- 1Plant Molecular Biology Laboratory, Agri Biotech Foundation, Hyderabad, India
- 2Cell, Molecular Biology and Genetic Engineering Group, International Crops Research Institute for the Semi-Arid Tropics, Hyderabad, India
- 3Crop improvement group, International Center for Genetic Engineering and Biotechnology, New Delhi, India
Eukaryotic translational initiation factor 4A belong to family of helicases, involved in multifunctional activities during stress and non-stress conditions. The eIF4A gene was isolated and cloned from semi-arid cereal crop of Pennisetum glaucum. In present study, the PgeIF4A gene was expressed under the regulation of stress inducible Arabidopsis rd29A promoter in groundnut (cv JL-24) with bar as a selectable marker. The de-embryonated cotyledons were infected with Agrobacterium tumefaciens (LBA4404) carrying rd29A:PgeIF4A construct and generated high frequency of multiple shoots in phosphinothricin medium. Twenty- four T0 plants showed integration of both nos-bar and rd29A-PgeIF4A gene cassettes in genome with expected amplification products of 429 and 654 bps, respectively. Transgene copy number integration was observed in five T0 transgenic plants through Southern blot analysis. Predicted Mendelian ratio of segregation (3:1) was noted in transgenic plants at T1 generation. The T2 homozygous lines (L1-5, L8-2, and L16-2) expressing PgeIF4A gene were exhibited superior growth performance with respect to phenotypic parameters like shoot length, tap root length, and lateral root formation under simulated drought and salinity stresses compared to the wild type. In addition, the chlorophyll retention was found to be higher in these plants compared to the control plants. The quantitative real time—PCR results confirmed higher expression of PgeIF4A gene in L1-5, L8-3, and L16-2 plants imposed with drought/salt stress. Further, the salt stress tolerance was associated with increase in oxidative stress markers, such as superoxide dismutase accumulation, reactive oxygen species scavenging, and membrane stability in transgenic plants. Taken together our results confirmed that the PgeIF4A gene expressing transgenic groundnut plants exhibited better adaptation to stress conditions.
Introduction
Groundnut or peanut (Arachis hypogea L.) is one of the important food legumes and oil seed crops grown in the semi-arid tropics of the world. It is being cultivated on over 25.2 million ha worldwide with a total production of 41.2 million tons with an average yield of 1.67 tons/ha. India is the second largest producer of groundnut accounting 8 million tons from 6 million ha (FAOSTAT, 2014) which needs to be increased up to 14.8 million tons by 2020 to meet the growing demand. Most of the cultivated groundnut varieties are highly sensitive to abiotic and biotic stresses such as drought, salinity, low temperature, insects, fungal, viral, and bacterial diseases (Kumar and Kirti, 2015).
Conventional breeding approaches for abiotic stress tolerance in groundnut has met with limited success due to non-availability of desired QTLs and levels of polymorphisms in cultivated varieties. As groundnut is self-pollinating crop, transfer of genes into sensitive popular varieties through marker-assisted selection may leads to linkage drag (Bhatnagar-Mathur et al., 2014). An alternative, genetic engineering approach offers novel solution by transferring alien genes for improving groundnut varieties for abiotic stress tolerance.
Different classes of genes related to abiotic stress have been used to express in groundnut for improvement of stress tolerance. Overexpression of AtNHX (Na+/H+ antiporter) gene in groundnut leads to compartmentalization of Na+ ions in vacuoles, thereby improved tolerance against salinity and limited water conditions (Asif et al., 2011). A bacterial mtlD gene encoding mannitol 1-phosphate dehydrogenase when expressed in groundnut cultivar GG20 under a constitutive promoter CaMV35s conferred drought tolerance through accumulation of mannitol (Bhauso et al., 2014). The genes from Salicornia brachiata, ascorbate peroxidase (SbAPX), and abscisic acid stress ripening (SbASR) genes when expressed in groundnut displayed salt and drought stress tolerance coupled with more chlorophyll retention and enhanced levels of relative water content under normal/stress conditions (Singh et al., 2014; Tiwari et al., 2015). The transcription factors from Arabidopsis, AtNAC2 (Patil et al., 2014), AtDREB1A (Sarkar et al., 2014, 2016) and horsegram, MuNAC4 (Pandurangaiah et al., 2014) were exhibited drought and salt stress tolerance in groundnut by reducing the membrane damage and improving scavenging of reactive oxygen species scavenging (ROS). Groundnut transgenic plants (cv TMV-2) simultaneously expressing three TFs (AtDREB2A, AtHB7, and AtABF3) displayed to increase drought, salinity, and oxidative stress tolerance compared to wild type (Pruthvi et al., 2014). The AtDREB1A transcription factor was expressed in groundnut under the regulation of either rd29A or CaMV35s promoter separately. The phenotype of 35s:AtDREB1A exhibited delay in germination with severe growth retardation. However, positive growth regulation was seen in rd29A:AtDREB1A expressing plants and thereby improved 40% transpiration efficiency under limited water conditions (Bhatnagar-Mathur et al., 2014). Thus, expression of the stress related genes under the regulation of stress inducible promoters could be more rewarding rather than constitutive expression to minimize negative effects on plant growth performance (Sarkar et al., 2016).
Eukaryotic translational initiation factor 4A proteins belong to a family of DEAD box DNA/RNA helicases which catalyze the unwinding of stable RNA or DNA duplex during translation process. eIF4A is a prototype member of DEAD box helicase family protein that mediates the unwinding of double stranded RNA and binds to 40s ribosomal subunit during translational initiation process. Further these proteins stimulate stress induced pathways which mediate the stress tolerance (Tuteja et al., 2014). The eIF4A participates in ATP-dependent unwinding of the mRNA (Rogers et al., 2002; Linder, 2006). Plant based eIF4A was first reported in association with a cyclin dependent kinase during active cell proliferation (Hutchins et al., 2004). The eIF4A genes associated with abiotic stress tolerance have been identified from several plant species like tobacco (Owtrim et al., 1991), rice (Nishi et al., 1993), pea (Pham et al., 2000; Vashisht et al., 2005), and wheat (Metz and Browning, 1993).
The role of eIF4A genes in abiotic stress tolerance was studied through transgenic approach. A pea DNA helicase (PDH45) homologous to eIF4A when expressed in tobacco and rice imparted salt stress tolerance with minimal accumulation of sodium in transgenic plants (Sanan-Mishra et al., 2005; Nath et al., 2015). PDH45 gene when expressed in groundnut variety (K-134) showed high tolerance against moisture stress (Manjulatha et al., 2014). Multiple stress responsive genes such as OsAlfin (alfalfa zinc finger), PDH45 and PgHSF4 (Pennisetum glaucum heat shock factor) simultaneously expressed in groundnut conferred tolerance to simulated drought stress and enhanced yield (Ramu et al., 2015). Constitutive expression of PDH45 gene along with EaDREB2 was conferred higher salinity tolerance in sugarcane (Augustine et al., 2015).
Pearl millet [Pennisetum glaucum (L.) R. Br.], a hardy C4 plant, exhibits better adaptation to harsh arid or semi-arid climates as compared to other cereals (Yadav, 2010), thereby making it an ideal depository of several stress regulatory genes. In this study, we have cloned the cDNA encoding for eIF4A gene of P. glaucum and transferred into JL-24 variety through Agrobacterium. The transgenic groundnut plants were evaluated for their stress tolerance under different conditions and for their molecular and phenotypic features. This is the first report on evaluation and expression of eIF4A gene of P. glaucum in groundnut for revealing its role in drought and salinity stress tolerance.
Materials and Methods
Cloning of PgeIF4A Gene in Binary Vector under Stress Inducible Promoter rd29A
The cDNA encoding for PgeIF4A gene was isolated from stress induced seedlings of P. glaucum by using homology based gene cloning approach. Degenerate primers were designed based on conserved sequences found in the closely related monocot species. The full length gene was amplified using specific primers PgeIF4AF -Nde I and PgeIF4AR -Not I. The rd29A promoter (Accession no: AY973635) was isolated from Arabidopsis genomic DNA by using promoter specific primers overhang with restriction enzymes, Kpn I in sense primer and Nde I in anti-sense primer. The PgeIF4A gene fused in sense orientation at Nde I–Not I sites at downstream to the rd29A promoter (Kpn I–Nde I) and upstream to poly A terminator (Not I–Sac I) in plant expression vector, pGreen0229 (www.pgreen.ac.uk/) with bar (bialophos aminotransferase driven by nopaline synthase promoter) as a selectable marker. The recombinant rd29A:PgeIF4A:Poly A construct was then confirmed by restriction and sequence analysis.
Agrobacterium Transformation of PgeIF4A through Electroporation
The pGreen0229 vector harboring rd29A-PgeIF4A–poly A cassette and pSoup were co-transformed into Agrobacterium tumefaciens (LBA4404) with following condition of 2.5 kV for 5 μS by multi- electroporator (Eppendorf International, Germany). Electroporated samples were immediately transferred into 1 ml YEM (yeast extract mannitol) liquid medium and incubated for 5 h at 28°C with 200 rpm. Then the cells were harvested and plated on YEM medium supplemented with 50 mg/L kanamycin, 20 mg/L rifampicin, and 50 mg/L streptomycin. The recombinant colonies were observed after 48 h of incubation at 28°C and confirmed the positive colonies by PCR.
Seed Sterilization and Explants Preparation
Seeds of groundnut (cv JL-24) were collected from International Crops Research Institute for the Semi Arid Tropics (ICRISAT), Patancheru, India. Seeds were surface sterilized with 0.1% mercuric chloride for 8 min, followed through rinsing with sterile water and soaked for 4 h in distilled water. The seed coat was peeled off and the cotyledons were sliced vertically to prepare de-embryonated half cotyledon (DEC) aseptically which was used for Agrobacterium transformation.
Agrobacterium Mediated Groundnut Transformation with PgeIF4A Using DEC Explants
Healthy half DECs were infected with A. tumefaciens (LBA4404) suspension culture (OD600 = 0.5–0.6) by gentle shaking for 10 min and dried them on sterile Whatman filter paper under aseptic conditions. Then, the explants were co-cultivated for 48 h on MS basal medium (Murashige and Skoog, 1962) + B5 vitamins (Gamborg et al., 1968) supplemented with 3% sucrose, solidified with 0.8% agar and 100 μM acetosyringone. Further, the explants were washed with cefotaxime (250 mg/L) for the suppression of Agrobacterium. The explants transferred to shoot induction medium supplemented with MS salts + B5 + 24.5 mg/L of benzylaminopurine (BAP) + 21.5 mg/L of 2, 4—Dichlorophenoxy acetic acid (2,4-D) + 5 mg/L phosphinothricin (PPT) and 3% sucrose at pH 5.8 solidified with 0.8% agar. Cultures were maintained at 26 ± 1°C at 16 h photoperiod with white fluorescent light intensity of 60 mE m2s−1. Resistant induced shoots from un-differentiated calli at the stage of 3–5 cm length were transformed to shoot elongation medium supplemented with BAP (2 mg/L) and allowed to grow further for another 2 weeks to attain shoot elongation up to 7–9 cm. Elongated shoots were sub cultured into rooting medium containing NAA (0.5 mg/L) + PPT (5 mg/L) incubated for about 15 days. After root formation, the plantlets were transferred to transgenic glasshouse for further hardening and grown them till attaining the maturity. Seeds were harvested from the putative transformed and control plants for further analysis.
Molecular Confirmation of Transgenic Plants
Genomic DNA from un-transformed control and transformed plants (T0) was isolated using modified CTAB method (Doyle and Doyle, 1987). Transgene (rd29A-PgeIF4A) and marker gene (nos-bar) cassettes were PCR amplified using primer sets rd29A F1: PgeIF4A R1 and nos F1: bar R1 respectively (Supplementary Table 1). The PCR reaction was performed using the thermal profile: one cycle of initial denaturation at 94°C for 3 min; 35 cycles with repetition of 94°C for 1 min (denaturation), 55°C for 1 min (annealing), 72°C for 1 min (extension). The 50 μl PCR reaction mixture contained 200 ng of genomic DNA, 5 μl 10 X Taq buffer, 1 μl dNTP (10 mM), 1 μl forward primer (150 ng), 1 μl reverse primer (150 ng), 1 μl Taq (5 U) DNA polymerase enzyme and rest all make up to 50 μl with milli Q water. The amplified products were electrophoressed on 1% agarose gel and visualized on gel documentation system (G-Box Syngene, UK).
Copy Number Detection by Southern Blotting
Genomic DNA (20 μg) from control and transformed plants (T0) was digested using 30 U of Xho I (Fermentas life sciences), single cutter of T-DNA and non-cutter of PgeIF4A gene. The digested samples were size fractionated on agarose gel (0.8%) for 16 h at 30 V. The depurinated and denatured DNA fragments were subsequently transferred to positively charged Hybond N+ nylon membrane (GE Health Care, USA). Biotin labeled PgeIF4A gene was used as probe. Pre-Hybridization, washing and detection of transgene signals were carried out by following manufacturer's instructions (Biotin DNA labeling and chromogenic detection kit-Thermo Scientifics, USA). The seeds obtained from Southern positive were used for further transgene segregation analysis.
Transgene Segregation Analysis
The T1 seeds were collected from transgene integrated plants and used for segregation analysis. The goodness of fit of the ratio was tested using Chi-square test. Gene specific primers were used for the amplification of coding region of PgeIF4A. Leaves of 2-week-old plants of control and transgenic lines (T1) were dipped in PPT (5 mg/L) solution and assessed the herbicide resistance after 10 days.
Phenotypic Characterization of Transformed Seedlings (T2) through Drought and Salinity Stress Assays
Mannitol and NaCl were used for simulating drought and salt stress conditions in transgenic plants at seedling stage. T2 seeds (L1-5, L8-3, and L16-2) were placed on half strength MS medium supplemented with mannitol (0, 200, and 300 mM) and NaCl (0, 100, and 200 mM), incubated for 15 days at 25 ± 2°C under 16 h light /8 h dark photo-period. Three seedlings constituted one biological replicate. The phenotypic growth parameters like shoot length, tap root length and number of lateral roots were recorded to evaluate the effect of drought and salinity stresses on transgenic lines. All experiments were repeated thrice and the data statistically analyzed.
Leaf Disc Senescence Assay
Leaf discs (six discs per each biological sample with 1.0 cm diameter) from fully expanded leaves of 1-month-old plants (T2 plants of lines L1-5, L8-3, and L16-2) were excised using cork borer. The discs were floated on 5 ml solution of 0 (distilled water as control), 200, 300 mM mannitol and 100, 200 mM NaCl to simulate drought and salinity stress, respectively. These discs were incubated for 4 days under continuous illumination at 26°C for leaf senescence assay. Then, the leaf discs were macerated using mortar and pestle and diluted them in 80% acetone. The absorbance of the samples was recorded at A664 and A667 using spectrophotometer. The chlorophyll A, B, and total amount were calculated as described by Arnon (1949). Experiment was carried out as triplicates and the data was analyzed statistically.
RNA Isolation, cDNA Synthesis, and Quantitative Real—Time PCR
The total leaf RNA isolated using Tri-reagent (Sigma Aldrich) from the transgenic T2 lines as per the manufacturer's instructions (Sigma Aldrich). The quality and quantity of total RNA was assessed by Nano spectrophotometer (GE Health Care, USA) and RNA gel electrophoresis. The total RNA (5 μg) was reverse transcribed to cDNA using oligo dT primer as per manufacturer's instructions (iScript cDNA synthesis kit). The quantitative-real time PCR (qRT-PCR) was performed in Realplex (Eppendort, Germany) real time system. The gene specific primers and reference gene primers were designed using Primer 3.0 software to amplify the product of PgeIF4A and glucose 6 phosphate-1-dehydrogenase (G6PD, housekeeping internal groundnut gene; Reddy et al., 2013). The qRT-PCR reaction was performed in total 10 μl reaction containing 5 μl of 2X SensiFAST™ SYBR No-ROX (Bioline, UK) mix, 400 nM of each primer (Eurofins Scientifics) 1.0 μl diluted cDNA and nuclease free water to make the final volume. The thermal cycles were as follows: 95°C for 10 min followed by 40 cycles at 95°C for 15 s and 61°C for 1 min. After the qRT-PCR reaction was completed, a melting curve was generated to analyze the specificity of each gene by increasing the temperature from 60 to 95°C. The samples collected from three independent plants with three technical replicates. The threshold cycle (Ct) values were normalized using G6PD reference gene. The expression levels of PgeIF4A transcripts in different transgenic plants grown under control and stress treated were analyzed using qBase plus software (ver: 2.4; Biogazelle, Belgium; Hellemans et al., 2007).
Biochemical Characterization of Transgenic Plants Exposed to Salinity Stress
To determine the salinity induced oxidative stress, 1 month old control and transgenic plants (L1-5, L8-3, and L16-2) were exposed to NaCl (250 mM) treatment for 10 days and maintained them under controlled greenhouse conditions.
Assessment of Superoxide Dismutase (SOD) Activity
The SOD activity was assayed by monitoring the percentage of photochemical reduction of nitro bluetetrazolium (NBT) in leaf tissues according to a protocol described by Beyer and Fridovich (1987). The reaction mixture consisting of methionine (30 mg/mL), NBT (1.41 mg/mL), and triton X—100 (0.5%). To this reaction mixture, 50 μg of protein and 10 μl of riboflavin (0.44 mg/mL) were added and immediately kept under cool fluorescent light (500 μmol. m−2. s−1) to perform the reaction. The photo reduction of NBT (blue formazan production) was measured at 560 nm using spectrophotometer.
Assessment of Lipid Peroxidation through TBARS Assay
The lipid peroxidation was determined by measuring malondialdehyde (MDA) content in leaf tissues through TBARS (thio barbutaric acid reactive substances) assay. Hundred milligrams of leaf tissue was homogenized in 2 mL of 0.1% TCA and centrifuged at 10,000 rpm for about 10 min. To 1 mL of supernatant 2 mL of 20% TCA and 2 mL of 0.5% TBA was added and incubated in water bath at 95 °C for 30 min, immediately cooled on ice and then centrifuged at 10,000 rpm for 10 min. The absorbance was measured at 532 and 600 nm. The value of absorbance at 600 nm was subtracted from absorbance at 532 nm. The MDA content was calculated using extinction coefficient of 155 mM−1 cm−1 (Arnon, 1949).
Cell Membrane Stability/Electrolyte Leakage
Electrolyte leakage (EL) was determined by following the standard protocol described by Kumari et al. (2015). Briefly, the leaf discs of control and transgenics were washed thoroughly with distilled water and incubated in 20 mL of deionized water for 2 h at 25°C. Electrolyte extracts drained into medium was recorded as E1 using electro conductivity meter. Later, the leaf discs were boiled for 30 min, subsequently allowed the samples to cool down and total electrolyte leakage was recorded (E2). Similarly, the EL was measured from control plants also. Total EL (E2) was determined after autoclaving the samples. The relative electrolyte leakage was calculated as (E1/E2) × 100.
3, 3′ Diaminobenzidine (DAB) Assay
In vitro localization of H2O2 was determined by DAB assay as described by Kumari et al. (2015). Leaves of control and transgenic plants were vacuum infiltrated in 1 mg/L freshly prepared DAB solution (pH 3.8) for about 10 min at room temperature. The samples were then placed under light until dark spots appeared. The stained leaves were fixed with 3:1:1 ethanol: acetic acid: glycerol (v/v) solution.
Statistical Analysis
The experiments were designed as triplicates (three biological samples) and data analyzed statistically. To perform one-way and two-way ANOVA analysis, CoStat version 6.204 statistical program package (Cohort Software, Monterey, CA, USA) were used. One-way ANOVA was carried out to test the difference between the transgenic lines (L1-5, L8-3, and L16-2) and control. Means were compared using Tukey-Kramer test and LSD at P ≤ 0.05 (5% level). Subsequently, two-way ANOVA was used to assess treatment (T), plant lines (L), and plant lines by-treatment interactions (L × T) in two different stress treatments (Mannitol and NaCl).
Results
Cloning and In-silico Analysis of PgeIF4A Gene
The full length cDNA of PgeIF4A gene was isolated and cloned from P. glaucum using homology based cloning approach. The cloned gene sequence was deposited in the NCBI Genbank (Accession no: EU856535). The PgeIF4A gene consists of 1224 bp encoding for 407 amino acids with predicted molecular mass 45.2 kDa and isoelectric pH 6.10. The PgeIF4A protein consists of important motifs like RNA helicase/DEAD box Q-motif, DEAD box domain and RNA-helicase C-terminal (Figure 1A). These domains play major role in replication, recombination, splicing, ribosome biogenesis, RNA degradation, and repair mechanism. The domains of PgeIF4A protein were depicted in 3D modeling design. In this protein total 19 α-Helices and 13 β-sheets are present (Figure 1B). It was validated using UCSF-chimera, Rasmol, Phymol (Pettersen et al., 2004; Maheshwari and Brylinski, 2015). The phylogenetic analysis revealed that the protein belongs to the DEAD box helicase and shared highly conserved regions with Setaria italica (Figure 1C).
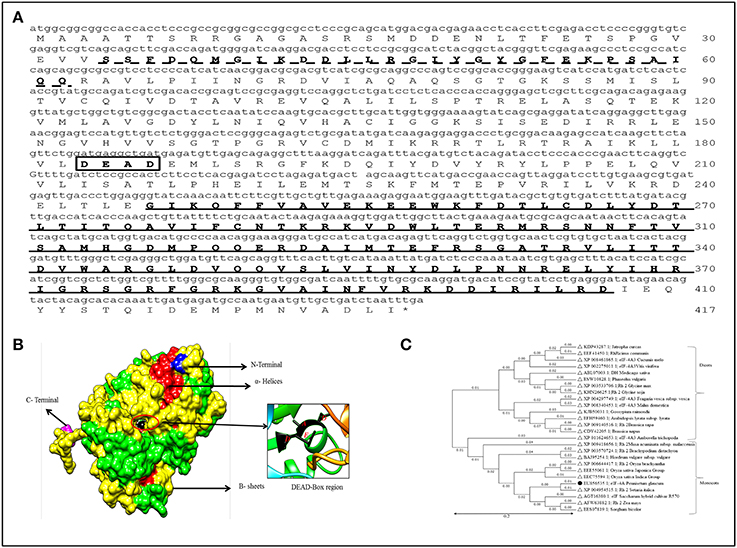
Figure 1. Sequence analysis of PgeIF4A gene and protein. (A) Schematic Representation of three motifs including RNA helicase/DEAD box Rec-Q-motif (34–62, indicated as dotted line), DEAD box domain (183–186, in box), and RNA-helicase C-terminal (246–407) in PgeIF4A protein. (B) Predicted three dimensional structure of PgeIF4A protein showing various domains in different colors, blue represented N-terminal region, magenta for C-terminal, red for α-Helices and green for β-sheets. DEAD box region highlighted in red color circle which harbor Asp-183 in β-5, Glu-184 in linked region and Ala-185, Asp-186 presented in α-9 regions. (C) Phylogenetic tree constructed based on deduced amino acid sequences of various eIF4A from closely related species. Protein names, accession numbers, and species names were indicated at each branch. The phylogenetic tree generated using Neighbor Joining (NJ) method and viewed using MEGA4 software.
Generation of PgeIF4A Expressing Groundnut Transgenic Plants
The Agrobacterium tumefaciens (LBA4404) carrying vector, pGreen0229: rd29A: PgeIF4A: poly A with nos-bar gene as selectable marker (Figure 2A) was used to infect 742 DECs (Figure 2B) prepared from matured groundnut seeds. Out of these 225 explants induced calli in the medium supplemented with phosphinothricin (PPT), BAP and 2, 4-D with a frequency of 30.3% (Figures 2C–E; Table 1). Subsequently these proliferated resistant calli differentiated into shoots in 2 weeks. About 38 green shoots from resistant calli (16.8%) differentiated into multiple shoots in 30–40 days (Figures 2F–H). The PPT resistant shoots were continuously grown and maintained on selective media. Roots generated on NAA supplemented medium. About 30 rooted plants were acclimatized under controlled greenhouse conditions (Figure 2I). The hardened plants grew normally and set flowers and pods. These plants were used for further molecular analysis.
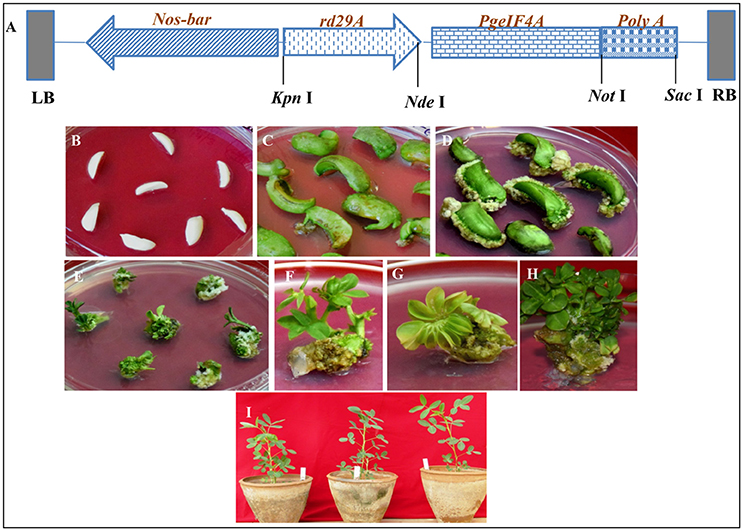
Figure 2. Schematic representation of T-DNA region of recombinant plant transformation vector pGreen0229-rd29A:PgeIF4A:poly A and groundnut transformation. (A) PgeIF4A gene was cloned under stress inducible promoter rd29A and poly A terminator, bar (Bialaphos amino transferase gene) as a selectable marker which driven by nos (nopaline synthase) promoter and nos-terminator. LB, left boarder; RB, right boarder. (B) De-embryonated half cotyledons (DEC) prepared from matured seeds. (C,D) DEC producing calli. (E) Phosphinothricin resistant calli producing shoots. (F–H) Shoot induction and multiple shoot formation. (I) Putative transgenic plants (T0) growing in glass house.

Table 1. Genetic transformation and regeneration frequency of peanut DEC explants of groundnut cv JL-24.
Molecular Confirmation of PgeIF4A Transgene Integration
The PCR was performed for detecting the presence of PgeIF4A and bar gene cassettes at T0 generation in putative transgenic plants. Out of 30 phosphinothricin resistant (T0) plants, 24 showed amplification of expected 654 bp (Figure 3A) and 429 bp (Figure 3B) fragments of gene and marker gene cassettes, respectively. The products were amplified by using rd29A F1-PgeIF4A R1 (rd29A promoter-eIF4A gene junction) and nos F1-bar R1 (nos-bar junction) primer sets (Supplementary Table 1), respectively. The primers were designed one from promoter region another from gene region to avoid non-specific amplification of products. However, control plants failed such amplification of neither of them. The transformation frequency of JL-24 transgenics was noted as 11% based on PCR results. Both PCR amplified nos-bar and rd29A-PgeIF PCR products from transgenic plants were further sequenced using Sanger's dideoxy method (Eurofins Genomics India) and annotated the sequences using multiple sequence alignment software (http://www.ebi.ac.uk/Tools/msa/clustalw2/). Both gene and marker sequences were integrated in groundnut genome exactly matched with vector T-DNA (Figures 3C,D). It indicated that the transgene was integrated in JL-24 plants.
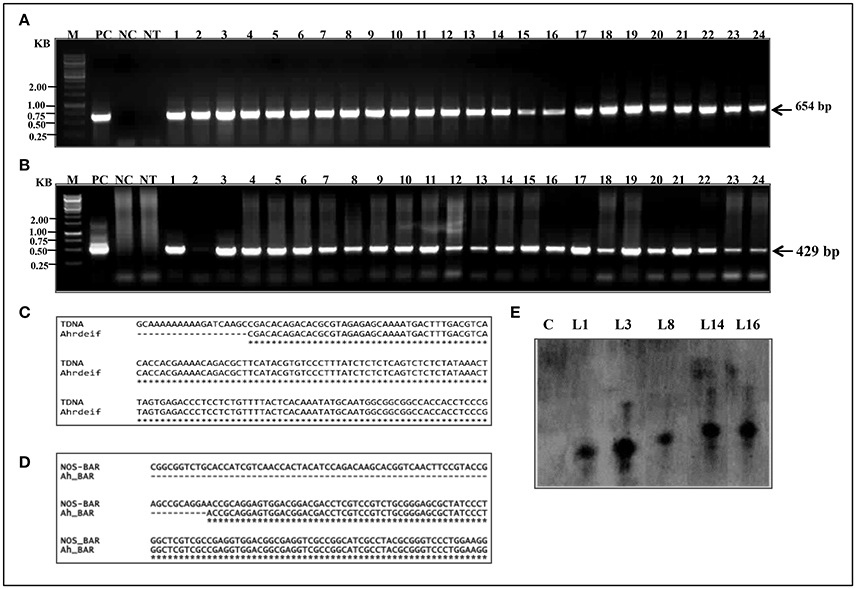
Figure 3. Molecular confirmation of putative transgenic groundnut plants (T0). (A) PCR amplification representing the junction of rd29A-PgeIF4A (654bp). (B) Junction of nos-bar marker gene. M, 1 kb marker; PC, positive control (plasmid DNA); NC, negative control; NT, non-transgenic plant; lanes 1–24, transgenic plant samples. (C,D) Alignment of nucleotide sequences of PCR amplified products Ah_rdeIF) and Ah_BAR from transgenic plants with recombinant vector pGreen0229-PgeIF4A sequence. (E) Depicted picture showing the analysis of Southern blot hybridization. The genomic DNA (20 μg) from control and transformed lines were digested with Xho I, size fractionated on 0.8% agarose gel, transferred to nylon membrane (Hybond N+), hybridized with biotin labeled eIF4A probe and detection of chromogenic signal was mediated through alkaline phosphatase reaction. Lane C, non-transgenic plant; Lane L1, L3, L8, L14, and L16 transgenic (T0) plants.
Southern Blotting Analysis and Segregation Analysis
The gene integration and copy number of PgeIF4A into genome of transgenic lines were confirmed by Southern blotting using PgeIF4A gene as a probe. Among 16 plants tested, five plants showed gene integration. In five, L1 and L8 showed single copy integration, whereas L3, L14, and L16 exhibited thick and overlapping band, which could be due to integration of two copies in these three plants (Figure 3E). However, no hybridization signal was observed in non-transgenic plants. BLASTn analysis of PgeIF4A probe sequence used in Southern hybridization did not show any similarity with Arachis hypogea native gene. It confirmed that the heterologous transgene (PgeIF4A) was integrated into groundnut transgenic plants.
T1 progeny seeds collected from single copy integrated southern positive (T0) plants were raised in glass house to determine the inheritance pattern of the transgene. The T1 progeny of L1, L8, and L16 was tested with PCR to detect the transgene. These lines exhibited the predicted Mendelian ratio as 3:1 (Figure 4A and Table 2). Simultaneously, all these T1 plants were again checked for their herbicide tolerance through PPT dip assay. The PCR positive plants showed the herbicide tolerance even after 10 days of exposure to PPT (Figure 4B). However, the control leaves were completely bleached out and wilted. It indicated that the transgenic plants expressed the bar gene and co segregated in the T1 generation which also followed the Mendelian law of segregation.
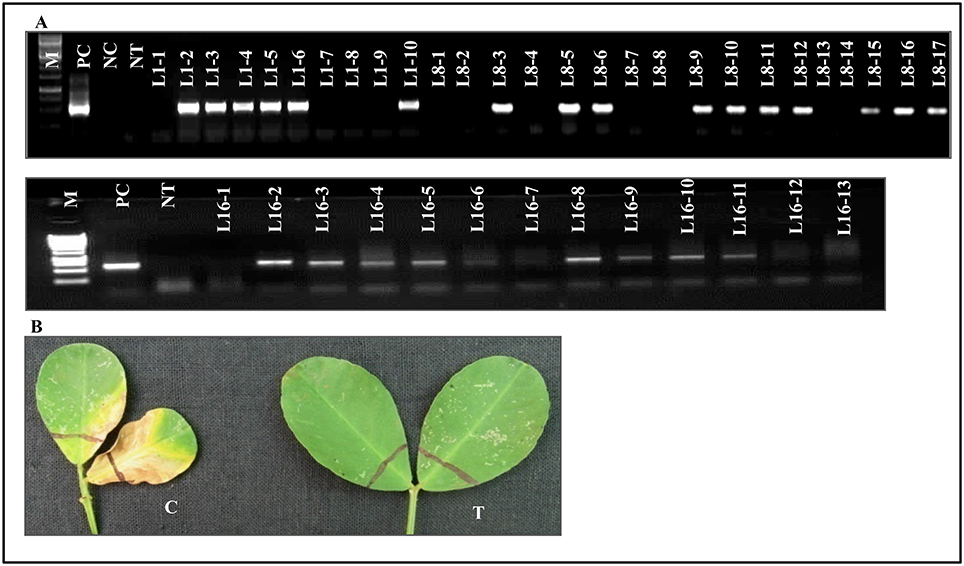
Figure 4. Transgene segregation analysis. (A) Depicted picture showing PCR amplification of eIF4A gene at T1 generation. L1-1 to L1-10, L8-1 to L8-17, and L16-1-L16-13 represents progeny of L1, L8, and L16 (T0) respectively. (B) Effect of PPT on leaves of groundnut transformants showing resistance to herbicide. C, wild type control leaf bleaching to herbicide; T, transgenic showing resistance to the herbicide.
Characterization of PgeIF4A Transgenic Plants under Simulated Drought Stress
The transformed plants germinated normally without growth penalty like control plants (Figures 5A,B). There was a significant difference in the seedling growth of the wild type and transgenic lines under stress conditions. The shoot length and tap root length of transgenics exhibited higher growth than the wild type plants. Interestingly, the tap root system of transgenics treated with mannitol developed better than the control plants grown under normal conditions at both concentrations of mannitol (200 and 300 mM; Figures 5C,D; Tables 3, 4). The lateral root formation was completely inhibited in control, whereas these roots enormously proliferated under both 200 and 300 mM mannitol concentrations in case of transgenic lines (Figure 5E; Tables 3, 4).
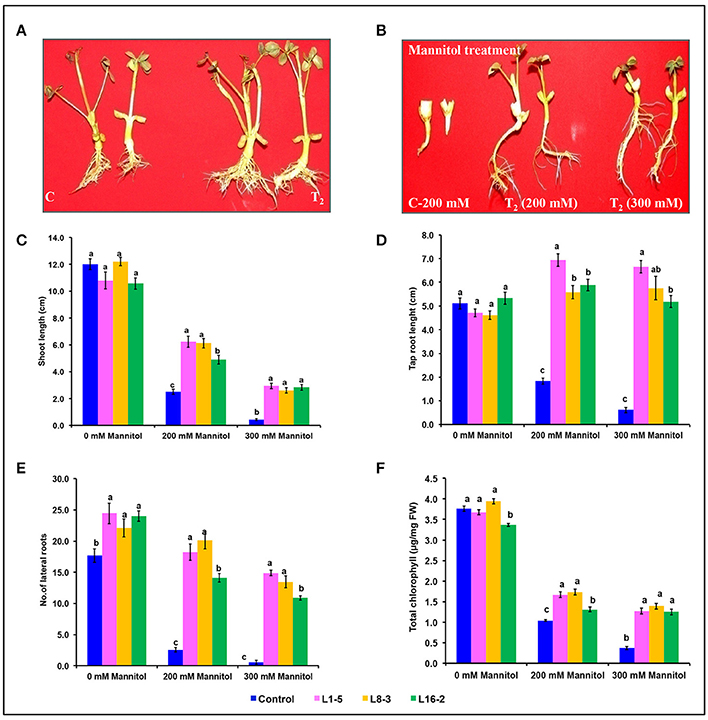
Figure 5. Characterization of eIF4A groundnut transgenic plants under simulated drought stress conditions. (A) Growth performance of PgeIF4A expressing transgenic plants (T2) and control (C) on plain MS medium. (B) Graphical representation of growth performance transgenic plants (T2) and control (C) on 200 and 300 mM mannitol. (C) Relative shoot length in cm. (D) Tap root length in cm. (E) Number of lateral roots. (F) Chlorophyll retention of transgenic and control plants grown under 0, 200, and 300 mM mannitol medium. All the experiments performed in triplicates and data represented as mean (n = three biological triplicates) using two way ANOVA with LSD and P < 0.005. Different alphabets indicated the significant differences between the treatments. Similar letters denotes non significant.
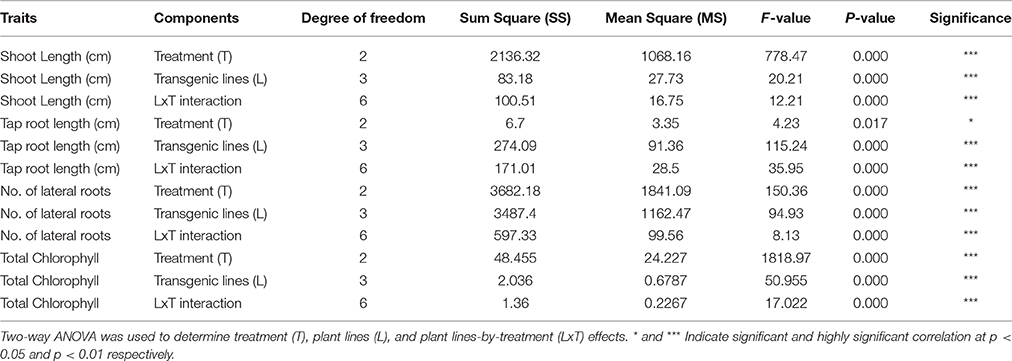
Table 3. Shoot length, tap root length, number of lateral roots, and total chlorophyll content of three transgenic plants and one control were subjected to different concentrations of mannitol stress treatments.
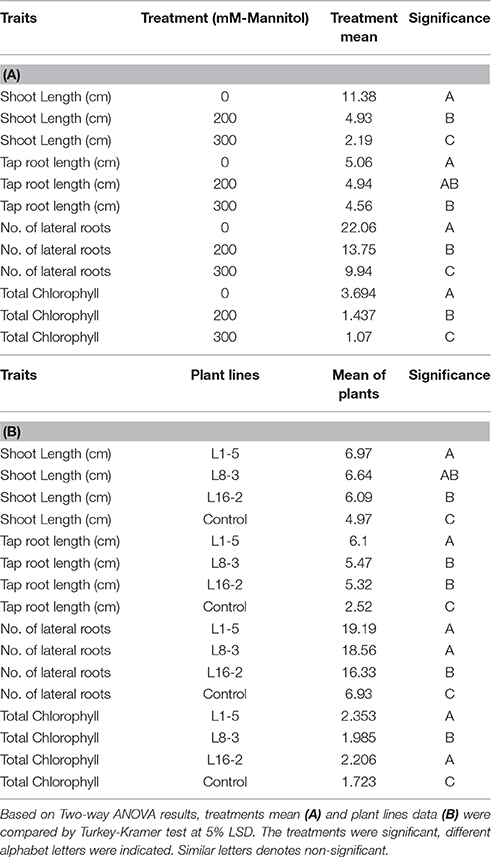
Table 4. Shoot length, tap root length, number of lateral roots, and total chlorophyll content of three transgenic plants and one control were subjected to mannitol stress treatments.
In leaf senescence assay, on 2nd day of incubation, the leaf discs from control had bleaching of the chlorophyll with increasing concentration of mannitol. However, minimal bleaching was observed in leaf discs from transgenic plants which stayed green like the controls without stress. Chlorophyll A, B and total chlorophyll content of these leaf discs after 4 days of stress imposition retained 1.9–2.3 μg/mg FW, when compared to 1.7 μg/mg FW in control. However, there was no significant difference observed in chlorophyll content, when the concentration of mannitol increased from 200 to 300 mM (Figure 5F). The results showed that stress induced expression of the transgene PgeIF4A conferred high level of drought tolerance.
Characterization of PgeIF4A Transgenic Plants under Simulated Salt Stress
The significant difference was observed in the shoot length, tap root length, and lateral root formation in wild type (C) and transgenic lines (L1-5, L8-3, and L16-2) under both concentrations of NaCl (100 and 200 mM). The shoot and tap root lengths of transgenics were noted as 10.46 and 3.48 cm compared with control plants as 6.57 and 1.8 cm, respectively, under 200 mM NaCl stress condition (Figures 6A–C). Formation of the lateral roots were significantly higher than the control under both concentrations of NaCl (Figure 6D). The growth of the transgenics at 200 mM NaCl were significantly lower for each of the line tested when compared to those at 100 mM (Figure 6; Tables 5, 6). However, the treated transgenic plants recovered very well when transferred to soil under normal growth conditions.
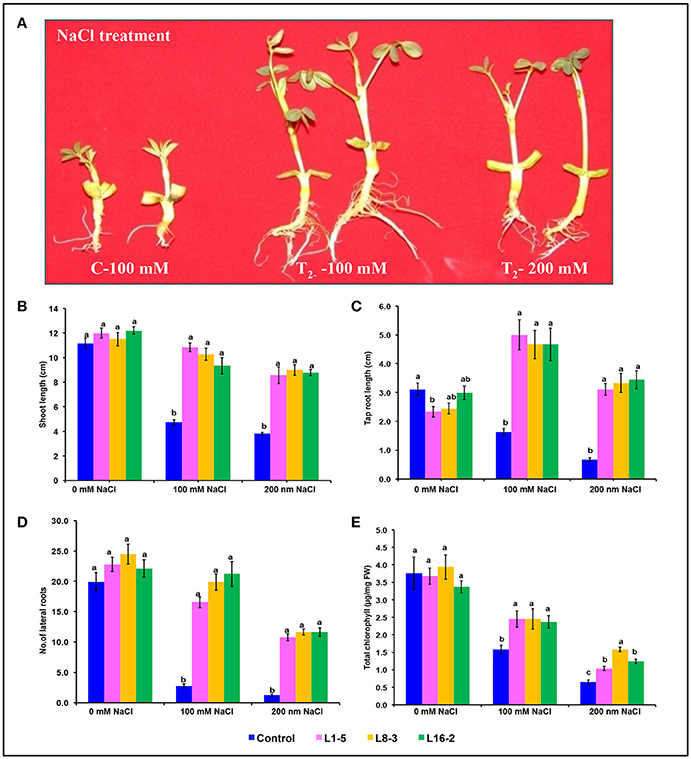
Figure 6. Characterization of PgeIF4A groundnut transgenic plants under simulated salinity stress conditions. (A) Growth performance of PgeIF4A expressing transgenic plants (T2) and control on 100 and 200 mM NaCl containing medium. (B) Graphical representation of relative shoot length in cm. (C) Tap root length in cm. (D) Number of lateral roots. (E) Chlorophyll retention of transgenic and control plants grown under 0, 100, and 200 mM NaCl medium. All the experiments performed in triplicates and data represented as mean (n = three biological triplicates) by using two way ANOVA with LSD and P < 0.005. Different alphabets indicated the significant differences between the treatments. Similar letters denotes non significant.
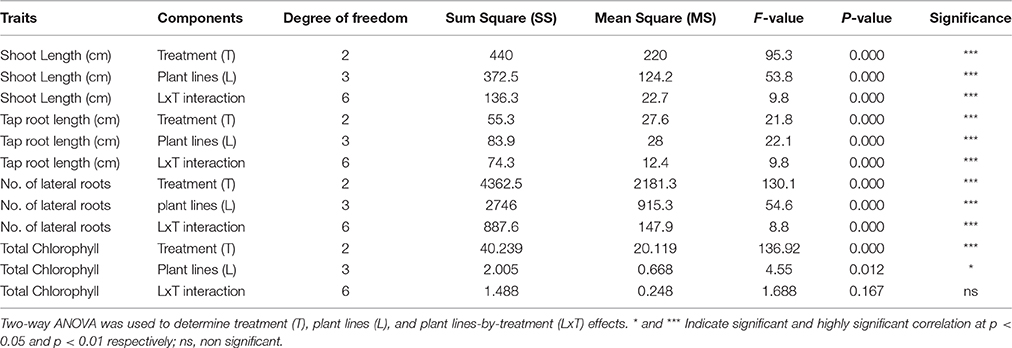
Table 5. Shoot length, tap root length, number of lateral roots, and total chlorophyll content of three transgenic plants and one control were subjected to different concentrations of NaCl (salinity) stress treatments.
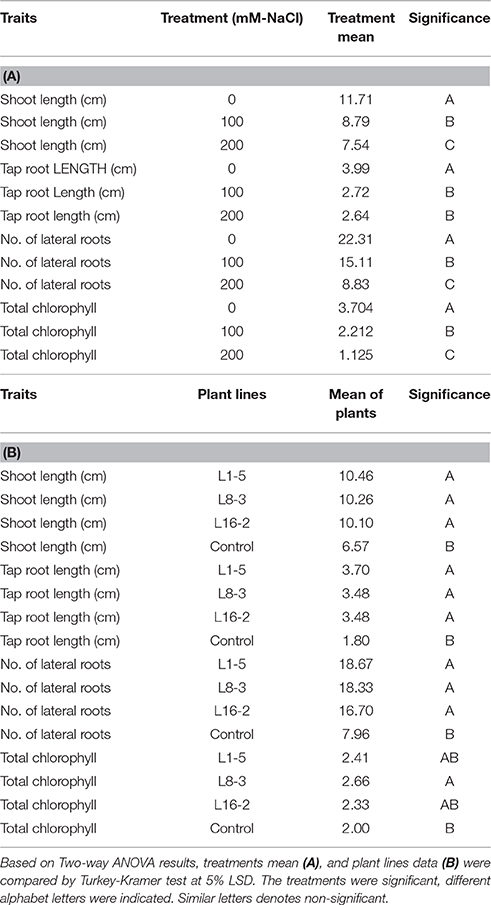
Table 6. Shoot length, tap root length, number of lateral roots, and total chlorophyll content of three transgenic plants and one control were subjected to different concentrations of NaCl (salinity) stress treatments.
In leaf senescence assay, on 2nd day of incubation, the leaf discs from control had bleaching of the chlorophyll as with increasing concentration of NaCl also. However, minimal bleaching was observed in leaf discs from transgenic plants. Total chlorophyll content of these leaf discs after 4 days of stress imposition was retained about 2.3–2.6 μg/mg FW, whereas in control it was observed 2.0 μg/mg FW (Figure 6E). As the concentration of NaCl (100–200 mM) increased the chlorophyll content decreased in accordance with phenotype observed (Figure 6E). The results showed that stress induced expression of the transgene PgeIF4A could have conferred high level of salt tolerance.
PgeIF4A Transcript Analysis by Semi Quantitative and qRT-PCR
The expression of PgeIF4A transcript was confirmed by semi quantitative PCR and qRT-PCR. The PgeIF4A transcript accumulated in homozygous T2 lines (L1-5, L8-3, and L16-2) grown under stress (mannitol and NaCl) conditions (Figure 7A). The qRT-PCR results showed that the PgeIF4A transcript could be detected in all three transgenic plants grown under normal and stress conditions (Figure 7B). The quantitative regulation of PgeIF4A in response to drought/salt stress was higher than the plants grown under normal growth conditions. The transgene expression in drought and salinity treated plants was about 4–5-folds higher than the transgenic lines grown under normal conditions (Figure 7B). It indicated that the transgene expression is positively correlated upon stress imposition.
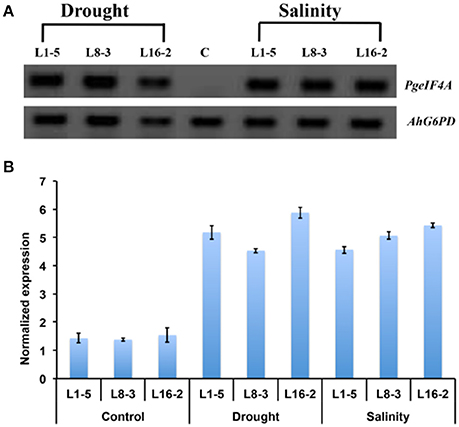
Figure 7. Expression of PgeIF4A transcript in transgenic groundnut (T2) lines. (A) Semi quantitative RT-PCR showing transcript expression pattern of PgeIF4A and G6PD (Glucose 6 phosphate 1 dehydrogenase) in control and transgenic lines grown under mannitol (drought) and NaCl (salinity) stress conditions. (B) qRT-PCR expression analysis of transgenic plants under un treated (control) and treated (drought and salinity) conditions. The Ct-values of the samples were normalized with G6PD house keeping gene. Lane C: non-transgenic, Lane: L1-5, L8-3, and L16-2 represented T2 transgenic lines.
Biochemical Analysis of Transgenic Plants under Salt Stress
The superoxide dismutase (SOD) activity in control and transgeni lines (T2) was assessed by measuring th percentage of NBT inhibition. The percentage of NBT inhibition in control was noted as 26.9% compared to the transgenic lines such as L1-5, L8-3, and L16-2 was 46.7, 33.3, and 37.2%, respectively (Figure 8A). It indicated that the superoxide dismutase accumulation was significantly increased, thery by improved the scavenging of reactive oxygen species in PgeIF4A expressing transgenic plants. The lipid peroxidation of control and transgenic lines were estimated in leaf tissues by measuring the MDA accumulation through TBARS assay. The higher MDA accumulation was observed in control (4.8 μmol/g FW) compared to transgenic lines L1-5, L8-3, and L16-2 (3.8, 3.9, and 3.4 μmol/g FW in, respectively (Figure 8B). The percentage of ion electrolyte leakage was higher in control (52.8%), compared to transgenic lines L1-5, L8-3, and L16-2 (41.6, 37.4, and 39.4% in, respectively; Figure 8C). It indicated that the electrolyte leakage in transgenics was significantly lower than the control plants, thereby enhanced the membrane stability during stress conditions. Further, 3, 3′ Diaminobenzidin (DAB) analysis detected in-situ H2O2 accumulation. H2O2 was visually detected by staining the leaves of PgeIF4A expressing transgenic and wild type plants under salinity stress (Figure 8D). The reddish brown color produced by DAB in transgenics was visually less compared to control plants, which indicated less H2O2 production in transgenic plants. Apart from these biochemical parameters, stress induced control plants showed leaf necrosis, bleaching of chlorophyll, and mortality compared to transgenic plants. The transgenic plants recovered completely (Figure 8E). The percentage of salt stress induced oxidative stress damage in L1-5, L8-3, and L16-2 lines was significantly lower than the control plants. The transgenic lines expressing PgeIF4A displayed improved membrane stability and RO scavenging capacity.
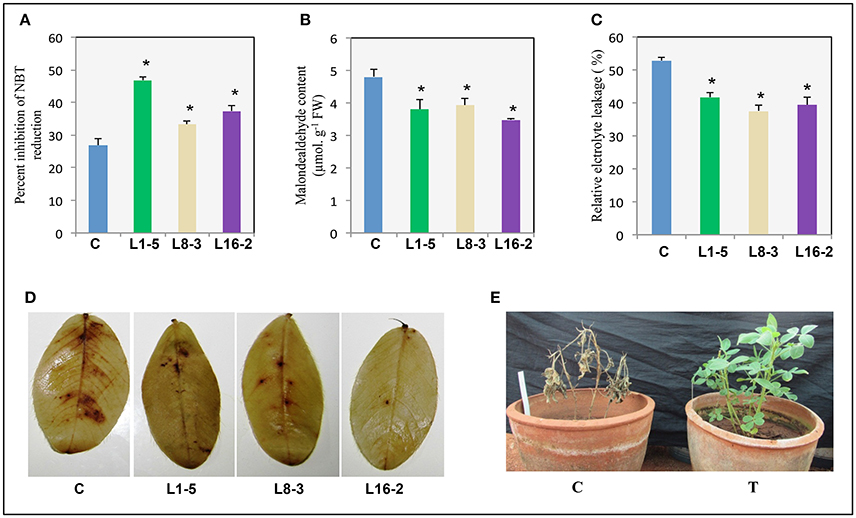
Figure 8. Response of transgenic plants expressing PgeIF4A to salinity stress. One month old control and transgenic plants were imposed with salinity stress (250 mM NaCl) for 2 weeks. (A) SOD activity (% inhibition of NBT). (B) Lipid peroxidation (malondialdehyde content). (C) Relative electrolyte leakage percentage. (D) DAB (3,3′-Diaminobenzidine) staining of WT and transgenic groundnut leaves. (E) Control (C) and PgeIF4A expressing transgenics (T) allowed to recovery after salinity treatment. Data represented as mean of ±SE (n = 3) using one way ANOVA. *Denotes significant difference between WT treated and transgenics (L1-5, L8-3, and L16-2) at p < 0.05.
Discussion
Eukaryotic translational initiation factor 4A belongs to the family of helicases, important proteins involved in several cellular and metabolic processes including abiotic stress tolerance in plants (Tuteja et al., 2014). Transgenic approaches have been exploited for expression of helicases from different species in tobacco, Arabidopsis, rice etc, conferred abiotic stress tolerance (Sanan-Mishra et al., 2005; Luo et al., 2009; Gill et al., 2013). Groundnut is one of important leguminous crops growing in semi arid and tropical regions. In current study, we have successfully developed transgenic groundnut cv JL24 by transferring PgeIF4A gene driven by stress inducible rd29A promoter. The DECs used as explants, since they were reported as explants having more regeneration capacity than any other explant in groundnut. The cut surfaces of DEC were acted as competent cells for the Agrobacterium infection and more amenable for multiple shoot proliferation from individual shoot (Tiwari and Tuli, 2012). Recovery of transformed multiple shoots was higher in DEC compared to immature embryos (Mehta et al., 2013). The putative transformed plants showed an amplification of both cassettes rd29A-PgeIF4A and nos-bar with 11% transformation efficiency. The transformation efficiency varied based on the different explants and genotypes, growth regulators used for generating transgenic plants (Mallikarjuna et al., 2016).
The transformed plants survived and displayed normal growth without any growth penalty under non stress and stress conditions. It could be due to stress inducible expression of the PgeIF4A gene in presence of rd29A promoter. The expression of OsDREB2A under the regulation of rd29A promoter conferred drought and salinity tolerance and minimized the negative growth effects under stress and non-stress condition in rice (Mallikarjuna et al., 2011). However, constitutively expressing AtDREB1A groundnut plants showed stunted growth and phenotypic abnormality when compared with those transgenics with the same gene under rd29A inducible expression (Bhatnagar-Mathur et al., 2007; Sarkar et al., 2016). Similarly, constitutive over expression of AtDREB1A gene caused growth retardation in transgenic Arabidopsis plants (Kasuga et al., 1999). The PgeIF4A expressing groundnut transgenic lines showed enhanced root and shoot growth over the control under stress conditions. Since the root system developed strongly, access to the uptake of nutrients could be increased in the plants. DREB1A expressing groundnut plants had better access of water uptake since the root system penetrated into deeper soil which resulted in significant tolerance under limited moisture conditions (Bhatnagar-Mathur et al., 2014; Sarkar et al., 2016).
The transgenic lines showed the accumulation of transgene transcript in three homozygous T2 lines grown under drought and salinity conditions. The qRT-PCR data showed that the expression of transgene was induced in three transgenic lines under drought and salt stress conditions. It could be due to the positive regulation of rd29A promoter which involved in stress inducible expression of transgene. Similarly, higher expression of AtDREB1A was seen in groundnut transgenic plants which were regulated by rd29A promoter (Sarkar et al., 2014, 2016). It indicated that there was positive correlation between regulation of transgene and stress tolerance. Similar kinds of results have been reported with mtlD, AtDREB1A expressing groundnut lines also (Bhauso et al., 2014; Sarkar et al., 2016).
The role of PgeIF4A gene at T2 generation was assessed by physiological and biochemical analysis. The number of branches and leaf area in transgenic plants increased better than control plants. Moreover, the thickness of the branches and shoots increased enormously in transformed plants. The shoot length, tap root length, and number of later roots of L1-5 and L8-3 significantly higher than L16-2 line at lower and higher concentrations of mannitol (Figures 5C–E). The shoot and taproot length of L1-5 line exhibited higher than other transgenic lines at lower and higher concentrations of mannitol and 100 mM NaCl. But at higher concentration of NaCl these lines showed similar performance (Figure 6). Interestingly, when tap root length increased, number of lateral root formation reduced under lower concentration of mannitol and NaCl. The tap root system was very well-developed with more robustness and well-adopted root hair in the transgenic plants. Moreover, the treated transgenic plants recovered very well-upon transferred them into soil.
In addition, the tap root of all transgenic lines showed higher growth under 100 mM than the plants grown under normal growth conditions. The transgenic lines L8-3 retained more chlorophyll than other two lines under both concentrations of mannitol and NaCl. However, at 200 mM NaCl there was a significant difference in chlorophyll retention between transgenic lines. The transgenic plants performed well without growth penalty which signifying that the plants were not been stunted due the regulation of stress induced rd29A promoter. The transgenic line L16-2 did not performed as like other two lines, this could be due to integration of two copies of transgenes in the genome. However, there was no much significance difference observed between transgenic lines and gene silencing was not occurred in L16-2 line. The transgenic plantlets expressing PDH45 exhibited higher survival rate, shoot and root growth in comparison with wild type plants under mannitol and salt stress conditions. The improved lateral root formation could be due to expression of related target genes under stress conditions (Manjulatha et al., 2014).
The membrane damage leads to accumulation of high MDA, electrolyte leakage and reduction in NBT inhibition levels in cells. Transgenic line L1-5 expressing PgeIF4A showed increased in NBT inhibition levels and less MDA, minimal electrolyte leakage which coupled with higher chlorophyll retention under stress conditions (Figure 8). However, there was no much significance difference in these parameters when compared with all transgenic lines. The cell membrane stability was enhanced by reducing the electrolyte leakage in transgenic plants. The transgenic plants possessed efficient anti oxidative machinery which protected the plant cells from ROS induced oxidative damage. In other studies also increase in level of oxidative markers were reported when heterologous genes expressed in groundnut such as AtNHX (Asif et al., 2011), PHD45 (Manjulatha et al., 2014), AtNAC2 (Patil et al., 2014), MuNAC4 (Pandurangaiah et al., 2014).
PgeIF4A expressing transgenic lines (T2) sensed the stress by way of coordinating with oxidative enzyme machinery. As a result high RO scavenging, more retention of chlorophyll in transgenic lines was noted and there by protected the plants by growing normally under stress and non-stress conditions. The improved JL-24 variety exhibited superior growth parameters under simulated stress condition. The plants showed tolerance to herbicide which could be additional feature to control the weeds. The eIF4A gene from P. glaucum played a key role in stress tolerance and these plants will be further validated under field conditions.
Conclusion
Groundnut cv JL-24 is an important high yielding and drought sensitive variety was used for improving drought and salt stress tolerant by transferring PgeIF4A gene through transgenic approach. In present study, three lines clearly showed drought, salt, and oxidative stress tolerance under simulated conditions. Among these lines, L1-5 exhibited super growth performance and scavenging free radicals under salt stress conditions. PgeIF4A expression in groundnut was strongly associated with enhancement of growth parameters and chlorophyll retention. This strong regulation could be due to firm regulated expression of PgeIF4A gene controlled by stress inducible rd29A promoter, which leads to the stress tolerance in subsequent generations.
Author Contributions
TS and GM: Planned and executed the experiments; JV: biochemical analysis, PS and MR: provided gene construct GM, TS, JV, and PS: Prepared manuscript.
Funding
Financial support received from Rashtriya Krishi Vikas Yojana (RKVY), Government of Andhra Pradesh.
Conflict of Interest Statement
The authors declare that the research was conducted in the absence of any commercial or financial relationships that could be construed as a potential conflict of interest.
Acknowledgments
Dr. J. S. Bentur: suggestions and corrections in MS, Prof. G. Pakki Reddy, Executive Director, ABF is specially acknowledged for his support.
Supplementary Material
The Supplementary Material for this article can be found online at: http://journal.frontiersin.org/article/10.3389/fpls.2017.00453/full#supplementary-material
References
Arnon, D. I. (1949). Copper enzymes in isolated chloroplasts. Polyphenoloxidase in Beta vulgaris. Plant Physiol. 24:1. doi: 10.1104/pp.24.1.1
Asif, M. A., Zafar, Y., Iqbal, J., Iqbal, M. M., Rashid, U., Ali, G. M., et al. (2011). Enhanced expression of AtNHX1, in transgenic groundnut (Arachis hypogaea L.) improves salt and drought tolerence. Mol. Biotechnol. 49, 250–256. doi: 10.1007/s12033-011-9399-1
Augustine, S. M., Narayan, J. A., Syamaladevi, D. P., Appunu, C., Chakravarthi, M., Ravichandran, V., et al. (2015). Overexpression of EaDREB2 and pyramiding of EaDREB2 with the pea DNA helicase gene (PDH45) enhance drought and salinity tolerance in sugarcane (Saccharum spp. hybrid). Plant Cell Rep. 34, 247–263. doi: 10.1007/s00299-014-1704-6
Beyer, W. F., and Fridovich, I. (1987). Assaying for superoxide dismutase activity: some large consequences of minor changes in conditions. Anal. Biochem. 161, 559–566. doi: 10.1016/0003-2697(87)90489-1
Bhatnagar-Mathur, P., Devi, M. J., Reddy, D. S., Lavanya, M., Vadez, V., Serraj, R., et al. (2007). Stress-inducible expression of AtDREB1A in transgenic peanut (Arachis hypogaea L.) increases transpiration efficiency under water-limiting conditions. Plant Cell Rep. 26, 2071–2082. doi: 10.1007/s00299-007-0406-8
Bhatnagar-Mathur, P., Rao, J. S., Vadez, V., Dumbala, S. R., Rathore, A., Yamaguchi-Shinozaki, K., et al. (2014). Transgenic peanut overexpressing the DREB1A transcription factor has higher yields under drought stress. Mol. Breed. 33, 327–340. doi: 10.1007/s11032-013-9952-7
Bhauso, T. D., Thankappan, R., Kumar, A., Mishra, G. P., Dobaria, J. R., and Rajam, M. V. (2014). Over-expression of bacterial mtlD gene confers enhanced tolerance to salt-stress and water-deficit stress in transgenic peanut (Arachis hypogaea) through accumulation of mannitol. Aust. J. Crop Sci. 8:413. doi: 10.1155/2014/125967
Doyle, J. J., and Doyle, J. L. (1987). A rapid DNA isolation procedure for small quantities of fresh leaf tissue. Photochembull 19, 11–15.
FAOSTAT (2014). FAO Statistics Division 2014. Available online at: http://faostat.fao.org/
Gamborg, O. L. C., Miller, R. A., and Ojima, K. (1968). Nutrient requirements of suspension cultures of soybean root cells. Exp. Cell Res. 50, 151–158. doi: 10.1016/0014-4827(68)90403-5
Gill, S. S., Tajrishi, M., Madan, M., and Tuteja, N. (2013). A DESD-box helicase functions in salinity stress tolerance by improving photosynthesis and antioxidant machinery in rice (Oryza sativa L. cv. PB1) Plant Mol. Biol. 82, 1–22. doi: 10.1007/s11103-013-0031-6
Hellemans, J., Mortier, G., De Paepe, A., Speleman, F., and Vandesompele, J. (2007). qBase relative quantification framework and software for management and automated analysis of real-time quantitative PCR data. Genome Biol. 8:R19. doi: 10.1186/gb-2007-8-2-r19
Hutchins, A. P., Roberts, G. R., Lloyd, C. W., and Doonan, J. H. (2004). In vivo interaction between CDKA and eIF4A: a possible mechanism linking translation and cell proliferation. FEBS Lett. 556, 91–94. doi: 10.1016/S0014-5793(03)01382-6
Kasuga, M., Liu, Q., Miura, S., Yamaguchi-Shinozaki, K., and Shinozaki, K. (1999). Improving plant drought, salt, and freezing tolerance by gene transfer of a single stress-inducible transcription factor. Nat. Biotechnol. 17, 287–291. doi: 10.1038/7036
Kumar, D., and Kirti, P. B. (2015). Pathogen-induced SGT1 of Arachis diogoi induces cell death and enhanced disease resistance in tobacco and peanut. Plant Biotechnol. J. 13, 73–84. doi: 10.1111/pbi.12237
Kumari, S., Joshi, R., Singh, K., Roy, S., Tripathi, A. K., Singh, P., et al. (2015). Expression of a cyclophilin OsCyp2-P isolated from a salt-tolerant landrace of rice in tobacco alleviates stress via ion homeostasis and limiting ROS accumulation. Funct. Integr. Genomics 15, 395–412. doi: 10.1007/s10142-014-0429-5
Linder, P. (2006). Dead-box proteins: a family affair—active and passive players in RNP remodeling. Nucleic Acids Res. 34, 4168–4180. doi: 10.1093/nar/gkl468
Luo, Y., Liu, Y. B., Dong, Y. X., Gao, X. Q., and Zhang, X. S. (2009). Expression of a putative alfalfa helicase increases tolerance to abiotic stress in Arabidopsis by enhancing the capacities for ROS scavenging and osmotic adjustment. J. Plant Physiol. 166, 385—394. doi: 10.1016/j.jplph.2008.06.018
Maheshwari, S., and Brylinski, M. (2015). Prediction of protein–protein interaction sites from weakly homologous template structures using meta-threading and machine learning. J. Mol. Recognit. 28, 35–48. doi: 10.1002/jmr.2410
Mallikarjuna, G., Mallikarjuna, K., Reddy, M. K., and Kaul, T. (2011). Expression of OsDREB2A transcription factor confers enhanced dehydration and salt stress tolerance in rice (Oryza sativa L.). Biotechnol. Lett. 33, 1689–1697. doi: 10.1007/s10529-011-0620-x
Mallikarjuna, G., Rao, T. S. R. B., and Kirti, P. B. (2016). Genetic engineering for peanut improvement: current status and prospects. Plant Cell Tissue Organ Cult. 125, 399–416. doi: 10.1007/s11240-016-0966-9
Manjulatha, M., Sreevathsa, R., Kumar, A. M., Sudhakar, C., Prasad, T. G., Tuteja, N., et al. (2014). Overexpression of a pea DNA helicase (PDH45) in peanut (Arachis hypogaea L.) confers improvement of cellular level tolerance and productivity under drought stress. Mol. Biotechnol. 56, 111–125. doi: 10.1007/s12033-013-9687-z
Mehta, R., Radhakrishnan, T., Kumar, A., Yadav, R., Dobaria, J. R., Thirumalaisamy, P. P., et al. (2013). Coat protein-mediated transgenic resistance of peanut (Arachis hypogaea L.) to peanut stem necrosis disease through Agrobacterium-mediated genetic transformation. Indian J. Virol. 24, 205–213. doi: 10.1007/s13337-013-0157-9
Metz, A. M., and Browning, K. S. (1993). Sequence of a cDNA encoding wheat eukaryotic protein synthesis initiation factor 4A. Gene 131, 299–300. doi: 10.1016/0378-1119(93)90310-Y
Murashige, T., and Skoog, F. (1962). A revised medium for rapid growth and bio assays with tobacco tissue cultures. Physiol. Plant. 15, 473–497. doi: 10.1111/j.1399-3054.1962.tb08052.x
Nath, M., Garg, B., Sahoo, R. K., and Tuteja, N. (2015). PDH45 overexpressing transgenic tobacco and rice plants provide salinity stress tolerance via less sodium accumulation. Plant Signal. Behav. 10:e992289. doi: 10.4161/15592324.2014.992289
Nishi, R., Kidou, S., Uchimiya, H., and Kato, A. (1993). Isolation and characterization of a rice cDNA which encodes the eukaryotic initiation factor 4A. Biochim. Biophys. Acta 1174, 293–294. doi: 10.1016/0167-4781(93)90201-N
Owtrim, G. W., Holfmann, S., and Kuhlemeir, C. (1991). Divergent genes for translation initiation factor elF-4A are coordinately expressed in tobacco. Nucleic Acids Res. 19, 5491–5496. doi: 10.1093/nar/19.20.5491
Pandurangaiah, M., Rao, G. L., Sudhakarbabu, O., Nareshkumar, A., Kiranmai, K., Lokesh, U., et al. (2014). Overexpression of horsegram (Macrotyloma uniflorum Lam. Verdc.) NAC transcriptional factor (MuNAC4) in groundnut confers enhanced drought tolerance. Mol. Biotechnol. 56, 758–769. doi: 10.1007/s12033-014-9754-0
Patil, M., Ramu, S., Jathish, P., Sreevathsa, R., Reddy, P. C., Prasad, T., et al. (2014). Overexpression of AtNAC2 (ANAC092) in groundnut (Arachis hypogaea L.) improves abiotic stress tolerance. Plant Biotechnol. Rep. 8, 161–169. doi: 10.1007/s11816-013-0305-0
Pettersen, E. F., Goddard, T. D., Huang, C. C., Couch, G. S., Greenblatt, D. M., Meng, E. C., et al. (2004). UCSF Chimera—a visualization system for exploratory research and analysis. J. Comput. Chem. 25, 1605–1612. doi: 10.1002/jcc.20084
Pham, X. H., Reddy, M. K., Ehtesham, N. Z., Matta, B., and Tuteja, N. (2000). A DNA helicase from Pisum sativum is homologous to translation initiation factor and stimulates topoisomerase I activity. Plant J. 24, 219–229. doi: 10.1046/j.1365-313x.2000.00869.x
Pruthvi, V., Narasimhan, R., and Nataraja, K. N. (2014). Simultaneous expression of abiotic stress responsive transcription factors, AtDREB2A, AtHB7 and AtABF3 improves salinity and drought tolerance in peanut (Arachis hypogaea L.). PLoS ONE 9:e111152. doi: 10.1371/journal.pone.0111152
Ramu, V. S., Swetha, T. N., Sheela, S. H., Babitha, C. K., Rohini, S., Reddy, M. K., et al. (2015). Simultaneous expression of regulatory genes associated with specific drought-adaptive traits improves drought adaptation in peanut. Plant Biotechnol. J. 14, 1008–1020. doi: 10.1111/pbi.12461
Reddy, D. S., Bhatnagar-Mathur, P., Cindhuri, K. S., and Sharma, K. K. (2013). Evaluation and validation of reference genes for normalization of quantitative real-time PCR based gene expression studies in peanut. PLoS ONE 8:e78555. doi: 10.1371/journal.pone.0078555
Rogers, G. W., Komar, A. A., and Merrick, W. C. (2002). eIF4A: the godfather of the DEAD box helicases. Prog. Nucleic Acid Res. Mol. Biol. 72, 307–331. doi: 10.1016/S0079-6603(02)72073-4
Sanan-Mishra, N., Pham, X. H., Sopory, S. K., and Tuteja, N. (2005). Pea DNA helicase 45 overexpression in tobacco confers high salinity tolerance without affecting yield. Proc. Natl. Acad. Sci. U.S.A. 102, 509–514. doi: 10.1073/pnas.0406485102
Sarkar, T., Radhakrishnan, T., Kumar, A., Mishra, G. P., and Dobaria, J. R. (2014). Heterologus expression of AtDREB1A gene in transgenic peanut conferred tolerance to drought and salinity stresses. PLoS ONE 9:e110507. doi: 10.1371/journal.pone.0110507
Sarkar, T., Thankappan, R., Kumar, A., Mishra, G. P., and Dobaria, J. R. (2016). Stress inducible expression of AtDREB1A transcription factor in transgenic peanut (Arachis hypogaea L.) conferred tolerance to soil-moisture deficit stress. Front. Plant Sci. 7:935. doi: 10.3389/fpls.2016.00935
Singh, N., Mishra, A., and Jha, B. (2014). Ectopic over-expression of peroxisomal ascorbate peroxidase (SbpAPX) gene confers salt stress tolerance in transgenic peanut (Arachis hypogaea). Gene 547, 119–125. doi: 10.1016/j.gene.2014.06.037
Tiwari, S., and Tuli, R. (2012). Optimization of factors for efficient recovery of transgenic peanut (Arachis hypogaea L.). Plant Cell Tissue Organ Cult. 109, 111–121. doi: 10.1007/s11240-011-0079-4
Tiwari, V., Chaturvedi, A. K., Mishra, A., and Jha, B. (2015). Introgression of the SbASR-1 gene cloned from a halophyte Salicornia brachiata enhances salinity and drought endurance in transgenic groundnut (Arachis hypogaea) and acts as a transcription Factor. PLoS ONE 10:e0131567. doi: 10.1371/journal.pone.0131567
Tuteja, N., Banu, M. S. A., Huda, K. M. K., Gill, S. S., Jain, P., Pham, X. H., et al. (2014). Pea p68, a DEAD-box helicase, provides salinity stress tolerance in transgenic tobacco by reducing oxidative stress and improving photosynthesis machinery. PLoS ONE 9:e98287. doi: 10.1371/journal.pone.0098287
Vashisht, A. A., Pradhan, A., Tuteja, R., and Tuteja, N. (2005). Cold-and salinity stress-induced bipolar pea DNA helicase 47 is involved in protein synthesis and stimulated by phosphorylation with protein kinase C. Plant J. 44, 76–87. doi: 10.1111/j.1365-313X.2005.02511.x
Keywords: de-embryonated cotyledons, nos-bar, rd29A, PgeIF4A, JL-24, segregation, transcript analysis
Citation: Santosh Rama Bhadra Rao T, Vijaya Naresh J, Sudhakar Reddy P, Reddy MK and Mallikarjuna G (2017) Expression of Pennisetum glaucum Eukaryotic Translational Initiation Factor 4A (PgeIF4A) Confers Improved Drought, Salinity, and Oxidative Stress Tolerance in Groundnut. Front. Plant Sci. 8:453. doi: 10.3389/fpls.2017.00453
Received: 07 December 2016; Accepted: 15 March 2017;
Published: 07 April 2017.
Edited by:
Chandrashekhar Pralhad Joshi, Michigan Technological University, USAReviewed by:
Pradeep Agarwal, Central Salt and Marine Chemicals Research Institute, IndiaSailendra Nath Sarkar, University of Calcutta, India
Copyright © 2017 Santosh Rama Bhadra Rao, Vijaya Naresh, Sudhakar Reddy, Reddy and Mallikarjuna. This is an open-access article distributed under the terms of the Creative Commons Attribution License (CC BY). The use, distribution or reproduction in other forums is permitted, provided the original author(s) or licensor are credited and that the original publication in this journal is cited, in accordance with accepted academic practice. No use, distribution or reproduction is permitted which does not comply with these terms.
*Correspondence: Garladinne Mallikarjuna, Z2FybGFkaW5uZW1hcmp1bkBnbWFpbC5jb20=