- 1Department of Mycology and Plant Pathology, Institute of Agricultural Sciences, Banaras Hindu University, Varanasi, India
- 2Institute of Environment and Sustainable Development, Banaras Hindu University, Varanasi, India
- 3Laboratory of Environmental Nanotechnology, Institute of Science and Technology of Sorocaba, São Paulo State University, São Paulo, Brazil
Nanotechnology representing a new frontier in modern agriculture is anticipated to become a major thrust in near future by offering potential applications. This integrating approach, i.e., agri-nanotechnology has great potential to cope with global challenges of food production/security, sustainability and climate change. However, despite the potential benefits of nanotechnology in agriculture so far, their relevance has not reached up to the field conditions. The elevating concerns about fate, transport, bioavailability, nanoparticles toxicity and inappropriateness of regulatory framework limit the complete acceptance and inclination to adopt nanotechnologies in agricultural sector. Moreover, the current research trends lack realistic approach that fail to attain comprehensive knowledge of risk assessment factors and further toxicity of nanoparticles toward agroecosystem components viz. plant, soil, soil microbiomes after their release into the environment. Hence in the present review we attempt to suggest certain key points to be addressed in the current and future agri-nanotechnology researches on the basis of recognized knowledge gaps with strong recommendation of incorporating biosynthesized nanoparticles to carry out analogous functions. In this perspective, the major points are as follows: (i) Mitigating risk assessment factors (responsible for fate, transport, behavior, bioavailability and toxicity) for alleviating the subsequent toxicity of nanoparticles. (ii) Optimizing permissible level of nanoparticles dose within the safety limits by performing dose dependent studies. (iii) Adopting realistic approach by designing the experiments in natural habitat and avoiding in vitro assays for accurate interpretation. (iv) Most importantly, translating environmental friendly and non-toxic biosynthesized nanoparticles from laboratory to field conditions for agricultural benefits.
Introduction
The development of nanotechnology has provided an exciting and novel frontier to nearly all fields of industrial applications with profound impact on human’s life (Linkov et al., 2011). This key enabling technology has evidenced broad and remarkable applications in diverse fields such as electronics, medicines, cosmetics, textiles, food science, energy sector and agriculture (Caruthers et al., 2007; Sharon et al., 2010; Sastry et al., 2011; NCPI, 2011). The worldwide popularity and expansion of nanotechnology industry could be anticipated by the fact that its market value will reach to US$ 75.8 Billion by 2020 due to its significant expansion at global level (Research and Markets, 2015). Indeed, the waves of nanotechnology-based researches have expeditiously contributed to global growth by delivering strong applications in many aforementioned industrial sectors. Simultaneously, it is a well-known fact that nanotechnology has tremendous potential to benefit society by revolutionizing the agricultural sector. Basically, this innovative technology has subsidized the agricultural based business sector with annual growth rate of 25% (US$ 1.08 billion). Moreover, it is estimated that integration of advanced nanotechnology in agriculture would thrust the global economic growth to ∼ US$ 3.4 trillion by 2020 (Sodano and Verneau, 2014; Sabourin and Ayande, 2015). This clearly accentuates the relevance of agri-nanotechnological researches equipped with comprehensive knowledge on its environmental impact, biosafety concerns and regulatory issues.
Nanotechnology exhibiting multidisciplinary applications is recognized as sixth most revolutionary technology in the modern era (Knell, 2010). Among the preceding revolutions introduced at different timescale, the Green revolution of 1960s and currently nanotechnology have immensely affected the agricultural field (NAAS, 2013). The Green revolution has confronted major drawbacks associated with productivity, constancy, sustainability and equity leading to urgent need of novel concepts for agricultural research and progress (Conway and Barbie, 1988). The increased dependency on chemical pesticides and fertilizers during and post Green revolution has generated serious issues related to sustainability, environmental impact and health hazards. As a result, the innovative approach of using environment friendly biofertilizers/biopesticides as alternative to agro-chemicals came in existence to ensure biosafety (Mishra et al., 2015). However, this exciting approach also comprised of some major issues of poor shelf life, their on-field stability, performance under fluctuating environmental conditions and most importantly the high required dose for maximum coverage area. Interestingly, nanoparticles based formulations have shown superiority over bioformulations in terms of confronting all these issues (Navrotsky, 2000; Auffan et al., 2009). As a result, contemporarily, the modern agriculture is embracing the innovative approach of nanotechnology to combat global challenges of crop production, food security, sustainability and climate change (Nair et al., 2010; Ghormade et al., 2011; Mishra et al., 2014a) (Figure 1). In addition to agriculture, it is important to consider that nanotechnological applications have also proved its relevance in all areas of food science including food processing, food safety through improved packaging, enhancing food nutrition and superior quality food contact materials (Amenta et al., 2015; Handford et al., 2015). However, the underexplored areas of this important aspect leading to apparent impediments, negative perceptions and hesitant adoption of nanotechnology cannot be overlooked. In this context, the present article discusses the key knowledge gaps and further highlights the promising approaches for future agri-nanotechnology researches. Moreover, considering the growing concern about nanotoxicity, we urge to recommend the agricultural application of biosynthesized nanoparticles to enhance agricultural sustainability.
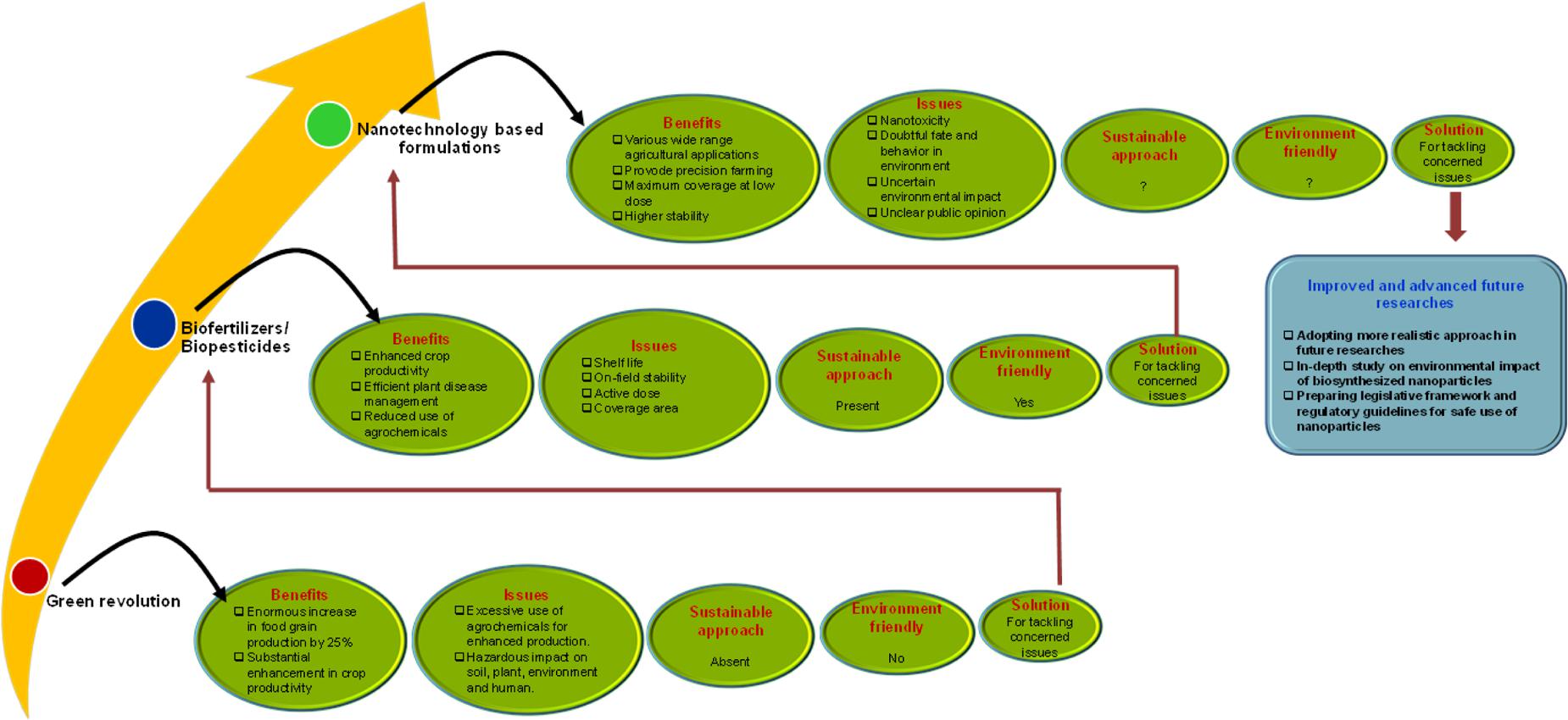
FIGURE 1. An overview of breakthrough revolutions in the field of agriculture with their respective benefits, issues and solutions. The schematic addresses the significance and superiority of nano-revolution over other two revolutions in agricultural sector.
Multifarious Applications of Nanotechnology in Agriculture and Identified Knowledge Gaps: An Overview
The potential benefits of nanotechnology in agricultural sector have created a great interest, as it can enhance agricultural productivity with low input of cost and energy. Importantly, nanotechnology by virtue of nanoparticles, has offered enormous potential applications in agriculture sector that include nanofertilizer, nanopesticide, nanoherbicide, nanosensor, and smart delivery systems for controlled release of agrochemicals (Salamanca-Buentello et al., 2005; Oliveira et al., 2014; Campos et al., 2014; Grillo et al., 2016). Additionally, nanotechnology based devices are also used for plant breeding and genetic engineering purposes (Jiang et al., 2013). The encouraging development of nanotechnological approaches in agriculture particularly for crop productivity and disease management is shown by current trends of publications and patents (Ghormade et al., 2011; Kah and Hofmann, 2014; Mishra et al., 2014b; Parisi et al., 2015; Mishra and Singh, 2016). To date, several studies have addressed how nanotechnological approach is benefiting the agricultural sector in number of ways. For examples: pesticides encapsulation in nanoparticles for their sustained release; nanoparticles mediated delivery of genetic material for crop improvement; carbon nanotube assisted seed germination of rain-fed crops; nanofertilizer for enhanced crop nutrition & crop productivity; nanopesticide for plant disease management; nanoherbicide for weed elimination and nanosensors for detection and forecast of pathogens and soil monitoring (Li et al., 2007; Barik et al., 2008; Wilson et al., 2008; Gan et al., 2010; Nair et al., 2010; Ghormade et al., 2011; Grillo et al., 2014; Mishra et al., 2014b; Oliveira et al., 2014; Campos et al., 2015a,b; Liu and Lal, 2015; Oliveira H. C et al., 2015; Oliveira J. L et al., 2015; Maruyama et al., 2016). Besides these, the probable impact of nanomaterials exposure on fate and accumulation of other organic and inorganic co-contaminants (being added to agricultural system such as pesticides, fertilizers, heavy metals etc.) is the recent development (Servin and White, 2016). Earlier report by De La Torre-Roche et al. (2013) suggest reduced uptake of pesticides by plants due to their association with carbon nanomaterials. This finding indicates beneficial impact of such interactions on reducing the pesticides residues in plants and edible parts. Given the importance to this aspect, such kind of studies must be promoted to remove the agricultural contaminants such as heavy metals and agrochemicals.
Undoubtedly, nanotechnology provides the possibility of precision farming (i.e., augmenting agricultural production with minimum input) in the era where elevating demand of sustainability compels to reduce the cost and excessive use of agricultural and natural resources (Chen and Yada, 2011). However, despite the exciting results obtained by involvement of ground-breaking nanotechnology in agriculture so far, their relevance have not yet reached up to the market. This is mainly attributed to small scale bench-top researches, ambiguous technical benefits, insufficient economic interest, biosafety concerns, regulatory issues and public opinion (Parisi et al., 2015). Additionally, the hovering apprehensions about fate, transport, bioavailability and toxicity of nanoparticles, limit the complete acceptance and willingness to adopt nanotechnology in agricultural sector. Nevertheless, nanotechnology renders precise capability to revolutionize the agricultural sector but at the same time it is also important to note that its concrete contribution to agriculture is still uncertain and at its nascent stage. Therefore, we need to include a system level approach providing more accurate informations on nanoparticles exposure and their risk in agricultural systems. In response to this, we highlight the future directions for improved agri-nanotechnology researches with special emphasis on; (i) optimization of the safe use of nanoparticles at permissible level for agricultural benefits by modulating the fate, behavior, bioavailability and toxicity determining factors (ii) advancement in experimental design and, (iii) incorporation of biosynthesized nanoparticles and assessing their relative advantages over nanoparticles from non-biological sources.
Concerning Risk Assessment Factors and Their Modulation for Safe Use of Nanomaterials in Agriculture
Undoubtedly, the current scenario is witnessing the successful advancement and remodeling of agricultural sector due to captivating scientific applications of nanotechnology. Inevitably, such advancement is urgently required to boost the agricultural production in order to feed growing global population, which is likely to reach 9 billion by 2050 (Chen and Yada, 2011). Considering the expected benefits of nanotechnology-based products in agriculture in near future, several countries across the world are giving considerable efforts in evaluating the suitability and compatibility of integration of nanotechnology with agriculture. In this regard, agricultural scientists are trying to fill the knowledge gaps regarding toxicity of nanoparticles toward agro-ecosystem components mainly plant, soil and soil biota. As soon as nanoparticles are released in the environment, they are subjected to the possible interactions with these agro-ecosystem components (Anjum et al., 2013; Mishra and Singh, 2015a). Therefore, researchers are taking efforts to understand and scrutinize the extent of these major interactions in order to gain functional knowledge about toxicity and probable impact of released nanoparticles on environment and agriculture. Moreover, such investigations would primarily contribute to determine the permissible level of nanoparticles within tolerable safety limits.
The plant-soil interaction is the main driving force for agricultural production, which is influenced by any alteration in physico-chemical properties of soil system. Notably, soil is actually the paramount sink of released nanoparticles and hence, their subsequent interaction with different soil components could have profound impact on the fate, transport and behavior of nanoparticles. For instance, few reports on the most popular and most studied silver nanoparticles (AgNPs) possessing antimicrobial property have clearly indicated the importance of soil pH, organic matter content and cation exchange capacity in controlling their fate, toxicity and bioavailability (Jacobson et al., 2005; Shoults-Wilson et al., 2011; Benoit et al., 2013). It has been observed that lower range of soil pH, organic matter content and cation exchange capacity obstructs sorption of Ag to soil resulting into enhanced risk of mobility, toxicity and bioavailability of AgNPs. In contrary, higher range of soil pH, organic matter content and cation exchange capacity facilitates Ag sorption to soil that prevent mobility, bioavailability and further toxicity of AgNPs (Shoults-Wilson et al., 2011; Benoit et al., 2013; Mishra and Singh, 2015a; Klitzke et al., 2015; Schlich and Hund-Rinke, 2015). Likewise, Wang P. et al. (2013) made comparative analysis of ZnO-NPs toxicity in solution culture and soil system. The authors advocated substantial reduction in the toxicity of ZnO-NPs in soil system. This is mainly attributed to a range of soil characteristics (pH and cation exchange capacity) which determine phytotoxicity of Zn in soil system while on other hand particle dissolution in solution culture caused more toxicity. Furthermore, loamy sand soil with pH 5.5 was reported to exhibit no phytotoxicity of ZnO-NPs at concentration of 2000 mg kg-1 toward Cucumis sativus (Kim et al., 2011) whereas loamy clay soil with pH 7.36 showed noticeable toxicity toward Triticum aestivum at concentration of 45.45 mg kg-1 (Du et al., 2011). Additionally, soil organic matter is considered as another important key factor influencing transport behavior of ZnO-NPs that eventually determines their further toxicity. Zhao et al. (2013) revealed positive effect of alginate in reducing the toxicity of ZnO-NPs toward Zea mays. The ZnO-NPs added to soil together with alginate at concentration of 400–800 mg kg-1 exhibited no reduction in plant biomass whereas significant reduction was observed without alginate. As evident from these findings that soil environment can assess the potential environmental risk of nanoparticles and therefore we should recommend soil ecotoxicity studies with nanoparticles to predict their long term maximum effects.
As noted above, nanoparticles introduced in the environment ultimately accumulate in the soil and their fate, transport and behavior is largely affected by soil characteristics. In addition to this, most of the exisiting literatures have also focused on determining the direct impact of released nanoparticles on soil microbial community structure (Hänsch and Emmerling, 2010; He et al., 2011; Simonin and Richaume, 2015). In this regard, initially, Ge et al. (2012) envisaged potential effect of TiO2 and ZnO-NPs on soil bacterial community in a dose dependent manner. Using DNA-based fingerprinting analysis, they observed reduced bacterial diversity with declining taxa of Rhizobiales, Bradyrhizobiaceae, and Bradyrhizobium (related to nitrogen fixation) in response to these nanoparticles treatment. However, some positive impact was also observed on Sphingomonadaceae and Streptomycetaceae. It is interesting to note here that TiO2 and ZnO-NPs have significantly altered the bacterial community structure with distinct impact on environmental processes. For instance, the declining taxa are closely associated with nitrogen fixation process whereas increasing taxa are likely to affect the decomposition process of organic pollutants and biopolymers. Further, Shahrokh et al. (2014) also revealed dose dependent effect of AgNPs on nitrate reductase activity of Rhizobium and Azotobacter, where low dose of AgNPs (0.2 ppm) facilitated nitrate reduction activity in Azotobacter. Based on findings of such studies it has been anticipated that the denitrifying bacterial community is assumed to be highly susceptible to nanoparticles toxicity (Throbäck et al., 2007; VandeVoort and Arai, 2012). Despite the clearly known impact of nanoparticles on soil microbial community, there exists a dearth of literature providing apparent connection between soil factors and toxic behavior of nanoparticles toward soil biota (Calder et al., 2012; Chunjaturas et al., 2014; Shah et al., 2014; Mishra and Singh, 2015a). In this context, Frenk et al. (2013) evidenced effect of copper oxide (CuO) and magnetite (Fe3O4) nanoparticles on soil bacterial community in two different soil types (sandy loam and sandy clay loam). Interestingly, more adverse effects of both nanoparticles were detected in sandy loam soil with CuO exhibiting relatively strong influence on community composition and bacterial activity. More specifically, Rhizobiales and Sphingobacteriaceae being the most targeted taxa, were negatively affected by CuO in sandy loam soil. However, limited effects were also noticed in sandy clay loam soil where 1% CuO decreased community composition and oxidative potential but on other side, Fe3O4 nanoparticles did not change the bacterial community structure. Based on this finding, it is worth mentioning here that occurrence of clay part in the soil actually contributed to diminished toxicity of nanoparticles (Schlich and Hund-Rinke, 2015). Most recently, Shen et al. (2015) demonstrated ecotoxicological effects of ZnO-NPs on soil microbes on the basis of parameters including ammonification, respiration, dehydrogenase activity and fluorescent diacetate hydrolase activity. The adverse effects of ZnO-NPs on soil microbes under microcosm set up were found to be more pronounced in acidic and neutral soil, while alkaline soil possessed relatively low toxicity. Similar to this, toxicity of TiO2 NPs was found to be mainly influenced by soil pH and organic matter as reported by Simonin et al. (2015). The authors noticed significant reduction on carbon mineralization (parameter to study microbial community) in soil with high pH and organic matter content. Evidently, these findings highlight the significance of identifying the major soil variables such as soil type, soil organic matter content and soil moisture while evaluating the toxicity of nanoparticles toward microbes in soil habitat. Most importantly, gaining precise knowledge on the probable impact of different soil properties on the toxicity behavior of nanomaterials would help in evading the release of nanoparticles in the soil environment favoring its toxicity. Moreover, adopting improved soil management practices such as mulching, enhancing soil organic matter content in order to reduce probable chances of nanoparticles toxicity would also be beneficial.
Phytotoxicity of nanoparticles is another prevalent topic of discussion as plants have plentiful opportunity to interact with nanoparticles due to large surface area of leaves and root system. In addition, risk of nanoparticles toxicity is higher in plants due to their miniscule size that can easily translocate within plant body. It is believed that nanoparticles enter within the plant body through surface adsorption or traversing through small openings of the plants (Dietz and Herth, 2011). The available literatures clearly point toward the all possible impact (positive, negative and neutral effects) of nanoparticles on plant system (Stampoulis et al., 2009; Khodakovskaya et al., 2009; Su et al., 2009; Lee et al., 2010; Cifuentes et al., 2010; Zhang et al., 2012). It is important to consider that phytotoxicity of nanoparticles is primarily dependent on their size and concentration. Accordingly, Ma et al. (2010) reviewed that nanoparticles of size less than 5 nm can easily be translocated through the cell wall pores while nanoparticles of size upto 20 nm moves through plasmodesmata. Moreover, small sized nanoparticles may cause phytotoxicity even at lower concentration owing to its easy uptake by the plants and their further translocation inside plant system (Rico et al., 2011; Wang J. et al., 2013). In case of the most common metallic nanoparticles i.e. AgNPs, nanoparticles size is considered to play a key role in their phytotoxicity behavior. It has been observed that small sized AgNPs in the range of 5-10 nm possess higher toxicity (Rico et al., 2011; Qian et al., 2013; Vannini et al., 2014).
Another study by Asli and Neumann (2009) reported mechanical mode of inhibition of TiO2-NPs (30 nm) treatment on hydraulic conductivity and transpiration rate of Z. mays grown in hydroponic solution, by decreasing cell wall pore size of root from 6.6 to 3 nm while, no significant inhibitory effect was observed in soil grown plants. In contrast, stimulating impact of TiO2-NPs (2.5 g L-1) application on fresh and dry weight of Spinacia oleracea has been noticed by Yang et al. (2007). In addition, this elevated response was also observed in case of chlorophyll, protein and total nitrogen content in leaves. The authors related the stimulating impact to improved nitrogen photoreduction where treatment of TiO2-NPs on exposure to sunlight enhanced reduction of N2 to NH3 in plants grown in nitrogen deficient solution. Similarly, Song et al. (2012) also reported positive impact on duckweed (Lemna minor) grown in culture media supplemented with TiO2-NPs at very low concentration of 0.5 g L-1 whereas, higher concentration caused significant damage to the plants. The ZnO-NPs being the most widely used metal oxide NPs, have also been reported to enhance the growth of mung (Vigna radiata) and chickpea (Cicer arietinum) grown on plant agar media at concentration of 20 mg L-1 and 1 mg L-1, respectively (Mahajan et al., 2011). Interestingly, Zhao et al. (2013) evidenced plant growth promoting effect of ZnO-NPs on Cucumis sativus grown in soil system at concentration of 400 and 800 mg kg-1. However, higher dose of ZnO-NPs beyond this concentration limit caused phytotoxic effects. Similar dose dependent effect has been observed in case of Cu-NPs that noticeably inhibited the growth of wheat (T. aestivum) and mung bean (Phaseolus radiatus) at concentration of 570 and 335 mg L-1 respectively. For homogenous exposure of nanoparticles suspension to the plants and to avert the possibility of precipitation, this test was conducted in plant agar media (Lee et al., 2008). Likewise, higher concentration of 1000 mg L-1 of Cu-NPs prominently reduced the growth of zucchini (Cucurbita pepo cv costata romanesco) grown in Hoagland solution (Stampoulis et al., 2009). On the contrary, it is worth mentioning here that stimulating impact was observed in lettuce (Lactuca sativa) plants grown in soil amended with 130 and 600 mg kg-1 of Cu-NPs (Shah and Belozerova, 2009). Typically, other type of nanomaterials, e.g., multi-walled carbon nanotubes (CNT) were also reported to enhance seed germination and root elongation of ryegrass at concentration of 2 g L-1, while no significant effects were noted on radish, lettuce, cucumber seeds (Lin and Xing, 2007). Likewise, application of 50 mg L-1 CNTs has also been indicated to enhance the yield of tomato by improving water use efficiency of plants (Khodakovskaya et al., 2013). Altogether, these studies strongly highlight the point that phytotoxic behavior of nanomaterials is largely dependent on concentration and plant growth system. Considering the relevance of nano-phytotoxicity in agro-ecosystem, it should be noted that soil is the main route through which plants are largely exposed to the released nanoparticles. Hence, pointing to this fact, more realistic approach must be incorporated in our experimental design to gain appropriate knowledge about the fate and risk of nanoparticles toxicity toward plants. Therefore, many researchers have started to consider this approach by avoiding hydroponics system so as to get more relevant toxicity data and unambiguous interpretation. With reference to this, previously, Zhu et al. (2008) interpreted nanofilter capability of soil system as it prevented uptake of Fe3O4-NPs by Cucurbita maxima, while 1.3% of 0.5 g L-1 Fe3O4-NPs (20 nm) was traced to translocate to the leaves when the plants were grown in hydroponics growth system. Likewise, CeO2 nanoparticles (37 nm) when applied to soil at 10 μg g-1 through 14 days irrigation, yielded neutral effect on maize plants because neither noticeable uptake nor any kind of growth inhibition was recorded (Birbaum et al., 2010). Additionally, Lee et al. (2012) also evidenced low toxicity of AgNPs toward Phaseolus radiatus and Sorghum bicolor grown in soil system as compared to agar. Most importantly, the response of plants to nanoparticles released in soil system is largely governed by soil parameters but unfortunately, there is lack of research on this aspect. However, only few studies have investigated this approach, for example, Jośko and Oleszczuk (2013) demonstrated non-toxic effect of ZnO-NPs toward Lepidium sativum grown in soil with higher level of cation exchange capacity. Likewise, Dimkpa et al. (2012) observed higher toxicity of CuO and ZnO nanoparticles toward T. aestivum grown in sandy soil. Based on these findings, there is clear evidence that soil parameters can modulate the probable phytotoxicity of nanoparticles. Therefore, considerable efforts must be undertaken in this direction for gaining detailed understanding of multitude of soil components affecting the plant-nanoparticles interaction in soil and for making reliable estimate of phyto-nanotoxicity.
In light of the above-mentioned reports, we assume that an understanding of the environmental impact of nanoparticles released in agro-ecosystem must include the analysis of basic risk assessment factors during the tripartite interactions of nanoparticles with plant, soil, and soil microbial community (Table 1). Indeed, the key soil factors mainly soil type, pH, organic matter content, soil moisture, govern the behavior, and toxicity of released nanoparticles toward plants and microbes. However, on other side, phytotoxicity of nanoparticles is largely regulated by their size, concentration and plant growth system. Here, the important point is to mitigate these risk assessment factors for alleviating the subsequent toxicity of nanoparticles.
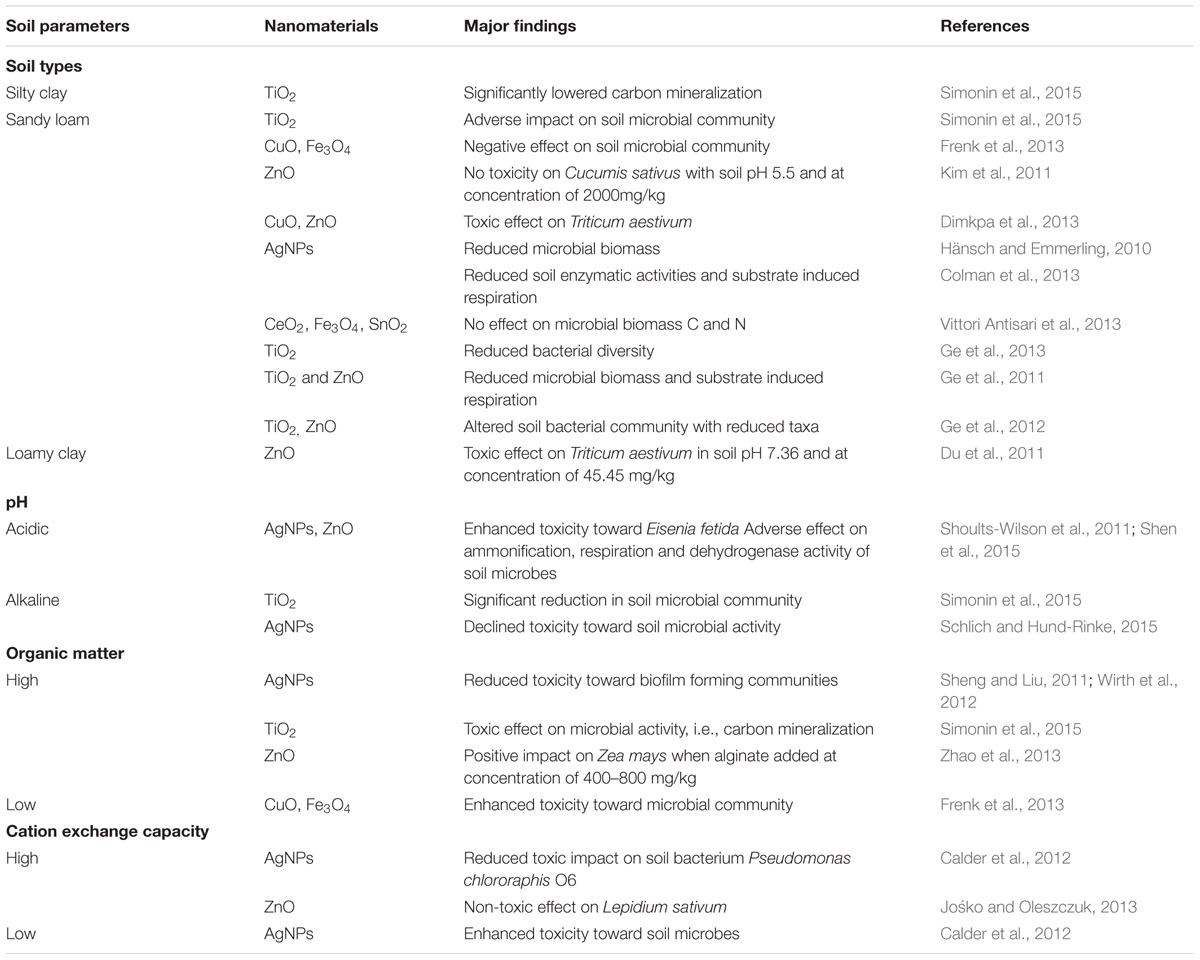
TABLE 1. Review of the possible interactions and impact of nanomaterials on soil microbes and plant under varying soil physico-chemical properties.
Comprehensive Understanding of the Interactions, Toxicity, and Fate of Biosynthesized Nanoparticles
The rapid development of nanotechnology has raised several issues of which synthesis protocols are gaining considerable attention. In general, a variety of physical and chemical protocols viz. laser pyrolysis or ablation, micro-emulsion sol-gel, ultrasonic fields, UV irradiation photochemical, reduction techniques etc. have been successfully employed for nanoparticles synthesis (Sastry et al., 2004; Yang and Aoki, 2005; Aslan et al., 2006; Cao and Hu, 2009). However, feasibility of these protocols is still ambiguous and contentious due to environmental risks of toxic and hazardous chemicals used for synthesis purpose (Li et al., 2011; Singh et al., 2016). Therefore, opting ecofriendly, non-toxic and sustainable methods for fabricating a myriad of nanoparticles is the current area of global interest. In this way, several biological agents such as bacteria, fungi, actinomycetes, plants and algae have been exploited for biosynthesis of nanoparticles (Ahmad et al., 2003; Singaravelu et al., 2007; Li et al., 2011; Mittal et al., 2013; Mishra et al., 2014b). The superiority of biological method for nanoparticles synthesis could be estimated by the fact that the whole process of synthesis is rapid, stable and requires a wide range of non-toxic biomolecules of low cost and most importantly provides more stable nanoparticles (Singh et al., 2016; Hussain et al., 2016). Moreover, shape and size of the nanoparticles can also be regulated by modifying the pH and temperature of the reaction mixture (Gericke and Pinches, 2006). Thus, several metal nanoparticles (Au, Ag, Fe, Pt, Ti, Zn, Mg etc.) have been successfully fabricated using biosynthetic approach (Kharissova et al., 2013). Interestingly, biosynthesized nanoparticles are found to show improved activity in comparison to those synthesized by physical and chemical methods (Sintubin et al., 2011). However, besides these advantages, the major concern associated with biological synthesis approach is the polydispersity of synthesized nanoparticles. But by optimizing the synthesizing conditions such as pH, temperature, salt concentration; the shape, size and dispersity of nanoparticles can be controlled (Pimprikar et al., 2009; Iravani et al., 2014).
In relation to agricultural perspective, biosynthesized nanoparticles offer efficient and environment-friendly applications particularly for plant growth promotion, plant disease management and stress tolerance (Mishra et al., 2016). In this context, Raliya et al. (2015) found stimulating impact of biosynthesized TiO2 nanoparticles using Aspergillus flavus on plant growth of Vigna radiata and rhizospheric microbial population. Likewise, Mishra et al. (2014b) reported strong antifungal activity of biosynthesized silver nanoparticles (AgNPs) against Bipolaris sorokiniana, spot blotch pathogen of wheat (T. aestivum). Additionally, biosynthesized AgNPs using Serratia sp. BHU-S4 were also found to inhibit melanin biosynthesis genes in B. sorokiniana (Mishra and Singh, 2015b). Apart from this, many earlier studies have confirmed the antimicrobial activity of biosynthesized AgNPs against broad range of phytopathogens pointing toward their exciting possibilities in agriculture (Krishnaraj et al., 2012; Gopinath and Velusamy, 2013; Lee et al., 2013; Paulkumar et al., 2014). Furthermore, Raliya et al. (2014) demonstrated positive effect of biosynthesized MgO nanoparticles using Aspergillus flavus on clusterbean (Cyamopsis tetragonoloba). Application of these nanoparticles at concentration of 15 mg L-1 resulted into enhanced root-shoot growth and chlorophyll pigment in clusterbean. Additionally, ZnO nanoparticles synthesized from Aspergillus fumigatus, showed significant improvement in overall plant health along with enhancement in rhizosphere microbial population, acid phosphatase, alkaline phosphatase and phytase activity in clusterbean rhizosphere (Raliya and Tarafdar, 2013). Correspondingly, Sabir et al. (2014) also proposed that application of ZnO-NPs can revolutionize the agricultural sector and could solve the current problem of food demand due to their antimicrobial and fertilizer action, especially if considered biogenic synthesis of these nanoparticles. The above-mentioned studies confirmed that agricultural applications of biosynthesized nanoparticles provide new insight on precision farming technology. Moreover, there is a growing interest in studying the fate, transport and toxicity of biosynthesized nanoparticles and hence more attention should be given in this direction.
Biosynthesis routes employ biological materials such as plant extracts, sugars, polyphenols, vitamins and microorganisms which are used as reducing and capping agents in synthesis process leading to more stabilized and biocompatible nanoparticles with higher longevity (Parsons et al., 2007; Kalaiarasi et al., 2010; Kharissova et al., 2013). Most importantly, the biofabricated nanoparticles exhibit relatively lower toxicity compared to chemically produced nanoparticles (Sanchez-Mendieta and Vilchis-Nestor, 2012; Varma, 2012; Órdenes-Aenishanslins et al., 2014). Consequently, with the growing public concern on the nanotoxicity and its direct or indirect environmental impact, considerable attention is required for employing biosynthesized nanoparticles for agricultural purposes. However, there is complete lack of studies aimed at toxicity, associated risk factors and environmental impact of biosynthesized nanoparticles. Furthermore, there is enormous scope of research in this underexplored, emerging and challenging area and hence, considerable efforts must be devoted to in-depth study on environmental impact of biosynthesized nanoparticles. Keeping this view in mind, we believe that meticulous application of biosynthesized nanoformulations in agricultural system would eventually remove its negative perception.
Regulatory Policies and Considerations
Regardless of the promising development of nanotechnology in varied fields, its agricultural applications have not been translated to meet global needs primarily due to shallow awareness and biosafety concerns. The foremost reason for scarcity of commercial development of nanotechnology in agriculture is geographically limited existence of legislative framework, regulatory guidelines and negative public opinion (Arts et al., 2014). The growing challenge of global food security and climate change strongly underline the commercial applications of nanotechnology-based products for agricultural sector (Rossi et al., 2014). Therefore, there is an urgent need to thoroughly assess the risk assessment and risk management factors associated with application of nanoparticles in agricultural sector, prior to implementation of regulatory guidelines (FAO/WHO, 2013; Amenta et al., 2015). In this context, several regulatory bodies viz. US Food and Drug Administration (USFDA), Organisation for Economic Cooperation and Development (OECD), International Standard Organization (ISO) are undertaking challenges in this direction thereby agencies including USFDA specifically enforces legislation on soil while, ISO and OECD only provide guidelines and suggestions to the regulatory bodies. Different approaches are being followed in OECD and non-OECD countries in regulating nanotechnology in agri/feed/food sectors (Amenta et al., 2015). The main EU regulation is the REACH (Registration, Evaluation, Authorisation and Restriction of Chemicals) which chiefly addresses the use of nanomaterials in plant protection products, food additives/supplements and food contact materials (European Commission, 2013). Globally, only EU and Switzerland have successfully established nano-specific legislative provisions particularly for agriculture, food and feed sector whereas, other non-EU countries have non-mandatory frameworks binding with non-legal guidance (OECD, 2013). However, it is important to note that due to uncertainty of regulatory frameworks and difference in opinion across the globe, the nanoparticles based products for agricultural benefits are not flourishing and facing difficulties in reaching to the market. Risk assessment and risk management are the top most priorities to be considered in framing regulatory policies for addressing biosafety issues. Moreover, sharing views and opinion on public platform across the globe would be helpful in dealing with efficient regulatory measures.
Recommendations for Future Research
Promising applications of nanotechnology in all areas cannot be overlooked. However, simultaneously uncertainty and negative perception vis-à-vis nanotechnological interventions in agricultural sector must be taken seriously. Hence, there is need to make extensive efforts in forwarding and improving the futuristic researches based on recognized knowledge gaps (Figure 2). In this context, we suggest the following key points:
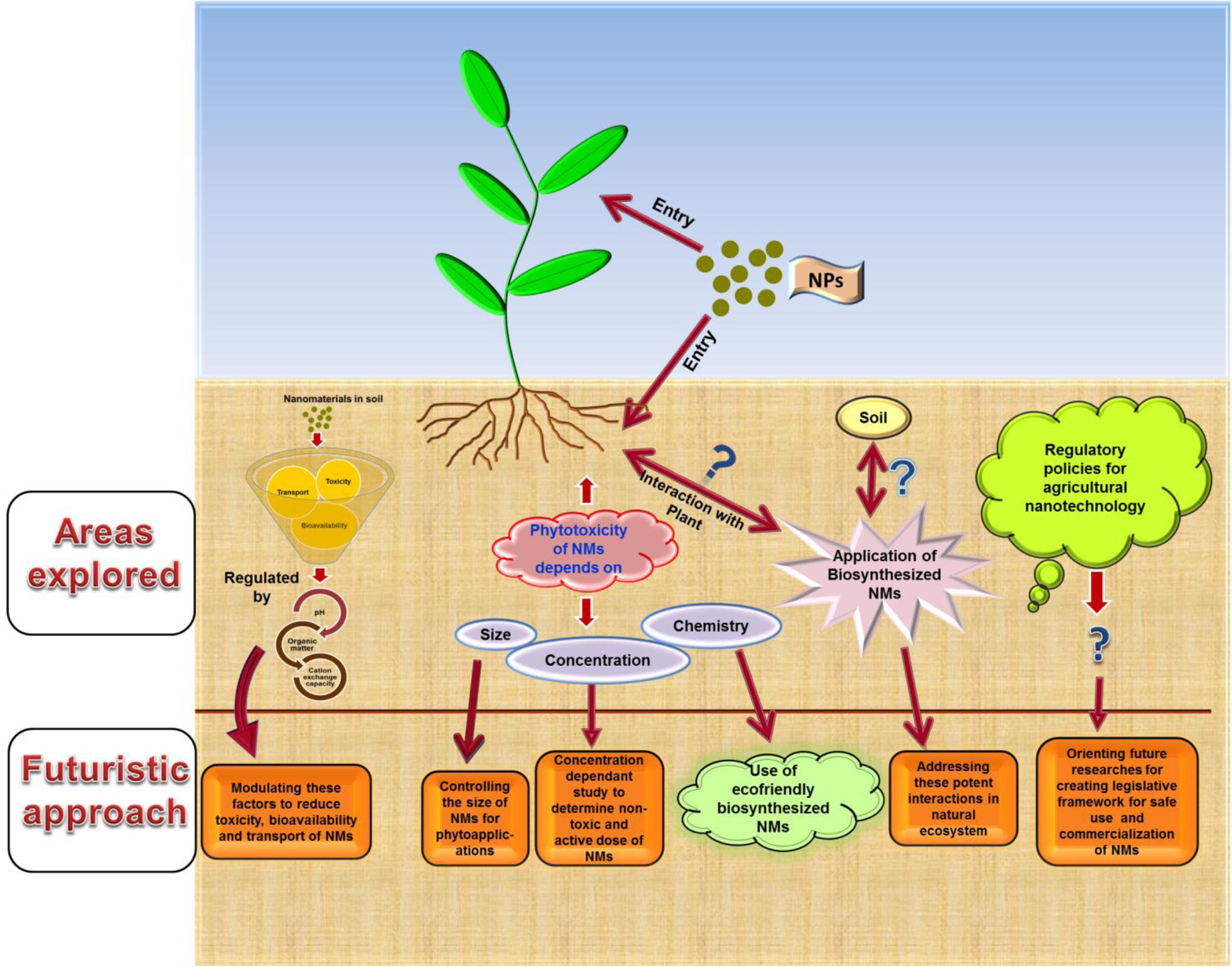
FIGURE 2. Schematic representation of the key points to be addressed in future researches on agri-nanotechnology for filling in the identified knowledge gaps.
✓ The future researches must be emphasized toward searching the ways to circumvent the risk factors associated with nanoparticles usage. Studying nanoparticles synthesis and bestowing few applications limited to laboratory conditions only could not contribute to the complete acceptance of nanotechnology in agricultural sector. Hence, scientific community must work together to improve the future researches based on more realistic approach.
✓ Validating the permissible level of nanoparticles dose within safety limits need to be explored and clarified. This could be achieved by attempting concentration dependent study in natural soil system in order to understand the accurate active and non-toxic dose of nanoparticles.
✓ An understanding of the transgenerational and trophic chain transfer effects of nanoparticles applications on plants must be included to gain comprehensive knowledge of nanotoxicity. Interestingly, selection of permissible level together with studying transgenerational and trophic chain transfer effects could provide adequate safety assessments.
✓ A clear overview of the soil physico-chemical characteristics of the agricultural fields where nanoparticles are to be applied may help in reducing their risk toward plant and soil biota. Altering soil environment in a way to modify the fate, transport and bioavailability of nanoparticles in order to reduce their subsequent toxicity could greatly achieve their safe and beneficial applications in agriculture. For example, advanced soil management practices for improving soil conditions could assist in reducing transport, bioavailability and further toxicity of nanoparticles with significant positive impact in agroecosystem.
✓ Finally, and most importantly, we strongly recommend the inclusion of biosynthesized nanoparticles as prerequisites for consequential and in-depth researches. Redeeming the environment-friendly approach of green synthesis of nanoparticles, it is believed that biosynthesized nanoparticles may possess relatively lesser or no toxicity and hence future researches must precisely focus on their practical utility. In addition, experimental design must be set in natural environment (growing the plants in soil) to give precise depiction of environmental impact of nanoparticles.
Author Contributions
SM and HS conceived the idea of review, provided inputs for specific sections, and edited the final draft. The primary manuscript was written by SM and CK. PA and LF provided specific comments. All the authors read and approved it for publication.
Conflict of Interest Statement
The authors declare that the research was conducted in the absence of any commercial or financial relationships that could be construed as a potential conflict of interest.
Acknowledgments
SM is grateful to the Department of Science and Technology, Government of India, New Delhi, for financial assistance under SERB-Start-Up Research Grant (Young Scientist) Scheme (YSS/2015/000082). LF would like to thank São Paulo State Research Foundation (Process #2015/15617-9).
References
Ahmad, A., Mukherjee, P., Senapati, S., Mandal, D., Khan, M. I., Kumar, R., et al. (2003). Extracellular biosynthesis of silver nanoparticles using the fungus Fusarium oxysporum. Colloids Surf. B Biointerfaces 28, 313–318. doi: 10.1016/S0927-7765(02)00174-1
Amenta, V., Aschberger, K., Arena, M., Bouwmeester, H., Moniz, F. B., Brandhoff, P., et al. (2015). Regulatory aspects of nanotechnology in the agri/feed/food sector in EU and non-EU countries. Regul. Toxicol. Pharmacol. 73, 463–476. doi: 10.1016/j.yrtph.2015.06.016
Anjum, N. A., Gill, S. S., Duarte, A. C., Pereira, E., and Ahmad, I. (2013). Silver nanoparticles in soil–plant systems. J. Nanopart. Res. 15, 1896. doi: 10.1007/s11051-013-1896-7
Arts, J. H. E., Hadi, M., Keene, A. M., Kreiling, R., Lyon, D., Maier, M., et al. (2014). A critical appraisal of existing concepts for the grouping of nanomaterials. Regul. Toxicol. Pharmacol. 70, 492–506. doi: 10.1016/j.yrtph.2014.07.025
Aslan, K., Holley, P., and Geddes, C. D. (2006). Metal-enhanced fluorescence from silver nanoparticledeposited polycarbonate substrates. J. Mater. Chem. 16, 2846–2852. doi: 10.1039/b604650a
Asli, S., and Neumann, P. M. (2009). Colloidal suspensions of clay or titanium dioxide NPs can inhibit leaf growth and transpiration via physical effects on root water transport. Plant Cell Environ. 32, 577–584. doi: 10.1111/j.1365-3040.2009.01952.x
Auffan, M., Rose, J., Bottero, J. Y., Lowry, G. V., Jolivet, J. P., and Wiesner, M. R. (2009). Towards a definition of inorganic nanoparticles from an environmental, health and safety perspective. Nat. Nanotechnol. 4, 634–641. doi: 10.1038/nnano.2009.242
Barik, T. K., Sahu, B., and Swain, V. (2008). Nanosilica-from medicine to pest control. Parasitol. Res. 103, 253–258. doi: 10.1007/s00436-008-0975-7
Benoit, R., Wilkinson, K. J., and Sauve, S. (2013). Partitioning of silver and chemical speciation of free Ag in soils amended with nanoparticles. Chem. Cent. J. 7:75. doi: 10.1186/1752-153X-7-75
Birbaum, K., Brogioli, R., Schellenberg, M., Martinoia, E., Stark, W. J., Günther, D., et al. (2010). No evidence for cerium dioxide nanoparticle translocation in maize plants. Environ. Sci. Technol. 44, 8718–8723. doi: 10.1021/es101685f
Calder, A. J., Dimkpa, C. O., McLean, J. E., Britt, D. W., Johnsonc, W., and Anderson, A. J. (2012). Soil components mitigate the antimicrobial effects of silver nanoparticles towards a beneficial soil bacterium, Pseudomonas chlororaphis O6. Sci. Total Environ. 429, 215–222. doi: 10.1016/j.scitotenv.2012.04.049
Campos, E. V., Oliveira, J. L., and Fraceto, L. F. (2014). Applications of controlled release systems for fungicides, herbicides, acaricides, nutrients, and plant growth hormones: a review. Adv. Sci. Eng. Med. 6, 373–387. doi: 10.1166/asem.2014.1538
Campos, E. V., Oliveira, J. L., Fraceto, L. F., and Singh, B. (2015a). Polysaccharides as safer release systems for agrochemicals. Agron. Sustain. Dev. 35, 47–66. doi: 10.1016/j.carbpol.2013.10.025
Campos, E. V., Oliveira, J. L., Goncalves, C. M., Pascoli, M., Pasquoto, T., Lima, R., et al. (2015b). Polymeric and solid lipid nanoparticles for sustained release of carbendazim and tebuconazole in agricultural applications. Sci. Rep. 5:13809. doi: 10.1038/srep13809
Cao, J., and Hu, X. (2009). Synthesis of gold nanoparticles using halloysites. e-J. Surf. Sci. Nanotechnol. 7, 813–815. doi: 10.1380/ejssnt.2009.813
Caruthers, S. D., Wickline, S. A., and Lanza, G. M. (2007). Nanotechnological applications in medicine. Curr. Opin. Biotechnol. 18, 26–30. doi: 10.1016/j.copbio.2007.01.006
Chen, H., and Yada, R. (2011). Nanotechnologies in agriculture: new tools for sustainable development. Trends Food Sci. Technol. 22, 585–594. doi: 10.1002/ps.1732
Chunjaturas, W., Ferguson, J. A., Rattanapichai, W., Sadowsky, M. J., and Sajjaphan, K. (2014). Shift of bacterial community structure in two Thai soil series affected by silver nanoparticles using ARISA. World J. Microbiol. Biotechnol. 30, 2119–2124. doi: 10.1007/s11274-014-1633-0
Cifuentes, Z., Custardoy, L., de la Fuente, J. M., Marquina, C., Ibarra, M. R., Rubiales, D., et al. (2010). Absorption and translocation to the aerial part of magnetic carbon-coated nanoparticles through the root of different crop plants. J. Nanobiotechnol. 8, 1–8. doi: 10.1186/1477-3155-8-26
Colman, B. P., Arnaout, C. L., Anciaux, S., Gunsch, C. K., Hochella, M. F. Jr., Kim, B., et al. (2013). Low concentrations of silver nanoparticles in biosolids cause adverse ecosystem responses under realistic field scenario. PLoS ONE 8:e57189. doi: 10.1371/journal.pone.0057189
Conway, G. R., and Barbie, E. B. (1988). After the green revolution: sustainable and equitable agricultural development. Futures 20, 651–670. doi: 10.1016/0016-3287(88)90006-7
De La Torre-Roche, R., Hawthorne, J., Deng, Y., Xing, B., Cai, W., Newman, L. A., et al. (2013). Multiwalled carbon nanotubes and c60 fullerenes differentially impact the accumulation of weathered pesticides in four agricultural plants. Environ. Sci. Technol. 47, 12539–12547. doi: 10.1021/es4034809
Dietz, K. J., and Herth, S. (2011). Plant nanotoxicology. Trends Plant Sci. 16, 582–589. doi: 10.1016/j.tplants.2011.08.003
Dimkpa, C. O., Latta, D. E., McLean, J. E., Britt, D. W., Boyanov, M. I., and Anderson, A. J. (2013). Fate of CuO and ZnO nano-and microparticles in the plant environment. Environ. Sci. Technol. 47, 4734–4742. doi: 10.1021/es304736y
Dimkpa, C. O., McLean, J. E., Britt, D. W., and Anderson, A. J. (2012). CuO and ZnO nanoparticles differently affect the secretion of fluorescent siderophores in the beneficial root colonizer, Pseudomonas chlororaphis O6. Nanotoxicology 6, 635–642. doi: 10.3109/17435390.2011.598246
Du, W., Sun, Y., Ji, R., Zhu, J., Wu, J., and Guo, H. (2011). TiO2 and ZnO nanoparticles negatively affect wheat growth and soil enzyme activities in agricultural soil. J. Environ. Monit. 13, 822–828. doi: 10.1039/c0em00611d
European Commission (2013). Proposal for a Regulation of the European Parliament and of the Council on Novel Foods. Available at: http://ec.europa.eu/food/food/biotechnology/novelfood/documents/novel-cloning_com2013-894_final_en.pdf
FAO/WHO (2013). State of the Art on the Initiatives and Activities Relevant to Risk Assessment and Risk Management of Nanotechnologies in the Food and Agriculture Sectors. Available at: http://www.fao.org/docrep/018/i3281e/i3281e.pdf
Frenk, S., Ben-Moshe, T., Dror, I., Berkowitz, B., and Minz, D. (2013). Effect of metal oxide nanoparticles on microbial community structure and function in two different soil types. PLoS ONE 8:e84441. doi: 10.1371/journal.pone.0084441
Gan, N., Yang, X., Xie, D., Wu, Y., and Wen, W. A. (2010). Disposable organophosphorus pesticides enzyme biosensor based on magnetic composite nanoparticles modified screen printed carbon electrode. Sensors 10, 625–638. doi: 10.3390/s100100625
Ge, Y., Priester, J. H., Van De Werfhorst, L. C., Schimel, J. P., and Holden, P. A. (2013). Potential mechanisms and environmental controls of TiO2 nanoparticle effects on soil bacterial communities. Environ. Sci. Technol. 47, 14411–14417. doi: 10.1021/es403385c
Ge, Y., Schimel, J. P., and Holden, P. A. (2011). Evidence for negative effects of TiO2 and ZnO nanoparticles on soil bacterial communities. Environ. Sci. Technol. 45, 1659–1664. doi: 10.3390/s100100625
Ge, Y., Schimel, J. P., and Holden, P. A. (2012). Identification of soil bacteria susceptible to TiO2 and ZnO nanoparticles. Appl. Environ. Microbiol. 78, 6749–6758. doi: 10.1128/AEM.00941-12
Gericke, M., and Pinches, A. (2006). Microbial production of gold nanoparticles. Gold Bull. 39, 22–28. doi: 10.1007/BF03215529
Ghormade, V., Deshpande, M. V., and Paknikar, K. M. (2011). Perspectives for nanobiotechnology enabled protection and nutrition of plants. Biotechnol. Adv. 29, 792–803. doi: 10.1016/j.biotechadv.2011.06.007
Gopinath, V., and Velusamy, P. (2013). Extracellular biosynthesis of silver nanoparticles using Bacillus sp. GP-23 and evaluation of their antifungal activity towards Fusarium oxysporum. Spectrochim. Acta A Mol. Biomol. Spectrosc. 106, 170–174. doi: 10.1016/j.saa.2012.12.087
Grillo, R., Abhilash, P. C., and Fraceto, L. F. (2016). Nanotechnology applied to bio-encapsulation of pesticides. J. Nanosci. Nanotechnol. 16, 1231–1234. doi: 10.1166/jnn.2016.12332
Grillo, R., Pereira, A. E. S., Nishisaka, C. S., de Lima, R., Oehlke, K., Greiner, R., et al. (2014). Chitosan/tripolyphosphate nanoparticles loaded with paraquat herbicide: an environmentally safer alternative for weed control. J. Hazard. Mater. 278, 163–171. doi: 10.1016/j.jhazmat.2014.05.079
Handford, C. E., Dean, M., Spence, M., Henchion, M., Elliott, C. T., and Campbell, K. (2015). Awareness and attitudes towards the emerging use of nanotechnology in the agri-food sector. Food Control 57, 24–34. doi: 10.1016/j.foodcont.2015.03.033
Hänsch, M., and Emmerling, C. (2010). Effects of silver nanoparticles on the microbiota and enzyme activity in soil. J. Plant Nutr. Soil Sci. 173, 554–558. doi: 10.1002/jpln.200900358
He, S., Feng, Y., Ren, H., Zhang, Y., Gu, N., and Lin, X. (2011). The impact of iron oxide magnetic nanoparticles on the soil bacterial community. J. Soils Sediments 11, 1408–1417. doi: 10.1007/s11368-011-0415-7
Hussain, I., Singh, N. B., Singh, A., Singh, H., and Singh, S. C. (2016). Green synthesis of nanoparticles and its potential application. Biotechnol. Lett. 38, 545–560. doi: 10.1007/s10529-015-2026-7
Iravani, S., Korbekandi, H., Mirmohammadi, S. V., and Zolfaghari, B. (2014). Synthesis of silver nanoparticles: chemical, physical and biological methods. Res. Pharm. Sci. 9, 385–406.
Jacobson, A. R., McBride, M. B., Baveye, P., and Steenhuis, T. S. (2005). Environmental factors determining the trace-level sorption of silver and thallium to soils. Sci. Total Environ. 345, 191–205. doi: 10.1016/j.scitotenv.2004.10.027
Jiang, S., Eltoukhy, A. A., Love, K. T., Langer, R., and Anderson, D. G. (2013). Lipidoid-coated iron oxide nanoparticles for efficient DNA and siRNA delivery. Nano lett. 13, 1059–1064. doi: 10.1021/nl304287a
Jośko, I., and Oleszczuk, P. (2013). Influence of soil type and environmental conditions on ZnO, TiO 2 and Ni nanoparticles phytotoxicity. Chemosphere 92, 91–99. doi: 10.1016/j.chemosphere.2013.02.048
Kah, M., and Hofmann, T. (2014). Nanopesticide research: current trends and future priorities. Environ. Int. 63, 224–235. doi: 10.1016/j.envint.2013.11.015
Kalaiarasi, R., Jayallakshmi, N., and Venkatachalam, P. (2010). Phytosynthesis of nanoparticles and its applications. Plant Cell Biotechnol. Mol. Biol. 11, 1–16.
Kharissova, O. V., Dias, H. V. R., Kharisov, B. I., Pe’rez, B. O., and Pe’rez, V. M. J. (2013). The greener synthesis of nanoparticles. Trends Biotechnol. 31, 240–248. doi: 10.1016/j.tibtech.2013.01.003
Khodakovskaya, M., Dervishi, E., Mahmood, M., Xu, Y., Li, Z., Watanabe, F., et al. (2009). Carbon nanotubes are able to penetrate plant seed coat and dramatically affect seed germination and plant growth. ACS Nano 3, 3221–3227. doi: 10.1021/nn900887m
Khodakovskaya, M. V., Kim, B., Kim, J. N., Alimohammadi, M., Dervishi, E., Mustafa, T., et al. (2013). Carbon nanotubes as plant growth regulators: effects on tomato growth, reproductive system, and soil microbial community. Small 9, 115–123. doi: 10.1002/smll.201201225
Kim, S., Kim, J., and Lee, I. (2011). Effects of Zn and ZnO nanoparticles and Zn2+ on soil enzyme activity and bioaccumulation of Zn in Cucumis sativus. Chem. Ecol. 27, 49–55. doi: 10.1080/02757540.2010.529074
Klitzke, S., Metreveli, G., Peters, A., Schaumann, G. E., and Lang, F. (2015). The fate of silver nanoparticles in soil solution-Sorption of solutes and aggregation. Sci. Total Environ. 535, 54–60. doi: 10.1016/j.scitotenv.2014.10.108
Knell, M. (2010). “Nanotechnology and the sixth technological revolution,” in Nanotechnology and the Challenges of Equity, Equality and Development, eds S. E. Cozzens and J. M. Wetmore (Dordrecht: Springer), 127–143. doi: 10.1007/978-90-481-9615-9_8
Krishnaraj, C., Jagan, G., Ramachandran, R., Abirami, S. M., Mohan, N., and Kalaichelvan, P. T. (2012). Effect of biologically synthesized silver nanoparticles on Bacopa monnieri L. Wettst. plant growth metabolism. Process Biochem. 47, 651–658. doi: 10.1016/j.procbio.2012.01.006
Lee, C. W., Mahendra, S., Zodrow, K., Li, D., Tsai, Y. C., Braam, J., et al. (2010). Developmental phytotoxicity of metal oxide nanoparticles to Arabidopsis thaliana. Environ. Toxicol. Chem. 29, 669–675. doi: 10.1002/etc.58
Lee, K. J., Park, S. H., Govarthanan, M., Hwang, P. H., Seo, Y. S., Cho, M., et al. (2013). Synthesis of silver nanoparticles using cow milk and their antifungal activity against phytopathogens. Mater. Lett. 105, 128–131. doi: 10.1016/j.matlet.2013.04.076
Lee, W. M., An, Y. J., Yoon, H., and Kweon, H. S. (2008). Toxicity and bioavailability of copper nanoparticles to the terrestrial plants mung bean (Phaseolus radiatus) and wheat (Triticum aestivum): plant agar test for water-insoluble nanoparticles. Environ. Toxicol. Chem. 27, 1915–1921. doi: 10.1897/07-481.1
Lee, W.-M., Kwak, J. I., and An, Y.-J. (2012). Effect of silver nanoparticles in crop plants Phaseolus radiatus and Sorghum bicolor: media effect on phytotoxicity. Chemosphere 86, 491–499. doi: 10.1016/j.chemosphere.2011.10.013
Li, X., Xu, H., Chen, Z. S., and Chen, G. (2011). Biosynthesis of nanoparticles by microorganisms and their applications. J. Nanomater. 2011:270974. doi: 10.1155/2011/270974
Li, Z. Z., Chen, J. F., Liu, F., Liu, A. Q., Wang, Q., and Sun, H. Y. (2007). Study of UV-shielding properties of novel porous hollow silica nanoparticle carriers for avermectin. Pest Manag. Sci. 63, 241–246. doi: 10.1002/ps.1301
Lin, D., and Xing, B. (2007). Phytotoxicity of nanoparticles: inhibition of seed germination and root growth. Environ. Pollut. 150, 243–250. doi: 10.1016/j.envpol.2007.01.016
Linkov, I., Bates, M. E., Canis, L. J., Seager, T. P., and Keisler, J. M. (2011). A decision-directed approach for prioritizing research into the impact of nanomaterials on the environment and human health. Nat. Nanotechnol. 6, 784–787. doi: 10.1038/nnano.2011.163
Liu, R., and Lal, R. (2015). Potentials of engineered nanoparticles as fertilizers for increasing agronomic productions. Sci. Total Environ. 514, 131–139. doi: 10.1016/j.scitotenv.2015.01.104
Ma, X. M., Geiser-Lee, J., Deng, Y., and Kolmakov, A. (2010). Interactions between engineered NPs (ENPs) and plants: phytotoxicity, uptake and accumulation. Sci. Total Environ. 408, 3053–3061. doi: 10.1016/j.scitotenv.2010.03.031
Mahajan, P., Dhoke, S. K., and Khanna, A. S. (2011). Effect of nano-ZnO particle suspension on growth of mung (Vigna radiata) and gram (Cicer arietinum) seedlings using plant agar method. J. Nanotechnol. 7:696535. doi: 10.1155/2011/696535
Maruyama, C. R., Guilger, M., Pascoli, M., Bileshy-José, N., Abhilash, P. C., Fraceto, L. F., et al. (2016). Nanoparticles based on chitosan as carriers for the combined herbicides imazapic and imazapyr. Sci. Rep. 6:19768. doi: 10.1038/srep19768
Mishra, S., Keswani, C., Singh, A., Singh, B. R., Singh, S. P., and Singh, H. B. (2016). “Microbial nanoformulation: exploring potential for coherent nano-farming,” in The Handbook of Microbial Bioresourses, eds V. K. Gupta, G. D. Sharma, M. G. Tuohy, and R. Gaur (London: CABI), 107–120.
Mishra, S., Singh, A., Keswani, C., Saxena, A., Sarma, B. K., and Singh, H. B. (2015). “Harnessing plant-microbe interactions for enhanced protection against phytopathogens,” in Plant Microbes Symbiosis: Applied Facets, ed. N. K. Arora (New Delhi: Springer), 111–125.
Mishra, S., Singh, A., Keswani, C., and Singh, H. B. (2014a). Nanotechnology: exploring potential application in agriculture and its opportunities and constraints. Biotech Today 4, 9–14. doi: 10.5958/2322-0996.2014.00011.8
Mishra, S., Singh, B. R., Singh, A., Keswani, C., Naqvi, A. H., and Singh, H. B. (2014b). Biofabricated silver nanoparticles act as a strong fungicide against Bipolaris sorokiniana causing spot blotch disease in wheat. PLoS ONE 9:e97881. doi: 10.1371/journal.pone.0097881
Mishra, S., and Singh, H. B. (2015a). Biosynthesized silver nanoparticles as a nanoweapon against phytopathogens: exploring their scope and potential in agriculture. Appl. Microbiol. Biotechnol. 99, 1097–1107. doi: 10.1007/s00253-014-6296-0
Mishra, S., and Singh, H. B. (2015b). Silver nanoparticles mediated altered gene expression of melanin biosynthesis genes in Bipolaris sorokiniana. Microbiol. Res. 172, 16–18. doi: 10.1016/j.micres.2015.01.006
Mishra, S., and Singh, H. B. (2016). Preparation of biomediated metal nanoparticles. Indian Patent Filed 201611003248.
Mittal, A. K., Chisti, Y., and Banerjee, U. C. (2013). Synthesis of metallic nanoparticles using plant extracts. Biotechnol. Adv. 31, 346–356. doi: 10.1016/j.biotechadv.2013.01.003
NAAS (2013). Nanotechnology in Agriculture: Scope and Current Relevance. Policy Paper No. 63. New Delhi: National Academy of Agricultural Sciences, 20.
Nair, R., Varghese, S. H., Nair, B. G., Maekawa, T., Yoshida, Y., and Kumar, D. S. (2010). Nanoparticulate material delivery to plants. Plant Sci. 179, 154–163. doi: 10.1016/j.plantsci.2010.04.012
Navrotsky, A. (2000). Nanomaterials in the environment, agriculture, and technology (NEAT). J. Nanopart. Res. 2, 321–323. doi: 10.1023/A:1010007023813
NCPI (2011). Nanotechnology Consumer Products Inventory. Available at: http://www.nanotechproject.org
OECD (2013). Regulatory Frameworks for Nanotechnology in Foods and Medical Products. Summary Results of a Survey Activity. Paris: OECD. doi: 10.1787/5k47w4vsb4s4-en
Oliveira, H. C., Stolf-Moreira, R., Martinez, C. B. R., Grillo, R., de Jesus, M. B., and Fraceto, L. F. (2015). Nanoencapsulation enhances the post-emergence herbicidal activity of atrazine against mustard plants. PLoS ONE 10:e132971. doi: 10.1371/journal.pone.0132971
Oliveira, J. L., Campos, E. V., Bakshi, M., Abhilash, P. C., and Fraceto, L. F. (2014). Application of nanotechnology for the encapsulation of botanical insecticides for sustainable agriculture: prospects and promises. Biotechnol. Adv. 32, 1550–1561. doi: 10.1016/j.biotechadv.2014.10.010
Oliveira, J. L., Campos, E. V., Goncalves, C. M., Pasquoto, T., de Lima, R., and Fraceto, L. F. (2015). Solid lipid nanoparticles co-loaded with simazine and atrazine: preparation, characterization, and evaluation of herbicidal activity. J. Agric. Food Chem. 63, 422–432. doi: 10.1021/jf5059045
Órdenes-Aenishanslins, N. A., Saona, L. A., Durán-Toro, V. M., Monrás, J. P., Bravo, D. M., and Pérez-Donoso, J. M. (2014). Use of titanium dioxide nanoparticles biosynthesized by Bacillus mycoides in quantum dot sensitized solar cells. Microb. Cell Fact. 13, 90. doi: 10.1186/s12934-014-0090-7
Parisi, C., Vigani, M., and Rodriguez-Cerezo, E. (2015). Agricultural nanotechnologies: What are the current possibilities? Nano Today 10, 124–127. doi: 10.1016/j.bios.2015.11.086
Parsons, J. G., Peralta-Videa, J. R., and Gardea-Torresdey, J. L. (2007). Use of plants in biotechnology: synthesis of metal nanoparticles by inactivated plant tissues, plant extracts, and living plants. Dev. Environ. Sci. 5, 463–485. doi: 10.1016/s1474-8177(07)05021-8
Paulkumar, K., Gnanajobitha, G., Vanaja, M., Rajeshkumar, S., Malarkodi, C., Pandian, K., et al. (2014). Piper nigrum leaf and stem assisted green synthesis of silver nanoparticles and evaluation of its antibacterial activity against agricultural plant pathogens. Sci. World J. 2014:829894. doi: 10.1155/2014/829894
Pimprikar, P. S., Joshi, S. S., Kumar, A. R., Zinjarde, S. S., and Kulkarni, S. K. (2009). Influence of biomass and gold salt concentration on nanoparticle synthesis by the tropical marine yeast Yarrowia lipolytica NCIM 3589. Colloids Surf. B Biointerfaces 74, 309–316. doi: 10.1016/j.colsurfb.2009.07.040
Qian, H., Peng, X., Han, X., Ren, J., Sun, L., and Fu, Z. (2013). Comparison of the toxicity of silver nanoparticles and silver ion on the growth of terrestrial plant model Arabidopsis thaliana. J. Environ. Sci. 25, 1947–1956. doi: 10.1016/S1001-0742(12)60301-5
Raliya, R., Biswas, P., and Tarafdar, J. C. (2015). TiO2 nanoparticle biosynthesis and its physiological effect on mung bean (Vigna radiata L.). Biotechnol. Rep. 5, 22–26. doi: 10.1016/j.btre.2014.10.009
Raliya, R., and Tarafdar, J. C. (2013). ZnO nanoparticle biosynthesis and its effect on phosphorous-mobilizing enzyme secretion and gum contents in clusterbean (Cyamopsis tetragonoloba L.). Agric. Res. 2, 48–57. doi: 10.1007/s40003-012-0049-z
Raliya, R., Tarafdar, J. C., Singh, S. K., Gautam, R., Choudhary, K., Maurino Veronica, G., et al. (2014). MgO nanoparticles biosynthesis and its effect on chlorophyll contents in the leaves of Clusterbean (Cyamopsis tetragonoloba L.). Adv. Sci. Eng. Med. 6, 538–545. doi: 10.1166/asem.2014.1540
Research and Markets (2015). Global Nanotechnology Market Outlook 2015–2020. Available at: http://www.prnewswire.com/news-releases/global-nanotechnology-market-outlook-2015-2020---industry-will-grow-to-reach-us-758-billion-507155671.html
Rico, C. M., Majumdar, S., Duarte-Gardea, M., Peralta-Videa, J. R., and GardeaTorresdey, J. L. (2011). Interaction of nanoparticles with edible plants and their possible implications in the food chain. J. Agric. Food Chem. 59, 3485–3498. doi: 10.1021/jf104517j
Rossi, M., Cubadda, F., Dini, L., Terranova, M. L., Aureli, F., Sorbo, A., et al. (2014). Scientific basis of nanotechnology, implications for the food sector and future trends. Trends Food Sci. Technol. 40, 127–148. doi: 10.1016/j.tifs.2014.09.004
Sabir, S., Arshad, M., and Chaudhari, S. K. (2014). Zinc oxide nanoparticles for revolutionizing agriculture: synthesis and applications. Sci. World J. 2014, 925494. doi: 10.1155/2014/925494
Sabourin, V., and Ayande, A. (2015). Commercial opportunities and market demand for nanotechnologies in agribusiness sector. J. Technol. Manag. Innov. 10, 40–51. doi: 10.4067/S0718-27242015000100004
Salamanca-Buentello, F., Persad, D. L., Court, E. B., Martin, D. K., Daar, A. S., and Singer, P. A. (2005). Nanotechnology and the developing world. PLoS Med. 2:e97. doi: 10.1371/journal.pmed.0020097
Sanchez-Mendieta, V., and Vilchis-Nestor, A. R. (2012). “Green synthesis of noble metal (Au, Ag, Pt) nanoparticles, assisted by plant-extracts,” in Noble Metals, ed. S. Yen-Hsun (Rijeka: INTECH), 391–408. doi: 10.5772/34335
Sastry, M., Ahmad, A., Khan, M. I., and Kumar, R. (2004). “Microbial nanoparticle production,” in Nanobiotechnology, eds C. M. Niemeyer and C. A. Mirkin (Weinheim: Wiley), 126–135.
Sastry, R. K., Rashmi, H. B., and Rao, N. H. (2011). Nanotechnology for enhancing food security in India. Food Policy 36, 391–400. doi: 10.1016/j.foodpol.2010.10.012
Schlich, K., and Hund-Rinke, K. (2015). Influence of soil properties on the effect of silver nanomaterials on microbial activity in five soils. Environ. Pollut. 196, 321–330. doi: 10.1016/j.envpol.2014.10.021
Servin, A. D., and White, J. C. (2016). Nanotechnology in agriculture: next steps for understanding engineered nanoparticle exposure and risk. NanoImpact 1, 9–12. doi: 10.1016/j.impact.2015.12.002
Shah, V., and Belozerova, I. (2009). Influence of metal nanoparticles on the soil microbial community and germination of lettuce seeds. Water Air Soil Pollut. 197, 143–148. doi: 10.1007/s11270-008-9797-6
Shah, V., Collins, D., Walker, V. K., and Shah, S. (2014). The impact of engineered cobalt, iron, nickel and silver nanoparticles on soil bacterial diversity under field conditions. Environ. Res. Lett. 9:024001. doi: 10.1088/1748-9326/9/2/024001
Shahrokh, S., Hosseinkhani, B., and Emtiazi, G. (2014). The impact of nano-silver on bacterial aerobic nitrate reductase. J. Bioprocess. Biotechnol. 4:162. doi: 10.4172/2155-9821.1000162
Sharon, M., Choudhary, A., and Kumar, R. (2010). Nanotechnology in agricultural diseases and food safety. J. Phytol. 4, 83–92.
Shen, Z., Chen, Z., Hou, Z., Li, T., and Lu, X. (2015). Ecotoxicological effect of zinc oxide nanoparticles on soil microorganisms. Front. Environ. Sci. Eng. 9, 912–918. doi: 10.1007/s11783-015-0789-7
Sheng, Z., and Liu, Y. (2011). Effects of silver nanoparticles on wastewater biofilms. Water Res. 45, 6039–6050. doi: 10.1016/j.watres.2011.08.065
Shoults-Wilson, W. A., Reinsch, B. C., Tsyusko, O. V., Bertsch, P. M., Lowry, G. V., and Unrine, J. M. (2011). Role of particle size and soil type in toxicity of silver nanoparticles to earthworms. Soils Sci. Soc. Am. J. 75, 365–377. doi: 10.1016/j.envpol.2016.08.016
Simonin, M., Guyonnet, J. P., Martins, J. M., Ginot, M., and Richaume, A. (2015). Influence of soil properties on the toxicity of TiO2 nanoparticles on carbon mineralization and bacterial abundance. J. Hazard. Mater. 283, 529–535. doi: 10.1016/j.jhazmat.2014.10.004
Simonin, M., and Richaume, A. (2015). Impact of engineered nanoparticles on the activity, abundance, and diversity of soil microbial communities: a review. Environ. Sci. Pollut. Res. 22, 13710–13723. doi: 10.1007/s11356-015-4171-x
Singaravelu, G., Arockiamary, J. S., Ganesh Kumar, V., and Govindraju, K. (2007). A novel extracellular synthesis of monodisperse gold nanoparticles using marine alga, Sargassum wightii Greville. Colloid Surf. B Biointerface 57, 97–101. doi: 10.1016/j.colsurfb.2007.01.010
Singh, P., Kim, Y. J., Zhang, D., and Yang, D. C. (2016). Biological synthesis of nanoparticles from plants and microorganisms. Trends Biotechnol. 34, 588–599. doi: 10.1016/j.tibtech.2016.02.006
Sintubin, L., Gusseme, D. B., Meeren, V. P., Pycke, B. F. G., Verstraete, W., and Boon, N. (2011). The antibacterial activity of biogenic silver and its mode of action. Appl. Microbiol. Biotechnol. 91, 153–162. doi: 10.1007/s00253-011-3225-3
Sodano, V., and Verneau, F. (2014). Competition policy and food sector in the european union. J. Int. Food Agribus. Mark. 26, 155–172. doi: 10.1080/08974438.2013.833576
Song, G., Gao, Y., Wu, H., Hou, W., Zhang, C., and Ma, H. (2012). Physiological effect of anatase TiO2 nanoparticles on Lemna minor. Environ. Toxicol. Chem. 31, 2147–2152. doi: 10.1002/etc.1933
Stampoulis, D., Sinha, S. K., and White, J. C. (2009). Assay-dependent phytotoxicity of nanoparticles to plants. Environ. Sci. Technol. 43, 9473–9479. doi: 10.1021/es901695c
Su, M., Liu, H., Liu, C., Qu, C., Zheng, L., and Hong, F. (2009). Promotion of nano-anatase TiO2 on the spectral responses and photochemical activities of D1/D2/Cyt b559 complex of spinach. Spectrochim. Acta A Mol. Biomol. Spectrosc. 72, 1112–1116. doi: 10.1016/j.saa.2009.01.010
Throbäck, I. N., Johansson, M., Rosenquist, M., Pell, M., Hansson, M., and Hallin, S. (2007). Silver (Ag+) reduces denitrification and induces enrichment of novel nirK genotypes in soil. FEMS Microbiol. Lett. 270, 189–194. doi: 10.1111/j.1574-6968.2007.00632.x
VandeVoort, A. R., and Arai, Y. (2012). Effect of silver nanoparticles on soil denitrification kinetics. Ind. Biotechnol. 8, 358–364. doi: 10.1089/ind.2012.0026
Vannini, C., Domingo, G., Onelli, E., De Mattia, F., Bruni, I., Marsoni, M., et al. (2014). Phytotoxic and genotoxic effects of silver nanoparticles exposure on germinating wheat seedlings. J. Plant Physiol. 171, 1142–1148. doi: 10.1016/j.jplph.2014.05.002
Varma, R. S. (2012). Greener approach to nanomaterials and their sustainable applications. Curr. Opin. Chem. Eng. 1, 123–128. doi: 10.1016/j.coche.2011.12.002
Vittori Antisari, L., Carbone, S., Gatti, A., Vianello, G., and Nannipieri, P. (2013). Toxicity of metal oxide (CeO2, Fe3O4, SnO2) engineered nanoparticles on soil microbial biomass and their distribution in soil. Soil Biol. Biochem. 60, 87–94. doi: 10.1016/j.soilbio.2013.01.016
Wang, J., Koo, Y., Alexander, A., Yang, Y., Westerhof, S., Zhang, Q., et al. (2013). Phytostimulation of poplars and Arabidopsis exposed to silver nanoparticles and Ag+ at sublethal concentrations. Environ. Sci. Technol. 47, 5442–5449. doi: 10.1021/es4004334
Wang, P., Menzies, N. W., Lombi, E., McKenna, B. A., Johannessen, B., Glover, C. J., et al. (2013). Fate of ZnO nanoparticles in soils and cowpea (Vigna unguiculata). Environ. Sci. Technol. 47, 13822–13830. doi: 10.1021/es403466p
Wilson, M. A., Tran, N. H., Milev, A. S., Kannangara, G. S. K., Volk, H., and Lu, G. H. M. (2008). Nanomaterials in soils. Geoderma 146, 291–302. doi: 10.1016/j.geoderma.2008.06.004
Wirth, S. M., Lowry, G. V., and Tilton, R. D. (2012). Natural organic matter alters biofilm tolerance to silver nanoparticles and dissolved silver. Environ. Sci Technol. 46, 12687–12696. doi: 10.1021/es301521p
Yang, F., Liu, C., Gao, F., Su, M., Wu, X., Zheng, L., et al. (2007). The improvement of spinach growth by nano-anatase TiO2 treatment is related to nitrogen photoreduction. Biol. Trace Elem. Res. 119, 77–88. doi: 10.1007/s12011-007-0046-4
Yang, N., and Aoki, K. (2005). Voltammetry of the silver alkylcarboxylate nanoparticles in suspension. Electrochim. Acta 50, 4868–4872. doi: 10.1016/j.electacta.2005.02.071
Zhang, P., Ma, Y., Zhang, Z., He, X., Guo, Z., Tai, R., et al. (2012). Comparative toxicity of nanoparticulate/bulk Yb2O3and YbCl3to cucumber (Cucumis sativus). Environ. Sci. Technol. 46, 1834–1841. doi: 10.1021/es2027295
Zhao, L., Hernandez-Viezcas, J. A., Peralta-Videa, J. R., Bandyopadhyay, S., Peng, B., Munoz, B., et al. (2013). ZnO nanoparticle fate in soil and zinc bioaccumulation in corn plants (Zea mays) influenced by alginate. Environ. Sci. Processes Impacts 15, 260–266. doi: 10.1039/C2EM30610G
Keywords: agriculture, nanotechnology, biosynthesized nanoparticles, toxicity, bioavailability, sustainability, phytopathogens, soil
Citation: Mishra S, Keswani C, Abhilash PC, Fraceto LF and Singh HB (2017) Integrated Approach of Agri-nanotechnology: Challenges and Future Trends. Front. Plant Sci. 8:471. doi: 10.3389/fpls.2017.00471
Received: 15 January 2017; Accepted: 17 March 2017;
Published: 04 April 2017.
Edited by:
Susana Araújo, Universidade Nova de Lisboa, PortugalReviewed by:
Marta Marmiroli, University of Parma, ItalyFabián Fernández-Luqueño, Centro de Investigación y de Estudios Avanzados del Instituto Politécnico Nacional, Mexico
Copyright © 2017 Mishra, Keswani, Abhilash, Fraceto and Singh. This is an open-access article distributed under the terms of the Creative Commons Attribution License (CC BY). The use, distribution or reproduction in other forums is permitted, provided the original author(s) or licensor are credited and that the original publication in this journal is cited, in accordance with accepted academic practice. No use, distribution or reproduction is permitted which does not comply with these terms.
*Correspondence: Harikesh Bahadur Singh, aGJzMUByZWRpZmZtYWlsLmNvbQ== Leonardo F. Fraceto, bGVvbmFyZG9Ac29yb2NhYmEudW5lc3AuYnI=