- Department of Biology and Biochemistry, University of Bath, Bath, UK
S-acylation, also known as S-palmitoylation or palmitoylation, is a reversible post-translational lipid modification in which long chain fatty acid, usually the 16-carbon palmitate, covalently attaches to a cysteine residue(s) throughout the protein via a thioester bond. It is involved in an array of important biological processes during growth and development, reproduction and stress responses in plant. S-acylation is a ubiquitous mechanism in eukaryotes catalyzed by a family of enzymes called Protein S-Acyl Transferases (PATs). Since the discovery of the first PAT in yeast in 2002 research in S-acylation has accelerated in the mammalian system and followed by in plant. However, it is still a difficult field to study due to the large number of PATs and even larger number of putative S-acylated substrate proteins they modify in each genome. This is coupled with drawbacks in the techniques used to study S-acylation, leading to the slower progress in this field compared to protein phosphorylation, for example. In this review we will summarize the discoveries made so far based on knowledge learnt from the characterization of protein S-acyltransferases and the S-acylated proteins, the interaction mechanisms between PAT and its specific substrate protein(s) in yeast and mammals. Research in protein S-acylation and PATs in plants will also be covered although this area is currently less well studied in yeast and mammalian systems.
Introduction
Lipid modification is a common mechanism in organisms, in which a fatty acid attaches to specific amino acid residues, leading to increased hydrophobicity of proteins which aids their anchoring to membranes or specific lipid rafts (Levental et al., 2010). The three most commonly known lipid modifications are N-myristoylation, prenylation and S-acylation (Figure 1). N-myristoylation is an irreversible, co-translational protein modification in which 14-carbon myristoyl group is covalently attached to N-terminal glycine residue via an amide bond (Martin et al., 2011). Prenylation is a post-translational lipid modification which involves the transfer of either a 15-carbon farnesyl or a 20-carbon geranyl-geranyl moiety to CaaX C-terminal cysteine of the target protein. S-acylation, more commonly known as S-palmitoylation, is a post-translational lipid modification in which a long chain fatty acid, usually the 16-carbon palmitate, covalently attaches to the specific cysteine residue(s) throughout the protein via a thioester bond (Resh, 2006; Greaves and Chamberlain, 2011).
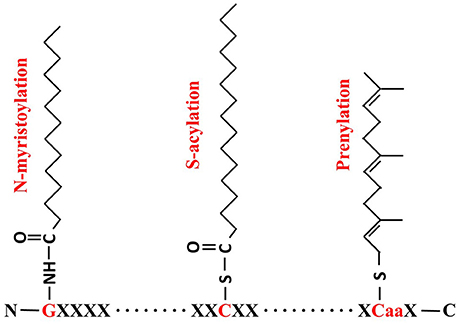
Figure 1. Formulae of N-myristoylation, S-acylation and prenylation. For N- myristoylation, a 14-carbon myristoyl group is covalently attached by an amide bond to the alpha-amino group of an N-terminal glycine (G, in red); S-acylation is the attachment of a 16-carbon palmitate to cysteine residue (C, in red) via thioester bond; and Prenylation makes a 15-carbon farnesyl link to the CaaX cysteine residue in C-termini.
It is noteworthy that three types of protein palmitoylation are found so far, including S-palmitoylation, N-palmitoylation and O-palmitoylation. While S-palmitoylation can occur at any Cys residues along the protein sequence in which the palmitate is reversibly attached via thioester bond as shown in Figure 1, N-palmitoylation is a stable lipid modification at the N-terminal residue (very often Cys) through amide linkage. A small group of secreted proteins have been identified as N-palmitoylated proteins, including the epidermal growth factor (EGF) like ligand “Spitz” and Hedgehog family members in Drosophila and mammals (Pepinsky et al., 1998; Chamoun et al., 2001; Miura et al., 2006; Buglino and Resh, 2012). Since N-palmitoylation can be easily converted by S-palmitoyl migration, it is still not very clear whether N-palmitoylation is an independent enzyme-catalyzed reaction or just from S- to N-palmitoyl transfer (Ji et al., 2016). Less frequently, palmitoyl group can also be linked to a serine residue through ester bond via the so-called O-palmitoyltion. The identified O-palmitoylated targets so far include Wnt/Wg proteins and the peptide hormone preghrelin (Takada et al., 2006; Yang et al., 2008). Although, palmitate is thought to be the most common fatty acid found to be attached to S-palmitoylated proteins recent studies proved that other acyl groups such as stearate (C18:0) or oleate (C18:1) are also accepted in S-palmitoylation. Therefore, S-acylation is a more representative term than palmitoylation (Jones et al., 1997; Sorek et al., 2007; Hurst and Hemsley, 2015). In contrast to other lipid modification, such as myristoylation, prenylation, N-palmitoylation or O-palmitoylation, S-acylation is a unique posttranslational modification in that it is usually reversible (Fukata and Fukata, 2010). As such it is important for cellular protein sorting, vesicle trafficking, activation state control, protein stability, membrane microdomain partitioning of protein and protein complex assembly (Greaves and Chamberlain, 2007; Baekkeskov and Kanaani, 2009; Charollais and Van Der Goot, 2009; Hemsley, 2009; Hemsley et al., 2013).
Some other lipid modifications, such as Glycosylphosphatidylinositol (GPI) and glycosylinositolphosphorylceramide (GIPC) anchors can link the whole glycolipids to the protein instead of the simple fatty acid or polyisoprene group (Hemsley, 2015). GPI and GIPC anchors modify proteins at the lumen side instead of in the cytosol as do the other three lipid modifications (Ganesan and Levental, 2015). Lipid modifications which are only found in specific proteins, such as cholesterol addition at the C-terminal glycine of proteins have also been reported (Buglino and Resh, 2012). All these lipid modifications are widely present in mammals and plants (except for N- or O- palmitoylation which is only found in mammals so far) and all play important roles during growth and development through the modification of an array of proteins.
Although all lipid modifications can facilitate the attachment of proteins to membranes, modification with palmitoyl groups provide more affinity, about 10 times stronger than myristoyl groups and 100 times than farnesyl groups (Silvius and L'heureux, 1994; Hemsley, 2009).
S-acylation
S-acylation can occur both on soluble and transmembrane proteins (Roth et al., 2006; Blaskovic et al., 2013). S-acylation of soluble proteins allows their association with membranes, trafficking, regulation and signaling (Roth et al., 2006; Blaskovic et al., 2013). For example, a constitutive de/re-acylation of H- and N- small Rat sarcoma (Ras) drives their subcellular localization from plasma membrane (PM) to Golgi which initiates RAS activation (Rocks et al., 2005). Although, the direct mechanism of S-acylation on transmembrane proteins is not very clear it is thought that it plays multiple roles in altering signaling capacity (Merrick et al., 2011), reducing activity (Huang et al., 2010), trafficking modification (Abrami et al., 2008; Flannery et al., 2010) and changing stability of these proteins (Abrami et al., 2006; Maeda et al., 2010; Blaskovic et al., 2013). For example, S-acylation of transmembrane proteins, such as death receptor 4 (Oh et al., 2012), β-secretase BACE1 (Motoki et al., 2012), cannabinoid receptor (Oddi et al., 2012) and influenza virus M2 protein (Thaa et al., 2011), can promote their association with membrane lipid rafts. However, for some peripheral membrane proteins such as transferrin receptor and caveolin, their palmitoylation sites are localized to non-raft domains, therefore palmitoylation is not necessary for their raft localization (Alvarez et al., 1990; Dietzen et al., 1995; Charollais and Van Der Goot, 2009). In the case of the tumor endothelial marker 8 (TEM8) palmitoylation was actually found to negatively regulate its raft association (Abrami et al., 2006).
S-acylation in Yeast
A proteomic method using the acyl-biotinyl exchange (ABE) chemistry combining with the traditional [3H] palmitate in vivo labeling protocol identified 48 S-acylated proteins that span a wide range of cellular functions in Saccharomyces cerevisiae (Roth et al., 2006). These include a large number of SNAREs (soluble N-ethylmaleimide-sensitive fusion protein-attachment protein receptor) that are involved in vesicle fusion. Redundant SNAREs, such as plasma membrane (PM) localized synaptobrevin homologs Snc1 and Snc2, were first identified to be S-acylated proteins in 1995 (Couve et al., 1995), and subsequently confirmed independently (Valdez-Taubas and Pelham, 2005; Roth et al., 2006). Ykt6 is another commonly known S-acylated SNARE. It requires both C-terminal prenylation and palmitoylation to target to the membrane, which is different from all other single transmembrane domain (TMD) containing SNAREs (Fukasawa et al., 2004). Tlg1 lacking S-acylation undergoes ubiquitination, implying S-acylation can protect proteins from degradation (Valdez-Taubas and Pelham, 2005). Other SNAREs that have been confirmed to be S-acylated are Sso1, Sso2, Vam3, Tlg2, and Syn8 (Valdez-Taubas and Pelham, 2005; Roth et al., 2006). S-acylation is also very common in many important signaling proteins, such as the heterotrimeric G protein alpha and gamma subunits Gpa1 (Song and Dohlman, 1996; Song et al., 1996), Gpa2 (Harashima and Heitman, 2005), and Gγ (Ste18, Hirschman and Jenness, 1999); small monomeric G proteins (GTPases) such as Rho1, Rho2 (Roth et al., 2006), Rho3 (Zhang et al., 2013), Ras1 and Ras2 (Deschenes et al., 1990; Mitchell et al., 1994; Bartels et al., 1999). A recent study shows that the pathogenesis, morphogenesis and sexual differentiation of an encapsulated yeast Cryptococcus neoformans is achieved through the important roles that S-acylation plays in modulating the localization of Ras1 (Nichols et al., 2015). Interestingly, all of these signaling proteins acquire prenylation or myristoylation before S-acylation occurs (Roth et al., 2006).
In addition, many amino acid permeases (AAP) were proved to be S-acylated (Roth et al., 2006). For example, the yeast type I casein kinases, Yck1, Yck2, and Yck3, which play important roles in cellular morphology, bud emergence and endocytosis of mating pheromone receptor, are membrane localized via S-acylation for function (Roth et al., 2006, 2011). ENV7 (late endosome and vacuole interface) encodes a protein kinase that plays important roles in vacuole morphology, and its proper membrane localization and function relies on S-acylation of the N-terminal triple cysteines motif (C13C14C15) (Manandhar et al., 2013, 2014; Cocca, 2014). S-acylation of telomere-binding protein Rif1 anchored it to the inner nuclear membrane, which influences its role in heterochromatin dynamics (Park et al., 2011). Mutagenesis of cysteine in different positions of Arsenite permease Acr3p can cause its completely or partially dysfunction as a low affinity As(III)/H+ and Sb(III)/H+ antiporter, and Cys90 which localizes in the cytosolic loop but in close proximity to transmembrane regions has the high possibility to be S-acylated (Maciaszczyk-Dziubinska et al., 2014). It was also reported that S-acylation is necessary for the export of chitin synthase Chs3 from ER (Lam et al., 2006). The information described in this section is summarized in Table 1.
S-acylation in Mammals
Following study of S-acylation in yeast research that has extended to mammalian systems considerable knowledge has been gained in recent years, revealing the involvement of protein S-acylation in the regulation of growth, development, and cancer and disease status. For example, a global rat neural palmitoyl-proteome characterized almost 300 S-acylated proteins, again with the ABE method adapted from the yeast study (Kang et al., 2008). Similarly 331 S-acylated proteins were identified from human prostate cancer cells (Yang et al., 2010), 57 from human B lymphoid cells (Ivaldi et al., 2012) and 150 from endothelial cells (Marin et al., 2012). By bio-orthogonal labeling of S-acylated proteins with 17-octadecynoic acid (ODYA) about 125 and over 400 S-acylated proteins were identified from human Jurkat T-cells and mouse T-cell hybridoma cells, respectively (Martin and Cravatt, 2009; Martin et al., 2012).
It is worth noting that proteins that have been proved to be S-acylated in yeast, their homologous proteins in mammals tend to be also S-acylated. For instance, many human SNAREs were proved to be also S-acylated (Greaves et al., 2010), S-acylation of α subunits of G proteins is necessary for their membrane localization and function (Wedegaertner et al., 1993; Grassie et al., 1994; Ponimaskin et al., 1998, 2000). However, G-protein γ subunits have not been reported to be S-acylated in mammals. Many G-protein-coupled receptors (GPCRs) (Blaskovic et al., 2013) and Ras GTPase (Rocks et al., 2005) are also S-acylated. Mitochondrial targeting of a microphage protein phospholipid scramblase 3 (Plscr3) is dependent on its S-acylation (Merrick et al., 2011).
Some S-acylated proteins in mammals can also make themselves avoid degradation by attaching a palmitate molecule. For instance, LRP6 (lipoprotein-receptor-related protein 6) is S-acylated and the removal of acyl group leads to destabilization or ubiquitination (Abrami et al., 2008). Similarly, the palmitoylation of TEM8 (Abrami et al., 2006), CCR5 (chemokine and HIV receptor) (Percherancier et al., 2001) and Rhodopsins (Maeda et al., 2010) prevents the degradation of these proteins. It was also reported that for some other proteins, their degradation depends on the S-acylation. For example, a cancer-promoting protein CDCP1 (CUB domain-containing protein 1) is degraded upon S-acylation, leading to a decrease of ovarian cancer cell migration (Adams et al., 2015). Therefore, it seems that S-acylation can play opposite roles in protein degradation.
Many signaling proteins involved in keeping T-cell homeostasis are S-acylated, such as T-cell co-receptors CD4 and CD8, tyrosine kinases Lck and Fyn, and adaptor proteins LAT (linker for activation of T cells) and Cbp/PAG (Bijlmakers, 2009; Hundt et al., 2009; Akimzhanov and Boehning, 2015). S-acylation of Lck at both Cys3 and Cys5, which are redundant for the function of Lck, is essential for propagating T-cell receptor signaling and releasing apoptotic calcium (Akimzhanov and Boehning, 2015). Similarly, LAT is also a dual (Cys26 and Cys29) S-acylated protein which is required for T cell development and activation. However, S-acylation of Cys26 alone is enough for its PM localization and proper function (Hundt et al., 2009).
S-acylation of synaptic proteins is important for synaptic plasticity, and the key S-acylated synaptic proteins include postsynaptic density protein PSD-95, δ-catenin, gephyrin, A-kinase anchoring protein AKAP79 and 150, the small GTPase Cdc42. Lack of S-acylation of these proteins lead to impaired performance on learning and memory tasks (Brigidi et al., 2015). Huntington's disease is a neurodegenerative disorder caused by mutation in the gene encoding the S-acylated Huntingtin (HTT) (Butland et al., 2014). Defects in S-acylation can also cause mental problems such as schizophrenia and X-linked mental retardation (XLMR), however, the specific S-acylated target proteins involved in this process have not been isolated (Mukai et al., 2004; Raymond et al., 2007). Alzheimer's disease (AD) is a neurodegenerative dementia which accounts for 60–70% of cases of dementia. Many studies have demonstrated that S-acylation plays very important roles in the pathogenesis of AD, and the related S-acylated proteins include β- and γ-secretase enzymes, and the major APP (amyloid precursor protein) cleaving enzyme BACE1, which are S-acylated at four sites (Benjannet et al., 2001; Hornemann, 2015).
Autophagic protein microtubule-associated protein 1 light chain-3B (LC3B) is a positive regulator of chronic obstructive pulmonary diseases such as emphysema. LC3B is associated with the extrinsic apoptotic factor Fas, and their interaction is mediated by caveolin-1 (Cav-1). Interestingly, both Fas and Cav-1 are S-acylated proteins (Chen et al., 2010). S-acylation of the bone developmental regulator membrane type1-metalloprotease (MT1-MMP) is a key modulator of bone homeostasis (Song et al., 2014). Goltz syndrome, caused by loss of function of the S-acylated protein Porcupine (Galli et al., 2007; Hornemann, 2015), is an X-linked dominant form of ectodermal dysplasia, which is primarily characterized by skin manifestations as atrophic and hypoplastic areas and results in osseous defects and dental anomalies later (Wang et al., 2007).
An increasing number of reports indicate that S-acylation is involved in cancer. For instance, Ras is a negative regulator of cell proliferation, and S-acylation of Ras maintains its steady state plasma membrane localization which is essential for transduction of extracellular proliferative signals (Rocks et al., 2005; Schmick et al., 2015). S-acylation of the neurotensin receptor 1 (NTSR-1), a key mediator in breast, pancreas, prostate, colon and lung cancers, is essential for its localization and efficient signaling (Heakal et al., 2011). The induction of apoptosis is an efficient way to stop tumor development, many proteins involved in apoptosis are S-acylated including FasL (Fas Ligand; Guardiola-Serrano et al., 2010), FasR (Fas receptor; Chakrabandhu et al., 2007), DR4 (a receptor of the tumor necrosis factor-related apoptosis-inducing ligand; Rossin et al., 2009), DCC (deleted in colorectal cancer; Furne et al., 2006), UNC5H (Maisse et al., 2008) and BAX (BCL-2-associated X) (Fröhlich et al., 2014). The spread of cancer cells from their original site to other parts of the body is through metastasis. It was reported that S-acylation of metastasis-associated proteins KAT1/CD82, CD9, and CD151 is essential for their function of suppressing metastasis or inhibiting tumor cell adhesion and migration (Zhou et al., 2004; Hemler, 2014; Termini et al., 2014). Integrin β4 (ITGβ4) can interact with growth factor receptors and enhance invasive potential of cancer cells (Soung and Chung, 2011). This is helped by the S-acylation of ITGβ4 which is required for its lipid raft localization in the membrane and signaling activity. The level of ITGβ4 S-acylation is correlated with the invasive potential of breast cancer cells (Coleman et al., 2015). Another S-acylated protein related to breast cancer is CD44 which negatively regulates cell migration (Xie et al., 2009). Endothelial nitric oxide synthase (eNOS), which localizes through S-acylation to the Golgi complex and PM cholesterol-rich microdomains, promotes angiogenesis and tumorigenesis (Fernández-Hernando et al., 2006; Wei et al., 2011). Table 2 lists the identified S-acylated proteins in mammals described in this section.
The above studies clearly demonstrate that S-acylation is involved in a wide range of human diseases including mal-development, infectious diseases, autoimmune diseases, neuropsychiatric disorders, dermatosis, osteoporosis, and cancer (Ivaldi et al., 2012; Chavda et al., 2014; Hornemann, 2015; Yeste-Velasco et al., 2015). Understanding S-acylation will provide invaluable information to the insight of disease processes which in turn will aid the development of drugs to control and target these various diseases. Therefore, the relationship between protein S-acylation and disease in human becomes a hot research topic in the medical field in recent years.
S-acylation in Plants
Our understanding of plant S-acylation is rudimentary and the limited knowledge comes mainly from targeted studies on the functional characterization of individual proteins that happen to be S-acylated, including, mainly heterotrimeric G protein and some small monomeric G-proteins. For instance, the α subunit GPA1 and γ subunit AGG2 of plant heterotrimeric G protein are S-acylated. GPA1 has dual lipid modification with a myristoylation site at the G2 position and an adjacent S-acylation site at the C5 position, ensuring its localization to the PM (Adjobo-Hermans et al., 2006). Apart from promoting PM localization, S-acylation of GPA1 may also stabilize the newly formed heterotrimer. AGG2 is S-acylated at Golgi before delivered to the PM, and its membrane localization is dependent on its prenylation and S-acylation (Zeng et al., 2007). Therefore, S-acylation may act as a membrane targeting signal and restricts AGG2 shuttle in and out of PM (Zeng et al., 2007; Hemsley, 2009). Some small GTPases are also known to be S-acylated. For instance, S-acylation of AtROP6 is responsible for its activation and inactivation cycles (Sorek et al., 2007). AtROP9 and AtROP10, which are involved in ABA signaling, contain 3 and 2 S-acylation sites respectively (Lavy et al., 2002; Zheng et al., 2002; Hemsley, 2009). For AtRABF1 (ARA6), both S-acylation and myristoylation are essential for its prevacuolar compartment localization (Ueda et al., 2001).
Proteins involved in Ca2+ signaling such as calcineurin B-Like proteins AtCBL1, AtCBL2, AtCBL3, and AtCBL6 in Arabidopsis (Batistic et al., 2008, 2012); calcium dependent protein kinases OsCPK2 in rice (Martin and Busconi, 2000); LeCPK1 in tomato (Leclercq et al., 2005); MtCPK3 in Medicago truncatula (Gargantini et al., 2006) and StCDPK1 in Solanum tuberosum (Raíces et al., 2003) were reported to be S-acylated. AtCBL1 is a dually lipid modified protein, in which myristoylation targets it to the endoplasmic reticulum (ER), but the trafficking from ER to PM and subsequent PM anchoring depends on S-acylation (Batistic et al., 2008).
Other S-acylated proteins are the pathogenesis related proteins such as RPM1 interacting protein 4 (RIN4) and leucine-rich repeat receptor like kinase (FLS2) (Kim et al., 2005; Hemsley et al., 2013; Running, 2014; Boyle et al., 2016); NDR1/HIN1-like (NHL) stress response proteins (Hemsley et al., 2013; Hurst and Hemsley, 2015); POLTERGEIST (POL) and POLTERGEIST LIKE 1 (PLL1) (their PM localization is dependent on both myristoylation and S-acylation at their N-termini) (Gagne and Clark, 2010); the Lost In Pollen tube guidance 1 (LIP1) and 2 (LIP2), mutations of their S-acylation sites abolished PM localization. Although, individual knockout mutant of LIP1 and LIP2 did not have any defects the double mutant can cause sterility due to loss of pollen tube guidance (Liu et al., 2013). S-acylation of remorin proteins, a group of well-known plasma membrane marker proteins, contribute to their subcellular localization (Konrad et al., 2014). Very recently, Kumar and his coworkers confirmed that a number of the catalytic subunits of cellulose synthase complex (CSC) in Arabidopsis are S-acylated. These include the cellulose synthase A 1 (CESA1), CESA4, CESA6, CESA7, and CESA8 where up to 6 S-acylation sites were in each of these proteins (Kumar et al., 2016). SGN1, a receptor-like cytoplasmic kinase (RLCK), localizes in a strictly polar fashion to the endodermal outer plasma membrane, and this is dependent on the S-acylation of N-termini (Alassimone et al., 2016; See summary in Table 3).
On a proteomic level Hemsley and coworkers identified about 600 putative S-acylated proteins from Arabidopsis using a biotin switch isobaric tagging for relative and absolute quantification (Hemsley et al., 2013). These proteins are involved in many processes across plant growth, development and stress responses, including the mitogen-activated protein kinases (MAPKs), leucine-rich repeat receptor-like kinases (LRR-RLKs) and RLK superfamily members, integral membrane transporters, ATPases, SNAREs and others. Similarly, about 450 S-acylated proteins were identified from Poplar cell suspension very recently. Except for the commonly known intracellular trafficking related proteins such as protein kinases, SNAREs, band 7 family proteins and tetraspanins, some cell wall related proteins were also found to be S-acylated (Srivastava et al., 2016). These results greatly expand the range of functions of protein S-acylation involves in plants, demonstrating the important roles of protein S-acylation in plant growth, development and stress signaling.
S-acylation in Other Organisms
S-acylated proteins were also identified from other organisms. For example, more than 400 putative S-acylated proteins were isolated from the most severe human malaria causing parasite Plasmodium falciparum, involved in almost all the stages of its life cycle (Hodson et al., 2015). A number of S-acylated proteins are localized in the inner membrane complex (IMC). IMC is a membranous two layered structure located underneath the plasma membrane, which IMC plays central roles in host cell invasion and cytokinesis in P. falciparum (Cavalier-Smith, 1993; Wetzel et al., 2015). In another parasite Toxoplasma gondii, S-acylated proteins were also proved to be involved in many physiological processes including motility, invasion and division (Beck et al., 2010; Frénal et al., 2010, 2014). In Aspergillus fumigatus, one of the most common species that cause the invasive aspergillosis in individuals with an immunodeficiency, Ras pathway signaling is its critical virulence determinant and the properly localized and activated Ras is dependent on a series of posttranslational lipid modification including S-acylation (Al Abdallah and Fortwendel, 2015). Study also showed that S-acylation is essential for spermatogenesis of Caenorhabditis elegans (Gleason et al., 2006).
S-acylation not only occurs on proteins synthesized in eukaryotic cells but also for proteins secreted by prokaryotic bacteria and viruses and subsequently S-acylated by their eukaryotic hosts. For example, Legionella and other bacterial pathogens can secrete effectors that mimic the substrates of host lipid transferases, which can help them target the proper host membranes after S-acylation and other lipid modifications (Ivanov and Roy, 2013). A group of cysteine protease type III effectors secreted by the plant pathogen Pseudomonas syringae, rely on their S-acylation by the host cells to be targeted to plasma membrane and activated (Dowen et al., 2009). S-acylation can also take place on viral proteins and in fact the first reported S-acylated protein was a glycoprotein from Vesicular stomatitis virus (Schmidt and Schlesinger, 1979; Hurst and Hemsley, 2015). Another viral S-acylated protein is the hemagglutinin of Influenza virus. S-acylation of its all three cysteine residues by the host cell S-acylation machinery is essential for the replication and infection of the virus (Zurcher et al., 1994; Wagner et al., 2005; Brett et al., 2014).
Protein S-Acyl Transferases (PATs)
While spontaneous palmitoylation does occur on some proteins in the cells (i.e., Bizzozero et al., 2001; Kümmel et al., 2006; Kostiuk et al., 2008) it is generally accepted that S-acylation is an enzymatic process catalyzed by a family of proteins, the Protein S-Acyl Transferases (PATs for short). This is because research on PATs was much delayed compared to that on the S-acylated proteins. The first PAT, Akr1 was identified from S. cerevisiae in 2002 which is 20 years later than S-acylation of protein first reported (Schmidt and Schlesinger, 1979; Bartels et al., 1999; Roth et al., 2002). Since then, the significance of this enzyme family has been gradually recognized by studies carried out by an increasing number of researchers in this field, leading to the great enrichment of our knowledge of PATs, particularly in yeast and mammals.
The Structure and Functional Domains of PATs
Compared to the numbers of enzymes that catalyze the N-myristoylation or prenylation, there are much more DHHC-containing PATs existing in eukaryotes. In contrast to the cytoplasmic catalyzing enzymes for S-prenylation and N-myristoylation, PATs are transmembrane proteins with 4-6 TMDs and cytosolic N- and C- terminii (Hemsley et al., 2013). Most importantly, PATs also have a highly conserved catalytic Asp-His-His-Cys Cysteine Rich Domain (DHHC-CRD) of ~50 amino acids (Roth et al., 2002). This domain was proposed as Cx2Cx9HCx2Cx4DHHCx5Cx4Nx3F (Mitchell et al., 2006), usually residing on the cytoplasmic face of membranes between transmembrane domains (TMD) 2 and 3 of PATs (Gottlieb et al., 2015). It was reported that mutation of cysteine in DHHC domain inhibits both acyl intermediate formation and acyl chain transfer activity of PATs (Mitchell et al., 2006; Gottlieb et al., 2015). Indeed, when cysteine residue in the DHHC motif of AtPAT24, AtPAT10 and AtPAT14 of Arabidopsis was mutated to alanine or serine, all 3 AtPATs lost their PAT activities (Hemsley et al., 2005; Qi et al., 2013; Li et al., 2016). The DHHC-CRD domain in Swf1 cannot be replaced by those from Pfa3, Pfa4 or Erf2, and similar results were also found for Pfa3, the DHHC-CRD of which cannot be replaced by that of Swf1 or Erf2. The irreplaceability of DHHC-CRD demonstrates interaction between this domain and other regions is required for proper PAT function (Montoro et al., 2011). Although, the acyl intermediate happened on the cysteine residue in the DHHC motif study on human DHHC3 showed that mutation of other conserved cysteines in the CRD also decreased its activity (Gottlieb et al., 2015). In addition, cysteine residues within a novel CCX7−−13C(S/T) motif downstream of the conserved DHHC-CRD of human PATs DHHC5, DHHC6 and DHHC8 were also proved to be S-acylated (Yang et al., 2010). Therefore, it seems that cysteine residues in the DHHC-CRD as well as other motifs play joint roles in of PATs auto-acylation and subsequent transfer of the fatty acid to their substrate proteins. It is also interesting to note that many residues in the DHHC-CRD domain are reported to determine substrate specificity of a PAT, such as A145 and K148 in Swf1 (Montoro et al., 2011).
Some PATs also have an N-terminal ankyin repeat (AR) domain. Usually two AR containing DHHC PATs are found in per genome, such as in mammals (Fukata et al., 2004), yeast (Roth et al., 2006), fly (Bannan et al., 2008), apicomplexan parasite (Frénal et al., 2013), nematode (Edmonds and Morgan, 2014), and plants (Yuan et al., 2013). It is thought that AR can help these PAT to recognize its specific targets for S-acylation (Lemonidis et al., 2015). However, other functions of AR that are independent from S-acylation were also found in some such PATs (Harada et al., 2003; Hemsley and Grierson, 2011; Yang and Cynader, 2011). For example, AR is essential for Akr1 to interact with Gβγ dimer and to suppress cell cycle arrest induced by β subunit, and this process does not require Akr1 being a functional PAT (Hemsley and Grierson, 2011).
Both the N- and C-termini of all PATs characterized so far are highly variable and cytosolic. The highly variability of N- and C- terminal domains are believed to be essential for substrate specificity of PATs, even though there have no experimental evidence to support this at present (Huang et al., 2009; Greaves et al., 2010; Montoro et al., 2011). Many PATs also have a conserved aspartate-proline-glycine (DPG) motif close to the second TMD, and a threonine-threonine-asparagine-glutamate (TTxE) motif adjacent to the last TMD. Both of them are cytosolic but their roles in the function of PATs are still waiting to be explored. Another important region that contains 16-amino acids and is conserved in 70% eukaryotic PATs is the PaCCT motif (Palmitoyltransferase Conserved C-Terminus). Absence of the PaCCT motif abolished the function of Pfa3 in yeast; the tyrosine residue within this motif of Swf1 is essential for its PAT activity toward Tlg1 (González et al., 2009).
The general structure and the functional/conserved domains of PATs is illustrated in Figure 2.
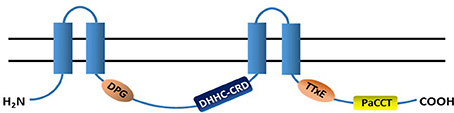
Figure 2. Topology structure and conserved domains of PATs. Most PATs have 4 transmembrane domains (TMDs, blue columns) and their N- and C-termini are in the cytoplasm. A highly conserved catalytic DHHC-CRD (aspartate-histidine-histidine-cysteine cysteine rich domain) resides between the 2nd- and 3rd-TMDs. The majority of PATs also have the DPG (aspartate-proline-glycine), TTxE (threonine-threonine-any amino acid-glutamic acid) and PaCCT (Palmitoyltransferase Conserved C-Terminus) domains, and all of them are cytosolic.
DHHC Proteins are Commonly Found in Eukaryotes
Since the first DHHC containing protein, Akr1 was found and proved to be a PAT from yeast in 2002, significant advances have been made in understanding of DHHC protein family in yeast, mammals, worm and plants. So far, 6 of the 7 yeast DHHC proteins have been confirmed to be PATs, and they are Akr1 (Lobo et al., 2002; Roth et al., 2002), Erf2 (Valdez-Taubas and Pelham, 2005), Swf1 (Smotrys et al., 2005), Pfa3 (Hou et al., 2009), Pfa4 (Smotrys et al., 2005), and Pfa5 (Roth et al., 2006). Akr2 is highly homologous to Akr1 with a typical DHHC-CRD and two ARs, however, akr2 mutant did not show any remarkable phenotype and there is no direct evidence to show whether it has PAT catalytic activity or not (Kihara et al., 2005; Linder and Deschenes, 2007).
Among the 22 human DHHC proteins (DHHC1-22), 17 were proved to have PAT activities excluding DHHC4, 11, 13, 19, and 22 (Ohno et al., 2012). One more DHHC protein (DHHC23) was found in mice but its homolog cannot be found in human (Ohno et al., 2006; Greaves and Chamberlain, 2010). Caenorhabditis elegans has 15 DHHC-PATs, but so far only 1, SPE10 (spermatogenesis 10), was characterized in some detail and showed that it is essential for membranous organelles to deliver fibrous bodies to the spermatid (Gleason et al., 2006).
Plant genomes also possess various numbers of DHHC containing protein sequences. A recent survey from 31 plant species with complete genomes including Arabidopsis, identified 804 DHHC proteins. The numbers of DHHC proteins were variable in different species from 6 in Volvox carteri to 52 in Panicum virgatum. Expression pattern of DHHC proteins in Zea mays and their response to phytohormones and abiotic stress showed that these DHHC proteins may play important roles in plant growth and development as well as stress responses (Yuan et al., 2013). Arabidopsis has 24 DHHC-containing proteins, named as ATPAT1-24 (Hemsley et al., 2005; Batistič, 2012). According to their phylogenetic relationship they are divided into 3 main groups, where group A has the most members including AtPATs 1–9, group B consists AtPATs 11–16, group C is made of AtPATs 18–22, whilst AtPAT10, 17, 23, and 24 do not belong to any groups (Batistič, 2012). All these putative PATs have 4 TMDs except for AtPAT15 and AtPAT17 where 3 and 6 TMDs are found respectively.
Similar to what was found in yeast and mammals, Arabidopsis genome also has 2 ankyrin repeats containing PATs, AtPAT23 and AtPAT24 that are highly homologous. Being the first PAT identified in higher plant, AtPAT24 was confirmed to be an S-acyl transferase because it not only auto-acylated but also rescued the yeast PAT Akr1 knockout mutant akr1 for its morphological and temperature sensitive defects. AtPAT24 can also restore the correct localization of one of the Akr1 palmitoylating proteins, the yeast casein kinase 2 (Yck2). The AtPAT24 loss-of-function mutant tip1 exhibites defects in cell size control, pollen tube and root hair growth, as well as cell polarity (Hemsley et al., 2005). Following this, the biological functions of 3 other AtPATs have also been characterized in some detail recently. For example, three T-DNA insertion mutant alleles were identified for AtPAT10 and all showed pleiotropic defects, including cell expansion, cell division, vascular patterning, fertility and salt stress in Arabidopsis (Qi et al., 2013; Zhou et al., 2013). Single mutants of atpat13 and atpat14 are semi-dwarf and show precocious leaf senescence, and the double mutant atpat13 atpat14 has even stronger phenotype than each of their parent single mutant plants (Lai et al., 2015; Li et al., 2016).
DHHC containing proteins were also identified from other organisms. For example, 22 such proteins were found in Drosophila (Bannan et al., 2008), 18 in T. gondii, 17 in Neospora caninum, 12 in Trypanosome brucei, 12 in P. falciparum, 11 in Plasmodium bergbei, 10 in Cryptosporidium species, 9 in Theileria parva, 8 in Babesia bovis and 6 in Eimeria tenella (Frénal et al., 2013). It was reported that TgDHHC7 from T. gondii is essential for rhoptry organelles localization and parasite invasion (Frénal et al., 2013).
Expression Pattern and Subcellular Localization of PATs
The spatial and temporal expression patterns of genes are very important for their cellular functions. However, only very limited information available for the expression patterns of PAT family proteins so far. It was shown that the humans DHHC1, 3–10, 12–14, 16–18, and 20–22, are ubiquitously expressed in different tissues. DHHC19 had very high expression level in testis with weak expression in thymus and small intestine while DHHC11 was only expressed in testis. In addition, only very low level of DHHC2 transcript was present in kidney and testis, and similar low levels of DHHC15 transcript were found in heart, brain, lung, kidney, thymus, and small intestine (Ohno et al., 2006).
The expression patterns of DHHC proteins in Drosophila were also analyzed. Among them, CG1407, CG5620, CG6017, CG6627, and CG17257 exhibited maternal expression and were enriched in neural tissues, with transcripts of all of them except for CG1407 also detected in larval brains. Some DHHC proteins were only expressed in testis, such as CG4483, CG4956, CG13029, CG17075, CG17195-17198, CG17287, and CG18810, and others expressed only in ovary, such as CG5880, CG6017, and CG34449 (Bannan et al., 2008).
Study of the expression patterns of PATs in parasite also showed that most of them have ubiquitous expression with a few being more tissue or developmental stage specific. For instance, TgDHHC18 is specially expressed in bradyzoites, TgDHHC10 at oocyst stage, PfDHHC6 and PfDHHC10 at gametocyte stage (López-Barragán et al., 2011; Frénal et al., 2013).
In Arabidopsis, 19 of the 24 AtPATs expressed in a broad and constant pattern with transcripts detected in most tissues at all different developmental stages in Arabidopsis. However, AtPAT1, 2, 3, 11, and 21 had relatively low expression levels than other AtPATs, and AtPAT2 and 3 also exhibited stronger expression in pollen (Batistič, 2012; Yuan et al., 2013). In Oryza sative, 26 of the 30 OsPATs can express in more than one type of tissue, among which OsPAT29 was only expressed during germination stage, OsPAT21 and OsPAT26 were only expressed in the internode and stamen respectively. The transcripts of OsPAT13 and OsPAT28 were barely detectable in the tissues examined (Yuan et al., 2013). In Zea mays 28 of 38 ZmPATs have extensive expression in different developmental stages and tissues with ZmPAT13 and ZmPAT22 having higher expression in anther (Yuan et al., 2013). However, in Glycine max, specific, rather than broad expression patterns were found where 7 GmPATs were specifically expressed in the flowering stage and the transcripts of the remaining 11 GmPATs were detected in all developmental stages except for flowering stage (Yuan et al., 2013).
Therefore, it is clear that most PATs exhibit a broad expression pattern in different developmental stages and tissues with a few stage or tissue specific PATs in all organisms reported. This suggests that most PATs are involved in a broad range of functions in a given organism, such as Arabidopsis.
PATs distributed in the entire endomembrane system in the cell and this locality nature of PATs may determine the specific set of proteins they modify. For example, the plasma membrane-localized Pfa5 in yeast and DHHC5, 20 and 21 in human are the PATs involved in S-acylation mediated signal transduction of PM localized heterotrimeric G protein alpha subunit Gsα, the β2-adrenergic receptor and endothelial nitric oxide synthase (Mumby et al., 1994; Robinson et al., 1995; Loisel et al., 1996; Ohno et al., 2006). The ER- and Golgi-localized DHHC proteins may be responsible for palmitoylation of de novo synthesized proteins during the processes of membrane localization and delivery to other organelles (Ohno et al., 2006). The tonoplast-localized Pfa3 in yeast palmitoylates Vac8p for its vacuolar membrane targeting (Hou et al., 2005; Smotrys et al., 2005). Therefore, to determine the subcellular localization of individual PAT is very important in order to understand its function by identifying the substrate protein(s) it modifies and signaling pathways it is involved.
Although, PATs are found in all endomembrane systems in the eukaryotic cell they have different preference in different species as to which endomembrane compartment they are localized. For instance, in yeast, 3 PATs, Swf1, Pfa4, and Erf2 are localized at ER; Akr1 and Akr2 are localized at Golgi; while Pfa3 and Pfa5 are localized at vacuole and plasma membrane respectively (Valdez-Taubas and Pelham, 2005; Ohno et al., 2006). In human, 8 PATs are localized at ER (DHHC1, 6, 10, 11, 13, 14, 16, and 19), 7 at Golgi (DHHC3, 4, 7, 8, 15, 17, and 18), 2 at PM (DHHC5 and 20), 4 at both ER and Golgi (DHHC2, 9, 12, and 22), and 1 at both Golgi and PM (DHHC21) (Ohno et al., 2006).
Similar to the mammalian PATs, DHHC-PATs in Drosophila are also mainly localized at ER (14: CG4483, CG4676, CG4956, CG5196, CG5620, CG6627, CG10344, CG13029, CG17075, CG17195, CG17196, CG17197, CG17198, and CG17287) and Golgi (6: CG5880, CG6017, CG6618, CG8314, CG17257, and CG18810). The only exception is CG1407 which is localized at PM (Bannan et al., 2008). In Apicomplexan, such as T. gondii, TgDHHCs are not only localized on the common organelles such as Golgi (TgDHHC1, 5, 6, 9, 11, 12, 15, and 17), ER (TgDHHC3, 8, and 16), PM (TgDHHC4 and 13), but also the Apicomplexan-specific organelles such as IMC (TgDHHC2 and 14) and rhoptries (TgDHHC7) (Frénal et al., 2013).
In some contrast to the subcellular localization described above, studies carried out on transiently expressed in tobacco leaves of the 24 Arabidopsis PATs show that 9 of them are localized on PM, including AtPAT04-09, 12, 19, and 21. Therefore, it was proposed that PM is the main site for S-acylation in Arabidopsis plant (Batistič, 2012). This is different from mammalian PATs where most of them are localized in Golgi and therefore Golgi is thought to be the major S-acylation machinery (Ohno et al., 2006; Batistič, 2012). It is also interesting to note that many AtPATs that are localized on ER and PM are also associated with vesicles around them. For example, AtPAT3, 15, 17, and 18 are localized at ER as well as on the vesicles associated with them; AtPAT13, 20, and 22 at PM and also vesicles (Batistič, 2012). AtPAT10, 14, 16, 23, and 24 are mainly localized at Golgi, and the Golgi-localization of AtPAT10 and AtPAT14 were further confirmed in stably transformed Arabidopsis plants (Qi et al., 2013; Li et al., 2016). While the main residence of AtPAT01 and AtPAT02 are the endosomal compartments AtPAT10 and AtPAT11 were found on the tonoplast (Batistič, 2012; Qi et al., 2013; Zhou et al., 2013). It is noteworthy that a few Arabidopsis PATs have dual subcellular localizations, such as AtPAT10 (Golgi and tonoplast) and AtPAT13/20/22 (PM and vesicles) (Batistič, 2012; Qi et al., 2013). Similar observations were also made with some mammalian PATs (Valdez-Taubas and Pelham, 2005; Ohno et al., 2006). However, the significance of this dual-localization nature of PATs is currently unknown.
Little is known about how the PAT proteins achieve their respective localization in the cell. A recent study show that the lysine-based sorting signals KXX and KKXX are present in the mammalian DHHC4 and DHHC6, respectively, and it is these motifs that restrict their localization to the ER (Gorleku et al., 2011). It is also revealed that the C-terminal 68 amino acids of the mammalian DHHC2 play an important role to define its subcellular localization to the ER and Golgi (Fukata et al., 2013). However, there is currently no information available on how plant PAT are targeted to individual membranes in the cell.
The Identified PAT/Substrate Pairs
As an enzyme PAT carries out its function mainly through substrate protein(s) it S-acylates. Therefore, to understand how PATs operate it is important to identify the target proteins they modify. However, to match an individual PAT and its S-acylated substrate proteins has proved to be a very difficult task so far. This is because: (1) the number of potential S-acylated proteins far exceed the number of their modifying PATs. For example, there are 7 PATs in yeast, however, ~50 S-acylated proteins were identified by a proteomic approach (Roth et al., 2006). Similarly, much more S-acylated proteins were isolated than the number of PATs present in mammals and Arabidopsis (Martin and Cravatt, 2009; Hemsley et al., 2013). Therefore, it seems most likely that at least some if not all PATs can S-acylate multiple substrate proteins, i.e., PATs do not have strict substrate specificity; (2) Many substrate proteins can be modified by more than one PATs. For instance, in yeast, the S-acylation of Vac8 is only partially reduced in the yeast PAT knock-out strain pfa3, thus it is most likely that Vac8 is S-acylated by Pfa3 as well as one another or other PATs (Smotrys et al., 2005). Similarly, Ras2 S-acylation is only partially suppressed in the absence of Erf2 hence other PATs are also capable to S-acylate Ras2 (Roth et al., 2006; Montoro et al., 2011). Therefore, these PATs have specific yet overlapping substrate specificity. For some peripheral membrane proteins in mammalian cells, their S-acylation is devoid of specificity altogether (Rocks et al., 2010). However, reports show that some PATs do have their preferentially modified proteins. For example, Swf1 in yeast prefers to function on transmembrane proteins that have cysteines close to TMDs (Roth et al., 2006). In human, integrin α4β6 is strictly modified by DHHC3 (Sharma et al., 2012); (3) No consensus sequences in S-acylated proteins have been found. Although many S-acylated proteins have been identified and some of them are S-acylated by the same PAT, there are no consensus sequences characterized in these proteins (Montoro et al., 2011).
In yeast, each of the five PATs have been mapped to one or more substrate proteins. However, the total number of these individual substrate proteins are still far less than ~50 S-acylated proteins identified (Roth et al., 2006). For example, Akr1 S-acylates casein kinases Yck1, Yck2, and Yck3 (Roth et al., 2002). It also S-acylates sphingosine kinase Lcb4 because 60-80% of reduction in S-acylation of Lcb4 was found in akr1 mutant yeast (Kihara et al., 2005). Other proteins that are also S-acylated by Akr1 are Meh1, Sna4 and the unknown function proteins such as Ypl199c, Ykl047w, and Ypl236c (Roth et al., 2006). Therefore, Akr1 alone can S-acylate at least 9 substrate proteins in yeast. It was noted that Akr1 prefers hydrophilic proteins that tether to membranes solely through N- or C-terminal palmitoyl modifications (Roth et al., 2006). Erf2 is responsible for the S-acylation of Ras and other signaling proteins such as Rho2, Rho3, Gpa1, Gpa2, and Ste18, all of which are heterolipidated (Bartels et al., 1999; Lobo et al., 2002; Roth et al., 2006; Zhang et al., 2013). Swf1 tends to S-acylate proteins that have juxta-TMD mapping cysteines, such as SNAREs (Valdez-Taubas and Pelham, 2005), mannosyltransferases including Mnn1, Mnn10 and Mnn11 and prion induction protein Pin2 (Roth et al., 2006). Pfa4 is devoted to the palmitoylation of a group of Amino Acid Permeases (AAPs). AAPs is a family of plasma membrane transporters with 12 TMDs and a conserved C-terminal Phe-Trp-Cys palmitoylation site. Experiments in C. neoformans showed that Pfa4 is also responsible for PM localization of Ras1 via palmitoylation (Merino et al., 2014). On the other hand, one substrate protein can be palmitoylated by multiple PATs. For example, S-acylation of Gpa2 is mediated by both Pfa5 and Erf2 (Roth et al., 2006; Greaves and Chamberlain, 2011; Zhang et al., 2013); Meh1 was S-acylated by Pfa3 and Akr1 (Greaves and Chamberlain, 2011); and an unknown protein Yg1108 was S-acylated equally by Erf2 and Pfa4 (Greaves and Chamberlain, 2011). However, so far the substrates of Akr2 has not been identified. Therefore, it is clear that both PATs and their substrate proteins are highly redundant in yeast. A summary of PATs and their substrate proteins in yeast is shown in Table 4.
Many substrate proteins of mammalian PATs have also been identified in recent years. These include GTP-binding proteins, cytoskeletal proteins, enzymes, neurotransmitter receptors and synaptic scaffolding proteins (Table 5). Similar to what is found in yeast, some proteins can be modified by more than one PAT and most PATs can modify multiple proteins such as DHHC2, 3, 5, 7, 8, 13, 15, 17, and 21 (Table 5). For instance, PSD-95, a protein that scaffolds receptors and signaling enzymes at the postsynapse (Topinka and Bredt, 1998) can be S-acylated by DHHC2, 3, 7, 8, 15, and 17 (Fukata et al., 2004, 2006; Greaves and Chamberlain, 2011; Butland et al., 2014). SNAP-25, a t-SNARE protein that regulates neurotransmitter release, is the substrate of DHHC2, 3, 7, 8, 15, and 17 (Greaves et al., 2009). S-acylation of a tyrosine kinase Fyn is mediated by DHHC2, 3, 7, 15, 20, and 21 (Mill et al., 2009). All these mentioned PAT/substrates and other pairs are listed in Table 5. On the other hand, one PAT can palmitoylate multiple substrate proteins. For example, DHHC2 palmitoylats cytoskeleton-associated protein 4 (CKAP4) and AKAP79/150 (Keith et al., 2012; Chavda et al., 2014); DHHC3 does integrin α6β4, Calmodulin-dependent protein kinase isoform 1γ (CaMKIγ), NMDA receptor subunits 2A and 2B (NR2A/B) and DR4 Takemoto-Kimura et al., 2007; Hayashi et al., 2009; Sharma et al., 2012; Yeste-Velasco et al., 2015; the S-acylation of Grip1b, δ-catenin, Flotillin-2, somatostatin receptor 5 and Ankyrin-G is carried out by DHHC5 (Brigidi et al., 2015). In the same fashion many other proteins are also palmitoylated by specific PATs (Table 5). Importantly, some DHHC proteins have been indicated to be involved in certain diseases, such as DHHC8 in schizophrenia, DHHC9 and 15 in X-linked mental retardation, DHHC17 in Huntington's disease and many PATs are involved in different types of cancer including DHHC2, 3, 7, 9, 11, 14, 17, 20, and 21 (Chavda et al., 2014; Yeste-Velasco et al., 2015). However, for some of them, their specific substrate proteins have not been identified.
Very little information is available for PAT/substrate pairs in other organisms. The only PAT/substrate pair characterized was in P. falciparum where PfDHHC1, an apicomplexan-specific and inner membrane complex-localized PAT, has identical expression pattern to two S-acylated proteins PfISP1 and PfISP3 (Wetzel et al., 2015).
In plant, the only putative PAT/substrates pairing identified is ATPAT10/AtCBL2, 3, 6. This was achieved by transient expression of AtCBL2, AtCBL3 and AtCBL6 in Arabidopsis protoplast, showing that the tonoplast localization of AtCBLs is lost in protoplast prepared from AtPAT10 loss-of-function mutant (Zhou et al., 2013).
Therefore, although many hundreds of S-acylated proteins, including putative ones isolated by large proteomic approaches were identified from different species at present, there are many more to come in the future due to the readily available proteomics facilities in large institutions. A framework for characterizing PAT/substrate selectivity is urgently required to set out to match individual PATs and their S-acylated substrate proteins in order to understand the mechanism of S-acylation in individual organism and in general.
De-S-acylation
Similar to phosphorylation and ubiquitiation, S-acylation process is reversible, which makes it a very important lipid modification of proteins (Hemsley, 2009). S-acylation turnover by de-S-acylation, can be constitutive or stimulated (Smotrys and Linder, 2004). Ras proteins were the first proteins to be reported to have dynamic S-acylation with different H-Ras has different de-S-acylation rates (Baker et al., 2003). S-acylation/ de-S-acylation of Fyn, a member of the Src kinase family, happens with a half-life of 1.5–2 h (Wolven et al., 1997; Zeidman et al., 2009). The de-S-acylation of Gα subunits is stimulated by the activation of G-protein-coupled receptors (Mumby et al., 1994; Linder and Deschenes, 2007). De-S-acylation of PSD-95 is enhanced by neuronal activity (El-Husseini et al., 2002).
At present only four protein thioesterases have been identified to catalyze the de-S-acylation process, including acyl protein thioesterases 1 (APT1) and 2 (APT2), palmitoyl thioesterases 1 (PPT1) and 2 (PPT2) (Tomatis et al., 2010; Hornemann, 2015). These enzymes carry out the de-S-acylation step in which the palmitate or other long chain fatty acids are removed from the S-acylated proteins (Linder and Deschenes, 2007). APT1 was first found in rat liver as a lysophospholipase and its substrates include Ras, Gα subunit, RGS4, SNAP-23, and eNOS (Toyoda et al., 1999; Akimzhanov and Boehning, 2015). APT2 was reported to de-S-acylate the growth-associated protein 43 (Tomatis et al., 2010). PPT1 is a soluble lipase that is localized in lysosomes and it is responsible for the degradation of S-acylated proteins (Linder and Deschenes, 2007; Chavda et al., 2014). The loss-of-function of PPT1 resulted in severe infantile neuronal ceroid lipofuscinosis (Vesa et al., 1995). PPT2 has very limited acyl protein thioesterase activity, which prefers de-S-acylating short-chain lipid substrate. Interestingly, study has shown that it is up-regulated in obesity (Bürger et al., 2012; Fox et al., 2012).
It is surprising that only four thioesterases have been identified so far yet many hundreds of S-acylated proteins were isolated from different genomes. The explanations for this could be: (1) thioesterases are broad specificity enzymes, each of which can de-S-acylate a wide range of substrates; (2) not all S-acylated proteins undergo de-S-acylation; (3) of course, it could be because many more thioesterases have not been found (Chavda et al., 2014). There currently no protein thioesterases have been identified from plant.
Mechanism of Protein S-acylation
It is well recognized that DHHC proteins transfer acyl group via a two-step catalytic mechanism in which the enzyme first modifies itself with palmitate (or other long chain fatty acids) in a process termed autoacylation. The enzyme then transfers the acyl group from itself onto its substrate proteins. However, the number and location of the S-acylated cysteines of a given PAT in the autoacylated intermediate is unknown. It is well accepted that the cysteine in the DHHC motif is the auto-S-acylation site because mutation in this residue results in loss of auto-acylation of many characterized PATs from yeast (Montoro et al., 2011), mammals (Ohno et al., 2012), and Arabidopsis (Hemsley et al., 2005; Qi et al., 2013). However, cysteines in other positions of PATs such as the CRD and other domains may also be autoacylated (Gottlieb et al., 2015). For instance, DHHC3 has 6 auto-S-acylation sites where 5 in the CRD, including Cys-132, Cys-133, Cys-146, Cys-157, and Cys-163, 1 in the N-terminal domain (Cys-24) (Gottlieb et al., 2015).
Techniques Used for Prediction and Confirmation of S-acylated Cysteines in Proteins
There is no consensus for sequences in the S-acylated proteins despite that many such proteins have been isolated through proteomics approach or individually confirmed via radioactive labeling or/and mutation studies. Nevertheless, it is noted that: (1) in some S-acylated soluble proteins the cysteine residues that are S-acylated are frequently surrounded by basic or hydrophobic amino acids, such as GAP-43 (Liu et al., 1993) at N-terminal motif, Yck2 (Roth et al., 2002) at C-terminal motif and SNAP-25b (Lane and Liu, 1997) at cysteine string motif (Smotrys and Linder, 2004); (2) in other S-acylated soluble proteins the Cys residue is located near the prenylated or myristoylated residues, resulting in the so-called dual lipidition. These proteins include the Arabidopsis α and γ subunits of heterotrimeric G protein (Adjobo-Hermans et al., 2006; Zeng et al., 2007), small GTPases (Deschenes et al., 1990; Bartels et al., 1999; Roth et al., 2006; Zhang et al., 2013); 3 for transmembrane proteins, the Cys residues are often situated in the cytoplasmic regions of membrane-spanning regions (Roth et al., 2006; Ohno et al., 2012). For instance, the S-acylation of C261-263 triplet in death receptor 4 (DR4) (Rossin et al., 2009) and C474 in β-secretase BACE1 (Motoki et al., 2012) promotes their association with lipid raft.
Based on the above information several software packages have been developed to predict the S-acylated cysteines, such as a clustering and scoring strategy known as CSS-Palm (Zhou et al., 2006), which has been updated to the latest version CSS-Palm 4.0 (freely available at http://csspalm.biocuckoo.org/), incremental feature selection (IFS)-Palm (Hu et al., 2011), weight, amino acid composition and position specific scoring (WAP)-Palm (Shi et al., 2013) and PalmPred (Kumari et al., 2014). All of them are on-line so that one can input the protein sequence of interest to predict the possibility of its S-acylation and where the Cys residues are located within the sequence. The prediction data from these platforms can then be confirmed experimentally. These techniques include:
1. PAT inhibitors. The palmitate analog, 2-bromopalmitate (2-BP) is the most commonly used inhibitor of S-acylation, which inhibits palmitoylation in cells and PAT activity of DHHC proteins in vitro (Webb et al., 2000; Fukata et al., 2004; Jennings et al., 2009). However, it lacks specificity and can also inhibit myristoylation and reduce de-acylation through inhibiting activities of acyl-protein thioesterases (Webb et al., 2000; Pedro et al., 2013). Tunicamycin and cerulenin are also used to inhibit S-acylation, but similar effect was found as 2-BP (Patterson and Skene, 1995; Lawrence et al., 1999). Recently, a compound, 2-(2-hydroxy-5-nitro-benzylidene)-benzo[b]thiophen-3-one, was shown to have more specificity, but it does not have selectivity for specific PAT, i.e., it inhibits activities of all PATs (Jennings et al., 2009), which means it still cannot be used to study the function of individual PAT. Therefore, it is clear that results obtained from these inhibitors should be further validated by mutational or biochemical analysis.
2. Mutational analysis to change the potential S-acylated Cysteine to alanine or serine. Both alanine and serine were frequently used to replace the cysteine to achieve similar results (Hemsley et al., 2005; Qi et al., 2013; Li et al., 2016). Cysteine and serine have very similar structure, when the cysteine is mutated to serine it can maintain the size and the properties of the putative S-acylated protein, in this case, serine is a better substitution for cysteine. However, compare to alanine, serine is more hydrophilic than cysteine and might also cause unwanted side chain effects (Nagano et al., 1999). In this specific study, both alanine and serine as the substitutions for cysteine are accepted so far. This is followed by comparing the effect on the differences of functions or the subcellular localizations to native protein. If a difference was found the proteins were most likely S-acylated at the cysteine residues that were mutated.
3. Biochemical assays to analyze the attachment of fatty acids of the individual proteins. This includes: (1) traditionally feed with tritiated fatty acids followed by exposures to X-ray film (Lavy et al., 2002); (2) azido-alkyne CLICK-chemistry (Martin and Cravatt, 2009); (3) Acyl-exchange, or Biotin-switch assay (Wan et al., 2007; Hemsley et al., 2008); and (4) direct resin immobilization (Forrester et al., 2011).
4. Direct detection of the S-acyl group by gas chromatography–mass spectrometry (GC-MS) analysis. The identification of lipid groups attached to proteins can help to understand the biophysical properties of the protein. This method has been successfully used to demonstrate S-acylation of CBL1 and CBL2, which are attached by palmitate and/or stearate (Batistic et al., 2008, 2012).
Specificities of PAT-Substrate Interaction
Although, it is generally accepted that PATs are lacking specificity toward their substrate proteins and vice versa studies in yeast showed that some PATs do exhibit preference to some substrate protein(s) compared than others. For instance, Akr1 prefers to S-acylate soluble proteins at their N- or C-terminus, such as Ypl236c S-acylated at N-terminal cysteine and Yck1 S-acylated at C-terminal cysteine. Erf2 and Pfa5 show preference for pre-lipidated substrates, such as prenylated Ras1 and Ras2, myristoylated Gpa1 and Gpa2. Swf1 and Pfa4, on the other hand, prefer single and multiple transmembrane proteins such as SNAREs and AAPs (Roth et al., 2006; Ohno et al., 2012). Similar conclusions were made from studies of mammalian DHHC proteins where it was found that DHHC3, 7, 8, and 14–17 had high activities toward soluble proteins, while DHHC2, 20 and 21 were highly active to integral membrane proteins (Ohno et al., 2012). However, it was also noted that most DHHC proteins in both yeast and mammals had overlap activity to modify pre-lipidated substrates, such as 4 yeast PATs and 16 mammalian PATs can all S-acylate the myristoylated Gpa2 (Ohno et al., 2012).
The question here is how PATs and their substrates recognize each other? To address this, studies were carried out on some PATs and their S-acylated proteins in mammalian system. It was reported that the AR domain of the two ankyrin repeat containing PATs DHHC13 and 17 in mammals can act as substrate-recruiting signal and recognizes the [VIAP][VIT]XXQP motif that is shared between some S-acylated proteins including SNAP25/23, CSP, HTT, and CLIP3 (Lemonidis et al., 2015). Fusing the AR domain of DHHC17 to the N terminus of DHHC3 that lacks an AR domain, can make DHHC3 a PAT for HTT which also supports the notion that the AR domain contributes to the substrate specificity of DHHC17 for HTT (Huang et al., 2009). DHHC3 and 7 interact with GABAAγ2 through a 14-amino acid cysteine rich domain (Fang et al., 2006). DHHC7 has two splicing isoforms, the longer one has additional 111 bp compared to the shorter one, which might possess its tissue-specific function since it expresses specifically in placenta, lung, liver, thymus and small intestine (Ohno et al., 2006). The recognition and S-acylation of PSD95 by DHHC17 depend on the N-terminal 13 amino acids of PSD-95 (Huang et al., 2009). The cysteine rich “CCPCC” motif of PI4KII is required for its S-acylation by DHHC3 and DHHC7 (Lu et al., 2012). Subtle changes in the S-acylation domains of proteins can alter their PAT specificity, which were proved from SNAP23/25 (Greaves et al., 2010). For instance, a SNAP25 mutant which lacks a proline located 25 residues downstream of the S-acylated domain can only be modified by DHHC3 but not DHHC17 (Greaves et al., 2009). Therefore, specific domains in PATs and their substrate proteins are required for recognition and S-acylation to occur.
Subcellular localizations of PATs can have a profound effect on the type of proteins it can S-acylate (Greaves and Chamberlain, 2011). A transmembrane protein might only have access to be S-acylated by the PATs localized on the same membrane. For example, the PM-localized Gpa1 is S-acylated by the PM-localized Pfa5 in yeast (Ohno et al., 2006); tonoplast-localization of AtCBL2 and AtCBL3 is via S-acylation carried out by AtPAT10 which is also localized on tonoplast in Arabidopsis (Zhou et al., 2013).
For S-acylation of a protein to occur its prior membrane attachment via TMD, another lipid modification or protein-protein interaction is often acquired (Hemsley, 2015). For instance, TEM8 localizes at PM with one TMD, S-acylation of which negatively regulate its raft association (Abrami et al., 2006). Some proteins require another lipid modification such as myristoylation to target to certain membrane first before the S-acylation can take place. AtCBL1 is one of these proteins where myristoylation targets it on the ER, then the unknown ER-localized PAT S-acylates the myristoylated AtCBL1. This dual-lipidated AtCBL1 can subsequently be trafficked to the PM (Batistic et al., 2008). It was reported that the N-terminal 12-amino acid peptide of AtCBLs is sufficient to mediate the dual lipid modification and target to PM or tonoplast (Batistic et al., 2008). Therefore, the localization of a specific PAT for a given S-acylated substrate protein relies on where this protein is localized after the first lipid modification.
Important Molecules That Are Involved in S-acylation
Special molecules have either positive or negative effect on S-acylation of certain proteins. These molecules could be another protein, hormone, ions or protein inhibitors. For instance, although most DHHC-PATs in mammals can catalyze S-acylation independently DHHC9 needs a Golgi-localized protein GCP16 to specially palmitoylate H- and N-Ras (Swarthout et al., 2005). Ykt6, which possibly works as a co-factor of Pfa3 enhanced the S-acylation and vacuole localization of Vac8 (Dietrich et al., 2004; Hou et al., 2005; Meiringer and Ungermann, 2006). S-acylation and localization of Ras protein is catalyzed by the Erf2p-Erf4p complex in yeast (Zhao et al., 2002). Zinc ion is tightly bound to the cysteine rich domain of the DHHC3, which is essential for its structural integrity and PAT activity (Gottlieb et al., 2015). Selenoprotein K (SelK), an 11-kDa endoplasmic reticulum protein of unknown function (Shchedrina et al., 2011) is required for the S-acylation of both IP3R (inositol-1, 4, 5-triphosphate receptor) and CD36 (Fredericks and Hoffmann, 2015). S-acylation of PI4KII is cholesterol-dependent (Lu et al., 2012). Some compounds might have negative effect to specific S-acylation, such as curcumin can prevent S-acylation of integrin β4 by DHHC3 in breast cancer cells (Coleman et al., 2015). Understanding the involvement of these molecules in S-acylation could provide important information in designing and developing new drugs to target the disease and cancer-related S-acylation machinery.
Conclusion and Future Perspectives
Ever since the discovery of the first S-acyltransferase, Akr1 from yeast in 2002, which lead to the realization of protein S-acylation being an enzymatic process rather than a simultaneous addition of a long chain fatty acid to proteins, research on S-acylation of proteins has accelerated in a remarkable speed in the past decade. Yeast, as a simple unicellular model eukaryote, has been the first choice for researchers to study S-acylation. The knowledge learnt from the yeast system has then been applied in guiding similar studies in other organisms. As such the important roles of protein S-acylation in growth and development, especially in different human diseases, such as cancers, have hence attracted much attention and become a hot area of research in recent years.
Although, progress has been made toward understanding various aspects of protein palmitoylation the corresponding research in plants is trailing behind that in yeast and mammals. Judging from the wide arrays of S-acylated proteins identified recently from Arabidopsis and Poplar by proteomic studies it is clear that S-acylation plays variable and important roles in plant growth, development and environmental adaption (Hemsley et al., 2013; Srivastava et al., 2016). The knowledge learnt and methodologies developed from yeast and mammals will no doubt provide important clues and necessary tools for us to conduct more efficient research on S-acylation in plants in the coming years. Specifically, (1) the roles of the remaining 21 AtPATs in Arabidopsis will need to be characterized. PATs from other plant species, such as poplar, especially with its S-acylated proteins being isolated recently, will also need to be characterized to see if plant PATs share functional similarity, this will further validate the data obtained from Arabidopsis PATs so far; (2) To match individual PATs with their S-acylated substrate proteins in Arabidopsis and poplar. At present, the only plant PAT with tentative mapped substrate proteins is the Arabidopsis AtPAT10 where it was found that the tonoplast localization of transiently expressed CBL2,3,6 were lost in the protoplast prepared from leaf cells of AtPAT10 loss-of-function mutant plant (Zhou et al., 2013). This indicates that AtPAT10 functions in calcium signaling and salt stress through the actions of these CBLs. Similar approaches could be used to map other PAT/substrate(s) pairs in Arabidopsis and poplar. This will provide further insights to substrate specificity of PATs and molecular mechanisms how PATs function in plants; (3) De-palmitoylation enzymes. S-acylation of proteins is a reversible process where S-acylation is catalyzed by PATs and De-palmitoylation is by acyl protein thioesterases. While 4 such enzymes have been identified and characterized from mammals none from plant. Therefore, research in this area is paramount.
Author Contributions
YL and BQ both contributed in the writing and approving it for publication.
Funding
This review was produced as part of project about “Protein S-acyl transferases,” which was funded by National Natural Science Foundation of China (Grant No. 31170233 to BQ).
Conflict of Interest Statement
The authors declare that the research was conducted in the absence of any commercial or financial relationships that could be construed as a potential conflict of interest.
References
Abrami, L., Kunz, B., Iacovache, I., and Van Der Goot, F. G. (2008). Palmitoylation and ubiquitination regulate exit of the Wnt signaling protein LRP6 from the endoplasmic reticulum. Proc. Natl. Acad. Sci. U.S.A. 105, 5384–5389. doi: 10.1073/pnas.0710389105
Abrami, L., Leppla, S. H., and Van Der Goot, F. G. (2006). Receptor palmitoylation and ubiquitination regulate anthrax toxin endocytosis. J. Cell Biol. 172, 309–320. doi: 10.1083/jcb.200507067
Adams, M., Harrington, B., He, Y., Davies, C., Wallace, S., Chetty, N., et al. (2015). EGF inhibits constitutive internalization and palmitoylation-dependent degradation of membrane-spanning procancer CDCP1 promoting its availability on the cell surface. Oncogene 34, 1375–1383. doi: 10.1038/onc.2014.88
Adjobo-Hermans, M. J., Goedhart, J., and Gadella, T. W. (2006). Plant G protein heterotrimers require dual lipidation motifs of Gα and Gγ and do not dissociate upon activation. J. Cell Sci. 119, 5087–5097. doi: 10.1242/jcs.03284
Akimzhanov, A. M., and Boehning, D. (2015). Rapid and transient palmitoylation of the tyrosine kinase Lck mediates Fas signaling. Proc. Natl. Acad. Sci. U.S.A. 112, 11876–11880. doi: 10.1073/pnas.1509929112
Al Abdallah, Q., and Fortwendel, J. R. (2015). Exploration of Aspergillus fumigatus Ras pathways for novel antifungal drug targets. Front. Microbiol. 6:128. doi: 10.3389/fmicb.2015.00128
Alassimone, J., Fujita, S., Doblas, V. G., Van Dop, M., Barberon, M., Kalmbach, L., et al. (2016). Polarly localized kinase SGN1 is required for Casparian strip integrity and positioning. Nat. Plants 2:16113. doi: 10.1038/nplants.2016.113
Alvarez, E., Gironès, N., and Davis, R. J. (1990). A point mutation in the cytoplasmic domain of the transferrin receptor inhibits endocytosis. Biochem. J. 267, 31–35. doi: 10.1042/bj2670031
Antinone, S. E., Ghadge, G. D., Lam, T. T., Wang, L., Roos, R. P., and Green, W. N. (2013). Palmitoylation of superoxide dismutase 1 (SOD1) is increased for familial amyotrophic lateral sclerosis-linked SOD1 mutants. J. Biol. Chem. 288, 21606–21617. doi: 10.1074/jbc.M113.487231
Babu, P., Deschenes, R. J., and Robinson, L. C. (2004). Akr1p-dependent palmitoylation of Yck2p yeast casein kinase 1 is necessary and sufficient for plasma membrane targeting. Journal of Biol. Chem. 279, 27138–27147. doi: 10.1074/jbc.M403071200
Baekkeskov, S., and Kanaani, J. (2009). Palmitoylation cycles and regulation of protein function (Review). Mol. Membr. Biol. 26, 42–54. doi: 10.1080/09687680802680108
Baker, T. L., Zheng, H., Walker, J., Coloff, J. L., and Buss, J. E. (2003). Distinct rates of palmitate turnover on membrane-bound cellular and oncogenic H-ras. J. Biol. Chem. 278, 19292–19300. doi: 10.1074/jbc.M206956200
Bannan, B. A., Van Etten, J., Kohler, J. A., Tsoi, Y., Hansen, N. M., Sigmon, S., et al. (2008). The Drosophila protein palmitoylome: characterizing palmitoyl-thioesterases and DHHC palmitoyl-transferases. Fly 2, 198–214. doi: 10.4161/fly.6621
Bartels, D. J., Mitchell, D. A., Dong, X., and Deschenes, R. J. (1999). Erf2, a novel gene product that affects the localization and palmitoylation of Ras2 in Saccharomyces cerevisiae. Mol. Cell. Biol. 19, 6775–6787. doi: 10.1128/MCB.19.10.6775
Batistič, O. (2012). Genomics and localization of the Arabidopsis DHHC-Cysteine-rich domain S-Acyltransferase protein family. Plant Physiol. 160, 1597–1612. doi: 10.1104/pp.112.203968
Batistic, O., Rehers, M., Akerman, A., Schlücking, K., Steinhorst, L., Yalovsky, S., et al. (2012). S-acylation-dependent association of the calcium sensor CBL2 with the vacuolar membrane is essential for proper abscisic acid responses. Cell Res. 22, 1155–1168. doi: 10.1038/cr.2012.71
Batistic, O., Sorek, N., Schültke, S., Yalovsky, S., and Kudla, J. (2008). Dual fatty acyl modification determines the localization and plasma membrane targeting of CBL/CIPK Ca2+ signaling complexes in Arabidopsis. Plant Cell 20, 1346–1362. doi: 10.1105/tpc.108.058123
Baumgart, F., Corral-Escariz, M., Pérez-Gil, J., and Rodríguez-Crespo, I. (2010). Palmitoylation of R-Ras by human DHHC19, a palmitoyl transferase with a CaaX box. Biochim. et Biophys. Acta 1798, 592–604. doi: 10.1016/j.bbamem.2010.01.002
Beck, J. R., Rodriguez-Fernandez, I. A., De Leon, J. C., Huynh, M.-H., Carruthers, V. B., Morrissette, N. S., et al. (2010). A novel family of Toxoplasma IMC proteins displays a hierarchical organization and functions in coordinating parasite division. PLoS Pathog. 6:e1001094. doi: 10.1371/journal.ppat.1001094
Benjannet, S., Elagoz, A., Wickham, L., Mamarbachi, M., Munzer, J. S., Basak, A., et al. (2001). Post-translational processing of β-Secretase (β-Amyloid-converting Enzyme) and its ectodomain shedding the pro-and transmembrane/cytosolic domains affect its cellular activity and amyloid-β production. J. Biol. Chem. 276, 10879–10887. doi: 10.1074/jbc.M009899200
Bijlmakers, M.-J. (2009). Protein acylation and localization in T cell signaling (Review). Mol. Membr. Biol. 26, 93–103. doi: 10.1080/09687680802650481
Bizzozero, O. A., Bixler, H. A., and Pastuszyn, A. (2001). Structural determinants influencing the reaction of cysteine-containing peptides with palmitoyl-coenzyme A and other thioesters. Biochim. Biophys. Acta 1545, 278–288. doi: 10.1016/S0167-4838(00)00291-0
Blaskovic, S., Blanc, M., and Goot, F. G. (2013). What does S-palmitoylation do to membrane proteins? FEBS J. 280, 2766–2774. doi: 10.1111/febs.12263
Boyle, P. C., Schwizer, S., Hind, S. R., Kraus, C. M., Diaz, S. T., He, B., et al. (2016). Detecting N-myristoylation and S-acylation of host and pathogen proteins in plants using click chemistry. Plant Methods 12, 38. doi: 10.1186/s13007-016-0138-2
Brett, K., Kordyukova, L. V., Serebryakova, M. V., Mintaev, R. R., Alexeevski, A. V., and Veit, M. (2014). Site-specific S-acylation of influenza virus hemagglutinin the location of the acylation site relative to the membrane border is the decisive factor for attachment of stearate. J. Biol. Chem. 289, 34978–34989. doi: 10.1074/jbc.M114.586180
Brigidi, G. S., Santyr, B., Shimell, J., Jovellar, B., and Bamji, S. X. (2015). Activity-regulated trafficking of the palmitoyl-acyl transferase DHHC5. Nat. Commun. 6:8200. doi: 10.1038/ncomms9200
Brigidi, G. S., Sun, Y., Beccano-Kelly, D., Pitman, K., Mobasser, M., Borgland, S. L., et al. (2014). Palmitoylation of [delta]-catenin by DHHC5 mediates activity-induced synapse plasticity. Nat. Neurosci. 17, 522–532. doi: 10.1038/nn.3657
Buglino, J. A., and Resh, M. D. (2012). Palmitoylation of Hedgehog proteins. Vitam. Horm. 88, 229. doi: 10.1016/B978-0-12-394622-5.00010-9
Bürger, M., Zimmermann, T. J., Kondoh, Y., Stege, P., Watanabe, N., Osada, H., et al. (2012). Crystal structure of the predicted phospholipase LYPLAL1 reveals unexpected functional plasticity despite close relationship to acyl protein thioesterases. J. Lipid Res. 53, 43–50. doi: 10.1194/jlr.M019851
Butland, S. L., Sanders, S. S., Schmidt, M. E., Riechers, S.-P., Lin, D. T., Martin, D. D., et al. (2014). The palmitoyl acyltransferase HIP14 shares a high proportion of interactors with huntingtin: implications for a role in the pathogenesis of Huntington's disease. Hum. Mol. Genet. 23, 4142–4160. doi: 10.1093/hmg/ddu137
Chakrabandhu, K., Hérincs, Z., Huault, S., Dost, B., Peng, L., Conchonaud, F., et al. (2007). Palmitoylation is required for efficient Fas cell death signaling. EMBO J. 26, 209–220. doi: 10.1038/sj.emboj.7601456
Chamoun, Z., Mann, R. K., Nellen, D., Von Kessler, D. P., Bellotto, M., Beachy, P. A., et al. (2001). Skinny hedgehog, an acyltransferase required for palmitoylation and activity of the hedgehog signal. Science 293, 2080–2084. doi: 10.1126/science.1064437
Charollais, J., and Van Der Goot, F. G. (2009). Palmitoylation of membrane proteins (Review). Mol. Membr. Biol. 26, 55–66. doi: 10.1080/09687680802620369
Charych, E. I., Jiang, L.-X., Lo, F., Sullivan, K., and Brandon, N. J. (2010). Interplay of palmitoylation and phosphorylation in the trafficking and localization of phosphodiesterase 10A: implications for the treatment of schizophrenia. J. Neurosci. 30, 9027–9037. doi: 10.1523/JNEUROSCI.1635-10.2010
Chavda, B., Arnott, J. A., and Planey, S. L. (2014). Targeting protein palmitoylation: selective inhibitors and implications in disease. Expert Opin. Drug Discov. 9, 1005–1019. doi: 10.1517/17460441.2014.933802
Chen, Z. H., Lam, H. C., Jin, Y., Kim, H. P., Cao, J., Lee, S. J., et al. (2010). Autophagy protein microtubule-associated protein 1 light chain-3B (LC3B) activates extrinsic apoptosis during cigarette smoke-induced emphysema. Proc. Natl. Acad. Sci. U.S.A. 107, 18880–18885. doi: 10.1073/pnas.1005574107
Cocca, S. M. (2014). Phylogenetic Analysis, Modeling and Experimental Studies of the Saccharomyces cerevisiae Palmitoylated Protein Kinase Gene, ENV7. Long Beach, CA: California State University.
Coleman, D. T., Soung, Y. H., Surh, Y.-J., Cardelli, J. A., and Chung, J. (2015). Curcumin prevents palmitoylation of integrin β4 in breast cancer cells. PLoS ONE 10:e0125399. doi: 10.1371/journal.pone.0125399
Couve, A., Protopopov, V., and Gerst, J. E. (1995). Yeast synaptobrevin homologs are modified posttranslationally by the addition of palmitate. Proc. Natl. Acad. Sci. U.S.A. 92, 5987–5991. doi: 10.1073/pnas.92.13.5987
Deschenes, R. J., Resh, M. D., and Broach, J. (1990). Acylation and prenylation of proteins. Curr. Opin. Cell Biol. 2, 1108–1113. doi: 10.1016/0955-0674(90)90164-A
Dietzen, D. J., Randall Hastings, W., and Lublin, D. M. (1995). Caveolin is palmitoylated on multiple cysteine residues PALMITOYLAT ION IS NOT NECESSARY FOR LOCALIZATION OF CAVEOLIN TO CAVEOLAE. J. Biol. Chem. 270, 6838–6842. doi: 10.1074/jbc.270.12.6838
Dietrich, L. E., Gurezka, R., Veit, M., and Ungermann, C. (2004). The SNARE Ykt6 mediates protein palmitoylation during an early stage of homotypic vacuole fusion. EMBO J. 23, 45–53. doi: 10.1038/sj.emboj.7600015
Dowen, R. H., Engel, J. L., Shao, F., Ecker, J. R., and Dixon, J. E. (2009). A family of bacterial cysteine protease type III effectors utilizes acylation-dependent and-independent strategies to localize to plasma membranes. J. Biol. Chem. 284, 15867–15879. doi: 10.1074/jbc.M900519200
Edmonds, M. J., and Morgan, A. (2014). A systematic analysis of protein palmitoylation in Caenorhabditis elegans. BMC Genomics 15:841. doi: 10.1186/1471-2164-15-841
El-Husseini, A. E. D., Schnell, E., Dakoji, S., Sweeney, N., Zhou, Q., Prange, O., et al. (2002). Synaptic strength regulated by palmitate cycling on PSD-95. Cell 108, 849–863. doi: 10.1016/S0092-8674(02)00683-9
Fang, C., Deng, L., Keller, C. A., Fukata, M., Fukata, Y., Chen, G., et al. (2006). GODZ-mediated palmitoylation of GABAA receptors is required for normal assembly and function of GABAergic inhibitory synapses. J. Neurosci. 26, 12758–12768. doi: 10.1523/JNEUROSCI.4214-06.2006
Fernández-Hernando, C., Fukata, M., Bernatchez, P. N., Fukata, Y., Lin, M. I., Bredt, D. S., et al. (2006). Identification of Golgi-localized acyl transferases that palmitoylate and regulate endothelial nitric oxide synthase. J. Cell Biol. 174, 369–377. doi: 10.1083/jcb.200601051
Flannery, A. R., Czibener, C., and Andrews, N. W. (2010). Palmitoylation-dependent association with CD63 targets the Ca2+ sensor synaptotagmin VII to lysosomes. J. Cell Biol. 191, 599–613. doi: 10.1083/jcb.201003021
Forrester, M. T., Hess, D. T., Thompson, J. W., Hultman, R., Moseley, M. A., Stamler, J. S., et al. (2011). Site-specific analysis of protein S-acylation by resin-assisted capture. J. Lipid Res. 52, 393–398. doi: 10.1194/jlr.D011106
Fox, C. S., Liu, Y., White, C. C., Feitosa, M., Smith, A. V., Heard-Costa, N., et al. (2012). Genome-wide association for abdominal subcutaneous and visceral adipose reveals a novel locus for visceral fat in women. PLoS Genet. 8:e1002695. doi: 10.1371/journal.pgen.1002695
Fredericks, G. J., and Hoffmann, P. R. (2015). Selenoprotein K and protein palmitoylation. Antioxid. Redox Signal. 23, 854–862. doi: 10.1089/ars.2015.6375
Frénal, K., Kemp, L. E., and Soldati-Favre, D. (2014). Emerging roles for protein S-palmitoylation in Toxoplasma biology. Int. J. Parasitol. 44, 121–131. doi: 10.1016/j.ijpara.2013.09.004
Frénal, K., Polonais, V., Marq, J.-B., Stratmann, R., Limenitakis, J., and Soldati-Favre, D. (2010). Functional dissection of the apicomplexan glideosome molecular architecture. Cell Host Microbe 8, 343–357. doi: 10.1016/j.chom.2010.09.002
Frénal, K., Tay, C. L., Mueller, C., Bushell, E. S., Jia, Y., Graindorge, A., et al. (2013). Global analysis of apicomplexan protein S-Acyl transferases reveals an enzyme essential for invasion. Traffic 14, 895–911. doi: 10.1111/tra.12081
Fröhlich, M., Dejanovic, B., Kashkar, H., Schwarz, G., and Nussberger, S. (2014). S-palmitoylation represents a novel mechanism regulating the mitochondrial targeting of BAX and initiation of apoptosis. Cell Death Dis. 5, e1057. doi: 10.1038/cddis.2014.17
Fukasawa, M., Varlamov, O., Eng, W. S., Söllner, T. H., and Rothman, J. E. (2004). Localization and activity of the SNARE Ykt6 determined by its regulatory domain and palmitoylation. Proc. Natl. Acad. Sci. U.S.A. 101, 4815–4820. doi: 10.1073/pnas.0401183101
Fukata, M., Fukata, Y., Adesnik, H., Nicoll, R. A., and Bredt, D. S. (2004). Identification of PSD-95 palmitoylating enzymes. Neuron 44, 987–996. doi: 10.1016/j.neuron.2004.12.005
Fukata, Y., Dimitrov, A., Boncompain, G., Vielemeyer, O., Perez, F., and Fukata, M. (2013). Local palmitoylation cycles define activity-regulated postsynaptic subdomains. J. Cell Biol. 202, 145–161. doi: 10.1083/jcb.201302071
Fukata, Y., and Fukata, M. (2010). Protein palmitoylation in neuronal development and synaptic plasticity. Nat. Rev. Neurosci. 11, 161–175. doi: 10.1038/nrn2788
Fukata, Y., Iwanaga, T., and Fukata, M. (2006). Systematic screening for palmitoyl transferase activity of the DHHC protein family in mammalian cells. Methods 40, 177–182. doi: 10.1016/j.ymeth.2006.05.015
Furne, C., Corset, V., Hérincs, Z., Cahuzac, N., Hueber, A.-O., and Mehlen, P. (2006). The dependence receptor DCC requires lipid raft localization for cell death signaling. Proc. Natl. Acad. Sci. U.S.A. 103, 4128–4133. doi: 10.1073/pnas.0507864103
Gagne, J. M., and Clark, S. E. (2010). The Arabidopsis stem cell factor POLTERGEIST is membrane localized and phospholipid stimulated. Plant Cell 22, 729–743. doi: 10.1105/tpc.109.068734
Galli, L. M., Barnes, T. L., Secrest, S. S., Kadowaki, T., and Burrus, L. W. (2007). Porcupine-mediated lipid-modification regulates the activity and distribution of Wnt proteins in the chick neural tube. Development 134, 3339–3348. doi: 10.1242/dev.02881
Ganesan, L., and Levental, I. (2015). Pharmacological inhibition of protein lipidation. J. Membr. Biol. 248, 929–941. doi: 10.1007/s00232-015-9835-4
Gargantini, P. R., Gonzalez-Rizzo, S., Chinchilla, D., Raices, M., Giammaria, V., Ulloa, R. M., et al. (2006). A CDPK isoform participates in the regulation of nodule number in Medicago truncatula. Plant J. 48, 843–856. doi: 10.1111/j.1365-313X.2006.02910.x
Gleason, E. J., Lindsey, W. C., Kroft, T. L., Singson, A. W., and L'hernault, S. W. (2006). spe-10 encodes a DHHC–CRD zinc-finger membrane protein required for endoplasmic reticulum/Golgi membrane morphogenesis during Caenorhabditis elegans spermatogenesis. Genetics 172, 145–158. doi: 10.1534/genetics.105.047340
González, M. A., Quiroga, R., Maccioni, H., and Valdez, T. J. (2009). A novel motif at the C-terminus of palmitoyltransferases is essential for Swf1 and Pfa3 function in vivo. Biochem. J. 419, 301–308. doi: 10.1042/BJ20080921
Gorleku, O. A., Barns, A.-M., Prescott, G. R., Greaves, J., and Chamberlain, L. H. (2011). Endoplasmic reticulum localization of DHHC palmitoyltransferases mediated by lysine-based sorting signals. J. Biol. Chem. 286, 39573–39584. doi: 10.1074/jbc.M111.272369
Gottlieb, C. D., Zhang, S., and Linder, M. E. (2015). The Cysteine-rich Domain of the DHHC3 Palmitoyltransferase Is Palmitoylated and Contains Tightly Bound Zinc. J. Biol. Chem. 290, 29259–29269. doi: 10.1074/jbc.M115.691147
Grassie, M. A., Mccallum, J., Guzzi, F., Magee, A., Milligan, G., and Parenti, M. (1994). The palmitoylation status of the G-protein Go1 α regulates its activity of interaction with the plasma membrane. Biochem. J. 302, 913–920. doi: 10.1042/bj3020913
Greaves, J., and Chamberlain, L. H. (2007). Palmitoylation-dependent protein sorting. J. Cell Biol. 176, 249–254. doi: 10.1083/jcb.200610151
Greaves, J., and Chamberlain, L. H. (2010). S-acylation by the DHHC protein family. Biochem. Soc. Trans. 38, 522–524. doi: 10.1042/BST0380522
Greaves, J., and Chamberlain, L. H. (2011). DHHC palmitoyl transferases: substrate interactions and (patho) physiology. Trends Biochem. Sci. 36, 245–253. doi: 10.1016/j.tibs.2011.01.003
Greaves, J., Gorleku, O. A., Salaun, C., and Chamberlain, L. H. (2010). Palmitoylation of the SNAP25 protein family specificity and regulation by dhhc palmitoyl transferases. J. Biol. Chem. 285, 24629–24638. doi: 10.1074/jbc.M110.119289
Greaves, J., Prescott, G. R., Fukata, Y., Fukata, M., Salaun, C., and Chamberlain, L. H. (2009). The hydrophobic cysteine-rich domain of SNAP25 couples with downstream residues to mediate membrane interactions and recognition by DHHC palmitoyl transferases. Mol. Biol. Cell 20, 1845–1854. doi: 10.1091/mbc.E08-09-0944
Greaves, J., Salaun, C., Fukata, Y., Fukata, M., and Chamberlain, L. H. (2008). Palmitoylation and membrane interactions of the neuroprotective chaperone cysteine-string protein. J. Biol. Chem. 283, 25014–25026. doi: 10.1074/jbc.M802140200
Guardiola-Serrano, F., Rossin, A., Cahuzac, N., Lückerath, K., Melzer, I., Mailfert, S., et al. (2010). Palmitoylation of human FasL modulates its cell death-inducing function. Cell Death Dis. 1, e88. doi: 10.1038/cddis.2010.62
Harada, T., Matsuzaki, O., Hayashi, H., Sugano, S., Matsuda, A., and Nishida, E. (2003). AKRL1 and AKRL2 activate the JNK pathway. Genes Cells 8, 493–500. doi: 10.1046/j.1365-2443.2003.00650.x
Harashima, T., and Heitman, J. (2005). Gα subunit Gpa2 recruits kelch repeat subunits that inhibit receptor-G protein coupling during cAMP-induced dimorphic transitions in Saccharomyces cerevisiae. Mol. Biol. Cell 16, 4557–4571. doi: 10.1091/mbc.E05-05-0403
Hayashi, T., Rumbaugh, G., and Huganir, R. L. (2005). Differential regulation of AMPA receptor subunit trafficking by palmitoylation of two distinct sites. Neuron 47, 709–723. doi: 10.1016/j.neuron.2005.06.035
Hayashi, T., Thomas, G. M., and Huganir, R. L. (2009). Dual palmitoylation of NR2 subunits regulates NMDA receptor trafficking. Neuron 64, 213–226. doi: 10.1016/j.neuron.2009.08.017
Heakal, Y., Woll, M. P., Fox, T., Seaton, K., Levenson, R., and Kester, M. (2011). Neurotensin receptor-1 inducible palmitoylation is required for efficient receptor-mediated mitogenic-signaling within structured membrane microdomains. Cancer Biol. Ther. 12, 427–435. doi: 10.4161/cbt.12.5.15984
Hemler, M. E. (2014). Tetraspanin proteins promote multiple cancer stages. Nat. Rev. Cancer 14, 49–60. doi: 10.1038/nrc3640
Hemsley, P. A. (2009). Protein S-acylation in plants (Review). Mol. Membr. Biol. 26, 114–125. doi: 10.1080/09687680802680090
Hemsley, P. A. (2015). The importance of lipid modified proteins in plants. New Phytol. 205, 476–489. doi: 10.1111/nph.13085
Hemsley, P. A., and Grierson, C. S. (2011). The ankyrin repeats and DHHC S-acyl transferase domain of AKR1 act independently to regulate switching from vegetative to mating states in yeast. PLoS ONE 6:e28799. doi: 10.1371/journal.pone.0028799
Hemsley, P. A., Kemp, A. C., and Grierson, C. S. (2005). The TIP GROWTH DEFECTIVE1 S-acyl transferase regulates plant cell growth in Arabidopsis. Plant Cell 17, 2554–2563. doi: 10.1105/tpc.105.031237
Hemsley, P. A., Taylor, L., and Grierson, C. S. (2008). Assaying protein palmitoylation in plants. Plant Methods 4, 1. doi: 10.1186/1746-4811-4-2
Hemsley, P. A., Weimar, T., Lilley, K. S., Dupree, P., and Grierson, C. S. (2013). A proteomic approach identifies many novel palmitoylated proteins in Arabidopsis. New Phytol. 197, 805–814. doi: 10.1111/nph.12077
Hirschman, J. E., and Jenness, D. D. (1999). Dual lipid modification of the yeast Gγ subunit Ste18p determines membrane localization of Gβγ. Mol. Cell. Biol. 19, 7705–7711. doi: 10.1128/MCB.19.11.7705
Hodson, N., Invergo, B., Rayner, J. C., and Choudhary, J. S. (2015). Palmitoylation and palmitoyl-transferases in Plasmodium parasites. Biochem. Soc. Trans. 43, 240–245. doi: 10.1042/BST20140289
Hornemann, T. (2015). Palmitoylation and depalmitoylation defects. J. Inherit. Metab. Dis. 38, 179–186. doi: 10.1007/s10545-014-9753-0
Hou, H., Subramanian, K., Lagrassa, T. J., Markgraf, D., Dietrich, L. E., Urban, J., et al. (2005). The DHHC protein Pfa3 affects vacuole-associated palmitoylation of the fusion factor Vac8. Proc. Natl. Acad. Sci. U.S.A. 102, 17366–17371. doi: 10.1073/pnas.0508885102
Hou, H., John Peter, A. T., Meiringer, C., Subramanian, K., and Ungermann, C. (2009). Analysis of DHHC acyltransferases implies overlapping substrate specificity and a two-step reaction mechanism. Traffic 10, 1061–1073. doi: 10.1111/j.1600-0854.2009.00925.x
Hu, L. L., Wan, S. B., Niu, S., Shi, X. H., Li, H. P., Cai, Y. D., et al. (2011). Prediction and analysis of protein palmitoylation sites. Biochimie 93, 489–496. doi: 10.1016/j.biochi.2010.10.022
Huang, K., Kang, M. H., Askew, C., Kang, R., Sanders, S. S., Wan, J., et al. (2010). Palmitoylation and function of glial glutamate transporter-1 is reduced in the YAC128 mouse model of Huntington disease. Neurobiol. Dis. 40, 207–215. doi: 10.1016/j.nbd.2010.05.027
Huang, K., Sanders, S., Singaraja, R., Orban, P., Cijsouw, T., Arstikaitis, P., et al. (2009). Neuronal palmitoyl acyl transferases exhibit distinct substrate specificity. FASEB J. 23, 2605–2615. doi: 10.1096/fj.08-127399
Huang, K., Yanai, A., Kang, R., Arstikaitis, P., Singaraja, R. R., Metzler, M., et al. (2004). Huntingtin-interacting protein HIP14 is a palmitoyl transferase involved in palmitoylation and trafficking of multiple neuronal proteins. Neuron 44, 977–986. doi: 10.1016/j.neuron.2004.11.027
Hundt, M., Harada, Y., De Giorgio, L., Tanimura, N., Zhang, W., and Altman, A. (2009). Palmitoylation-dependent plasma membrane transport but lipid raft-independent signaling by linker for activation of T cells. J. Immunol. 183, 1685–1694. doi: 10.4049/jimmunol.0803921
Hurst, C. H., and Hemsley, P. A. (2015). Current perspective on protein S-acylation in plants: more than just a fatty anchor? J. Exp. Bot. 66, 1599–1606. doi: 10.1093/jxb/erv053
Ivaldi, C., Martin, B. R., Kieffer-Jaquinod, S., Chapel, A., Levade, T., Garin, J., et al. (2012). Proteomic analysis of S-acylated proteins in human B cells reveals palmitoylation of the immune regulators CD20 and CD23. PLoS ONE 7:e37187. doi: 10.1371/journal.pone.0037187
Ivanov, S. S., and Roy, C. (2013). “Host lipidation: a mechanism for spatial regulation of Legionella effectors,” in Molecular Mechanisms in Legionella Pathogenesis, ed H. Hilbi (Springer), 135–154.
Jennings, B. C., Nadolski, M. J., Ling, Y., Baker, M. B., Harrison, M. L., Deschenes, R. J., et al. (2009). 2-Bromopalmitate and 2-(2-hydroxy-5-nitro-benzylidene)-benzo [b] thiophen-3-one inhibit DHHC-mediated palmitoylation in vitro. J. Lipid Res. 50, 233–242. doi: 10.1194/jlr.M800270-JLR200
Ji, Y., Bachschmid, M. M., Costello, C. E., and Lin, C. (2016). S-to N-Palmitoyl transfer during proteomic sample preparation. J. Am. Soc. Mass Spectrom. 27, 677–685. doi: 10.1007/s13361-015-1319-3
Jones, T. L., Degtyarev, M. Y., and Backlund, P. S. (1997). The stoichiometry of Gαs palmitoylation in its basal and activated states. Biochemistry 36, 7185–7191. doi: 10.1021/bi9628376
Kang, R., Wan, J., Arstikaitis, P., Takahashi, H., Huang, K., Bailey, A. O., et al. (2008). Neural palmitoyl-proteomics reveals dynamic synaptic palmitoylation. Nature 456, 904–909. doi: 10.1038/nature07605
Keith, D. J., Sanderson, J. L., Gibson, E. S., Woolfrey, K. M., Robertson, H. R., Olszewski, K., et al. (2012). Palmitoylation of A-kinase anchoring protein 79/150 regulates dendritic endosomal targeting and synaptic plasticity mechanisms. J. Neurosci. 32, 7119–7136. doi: 10.1523/JNEUROSCI.0784-12.2012
Keller, C. A., Yuan, X., Panzanelli, P., Martin, M. L., Alldred, M., Sassoè-Pognetto, M., et al. (2004). The γ2 subunit of GABAA receptors is a substrate for palmitoylation by GODZ. J. Neurosci. 24, 5881–5891. doi: 10.1523/JNEUROSCI.1037-04.2004
Kihara, A., Kurotsu, F., Sano, T., Iwaki, S., and Igarashi, Y. (2005). Long-chain base kinase Lcb4 Is anchored to the membrane through its palmitoylation by Akr1. Mol. Cell. Biol. 25, 9189–9197. doi: 10.1128/MCB.25.21.9189-9197.2005
Kim, M. G., Da Cunha, L., Mcfall, A. J., Belkhadir, Y., Debroy, S., Dangl, J. L., et al. (2005). Two Pseudomonas syringae type III effectors inhibit RIN4-regulated basal defense in Arabidopsis. Cell 121, 749–759. doi: 10.1016/j.cell.2005.03.025
Kokkola, T., Kruse, C., Roy-Pogodzik, E.-M., Pekkinen, J., Bauch, C., Hönck, H.-H., et al. (2011). Somatostatin receptor 5 is palmitoylated by the interacting ZDHHC5 palmitoyltransferase. FEBS Lett. 585, 2665–2670. doi: 10.1016/j.febslet.2011.07.028
Konrad, S. S., Popp, C., Stratil, T. F., Jarsch, I. K., Thallmair, V., Folgmann, J., et al. (2014). S-acylation anchors remorin proteins to the plasma membrane but does not primarily determine their localization in membrane microdomains. New Phytol. 203, 758–769. doi: 10.1111/nph.12867
Kostiuk, M. A., Corvi, M. M., Keller, B. O., Plummer, G., Prescher, J. A., Hangauer, M. J., et al. (2008). Identification of palmitoylated mitochondrial proteins using a bio-orthogonal azido-palmitate analogue. FASEB J. 22, 721–732. doi: 10.1096/fj.07-9199com
Kumar, M., Wightman, R., Atanassov, I., Gupta, A., Hurst, C. H., Hemsley, P. A., et al. (2016). S-Acylation of the cellulose synthase complex is essential for its plasma membrane localization. Science 353, 166–169. doi: 10.1126/science.aaf4009
Kumari, B., Kumar, R., and Kumar, M. (2014). PalmPred: an SVM based palmitoylation prediction method using sequence profile information. PLoS ONE 9:e89246. doi: 10.1371/journal.pone.0089246
Kümmel, D., Heinemann, U., and Veit, M. (2006). Unique self-palmitoylation activity of the transport protein particle component Bet3: a mechanism required for protein stability. Proc. Natl. Acad. Sci. U.S.A. 103, 12701–12706. doi: 10.1073/pnas.0603513103
Lai, J., Yu, B., Cao, Z., Chen, Y., Wu, Q., Huang, J., et al. (2015). Two homologous protein S-acyltransferases, PAT13 and PAT14, cooperatively regulate leaf senescence in Arabidopsis. J. Exp. Bot. 66, 6345–6353. doi: 10.1093/jxb/erv347
Lakkaraju, A. K., Abrami, L., Lemmin, T., Blaskovic, S., Kunz, B., Kihara, A., et al. (2012). Palmitoylated calnexin is a key component of the ribosome–translocon complex. EMBO J. 31, 1823–1835. doi: 10.1038/emboj.2012.15
Lam, K. K., Davey, M., Sun, B., Roth, A. F., Davis, N. G., and Conibear, E. (2006). Palmitoylation by the DHHC protein Pfa4 regulates the ER exit of Chs3. J. Cell Biol. 174, 19–25. doi: 10.1083/jcb.200602049
Lane, S. R., and Liu, Y. (1997). Characterization of the palmitoylation domain of SNAP-25. J. Neurochem. 69, 1864–1869. doi: 10.1046/j.1471-4159.1997.69051864.x
Lavy, M., Bracha-Drori, K., Sternberg, H., and Yalovsky, S. (2002). A cell-specific, prenylation-independent mechanism regulates targeting of type II RACs. Plant Cell 14, 2431–2450. doi: 10.1105/tpc.005561
Lawrence, D. S., Zilfou, J. T., and Smith, C. D. (1999). Structure-activity studies of cerulenin analogues as protein palmitoylation inhibitors. J. Med. Chem. 42, 4932–4941. doi: 10.1021/jm980591s
Leclercq, J., Ranty, B., Sanchez-Ballesta, M.-T., Li, Z., Jones, B., Jauneau, A., et al. (2005). Molecular and biochemical characterization of LeCRK1, a ripening-associated tomato CDPK-related kinase. J. Exp. Bot. 56, 25–35. doi: 10.1093/jxb/eri003
Lemonidis, K., Sanchez-Perez, M. C., and Chamberlain, L. H. (2015). Identification of a novel sequence motif recognized by the ankyrin repeat domain of zDHHC17/13 S-Acyltransferases. J. Biol. Chem. 290, 21939–21950. doi: 10.1074/jbc.M115.657668
Levental, I., Lingwood, D., Grzybek, M., Coskun, Ü., and Simons, K. (2010). Palmitoylation regulates raft affinity for the majority of integral raft proteins. Proc. Natl. Acad. Sci. U.S.A. 107, 22050–22054. doi: 10.1073/pnas.1016184107
Li, Y., Martin, B. R., Cravatt, B. F., and Hofmann, S. L. (2012). DHHC5 protein palmitoylates flotillin-2 and is rapidly degraded on induction of neuronal differentiation in cultured cells. J. Biol. Chem. 287, 523–530. doi: 10.1074/jbc.M111.306183
Li, Y., Scott, R., Doughty, J., Grant, M., and Qi, B. (2016). Protein S-Acyltransferase 14: a specific role for palmitoylation in leaf senescence in Arabidopsis. Plant Physiol. 170, 415–428. doi: 10.1104/pp.15.00448
Linder, M. E., and Deschenes, R. J. (2007). Palmitoylation: policing protein stability and traffic. Nat. Rev. Mol. Cell Biol. 8, 74–84. doi: 10.1038/nrm2084
Liu, J., Zhong, S., Guo, X., Hao, L., Wei, X., Huang, Q., et al. (2013). Membrane-Bound RLCKs LIP1 and LIP2 are essential male factors controlling male-female attraction in Arabidopsis. Curr. Biol. 23, 993–998. doi: 10.1016/j.cub.2013.04.043
Liu, Y., Fisher, D. A., and Storm, D. R. (1993). Analysis of the palmitoylation and membrane targeting domain of neuromodulin (GAP-43) by site-specific mutagenesis. Biochemistry 32, 10714–10719. doi: 10.1021/bi00091a023
Lobo, S., Greentree, W. K., Linder, M. E., and Deschenes, R. J. (2002). Identification of a Ras palmitoyltransferase in Saccharomyces cerevisiae. J. Biol. Chem. 277, 41268–41273. doi: 10.1074/jbc.M206573200
Loisel, T. P., Adam, L., Hebert, T. E., and Bouvier, M. (1996). Agonist stimulation increases the turnover rate of β2AR-bound palmitate and promotes receptor depalmitoylation. Biochemistry 35, 15923–15932. doi: 10.1021/bi9611321
López-Barragán, M. J., Lemieux, J., Qui-ones, M., Williamson, K. C., Molina-Cruz, A., Cui, K., et al. (2011). Directional gene expression and antisense transcripts in sexual and asexual stages of Plasmodium falciparum. BMC Genomics 12:587. doi: 10.1186/1471-2164-12-587
Lu, D., Sun, H.-Q., Wang, H., Barylko, B., Fukata, Y., Fukata, M., et al. (2012). Phosphatidylinositol 4-kinase IIα is palmitoylated by Golgi-localized palmitoyltransferases in cholesterol-dependent manner. J. Biol. Chem. 287, 21856–21865. doi: 10.1074/jbc.M112.348094
Maciaszczyk-Dziubinska, E., Migocka, M., Wawrzycka, D., Markowska, K., and Wysocki, R. (2014). Multiple cysteine residues are necessary for sorting and transport activity of the arsenite permease Acr3p from Saccharomyces cerevisiae. Biochim. Biophys. Acta 1838, 747–755. doi: 10.1016/j.bbamem.2013.11.013
Maeda, A., Okano, K., Park, P. S.-H., Lem, J., Crouch, R. K., Maeda, T., et al. (2010). Palmitoylation stabilizes unliganded rod opsin. Proc. Natl. Acad. Sci. U.S.A. 107, 8428–8433. doi: 10.1073/pnas.1000640107
Maisse, C., Rossin, A., Cahuzac, N., Paradisi, A., Klein, C., Haillot, M.-L., et al. (2008). Lipid raft localization and palmitoylation: identification of two requirements for cell death induction by the tumor suppressors UNC5H. Exp. Cell Res. 314, 2544–2552. doi: 10.1016/j.yexcr.2008.06.001
Manandhar, S. P., Calle, E. N., and Gharakhanian, E. (2014). Distinct palmitoylation events at the amino-terminal conserved cysteines of Env7 direct its stability, localization, and vacuolar fusion regulation in S. cerevisiae. J. Biol. Chem. 289, 11431–11442. doi: 10.1074/jbc.M113.524082
Manandhar, S. P., Ricarte, F., Cocca, S. M., and Gharakhanian, E. (2013). Saccharomyces cerevisiae Env7 is a novel serine/threonine kinase 16-related protein kinase and negatively regulates organelle fusion at the lysosomal vacuole. Mol. Cell. Biol. 33, 526–542. doi: 10.1128/MCB.01303-12
Martin, B. R., and Cravatt, B. F. (2009). Large-scale profiling of protein palmitoylation in mammalian cells. Nat. Methods 6, 135–138. doi: 10.1038/nmeth.1293
Martin, B. R., Wang, C., Adibekian, A., Tully, S. E., and Cravatt, B. F. (2012). Global profiling of dynamic protein palmitoylation. Nat. Methods 9, 84–89. doi: 10.1038/nmeth.1769
Martin, D. D. O., Beauchamp, E., and Berthiaume, L. G. (2011). Post-translational myristoylation: fat matters in cellular life and death. Biochimie 93, 18–31. doi: 10.1016/j.biochi.2010.10.018
Marin, E. P., Derakhshan, B., Lam, T. T., Davalos, A., and Sessa, W. C. (2012). Endothelial cell palmitoylproteomic identifies novel lipid-modified targets and potential substrates for protein acyl transferases. Circ. Res. 110, 1336–1344. doi: 10.1161/CIRCRESAHA.112.269514
Martin, M. L., and Busconi, L. (2000). Membrane localization of a rice calcium-dependent protein kinase (CDPK) is mediated by myristoylation palmitoylation. Plant J. 24, 429–435.
Mccormick, P. J., Dumaresq-Doiron, K., Pluviose, A. S., Pichette, V., Tosato, G., and Lefrancois, S. (2008). Palmitoylation controls recycling in lysosomal sorting and trafficking. Traffic 9, 1984–1997. doi: 10.1111/j.1600-0854.2008.00814.x
Meiringer, C. T., and Ungermann, C. (2006). Probing protein palmitoylation at the yeast vacuole. Methods 40, 171–176. doi: 10.1016/j.ymeth.2006.06.020
Merino, M. C., Zamponi, N., Vranych, C. V., Touz, M. C., and Ropolo, A. S. (2014). Identification of Giardia lamblia DHHC Proteins and the Role of Protein S-palmitoylation in the Encystation Process. PLoS Negl. Trop. Dis. 8:e2997. doi: 10.1371/journal.pntd.0002997
Merrick, B. A., Dhungana, S., Williams, J. G., Aloor, J. J., Peddada, S., Tomer, K. B., et al. (2011). Proteomic profiling of S-acylated macrophage proteins identifies a role for palmitoylation in mitochondrial targeting of phospholipid scramblase 3. Mol. Cell. Proteomics 10:M110. 006007. doi: 10.1074/mcp.M110.006007
Mill, P., Lee, A. W., Fukata, Y., Tsutsumi, R., Fukata, M., Keighren, M., et al. (2009). Palmitoylation regulates epidermal homeostasis and hair follicle differentiation. PLoS Genet. 5:e1000748. doi: 10.1371/journal.pgen.1000748
Mitchell, D. A., Farh, L., Marshall, T. K., and Deschenes, R. J. (1994). A polybasic domain allows nonprenylated Ras proteins to function in Saccharomyces cerevisiae. J. Biol. Chem. 269, 21540–21546.
Mitchell, D. A., Vasudevan, A., Linder, M. E., and Deschenes, R. J. (2006). Thematic review series: lipid posttranslational modifications. Protein palmitoylation by a family of DHHC protein S-acyltransferases. J. Lipid Res. 47, 1118–1127. doi: 10.1194/jlr.R600007-JLR200
Miura, G. I., Buglino, J., Alvarado, D., Lemmon, M. A., Resh, M. D., and Treisman, J. E. (2006). Palmitoylation of the EGFR ligand Spitz by Rasp increases Spitz activity by restricting its diffusion. Dev. Cell 10, 167–176. doi: 10.1016/j.devcel.2005.11.017
Montoro, A. G., Ramirez, S. C., Quiroga, R., and Taubas, J. V. (2011). Specificity of transmembrane protein palmitoylation in yeast. PLoS ONE 6:e16969. doi: 10.1371/journal.pone.0016969
Motoki, K., Kume, H., Oda, A., Tamaoka, A., Hosaka, A., Kametani, F., et al. (2012). Neuronal β-amyloid generation is independent of lipid raft association of β-secretase BACE1: analysis with a palmitoylation-deficient mutant. Brain Behav. 2, 270–282. doi: 10.1002/brb3.52
Mukai, J., Dhilla, A., Drew, L. J., Stark, K. L., Cao, L., Macdermott, A. B., et al. (2008). Palmitoylation-dependent neurodevelopmental deficits in a mouse model of 22q11 microdeletion. Nat. Neurosci. 11, 1302–1310. doi: 10.1038/nn.2204
Mukai, J., Liu, H., Burt, R. A., Swor, D. E., Lai, W.-S., Karayiorgou, M., et al. (2004). Evidence that the gene encoding ZDHHC8 contributes to the risk of schizophrenia. Nat. Genet. 36, 725–731. doi: 10.1038/ng1375
Mumby, S. M., Kleuss, C., and Gilman, A. G. (1994). Receptor regulation of G-protein palmitoylation. Proc. Natl. Acad. Sci. U.S.A. 91, 2800–2804. doi: 10.1073/pnas.91.7.2800
Nagano, N., Ota, M., and Nishikawa, K. (1999). Strong hydrophobic nature of cysteine residues in proteins. FEBS Lett. 458, 69–71. doi: 10.1016/S0014-5793(99)01122-9
Nichols, C. B., Ost, K. S., Grogan, D. P., Pianalto, K., Hasan, S., and Alspaugh, J. A. (2015). Impact of protein palmitoylation on the virulence potential of Cryptococcus neoformans. Eukaryotic Cell 14, 626–635. doi: 10.1128/EC.00010-15
Oddi, S., Dainese, E., Sandiford, S., Fezza, F., Lanuti, M., Chiurchiù, V., et al. (2012). Effects of palmitoylation of Cys415 in helix 8 of the CB1 cannabinoid receptor on membrane localization and signalling. Br. J. Pharmacol. 165, 2635–2651. doi: 10.1111/j.1476-5381.2011.01658.x
Oh, Y., Jeon, Y., Hong, G., Kim, I., Woo, H., and Jung, Y. (2012). Regulation in the targeting of TRAIL receptor 1 to cell surface via GODZ for TRAIL sensitivity in tumor cells. Cell Death Different. 19, 1196–1207. doi: 10.1038/cdd.2011.209
Ohno, Y., Kashio, A., Ogata, R., Ishitomi, A., Yamazaki, Y., and Kihara, A. (2012). Analysis of substrate specificity of human DHHC protein acyltransferases using a yeast expression system. Mol. Biol. Cell 23, 4543–4551. doi: 10.1091/mbc.E12-05-0336
Ohno, Y., Kihara, A., Sano, T., and Igarashi, Y. (2006). Intracellular localization and tissue-specific distribution of human and yeast DHHC cysteine-rich domain-containing proteins. Biochim. Biophys. Acta 1761, 474–483. doi: 10.1016/j.bbalip.2006.03.010
Ohyama, T., Verstreken, P., Ly, C. V., Rosenmund, T., Rajan, A., Tien, A.-C., et al. (2007). Huntingtin-interacting protein 14, a palmitoyl transferase required for exocytosis and targeting of CSP to synaptic vesicles. J. Cell Biol. 179, 1481–1496. doi: 10.1083/jcb.200710061
Park, S., Patterson, E. E., Cobb, J., Audhya, A., Gartenberg, M. R., and Fox, C. A. (2011). Palmitoylation controls the dynamics of budding-yeast heterochromatin via the telomere-binding protein Rif1. Proc. Natl. Acad. Sci. U.S.A. 108, 14572–14577. doi: 10.1073/pnas.1105262108
Patterson, S. I., and Skene, J. (1995). Inhibition of dynamic protein palmitoylation in intact cells with tunicamycin. Meth. Enzymol. 250, 284. doi: 10.1016/0076-6879(95)50079-0
Pedro, M. P., Vilcaes, A. A., Tomatis, V. M., Oliveira, R. G., Gomez, G. A., and Daniotti, J. L. (2013). 2-Bromopalmitate reduces protein deacylation by inhibition of acyl-protein thioesterase enzymatic activities. PLoS ONE 8:e75232. doi: 10.1371/journal.pone.0075232
Pepinsky, R. B., Zeng, C., Wen, D., Rayhorn, P., Baker, D. P., Williams, K. P., et al. (1998). Identification of a palmitic acid-modified form of human Sonic hedgehog. J. Biol. Chem. 273, 14037–14045. doi: 10.1074/jbc.273.22.14037
Percherancier, Y., Planchenault, T., Valenzuela-Fernandez, A., Virelizier, J.-L., Arenzana-Seisdedos, F., and Bachelerie, F. (2001). Palmitoylation-dependent control of degradation, life span, and membrane expression of the CCR5 receptor. J. Biol. Chem. 276, 31936–31944. doi: 10.1074/jbc.M104013200
Ponimaskin, E., Behn, H., Adarichev, V., Voyno-Yasenetskaya, T. A., Offermanns, S., and Schmidt, M. F. (2000). Acylation of Gα13 is important for its interaction with thrombin receptor, transforming activity and actin stress fiber formation. FEBS Lett. 478, 173–177. doi: 10.1016/S0014-5793(00)01845-7
Ponimaskin, E., Dityateva, G., Ruonala, M. O., Fukata, M., Fukata, Y., Kobe, F., et al. (2008). Fibroblast growth factor-regulated palmitoylation of the neural cell adhesion molecule determines neuronal morphogenesis. J. Neurosci. 28, 8897–8907. doi: 10.1523/JNEUROSCI.2171-08.2008
Ponimaskin, E., Harteneck, C., Schultz, G., and Schmidt, M. F. (1998). A cysteine-11 to serine mutant of Gα12 impairs activation through the thrombin receptor. FEBS Lett. 429, 370–374. doi: 10.1016/S0014-5793(98)00638-3
Qi, B., Doughty, J., and Hooley, R. (2013). A Golgi and tonoplast localized S-acyl transferase is involved in cell expansion, cell division, vascular patterning and fertility in Arabidopsis. New Phytol. 200, 444–456. doi: 10.1111/nph.12385
Raíces, M., Ulloa, R. M., Macintosh, G. C., Crespi, M., and Téllez-Iñón, M. T. (2003). StCDPK1 is expressed in potato stolon tips and is induced by high sucrose concentration. J. Exp. Bot. 54, 2589–2591. doi: 10.1093/jxb/erg282
Raymond, F. L., Tarpey, P. S., Edkins, S., Tofts, C., O'meara, S., Teague, J., et al. (2007). Mutations in ZDHHC9, which encodes a palmitoyltransferase of NRAS and HRAS, cause X-linked mental retardation associated with a Marfanoid habitus. Am. J. Hum. Genet. 80, 982–987. doi: 10.1086/513609
Resh, M. D. (2006). Palmitoylation of ligands, receptors, and intracellular signaling molecules. Sci. Signal. 2006:re14. doi: 10.1126/stke.3592006re14
Robinson, L. J., Busconi, L., and Michel, T. (1995). Agonist-modulated palmitoylation of endothelial nitric oxide synthase. J. Biol. Chem. 270, 995–998. doi: 10.1074/jbc.270.3.995
Rocks, O., Gerauer, M., Vartak, N., Koch, S., Huang, Z.-P., Pechlivanis, M., et al. (2010). The palmitoylation machinery is a spatially organizing system for peripheral membrane proteins. Cell 141, 458–471. doi: 10.1016/j.cell.2010.04.007
Rocks, O., Peyker, A., Kahms, M., Verveer, P. J., Koerner, C., Lumbierres, M., et al. (2005). An acylation cycle regulates localization and activity of palmitoylated Ras isoforms. Science 307, 1746–1752. doi: 10.1126/science.1105654
Rossin, A., Derouet, M., Abdel-Sater, F., and Hueber, A. (2009). Palmitoylation of the TRAIL receptor DR4 confers an efficient TRAIL-induced cell death signalling. Biochem. J. 419, 185–192. doi: 10.1042/BJ20081212
Roth, A. F., Feng, Y., Chen, L., and Davis, N. G. (2002). The yeast DHHC cysteine-rich domain protein Akr1p is a palmitoyl transferase. J. Cell Biol. 159, 23–28. doi: 10.1083/jcb.200206120
Roth, A. F., Papanayotou, I., and Davis, N. G. (2011). The yeast kinase Yck2 has a tripartite palmitoylation signal. Mol. Biol. Cell 22, 2702–2715. doi: 10.1091/mbc.E11-02-0115
Roth, A. F., Wan, J., Bailey, A. O., Sun, B., Kuchar, J. A., Green, W. N., et al. (2006). Global analysis of protein palmitoylation in yeast. Cell 125, 1003–1013. doi: 10.1016/j.cell.2006.03.042
Running, M. P. (2014). The role of lipid post-translational modification in plant developmental processes. Front. Plant. Sci. 5:50. doi: 10.3389/fpls.2014.00050
Saleem, A. N., Chen, Y.-H., Baek, H. J., Hsiao, Y.-W., Huang, H.-W., Kao, H.-J., et al. (2010). Mice with alopecia, osteoporosis, and systemic amyloidosis due to mutation in Zdhhc13, a gene coding for palmitoyl acyltransferase. PLoS Genet. 6:e1000985. doi: 10.1371/journal.pgen.1000985
Schmick, M., Kraemer, A., and Bastiaens, P. I. (2015). Ras moves to stay in place. Trends Cell Biol. 25, 190–197. doi: 10.1016/j.tcb.2015.02.004
Schmidt, M. F., and Schlesinger, M. J. (1979). Fatty acid binding to Vesicular stomatitis virus glycoprotein: a new type of post-translational modification of the viral glycoprotein. Cell 17, 813–819. doi: 10.1016/0092-8674(79)90321-0
Sharma, C., Yang, X. H., and Hemler, M. E. (2008). DHHC2 affects palmitoylation, stability, and functions of tetraspanins CD9 and CD151. Mol. Biol. Cell. 19, 3415–3425. doi: 10.1091/mbc.E07-11-1164
Sharma, C., Rabinovitz, I., and Hemler, M. E. (2012). Palmitoylation by DHHC3 is critical for the function, expression, and stability of integrin α6β4. Cell. Mol. Life Sci. 69, 2233–2244. doi: 10.1007/s00018-012-0924-6
Shchedrina, V. A., Everley, R. A., Zhang, Y., Gygi, S. P., Hatfield, D. L., and Gladyshev, V. N. (2011). Selenoprotein K binds multiprotein complexes and is involved in the regulation of endoplasmic reticulum homeostasis. J. Biol. Chem. 286, 42937–42948. doi: 10.1074/jbc.M111.310920
Shi, S.-P., Sun, X.-Y., Qiu, J.-D., Suo, S.-B., Chen, X., Huang, S.-Y., et al. (2013). The prediction of palmitoylation site locations using a multiple feature extraction method. J. Mol. Graph. Model. 40, 125–130. doi: 10.1016/j.jmgm.2012.12.006
Shmueli, A., Segal, M., Sapir, T., Tsutsumi, R., Noritake, J., Bar, A., et al. (2010). Ndel1 palmitoylation: a new mean to regulate cytoplasmic dynein activity. EMBO J. 29, 107–119. doi: 10.1038/emboj.2009.325
Silvius, J. R., and L'heureux, F. (1994). Fluorometric evaluation of the affinities of isoprenylated peptides for lipid bilayers. Biochemistry 33, 3014–3022. doi: 10.1021/bi00176a034
Singaraja, R. R., Kang, M. H., Vaid, K., Sanders, S. S., Vilas, G. L., Arstikaitis, P., et al. (2009). Palmitoylation of ATP-binding cassette transporter A1 is essential for its trafficking and function. Circ. Res. 105, 138–147. doi: 10.1161/CIRCRESAHA.108.193011
Smotrys, J. E., and Linder, M. E. (2004). Palmitoylation of intracellular signaling proteins: regulation and function. Annu. Rev. Biochem. 73, 559–587. doi: 10.1146/annurev.biochem.73.011303.073954
Smotrys, J. E., Schoenfish, M. J., Stutz, M. A., and Linder, M. E. (2005). The vacuolar DHHC-CRD protein Pfa3p is a protein acyltransferase for Vac8p. J. Cell Biol. 170, 1091–1099. doi: 10.1083/jcb.200507048
Song, I.-W., Li, W.-R., Chen, L.-Y., Shen, L.-F., Liu, K.-M., Yen, J. J., et al. (2014). Palmitoyl acyltransferase, Zdhhc13, facilitates bone mass acquisition by regulating postnatal epiphyseal development and endochondral ossification: a mouse model. PLoS ONE 9:e92194. doi: 10.1371/journal.pone.0092194
Song, J., and Dohlman, H. G. (1996). Partial constitutive activation of pheromone responses by a palmitoylation-site mutant of a G protein α subunit in yeast. Biochemistry 35, 14806–14817. doi: 10.1021/bi961846b
Song, J., Hirschman, J., Gunn, K., and Dohlman, H. G. (1996). Regulation of membrane and subunit interactions by N-myristoylation of a G protein α subunit in yeast. J. Biol. Chem. 271, 20273–20283. doi: 10.1074/jbc.271.34.20273
Sorek, N., Poraty, L., Sternberg, H., Bar, E., Lewinsohn, E., and Yalovsky, S. (2007). Activation status-coupled transient S acylation determines membrane partitioning of a plant Rho-related GTPase. Mol. Cell. Biol. 27, 2144–2154. doi: 10.1128/MCB.02347-06
Soung, Y. H., and Chung, J. (2011). Curcumin inhibition of the functional interaction between integrin α6β4 and the epidermal growth factor receptor. Mol. Cancer Ther. 10, 883–891. doi: 10.1158/1535-7163.MCT-10-1053
Srivastava, V., Weber, J. R., Malm, E., Fouke, B. W., and Bulone, V. (2016). Proteomic analysis of a poplar cell suspension culture suggests a major role of protein S-acylation in diverse cellular processes. Front. Plant Sci. 7:477. doi: 10.3389/fpls.2016.00477
Swarthout, J. T., Lobo, S., Farh, L., Croke, M. R., Greentree, W. K., Deschenes, R. J., et al. (2005). DHHC9 and GCP16 constitute a human protein fatty acyltransferase with specificity for H-and N-Ras. J. Biol. Chem. 280, 31141–31148. doi: 10.1074/jbc.M504113200
Takada, R., Satomi, Y., Kurata, T., Ueno, N., Norioka, S., Kondoh, H., et al. (2006). Monounsaturated fatty acid modification of Wnt protein: its role in Wnt secretion. Dev. Cell 11, 791–801. doi: 10.1016/j.devcel.2006.10.003
Takemoto-Kimura, S., Ageta-Ishihara, N., Nonaka, M., Adachi-Morishima, A., Mano, T., Okamura, M., et al. (2007). Regulation of dendritogenesis via a lipid-raft-associated Ca 2+/calmodulin-dependent protein kinase CLICK-III/CaMKIγ. Neuron 54, 755–770. doi: 10.1016/j.neuron.2007.05.021
Termini, C. M., Cotter, M. L., Marjon, K. D., Buranda, T., Lidke, K. A., and Gillette, J. M. (2014). The membrane scaffold CD82 regulates cell adhesion by altering α4 integrin stability and molecular density. Mol. Biol. Cell 25, 1560–1573. doi: 10.1091/mbc.E13-11-0660
Thaa, B., Levental, I., Herrmann, A., and Veit, M. (2011). Intrinsic membrane association of the cytoplasmic tail of influenza virus M2 protein and lateral membrane sorting regulated by cholesterol binding and palmitoylation. Biochem. J. 437, 389–397. doi: 10.1042/BJ20110706
Thomas, G. M., Hayashi, T., Chen, C.-M., Chiu, S.-L., and Huganir, R. L. (2012). Palmitoylation by DHHC5/8 targets GRIP1 to dendritic endosomes to regulate AMPA-R trafficking. Neuron 73, 482–496. doi: 10.1016/j.neuron.2011.11.021
Tian, L., Mcclafferty, H., Jeffries, O., and Shipston, M. J. (2010). Multiple palmitoyltransferases are required for palmitoylation-dependent regulation of large conductance calcium-and voltage-activated potassium channels. J. Biol. Chem. 285, 23954–23962. doi: 10.1074/jbc.M110.137802
Tomatis, V. M., Trenchi, A., Gomez, G. A., and Daniotti, J. L. (2010). Acyl-protein thioesterase 2 catalizes the deacylation of peripheral membrane-associated GAP-43. PLoS ONE 5:e15045. doi: 10.1371/journal.pone.0015045
Topinka, J. R., and Bredt, D. S. (1998). N-terminal palmitoylation of PSD-95 regulates association with cell membranes and interaction with K+ channel K v 1.4. Neuron 20, 125–134. doi: 10.1016/S0896-6273(00)80440-7
Toyoda, T., Sugimoto, H., and Yamashita, S. (1999). Sequence, expression in Escherichia coli, and characterization of lysophospholipase II. Biochim. Biophys. Acta 1437, 182–193. doi: 10.1016/S1388-1981(99)00007-4
Tsutsumi, R., Fukata, Y., Noritake, J., Iwanaga, T., Perez, F., and Fukata, M. (2009). Identification of G protein α subunit-palmitoylating enzyme. Mol. Cell. Biol. 29, 435–447. doi: 10.1128/MCB.01144-08
Ueda, T., Yamaguchi, M., Uchimiya, H., and Nakano, A. (2001). Ara6, a plant-unique novel type Rab GTPase, functions in the endocytic pathway of Arabidopsis thaliana. EMBO J. 20, 4730–4741. doi: 10.1093/emboj/20.17.4730
Valdez-Taubas, J., and Pelham, H. (2005). Swf1-dependent palmitoylation of the SNARE Tlg1 prevents its ubiquitination and degradation. EMBO J. 24, 2524–2532. doi: 10.1038/sj.emboj.7600724
Vesa, J., Hellsten, E., Verkruyse, L. A., Camp, L. A., Rapola, J., Santavuori, P., et al. (1995). Mutations in the palmitoyl protein thioesterase gene causing infantile neuronal ceroid lipofuscinosis. Nature. 376, 584–587. doi: 10.1038/376584a0
Vetrivel, K. S., Meckler, X., Chen, Y., Nguyen, P. D., Seidah, N. G., Vassar, R., et al. (2009). Alzheimer disease Aβ production in the absence of S-palmitoylation-dependent targeting of BACE1 to lipid rafts. J. Biol. Chem. 284, 3793–3803. doi: 10.1074/jbc.M808920200
Wagner, R., Herwig, A., Azzouz, N., and Klenk, H. D. (2005). Acylation-mediated membrane anchoring of avian influenza virus hemagglutinin is essential for fusion pore formation and virus infectivity. J. Virol. 79, 6449–6458. doi: 10.1128/JVI.79.10.6449-6458.2005
Wan, J., Roth, A. F., Bailey, A. O., and Davis, N. G. (2007). Palmitoylated proteins: purification and identification. Nat. Protoc. 2, 1573–1584. doi: 10.1038/nprot.2007.225
Wang, X., Sutton, V. R., Peraza-Llanes, J. O., Yu, Z., Rosetta, R., Kou, Y.-C., et al. (2007). Mutations in X-linked PORCN, a putative regulator of Wnt signaling, cause focal dermal hypoplasia. Nat. Genet. 39, 836–838. doi: 10.1038/ng2057
Webb, Y., Hermida-Matsumoto, L., and Resh, M. D. (2000). Inhibition of protein palmitoylation, raft localization, and T cell signaling by 2-bromopalmitate and polyunsaturated fatty acids. J. Biol. Chem. 275, 261–270. doi: 10.1074/jbc.275.1.261
Wei, X., Schneider, J. G., Shenouda, S. M., Lee, A., Towler, D. A., Chakravarthy, M. V., et al. (2011). De novo lipogenesis maintains vascular homeostasis through endothelial nitric-oxide synthase (eNOS) palmitoylation. J. Biol. Chem. 286, 2933–2945. doi: 10.1074/jbc.M110.193037
Wedegaertner, P. B., Chu, D., Wilson, P., Levis, M. J., and Bourne, H. (1993). Palmitoylation is required for signaling functions and membrane attachment of Gq alpha and Gs alpha. J. Biol. Chem. 268, 25001–25008.
Wetzel, J., Herrmann, S., Swapna, L. S., Prusty, D., John Peter, A. T., Kono, M., et al. (2015). The role of palmitoylation for protein recruitment to the inner membrane complex of the malaria parasite. J. Biol. Chem. 290, 1712–1728. doi: 10.1074/jbc.M114.598094
Wolven, A., Okamura, H., Rosenblatt, Y., and Resh, M. (1997). Palmitoylation of p59fyn is reversible and sufficient for plasma membrane association. Mol. Biol. Cell 8, 1159–1173. doi: 10.1091/mbc.8.6.1159
Xie, Y., Wolff, D. W., Wei, T., Wang, B., Deng, C., Kirui, J. K., et al. (2009). Breast cancer migration and invasion depend on proteasome degradation of regulator of G-protein signaling 4. Cancer Res. 69, 5743–5751. doi: 10.1158/0008-5472.CAN-08-3564
Yang, G., and Cynader, M. S. (2011). Palmitoyl acyltransferase zD17 mediates neuronal responses in acute ischemic brain injury by regulating JNK activation in a signaling module. J. Neurosci. 31, 11980–11991. doi: 10.1523/JNEUROSCI.2510-11.2011
Yang, J., Brown, M. S., Liang, G., Grishin, N. V., and Goldstein, J. L. (2008). Identification of the acyltransferase that octanoylates ghrelin, an appetite-stimulating peptide hormone. Cell 132, 387–396. doi: 10.1016/j.cell.2008.01.017
Yang, W., Di Vizio, D., Kirchner, M., Steen, H., and Freeman, M. R. (2010). Proteome scale characterization of human S-acylated proteins in lipid raft-enriched and non-raft membranes. Mol. Cell. Proteomics 9, 54–70. doi: 10.1074/mcp.M800448-MCP200
Yeste-Velasco, M., Linder, M. E., and Lu, Y.-J. (2015). Protein S-palmitoylation and cancer. Biochim. Biophys. Acta 1856, 107–120. doi: 10.1016/j.bbcan.2015.06.004
Yuan, X., Zhang, S., Sun, M., Liu, S., Qi, B., and Li, X. (2013). Putative DHHC-Cysteine-Rich domain S-Acyltransferase in plants. PLoS ONE 8:75985. doi: 10.1371/journal.pone.0075985
Zeidman, R., Jackson, C. S., and Magee, A. I. (2009). Protein acyl thioesterases (Review). Mol. Membr. Biol. 26, 32–41. doi: 10.1080/09687680802629329
Zeng, Q., Wang, X., and Running, M. P. (2007). Dual lipid modification of Arabidopsis Gγ-subunits is required for efficient plasma membrane targeting. Plant Physiol. 143, 1119–1131. doi: 10.1104/pp.106.093583
Zhang, J., Planey, S. L., Ceballos, C., Stevens, S. M., Keay, S. K., and Zacharias, D. A. (2008). Identification of CKAP4/p63 as a major substrate of the palmitoyl acyltransferase DHHC2, a putative tumor suppressor, using a novel proteomics method. Mol. Cell. Proteomics 7, 1378–1388. doi: 10.1074/mcp.M800069-MCP200
Zhang, M. M., Wu, P.-Y. J., Kelly, F. D., Nurse, P., and Hang, H. C. (2013). Quantitative control of protein S-palmitoylation regulates meiotic entry in fission yeast. PLoS Biol. 11:e1001597. doi: 10.1371/journal.pbio.1001597
Zhao, L., Lobo, S., Dong, X., Ault, A. D., and Deschenes, R. J. (2002). Erf4p and Erf2p form an endoplasmic reticulum-associated complex involved in the plasma membrane localization of yeast Ras proteins. J. Biol. Chem. 277, 49352–49359. doi: 10.1074/jbc.M209760200
Zheng, Z. L., Nafisi, M., Tam, A., Li, H., Crowell, D. N., Chary, S. N., et al. (2002). Plasma membrane—associated ROP10 small GTPase is a specific negative regulator of abscisic acid responses in Arabidopsis. Plant Cell 14, 2787–2797 doi: 10.1105/tpc.005611
Zhou, B., Liu, L., Reddivari, M., and Zhang, X. A. (2004). The palmitoylation of metastasis suppressor KAI1/CD82 is important for its motility-and invasiveness-inhibitory activity. Cancer Res. 64, 7455–7463. doi: 10.1158/0008-5472.CAN-04-1574
Zhou, F., Xue, Y., Yao, X., and Xu, Y. (2006). CSS-Palm: palmitoylation site prediction with a clustering and scoring strategy (CSS). Bioinformatics 22, 894–896. doi: 10.1093/bioinformatics/btl013
Zhou, L. Z., Li, S., Feng, Q.-N., Zhang, Y.-L., Zhao, X., Zeng, Y.-L., et al. (2013). Protein S-ACYL Transferase10 is critical for development and salt tolerance in Arabidopsis. Plant Cell 25, 1093–1107 doi: 10.1105/tpc.112.108829
Keywords: lipid modification, S-acylation, PATs, substrate recognition and specificity, yeast, mammalian, plants
Citation: Li Y and Qi B (2017) Progress toward Understanding Protein S-acylation: Prospective in Plants. Front. Plant Sci. 8:346. doi: 10.3389/fpls.2017.00346
Received: 14 January 2017; Accepted: 28 February 2017;
Published: 24 March 2017.
Edited by:
Markus Geisler, University of Fribourg, SwitzerlandReviewed by:
Oliver Batistic, University of Münster, GermanyFrantisek Baluska, University of Bonn, Germany
Copyright © 2017 Li and Qi. This is an open-access article distributed under the terms of the Creative Commons Attribution License (CC BY). The use, distribution or reproduction in other forums is permitted, provided the original author(s) or licensor are credited and that the original publication in this journal is cited, in accordance with accepted academic practice. No use, distribution or reproduction is permitted which does not comply with these terms.
*Correspondence: Baoxiu Qi, YnNzYnFAYmF0aC5hYy51aw==