centrifugation at 13,000 × g for 5 min. The absorbance of the supernatant was measured at wavelengths of 646.8 and 663.2 nm using a spectrophotometer. Total chlorophyll content was calculated using the following formula (Lichtenthaler, 1987): Total chlorophyll (mg/fresh weight or fw) = (7.15 ∗ A663.2 + 18.71 ∗ A646.8)/1000/fw of leaves.
Quantitative RT-PCR
Bacterial suspension of B. velezensis YC7010 and 40 aphids were used to treat 4 weeks old Arabidopsis plants as described previously. Leaves were collected at 0, 12, 24, 48, and 72 h after aphid infestation of treated and untreated plants. Collected samples were frozen and ground in liquid nitrogen to a fine powder. Total RNA was extracted by using RNA extraction kit (Qiagen RNeasy Plant Mini Kit) and used to synthesis of complementary DNA using QuantiTect Reverse Transcription Kit according to the manufacturer’s instruction. Using SYBR Green Master Mix (Bio-Rad), quantitative reverse transcription RT-PCR reactions were performed according to the manufacturer’s protocol. Primers for candidate genes were designed using Primer3 software. Primer sequences are provided in Supplementary Table S1. To quantitatively determine the accumulation levels of genes, 2-ΔΔCt method (Livak and Schmittgen, 2001) was used. Expression of genes was normalized against Arabidopsis UBIQUITIN10 (AT4G05320) as an internal reference. All experiments were repeated three times and each real time PCR sample was run in triplicates.
Statistical Analysis
All data were subjected to analysis of variance (ANOVA). Mean differences were estimated by Tukey’s honestly significant difference (HSD) using statistical software SPSS 17 (SPSS Inc., Chicago, IL, USA) and Sigma plot (version 12). Statistical significance was considered when P value was less than 0.05.
Results
B. velezensis YC7010 Induces Systemic Resistance to Aphids in Arabidopsis
To determine whether B. velezensis YC7010 could induce resistance against aphids, both no-choice and choice tests were performed after drenching bacterial suspension to the rhizosphere of Arabidopsis. Such bacterial treatment significantly (P < 0.01) reduced the number of aphids than untreated control (Figures 1A,B). In the no-choice test, the number of aphids on bacterial treated plants was 20.75 per plant, which was significantly (P < 0.01) less than 35.50 on untreated plants. In the choice test, the number of aphids on bacterial treated plants was 11.38 per plant, which was also significantly (P < 0.01) lower than 22.00 on untreated plants. In the no-choice test and the choice test, the numbers of aphids on treated plants were reduced by 41.55 and 48.27%, respectively, by bacterial treatment when compared to those without bacterial treatment. In agreement with these results, aphids on bacterial treated plants showed significantly less excretion of honeydew, indicating less food intake at all time points at 3, 4 or 5 days after aphid infestation (Figures 1C,D).
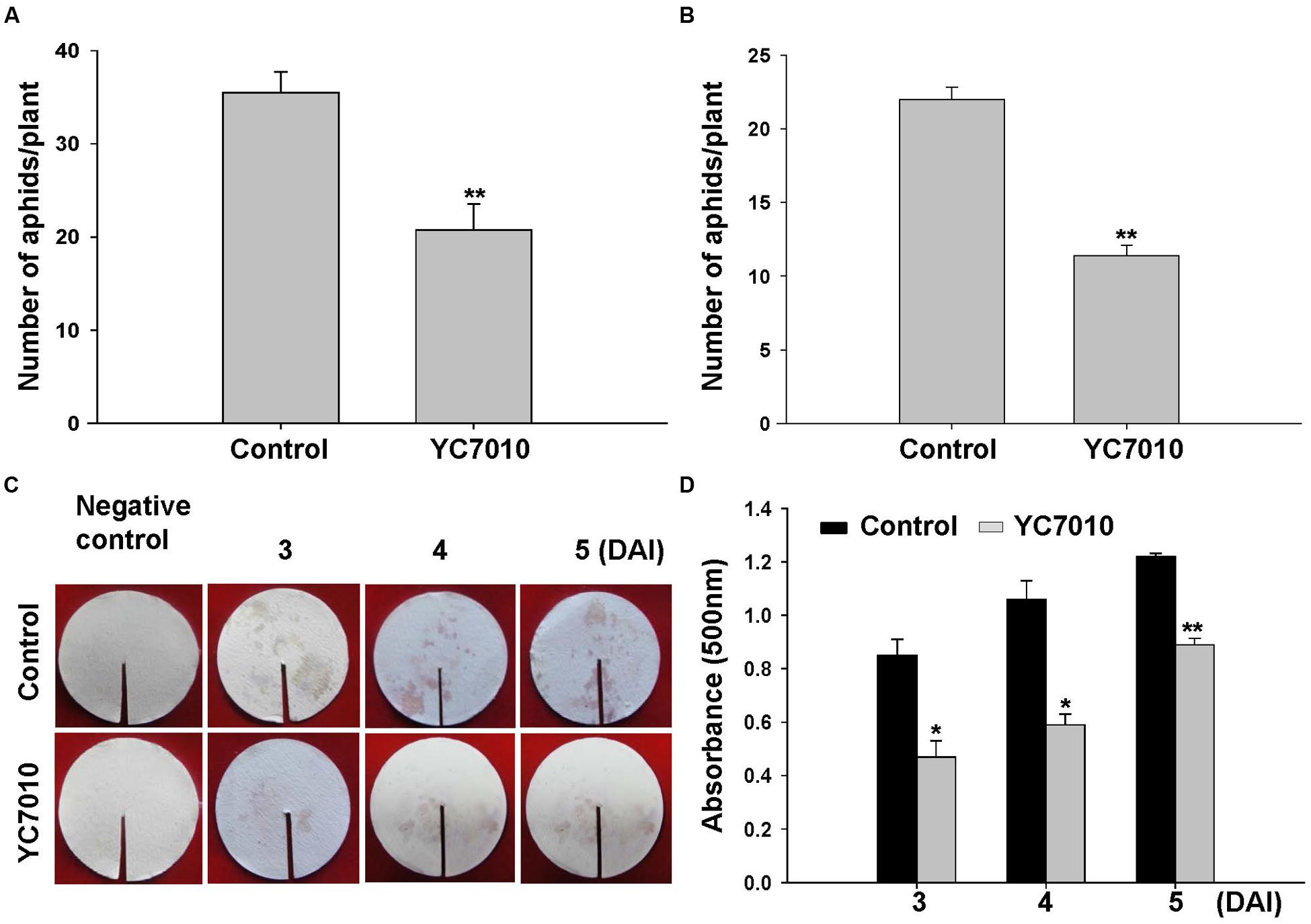
FIGURE 1. Induction of systemic resistance to green peach aphids by treating Arabidopsis Col-0 plants with B. velezensis YC7010. Bacterial suspension (2 × 107 CFU/ml) was drenched to the rhizosphere of 4-weeks old Arabidopsis plants while 10 mM MgSO4 solution was used as negative control. (A) No-choice test. Five second-instar nymphs were placed at 5 days after bacterial inoculation and the total number of aphids on the leaves was counted at 7 days later after bacterial inoculation. (B) Choice test. The number of settled aphids was counted at 24 h after releasing 35 adults between two plants of the treated plants and control plants. (C) Effect of bacteria inoculation on aphid excretion of honeydew. The quantity of excreted honeydew was measured with intensity of ninhydrin stains. (D) Quantity of honeydew secretion was determined at optical density of 500 nm. DAI, days after infestation of aphids. Data were analyzed by independent Student’s t-tests. Bars represent mean values ± standard error (SE). Statistical significance for treatment effects is marked (∗P < 0.05; ∗∗P < 0.01). All experiments were conducted three times with similar results.
B. velezensis YC7010-Induced Systemic Resistance Is Dependent on Hydrogen Peroxide Production, Cell Death, and Callose Deposition in Arabidopsis
The effect of bacterial treatment on the accumulation of H2O2, cell death and callose deposition was observed at 6 days after aphid infestation. The accumulation of H2O2 and the level of H2O2 production at 12, 24, 48, and 72 h after infestation (HAI) of aphids in bacterial inoculated plants were significantly higher than those in untreated plants. The highest content of H2O2 was 8.12 μmol g-1 fw at 24 HAI in bacterial treated leaves. It was decreased to 6.00 μmol g-1fw at 48 HAI. However, this was still significantly higher than that in untreated plants after aphid infestation (Figures 2A,B). More cell death and callose deposition were detected in bacterial treated Arabidopsis plants comparing to those of untreated plants after aphid infestation (Figures 2C–E). A combination of bacterial treatment and aphid infestation led to significantly more H2O2 production, cell death and callose deposition, which might have contributed to effective defense response against aphids.
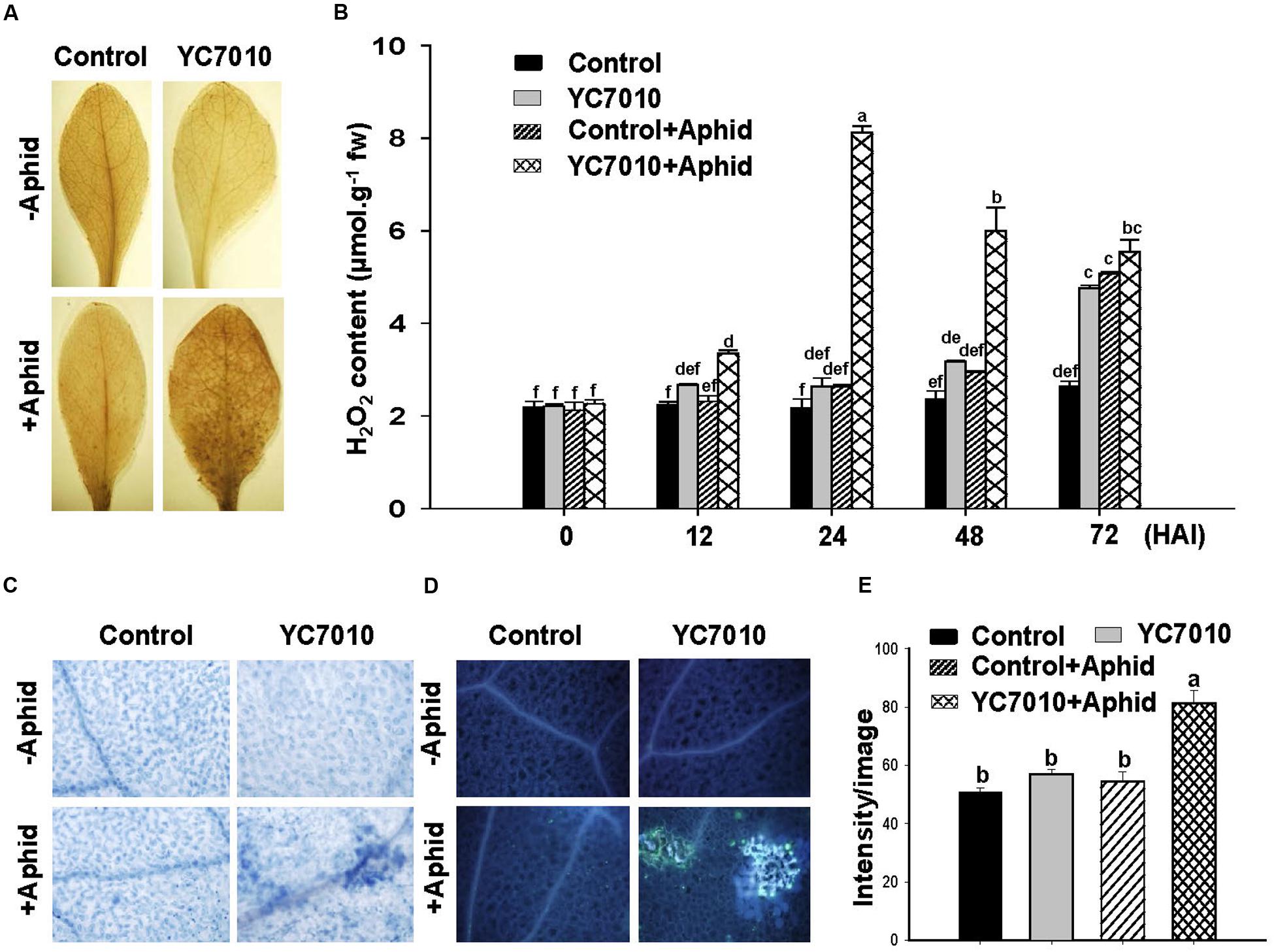
FIGURE 2. B. velezensis YC7010-mediated priming for activation of H2O2 accumulation, cell death, and callose deposition in the leaves of Arabidopsis Col-0 plants against aphid infestation. Bacterial suspension (2 × 107 CFU/ml) was used to treat 4-weeks old Arabidopsis plants and 40 aphids were placed at 5 days after bacterial treatment. (A) Arabidopsis leaves stained with 3,3’-diaminobenzidine, an indicator of H2O2, at 6 days after aphid infestation. (B) Content of H2O2 accumulated in the Arabidopsis leaves treated with bacteria at 0, 12, 24, 48, and 72 h after infestation (HAI) of aphids. (C) Arabidopsis leaves stained with trypan blue, a cell death indicator, at 6 days after aphid infestation. (D) Callose deposition in Arabidopsis leaves stained with aniline blue at 6 days after aphid infestation. (E) Quantification of the callose fluorescence on representative images using image intensity analysis with IMAGEJ software. Data were analyzed by one-way ANOVA. Means with different letters were significantly different (P < 0.05).
B. velezensis YC7010-Induced Systemic Resistance is Independent of SA, JA, ET, or ABA in Arabidopsis
To evaluate whether hormones such as SA, JA, ET, and ABA might play a role in B. velezensis YC7010 induced resistance to aphids, Arabidopsis plants such as wild ecotype Col-0, NahG, sid2, jar1, ein2-1 and abi2-2 were used in choice and no-choice tests. In both no-choice and choice tests, root drenching with bacterial suspension resulted in significant (P < 0.05) reduction in the number of aphids without significant difference among the Col-0, NahG and mutants (sid2, jar1, ein2-1 or abi2-2) (Figures 3A,B). These results showed that B. velezensis YC7010 could confer resistance to aphids in all treated Arabidopsis plants regardless of mutation. Accumulation of H2O2 and cell death were also observed in all bacterial treated Col-0, NahG, and mutants. Both H2O2 accumulation and cell death were found in bacterial treated Col-0, NahG, sid2, jar1, ein2-1, or abi2-2 plants. However, H2O2 accumulation and cell death were not detected in untreated control plants at 6 days after aphid infestation (Figures 3C,D). Collectively, these results indicate that the reduction in the number of aphids, H2O2 accumulation and cell death induced by B. velezensis YC7010 is not dependent on SA, JA, ET, or ABA in Arabidopsis.
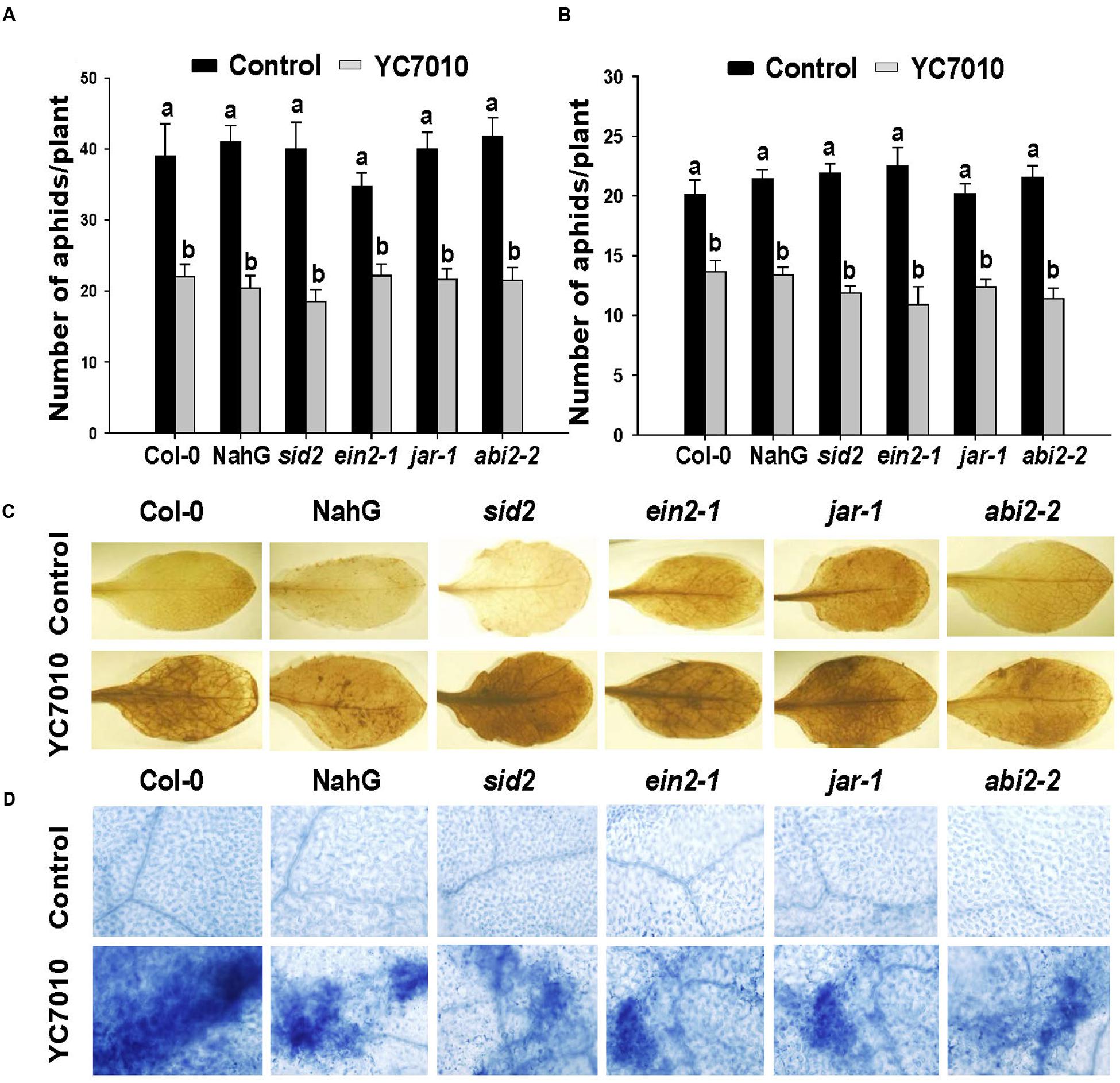
FIGURE 3. B. velezensis YC7010-induced systemic resistance to aphids, H2O2 accumulation and cell death in Arabidopsis plants were SA, JA, ET, and ABA independent. (A) No-choice test on different genotypes. Five second-instar nymphs were placed at 5 days after bacterial inoculation and the total number of aphids on the leaves was counted at 7 days later after bacterial inoculation. (B) Choice test on different genotypes. The number of settled aphids was counted at 24 h after releasing 35 adults between two plants of the treated plants and control plants. (C) Representative leaf images of 3,3’-diaminobenzidine staining (H2O2 indicator). (D) Trypan blue staining (cell death indicator). Untreated control (top) or treated plants (bottom) at 6 days after aphids infestation. Data were analyzed by one-way ANOVA. Means with different letters were significantly different (P < 0.05).
B. velezensis YC7010-Induced Aphid Resistance is Dependent on Interactions among PAD4, BIK1, and SAG13
To investigate whether B. velezensis YC7010-induced aphid resistance was dependent on PAD4 associated with BIK1, we examined aphid performance on wild type Col-0 and mutants (pad4, bik1, and bik1pad4) of Arabidopsis treated with B. velezensis YC7010 (Figure 4). In both no-choice and choice tests, no significant difference in the number of aphids between bacterial treated and untreated all mutants was found. However, significantly less number of aphids was found on bacteria treated Col-0 Arabidopsis than that on untreated control Arabidopsis (Figures 4A,B). On the other hand, the number of aphids on bacterial treated or untreated pad4 and bik1pad4 mutants was higher than that on bik1 (Figure 4A). Interestingly, in the no-choice test, aphid growth was suppressed in both treated and untreated bik1 mutants. However, in the choice test, the number of aphids was not significantly different on these mutants regardless of bacterial treatment (Figure 4B). Accumulation of H2O2, cell death and callose were observed on bacterial inoculated Col-0, bik1 and untreated bik1 mutants after aphid infestation. However, none of these responses was detected on bacterial treated or untreated control pad4 or bik1pad4 mutants even with aphid infestation (Figures 4C–E). These results suggest that PAD4 is required for H2O2 accumulation, cell death and callose deposition in Arabidopsis. More H2O2 accumulation, cell death and callose deposition were found on bacterial treated Col-0 and bik1 plants treated with or without B. velezensis YC7010. Therefore, we investigated the expression patterns of PAD4 and BIK1 genes in Col-0 plants. At every time point, the expression level of PAD4 was higher in B. velezensis YC7010 treated plants than that in untreated plants (Figure 5A). The highest expression level of PAD4 was found in bacterial treated plants at 48 HAI of aphids. On the contrary, the expression level of BIK1 was higher in untreated plant compared to that in bacterial treated plants with aphid infestation (Figure 5B). As PAD4 can stimulate premature leaf senescence in aphids-infested Arabidopsis plants, we investigated whether the expression level of senescence associated SAG13 gene was affected by B. velezensis YC7010 in Col-0 plants. The expression level of SAG13 was higher in bacterial treated plants than in untreated plants at all time points after aphid infestation (Figure 5C). The highest expression was found in bacterial treated plants at 72 HAI of aphids. Lower chlorophyll content was found in most bacterial treated plants (except pad4, bik1, and bik1pad4) compared to that in untreated control Col-0 plants (Supplementary Figure S1). The contents of chlorophyll in the leaves of pad4, bik1, and bik1pad4 mutants in treated and untreated plants were similar to each other. The chlorophyll content in bik1 mutant was lower than that in pad4 or bik1pad4 mutant (treated or untreated), although the difference was not statistically significant. Bacterial count in the roots of bik1 mutant was higher than that of Col-0 (Figure 6), indicating that root colonization of B. velezensis YC7010 was suppressed by BIK1 in Arabidopsis plants.
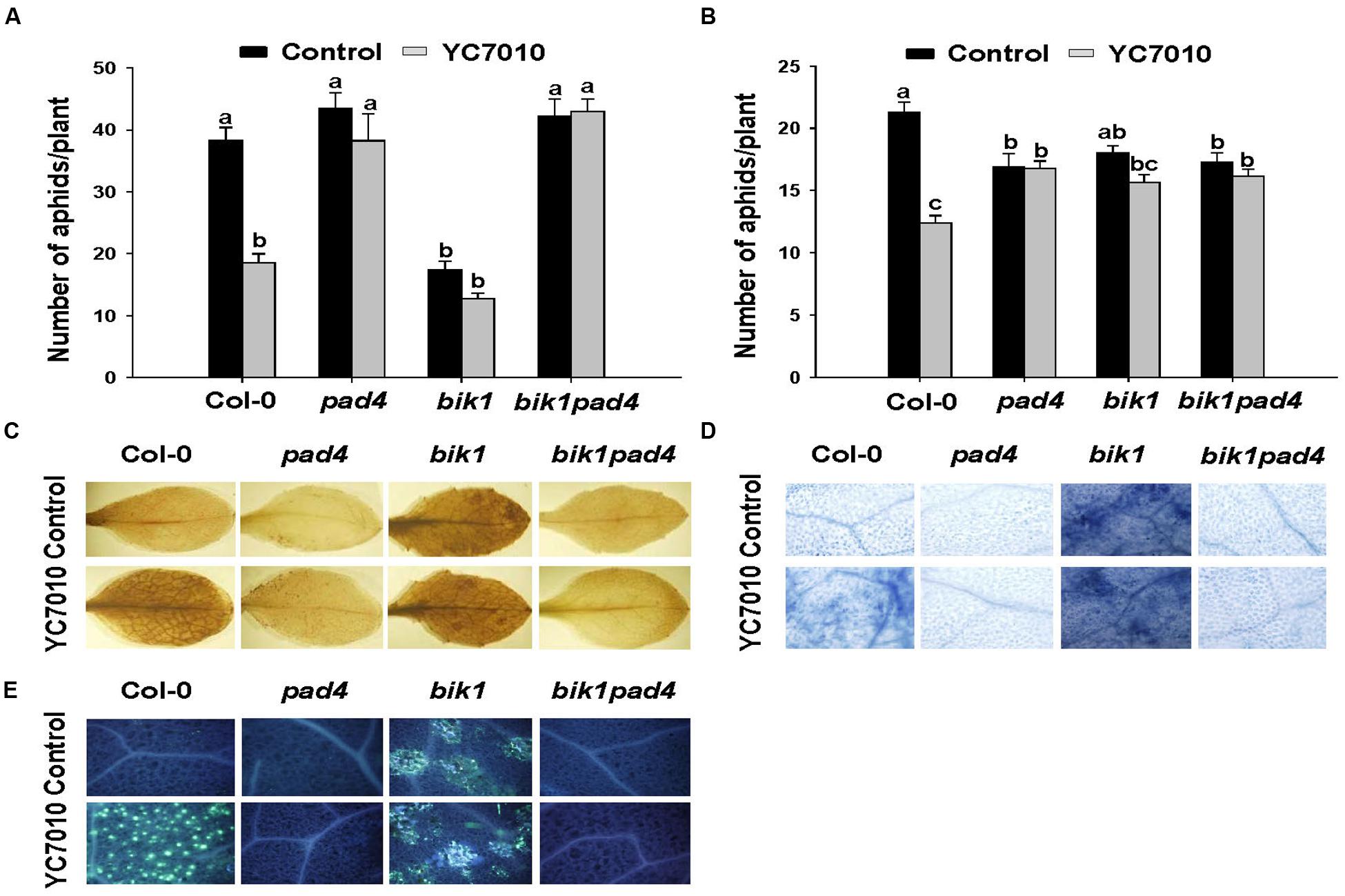
FIGURE 4. B. velezensis YC7010-induced systemic resistance to aphids, H2O2 accumulation, cell death, and callose deposition in Arabidopsis plants were PAD4 dependent. (A) No-choice test on different genotypes. Five second-instar nymphs were placed at 5 days after bacterial inoculation and the total number of aphids on the leaves was counted at 7 days later after bacterial inoculation. (B) Choice-test on different genotypes. The number of settled aphids was counted at 24 h after releasing 35 adults between two plants of the treated plants and control plants. (C) Representative leaf images of 3,3’-diaminobenzidine staining (H2O2 indicator). (D) Trypan blue staining (cell death indicator). (E) Callose deposition in Arabidopsis leaves stained with aniline blue. Untreated control (top) or treated plants (bottom) at 6 days after aphids infestation. Data were analyzed by one-way ANOVA. Means with different letters were significantly different (P < 0.05).
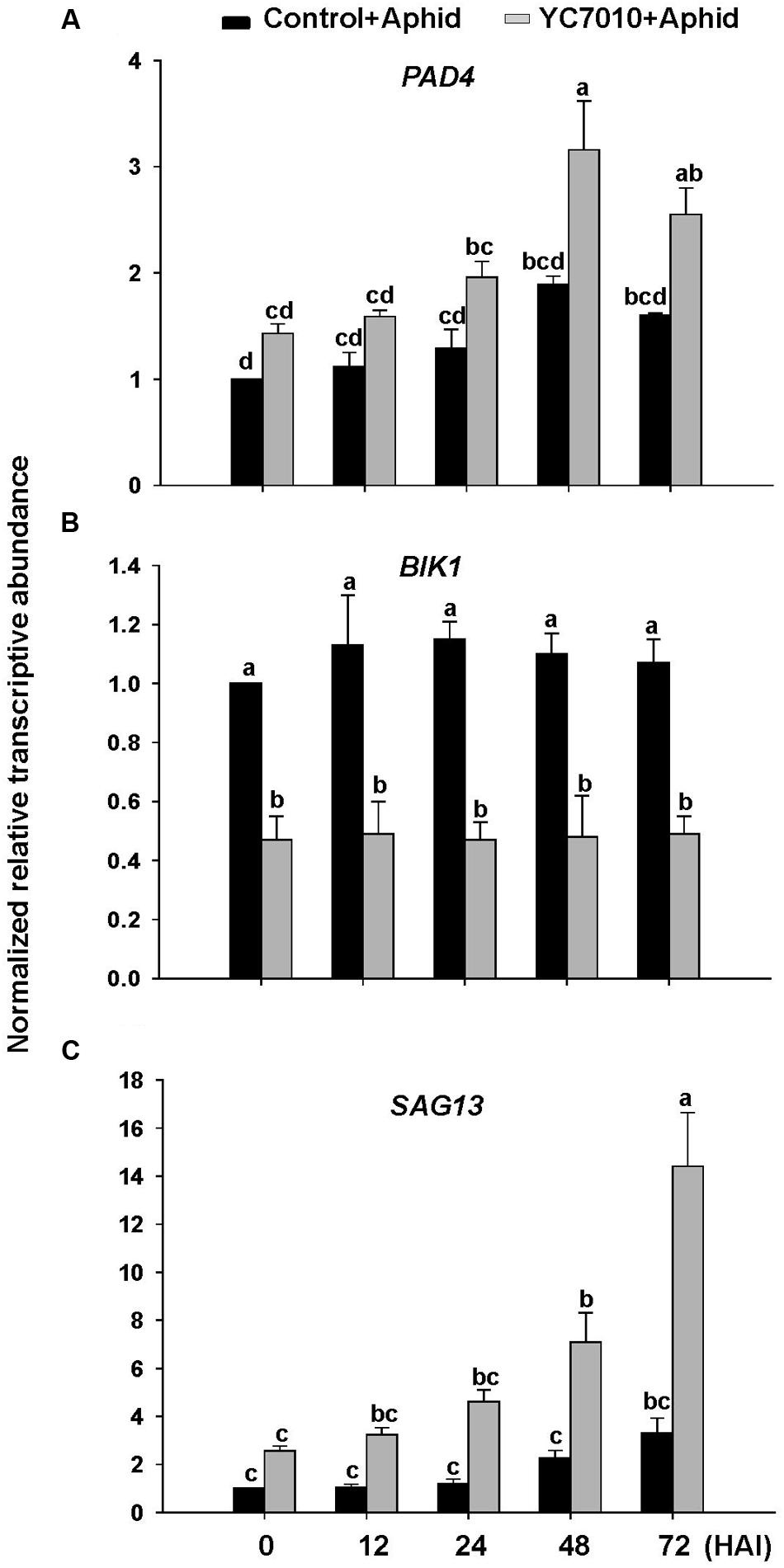
FIGURE 5. Gene expression in leaves of Arabidopsis Col-0 plants after infestation with aphids. Relative transcriptional levels of (A) PAD4, (B) BIK1, and (C) SAG13. Arabidopsis plants were treated with B. velezensis YC7010. At 5 days after treatment, 40 aphids were placed on both treated plants and control plants. Transcriptional levels were determined at 0, 12, 24, 48, and 72 h after infestation (HAI). Error bars represent standard error of mean. Each real time PCR sample was run in triplicates. Data were analyzed by one-way ANOVA. Means with different letters were significantly different (P < 0.05).
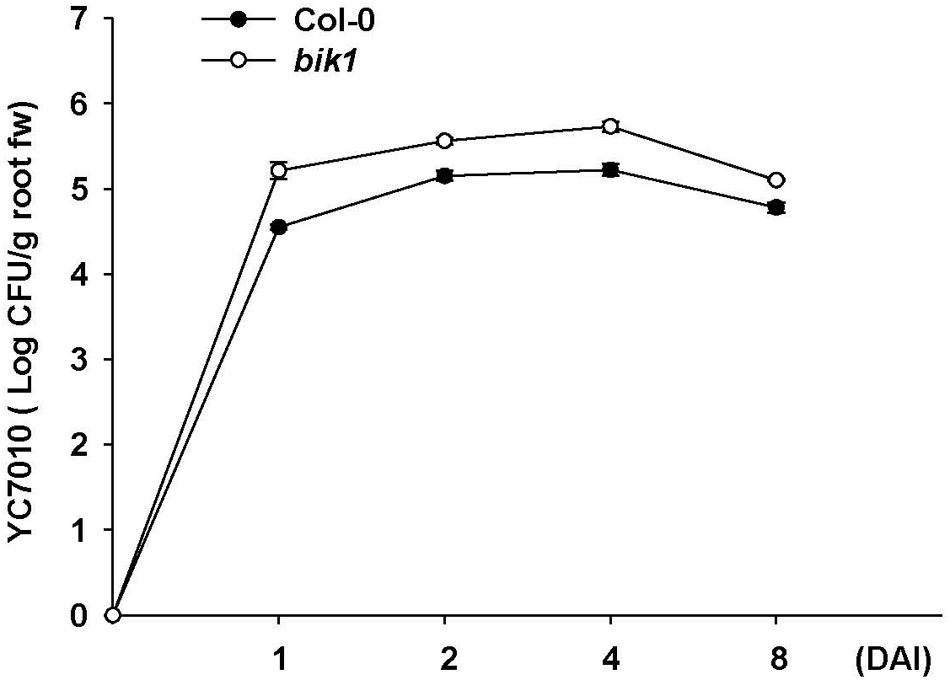
FIGURE 6. Population of B. velezensis YC7010 colonized in the root of Arabidopsis Col-0 or bik1 mutant plants. The number of bacterial colonies grown on 1/10 TSB agar media supplemented with chloramphenicol (40 μg/ml) for 48 h at 28°C was counted using root pieces sampled at 0, 1, 2, 4, and 8 days after inoculation (DAI). Error bars represent standard error of mean.
Discussion
Arabidopsis roots colonized by certain beneficial PGPR can activate ISR response that is effective against a broad range of plant pathogens (Van Oosten et al., 2008; Van Wees et al., 2008; Van der Ent et al., 2009). Likewise, some non-pathogenic rhizobacteria or endophytic bacteria commonly found inside roots also can enhance plant resistance against insect pests (Pineda et al., 2010; de Oliveira Araujo, 2015). These bacteria with ability to enhance plant growth and development have the potential to be utilized for biological control of numerous insect pests. We have previously identified an endophytic B. velezensis YC7010 with anti-microbial, plant growth-promoting and systemic resistance-inducing activities (Chung et al., 2015). In this study, our results demonstrated that root drenching of Arabidopsis with B. velezensis YC7010 suspension resulted in the establishment of an ISR against GPA, regardless of test methods (choice or no-choice tests, Figure 1). Up to date, few reports have been published on tritrophic interactions among bacteria, insects and host plants. Reports on ISR by endophytic bacteria is especially limited. Van de Mortel et al. (2012) have reported that colonization of Arabidopsis roots by non-pathogenic rhizobacteria can induce resistance against lepidopteran insect herbivore Spodoptera exigua, in agreement with our results. Less honeydew excretion by GPA also indicates less food intake from bacterial treated plants.
Inoculation of B. velezensis YC7010 enhanced H2O2 production, cell death, and callose deposition with aphid infestation in Arabidopsis (Figure 2). This indicates that ISR by this bacterial strain might be due to cellular responses, resulting in early cell death and callose deposition as HR. Insect feeding induced oxidative stress is an important component of plant defense to attacking insects. ROS detoxification may decrease antioxidant levels but increase toxic oxidation products in corn earworm infested soybean plants (Bi and Felton, 1995). Increased level of H2O2 and other oxidative products of ROS in plants can directly damage the midgut of insects and inhibit their growth. High mortality of insects by consumption of artificial diets containing H2O2 also supports the effect of ROS on the suppression of GPA (Liu et al., 2010). Another study has shown that higher level of H2O2 accumulation in rice (Oryza sativa) can enhance the resistance against phloem sap sucking BPH (Nilaparvata lugens) (Zhou et al., 2009). In addition, cellular accumulation of H2O2 can lead to plant cell death which may act as HR to GPA (Hoeberichts and Woltering, 2003). Cell death is considered as a plant defense factor against aphid by manipulating host nutritional quality in plant–microbe interactions (Goggin, 2007). Callose deposition is also an important defense mechanism that prevents insects from ingesting phloem sap (Hao et al., 2008). Microbes-mediated ISR is often associated with accumulation of H2O2, cell death, and deposition of callose in plant–pathogen interactions (Conrath et al., 2002; Jacobs et al., 2011). However, no such report has been published on three-way interaction of rhizobacteria (B. velezensis YC7010), host plants and aphids through ISR associated with these defense responses. It is possible that H2O2-induced cell death in infested and adjacent cells could limit photoassimilates flow to the feeding sites, which can move aphids away from their feeding sites. No discrete accumulation of H2O2 and callose deposition was detected in bacterial treated or untreated leaves before aphid infestation. However, accumulation of H2O2 and callose deposition were observed in leaves infested with GPA 6 days later. On the other hand, more H2O2 accumulation was found only in bacterial treated plants with GPA infestation at different time points (Figure 2). Similarly, accumulation of H2O2 and callose deposition was observed in Arabidopsis treated with rhizobacteria or inoculated by pathogens (Ahn et al., 2007). Primed plants show faster and/or stronger activation of cellular defenses when subsequently challenged by pathogen or insect attack, resulting in enhanced level of resistance (Conrath, 2011). These results suggest that B. velezensis YC7010 can induce priming responses against GPA in Arabidopsis, which is partially similar to results of previous studies, supporting the perception that ISR by beneficial microbes is commonly based on defense priming (Pieterse et al., 2014).
Our results showed that B. velezensis YC7010-mediated ISR to aphids were not dependent on SA, JA, ET, or ABA that were not associated with H2O2 accumulation or cell death in bacterial treated Arabidopsis plants (Figure 3). In other previous studies on aphid defense mechanisms, SA is not essential, while JA, ET, and ABA are not mainly involved in defense of Arabidopsis plants against aphid either (Pegadaraju et al., 2005; Lei et al., 2014). On the contrary, PAD4 was found to be required for induction of B. velezensis YC7010-mediated resistance to aphid in this study. In addition, root drenching with B. velezensis YC7010 enhanced the expression of PAD4 in Arabidopsis plants after aphid infestation (Figures 4 and 5A). Aphid feeding is well known to induce the expression of PAD4 which is required for cell death-mediated resistance. It has been reported that transgenic plants with overexpression of PAD4 can enhance their resistance against GPA more than wild type Arabidopsis Col-0 (Pegadaraju et al., 2005, 2007). In this study, the loss of BIK1 function promoted the induced resistance against aphid in the no-choice test without bacterial treatment. HRs such as H2O2 accumulation and cell death were observed in both bacterial treated and untreated plants. However, they were absent in bik1pad4 mutant, suggesting that BIK1 might not directly repress, but indirectly modulate cell death pathway through PAD4. Root drenching with B. velezensis YC7010 suppressed the expression of MTI related gene BIK1 (Figure 5B). However, the number of B. velezensis YC7010 in the root was higher in bik1 mutant than that in wild type Col-0, indicating that suppression of BIK1 might contribute to stable colonization of B. velezensis YC7010 (Figure 6). Similar to our results, it has been reported that root inoculation with Bacillus cereus AR156 can actively block immune responses in Arabidopsis roots in order to establish a compatible interaction with the host which is important for root colonization by bacteria (Niu et al., 2011). Lei et al. (2014) have also shown that PAD4 expression is much higher in bik1 mutant to suppress aphids. These results collectively demonstrate that root drenching of B. velezensis YC7010 can effectively suppress the growth of aphids in leaves via expression of PAD4 which depends on the suppression of BIK1 in Arabidopsis.
The colonization of Arabidopsis roots by B. velezensis YC7010 resulted in enhanced expression level of SAG13 as well as chlorophyll loss in leaves upon aphid infestation (Figure 5C; Supplementary Figure S1). Senescence-associated processes have negative effect on aphid growth. For example, a gall aphid induced premature senescence in Pistacia palaestina trees has been shown to be correlated with reduced performance of another aphid feeding on the same leaflet (Inbar et al., 1995). However, PAD4 stimulates the premature senescence of leaves which can confer resistance to aphids (Pegadaraju et al., 2005). These results suggested that root drenching of B. velezensis YC7010 can elevate the expression of PAD4 and activate premature leaf senescence which is involved in resistance to aphid.
In summary, B. velezensis YC7010 root treatment could induce primed systemic resistance against GPA in Arabidopsis. Root colonization of Arabidopsis by B. velezensis YC7010 suppressed the expression level of BIK1, resulting in higher expression level of PAD4 and SAG13 in bacterial treated plants. Enhanced expression of PAD4 triggered more rapid H2O2 accumulation, cell death, and callose deposition in bacterial treated Arabidopsis than in untreated plants after aphid infestation. To the best of our knowledge, this is the first report on the mechanism of ISR against aphid by an endophytic PGPR. In this aspect, the results of this study will be helpful for developing environmental friendly management strategies for insect pests using beneficial endophytic bacteria.
Author Contributions
This study was designed by YC, MR, AK, and MTH. All experiments in this study were performed by MR. Data was analyzed by MR, AK, and MTH. The manuscript was written by YR and MR.
Funding
This work was carried out with the support of “Cooperative Research Program for Agriculture Science and Technology Development (PJ01104901)” funded by Rural Development Administration, Republic of Korea. MR, AK, and MTH were supported by a scholarship from the BK21 Plus Program, the Ministry of Education, Republic of Korea.
Conflict of Interest Statement
The authors declare that the research was conducted in the absence of any commercial or financial relationships that could be construed as a potential conflict of interest.
Acknowledgments
We thank Jian-Min Zhou (Institute of Genetics and Developmental Biology, Chinese Academy of Sciences, Beijing, China) and Tesfaye Mengiste (Department of Botany and Plant Pathology, Purdue University, USA) for providing Arabidopsis mutants bik1 and bik1pad4. We also thank Monica Höfte (Department of Crop Protection, Ghent University, Belgium) for giving good suggestions and comments on the manuscript.
Supplementary Material
The Supplementary Material for this article can be found online at: http://journal.frontiersin.org/article/10.3389/fpls.2017.00211/full#supplementary-material
References
Ahn, I. P., Lee, S. W., and Suh, S. C. (2007). Rhizobacteria-induced priming in Arabidopsis is dependent on ethylene, jasmonic acid, and NPR1. Mol. Plant Microbe Interact. 20, 759–768. doi: 10.1094/MPMI-20-7-0759
Bi, J. L., and Felton, G. W. (1995). Foliar oxidative stress and insect herbivory: primary compounds, secondary metabolites, and reactive oxygen species as components of induced resistance. J. Chem. Ecol. 21, 1511–1530. doi: 10.1007/BF02035149
Blackman, R. L., and Eastop, V. F. (2000). Aphids on the World’s Crops an Identification and Information Guide. New York, NY: Willey and Sons.
Boller, T., and Felix, G. (2009). A renaissance of elicitors: perception of microbe-associated molecular patterns and danger signals by pattern-recognition receptors. Annu. Rev. Plant Biol. 60, 379–406. doi: 10.1146/annurev.arplant.57.032905.105346
Chinchilla, D., Zipfel, C., Robatzek, S., Kemmerling, B., Nürnberger, T., Jones, J. D., et al. (2007). A flagellin-induced complex of the receptor FLS2 and BAK1 initiates plant defence. Nature 448, 497–500. doi: 10.1038/nature05999
Chung, E. J., Hossain, M. T., Khan, A., Kim, K. H., Jeon, C. O., and Chung, Y. R. (2015). Bacillus oryzicola sp. nov., an endophytic bacterium isolated from the roots of rice with antimicrobial, plant growth promoting, and systemic resistance inducing activities in rice. Plant Pathol. J. 31, 1–13. doi: 10.5423/PPJ.OA.12.2014.0136
Clay, N. K., Adio, A. M., Denoux, C., Jander, G., and Ausubel, F. M. (2009). Glucosinolate metabolites required for an Arabidopsis innate immune response. Science 323, 95–101. doi: 10.1126/science.1164627
Conrath, U. (2006). Systemic acquired resistance. Plant Signal. Behav. 1, 179–184. doi: 10.4161/psb.1.4.3221
Conrath, U. (2011). Molecular aspects of defence priming. Trends Plant Sci. 16, 524–531. doi: 10.1016/j.tplants.2011.06.004
Conrath, U., Pieterse, C. M., and Mauch-Mani, B. (2002). Priming in plant-pathogen interactions. Trends Plant Sci. 7, 210–216. doi: 10.1016/S1360-1385(02)02244-6
Cooper, W. R., and Goggin, F. L. (2005). Effects of jasmonate-induced defenses in tomato on the potato aphid, Macrosiphum euphorbiae. Entomol. Exp. Appl. 115, 107–115. doi: 10.1111/j.1570-7458.2005.00289.x
Coppola, V., Coppola, M., Rocco, M., Digilio, M. C., D’Ambrosio, C., Renzone, G., et al. (2013). Transcriptomic and proteomic analysis of a compatible tomato-aphid interaction reveals a predominant salicylic acid-dependent plant response. BMC Genomics 14:515. doi: 10.1186/1471-2164-14-515
de Oliveira Araujo, E. (2015). Rhizobacteria in the control of pest insects in agriculture. Afr. J. Plant Sci. 9, 368–373. doi: 10.5897/AJPS2015.1318
De Vos, M., Van Oosten, V. R., Van Poecke, R. M., Van Pelt, J. A., Pozo, M. J., Mueller, M. J., et al. (2005). Signal signature and transcriptome changes of Arabidopsis during pathogen and insect attack. Mol. Plant Microbe Interact. 18, 923–937. doi: 10.1094/MPMI-18-0923
Dunlap, C. A., Kim, S. J., Kwon, S. W., and Rooney, A. P. (2016). Bacillus velezensis is not a later heterotypic synonym of Bacillus amyloliquefaciens; Bacillus methylotrophicus, Bacillus amyloliquefaciens subsp. plantarum and ‘Bacillus oryzicola’ are later heterotypic synonyms of Bacillus velezensis based on phylogenomics. Int. J. Syst. Evol. Microbiol. 66, 1212–1217.
Durrant, W. E., and Dong, X. (2004). Systemic acquired resistance. Annu. Rev. Phytopathol. 42, 185–209. doi: 10.1146/annurev.phyto.42.040803.140421
Goggin, F. L. (2007). Plant-aphid interactions: molecular and ecological perspectives. Curr. Opin. Plant Biol. 10, 399–408. doi: 10.1016/j.pbi.2007.06.004
Gómez-Gómez, L., and Boller, T. (2000). FLS2: an LRR receptor-like kinase involved in the perception of the bacterial elicitor flagellin in Arabidopsis. Mol. Cell 5, 1003–1011. doi: 10.1016/S1097-2765(00)80265-8
Gutjahr, C., and Paszkowski, U. (2009). Weights in the balance: jasmonic acid and salicylic acid signaling in root-biotroph interactions. Mol. Plant Microbe Interact. 22, 763–772. doi: 10.1094/MPMI-22-7-0763
Hao, P., Liu, C., Wang, Y., Chen, R., Tang, M., Du, B., et al. (2008). Herbivore-induced callose deposition on the sieve plates of rice: an important mechanism for host resistance. Plant Physiol. 146, 1810–1820. doi: 10.1104/pp.107.111484
Hoeberichts, F. A., and Woltering, E. J. (2003). Multiple mediators of plant programmed cell death: interplay of conserved cell death mechanisms and plant-specific regulators. Bioessays 25, 47–57. doi: 10.1002/bies.10175
Hossain, M. T., Khan, A., Chung, E. J., Rashid, H., and Chung, Y. R. (2016). Biological control of rice bakanae by an endophytic Bacillus oryzicola YC7007. Plant Pathol. J. 32, 228–241. doi: 10.5423/PPJ.OA.10.2015.0218
Inbar, M., Eshel, A., and Wool, D. (1995). Interspecific competition among phloem-feeding insects mediated by induced host-plant sinks. Ecology 76, 1506–1515. doi: 10.2307/1938152
Jacobs, S., Zechmann, B., Molitor, A., Trujillo, M., Petutschnig, E., Lipka, V., et al. (2011). Broad-spectrum suppression of innate immunity is required for colonization of Arabidopsis roots by the fungus Piriformospora indica. Plant Physiol. 156, 726–740. doi: 10.1104/pp.111.176446
Jana, S., and Choudhuri, M. A. (1982). Glycolate metabolism of three submersed aquatic angiosperms during ageing. Aquat. Bot. 12, 345–354. doi: 10.1016/0304-3770(82)90026-2
Jones, J. D., and Dangl, J. L. (2006). The plant immune system. Nature 444, 323–329. doi: 10.1038/nature05286
Kennedy, J. S., Day, M. F., and Eastop, V. F. (1962). A Conspectus of Aphids as Vectors of Plant Viruses. London: Commonwealth Institute of Entomology.
Kerchev, P. I., Karpiñska, B., Morris, J. A., Hussain, A., Verrall, S. R., Hedley, P. E., et al. (2013). Vitamin C and the abscisic acid-insensitive 4 transcription factor are important determinants of aphid resistance in Arabidopsis. Antioxid. Redox Signal. 18, 2091–2105. doi: 10.1089/ars.2012.5097
Kettles, G. J., Drurey, C., Schoonbeek, H. J., Maule, A. J., and Hogenhout, S. A. (2013). Resistance of Arabidopsis thaliana to the green peach aphid, Myzus persicae, involves camalexin and is regulated by microRNAs. New Phytol. 198, 1178–1190. doi: 10.1111/nph.12218
Kim, J. H., and Jander, G. (2007). Myzus persicae (green peach aphid) feeding on Arabidopsis induces the formation of a deterrent indole glucosinolate. Plant J. 49, 1008–1019. doi: 10.1111/j.1365-313X.2006.03019.x
Lei, J., Finlayson, S. A., Salzman, R. A., Shan, L., and Zhu-Salzman, K. (2014). BOTRYTIS-INDUCED KINASE1 modulates Arabidopsis resistance to green peach aphids via PHYTOALEXIN DEFICIENT4. Plant Physiol. 165, 1657–1670. doi: 10.1104/pp.114.242206
Lichtenthaler, H. K. (1987). Chlorophyll and carotenoids: pigments of photosynthetic biomembranes. Methods Enzymol. 148, 350–382. doi: 10.1016/0076-6879(87)48036-1
Liu, X., Williams, C. E., Nemacheck, J. A., Wang, H., Subramanyam, S., Zheng, C., et al. (2010). Reactive oxygen species are involved in plant defense against a gall midge. Plant Physiol. 152, 985–999. doi: 10.1104/pp.109.150656
Liu, Z., Wu, Y., Yang, F., Zhang, Y., Chen, S., Xie, Q., et al. (2013). BIK1 interacts with PEPRs to mediate ethylene-induced immunity. Proc. Natl. Acad. Sci. U.S.A. 110, 6205–6210. doi: 10.1073/pnas.1215543110
Livak, K. J., and Schmittgen, T. D. (2001). Analysis of relative gene expression data using real-time quantitative PCR and the 2-ΔΔCT method. Methods 25, 402–408. doi: 10.1006/meth.2001.1262
Louis, J., Gobbato, E., Mondal, H. A., Feys, B. J., Parker, J. E., and Shah, J. (2012). Discrimination of Arabidopsis PAD4 activities in defense against green peach aphid and pathogens. Plant Physiol. 158, 1860–1872. doi: 10.1104/pp.112.193417
Louis, J., Leung, Q., Pegadaraju, V., Reese, J., and Shah, J. (2010). PAD4-dependent antibiosis contributes to the ssi2-conferred hyper-resistance to the green peach aphid. Mol. Plant Microbe Interact. 23, 618–627. doi: 10.1094/MPMI-23-5-0618
Louis, J., and Shah, J. (2014). Plant defence against aphids: the PAD4 signalling nexus. J. Exp. Bot. 66, 449–454. doi: 10.1093/jxb/eru454
Lu, D., Wu, S., Gao, X., Zhang, Y., Shan, L., and He, P. (2010). A receptor-like cytoplasmic kinase, BIK1, associates with a flagellin receptor complex to initiate plant innate immunity. Proc. Natl. Acad. Sci. U.S.A. 107, 496–501. doi: 10.1073/pnas.0909705107
Lugtenberg, B., and Kamilova, F. (2009). Plant-growth-promoting rhizobacteria. Annu. Rev. Microbiol. 63, 541–556. doi: 10.1146/annurev.micro.62.081307.162918
Matthews, R. E. F. (ed.). (1991). “Relationships between plant viruses and invertebrates,” in Plant Virology, 3rd Edn (San Diego, CA: Academic Press), 520–561. doi: 10.1016/B978-0-12-480553-8.50019-5
Millet, Y. A., Danna, C. H., Clay, N. K., Songnuan, W., Simon, M. D., Werck-Reichhart, D., et al. (2010). Innate immune responses activated in Arabidopsis roots by microbe-associated molecular patterns. Plant Cell 22, 973–990. doi: 10.1105/tpc.109.069658
Monaghan, J., and Zipfel, C. (2012). Plant pattern recognition receptor complexes at the plasma membrane. Curr. Opin. Plant Biol. 15, 349–357. doi: 10.1016/j.pbi.2012.05.006
Moran, P. J., Cheng, Y., Cassell, J. L., and Thompson, G. A. (2002). Gene expression profiling of Arabidopsis thaliana in compatible plant-aphid interactions. Arch. Insect Biochem. Physiol. 51, 182–203. doi: 10.1002/arch.10064
Morkunas, I., and Gabryś, B. (2011). Phytohormonal signaling in plant responses to aphid feeding. Acta Physiol. Plant. 33, 2057–2073. doi: 10.1007/s11738-011-0751-7
Nisbet, A. J., Woodford, J. A. T., and Strang, R. H. C. (1994). Quantifying aphid feeding on non-radioactive food sources. Entomol. Exp. Appl. 72, 85–89. doi: 10.1111/j.1570-7458.1994.tb01805.x
Niu, D. D., Liu, H. X., Jiang, C. H., Wang, Y. P., Wang, Q. Y., Jin, H. L., et al. (2011). The plant growth-promoting rhizobacterium Bacillus cereus AR156 induces systemic resistance in Arabidopsis thaliana by simultaneously activating salicylate-and jasmonate/ethylene-dependent signaling pathways. Mol. Plant Microbe Interact. 24, 533–542. doi: 10.1094/MPMI-09-10-0213
Pegadaraju, V., Knepper, C., Reese, J., and Shah, J. (2005). Premature leaf senescence modulated by the Arabidopsis PHYTOALEXIN DEFICIENT4 gene is associated with defense against the phloem-feeding green peach aphid. Plant Physiol. 139, 1927–1934. doi: 10.1104/pp.105.070433
Pegadaraju, V., Louis, J., Singh, V., Reese, J. C., Bautor, J., Feys, B. J., et al. (2007). Phloem-based resistance to green peach aphid is controlled by Arabidopsis PHYTOALEXIN DEFICIENT4 without its signaling partner ENHANCED DISEASE SUSCEPTIBILITY1. Plant J. 52, 332–341. doi: 10.1111/j.1365-313X.2007.03241.x
Phi, Q.-T., Park, Y.-M., Seul, K.-J., Ryu, C.-M., Park, S.-H., Kim, J.-G., et al. (2010). Assessment of root-associated Paenibacillus polymyxa groups on growth promotion and induced systemic resistance in pepper. J. Microbiol. Biotechnol. 20, 1605–1613.
Pieterse, C. M., Van der Does, D., Zamioudis, C., Leon-Reyes, A., and Van Wees, S. C. (2012). Hormonal modulation of plant immunity. Annu. Rev. Cell Dev. Biol. 28, 489–521. doi: 10.1146/annurev-cellbio-092910-154055
Pieterse, C. M., Zamioudis, C., Berendsen, R. L., Weller, D. M., Van Wees, S. C., and Bakker, P. A. (2014). Induced systemic resistance by beneficial microbes. Annu. Rev. Phytopathol. 52, 347–375. doi: 10.1146/annurev-phyto-082712-102340
Pineda, A., Zheng, S. J., van Loon, J. J., Pieterse, C. M., and Dicke, M. (2010). Helping plants to deal with insects: the role of beneficial soil-borne microbes. Trends Plant Sci. 15, 507–514. doi: 10.1016/j.tplants.2010.05.007
Rahman, A., Uddin, W., and Wenner, N. G. (2015). Induced systemic resistance responses in perennial ryegrass against Magnaporthe oryzae elicited by semi-purified surfactin lipopeptides and live cells of Bacillus amyloliquefaciens. Mol. Plant Pathol. 16, 546–558. doi: 10.1111/mpp.12209
Singh, R., Dangol, S., Chen, Y., Choi, J., Cho, Y. S., Lee, J. E., et al. (2016). Magnaporthe oryzae effector avr-pii helps to establish compatibility by inhibition of the rice NADP-malic enzyme resulting in disruption of oxidative burst and host innate immunity. Mol. Cells 39, 426–438. doi: 10.14348/molcells.2016.0094
Tjallingii, W. F. (2006). Salivary secretions by aphids interacting with proteins of phloem wound responses. J. Exp. Bot. 57, 739–745. doi: 10.1093/jxb/erj088
Valenzuela-Soto, J. H., Estrada-Hernández, M. G., Ibarra-Laclette, E., and Délano-Frier, J. P. (2010). Inoculation of tomato plants (Solanum lycopersicum) with growth-promoting Bacillus subtilis retards whitefly Bemisia tabaci development. Planta 231, 397–410. doi: 10.1007/s00425-009-1061-9
Van de Mortel, J. E., de Vos, R. C., Dekkers, E., Pineda, A., Guillod, L., Bouwmeester, K., et al. (2012). Metabolic and transcriptomic changes induced in Arabidopsis by the rhizobacterium Pseudomonas fluorescens SS101. Plant Physiol. 160, 2173–2188. doi: 10.1104/pp.112.207324
Van der Ent, S., Van Wees, S. C., and Pieterse, C. M. (2009). Jasmonate signaling in plant interactions with resistance-inducing beneficial microbes. Phytochemistry 70, 1581–1588. doi: 10.1016/j.phytochem.2009.06.009
Van Oosten, V. R., Bodenhausen, N., Reymond, P., Van Pelt, J. A., Van Loon, L. C., Dicke, M., et al. (2008). Differential effectiveness of microbially induced resistance against herbivorous insects in Arabidopsis. Mol. Plant Microbe Interact. 21, 919–930. doi: 10.1094/MPMI-21-7-0919
Van Wees, S. C., Van der Ent, S., and Pieterse, C. M. (2008). Plant immune responses triggered by beneficial microbes. Curr. Opin. Plant Biol. 11, 443–448. doi: 10.1016/j.pbi.2008.05.005
Zamioudis, C., and Pieterse, C. M. (2012). Modulation of host immunity by beneficial microbes. Mol. Plant Microbe Interact. 25, 139–150. doi: 10.1094/MPMI-06-11-0179
Zebelo, S., Song, Y., Kloepper, J. W., and Fadamiro, H. (2016). Rhizobacteria activates (+)-δ-cadinene synthase genes and induces systemic resistance in cotton against beet armyworm (Spodoptera exigua). Plant Cell Environ. 39, 935–943. doi: 10.1111/pce.12704
Zehnder, G., Kloepper, J., Tuzun, S., Yao, C., Wei, G., Chambliss, O., et al. (1997). Insect feeding on cucumber mediated by rhizobacteria-induced plant resistance. Entomol. Exp. Appl. 83, 81–85. doi: 10.1046/j.1570-7458.1997.00159.x
Zhang, J., Li, W., Xiang, T., Liu, Z., Laluk, K., Ding, X., et al. (2010). Receptor-like cytoplasmic kinases integrate signaling from multiple plant immune receptors and are targeted by a Pseudomonas syringae effector. Cell Host Microbe 7, 290–301. doi: 10.1016/j.chom.2010.03.007
Zhou, G., Qi, J., Ren, N., Cheng, J., Erb, M., Mao, B., et al. (2009). Silencing OsHI-LOX makes rice more susceptible to chewing herbivores, but enhances resistance to a phloem feeder. Plant J. 60, 638–648. doi: 10.1111/j.1365-313X.2009.03988.x
Zhu-Salzman, K., Salzman, R. A., Ahn, J. E., and Koiwa, H. (2004). Transcriptional regulation of sorghum defense determinants against a phloem-feeding aphid. Plant Physiol. 134, 420–431. doi: 10.1104/pp.103.028324
Keywords: Bacillus velezensis YC7010, aphid, Arabidopsis, induced systemic resistance, PAD4
Citation: Rashid MH, Khan A, Hossain MT and Chung YR (2017) Induction of Systemic Resistance against Aphids by Endophytic Bacillus velezensis YC7010 via Expressing PHYTOALEXIN DEFICIENT4 in Arabidopsis. Front. Plant Sci. 8:211. doi: 10.3389/fpls.2017.00211
Received: 29 October 2016; Accepted: 03 February 2017;
Published: 15 February 2017.
Edited by:
Kumar Krishnamurthy, Tamil Nadu Agricultural University, IndiaReviewed by:
William Underwood, USDA-ARS, USAZonghua Wang, Fujian Agriculture and Forestry University, China
Copyright © 2017 Rashid, Khan, Hossain and Chung. This is an open-access article distributed under the terms of the Creative Commons Attribution License (CC BY). The use, distribution or reproduction in other forums is permitted, provided the original author(s) or licensor are credited and that the original publication in this journal is cited, in accordance with accepted academic practice. No use, distribution or reproduction is permitted which does not comply with these terms.
*Correspondence: Young R. Chung, eXJjaHVuZ0BnbnUuYWMua3I=