- Faculty of Biotechnology and Horticulture, Institute of Plant Biology and Biotechnology, University of Agriculture in Krakow, Krakow, Poland
The prevalence of non-autonomous class II transposable elements (TEs) in plant genomes may serve as a tool for relatively rapid and low-cost development of gene-associated molecular markers. Miniature inverted-repeat transposable element (MITE) copies inserted within introns can be exploited as potential intron length polymorphism (ILP) markers. ILPs can be detected by PCR with primers anchored in exon sequences flanking the target introns. Here, we designed primers for 209 DcSto (Daucus carota Stowaway-like) MITE insertion sites within introns along the carrot genome and validated them as candidate ILP markers in order to develop a set of markers for genotyping the carrot. As a proof of concept, 90 biallelic DcS-ILP markers were selected and used to assess genetic diversity of 27 accessions comprising wild Daucus carota and cultivated carrot of different root shape. The number of effective alleles was 1.56, mean polymorphism informative content was 0.27, while the average observed and expected heterozygosity was 0.24 and 0.34, respectively. Sixty-seven loci showed positive values of Wright's fixation index. Using Bayesian approach, two clusters comprising four wild and 23 cultivated accessions, respectively, were distinguished. Within the cultivated carrot gene pool, four subclusters representing accessions from Chantenay, Danvers, Imperator, and Paris Market types were revealed. It is the first molecular evidence for root-type associated diversity structure in western cultivated carrot. DcS-ILPs detected substantial genetic diversity among the studied accessions and, showing considerable discrimination power, may be exploited as a tool for germplasm characterization and analysis of genome relationships. The developed set of DcS-ILP markers is an easily accessible molecular marker genotyping system based on TE insertion polymorphism.
Introduction
Transposable elements (TEs) are segments of DNA that can move themselves to new chromosomal location. They are prevalent in the genomes of both prokaryotes and eukaryotes, and account for a great subsection of the genetic variation in plants and animals. Some plant genomes are composed of transposable elements in more than two thirds, as the 77% of the maize genome (Meyers et al., 2001). Miniature inverted-repeat transposable elements (MITEs) are a special type of class II non-autonomous elements with a maximum of a few hundred base pairs in size (Hua-Van et al., 2005). Although they were first discovered in plant genomes (Bureau and Wessler, 1992, 1994), they have been also identified in a wide range of animal, eubacteria and archea genomes (Brügger et al., 2002; Feschotte et al., 2002). The two largest MITE families, Stowaway and Tourist, were identified as members of the Tc1/Mariner and the PIF/Harbinger superfamilies, respectively (Jiang et al., 2004). Stowaway MITEs were first described in the maize genome (Bureau and Wessler, 1994) as less than 500 bp long, forming a 2 bp TA TSD upon insertion. MITEs are usually present in many thousand copies per genome. 22,000 identified Stowaway MITEs were classified into 34 families in the Oryza sativa genome (Feschotte et al., 2003), whereas 18,000 MITE insertions were classified into 18 families in the Triticum spp. genome (Yaakov et al., 2013).
The ubiquity, genome-wide distribution and high copy numbers have provided genetic markers from both class I and class II TEs (Kumar and Hirochika, 2001). The abundance of MITE copies makes them highly useful source of polymorphism. To date, MITE Transposon Display (MITE-TD) and Inter-MITE Polymorphism (IMP) techniques exploiting the TIR sequences in Oryza sativa, Zea mays, Sorghum bicolor, Hordeum vulgare, and Daucus carota MITEs, have been developed (Chang et al., 2001; Park et al., 2003; Casa et al., 2004; Lee et al., 2005; Grzebelus et al., 2007). Some Stowaway MITEs identified to date were described as being preferentially inserted or retained in genic regions (Casa et al., 2000; Jiang et al., 2003). However, even though 54% of DcSto insertion sites in the carrot genome were located less than 2 kb away from or inside the coding sequences, random distribution of DcSto rather than preferential insertions around genes was proposed (Iorizzo et al., 2016).
Insertions within introns may provide a significant polymorphism. Intron polymorphisms, particularly intron length polymorphisms (ILPs), can be exploited as genetic markers used for gene mapping (Wydner et al., 1994) and population genetic surveys (Lessa, 1992). ILP takes advantage of the different rate of evolution of exons and introns that can result in conserved exon nucleotide sequences adjoined to more variable intron sequences. ILP can be detected by the polymerase chain reaction with a pair of primers anchored in the exons flanking the intron of interest (Wang et al., 2005). ILP markers are unique due to their gene-specifity, codominancy, conveniency, reliability and cost-efficiency. Furthermore, ILPs are characterized by high transferability among related plant species (Yang et al., 2007; Gupta et al., 2011). To date, studies on the development of ILP markers in plants have been restricted to few species (Wang et al., 2005; Huang et al., 2008; Chen et al., 2010; Gupta et al., 2011, 2012; Li et al., 2013; Muthamilarasan et al., 2014).
Carrot is the most widely grown member of Apiaceae family. Its progenitor, wild Daucus carota L., is a plant commonly occurring in the temperate climatic zones. To date, a range molecular tools facilitating genome analysis in context of evolutionary history of wild and cultivated carrot have been developed, i.e., DArT, SSR, and SNP markers (Cavagnaro et al., 2011; Iorizzo et al., 2013; Grzebelus et al., 2014) and a set of ca. 30 resequenced genomes (Iorizzo et al., 2016). The analyses showed clear evidence for the carrot germplasm separation into three distinct groups of wild, western cultivated (European and American germplasm) and eastern cultivated (Asian germplasm) carrot. The majority of modern cultivars belong to the western group. Several varietal types were distinguished within western carrots, based primarily on the storage root shape and size (Prohens and Nuez, 2008). Despite apparent phenotypic differences, previous studies have indicated absence of any apparent population structure in western carrots, suggesting no significant genetic separation among these varietal types (Bradeen et al., 2002; Iorizzo et al., 2013).
In this study, we performed (1) a genome-wide search for DcSto (Daucus carota Stowaway-like) MITE insertion-based intron length polymorphism markers, and (2) validation of candidate ILP markers in order to develop a panel for genotyping the carrot by means of applying a simple, cost- and time-efficient polymerase chain reaction.
Materials and Methods
Plant Materials
Twenty eight carrot accessions comprising four wild carrots of different origin, 23 western type carrot cultivars representing four types of root shape and a DH1 plant (Iorizzo et al., 2016) as the reference, were used for ILP validation (Table 1). Total genomic DNA was isolated from fresh young leaves using commercial DNeasy Plant Mini Kit (Qiagen) and used as the template for PCR amplification.
Development of ILP Markers
Coordinates of 4028 DcSto insertions belonging to 14 families were compared to coordinates of ca. 32 thousand genes annotated in the carrot reference DH1 genome assembly (Iorizzo et al., 2016; NCBI accession LNRQ01000000). 609 gene-associated DcSto insertion sites localized in introns were identified, of which 209 were manually selected for development of ILP markers. The criteria for initial selection were as followed: insertion sites were (1) free from any other annotated repetitive sequences, (2) present in introns not longer than 3.7 Kb, and (3) evenly distributed over each chromosome. Primer3 (Untergasser et al., 2012) and Primer-BLAST (Ye et al., 2012) were used to design PCR primer pairs anchored in exons flanking introns harboring the selected DcSto insertions. Primer pairs were designed to amplify fragments in a 400–3,700-bp range. The optimal annealing temperature was set to 58°C; and the size and GC content ranged from 18 to 23 bases and 40 to 60%, respectively.
Validation and Evaluation of DcS-ILP Markers
Candidate ILP markers were selected for experimental evaluation. Amplification was carried out in a 10 μL total volume containing 20 ng of genomic DNA, 0.5 μM each of forward and reverse primer, 0.25 mM of each dNTP (Thermo Fisher Scientific), 0.5 U Taq DNA polymerase (Thermo Fisher Scientific) and 1x Taq buffer. The PCR amplifications were performed in an Eppendorf MasterCycler Gradient using the following thermal profile: 94°C (120 s), 30 cycles of 94°C (30 s), 56°C (30 s), 68°C (120 s) and final step of 68°C (600 s). For primers generating ambiguous profiles, the annealing temperature was adjusted to 58, 59, or 60°C. PCR products were separated in 1% agarose gels run in 1x Tris-borate-EDTA buffer (pH 8.0) at a constant current of 5V/cm for about 2 h, stained with Midori Green (Nippon Genetics) and analyzed using GelDoc-It imaging system (UVP). GeneRuler 1 kb and 100 bp+ DNA Ladders (Thermo Fisher Scientific) were used to determine product sizes for each locus. The amplicons representing additional local rearrangements within introns were excised, purified using GenJET™ Gel Extraction Kit (Thermo Fisher Scientific), cloned into T/A cloning vector (Promega Corporation) and transformed into Escherichia coli, strain DH10B. Up to five recombinant colonies were selected and cultured overnight at 37°C in culture tubes containing 5 mL of Luria–Bertani medium and ampicillin (100 mg/L). Plasmids were purified using Wizard SV Minipreps KIT (Promega Corporation). Sequencing reactions were set up with universal primers sp6 and T7 using Big Dye terminator chemistry (Applied Biosystems), as recommended by manufacturer. Sequencing was carried out on ABI 3700 capillary sequencer (Applied Biosystems).The sequences were manually edited using BioEdit (Hall, 1999) and aligned to the sequences of predicted genes for which ILP primers were designed.
Recording of Electrophoretic Bands and Statistical Data Analysis
The ILP marker profiles were scored manually. Each allele was scored as: 1 (empty insertion site), 2 (occupied insertion site) or 0 (lack of amplification).The codominant marker matrix with diploid individuals was created (Supplementary Table 1) and used in GenAlEx 6.5 (Peakall and Smouse, 2006) for creating genetic distance matrix and analysis of molecular variance (AMOVA). Expected and observed heterozygosity (He and Ho), and fixation index (FIS) were computed using POPGENE 1.32 (Yeh et al., 2000). Polymorphism informative content (PIC) of n-allele locus, an indicator of a genetic marker's usefulness introduced by Botstein et al. (1980), was calculated as: , where pi and pj are the population frequency of the ith and jth allele. Genetic structure was inferred using Bayesian model-based software STRUCTURE 2.2.3 (Pritchard et al., 2008) without information on the accession origin. Ten independent iterations with an admixture and correlated allele frequencies model were performed. The length of the burn-in period and the number of Markov Chain Monte Carlo (MCMC) replications after the burn-in were assigned at 105 for each number of clusters (K) set from 1 to 27 and 1 to 23 for further subclustering. The estimation of K was provided by joining the log probability of data [LnP(D)] from STRUCTURE output and an ad hoc statistics ΔK (Evanno et al., 2005) based on the second rate of change of the log probability of data with respect to the number of clusters. In addition, CLUMPAK software (Kopelman et al., 2015) was used to confirm the selection of the best K. Based on the chosen K, each carrot accession was assigned to a subpopulation for which its membership value (Q) was higher than 0.6. AMOVA was performed using GenAlEx 6.5 to evaluate differentiation among the subpopulations. Principal coordinate analysis (PCoA) was conducted to visualize genetic diversity of the studied accessions.
Results
Development and Validation of the Candidate ILP Markers
Insertion sites of 209 DcSto MITEs within introns of annotated genes were chosen to develop Daucus carota Stowaway-like Intron Length Polymorphism (DcS-ILP) markers evenly distributed throughout the genome (Figure 1). The number of DcSto insertion sites evaluated per chromosome varied from 18 (chromosome 9) to 32 (chromosome 2), with an average of 23.22. Their density ranged from 1.37 (chromosome 2) to 2.57 per Mb (chromosome 1), with an average of 1.76.
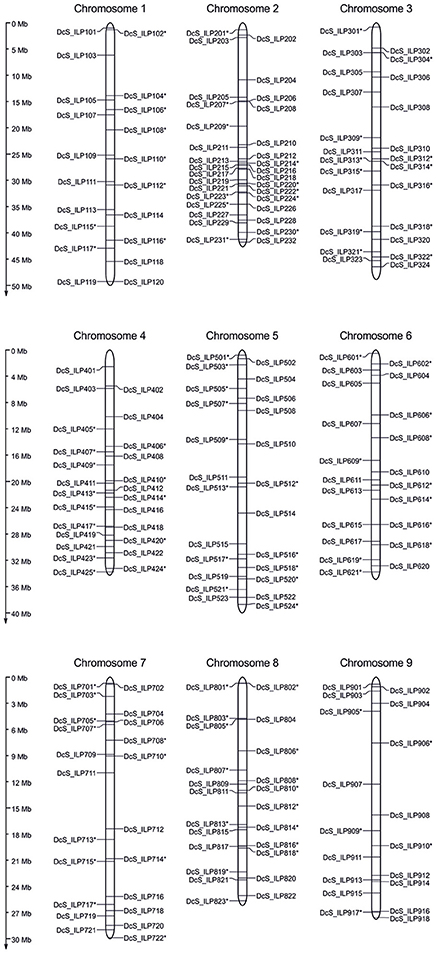
Figure 1. Physical genomic distribution of the 209 developed DcS-ILP markers on nine chromosomes of the carrot genome. The vertical bars correspond to the position of introns harboring DcSto insertions, selected for a development of ILP markers. Positively validated markers are marked by asterisk.
Upon PCR amplification, 100 of the 209 sites showed the expected DcSto insertion-based polymorphism, however, in case of 10 sites at least one additional amplicon was present in at least one accession (Figure 2). Sequencing of those amplicons revealed that none of the additional variants was related to the activity of the DcSto copy present in the reference genome (data not shown). Of the remaining 109 sites, six did not amplify efficiently; 32 were monomorphic for all tested plants; 13 showed a complex pattern resulting from nonspecific amplification, whereas 58 yielded polymorphic products not associated with DcSto insertions (i.e., sizes of PCR products did not correspond to the expected sizes of empty or occupied variants) (Table 2).
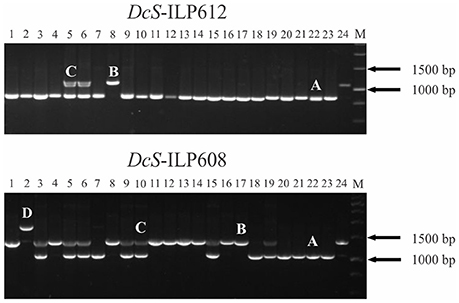
Figure 2. Amplification of DcS-ILP612 and DcS-ILP608 markers in 23 carrot cultivars and the reference genome. Carrot accessions from 1 to 24 are listed in Table 1. DcS-ILP612—amplification of two alleles corresponding to empty (A) and occupied (B) DcSto insertion site and heterozygote (C); DcS-ILP608—amplification of an additional allele (D) resulting from an unclassified rearrangement within the intron. M, 1 kb DNA Ladder.
The length of introns harboring the selected DcSto insertions varied from 449 to 3,637 bp. Based on the length of amplified introns, the developed markers were divided into six classes; I to V with intron size ranging from 400 to 3,400 bp, each at 600-bp interval, and class VI comprising introns longer than 3,400 bp (Table 3). Introns belonging to classes I to IV comprised 97.6% of all the developed markers. Class I and II markers were the most numerous, whereas class III markers showed the highest (55.6%) successful amplification rate indicating the most suitable length of introns considered for ILP markers. DcS-ILP markers of class V and VI were characterized by ambiguous amplification patterns, therefore not considered for further analyses.
Finally, 90 DcS-ILP (Supplementary Table 2) markers showing biallelic DcSto insertion polymorphism (Figure 2) were chosen for development of a panel for genotyping the carrot.
Assessment of Genetic Diversity
The utility of 90 biallelic DcS-ILP markers was verified by estimating the genetic diversity of the collection of 27 D. carota accessions comprising 23 cultivated and 4 wild populations. In total, 180 alleles were identified with an average of 2.0 per locus. 2.78% of the alleles were rare (frequency <0.05) and the mean effective number of alleles was 1.56. The observed heterozygosity for individual loci ranged from 0.04 to 0.56, with an average of 0.24, whereas the expected heterozygosity ranged from 0.04 to 0.51, with an average of 0.34. Shannon's index was from 0.09 to 0.69, with an average of 0.50. Among all the loci analyzed with the Wright's fixation index, 67 were positive. The PIC values ranged from 0.04 to 0.37, with an average of 0.27 (Supplementary Table 1).
STRUCTURE analysis based on 90 loci representing DcSto insertion-derived polymorphisms was performed to evaluate genetic structure of the 27 accessions. The value of ΔK statistics was the highest when two clusters were assumed [ΔK(2) = 297.64]. The increase in the number of assumed clusters resulted in low ΔK value [ΔK(>2) = 0.01–52.35]. Twenty three cultivated accessions were assigned to cluster 1 (C1) with membership coefficients (Q) ranging between 0.831 and 0.997, whereas cluster 2 (C2) comprised exclusively wild accessions with the Q value of 0.965–0.998 (Figure 3A). The level of genetic diversity within C1 (0.31) was slightly higher than within C2 (0.29).
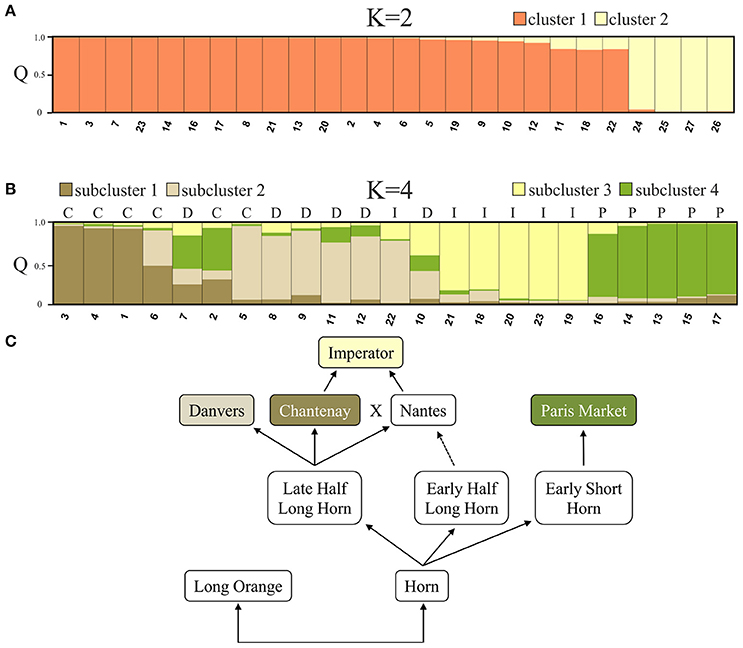
Figure 3. The genetic structure of the studied 27 accessions based on a Bayesian approach assuming two clusters comprising cultivated (cluster 1) and wild (cluster 2) accessions, exclusively (A). The analysis of the genetic structure within first cluster resulted in forming four subclusters, generally comprising accessions representing each of described storage root shapes: C, Chantenay; D, Danvers; I, Imperator; P, Paris Market (B). Assumed four gene pools reflect their breeding history as proposed by Banga (1963) (C). The numbers of accessions correspond to those listed in Table 1.
To evaluate the genetic structure of the 23 cultivated accessions further subclustering was performed on the accessions assigned to C1. The highest ΔK was observed for K = 21 [ΔK(21) = 22.77], K = 2 [ΔK(2) = 17.33] and K = 4 [ΔK(4) = 14.55]. ΔK values for K = 3, K = 5–20 and K = 22–23 were not significant (ΔK = 0.164–4.16). The mean value of log probability of the data was higher for K = 4 than for K = 21, and K = 2 [LnP(D)K= 4 = −1891.7, LnP(D)K= 2 = −1922.5, LnP(D)K= 21 = −2703.2], therefore four subclusters were chosen as the most probable genetic structure of the studied cultivated accessions. With K = 4, three accessions were assigned to subcluster SC1 with Q ranging between 0.928 and 0.962, six to subcluster SC2 with Q between 0.746 and 0.908, five to subcluster SC3 with Q between 0.825 and 0.954 and five to subcluster SC4 with Q between 0.782 and 0.922 (Figure 3B). Four accessions, namely Chantenay Red Cored, Chentenay Rex RS, Danvers 126, and Danvers could not be assigned to any of the subclusters due to high level of admixture (Q < 0.6). The overall Q proportion of each of the four types clearly distinguished (Q > 0.6) the membership of Chantenay root type in SC1 (Q = 0.605), Danvers root type in SC2 (Q = 0.626), Imperator root type in SC3 (Q = 0.785), and Paris Market root type in SC4 (Q = 0.884) (Table 4).
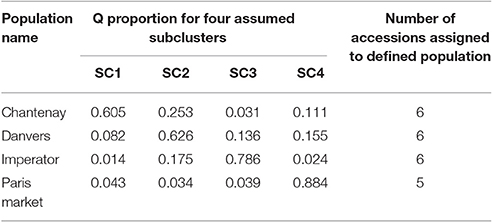
Table 4. The proportion of membership coefficients (Q) of each population defined by the type of root in each of the four subclusters.
AMOVA attributed 19% (P = 0.001) of the total genetic diversity to variation among the root types. The diversity of the 23 cultivated accessions was revealed by PCoA (Figure 4). Using the first three axes 31.7% of the total variation could be explained, with the 1st, 2nd, and 3rd axes explaining 12.1, 10.4, and 9.2%, respectively.
The above results suggested four separate groups in the collection of 23 cultivated carrots and the grouping generally corresponded with a postulated breeding history of western carrot types presented by Banga (1963), indicating that Chantenay and Danvers types originated from the Late Half Long Horn group, while Paris Market type descended from the Early Short Horn group. Both historical groups differ in terms of their storage root shape and earliness. In turn, the origin Imperator type was traced back to a cross between Chantenay and Nantes (Figure 3C).
Discussion
In the present study, we took advantage of intron length polymorphisms resulting from retained DcSto insertions in order to develop a set of ILP markers in the carrot. The DcSto elements used in the study comprised mostly two families, DcSto6 and DcSto1, the most numerous in the carrot genome and showing high percentage of insertions within coding regions (20 and 12%, respectively) (Iorizzo et al., 2016). The ubiquity of DcSto elements facilitated the selection of evenly distributed insertion sites for analysis, as well as equal coverage of the genome with the developed markers. 62.7% of the candidate markers were successfully amplified and 47.8% of them identified DcSto insertion polymorphisms. The success of amplification rate was lower in comparison with ILP markers in other plants, such as Vigna unguiculata (89%; Gupta et al., 2012), Glycine max (88.2%; Shu et al., 2010), Solanum lycopersicum (71%; Wang et al., 2010), probably as a result of high percentage of ambiguous amplification of introns longer than 2,200 bp. The length of intron is considered the main cause of PCR failure and generally, the successful amplification rate decreases with greater length of intron (Wang et al., 2010; Gupta et al., 2012). Polymorphism information content (PIC) has become the most widely used formula to measure the information content of molecular markers (Nagy et al., 2012). The mean PIC value of DcS-ILPs obtained for the studied Daucus carota accessions was higher compared to many of the developed ILP markers, e.g., Setaria italica (Gupta et al., 2011) and Hevea brasiliensis (Li et al., 2013), and comparable to study of Gupta et al. (2012) where 16 CILP loci were analyzed in 10 Vigna unguiculata accessions, with an average of 2.0 alleles per locus, and PIC value of 0.34. Differences in PIC values might be attributed to the various numbers of markers and accessions exploited in these studies. The average PIC value obtained in study of Huang et al. (2010), where 103 ILP loci were analyzed in 36 Oryza sativa accessions, was considerably higher (0.44) due to the higher number of alleles identified by rice ILPs (2.29 alleles per locus). As expected, the mean PIC value of the codominant DcS-ILPs was lower than the one obtained for the genomic SSR markers developed for the carrot (Rong et al., 2010; Cavagnaro et al., 2011). Similar results were reported for the comparative analysis of genetic diversity in Oryza sativa using ILP and genomic SSR markers (Huang et al., 2010). The developed DcS-ILPs showed discriminatory power comparable to that of dominant markers, e.g., DArT (Grzebelus et al., 2014). The values of Wright's fixation index which were significantly higher than zero, as well as the lower mean value of observed heterozygosity, indicated an excess of homozygous allelic states expected in advanced cultivars. DcS-ILP-based analysis of genetic structure of the studied accessions showed clear differentiation of wild and cultivated carrot, supporting earlier observations based on DArT, SSR and SNP genotyping (Cavagnaro et al., 2011; Iorizzo et al., 2013; Grzebelus et al., 2014). Bayesian clustering, on both accession and pre-defined population levels, revealed the presence of four gene pools that generally could be attributed to the shape of the storage root, namely: (1) Chantenay, (2) Danvers, (3) Imperator, and (4) Paris Market, and corresponding to their breeding history, as proposed by Banga (1963) (Figures 3B,C). Having said that, a substantial level of admixture was apparent for few investigated cultivars, possibly resulting from inter-type crosses aiming to derive an intermediate root morphology, e.g., longer or shorter roots. On the other hand, clear separation between the Paris Market type cultivars and the remaining three types confirms the postulated origin of the former from the Early Short Horn gene pool, opposed to Danvers and Chantenay types originating from the Late Half Long Horn gene pool. It is the first molecular evidence for a possible root-type associated structure of genetic diversity in western cultivated carrot. Nonetheless, a more extensive study ought to be conducted in order to substantiate this hypothesis. The results of PCoA were mostly consistent with Bayesian clustering indicating the presence of the above-mentioned genetic structure.
Conclusion
In this study, we showed that the abundance of class II transposable elements may serve as a tool for relatively rapid and low-cost development of gene-derived molecular markers for effective use in carrot genotyping studies. DcSto insertion-derived ILP markers detect substantial variation among carrot plants of different origin and can be exploited in germplasm characterization and analysis of genome relationships. In addition, DcS-ILP markers directly reflect the variation within the genes and could be potentially useful in gene tagging and genetic map construction. ILP markers share many advantages of SSR markers, i.e., codominant nature, locus specificity and high reproducibility, but provide more convenient and rapid detection. To our knowledge, the DcS-ILP markers developed in this study are a novel set of publicly available transposon-based markers in the carrot.
Author Contributions
AM, DG, and KS designed the study; KS, AM, and GM developed DcS-ILP markers; KS performed the validation of candidate DcS-ILP markers and the assessment of genetic diversity; KS, DG, AM, and GM drafted sections of the manuscript; KS and DG prepared the final version of the paper. All authors read, reviewed and approved the manuscript.
Funding
The research was financed from funds for basic research on crop improvement granted by the Polish Ministry of Agriculture and Rural Development in the years 2014–2016.
Conflict of Interest Statement
The authors declare that the research was conducted in the absence of any commercial or financial relationships that could be construed as a potential conflict of interest.
Supplementary Material
The Supplementary Material for this article can be found online at: http://journal.frontiersin.org/article/10.3389/fpls.2017.00725/full#supplementary-material
References
Banga, O. (1963). Main Types of the Western Carotene Carrot and Their Origin. Zwolle: N.V. Uitgevers-Maatschappij W.E.J. Tjeenk Willink.
Botstein, D., White, R. L., Skolnick, M., and Davis, R. W. (1980). Construction of a genetic linkage map in man using restriction fragment length polymorphisms. Am. J. Hum. Genet. 32, 314–331.
Bradeen, J. M., Bach, I. C., Briard, M., le Clerc, V., Grzebelus, D., Senalik, D. A., et al. (2002). Molecular diversity analysis of cultivated carrot (Daucus carota L.) and wild Daucus populations reveals a genetically nonstructured composition. J. Amer. Soc. Hort. Sci. 127, 383–391.
Brügger, K., Redder, P., She, Q., Confalonieri, F., Zivanovic, Y., and Garrett, R. A. (2002). Mobile elements in archaeal genomes. FEMS Microbiol. Lett. 206, 131–141. doi: 10.1016/S0378-1097(01)00504-3
Bureau, T. E., and Wessler, S. R. (1992). Tourist: a large family of small inverted repeat elements frequently associated with maize genes. Plant Cell 4, 1283–1294. doi: 10.1105/tpc.4.10.1283
Bureau, T. E., and Wessler, S. R. (1994). Stowaway: a new family of inverted repeat elements associated with the genes of both monocotyledonous and dicotyledonous plants. Plant Cell Online 6, 907–916. doi: 10.1105/tpc.6.6.907
Casa, A. M., Brouwer, C., Nagel, A., Wang, L., Zhang, Q., Kresovich, S., et al. (2000). The MITE family Heartbreaker (Hbr): molecular markers in maize. Proc. Natl. Acad. Sci. U.S.A. 97, 10083–10089. doi: 10.1073/pnas.97.18.10083
Casa, A. M., Nagel, A., and Wessler, S. R. (2004). MITE display. Methods Mol. Biol. 260, 175–188. doi: 10.1385/1-59259-755-6:175
Cavagnaro, P. F., Chung, S.-M., Manin, S., Yildiz, M., Ali, A., Alessandro, M. S., et al. (2011). Microsatellite isolation and marker development in carrot - genomic distribution, linkage mapping, genetic diversity analysis and marker transferability across Apiaceae. BMC Genomics 12:386. doi: 10.1186/1471-2164-12-386
Chang, R.-Y., O'Donoughue, L. S., and Bureau, T. E. (2001). Inter-MITE polymorphisms (IMP): a high throughput transposon-based genome mapping and fingerprinting approach. TAG Theor. Appl. Genet. 102, 773–781. doi: 10.1007/s001220051709
Chen, X., Zhang, G., and Wu, W. (2010). Investigation and utilization of intron length polymorphisms in conifers. New For. 41, 379–388. doi: 10.1007/s11056-010-9229-5
Evanno, G., Regnaut, S., and Goudet, J. (2005). Detecting the number of clusters of individuals using the software STRUCTURE: a simulation study. Mol. Ecol. 14, 2611–2620. doi: 10.1111/j.1365-294X.2005.02553.x
Feschotte, C., Swamy, L., and Wessler, S. R. (2003). Genome-wide analysis of mariner-like transposable elements in rice reveals complex relationships with Stowaway miniature inverted repeat transposable elements (MITEs). Genetics 163, 747–758.
Feschotte, C., Zhang, X., and Wessler, S. R. (2002). “Minature inverted-repeat transposable elements (MITEs) and their relationship with established DNA transposons,” in Mobile DNA II, eds L. Craig, R. Craigie, M. Gellert, and A. Lambowitz (Washington, DC: American Society for Microbiology Press), 1147–1158.
Grzebelus, D., Iorizzo, M., Senalik, D., Ellison, S., Cavagnaro, P., Macko-Podgorni, A., et al. (2014). Diversity, genetic mapping, and signatures of domestication in the carrot (Daucus carota L.) genome, as revealed by Diversity Arrays Technology (DArT) markers. Mol. Breed. 33, 625–637. doi: 10.1007/s11032-013-9979-9
Grzebelus, D., Jagosz, B., and Simon, P. W. (2007). The DcMaster transposon display maps polymorphic insertion sites in the carrot (Daucus carota L.) genome. Gene 390, 67–74. doi: 10.1016/j.gene.2006.07.041
Gupta, S., Bansal, R., and Gopalakrishna, T. (2012). Development of intron length polymorphism markers in cowpea [Vigna unguiculata (L.) Walp.] and their transferability to other Vigna species. Mol. Breed. 30, 1363–1370. doi: 10.1007/s11032-012-9722-y
Gupta, S., Kumari, K., Das, J., Lata, C., Puranik, S., and Prasad, M. (2011). Development and utilization of novel intron length polymorphic markers in foxtail millet (Setaria italica (L.) P. Beauv.). Genome 54, 586–602. doi: 10.1139/g11-020
Hall, T. (1999). BioEdit: a user-friendly biological sequence alignment editor and analysis program for Windows 95/98/NT. Nucleid Acids Symp. Ser. 41, 95–98.
Huang, M., Xie, F., Chen, L., Zhao, X., Jojee, L., and Madonna, D. (2010). Comparative analysis of genetic diversity and structure in rice using ILP and SSR markers. Rice Sci. 17, 257–268. doi: 10.1016/S1672-6308(09)60025-1
Huang, X., Lu, G., Zhao, Q., Liu, X., and Han, B. (2008). Genome-wide analysis of transposon insertion polymorphisms reveals intraspecific variation in cultivated rice. Plant Physiol. 148, 25–40. doi: 10.1104/pp.108.121491
Iorizzo, M., Ellison, S., Senalik, D., Zeng, P., Satapoomin, P., Huang, J., et al. (2016). A high-quality carrot genome assembly provides new insights into carotenoid accumulation and asterid genome evolution. Nat. Genet. 48, 657–666. doi: 10.1038/ng.3565
Iorizzo, M., Senalik, D. A., Ellison, S. L., Grzebelus, D., Cavagnaro, P. F., Allender, C., et al. (2013). Genetic structure and domestication of carrot (Daucus carota subsp. sativus) (Apiaceae). Am. J. Bot. 100, 930–938. doi: 10.3732/ajb.1300055
Jiang, N., Bao, Z., Zhang, X., Hirochika, H., Eddy, S. R., McCouch, S. R., et al. (2003). An active DNA transposon family in rice. Nature 421, 163–167. doi: 10.1038/nature01214
Jiang, N., Feschotte, C., Zhang, X., and Wessler, S. R. (2004). Using rice to understand the origin and amplification of miniature inverted repeat transposable elements (MITEs). Curr. Opin. Plant Biol. 7, 115–119. doi: 10.1016/j.pbi.2004.01.004
Kopelman, N. M., Mayzel, J., Jakobsson, M., Rosenberg, N. A., and Mayrose, I. (2015). Clumpak: a program for identifying clustering modes and packaging population structure inferences across K. Mol. Ecol. Resour. 15, 1179–1191. doi: 10.1111/1755-0998.12387
Kumar, A., and Hirochika, H. (2001). Applications of retrotransposons as genetic tools in plant biology. Trends Plant Sci. 6, 127–134. doi: 10.1016/S1360-1385(00)01860-4
Hua-Van, A., Le Rouzic, A., Maisonhaute, C., and Capy, P. (2005). Abundance, distribution and dynamics of retrotransposable elements and transposons: similarities and differences. Cytogenet. Genome Res. 110, 426–440. doi: 10.1159/000084975
Lee, J. K., Kwon, S.-J., Park, K.-C., and Kim, N.-S. (2005). Isaac-CACTA transposons: new genetic markers in maize and sorghum. Genome 48, 455–460. doi: 10.1139/g05-013
Lessa, E. (1992). Rapid surveying of DNA sequence variation in natural populations. Mol. Biol. Evol. 9, 323–330.
Li, D., Xia, Z., Deng, Z., Liu, X., and Feng, F. (2013). Development, characterization, genetic diversity and cross-species/genera transferability of ILP markers in rubber tree (Hevea brasiliensis). Genes Genomics 35, 719–731. doi: 10.1007/s13258-013-0122-4
Meyers, B. C., Tingey, S. V., and Morgante, M. (2001). Abundance, distribution, and transcriptional activity of repetitive elements in the maize genome. Genome Res. 11, 1660–1676. doi: 10.1101/gr.188201
Muthamilarasan, M., Venkata Suresh, B., Pandey, G., Kumari, K., Parida, S. K., and Prasad, M. (2014). Development of 5123 intron-length polymorphic markers for large-scale genotyping applications in foxtail millet. DNA Res. 21, 41–52. doi: 10.1093/dnares/dst039
Nagy, S., Poczai, P., Cernák, I., Gorji, A. M., Hegedűs, G., and Taller, J. (2012). PICcalc: an online program to calculate polymorphic information content for molecular genetic studies. Biochem. Genet. 50, 670–672. doi: 10.1007/s10528-012-9509-1
Park, K.-C., Lee, J. K., Kim, N.-H., Shin, Y.-B., Lee, J.-H., and Kim, N.-S. (2003). Genetic variation in Oryza species detected by MITE-AFLP. Genes Genet. Syst. 78, 235–243. doi: 10.1266/ggs.78.235
Peakall, R., and Smouse, P. E. (2006). GENALEX 6: genetic analysis in Excel. Population genetic software for teaching and research. Mol. Ecol. Notes 6, 288–295. doi: 10.1111/j.1471-8286.2005.01155.x
Pritchard, J. K., Wen, X., and Falush, D. (2008). Structure software: version 2.2.3. Chicago, IL: Univ. Chicago.
Prohens, J., and Nuez, F. (2008). Vegetables: Fabaceae, Liliaceae, Solanaceae, and Umbelliferae. New York, NY: Springer New York. doi: 10.1007/978-0-387-30443-4
Rong, J., Janson, S., Umehara, M., Ono, M., and Vrieling, K. (2010). Historical and contemporary gene dispersal in wild carrot (Daucus carota ssp. carota) populations. Ann. Bot. 106, 285–296. doi: 10.1093/aob/mcq108
Shu, Y., Li, Y., Zhu, Y., Zhu, Z., Lv, D., Bai, X., et al. (2010). Genome-wide identification of intron fragment insertion mutations and their potential use as SCAR molecular markers in the soybean. Theor. Appl. Genet. 121, 1–8. doi: 10.1007/s00122-010-1285-x
Untergasser, A., Cutcutache, I., Koressaar, T., Ye, J., Faircloth, B. C., Remm, M., et al. (2012). Primer3–new capabilities and interfaces. Nucleic Acids Res. 40:e115. doi: 10.1093/nar/gks596
Wang, X., Zhao, X., Zhu, J., and Wu, W. (2005). Genome-wide investigation of intron length polymorphisms and their potential as molecular markers in rice (Oryza sativa L.). DNA Res. 12, 417–427. doi: 10.1093/dnares/dsi019
Wang, Y., Chen, J., Francis, D. M., Shen, H., Wu, T., and Yang, W. (2010). Discovery of intron polymorphisms in cultivated tomato using both tomato and Arabidopsis genomic information. Theor. Appl. Genet. 121, 1199–1207. doi: 10.1007/s00122-010-1381-y
Wydner, K. S., Sechler, J. L., Boyd, C. D., and Passmore, H. C. (1994). Use of an intron length polymorphism to localize the tropoelastin gene to mouse Chromosome 5 in a region of linkage conservation with human chromosome 7. Genomics 23, 125–131. doi: 10.1006/geno.1994.1467
Yaakov, B., Ben-David, S., and Kashkush, K. (2013). Genome-wide analysis of Stowaway-like MITEs in wheat reveals high sequence conservation, gene association, and genomic diversification. Plant Physiol. 161, 486–496. doi: 10.1104/pp.112.204404
Yang, L., Jin, G., Zhao, X., Zheng, Y., Xu, Z., and Wu, W. (2007). PIP: a database of potential intron polymorphism markers. Bioinformatics 23, 2174–2177. doi: 10.1093/bioinformatics/btm296
Keywords: DcSto, genetic diversity structure, ILP, Stowaway-like MITEs, TEs
Citation: Stelmach K, Macko-Podgórni A, Machaj G and Grzebelus D (2017) Miniature Inverted Repeat Transposable Element Insertions Provide a Source of Intron Length Polymorphism Markers in the Carrot (Daucus carota L.). Front. Plant Sci. 8:725. doi: 10.3389/fpls.2017.00725
Received: 20 February 2017; Accepted: 19 April 2017;
Published: 09 May 2017.
Edited by:
Fulvio Cruciani, Sapienza University of Rome, ItalyReviewed by:
Christian Parisod, University of Neuchâtel, SwitzerlandTina T. Hu, Princeton University, USA
Copyright © 2017 Stelmach, Macko-Podgórni, Machaj and Grzebelus. This is an open-access article distributed under the terms of the Creative Commons Attribution License (CC BY). The use, distribution or reproduction in other forums is permitted, provided the original author(s) or licensor are credited and that the original publication in this journal is cited, in accordance with accepted academic practice. No use, distribution or reproduction is permitted which does not comply with these terms.
*Correspondence: Dariusz Grzebelus, ZC5ncnplYmVsdXNAb2dyLnVyLmtyYWtvdy5wbA==