- 1College of Life Sciences, Northwest A&F University, Yangling, China
- 2College of Forestry, Northwest A&F University, Yangling, China
The effect of arbuscular mycorrhizal fungus on the subcellular compartmentalization and chemical forms of lead (Pb) in Pb tolerance plants was assessed in a pot experiment in greenhouse conditions. We measured root colonization, plant growth, photosynthesis, subcellular compartmentalization and chemical forms of Pb in black locust (Robinia pseudoacacia L.) seedlings inoculated with Funneliformis mosseae isolate (BGC XJ01A) under a range of Pb treatments (0, 90, 900, and 3000 mg Pb kg-1 soil). The majority of Pb was retained in the roots of R. pseudoacacia under Pb stress, with a significantly higher retention in the inoculated seedlings. F. mosseae inoculation significantly increased the proportion of Pb in the cell wall and soluble fractions and decreased the proportion of Pb in the organelle fraction of roots, stems, and leaves, with the largest proportion of Pb segregated in the cell wall fraction. F. mosseae inoculation increased the proportion of inactive Pb (especially pectate- and protein-integrated Pb and Pb phosphate) and reduced the proportion of water-soluble Pb in the roots, stems, and leaves. The subcellular compartmentalization of Pb in different chemical forms was highly correlated with improved plant biomass, height, and photosynthesis in the inoculated seedlings. This study indicates that F. mosseae could improve Pb tolerance in R. pseudoacacia seedlings growing in Pb polluted soils.
Introduction
Lead (Pb) is one of the most widespread toxic metals (Fahr et al., 2013) and tends to remain in soil for long periods of time (LaBelle et al., 1987). Pb pollution may be naturally occurring or result from anthropogenic activities (Yadav, 2010), creating potential hazards to ecosystems (Lantzy and Mackenzie, 1979). Pb pollution has also been a serious threat to human health and food safety (Cuypers et al., 2016), especially harmful to young children (Datko-Williams et al., 2014). Over the last decade, evidence has been shown that Pb exposure-related health injury occur already at lower blood Pb levels than previously documented (Henry et al., 2015). Recently, the Centers for Disease Control and Prevention has lowered the reference value of blood Pb level to 5 μg dL-1 (Burns and Gerstenberger, 2014). To adhere the Pb reference value the diminishment of Pb level in soils is of high interest at present. As the demand for conversion of post-industrial lands continues to raise the remediation of Pb polluted soils represents one principal task to fulfill this requirement (Yousaf et al., 2016).
The established traditional techniques (e.g., washing, electrochemical methods, thermal processes, physical separation, stabilization/solidification, and burial) for clean-up of metal contaminated soils are generally expensive and harmful to soil microbial diversity (Rajkumar et al., 2012). Bioremediation has received increasing attention as it represents an eco-friendly and low-cost technology (Kotrba et al., 2009) using living plants (Janoušková and Pavlíková, 2010) or microorganisms (Brito et al., 2014) to remove toxic heavy metals (HM) in contaminated areas. Various plants growing in HM contaminated soils have developed specialized mechanisms for tolerating intracellular HM. Subcellular compartmentalization is an important way to eliminate the toxicity of HM in plants (Cobbett, 2003). Once taken up by plants, HM can exist in different chemical forms, including inorganic, water-soluble, pectate- and protein-integrated, undissolved phosphate and oxalate forms (Wu et al., 2005). For example, conversion of cadmium (Cd) into insoluble phosphate precipitates and pectate- or protein-bound forms is the primary means for reducing Cd mobility and toxicity in Nasturtium officinale (Wang J.B. et al., 2015). Copper (Cu) bound to the cell walls in fibrous roots of Malus sieversii mainly exists in phosphate and oxalate forms, which can explain some of the variation in Cu sensitivity in M. sieversii (Wang et al., 2016). Recently, Li et al. (2016) reported that the cell walls and intercellular spaces are the main location of Pb accumulation in the roots of Conyza canadensis. The cell walls restrict Pb uptake into plant roots and act as an important barrier to protect root cells (Wang Y. et al., 2015). Pb fixation by pectates and proteins in the cell walls and sequestration in the vacuoles were found to be responsible for Pb detoxification in Lolium perenne (He et al., 2015).
Arbuscular mycorrhizal fungi (AMF) are ubiquitous in terrestrial ecosystems (Sędzielewska-Toro and Delaux, 2016), forming symbiotic interactions with 80% of land plants. AMF can improve plant nutrient acquisition (Orrell and Bennett, 2013), enhance plant photosynthesis, and influence the fate of HM in both plants and the soil (Brito et al., 2014; Saia et al., 2015). Inoculation with Funneliformis mosseae resulted in a higher Pb tolerance of Eucalyptus grandis × urophylla and has been related to the retention of Pb in the roots, the binding of Pb to the cell walls, vacuolar compartmentalization of Pb in the soluble fraction, and increase in the proportion of less bioactive Pb (Liao et al., 2014). Thus, AMF may be used to advance plant-based environmental remediation by altering subcellular compartmentalization and chemical forms of HM in plants. Hence, it is necessary to select an appropriate HM-tolerant plants species that could form a symbiotic relationship with AMF.
Black locust (Robinia pseudoacacia L.) is a leguminous tree species widely planted on the Loess Plateau, China (Zhang et al., 2016). R. pseudoacacia is frequently found in HM contaminated areas and it may serve as an indicator of Pb pollution (Serbula et al., 2012). R. pseudoacacia plants are well grown and commonly colonized by AMF such as F. mosseae in the Qiandongshan lead–zinc polluted area (Yang et al., 2015b,c). Recent studies have shown that photosynthesis and antioxidant enzymes (e.g., superoxide dismutase and ascorbate peroxidases) in the leaves of R. pseudoacacia are enhanced by F. mosseae under Pb stress (Yang et al., 2015a). Additionally, F. mosseae is effective at accumulating Pb in plant root systems (Yang et al., 2015a). However, detailed information on the impact of F. mosseae on the subcellular compartmentalization and chemical forms of Pb in R. pseudoacacia under Pb stress are rare. To understand the efficiency of bioremediation of Pb in soils, there is a need to study the molecular mechanism how AMF colonization enhances Pb tolerance in woody legumes (Leguminosae) such as R. pseudoacacia.
One objective of our study was aimed to clarify whether F. mosseae is of significance for Pb tolerance in seedlings of R. pseudoacacia. For this purpose, inoculated as well as non-inoculated seedlings with F. mosseae were exposed to different Pb levels (0, 90, 900, and 3000 mg Pb kg-1 soil). The impact of Pb was studied on the basis of plant growth, gas exchange (CO2 assimilation, stomatal conductance for water vapor) and chlorophyll fluorescence. A second objective of this study was to give detailed insights in the detoxification mechanism of Pb from the molecular perspective, such as subcellular compartmentalization and conversion of Pb into inactive forms, and their alteration due to inoculation with F. mosseae. In this respect, the impact of F. mosseae on Pb tolerance was studied on the basis of Pb uptake in relation to the proportion of Pb located in different plant tissue, subcellular fractions and chemical forms.
Materials and Methods
Fungal Inoculum and Plant
Funneliformis mosseae (BGC XJ01A) spores were purchased from the Institute of Plant Nutrition and Resource, Beijing Academy of Agriculture and Forestry Sciences (Beijing, China). The fungus was propagated with fine sand for 3 months using Zea mays under greenhouse condition. The average colonization was 91.7%. The fungal inoculum consisted of sand, infected root fragments, external hyphae, and spores (∼26 spores g-1).
Robinia pseudoacacia L. is a Pb-tolerant tree species (Yang et al., 2015c). The seeds were collected from Northwest A&F University (Yangling, Shaanxi Province, China) in 2013. Seeds were surface sterilized with 10% H2O2 for 10 min, washed with distilled water for several times, and then soaked for 24 h before germinating on sterile filter paper in a Petri dish in an incubator at 28°C.
Growth Substrate
Soil used in this study was collected from the surface horizon (0–30 cm) on the campus of Northwest A&F University. The soil was air-dried, homogenized, and ground in a ceramic mill and passed through a 2 mm sieve before performing chemical analyses. After being mixed with washed fine sand (<2 mm), the substrate (sand/soil, 1:2 v/v) was autoclaved at 121°C for 2 h.
The properties of the soil were as follows (per kilogram of dry soil) after autoclaving: pH 7.66 (soil/water = 1:2.5, w/v), organic matter 14.85 g, ammonium-nitrogen 7.37 mg, nitrate-nitrogen 25.77 mg, available phosphorus 11.48 mg, and available potassium 128.96 mg, total Pb 6.58 mg. Measurements were performed according to the method described by Bao (2000).
The autoclaved substrate was divided into four subsamples. To three of the subsamples, Pb(NO3)2(aq) was added to produce substrates with different Pb levels, i.e., 90, 900, and 3000 mg kg-1 (mass of Pb/mass of dry soil) based on our pre-experiment (Yang et al., 2015c). An equivalent amount of distilled water was added to the control (0 mg Pb kg-1 soil). The four subsamples were supplied with an appropriate amount of NH4NO3 to compensate for the quantity of nitrate added as Pb(NO3)2. After addition of Pb solution, the growth substrate was allowed to stabilize for 1 month before used.
Experimental Design and Plant Culture
The experiment was setup as a 4 × 2 factorial design consisting of four Pb levels and one AMF inoculum and non-inoculated control which were arranged in a completely randomized design with 30 replicates per treatment combination. The experiment was performed from March to July 2014 in a greenhouse located at Northwest A&F University. Plants were kept at average room temperature (35/20°C, day/night) under a natural light regime during the period of plant growth. Soil moisture was determined by a soil moisture meter (Field Scout TDR 100, Spectrum Technologies Inc., Plainfield, IL, USA) and maintained at approximately 60% of field capacity by adding the amount of lost water to each pot daily.
Four uniform pre-germinated seeds were sown in each plastic pot (10 cm × 8 cm) with approximately 450 g growth substrate; each pot received 20 g fresh inoculum for mycorrhizal treatment or 20 g sterilized inoculum with 10 mL AMF-free filtrate (10 μm pore size) of unsterilized inoculum (soil:water = 1:10 w/v) as the non-mycorrhizal treatment (Sheng et al., 2008). The seedlings were thinned to one plant per pot 10 days after emergence. Plants were watered daily with tap water (35 mL) and supplemented with 0.25 × fresh Hoagland’s nutrient solution (35 mL) (Hoagland and Arnon, 1950) once a week throughout the growth stage.
After 4 months growth, the photosynthetic parameters and growth of six randomly selected plants per treatment were measured. The whole plants were washed with tap water to remove soil or dust deposits, and the roots were immersed in 20 mM Na2EDTA for 15 min to remove metal ions adhering to root surface (Chen et al., 2014). The plants were then washed with deionized water and dried with paper towels. Thereafter, the plants were separated into roots, stems, and leaves. The fresh roots (except for fine roots used for determination of AMF colonization), stems, and leaves were immediately frozen in liquid nitrogen (-196°C) and stored at -70°C for further analysis.
Physiological Measurements
Mycorrhizal Colonization and Growth Parameters
Mycorrhizal colonization (MC) was determined for fresh roots using the method described by Phillips and Hayman (1970). Then the MC was calculated according to Ban et al. (2015). Plant height and stem diameter (at 1 cm above the soil surface) were measured by precision straight edge (Deli 8200, Ningbo, China) and vernier caliper (Yifante ECV150C, Wuxi, China), respectively. Root dry weight, stem dry weight, and leaf dry weight were recorded after oven-drying to constant weight at 70°C. Total biomass was calculated as: root dry weight + stem dry weight + leaf dry weight.
Gas Exchange and Chlorophyll Fluorescence Parameters
Six healthy and functional leaves (youngest fully expanded from six different plants) were taken from each treatment and each leaf was measured five times for every photosynthetic parameter (Huang et al., 2011). Net CO2 assimilation rate (A), stomatal conductance to water vapor (gsw), intercellular CO2 concentration (Ci), and transpiration (E) were measured with a portable open-flow gas exchange system LI-6400 (LI-COR, USA) on a cloudless day from 9:00 to 11:30 a.m. in the glasshouse at 25°C. Automatic measurements were made under optimal conditions: photosynthetically active radiation 1000 ± 12 μmol m-2 s-1, CO2 concentration 350 ± 2 cm3 m-3, leaf temperature 28.0 ± 0.8°C, relative humidity 60%, ambient water vapor pressure 1.35 kPa, and flow rate of atmosphere 0.5 dm3 min-1.
Fluorescence assays were addressed to the same leaves as used for the photosynthetic measurements. The fluorescence parameters were measured at room temperature between 9:00 and 11:30 a.m. by a MINI-Imaging-PAM system (Imaging-PAM, Heinz Walz GmbH, Germany) as described by Gong et al. (2013). The seedlings were placed in darkness for 30 min and the minimal fluorescence in the dark-adapted state (Fo) was recorded. A saturating pulse of irradiation (2000 μmol m-2 s-1, 3 s) was applied to determine the maximal fluorescence (Fm) in the dark-adapted state. Then, the leaves were illuminated with actinic light (300 μmol m-2 s-1, 10 min) for evaluating the minimal fluorescence (Fo′) and maximal fluorescence (Fm′). The incident photosynthetically active irradiance (EPAR), effective photochemical efficiency of PSII (ΦPSII), and steady-state value of fluorescence (Fs) under actinic light were recorded. Using both light and dark fluorescence parameters, we calculated: (1) the maximal quantum yield of PSII in the dark-adapted state, Fv/Fm = (Fm - Fo)/Fm, (2) the effective photochemical efficiency of PSII, ΦPSII = (Fm′ - Fs)/Fm′, (3) the potential activity of PSII, Fv/Fo = (Fm - Fo)/Fo, (4) the photosynthetic electron transport rate, ETR = (Fm′ - Fs)/Fm′ × EPAR, (5) the photochemical quenching coefficient, qP = (Fm′ - Fs)/(Fm′ - Fo′), and (6) the non-photochemical quenching coefficient, qN = (Fm - Fm′)/Fm′.
Pb Analysis
To determine the fractions of Pb present in R. pseudoacacia, a fractionation procedure was adapted from a published protocol with slight modifications (Weigel and Jäger, 1980). Cells were separated by gradient centrifugation at 4°C into three different fractions: cell wall fraction (FI), organelle fraction (FII), and soluble fraction (FIII). In brief, 0.5 g of frozen tissues was homogenized in a pre-cold (4°C) extraction buffer [containing 50 mM Tris–HCl (pH 7.5), 250 mM sucrose, and 1.0 mM dithiothreitol (C4H10O2S2)] at the ratio of 1:10 (w/v) with a chilled mortar and a pestle. The homogenate was passed through a nylon cloth (80 μm mesh size) and liquid was squeezed from the residue. The residue on the cloth was washed twice with a homogenization buffer. The pooled washes, together with the first filtrate, were centrifuged at 300 × g for 30 s. The resulting pellet combined with the residue of nylon cloth filtration was designated as FI, containing mainly of cell walls and cell wall debris. The resulting supernatant solution was further centrifuged at 12,000 × g for 45 min. The pellet and supernatant solution were referred to as FII and FIII, respectively. The different fractions were dried at 70°C on an electric heating plate to a volume of approximately 1–2 mL and then wet-digested with concentrated acid HNO3/HClO4 (4:1, v/v). The recovery rate of Pb was calculated as: (cell wall fraction + organelle fraction + soluble fraction) Pb/total Pb × 100%. The proportion of Pb of the fraction was calculated with the following formula: Pb content in each fraction/total Pb content in the respective tissue × 100%.
To detect different chemical forms of Pb in plant tissues, we performed five extraction processes using for each a different extraction solvent (Wang et al., 2008), i.e., 80% ethanol (FEthanol; inorganic, soluble Pb), distilled water (Fd-H2O; organic, soluble Pb), 1 M sodium chloride (FNaCl; pectate and protein- Pb), 2% acetic acid (FHOAc; Pb phosphate), 0.6 M hydrochloric acid (FHCl; Pb oxalate), and Pb in residues (FResidue). Briefly, 0.5 g of frozen tissues were homogenized with a mortar and a pestle in liquid nitrogen (–196°C), diluted at a ratio of 1:50 (w/v) with the extraction solution, and then shaken for 22 h at 25°C. The resulting extracts were centrifuged at 5000 × g for 10 min. The precipitates were washed twice by re-suspending in the respective extraction medium, shaking at 25°C for 2 h, and centrifuging at 5000 × g for 10 min. The supernatants from each of the three repetitions were then pooled for each of the five extraction solutions. The resulting supernatant solvent from extraction solutions were evaporated on an electric heating plate to a volume of approximately 1–2 mL, followed by digestion with HNO3:HClO4 (4:1, v/v). To measure the Pb content in residues, plant materials were digested with HNO3–HClO4 (4:1, v/v) at the end of the sequential extraction. The recovery rate of Pb was calculated as: (FEthanol + FH2O + FNaCl + FHOAc + FHCl + FResidue) Pb/total Pb × 100%. The proportion of Pb of the chemical form was calculated as: Pb content of the chemical form/total Pb content in the respective tissue × 100%.
To determine the total Pb content in different tissues, oven-dried subsamples were ground into powder and sieved through a nylon mesh (100 μm). Then, 0.5 g aliquots of the samples were wet-digested in HNO3:HClO4 (4:1, v:v). Total Pb content as well as Pb content in different subcellular fractions and chemical forms was determined by atomic absorption spectrophotometry (Shimadzu AA-6300C, Kyoto, Japan). The flame composition was acetylene (flow rate 2 L min-1) and air (flow rate 15 L min-1). The working conditions of the instrument were as follows: current 7.5 mA, wavelength 217 nm, slit width 1.3 nm, burner height 7.5 mm, negative high voltage of photomultiplier tube 576 V, and auxiliary gas pressure 160 kPa.
Quality assurance and quality control for Pb in R. pseudoacacia was conducted using the standard reference material bush leaves (GBW07602), which was treated in the same way as the plant samples. The recovery for standard was approximately 95.3–108.5%. The standard solutions with different concentrations in the range of 1–10 μg mL-1 were aspirated in turn into flame and their absorbance values were recorded at 217 nm wavelength. The calibration curve was constructed by plotting on a linear graph paper using the absorbance of standards versus their concentrations. The correlation equations were defined as y = 0.019x + 0.004, R2 = 0.999. Reagent blanks and analytical duplicates were also used where appropriate, to ensure the accuracy and precision in the analysis. Pb content was determined three times for each sample and the relative standard deviation (SD) of Pb content was calculated (<4.0%).
Statistical Analysis
All values were expressed as mean ± SD (n = 6). The Kolmogorov–Smirnov test was applied to assess data normality and the Levene test for homogeneity of variance in SPSS 22.0 (SPSS Inc., Chicago, IL, USA). All the original datasets followed a normal distribution in this study. Potential differences among different Pb treatments were analyzed using two-way and three-way analysis of variance (ANOVA) followed by Duncan’s multiple comparison at P < 0.05. An independent t-test was performed to detect significant differences in plant growth and physiological parameters between the inoculated and non-inoculated controls within one Pb level. A two-factorial ANOVA and Pearson correlation analysis were performed to examine the influence of Pb exposure as well as the mycorrhizal treatment on physiological parameters as well as on the proportions of Pb in subcellular fractions and chemical forms and to detect possible correlations between these traits (n = 48). Graphs were drawn using SigmaPlot 10.0 (Systat Software, San Jose, CA, USA).
Results
Mycorrhizal Colonization and Plant Growth
None of the plants from non-inoculated treatments were colonized by F. mosseae. The symbiotic relationship between F. mosseae and R. pseudoacacia was well established in inoculated treatments irrespective of Pb treatment. Inoculated R. pseudoacacia showed 88.16% MC under control condition (0 mg Pb kg-1 soil), while low Pb treatment (90 mg Pb kg-1 soil) resulted in a slight increase in MC (93.56%). With increasing Pb level, MC of F. mosseae was significantly decreased and the lowest MC (49.77%) appeared at 3000 mg Pb kg-1 soil (Figure 1).
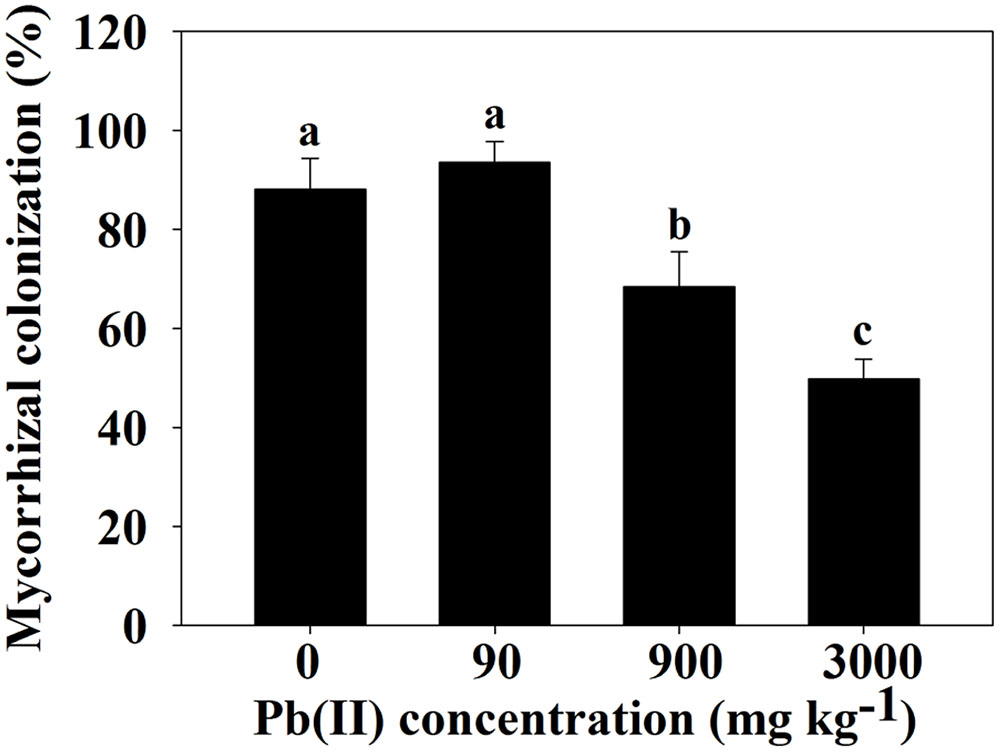
FIGURE 1. Colonization of Robinia pseudoacacia roots by Funneliformis mosseae in response to 0, 90, 900, and 3000 mg Pb kg-1 soil for 4 months. Shown are means ± SD (n = 6). Different lower case letters indicate significant differences among different Pb levels within inoculated treatment (P < 0.05; ANOVA with post hoc Duncan).
Under control conditions (0 mg Pb kg-1 soil), the growth parameters of R. pseudoacacia seedlings showed significant differences between non-inoculated and inoculated treatments. Inoculated seedlings showed significantly higher plant biomass (roots, stems, leaves, and total), plant height, and stem diameter than non-inoculated seedlings (Table 1). Compared to the controls, seedlings exposed to the lowest Pb level (90 mg Pb kg-1 soil) showed a significant improvement in plant growth, which was greater for inoculated seedlings than for non-inoculated seedlings (e.g., by 21% versus 16% in root dry weight). In contrast, exposure to higher Pb levels (900 and 3000 mg Pb kg-1 soil) resulted in a significant reduction in plant growth, which was greater for non-inoculated seedlings than for inoculated seedlings (e.g., by 41% versus 26% in root dry weight at 3000 mg Pb kg-1 soil; Figure 2).
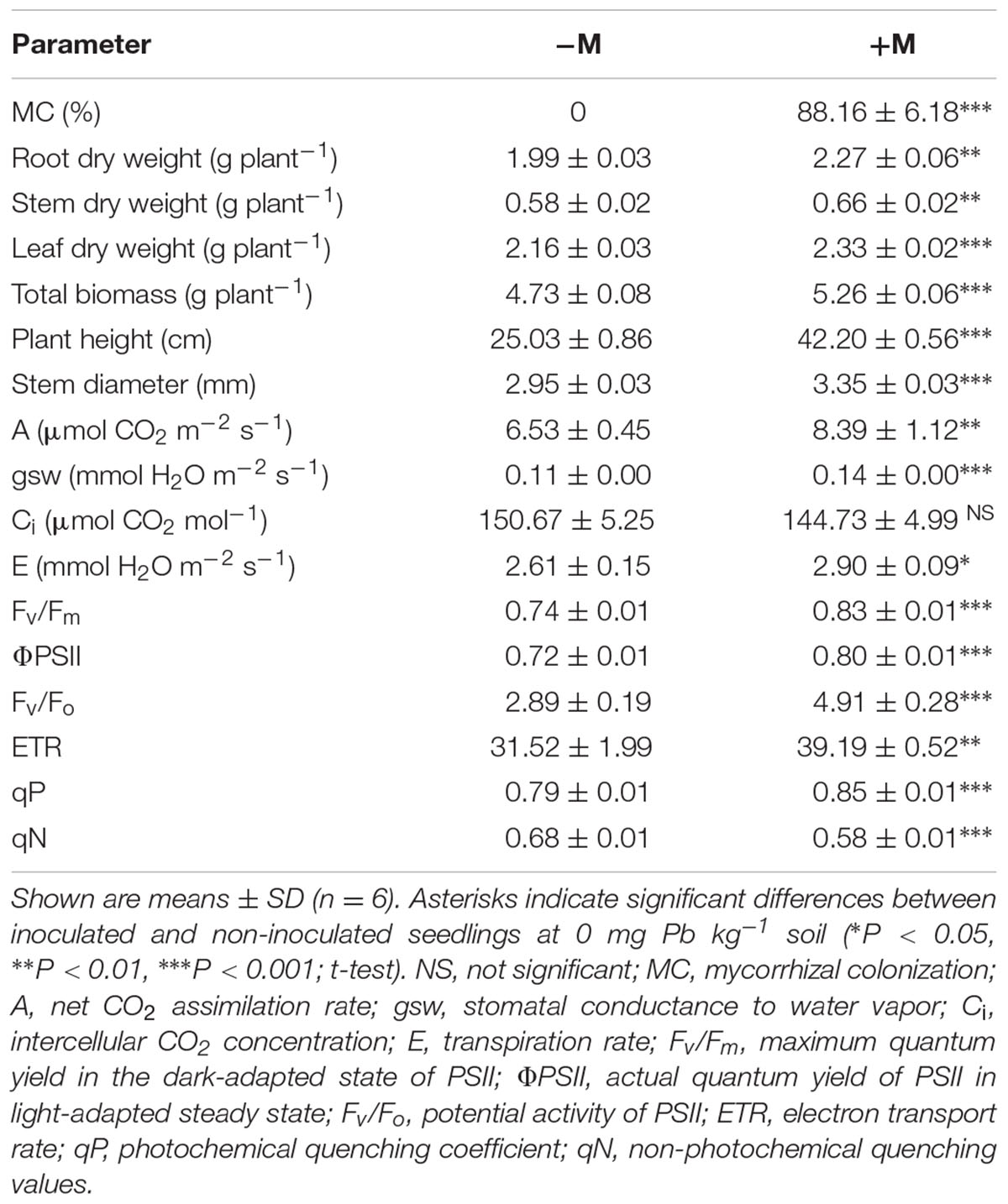
TABLE 1. Plant growth and physiological parameters of Robinia pseudoacacia seedlings with (+M) or without (-M) Funneliformis mosseae at 0 mg Pb kg-1 soil for 4 months.
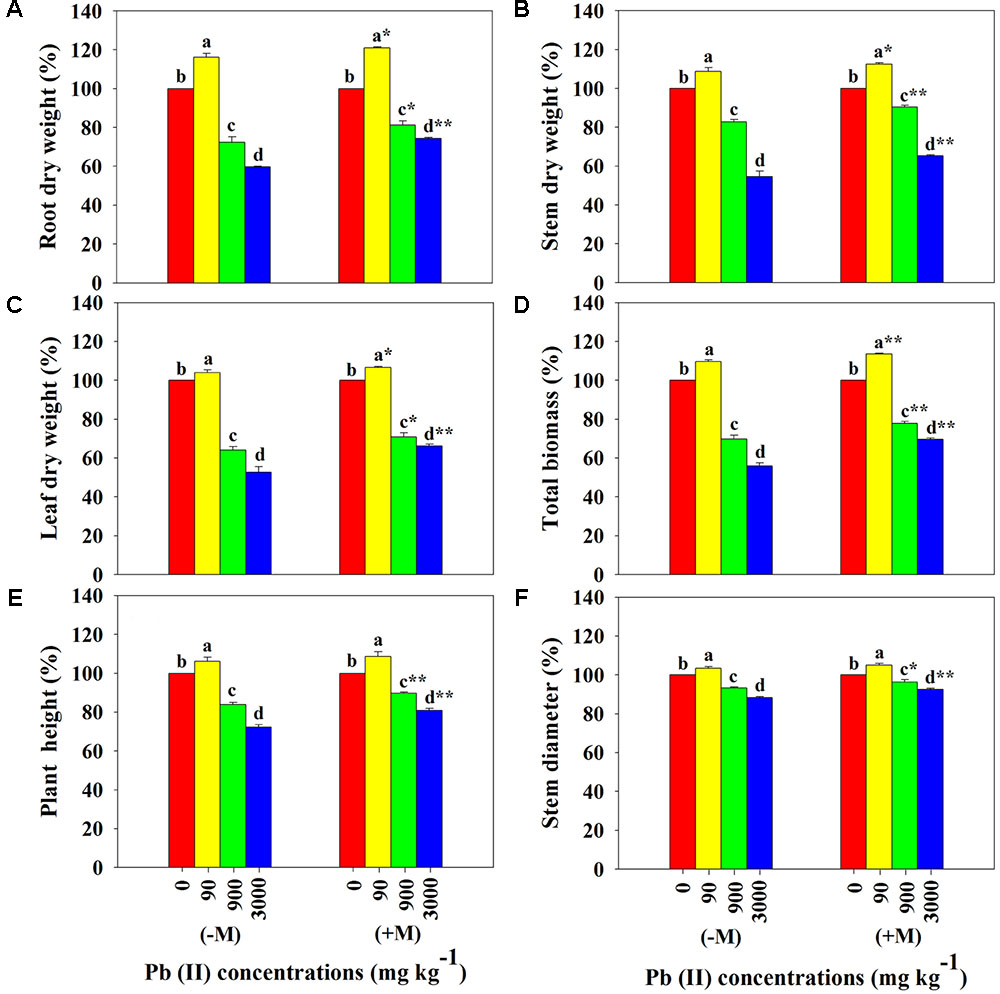
FIGURE 2. Relative differences in plant growth of Robinia pseudoacacia seedlings with (+M) or without (–M) Funneliformis mosseae in response to 0, 90, 900, and 3000 mg Pb kg-1 soil for 4 months. (A) Root dry weight, (B) stem dry weight, (C) leaf dry weight, (D) total biomass, (E) plant height, and (F) stem diameter. Shown are means ± SD (n = 6). Asterisks indicate significant differences between inoculated and non-inoculated seedlings within one Pb level (∗P < 0.05, ∗∗P < 0.01; t-test). Different lower case letters indicate significant differences among different Pb levels within inoculated or non-inoculated treatment (P < 0.05; ANOVA with post hoc Duncan).
Gas Exchange and Chlorophyll Fluorescence
In the leaves of control seedlings at 0 mg Pb kg-1 soil, gas exchange parameters (except for Ci) significantly differed between non-inoculated and inoculated treatments. Inoculated seedlings had significantly higher A, gsw, and E values compared to non-inoculated seedlings (Table 1). Compared to control data, A, gsw, and E were significantly increased at 90 mg Pb kg-1 soil and significantly reduced at 900 and 3000 mg Pb kg-1 soil in both inoculated and non-inoculated seedlings. However, the Ci values of both inoculated and non-inoculated seedlings were higher at 900 and 3000 mg Pb kg-1 soil compared to the respective seedlings at lower Pb level. Moreover, inoculated seedlings had significantly higher A, gsw, and E and lower Ci compared to non-inoculated seedlings at the highest Pb level (e.g., by 58, 22, 44, and 39% versus 86, 47, 71, and 72%, respectively, at 3000 mg Pb kg-1 soil; Figure 3).
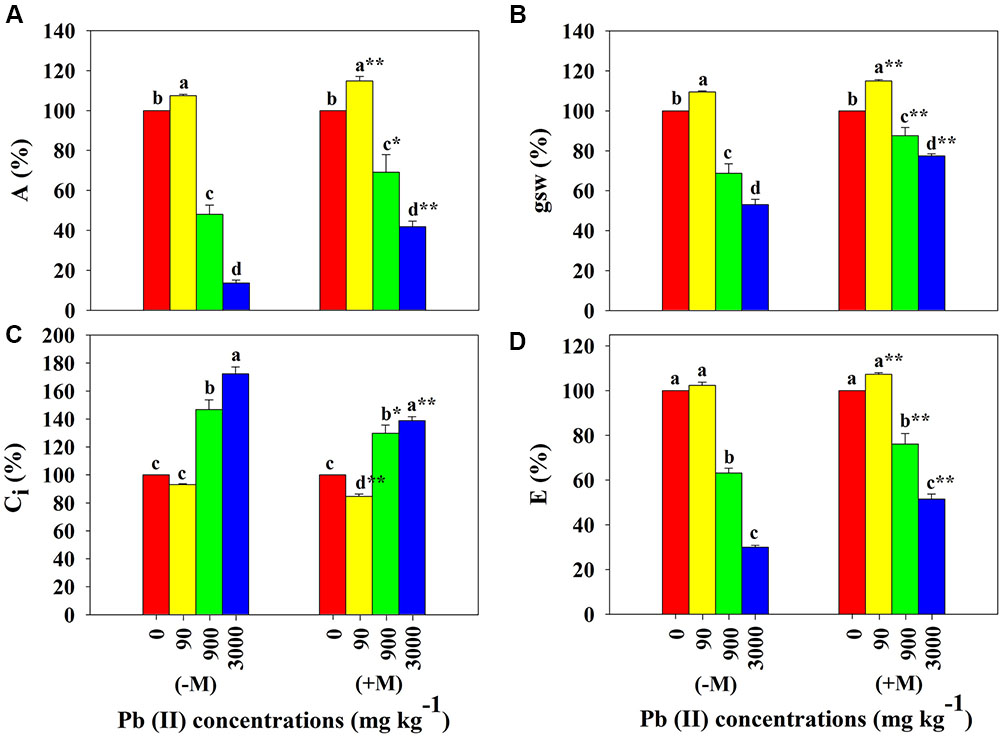
FIGURE 3. Relative differences in gas exchange in leaves of Robinia pseudoacacia seedlings with (+M) or without (–M) Funneliformis mosseae in response to 0, 90, 900, and 3000 mg Pb kg-1 soil for 4 months. (A) Net CO2 assimilation rate, A; (B) stomatal conductance to water vapor, gsw; (C) intercellular CO2 concentration, Ci; and (D) transpiration rate, E. Shown are means ± SD (n = 6). Asterisks indicate significant differences between inoculated and non-inoculated seedlings within one Pb level (∗P < 0.05, ∗∗P < 0.01; t-test). Different lower case letters indicate significant differences among different Pb levels within inoculated or non-inoculated treatment (P < 0.05; ANOVA with post hoc Duncan).
Under control conditions, we observed significantly higher Fv/Fm, ΦPSII, Fv/Fo, ETR, and qP, and significantly lower qN in the leaves of inoculated seedlings compared to non-inoculated seedlings (Table 1). Compared to control levels, Fv/Fm, ΦPSII, Fv/Fo, ETR, and qP of both inoculated and non-inoculated seedlings increased at 90 mg Pb kg-1 soil, followed by a significant reduction at 900 and 3000 mg Pb kg-1 soil. On the contrary, the qN showed a decrease at 90 mg Pb kg-1 soil, while an increase was found at 900 and 3000 mg Pb kg-1 soil. Inoculated seedlings showed significantly higher Fv/Fm, ΦPSII, Fv/Fo, ETR, and qP and significantly lower qN compared to non-inoculated seedlings at the highest Pb level (e.g., by 33, 28, 43, 31, 32, and 14% versus 39, 37, 75, 41, 39, and 28%, respectively, at 3000 mg Pb kg-1 soil; Figure 4 and Supplementary Figure S1).
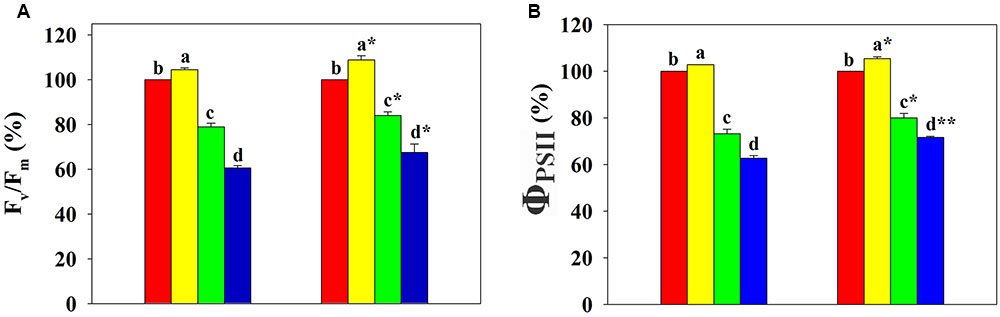
FIGURE 4. Relative differences in chlorophyll fluorescence in leaves of Robinia pseudoacacia seedlings with (+M) or without (–M) Funneliformis mosseae inoculation in response to 0, 90, 900, and 3000 mg Pb kg-1 soil for 4 months. (A) The maximum quantum yield in the dark-adapted state of PSII, Fv/Fm; and (B) the actual quantum yield of PSII in light-adapted steady state, ΦPSII. Shown are means ± SD (n = 6). Asterisks indicate significant differences between inoculated and non-inoculated seedlings within one Pb level (∗P < 0.05, ∗∗P < 0.01; t-test). Different lower case letters indicate significant differences among different Pb levels within inoculated or non-inoculated treatment (P < 0.05; ANOVA with post hoc Duncan).
Pb Uptake and Translocation
Under control conditions, no significant difference in Pb content between non-inoculated and inoculated treatments was detectable. Compared to the control conditions, exposure to Pb resulted in an increase in Pb content in all tissues for inoculated and non-inoculated seedlings in a concentration-dependent manner, with the highest Pb content found in the roots under all Pb treatments. Inoculated seedlings showed significantly higher Pb content in the roots and stems at 90, 900, and 3000 mg Pb kg-1 soil and significantly lower Pb content in the leaves at 3000 mg Pb kg-1 soil compared to non-inoculated seedlings under Pb exposure (Table 2).
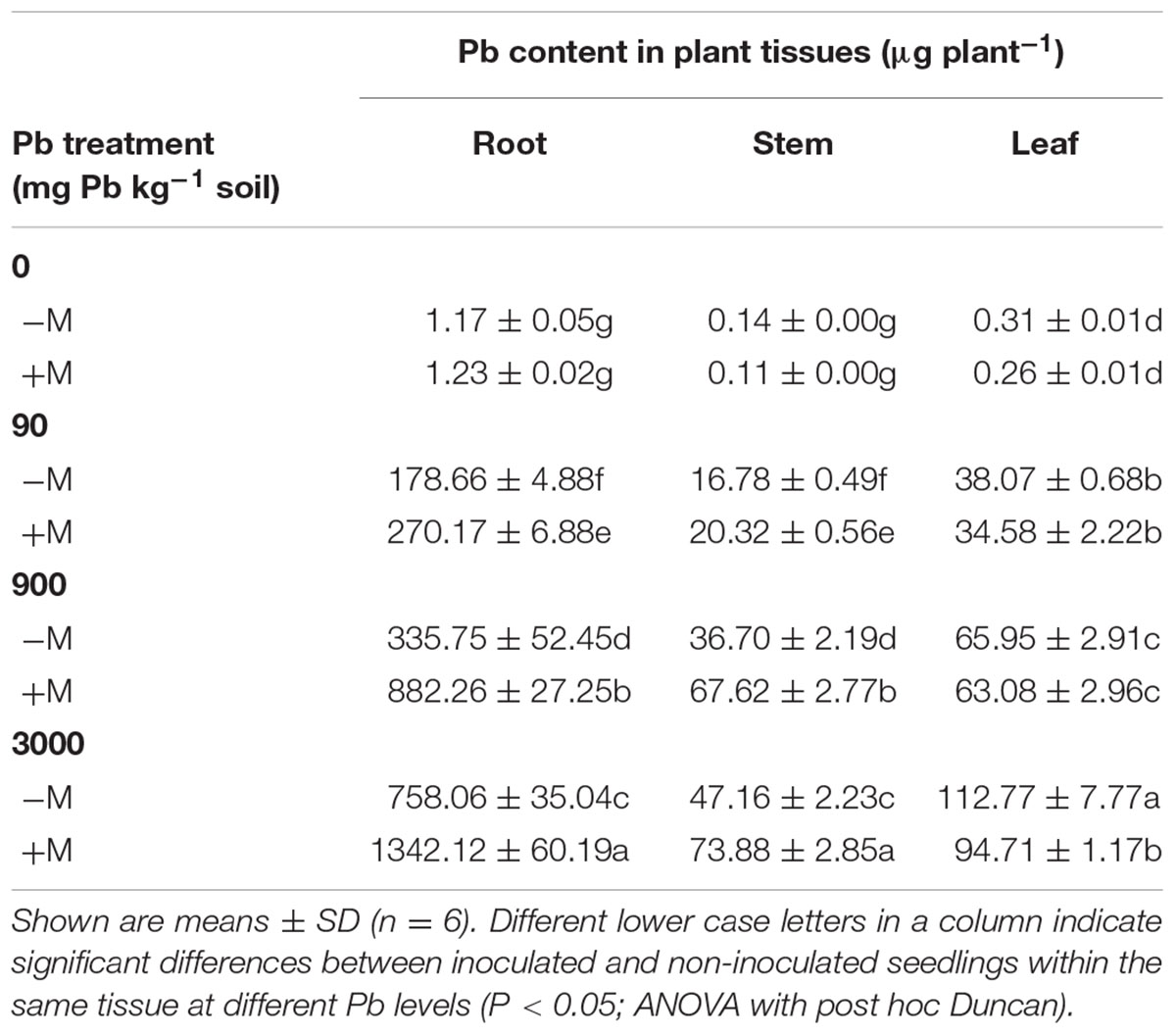
TABLE 2. Pb content in roots, stems, and leaves of Robinia pseudoacacia seedlings with (+M) or without (-M) Funneliformis mosseae exposed to 0, 90, 900, and 3000 mg Pb kg-1 soil for 4 months.
Subcellular Compartmentalization of Pb
The recovery rate of Pb was higher than 90% for most treatments. The proportion of Pb content in each subcellular fraction was related to the total Pb content accumulated in the respective tissue. Irrespective of Pb treatment or AMF inoculation, the proportion of Pb was highest in the FI fraction, ranging from 49 to 84% (Figure 5). Under control conditions, the proportions of Pb in the subcellular fractions of each tissue significantly differed between non-inoculated and inoculated seedlings. Both the FI and FIII were significantly higher and the FII was lower for inoculated seedlings compared to non-inoculated seedlings. Compared to control conditions, exposure to Pb (90, 900, and 3000 mg Pb kg-1 soil) resulted in a significant increase in the proportions of FI and FIII in all analyzed tissues in a concentration-dependent manner, and both fractions were significantly higher for inoculated seedlings than for non-inoculated seedlings (e.g., 62% versus 55% for FI in roots of seedlings at 90 mg Pb kg-1 soil). In contrast, the proportion of FII was significantly decreased in the tissues of seedlings under Pb exposure, and this fraction was significantly lower for inoculated seedlings compared to non-inoculated seedlings (e.g., 26% versus 39% in roots of seedlings at 90 mg Pb kg-1 soil; Figure 5).
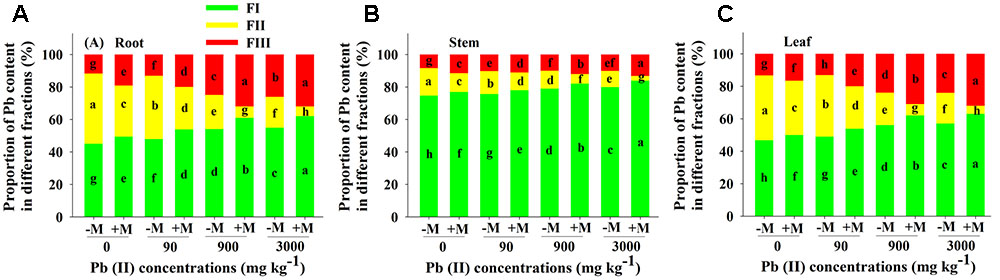
FIGURE 5. Proportion of Pb in different subcellular fractions in roots (A), stems (B), and leaves (C) of Robinia pseudoacacia seedlings with (+M) or without (–M) Funneliformis mosseae in response to 0, 90, 900, and 3000 mg Pb kg-1 soil for 4 months. Shown are means ± SD (n = 6). Different lower case letters within a column indicate significant differences between inoculated and non-inoculated seedlings within the same tissue among different Pb levels (P < 0.05; ANOVA with post hoc Duncan). Cells were separated by gradient centrifugation at 4°C into cell wall (FI), organelle (FII), and soluble (FIII) fractions.
Chemical Forms of Pb
The proportion of Pb content in each of chemical form was also related to the total Pb content accumulated in the respective tissue (Figure 6). For control seedlings (0 mg Pb kg-1 soil), the proportions of Pb in various chemical forms significantly differed between non-inoculated and inoculated seedlings. For example, inoculated seedlings showed significantly lower proportions (13–55%) of water-soluble Pb (including Fd-H2O and FEthanol) and significantly higher proportions (16–38%) of HOAc-exactable Pb (FHOAc) in the roots compared to non-inoculated seedlings.
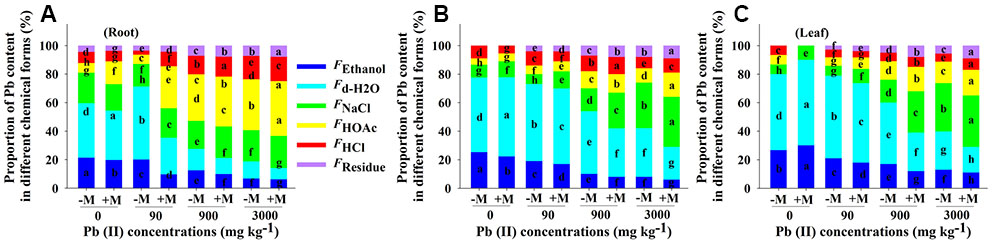
FIGURE 6. Proportion of Pb in different chemical forms in roots (A), stems (B), and leaves (C) of Robinia pseudoacacia seedlings with (+M) or without (–M) Funneliformis mosseae in response to 0, 90, 900, and 3000 mg Pb kg-1 soil for 4 months. Shown are means ± SD (n = 6). Different lower case letters within a column indicate significant differences between inoculated and non-inoculated seedlings within the same tissue among different Pb levels (P < 0.05; ANOVA with post hoc Duncan). Different chemical forms of Pb were sequentially extracted by 80% ethanol (FEthanol), deionized H2O Fd-H2O), 1 M NaCl (FNaCl), 2% HOAc (FHOAc), and 0.6 M HCl (FHCl). FResidue, Pb in residues.
Compared to controls, the lowest Pb level (90 mg Pb kg-1 soil) resulted in a significant increase in the proportion of Pb in Fd-H2O fraction in all analyzed tissues of the non-inoculated seedlings, whereas that of inoculated seedlings were significantly decreased. At higher Pb levels, the seedlings showed a significant reduction in the proportion of water-soluble Pb and a significant increase in the proportion of inactive Pb forms (including FNaCl, FHOAc, FHCl, and FResidue) in all tissues tested, irrespective of AMF inoculation. Additionally, inoculated seedlings retained significantly lower proportions of water-soluble Pb along with significantly higher proportions of inactive Pb forms compared to non-inoculated seedlings at 900 and 3000 mg Pb kg-1 soil (e.g., 21 and 79% versus 28 and 72%, respectively, at 900 mg Pb kg-1 soil, respectively; Figure 6).
Two-Factorial ANOVA and Correlation Analysis
The proportions of Pb content in different subcellular fractions and chemical forms were generally correlated with the relative differences in plant growth and physiological parameters of R. pseudoacacia seedlings (Supplementary Tables S1–S4). Additionally, Pb, AMF, and their interactions showed significant effects on plant growth and photosynthetic parameters (Supplementary Table S5).
Discussion
Pb has become ubiquitous in the soil due to natural deposits and intensive human activities (Fahr et al., 2013). AMF could offer an attractive system to facilitate plant-based environmental clean-up and strengthen plant tolerance to HM (Göhre and Paszkowski, 2006). In this study, we cultivated Pb-resistant R. pseudoacacia with F. mosseae exposed to different Pb levels in a pot experiment, in order to investigate the growth performance of R. pseudoacacia plants and Pb accumulation patterns in different tissues of R. pseudoacacia. The results indicate the potential of R. pseudoacacia for accumulation of Pb in phytoremediation and highlights the binding of inactive Pb forms to cell walls as a mechanism of Pb tolerance in plants with AMF.
Effects of AMF Symbiosis under Pb Stress
It is well documented that a symbiosis with AMF can improve photosynthesis, water use efficiency, and growth of plants (Chen et al., 2005; Zhu et al., 2011). In the current study, a symbiosis with F. mosseae showed significant positive effects on the gas exchange parameters, efficiency of PSII photochemistry, and plant growth in R. pseudoacacia seedlings under control conditions (0 mg Pb kg-1 soil; Figures 3, 4). This may be attributed to AMF regulation of the availability and uptake of water and nutrients by decreasing stomatal resistance, and increasing CO2 assimilation, and accelerating transpiration fluxes (Zhu et al., 2011). Low levels of HM (e.g., 50 μg L-1 Cu and Cd) have been shown to exert a stimulatory effect on plant growth of mycorrhizal (Acaulospora laevis and Glomus caledonium) and non-mycorrhizal Z. mays (Liao et al., 2003). Similar to these findings, across all Pb levels investigated in the present study, the lowest Pb level (90 mg Pb kg-1 soil) resulted in an increase in plant growth (e.g., higher total dry weight, plant height, and stem diameter; Figure 2) coinciding with an improvement of photosynthesis (e.g., higher A, gsw, E, Fv/Fm, and ΦPSII; Figures 3, 4) in R. pseudoacacia seedlings, regardless of mycorrhization with F. mosseae. This growth promotion effect of low Pb may be associated with stimulated metabolism (e.g., photosynthesis) and enzyme activities (e.g., superoxide dismutase and peroxidase) under low Pb stress (Schützendübel and Polle, 2002). Additionally, we found that seedlings inoculated with F. mosseae gained a higher performance in growth and photosynthesis than non-inoculated seedlings, consistent with previous findings in other plants. Chen et al. (2007) highlighted the importance of F. mosseae in accelerating growth of Coreopsis drummondii, Pteris vittata, and Trifolium repens in Cu mine tailings.
In this study, the application of higher Pb levels (900 and 3000 mg Pb kg-1 soil) resulted in an inhibition of photosynthesis, along with a decline in plant growth. The photosynthetic parameters of inoculated and non-inoculated seedlings exhibited similar patterns in response to Pb application; but the effect of AMF inoculation on photosynthetic parameters was strengthened at relatively low Pb level. In stressful conditions with HM, component disruption of the photosynthetic apparatus can occur and photosynthetic processes would be negatively affected in plants (Powles, 1984). Because of a significant reduction in ETR and ΦPSII in the leaves of R. pseudoacacia (Figure 4 and Supplementary Figure S1), it is assumed that higher Pb could destroy the PSII reaction center or disrupt electron transport in the photosynthetic apparatus. Nonetheless, we found that the photosynthetic parameters of inoculated seedlings were less impaired during exposure to higher Pb levels compared to non-inoculated seedlings. For instance, the relative difference in Fv/Fm was significantly lower for leaves of the inoculated seedlings compared to non-inoculated controls for the same Pb treatments (900 or 3000 mg Pb kg-1 soil). The higher performance of inoculated plants exposed to high Pb can be related to their improved availability of water and nutrients due to the AMF symbiosis. As we have normalized the physiological parameters of plants grown under Pb exposure to those under control conditions, the higher performance of inoculated plants under high Pb stress may also be attributed to other mechanisms that resist Pb uptake from soil and/or tolerate Pb within the cell (Wang Y. et al., 2015). AMF could facilitate Pb retention within the roots and stems, while reducing Pb accumulation in the leaves (Table 2). Thus, although significant Pb accumulation resulted in photosynthesis stress, it was seemed to be diminished for inoculated plants.
In this study, Pb accumulated in the roots, stems and leaves of R. pseudoacacia in a concentration-dependent manner, and Pb retention in the roots was significantly higher for inoculated seedlings compared to non-inoculated plants over the entire range of Pb levels applied (Table 2). AMF shows high tolerance to HM (Xu et al., 2012) and AMF symbiosis could create a more balanced environment for the roots by enriching HM at or in the mycorrhizal structure, decreasing free ion activity, and reducing toxicity (Chen et al., 2007). During symbiotic interaction between AMF and plants, hyphal network may functionally extend the root system of their hosts to take up HM from an enlarged soil volume (Göhre and Paszkowski, 2006). This explains the twofold higher Pb content in the roots of inoculated seedlings compared to non-inoculated seedlings of R. pseudoacacia (Table 2). The mitigation of negative impacts induced by HM could vary to a large extent, depending on HM species, soil HM level, fungal symbiotic partner, and/or plant environment (Hildebrandt et al., 2007). In the present study, the colonization of F. mosseae was markedly reduced in the roots of R. pseudoacacia at 900 and 3000 mg Pb kg-1 soil (Figure 1), indicating the negative effect of high Pb on the root colonization of AMF. A similar phenomenon was found by Weissenhorn and Leyval (1995) in the roots of Z. mays under Cd stress (up to 10 mg L-1), maybe due to limited hyphal extension and restricted spore germination at high HM levels (Weissenhorn et al., 1994). It is noteworthy that the root colonization rate by F. mosseae was not completely eliminated at 3000 mg Pb kg-1 soil in the current study. Thus, we assume that F. mosseae possesses a high tolerance toward Pb and it maybe a candidate for applications in reclamation of Pb polluted soils. This assumption needs to be tested by further long-term experiments.
Molecular Mechanism of Pb Tolerance
Under Pb stress, some molecular mechanisms are rapidly activated to minimize the potential toxicity of HM in plants (Wang Y. et al., 2015). Compartmentalization of HM is an important mechanism of detoxification/adaptation in plant cells (Zhao et al., 2015). After entering the cells, HM are bound to various subcellular compartments and exhibit different ecotoxicological significances. The cell walls, as the first protecting barrier, are mainly composed of polyoses (including cellulose, hemicellulose, and pectin) and proteins (Wang Y. et al., 2015). The negatively charged sites provided by functional groups, such as hydroxyl, carboxyl, amino, and aldehyde groups (Hu et al., 2012), can bind HM ions and limit their transport across the cell membrane (Bhatia et al., 2005; Arias et al., 2010). In the present study, a large proportion of Pb was found in the cell wall fraction in the roots, stems, and leaves of R. pseudoacacia seedlings across all Pb treatments, irrespective of AMF inoculation (Figure 5). This observation leads to the conclusion that the cell walls function as the primary barrier to Pb entry into the cytoplasm and limit organelles from suffering Pb toxicity in R. pseudoacacia. Further research is needed to evaluate the contribution of Pb sequestration in the cell walls of R. pseudoacacia roots.
The cell matrix between the cell walls and organelles can be regarded as an intracellular buffer (Arias et al., 2010). Soluble cellular components store HM, while they contain organo-ligands, mainly sulfur-rich peptides, organic alkali, and organic acids. Complexing metals with organo-ligands in these storage sites can decrease free ion activity and reduce HM toxicity (Bhatia et al., 2005). In the current study, the proportion of Pb in the soluble fraction was significantly increased in different tissues of R. pseudoacacia seedlings with increasing Pb level (Figure 5). This supports the hypothesis that complex formation of metals with organo-ligands is a molecular mechanism reducing HM toxicity in R. pseudoacacia seedlings. Meanwhile, the proportion of Pb in different subcellular fractions was significantly affected by AMF inoculation (Supplementary Table S5). Compared to the non-inoculated seedlings, R. pseudoacacia seedlings with F. mosseae showed significantly higher proportions of Pb in the cell wall and soluble fractions in the roots, stems, and leaves of R. pseudoacacia seedlings over the entire range of Pb level applied (Figure 5). This indicates that AMF facilitated the immobilization of Pb in the cell wall and soluble fractions of plant tissues, similar to the finding in Medicago sativa with Glomus intraradices under Cd stress (Wang et al., 2011). Moreover, the proportion of Pb in the subcellular compartments was highly associated with photosynthesis and plant growth of R. pseudoacacia seedlings (Supplementary Tables S1, S2). Thus, we conclude that the selective distribution of Pb in the cell wall and soluble fractions is a strategy for Pb tolerance and detoxification during the growth of R. pseudoacacia seedlings with AMF.
A high level of HM in plant tissue does not necessarily mean a high toxicity for a plant, since the HM may exist in chemical forms with low or no phytotoxicity (Fu et al., 2011). In the present study, we found that inoculation with F. mosseae promoted the conversion of Pb into inactivate forms (FNaCl, FHOAc, FHCl, and FResidue; Figure 6). The undissolved phosphate (FHOAc) and oxalate (FHCl) fractions of Pb have been already described as effective means in Pb detoxification (Zhang et al., 2013; Wang Y. et al., 2015) as they are less harmful than soluble Pb to plant cells. Wang et al. (2016) have indicated that the fibrous roots of apple tree showed largest proportions in the HOAc and HCl extractable Cu forms. Similarly, Zhao et al. (2015) showed that NaCl- and HOAc-extractable HM may be responsible for the adaptation of Porphyra yezoensis to Cd stress.
According to the high abundance of inactive forms of Pb in different tissues of inoculated plants, we therefore assume that (i) AMF may have a significant impact on the detoxification of Pb in plants through the transformation of Pb into the inactive forms, and (ii) transformation of Pb in inoculated seedlings may base on chelation of Pb by specific polar materials (e.g., hydroxyl or carboxyl groups) to form a non-toxic complex (Andrade et al., 2010), thereby contributing to improved plant growth and physiological performance. During exposure to high Pb, better photosynthesis and performance of PSII in the leaves of inoculated plants (e.g., higher gsw and Fv/Fm) may be attributed to the retention of Pb in different subcellular fractions and chemical forms in the roots, which could prevent the disruption of photosynthesis apparatus and membrane integrity. AMF symbiosis could improve the capacity of gas exchange, the efficiency of photochemistry and non-photochemistry of PSII, and regulate the energy bifurcation between photochemical and non-photochemical events in the leaves of seedlings.
Given its high stress tolerance and fast growth, R. pseudoacacia is considered suitable for soil and vegetation restoration in HM contaminated areas (Yang et al., 2015b,c). In this study, the majority of Pb was found to be retained in the roots of R. pseudoacacia under Pb stress, with a significantly higher accumulation in seedlings with F. mosseae. Compared with non-inoculated seedlings, the improved physiological parameters were highly associated with Pb compartmentalization in different chemical forms, including (1) increased proportion of Pb in the cell wall and soluble fractions, with the highest proportion of Pb in the cell wall fraction; and (2) increased proportion of inactive Pb, especially FNaCl and FHOAc, in plant tissues. These provide new insights into the role of AMF on Pb tolerance in woody legumes from a molecular perspective. From an ecological point of view, R. pseudoacacia inoculated with F. mosseae may be used for remediating Pb polluted soils.
Author Contributions
All authors participated in the conception of the topic after critically revising it for important intellectual content. LH and HZ wrote the manuscript. YS, YY, and HC assisted with data analysis, manuscript preparation, and revision. MT served as the primary investigator, conceived the project, and finalized the manuscript. All authors read and approved the final manuscript.
Funding
This research was supported by the National Natural Science Foundation of China (41671268, 31270639), the Program for Changjiang Scholars and Innovative Research Team in University of China (IRT1035), the Shaanxi Science and Technology Innovation Project Plan (2016KTCL02-07), and the Northwest A&F University Doctoral Research Start-Up Fund (Z109021503).
Conflict of Interest Statement
The authors declare that the research was conducted in the absence of any commercial or financial relationships that could be construed as a potential conflict of interest.
Acknowledgments
All authors thank Fengru Fang and Fei He for providing us with technical assistance.
Supplementary Material
The Supplementary Material for this article can be found online at: http://journal.frontiersin.org/article/10.3389/fpls.2017.00517/full#supplementary-material
References
Andrade, S. A. L., Silveira, A. P. D., and Mazzafera, P. (2010). Arbuscular mycorrhiza alters metal uptake and the physiological response of Coffea arabica seedlings to increasing Zn and Cu concentrations in soil. Sci. Total Environ. 408, 5381–5391. doi: 10.1016/j.scitotenv.2010.07.064
Arias, J. A., Peralta-Videa, J. R., Ellzey, J. T., Viveros, M. N., Ren, M., Mokgalaka-Matlala, N. S., et al. (2010). Plant growth and metal distribution in tissues of Prosopis juliflora-velutina grown on chromium contaminated soil in the presence of Glomus deserticola. Environ. Sci. Technol. 44, 7272–7279. doi: 10.1021/es1008664
Ban, Y., Xu, Z., Zhang, H., Chen, H., and Tang, M. (2015). Soil chemistry properties, translocation of heavy metals, and mycorrhizal fungi associated with six plant species growing on lead-zinc mine tailings. Ann. Microbiol. 65, 503–515. doi: 10.1007/s13213-014-0886-z
Bhatia, N. P., Walsh, K. B., and Baker, A. J. (2005). Detection and quantification of ligands involved in nickel detoxification in a herbaceous Ni hyperaccumulator Stackhousia tryonii Bailey. J. Exp. Bot. 56, 1343–1349. doi: 10.1093/jxb/eri135
Brito, I., Carvalho, M., Alho, L., and Goss, M. J. (2014). Managing arbuscular mycorrhizal fungi for bioprotection: Mn toxicity. Soil Biol. Biochem. 68, 78–84. doi: 10.1016/j.soilbio.2013.09.018
Burns, M. S., and Gerstenberger, S. L. (2014). Implications of the new centers for disease control and prevention blood lead reference value. Am. J. Public Health 104, e27–33. doi: 10.2105/AJPH.2013.301771
Chen, B. D., Zhu, Y. G., Duan, J., Xiao, X. Y., and Smith, S. E. (2007). Effects of the arbuscular mycorrhizal fungus Glomus mosseae on growth and metal uptake by four plant species in copper mine tailings. Environ. Pollut. 147, 374–380. doi: 10.1016/j.envpol.2006.04.027
Chen, L., Luo, S., Li, X., Wan, Y., Chen, J., and Liu, C. (2014). Interaction of Cd-hyperaccumulator Solanum nigrum L. and functional endophyte Pseudomonas sp. Lk9 on soil heavy metals uptake. Soil Biol. Biochem. 68, 300–308. doi: 10.1016/j.soilbio.2013.10.021
Chen, X., Wu, C., Tang, J., and Hu, S. (2005). Arbuscular mycorrhizae enhance metal lead uptake and growth of host plants under a sand culture experiment. Chemosphere 60, 665–671. doi: 10.1016/j.chemosphere.2005.01.029
Cobbett, C. (2003). Heavy metals and plants-model systems and hyperaccumulators. New Phytol. 159, 289–293. doi: 10.1046/j.1469-8137.2003.00832.x
Cuypers, A., Hendrix, S., dos Reis, R. A., De Smet, S., Deckers, J., Gielen, H., et al. (2016). Hydrogen peroxide, signaling in disguise during metal phytotoxicity. Front. Plant Sci. 7:470. doi: 10.3389/fpls.2016.00470
Datko-Williams, L., Wilkie, A., and Richmond-Bryant, J. (2014). Analysis of US soil lead (Pb) studies from 1970 to 2012. Sci. Total Environ. 468, 854–863. doi: 10.1016/j.scitotenv.2013.08.089
Fahr, M., Laplaze, L., Bendaou, N., Hocher, V., ElMzibri, M., Bogusz, D., et al. (2013). Effect of lead on root growth. Front. Plant Sci. 4:175. doi: 10.3389/fpls.2013.00175
Fu, X. P., Dou, C. M., Chen, Y. X., Chen, X. C., Shi, J. Y., Yu, M. G., et al. (2011). Subcellular distribution and chemical forms of cadmium in Phytolacca americana L. J. Hazard. Mater. 186, 103–107. doi: 10.1016/j.jhazmat.2010.10.122
Göhre, V., and Paszkowski, U. (2006). Contribution of the arbuscular mycorrhizal symbiosis to heavy metal phytoremediation. Planta 223, 1115–1122. doi: 10.1007/s00425-006-0225-0
Gong, M., Tang, M., Chen, H., Zhang, Q., and Feng, X. (2013). Effects of two Glomus species on the growth and physiological performance of Sophora davidii seedlings under water stress. New For. 44, 399–408. doi: 10.1007/s11056-012-9349-1
He, S., He, Z., Wu, Q., Wang, L., and Zhang, X. (2015). Effects of GA3 on plant physiological properties, extraction, subcellular distribution and chemical forms of Pb in Lolium perenne. Int. J. Phytoremediation 17, 1153–1159. doi: 10.1080/15226514.2015.1045124
Henry, H., Naujokas, M. F., Attanayake, C., Basta, N. T., Cheng, Z., Hettiarachchi, G. M., et al. (2015). Bioavailability-based in situ remediation to meet future lead (Pb) standards in urban soils and gardens. Environ. Sci. Technol. 49, 8948–8958. doi: 10.1021/acs.est.5b01693
Hildebrandt, U., Regvar, M., and Bothe, H. (2007). Arbuscular mycorrhiza and heavy metal tolerance. Phytochemistry 68, 139–146. doi: 10.1016/j.phytochem.2006.09.023
Hoagland, D. R., and Arnon, D. I. (1950). The water-culture method for growing plants without soil. Circ. Calif. Agric. Exp. Stat. 347, 32.
Hu, R., Sun, K., Su, X., Pan, Y. X., Zhang, Y. F., and Wang, X. P. (2012). Physiological responses and tolerance mechanisms to Pb in two xerophils: Salsola passerina Bunge and Chenopodium album L. J. Hazard. Mater. 205, 131–138. doi: 10.1016/j.jhazmat.2011.12.051
Huang, Z., Zou, Z., He, C., He, Z., Zhang, Z., and Li, J. (2011). Physiological and photosynthetic responses of melon (Cucumis melo L.) seedlings to three Glomus species under water deficit. Plant Soil 339, 391–399. doi: 10.1007/s11104-010-0591-z
Janoušková, M., and Pavlíková, D. (2010). Cadmium immobilization in the rhizosphere of arbuscular mycorrhizal plants by the fungal extraradical mycelium. Plant Soil 332, 511–520. doi: 10.1007/s11104-010-0317-2
Kotrba, P., Najmanova, J., Macek, T., Ruml, T., and Mackova, M. (2009). Genetically modified plants in phytoremediation of heavy metal and metalloid soil and sediment pollution. Biotechnol. Adv. 27, 799–810. doi: 10.1016/j.biotechadv.2009.06.003
LaBelle, S. J., Lindahl, P. C., Hinchman, R. R., Ruskamp, J., and McHugh, K. (1987). Pilot Study of the Relationship of Regional Road Traffic to Surface-Soil Lead Levels in Illinois (No. ANL/ES-154). Lemont, IL: Argonne National Lab.
Lantzy, R. J., and Mackenzie, F. T. (1979). Atmospheric trace metals: global cycles and assessment of man’s impact. Geochim. Cosmochim. Acta 43, 511–525. doi: 10.1016/0016-7037(79)90162-5
Li, Y., Zhou, C., Huang, M., Luo, J., Hou, X., Wu, P., et al. (2016). Lead tolerance mechanism in Conyza canadensis: subcellular distribution, ultrastructure, antioxidative defense system, and phytochelatins. J. Plant Res. 129, 251–262. doi: 10.1007/s10265-015-0776-x
Liao, J. P., Lin, X. G., Cao, Z. H., Shi, Y. Q., and Wong, M. H. (2003). Interactions between arbuscular mycorrhizae and heavy metals under sand culture experiment. Chemosphere 50, 847–853. doi: 10.1016/S0045-6535(02)00229-1
Liao, Y. J., Tian, Y., Wang, F., and Wang, W. S. (2014). Study on the Pb-tolerance mechanism of Eucalyptus under the role of arbuscular mycorrhizal fungi. Genomics Appl. Biol. 3, 027.
Orrell, P., and Bennett, A. E. (2013). How can we exploit above-belowground interactions to assist in addressing the challenges of food security. Front. Plant Sci. 4:432. doi: 10.3389/fpls.2013.00432
Phillips, J. M., and Hayman, D. S. (1970). Improved procedures for clearing roots and staining parasitic and vesicular-arbuscular mycorrhizal fungi for rapid assessment of infection. Trans. Br. Mycol. Soc. 55, 158–IN18.
Powles, S. B. (1984). Photoinhibition of photosynthesis induced by visible light. Annu. Rev. Plant Physiol. 35, 15–44. doi: 10.1146/annurev.pp.35.060184.000311
Rajkumar, M., Sandhya, S., Prasad, M. N. V., and Freitas, H. (2012). Perspectives of plant-associated microbes in heavy metal phytoremediation. Biotechnol. Adv. 30, 1562–1574. doi: 10.1016/j.biotechadv.2012.04.011
Saia, S., Rappa, V., Ruisi, P., Abenavoli, M. R., Sunseri, F., Giambalvo, D., et al. (2015). Soil inoculation with symbiotic microorganisms promotes plant growth and nutrient transporter genes expression in durum wheat. Front. Plant Sci. 6:815. doi: 10.3389/fpls.2015.00815
Schützendübel, A., and Polle, A. (2002). Plant responses to abiotic stresses: heavy metal-induced oxidative stress and protection by mycorrhization. J. Exp. Bot. 53, 1351–1365. doi: 10.1093/jexbot/53.372.1351
Sędzielewska-Toro, K., and Delaux, P. M. (2016). Mycorrhizal symbioses: today and tomorrow. New Phytol. 209, 917–920. doi: 10.1111/nph.13820
Serbula, S. M., Miljkovic, D. D., Kovacevic, R. M., and Ilic, A. A. (2012). Assessment of airborne heavy metal pollution using plant parts and top soil. Ecotox. Environ. Saf. 76, 209–214. doi: 10.1016/j.ecoenv.2011.10.009
Sheng, M., Tang, M., Chen, H., Yang, B., Zhang, F., and Huang, Y. (2008). Influence of arbuscular mycorrhizae on photosynthesis and water status of maize plants under salt stress. Mycorrhiza 18, 287–296. doi: 10.1007/s00572-008-0180-7
Wang, J. B., Su, L. Y., Yang, J. Z., Yuan, J. G., Yin, A. G., Qiu, Q., et al. (2015). Comparisons of cadmium subcellular distribution and chemical forms between low-Cd and high-Cd accumulation genotypes of watercress (Nasturtium officinale L. R. Br.). Plant Soil 396, 325–337. doi: 10.1007/s11104-015-2580-8
Wang, Q. Y., Liu, J. S., and Hu, B. (2016). Integration of copper subcellular distribution and chemical forms to understand copper toxicity in apple trees. Environ. Exp. Bot. 123, 125–131. doi: 10.1016/j.envexpbot.2015.11.014
Wang, X., Liu, Y., Zeng, G., Chai, L., Song, X., Min, Z., et al. (2008). Subcellular distribution and chemical forms of cadmium in Bechmeria nivea (L.) Gaud. Environ. Exp. Bot. 62, 389–395. doi: 10.1016/j.envexpbot.2007.10.014
Wang, Y., Huang, J., and Gao, Y. (2011). Arbuscular mycorrhizal colonization alters subcellular distribution and chemical forms of cadmium in Medicago sativa L. and resists cadmium toxicity. PLoS ONE 7:e48669. doi: 10.1371/journal.pone.0048669
Wang, Y., Shen, H., Xu, L., Zhu, X., Li, C., Zhang, W., et al. (2015). Transport, ultrastructural localization, and distribution of chemical forms of lead in radish (Raphanus sativus L.). Front. Plant Sci. 6:293. doi: 10.3389/fpls.2015.00293
Weigel, H. J., and Jäger, H. J. (1980). Subcellular distribution and chemical form of cadmium in bean plants. Plant Physiol. 65, 480–482. doi: 10.1104/pp.65.3.480
Weissenhorn, I., Glashoff, A., Leyval, C., and Berthelin, J. (1994). Differential tolerance to Cd and Zn of arbuscular mycorrhizal (AM) fungal spores isolated from heavy metal-polluted and unpolluted soils. Plant Soil 167, 189–196. doi: 10.1007/BF00007944
Weissenhorn, I., and Leyval, C. (1995). Root colonization of maize by a Cd-sensitive and a Cd-tolerant Glomus mosseae and cadmium uptake in sand culture. Plant Soil 175, 233–238. doi: 10.1007/BF00011359
Wu, F. B., Dong, J., Qian, Q. Q., and Zhang, G. P. (2005). Subcellular distribution and chemical form of Cd and Ca-Zn interaction in different barley genotypes. Chemosphere 60, 1437–1446. doi: 10.1016/j.chemosphere.2005.01.071
Xu, Z. Y., Tang, M., Chen, H., Ban, Y. H., and Zhang, H. H. (2012). Microbial community structure in the rhizosphere of Sophora viciifolia grown at a lead and zinc mine of northwest China. Sci. Total Environ. 435, 453–464. doi: 10.1016/j.scitotenv.2012.07.029
Yadav, S. K. (2010). Heavy metals toxicity in plants: an overview on the role of glutathione and phytochelatins in heavy metal stress tolerance of plants. S. Afr. J. Bot. 76, 167–179. doi: 10.1016/j.sajb.2009.10.007
Yang, Y., Han, X., Liang, Y., Ghosh, A., Chen, J., and Tang, M. (2015a). The combined effects of arbuscular mycorrhizal fungi (AMF) and Lead (Pb) stress on Pb accumulation, plant growth parameters, photosynthesis, and antioxidant enzymes in Robinia pseudoacacia L. PLoS ONE 10:e0145726. doi: 10.1371/journal.pone.0145726
Yang, Y., Liang, Y., Ghosh, A., Song, Y., Chen, H., and Tang, M. (2015b). Assessment of arbuscular mycorrhizal fungi status and heavy metal accumulation characteristics of tree species in a lead-zinc mine area: potential applications for phytoremediation. Environ. Sci. Pollut. Res. Int. 22, 13179–13193. doi: 10.1007/s11356-015-4521-8
Yang, Y., Song, Y., Scheller, H. V., Ghosh, A., Ban, Y., Chen, H., et al. (2015c). Community structure of arbuscular mycorrhizal fungi associated with Robinia pseudoacacia in uncontaminated and heavy metal contaminated soils. Soil Biol. Biochem. 86, 146–158. doi: 10.1016/j.soilbio.2015.03.018
Yousaf, B., Liu, G., Wang, R., Imtiaz, M., Rizwan, M. S., Zia-ur-Rehman, M., et al. (2016). The importance of evaluating metal exposure and predicting human health risks in urban-periurban environments influenced by emerging industry. Chemosphere 150, 79–89. doi: 10.1016/j.chemosphere.2016.02.007
Zhang, H., Liu, Z., Chen, H., and Tang, M. (2016). Symbiosis of arbuscular mycorrhizal fungi and Robinia pseudoacacia L. improves root tensile strength and soil aggregate stability. PLoS ONE 11:e0153378. doi: 10.1371/journal.pone.0153378
Zhang, Z., Liu, C., Wang, X., and Shi, G. (2013). Cadmium-induced alterations in morphophysiology of two peanut cultivars differing in cadmium accumulation. Acta Physiol. Plant. 35, 2105–2112. doi: 10.1007/s11738-013-1247-4
Zhao, Y., Wu, J., Shang, D., Ning, J., Zhai, Y., Sheng, X., et al. (2015). Subcellular distribution and chemical forms of cadmium in the edible seaweed, Porphyra yezoensis. Food Chem. 168, 48–54. doi: 10.1016/j.foodchem.2014.07.054
Keywords: arbuscular mycorrhizal fungus, symbiosis, bioremediation, Pb, plant tolerance
Citation: Huang L, Zhang H, Song Y, Yang Y, Chen H and Tang M (2017) Subcellular Compartmentalization and Chemical Forms of Lead Participate in Lead Tolerance of Robinia pseudoacacia L. with Funneliformis mosseae. Front. Plant Sci. 8:517. doi: 10.3389/fpls.2017.00517
Received: 31 May 2016; Accepted: 23 March 2017;
Published: 10 April 2017.
Edited by:
Mohamed Hijri, Université de Montréal, CanadaReviewed by:
Doerte Randewig, Swedish University of Agricultural Sciences, SwedenAmadou Bâ, Université des Antilles, France
Copyright © 2017 Huang, Zhang, Song, Yang, Chen and Tang. This is an open-access article distributed under the terms of the Creative Commons Attribution License (CC BY). The use, distribution or reproduction in other forums is permitted, provided the original author(s) or licensor are credited and that the original publication in this journal is cited, in accordance with accepted academic practice. No use, distribution or reproduction is permitted which does not comply with these terms.
*Correspondence: Ming Tang, dGFuZ21AbndzdWFmLmVkdS5jbg==