- 1Institute of Pomology, Jiangsu Academy of Agricultural Sciences and Jiangsu Key Laboratory for Horticultural Crop Genetic Improvement, Nanjing, China
- 2College of Agronomy and Resources and Environment, Tianjin Agricultural University, Tianjin, China
- 3Department of Agronomy, Purdue University, West Lafayette, IN, USA
- 4Department of Crop Genetics and Breeding, Sichuan Agricultural University, Chengdu, China
- 5College of Pastoral Agriculture Science and Technology, Lanzhou University, Lanzhou, China
Perennial ryegrass is a popular cool-season grass species due to its high quality for forage and turf. The objective of this study was to identify associations of candidate genes with growth and physiological traits to submergence stress and recovery after de-submergence in a global collection of 94 perennial ryegrass accessions. Accessions varied largely in leaf color, plant height (HT), leaf fresh weight (LFW), leaf dry weight (LDW), and chlorophyll fluorescence (Fv/Fm) at 7 days of submergence and in HT, LFW and LDW at 7 days of recovery in two experiments. Among 26 candidate genes tested by various models, single nucleotide polymorphisms (SNPs) in 10 genes showed significant associations with traits including 16 associations for control, 10 for submergence, and 8 for recovery. Under submergence, Lp1-SST encoding sucrose:sucrose 1-fructosyltransferase and LpGA20ox encoding gibberellin 20-oxidase were associated with LFW and LDW, and LpACO1 encoding 1-aminocyclopropane-1-carboxylic acid oxidase was associated with LFW. Associations between Lp1-SST and HT, Lp6G-FFT encoding fructan:fructan 6G-fructosyltransferase and Fv/Fm, LpCAT encoding catalase and HT were also detected under submergence stress. Upon de-submergence, Lp1-SST, Lp6G-FFT, and LpPIP1 encoding plasma membrane intrinsic protein type 1 were associated with LFW or LDW, while LpCBF1b encoding C-repeat binding factor were associated with HT. Nine significant SNPs in Lp1-SST, Lp6G-FFT, LpCAT, and LpACO1 resulted in amino acid changes with five substitutions found in Lp1-SST under submergence or recovery. The results indicated that allelic diversity in genes involved in carbohydrate and antioxidant metabolism, ethylene and gibberellin biosynthesis, and transcript factor could contribute to growth variations in perennial ryegrass under submergence stress and recovery after de-submergence.
Introduction
Plant species or cultivars differ in growth responses to submergence stress. Escape or quiescence can be a strategy for plant survival from submergence stress (Bailey-Serres and Voesenek, 2008). Escape type plants show rapid shoot elongation to potentially grow above the water and re-stablish air contact. In contrast, quiescence type plants conserve energy by minimizing shoot elongation under the water, allowing plants to generate new tissues after de-submergence (Perata and Voesenek, 2007; Colmer and Voesenek, 2009). The severity and duration of stress, plant species and cultivars, and variation of environmental conditions all influence submergence tolerance, as well as the capability of plant regrowth following de-submergence.
Numerous physiological and molecular alterations are involved in genotypic variations in response to submergence stress and recovery (Vashisht et al., 2011; Yu et al., 2012; Van Veen et al., 2013; Campbell et al., 2015). Carbohydrate consumption and conservation play an important role in stress tolerance, and depletion of carbohydrates under submergence stress largely influenced plant growth and survival (Setter and Laureles, 1996; Sasidharan et al., 2013; Liu and Jiang, 2016). In C3 cool-season temperate grasses, fructan is a major component of non-structural carbohydrate reserve (Chatterton et al., 1989). Decreases in fructan and total water soluble carbohydrate (WSC) content were found in perennial ryegrass exposed to submergence stress, but the tolerant accession had relatively higher levels of fructan and WSC than the intolerant one (Yu et al., 2012). WSC and fructan contents decreased to a similar level in tolerant species of alligatorweed under submergence, but de-submerged plants showed rapid recovery of carbohydrate, which was independent from stored carbohydrate reserves at the starting point of recovery (Ye et al., 2016). It seems that both carbohydrate utilization at the end of submergence and recovery of photosynthesis after de-submergence could be associated with the rate of regrowth (Luo et al., 2011; Yu et al., 2012).
Submergence-induced plant growth alterations are also mediated by phytohormone interactions (Voesenek et al., 2003; Tamang and Fukao, 2015). Under hypoxic conditions, the activities of ethylene biosynthetic enzymes were stimulated, causing increased ethylene levels (Sasidharan and Voesenek, 2015). In rice, an increase in the level of endogenous ethylene and ethylene-mediated gibberellin biosynthesis were observed in both escape and quiescence types in response to submergence stress (Tamang and Fukao, 2015). Through interactions between ethylene, brassinosteroids (BR), SUB1A and SKs, gibberellin-mediated shoot elongation either increased to grow out of the water (escape) or was suppressed for carbohydrate conservation during submergence (quiescence). The stimulated elongation of petioles under submergence stress was associated with accumulated ethylene in submerged petioles, a fast and substantial decrease of the endogenous abscisic acid (ABA) concentration, and a certain level of endogenous auxin and gibberellin in marsh dock (Voesenek et al., 2003). Similar results in lotus demonstrated that the increased ethylene altered the balance between ABA and GA, which contributed to the submergence-induced petiole elongation (Jin et al., 2017).
Antioxidant metabolisms may promote submergence tolerance and recovery upon reoxygenation in plants (Tan et al., 2010; Liu and Jiang, 2015, 2016). The increased shoot activities of catalase (CAT) and peroxidase (POD) were more pronounced in relatively slow-growing genotypes, while greater reductions in root activities of superoxide dismutase (SOD), CAT, POD and ascorbate peroxidase (APX) treatments and increased malondialdehyde concentrations were found in the fast growing genotypes of perennial ryegrass (Liu and Jiang, 2015). Submergence also decreased activities of SOD and APX but increased CAT and POD activities in two creeping bentgrass cultivars (Liu and Jiang, 2016). Moreover, reoxygenation after de-submergence can cause oxidative injury due to increased oxygen uptake and accelerated mitochondrial activities, potentially leading to lipid peroxidation and membrane leakage (Blokhina et al., 2003). A study by Yuan et al. (2017) found that the Jasmonate-inducible accumulation of antioxidants may alleviate oxidative damage caused by reoxygenation in Arabidopsis thaliana, improving plant survival after submergence. The results suggest activation of the antioxidant defense system by the reoxygenation process could reduce oxidative damage and maintain cellular redox homeostasis (Biemelt et al., 1998; Skutnik and Rychter, 2009).
Transcriptional profiling of genotypes contrasting submergence tolerance revealed numerous genes in response to submergence stress (Tamang et al., 2014; Campbell et al., 2015; Chen et al., 2016; Rivera-Contreras et al., 2016). A comparison of a fast-growing escape type of marsh dock with a slow-growing quiescence type of common sorrel identified molecular processes related to carbon starvation, toxins, and ion homeostasis that explained the adaptive growth differences in these two species (Van Veen et al., 2013). Root transcript profiling showed that glycolysis and fermentation genes and a gene encoding sucrose synthase were more strongly induced in less submergence tolerant great yellowcress than in tolerant creeping yellowcress (Sasidharan et al., 2013). Upon de-submergence, a large portion of reoxygenation-responsive genes were identified but also significantly overlapped with submergence-responsive genes in soybean plants (Tamang et al., 2014). The results indicate the induction or down-regulation of certain genes is associated with plant responses during and after submergence stress. These genes could be core conserved, genotype- and organ-specific in fulfilling their role in mediating submergence responses of plants (Tamang et al., 2014; Van Veen et al., 2016). Through genome-wide association analysis combined with biparental QTL mapping approaches, candidate gene HXK6 encoding a hexokinase was identified, with its role in mediating seed germination and contributing to the differences in coleoptile growth between Japonica and Indica varieties under submerged condition (Hsu and Tung, 2015). In Arabidopsis thaliana, genome wide association analysis on 81 natural accessions detected 77 genes (within 10 kb of the associated SNP markers) significantly associated with submergence response and involved in many physiological processes including carbon starvation and fermentation (Vashisht et al., 2016). These genes are important regulators for controlling diverse plant responses to submerged conditions.
Although a large number of genes have been identified in plants under submergence stress, little is known about whether allelic diversities of candidate genes involved in carbohydrate, hormone regulation and antioxidant metabolism cause variations in plant growth under submergence stress and recovery after de-submergence, especially in perennial grass species. Perennial ryegrass is one of the most important cool-season turf and forage grasses. This species has a diverse germplasm, diploid genetics, and more available genomic resources (Studer et al., 2012; Byrne et al., 2015) than other major economically important perennial forage and turf grass species, thus providing a good model for studying the genetic basis of submergence tolerance. We designed the experiment to identify associations of candidate genes with growth and physiological traits to submergence stress and recovery after de-submergence in perennial ryegrass. The knowledge gained from this study will reveal genetic mechanisms of submergence tolerance. The results would benefit germplasm enhancement in perennial ryegrass as well as in other major cool-season perennial grass species with more complex genomes.
Materials and Methods
Plant Materials and Growing Conditions
A global collection of 94 perennial ryegrass accessions was used in this study including 30 wild, 33 cultivars or cultivated, and 31 uncertain materials according to the germplasm bank classification (Yu et al., 2011). The selection of plant materials was based on geographical locations of accessions to maximize ecotype diversity (Figure 1 and Supplemental Table S1). A single seed from each accession was sown in a greenhouse into a plastic pot (4-cm diameter, 9-cm deep) containing a sandy-loam soil with a pH of 6.9. Each accession was propagated through tillers multiple times to ensure genetic uniformity. Two experiments (Experiments 1 and 2) were conducted in a greenhouse in 2009 and 2010 using newly propagated plants (Yu et al., 2012). The duration of Experiment 1 was from October 6 to November 21, 2009, and from December 23, 2009 to February 5, 2010 for Experiment 2. Detailed information of environmental conditions during plant growth was described previously (Yu et al., 2012). Prior to submergence treatment, all the plants were cut to about 5–6 cm above the soil surface to obtain a uniform height.
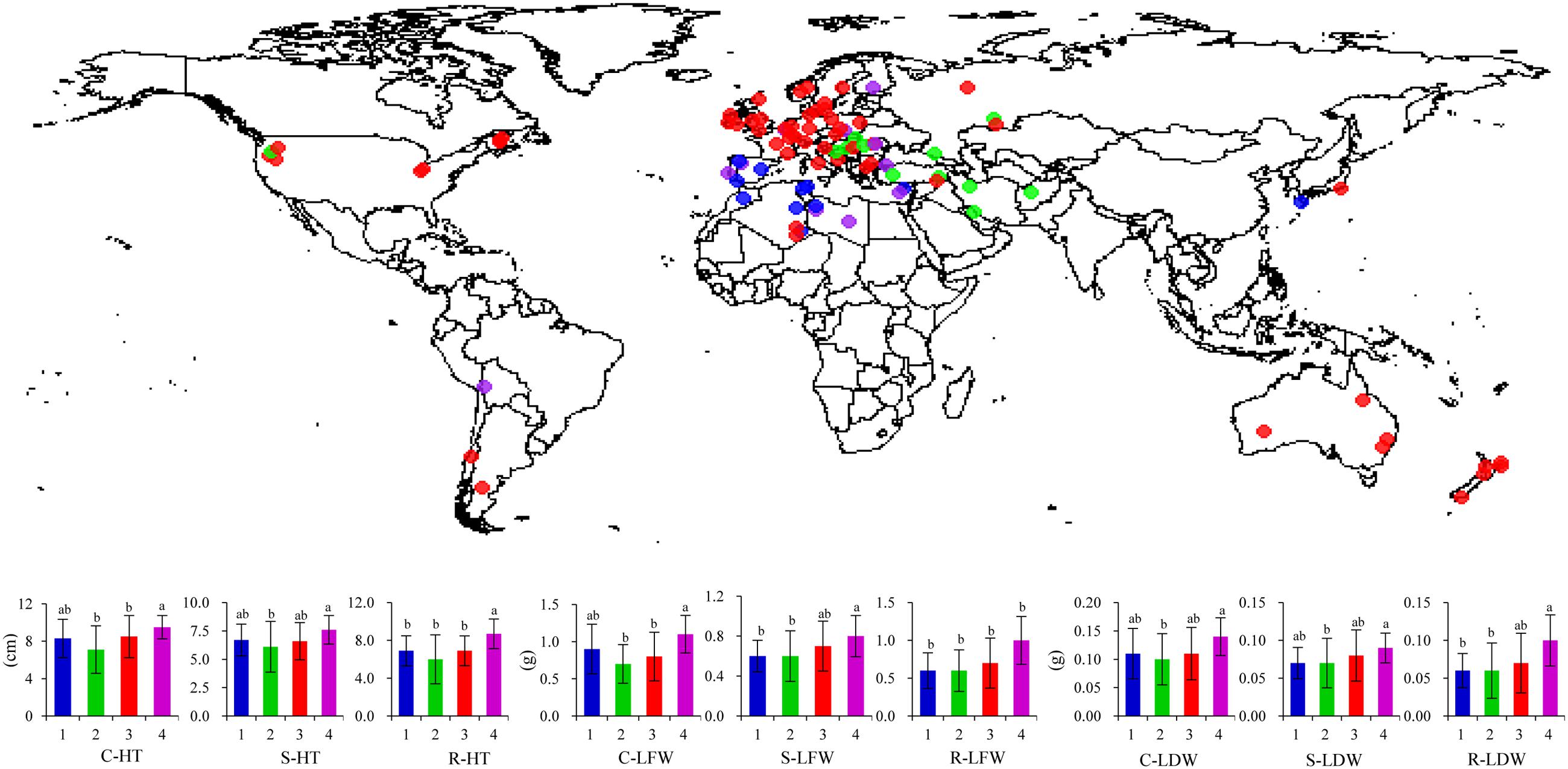
FIGURE 1. Germplasm origin and population structure-affected traits among worldwide perennial ryegrass accessions. Accessions are color-coded by subgroup type identified previously based on population structure (Yu et al., 2011). Accessions with the same color belong to the same subgroup. HT, plant height; LFW, leaf fresh weight; LDW, leaf dry weight; C, S, and R represent the non-stress control, submergence, and recovery after de-submergence, respectively. Means followed by same letter are not significantly different at P < 0.05 within a trait for a given subgroup (column). Bars indicate standard deviation.
Submergence Treatment and Recovery
Submergence stress was imposed by submerging the grass pots in (86 cm length × 38 cm width × 30 cm height) plastic containers with tap water kept at 5 cm above the top of the grasses. The stress treatments began on November 14 of 2009 for Experiment 1 and were repeated on January 29 of 2010 for Experiment 2, lasting 7 days for both. After 7 days of stress, the submerged plants were taken out of the water to allow recovery for 7 days. The environmental conditions during the treatment period were described previously (Yu et al., 2012).
Phenotypic Traits
Leaf color, plant height (HT), leaf fresh weight (LFW), leaf dry weight (LDW), and chlorophyll fluorescence (Fv/Fm) were assessed for indications of plant growth and physiological responses to submergence stress. Leaf color was visually rated on a scale of 1 (yellow) to 9 (dark green). Grass were cut to a similar height prior to stress, the measured HT referred to plant growth occurring only during the 7-day treatments. Leaves corresponding to this HT were cut as measurement of LFW, and LDW was determined after the tissues were dried in an oven at 80°C for 3 days. Fv/Fm was determined in the dark on randomly selected leaves using a fluorescent meter (OS-30P, OPTI-Sciences, Hudson, NH, USA). Only HT, LFW and LDW were evaluated after de-submergence, but not leaf color and Fv/Fm due to minimum differences in these two measurements for both experiments.
Experimental Design and Repeatability of Traits
The experiment was a split plot design with three replications for both experiments (Yu et al., 2012). The main plot was the submergence treatment and the subplot was the set of accessions. The grass pots were completely randomly assigned into containers within treatment regimes, respectively. The repeatability of phenotypic traits across two experiments as reflected by heritability (h2) was calculated using PROC MIXED (SAS Institute, Version 9.1, Cary, NC, USA). The h2 was calculated as follows:
where is the variance component for genotypes, σ2ge for genotype-by-environment, for error; r is the number of replications, and l is number of environment. Based on the outcome of h2, least square means were estimated for each accession across two experiments.
Genotyping
The population was genotyped by using 109 published genome-wide simple sequence repeat markers in perennial ryegrass (Kubik et al., 2001; Jensen et al., 2005; Gill et al., 2006; King et al., 2008). The detailed procedures of DNA extraction, PCR amplification, and allele identification for this population were described previously (Yu et al., 2011). Population structure (Q) was determined by using STRUCTURE 2.3.2 software (Pritchard et al., 2000) and pairwise relative kinship (K) was determined using SPAGeDi (Hardy and Vekemans, 2002) and both were assessed previously by Yu et al. (2011).
Gene Sequencing and SNP Identification
Twenty-six candidate genes in the functions of gibberellic acid biosynthesis, ethylene biosynthesis, kinase, dehydration protection, aquaporin, transcription factor, and anaerobic, carbohydrate and antioxidant metabolisms related to growth and submergence tolerance were selected for sequencing. Of them, sequences of genes involved in antioxidant metabolism (LpCAT, LpChl Cu-ZnSOD, LpCyt Cu-ZnSOD, LpGPX, LpMnSOD, LpFeSOD, LpAPX, LpMDAR, LpDHAR, LpGR), kinase (LpMAPK), dehydration (LpLEA3), aquaporin (LpPIP1 and LpTIP1), and transcription factor (LpCBF1b, LpCBF3b, LpCBF3c, and LpCB4b) for this perennial ryegrass population were obtained previously (Yu et al., 2013, 2015). Additional candidate genes including gene bank accession AY014277.1 for GA20ox, AY551432.1 for GA2ox4, AM407402.1 for 1-SST, AM407401 for 6G-FFT, AM407403.1 for 6-SFT, XM_003573365.3 for LDH, XM_003580260.1 for PDC, Bradi4g31820 for ACO1, and Bradi1g10030.1 for ACS were sequenced in the population (Table 2).
Direct genomic DNA sequencing often results in unclean sequence readings due to the high outcrossing and heterozygous nature of perennial ryegrass, thus, the more problematic introns were excluded for sequencing. We designed primers based on a single long exon for these genes (Supplementary Table S2) and genome DNA was used as the PCR template. Sequencing reactions were performed using Big Dye Terminator kit version 3.1 (Applied Biosystems, Carlsbad, CA, USA) and sequencing was conducted using an ABI 3730 genetic analyzer according to the manufacturer’s instructions (Applied Biosystems, Carlsbad, CA, USA) in the Genomic Center at Purdue University. SNPs were identified using the NovoSNP program 3.0.1 Microsoft Windows Platform version (Weckx et al., 2005).
Nucleotide Diversity and Linkage Disequilibrium (LD)
The nucleotide diversity (π), nucleotide polymorphism (𝜃), and Tajima’s D were analyzed with SNPs for each gene using TASSEL (Bradbury et al., 2007). The LD was also calculated for candidate genes using TASSEL. The generated r2 value was plotted against the physical distance among each pair of SNPs. Overall LD was generated by pooling data from all candidate genes.
Association Analysis
Quantile-quantile (Q-Q) plots for model comparisons of simple linear (S), Q, K and Q + K across all traits were analyzed using R, and the best fit model was selected for association analysis of each trait. Associations between candidate genes and traits of leaf color, HT, LFW, LDW, and Fv/Fm were analyzed using TASSEL 2.1 software with the following three data set: (1) well-drained control (C); (2) submergence stress (S); (3) Recovery after de-submergence (R). Minor alleles with frequency < 5% were removed prior to association analysis. Associations were considered to be significant only at a P-value lower than the Pthreshold-value, calculated using Pthreshold = 0.05/N, where N was the number of SNPs in a candidate gene.
Functional Substitution and Phylogenetic Tree
The putative functional amino acid substitutions were analyzed only for genes significantly associated with traits. A full length gene sequence was obtained from NCBI or from perennial ryegrass genome assembly (Byrne et al., 2015) for anchoring SNP positions in each gene. Amino acid sequences of each peptide were then inferred and compared using NCBI to identify amino acid substitutions. A phylogenetic tree was constructed in MEGA 6 (Tamura et al., 2013), incorporating the Neighbor-Joining method (Saitou and Nei, 1987) with bootstrap analysis of 1000 replicates. The species used for constructing the Neighbor-Joining Tree included perennial ryegrass, tall fescue, Brachypodium distachyon, wheat, barley, rye, maize, rice, oats, and foxtail millet.
Results
Trait Variations and Repeatability
For all accessions across two experiments, large variations in traits were observed under the control, submergence stress, and recovery periods (Table 1). Specifically, C-color ranged from 3.7 to 9.0 with a mean of 6.0 and S-Color ranged from 1.7 to 6.8 with a mean of 3.5. Fv/Fm varied from 0.71 to 0.82 for the control plants and from 0.66 to 0.81 for the submerged plants. The minimum and maximum HT were 2.0- and 16.3-cm for control, 1.44-and 11.7-cm for submergence, and 0.77- and 11.6-cm for recovery, respectively. LFW and LDW spanned from 0.34- to 1.7-g and 0.04- to 0.23-g for the control, 0.19- to 1.57-g and 0.01- to 0.19-g for submergence, and 0.17- to 1.62-g and 0.02- to 0.17-g for recovery, respectively.
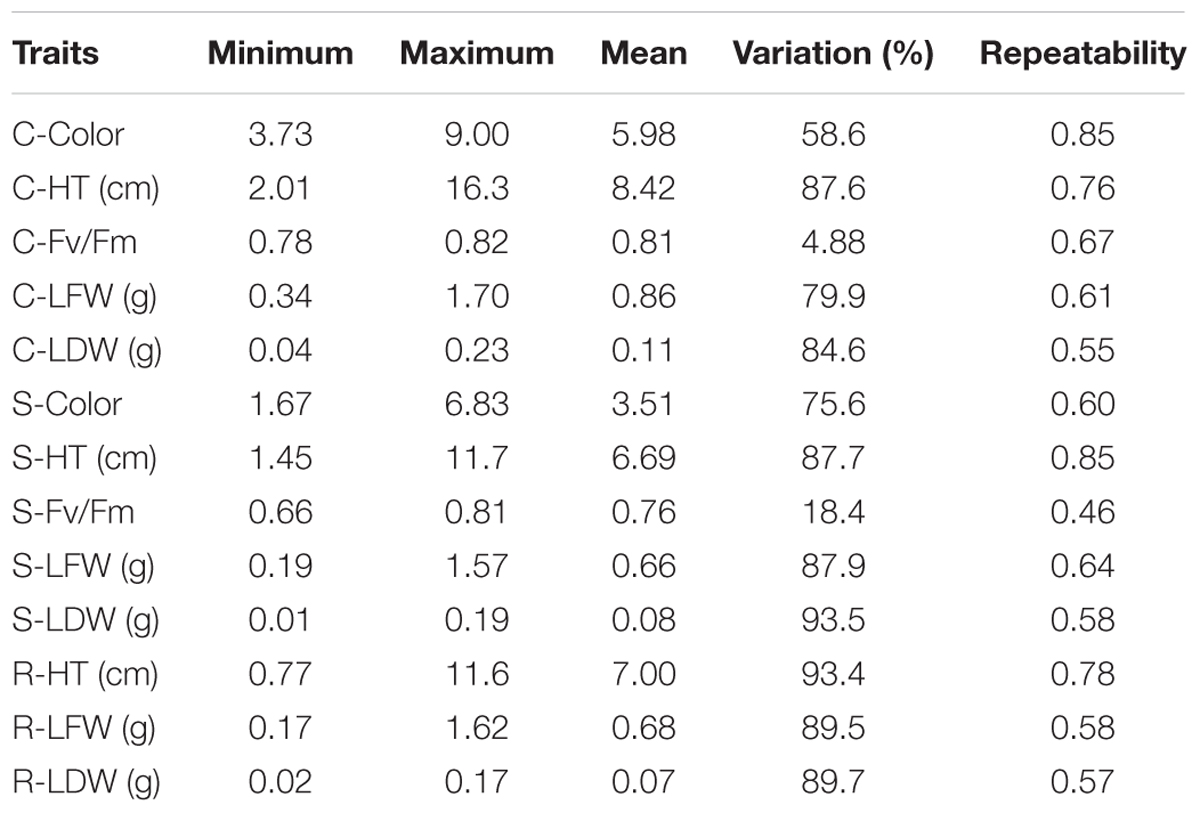
TABLE 1. Range and mean values, percentage of variation, and repeatability of leaf color (color), plant height (HT), chlorophyll fluorescence (Fv/Fm), leaf fresh weight (LFW), leaf dry weight (LDW) under non-stress control (C), submergence stress (S), and recovery after de-submergence (R) in 94 perennial ryegrass accessions across two experiments.
Repeatability (h2) was calculated across two experiments. The h2 values of all traits were higher than 0.5 except for S-Fv/Fm (Table 1). The average h2 for traits under control, submergence, and recovery were 0.69, 0.62, and 0.65, respectively. The high repeatability over testing two environments allowed least square means (LSmeans) of individual traits to be calculated and used for association analyses of genes and traits.
Traits Within Population Structure
Four population structures (subgroups) were identified previously in this population with no obvious kinship (Yu et al., 2011). There were 11, 12, 60, and 11 accessions from subgroups 1–4, respectively (Figure 1). Subgroup 1 contained accessions mainly from southern Europe and northern Africa, and subgroup 2 consisted of accessions mainly from eastern Europe and western Asia. Subgroup 3 was the largest one with more diverse geographic locations mainly including accessions from northern Europe, western Europe, USA, Canada, Australia, and New Zealand. Accessions from subgroup 4 showed mixed geographic locations from northern Africa, eastern and southern Europe. The average values of all traits differed in four subgroups except for C-Fv/Fm, S-Fv/Fm, and S-color (Figure 1). On average, C-color was 5.3, 6.6, 6.2, and 5.0 in subgroup 1, 2, 3, and 4, respectively. Generally, the highest values of HT, LFW, and LDW were found in subgroup 4 and the lowest in subgroup 2 (Figure 1). No differences in traits were noted between subgroup 1 and 3. The separation in trait values of subgroups could be related to geographical locations of accessions.
Nucleotide Diversity, Linkage Disequilibrium and SNP Number
Across 26 candidate genes, the highest value of nucleotide diversity (π) was found in LpPIP1 (0.34) and the lowest in LpCBF1b (0.004) with a mean value of 0.091 (Table 2). Watterson’s 𝜃w ranged from 0.0086 for LpCBF1b to 0.15 for LpCBF3c with a mean value of 0.042 (Table 2). Positive Tajima’s D values were seen in all genes except for LpCBF1b, LpCBF3c, and LpGPX. No Tajima’s D was detected in LpCBF3b, LpMAPK, and LpMnSOD. For the LD pattern, the mean r2 for the pair of SNPs with all candidate genes was 0.16, ranging from 0.034 (LpCBF3c) to 0.48 (LpCBF1b) (Table 2). Overall, a rapid LD decay was shown in perennial ryegrass across all genes (Figure 2). LD decay decreasingly extended to more than 900 kb within r2 = 0.1. Within the sequencing length, a total of 863 SNPs were detected in all genes, ranging from 2 (LpCBF3b) to 121 (LpACO1) after minor SNPs (<5%) were removed (Table 2). The average SNP frequency was 1/48.3 bp, ranging from 1/5 bp in Lp1-SST to 1/357 bp in LpCBF3b.
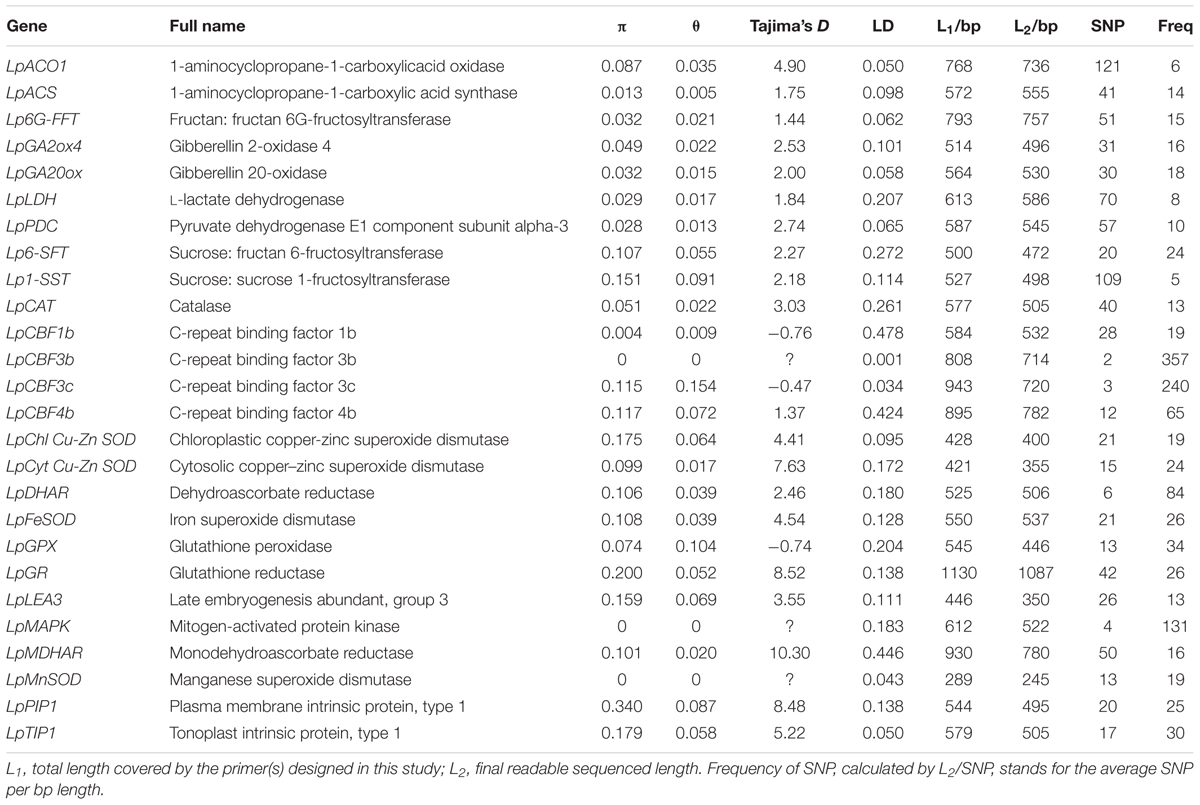
TABLE 2. Summary of genes used in this study, the number of single nucleotide polymorphism (SNP) sites, nucleotide diversity (π), nucleotide polymorphism (𝜃), linkage disequilibrium (LD), and Tajima’s D in each gene across a diverse perennial ryegrass population.
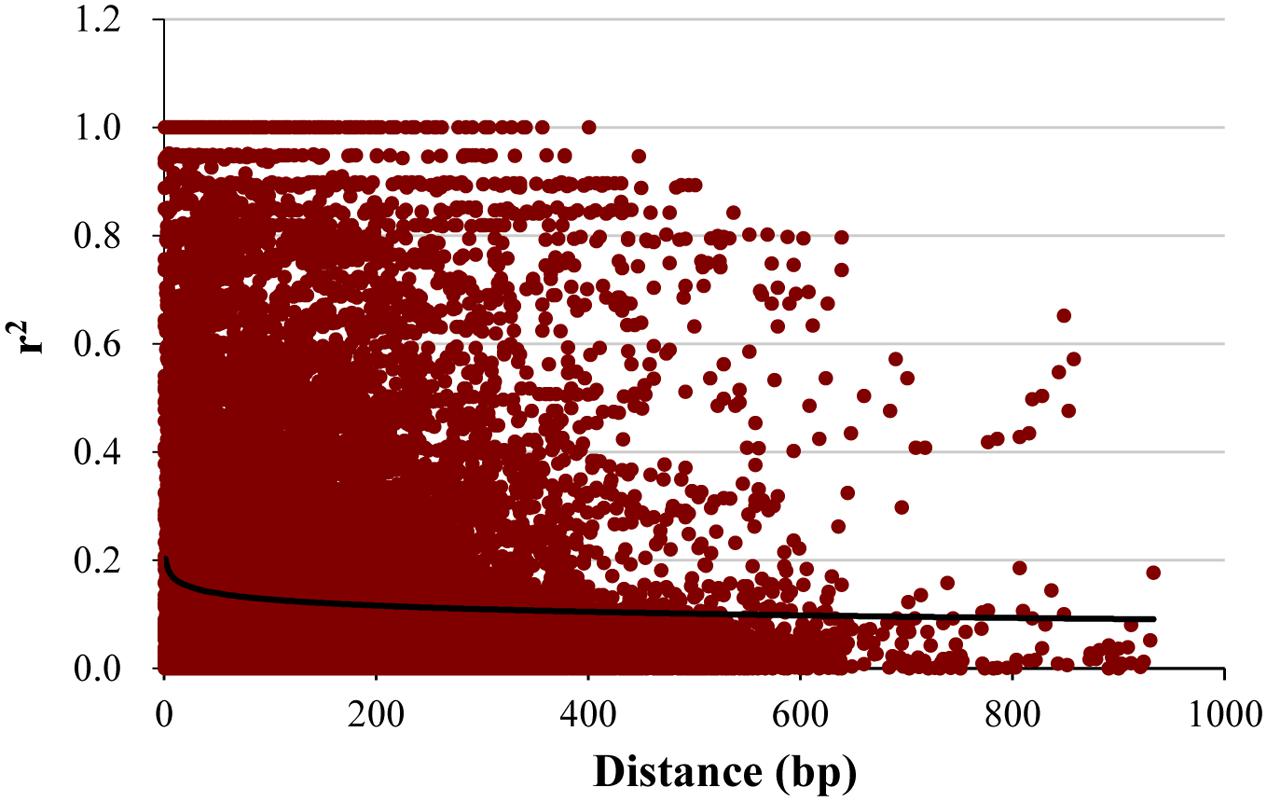
FIGURE 2. Linkage disequilibrium (LD) decay in perennial ryegrass. Plots of squared correlations of allele frequencies (r2) against physical distance between pairs of SNPs in the pooled 26 genes.
Gene-Trait Association
Quantile-quantile plots verified the adequate model for controlling false positives for each gene-trait association for the control, submergence, and recovery periods (Figure 3). The results between the observed and expected -log 10 (P) for associations of 26 genes with traits showed that either the S, Q, K or Q + K implemented model was suitable for analyzing gene-trait associations, depending on individual traits.
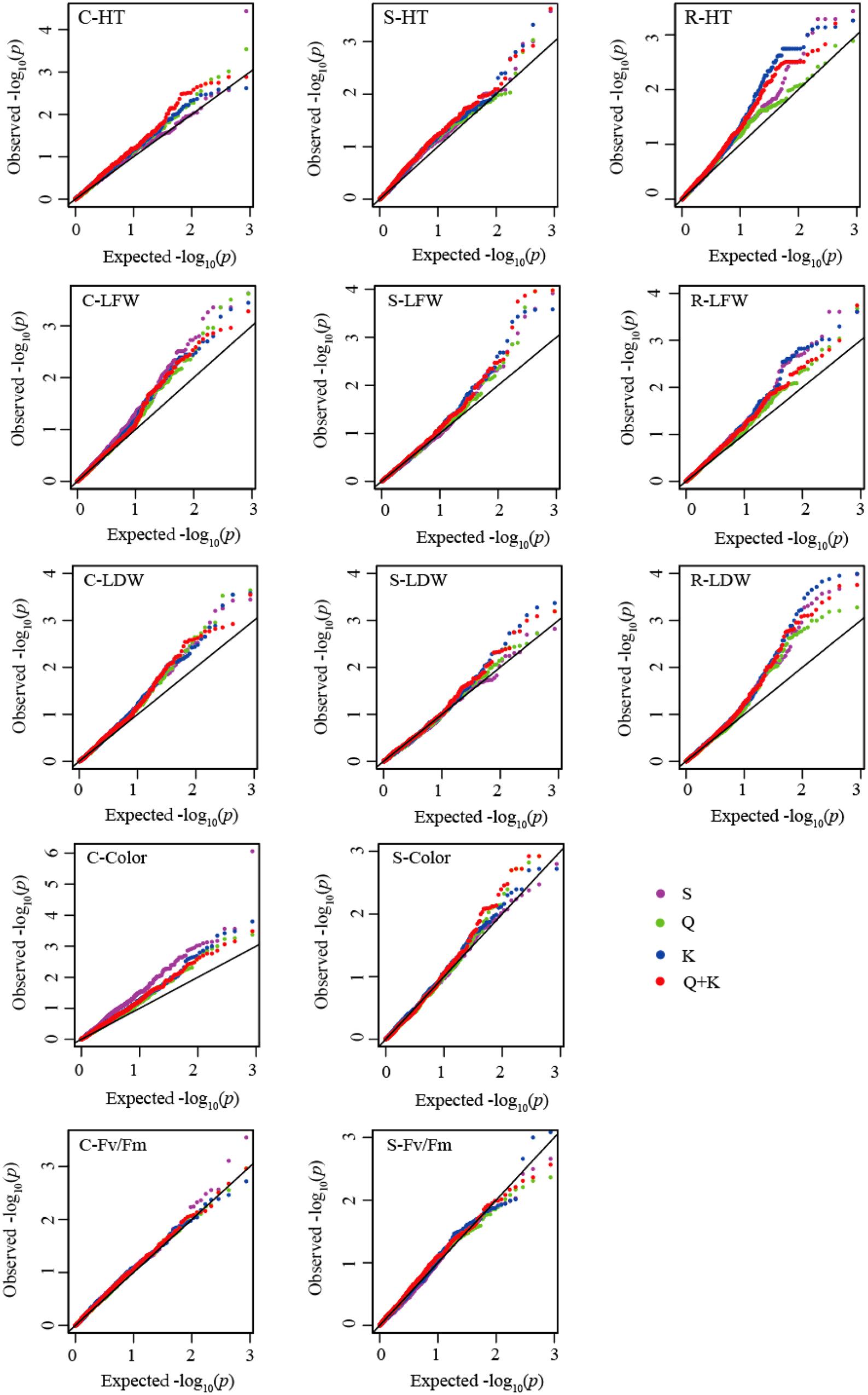
FIGURE 3. Quantile-quantile (QQ) plots for model comparisons with plant height (HT), leaf fresh weight (LFW), leaf dry weight (LDW), leaf color, and chlorophyll fluorescence (Fv/Fm) under non-stress control (C), submergence stress (S), and recovery after de-submergence (R). Leaf color and Fv/Fm were not collected after recovery, so that QQ plots were omitted for these two traits. The solid diagonal lines represent agreement between the observed and expected –log 10 (P) for associations of 26 genes with traits. Color lines represent agreement between the observed and expected –log 10 (P) value for gene-trait associations analyzed with simple liner (S), population structure (Q), relative kinship (K), and Q + K implemented model, respectively.
Through model testing mentioned above, 34 SNPs from 10 candidate genes exhibited significant associations with traits, including 16 SNPs for control, 10 for submergence, and 8 for recovery (Table 3, Supplementary Table S3). Multiple SNP sites in one particular gene were associated with different traits. For the control, SNPs from Lp1-SST, Lp6G-FFT, LpCBF1b, LpACO1, LpFeSOD, LpACS, and Lpcyto Cu-Zn SOD were associated with various traits. Under submergence, SNPs at position 1124 bp in Lp1-SST and at 1093 bp in LpCAT were associated with S-HT, and a SNP at 783 bp in Lp6G-FFT was associated with S-Fv/Fm. For S-LFW, significant associations were detected for SNPs at 909-, 1053-, 1087-, and 1124-bp in Lp1-SST and for a SNP at 469 bp in LpACO1. A SNP at 603 bp in LpGA20ox was associated with S-LFW and S-LDW under submergence. After de-submergence, a SNP at 1161 bp in Lp1-SST and a SNP at 267 bp in LpCBF1b were associated with R-HT. For Lp1-SST, SNPs at 871 bp was associated with R-LDW and SNP at 1091 bp associated with R-LFW and R-LDW. Significant associations were also found between SNPs at 835- and 938-bp in Lp6G-FFT and R-LFW and between a SNP at 1093 bp in LpCAT and R-LDW.
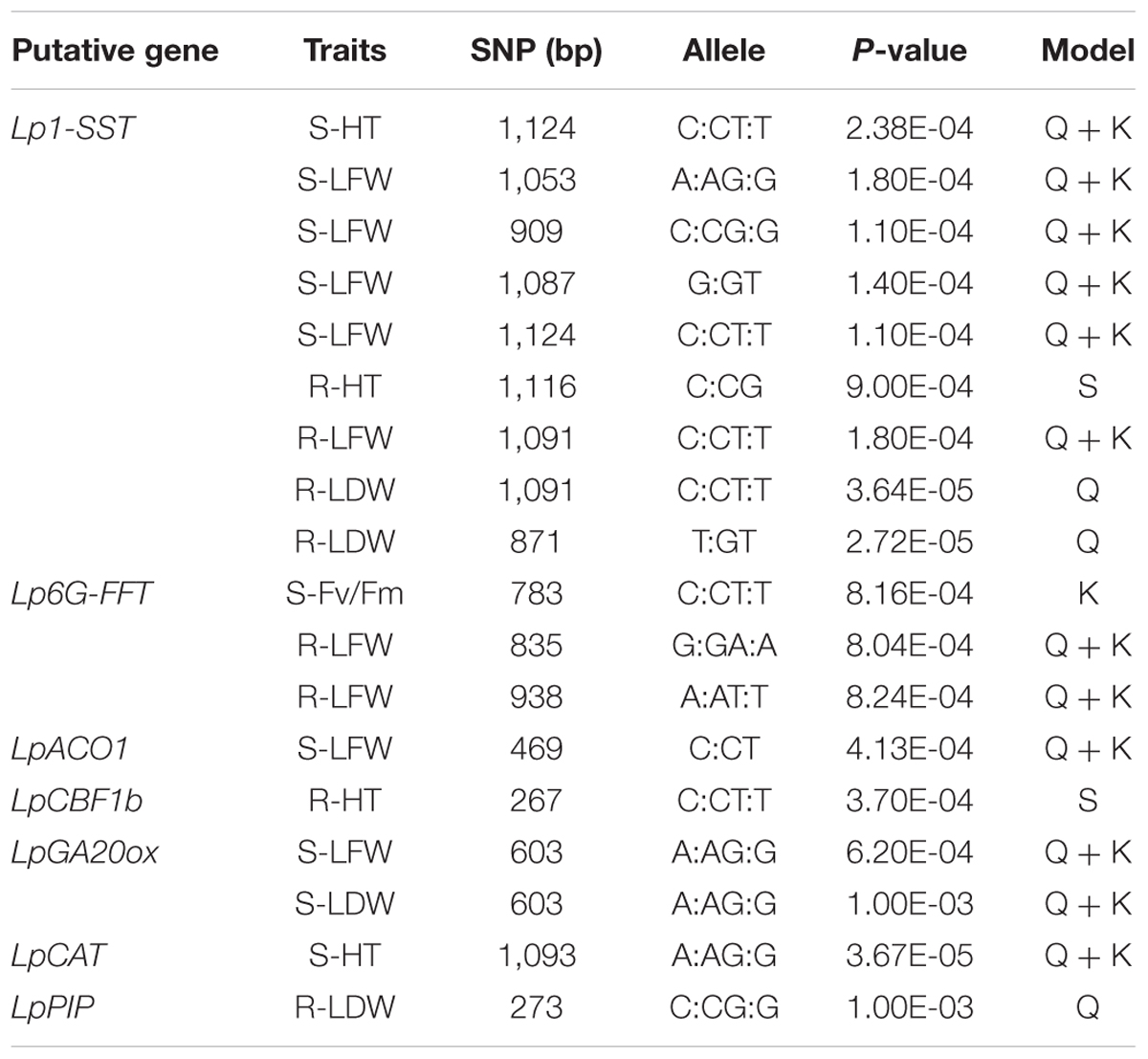
TABLE 3. Association of candidate genes with traits under submergence (S) and recovery (R) in 94 perennial ryegrass accessions.
Sequence variations and phenotypic differences of different alleles of Lp1-SST were compared under submergence or recovery after de-submergence (Figure 4). The mean values of S-HT and S-LDW under submergence at 1124 bp in Lp1-SST were significantly higher in the accessions carrying heterozygous C:T than the accessions carrying homozygous T:T and C:C. Similarly, at 1091 bp, accessions with heterozygous C:T had higher R-LFW and R-LDW after recovery than the accessions with homozygous T:T and C:C.
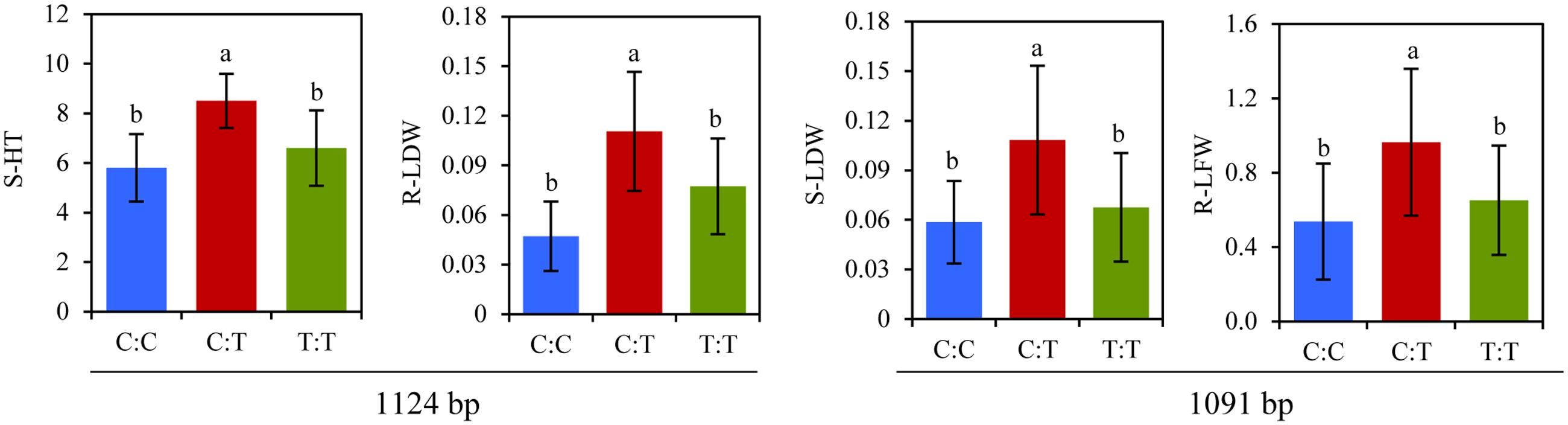
FIGURE 4. Allelic variations of SNP at 1124 bp in Lp1-SST associated with plant height under submergence stress (S-HT) and leaf dry weight after recovery (R-LDW), and at 1091 bp associated with leaf fresh weight under submergence stress (S-LDW) and with leaf fresh weight after recovery (R-LFW) in perennial ryegrass accessions. Columns with the same letter were not significantly different at P < 0.05. Bars indicate standard deviation.
Amino Acid Substitutions
The predicted amino acid substitutions were analyzed only for significant SNPs identified under submergence and recovery. Nine SNP positions in four genes resulted in amino acid changes (Table 4). Five SNPs at position 871-, 1087-, 1091-, 1116-, and 1124-bp in Lp1-SST had nucleotide substitutions from TCA to GCA, GCC to TCC, GTC to GCC, GAC to GAG, and CTG to CCG, causing amino acid substitutions from tyrosine (Y) to aspartic acid (D), alanine (A) to serine (S), valine (V) to A, D to Glutamic acid, (E) and leucine (L) to proline (P), respectively. A SNP at 835 bp in Lp6G-FFT showed one nucleotide substitution from GTC to ATC, resulting in an amino acid change from V to isoleucine (I), while a SNP at 938 bp had a substitution from TAC to TTC with the amino acid change from Y to phenylalanine (F). Nucleotide substitution also occurred at 1093 bp for LpCAT and 469 bp for LpACO1, leading to amino acid changes from V to methionine (M) and P to S, respectively.
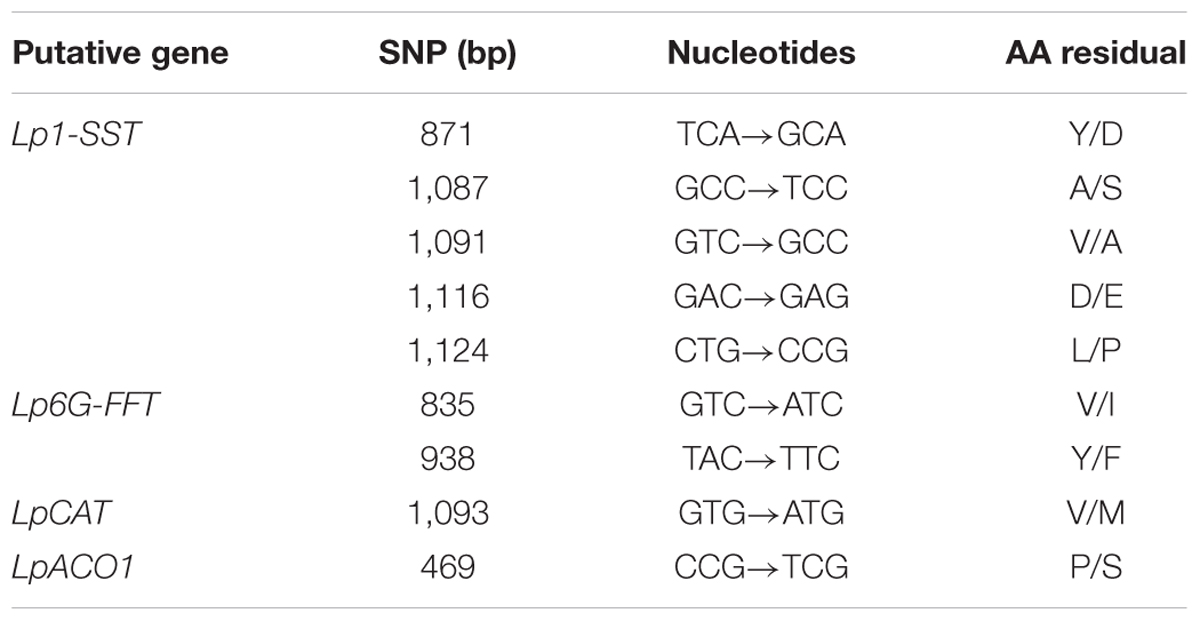
TABLE 4. Amino acid substitution in the loci from candidate genes significantly associated with submergence and recovery traits.
Phylogenetic Analysis
Phylogenetic analysis of protein sequences in the selected species revealed that perennial ryegrass Lp1-SST was more closely related to that in wheat, barley, rye, and tall fescue than that in Brachypodium distachyon, rice and foxtail millet (Figure 5). Based on LpCAT sequences, perennial ryegrass was closer to wheat, barley, and Brachypodium distachyon than that in rice, maize, foxtail millet, and tall fescue (Figure 5). Lp6G-FFT in perennial ryegrass was more closely related to foxtail millet than that in Kentucky bluegrass, wheat, tall fescue, and barley (Supplementary Figure S1). LpACO1 in perennial ryegrass was more closely related to Brachypodium distachyon and foxtail millet and barley than maize, wheat, rice, and creeping bentgrass (Supplementary Figure S1).
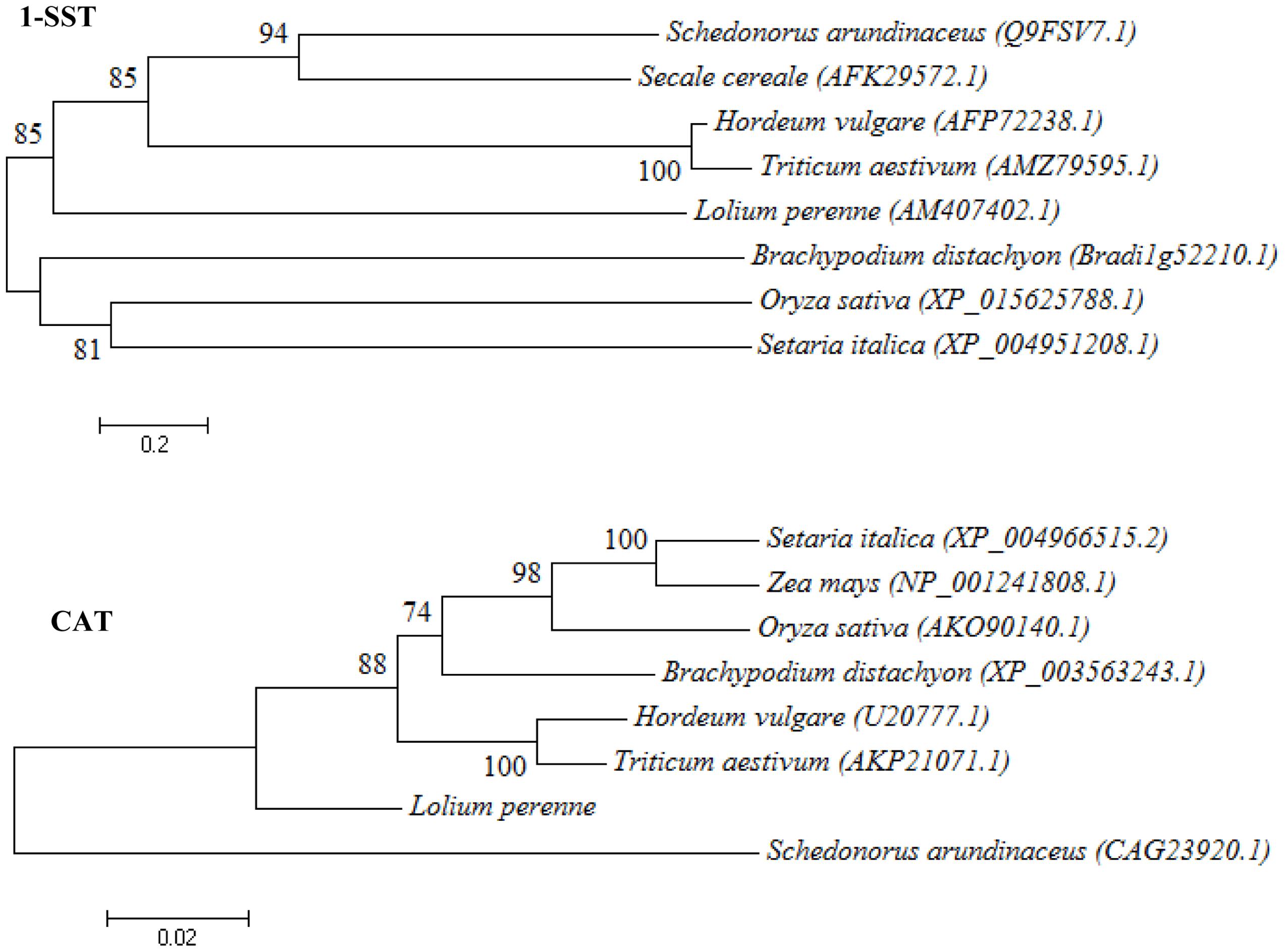
FIGURE 5. Phylogenetic tree of sucrose: sucrose 1-fructosyltransferase (1-SST) and C-repeat-binding factor 1b (CBF1b) protein sequence from plant species. NCBI protein accession numbers were used to indicate homologous protein.
Discussion
Plant growth and physiological status are closely associated with plant survival from submergence stress. Perennial ryegrass accessions differed significantly in leaf color, Fv/Fm, HT, LFW, and LDW in response to submergence stress. Most of these traits were affected by population structure (Figure 1), indicating that structured traits may be related to a geographical origin of the accessions. Larger variations in these traits found in submerged plants more than in the control plants, except for HT, suggested a wide range of submergence response in the perennial ryegrass collection. The differences in HT among accessions may be due to their variation in natural growth habits, although submergence-induced elongation was observed in some perennial ryegrass accessions (Yu et al., 2012). In Arabidopsis thaliana, submergence tolerance was negatively correlated with underwater petiole elongation in 86 accessions (Vashisht et al., 2011). The results indicate complex responses of plants to submergence stress, influenced by many factors including the severity and duration of stress, plant growth status, as well as species and cultivars.
Natural variations in plant response to submergence stress provide an important basis for analyzing gene and trait associations. Carbohydrate metabolisms play a critical role in submergence tolerance. In temperate grasses, fructan biosynthesis is mainly controlled by fructosyltransferases (FTs) including sucrose:sucrose 1-fructosyltransferase (1-SST), fructan:fructan 1-fructosyltransferase (1-FFT), fructan:fructan 6G-fructosyltransferase (6G-FFT), and sucrose:fructan 6-fructosyltransferase (6-SFT) (Gallagher et al., 2015). 1-SST catalyzes the first reaction of the pathway. In this study, significant associations of SNPs at multiple sites in Lp1-SST with LFW, LDW, and HT under submergence as well as with LFW and RDW after recovery, demonstrated that allelic diversity of Lp1-SST contributed to diverse growth responses during or after stress (Figure 4). In particular, multiple amino acid substitutions caused by SNP changes indicated the importance of this gene in conferring submergence tolerance and recovery. Associations of Lp6G-FFT with S-Fv/Fm and with R-LFW after recovery were also identified, especially amino acid changes occurring at SNPs at 835 and 938 bp for R-LFW. The results indicated that Lp1-SST and Lp6G-FFT could influence fructan biosynthesis and assist in submergence tolerance and regrowth in perennial ryegrass after recovery from de-submergence.
Submergence enhances ethylene biosynthesis (Voesenek et al., 1993). 1-aminocyclopropane-1-carboxylic acid synthase (ACS) and 1-aminocyclopropane-1-carboxylic acid oxidase (ACO) are two key enzymes in ethylene biosynthesis (Yang and Hoffman, 1984). Previous studies showed that both ACS and ACO are involved in submergence-induced responses in shoot elongation (Vriezen et al., 1999; Zhou et al., 2002). When submerged, expression of ACS1 and ACO1 and proteins were all up-regulated in bog yellowcress (Vriezen et al., 1999), suggesting transcription and translation regulation of these two enzymes in the ethylene biosynthesis pathway. In rice, the transcript levels of most OsACS and OsACO family members were induced by submergence stress, but to a larger extent in the OsACO family than in the OsACS family, indicating function-related differences between these two gene families during submergence (Guo et al., 2015). Association of a SNP at 469 bp in LpACO1 with R-LFW in this study supported the role of these two genes in mediating plant growth in perennial ryegrass. ACO is the last gene of the ethylene biosynthetic pathway. Particularly, the amino acid change from P to S caused by a SNP at 469 bp in LpACO1 suggested its importance in regulating growth responses of perennial ryegrass to submergence stress.
Gibberellin (GA) regulates plant growth and development, including stem elongation and leaf expansion (Colebrook et al., 2014). GA20oxidase (GA20ox) catalyzes three steps from GA53 to GA20 and GA12 to GA9. Additionally, GA3oxidase (GA3ox) catalyzes the final step from GA20 to GA1 and GA9 to GA4 (Ayano et al., 2014). In Arabidopsis thaliana, GA20ox1, 2, and 3 have an important role in growth and fertility (Plackett et al., 2012). The mutation of GA20ox1, 2, and 3 causes severe dwarfism and sterility (Plackett et al., 2012), and overexpression of GA20ox1 enhances plant growth (Nelissen et al., 2012). Deepwater rice cultivars differed in expression level of OsGA20ox2 under submergence conditions, and internode elongation may be caused by GA1 and GA4 accumulation after induction of the GA20ox gene in plants under deepwater (Ayano et al., 2014). Our results in association of GA20ox with S-LFW and S-LDW in perennial ryegrass supported the observation of regulation of GA20ox on plant growth under submergence stress, although such association did not cause amino acid change.
The C-repeat-binding factor (CBF) genes encoding transcriptional activators control the expression of genes containing the C-repeat-dehydration responsive element DNA regulatory element in their promoters (Gilmour et al., 2000). There were 10 putative CBF genes in perennial ryegrass, similar to either the HvCBF3 or HvCBF4 subgroups in barley (Tamura and Yamada, 2007). Overexpression of CBF genes inhibited plant growth (Achard et al., 2008; Zhou et al., 2014), which may be due to inhibition of GA-dependent elongation growth. A co-expression network of CBF genes was induced by submergence stress but was more activated in the tolerant line of maize (Campbell et al., 2015). Four LpCBF genes were tested for their relationship with traits, but only LpCBF1b was significantly associated with C-LDW, C-HT, and R-HT. The results suggest a role of LpCBF1b on regrowth for perennial ryegrass plants following submergence stress.
Transcriptomic analysis of submergence-tolerant and sensitive Brachypodium distachyon ecotypes reveals oxidative stress as a major tolerance factor (Rivera-Contreras et al., 2016). Among antioxidant enzymes, SOD scavenges to H2O2 (Bowler et al., 1992), and H2O2 can be decomposed by several pathways including the catalase (CAT) and ascorbate-glutathione cycles at different cellular locations (Mittler, 2002). The increased transcript levels of SOD or CAT were noted in maize (Zhang et al., 2009) and in creeping yellowcress under submergence stress (Sasidharan et al., 2013). The associations of Lpcyto Cu-Zn SOD and LpCAT with HT detected under non-stress or submergence indicated contributions of these genes to HT variations in perennial ryegrass accessions. In particular, a SNP at 1093 bp in LpCAT causing an amino acid change after recovery suggested a link between LpCAT and plant regrowth by potentially diminishing oxidative injury upon re-oxygenation.
Conclusion
Significant associations were identified between candidate genes and growth and physiological traits in perennial ryegrass under the non-stress, submerged and recovery after de-submergence conditions. Allelic variations in Lp1-SST, Lp6G-FFT, LpCAT, and LpACO1 caused amino acid substitutions, especially for Lp1-SST with multiple substitutions. The results suggest that allelic diversities of genes involved in carbohydrate and ethylene biosynthesis, antioxidant metabolism, and transcription factor may contribute to variable plant growth responses to submergence stress and recovery after stress in the perennial ryegrass population. This discovery illustrated an important genetic mechanism underlying submergence response, which will be valuable for further studies of functional and regulatory genes involved in submergence tolerance in perennial ryegrass or other perennial grass species with a more complex genome.
Author Contributions
XW conducted gene sequence and wrote the manuscript; YJ designed the experiments and led analyzing data and writing of the manuscript; XW, XZ, XS, XX, ZP, and HL analyzed data. All authors approved the manuscript.
Supplementary Material
The Supplementary Material for this article can be found online at: http://journal.frontiersin.org/article/10.3389/fpls.2017.00791/full#supplementary-material
Conflict of Interest Statement
The authors declare that the research was conducted in the absence of any commercial or financial relationships that could be construed as a potential conflict of interest.
References
Achard, P., Gong, F., Cheminant, S., Alioua, M., Hedden, P., and Genschik, P. (2008). The cold-inducible CBF1 factor–dependent signaling pathway modulates the accumulation of the growth-repressing DELLA proteins via its effect on gibberellin metabolism. Plant Cell 20, 2117–2129. doi: 10.1105/tpc.108.058941
Ayano, M., Kani, T., Kojima, M., Sakakibara, H., Kitaoka, T., Kuroha, T., et al. (2014). Gibberellin biosynthesis and signal transduction is essential for internode elongation in deepwater rice. Plant Cell Environ. 37, 2313–2324. doi: 10.1111/pce.12377
Bailey-Serres, J., and Voesenek, L. A. (2008). Flooding stress: acclimations and genetic diversity. Annu. Rev. Plant Biol. 59, 313–339. doi: 10.1146/annurev.arplant.59.032607.092752
Biemelt, S., Keetman, U., and Albrecht, G. (1998). Re-aeration following hypoxia or anoxia leads to activation of the antioxidative defense system in roots of wheat seedlings. Plant Physiol. 116, 651–658.
Blokhina, O., Virolainen, E., and Fagestedt, K. V. (2003). Antioxidants, oxidative damage, and oxygen deprivation stress: a review. Ann. Bot. 91, 179–194. doi: 10.1093/aob/mcf118
Bowler, C., Van Montagu, M., and Inze, D. (1992). Superoxide dismutase and stress tolerance. Ann. Rev. Plant Physiol. Plant Mol. Biol. 43, 83–116.
Bradbury, P. J., Zhang, Z., Kroon, D. E., Casstevens, T. M., Ramdoss, Y., and Buckler, E. S. (2007). TASSEL: software for association mapping of complex traits in diverse samples. Bioinformatics 23, 2633–2635. doi: 10.1093/bioinformatics/btm308
Byrne, S. L., Nagy, I., Pfeifer, M., Armstead, I., Swain, S., Studer, B., et al. (2015). A synteny-based draft genome sequence of the forage grass Lolium perenne. Plant J. 84, 816–826. doi: 10.1111/tpj.13037
Campbell, M. T., Proctor, C. A., Dou, Y., Schmitz, A. J., Phansak, P., Kruger, G. R., et al. (2015). Genetic and molecular characterization of submergence response identifies Subtol6 as a major submergence tolerance locus in maize. PLoS ONE 10:e0120385. doi: 10.1371/journal.pone.0120385
Chatterton, N. J., Harrison, P. A., Bennett, J. H., and Asay, K. H. (1989). Carbohydrate partitioning in 185 accessions of gramineae grown under warm and cool temperatures. J. Plant Physiol. 134, 169–179.
Chen, W., Yao, Q., Patil, G. B., Agarwal, G., Deshmukh, R. K., Lin, L., et al. (2016). Identification and comparative analysis of differential gene expression in soybean leaf tissue under drought and flooding stress revealed by RNA-Seq. Front. Plant Sci. 7:1044. doi: 10.3389/fpls.2016.01044
Colebrook, E. H., Thomas, S. G., Phillips, A. L., and Hedden, P. (2014). The role of gibberellin signalling in plant responses to abiotic stress. J. Exp. Biol. 217, 67–75. doi: 10.1242/jeb.089938
Colmer, T. D., and Voesenek, L. (2009). Flooding tolerance: suites of plant traits in variable environments. Funct. Plant Biol. 36, 665–681. doi: 10.1071/FP09144
Gallagher, J. A., Cairns, A. J., Thomas, D., Timms-Taravella, E., Skøt, K., Charlton, A., et al. (2015). Fructan synthesis, accumulation and polymer traits. II. Fructan pools in populations of perennial ryegrass (Lolium perenne L.) with variation for water-soluble carbohydrate and candidate genes were not correlated with biosynthetic activity and demonstrated constraints to polymer chain extension. Front. Plant Sci. 6:864. doi: 10.3389/fpls.2015.00864
Gill, G. P., Wilcox, P. L., Whittaker, D. J., Winz, R. A., Bickerstaff, P., Echt, C. E., et al. (2006). A framework linkage map of perennial ryegrass based on SSR markers. Genome 49, 354–364. doi: 10.1139/g05-120
Gilmour, S. J., Sebolt, A. M., Salazar, M. P., Everard, J. D., and Thomashow, M. F. (2000). Overexpression of the Arabidopsis CBF3 transcriptional activator mimics multiple biochemical changes associated with cold acclimation. Plant Physiol. 124, 1854–1865.
Guo, Y., Zhu, C., Gan, L., Ng, D., and Xia, K. (2015). Ethylene is involved in the complete-submergence induced increase in root iron and manganese plaques in Oryza sativa. Plant Growth Regul. 76, 259–268. doi: 10.1007/s10725-014-9996-7
Hardy, O. J., and Vekemans, X. (2002). SPAGeDi: a versatile computer program to analyse spatial genetic structure at the individual or population levels. Mol. Ecol. Notes 2, 618–620. doi: 10.1046/j.1471-8286.2002.00305.x
Hsu, S. K., and Tung, C. W. (2015). Genetic mapping of anaerobic germination-associated QTLs controlling coleoptile elongation in rice. Rice 8:38. doi: 10.1186/s12284-015-0072-3011-1598-4
Jensen, L. B., Muylle, H., Arens, P., Andersen, C. H., Holm, P. A., Ghesquiere, M., et al. (2005). Development and mapping of a public reference set of SSR markers in Lolium perenne L. Mol. Ecol. Notes 5, 951–957. doi: 10.1111/j.1471-8286.2005.01043.x
Jin, Q., Wang, Y., Li, X., Wu, S., Wang, Y., Luo, J., et al. (2017). Interactions between ethylene, gibberellin and abscisic acid in regulating submergence induced petiole elongation in Nelumbo nucifera. Aquat. Bot. 137, 9–15. doi: 10.1016/j.aquabot.2016.11.002
King, J., Thorogood, D., Edwards, K. J., Armstead, I. P., Roberts, L., Skøt, K., et al. (2008). Development of a genomic microsatellite library in perennial ryegrass (Lolium perenne) and its use in trait mapping. Ann. Bot. 101, 845–853. doi: 10.1093/aob/mcn016
Kubik, C., Sawkins, M., Meyer, W. A., and Gaut, B. S. (2001). Genetic diversity in seven perennial ryegrass (Lolium perenne L.) cultivars based on SSR markers. Crop Sci. 41, 1565–1572. doi: 10.2135/cropsci2001.4151565x
Liu, M., and Jiang, Y. (2015). Genotypic variation in growth and metabolic responses of perennial ryegrass exposed to short-term waterlogging and submergence stress. Plant Physiol. Biochem. 95, 57–64. doi: 10.1016/j.plaphy.2015.07.008
Liu, Q., and Jiang, Y. (2016). Exogenous application of nitrogen and cytokinin on growth, carbohydrate, and antioxidant metabolism of creeping bentgrass after de-submergence. HortScience 51, 1602–1606. doi: 10.21273/HORTSCI11357-16
Luo, F., Nagel, K. A., Scharr, H., Zeng, B., Schurr, U., and Matsubara, S. (2011). Recovery dynamics of growth, photosynthesis and carbohydrate accumulation after de-submergence: a comparison between two wetland plants showing escape and quiescence strategies. Ann. Bot. 107, 49–63. doi: 10.1093/aob/mcq212
Mittler, R. (2002). Oxidative stress, antioxidants and stress tolerance. Trends Plant Sci. 7, 405–410. doi: 10.1016/S1360-1385(02)02312-9
Nelissen, H., Rymen, B., Jikumaru, Y., Demuynck, K., Van Lijsebettens, M., Kamiya, Y., et al. (2012). A local maximum in gibberellin levels regulates maize leaf growth by spatial control of cell division. Curr. Biol. 22, 1183–1187. doi: 10.1016/j.cub.2012.04.065
Perata, P., and Voesenek, L. A. (2007). Submergence tolerance in rice requires Sub1A, an ethylene-response-factor-like gene. Trends Plant Sci. 12, 43–46. doi: 10.1016/j.tplants.2006.12.005
Plackett, A. R. G., Powers, S. J., Fernandez-Garcia, N., Urbanova, T., Takebayashi, Y., Seo, M., et al. (2012). Analysis of the developmental roles of the Arabidopsis gibberellin 20-oxidases demonstrates that GA20ox1,-2, and -3 are the dominant paralogs. Plant Cell 24, 941–960. doi: 10.1105/tpc.111.095109
Pritchard, J. K., Stephens, M., and Donnelly, P. (2000). Inference of population structure using multilocus genotype data. Genetics 155, 945–959.
Rivera-Contreras, I. K., Zamora-Hernández, T., Huerta-Heredia, A. A., Capataz-Tafur, J., Barrera-Figueroa, B. E., Juntawong, P., et al. (2016). Transcriptomic analysis of submergence-tolerant and sensitive Brachypodium distachyon ecotypes reveals oxidative stress as a major tolerance factor. Sci. Rep. 6:27686. doi: 10.1038/srep27686
Saitou, N., and Nei, M. (1987). The neighbor-joining method: a new method for reconstructing phylogenetic trees. Mol. Biol. Evol. 4, 406–425. doi: 10.1093/oxfordjournals.molbev.a040454
Sasidharan, R., Mustroph, A., Boonman, A., Akman, M., Ammerlaan, A. M., Breit, T., et al. (2013). Root transcript profiling of two Rorippa species reveals gene clusters associated with extreme submergence tolerance. Plant Physiol. 163, 1277–1292. doi: 10.1104/pp.113.222588
Sasidharan, R., and Voesenek, L. A. (2015). Ethylene-mediated acclimations to flooding stress. Plant Physiol. 169, 3–12. doi: 10.1104/pp.15.00387
Setter, T. L., and Laureles, E. V. (1996). The beneficial effect of reduced elongation growth on submergence tolerance in rice. J. Exp. Bot. 47, 1551–1559. doi: 10.1093/jxb/47.10.1551
Skutnik, M., and Rychter, A. M. (2009). Differential response of antioxidant systems in leaves and roots of barley subjected to anoxia and post-anoxia. J. Plant Physiol. 166, 926–937. doi: 10.1016/j.jplph.2008.11.010
Studer, B., Byrne, S., Nielsen, R. O., Panitz, F., Bendixen, C., Islam, M. S., et al. (2012). A transcriptome map of perennial ryegrass (Lolium perenne L.). BMC Genomics 13:140. doi: 10.1186/1471-2164-13-140
Tamang, B. G., and Fukao, T. (2015). Plant adaptation to multiple stresses during submergence and following desubmergence. Int. J. Mol. Sci. 16, 30164–30180. doi: 10.3390/ijms161226226
Tamang, B. G., Magliozzi, J. O., Maroof, M. A., and Fukao, T. (2014). Physiological and transcriptomic characterization of submergence and reoxygenation responses in soybean seedlings. Plant Cell Environ. 37, 2350–2365. doi: 10.1111/pce.12277
Tamura, K., Stecher, G., Peterson, D., Filipski, A., and Kumar, S. (2013). MEGA6: molecular evolutionary genetics analysis version 6.0. Mol. Biol. Evol. 30, 2725–2729. doi: 10.1093/molbev/mst197
Tamura, K., and Yamada, T. (2007). A perennial ryegrass CBF gene cluster is located in a region predicted by conserved synteny between Poaceae species. Theor. Appl. Genet. 114, 273–283. doi: 10.1007/s00122-006-0430-z
Tan, S., Zhu, M., and Zhang, Q. (2010). Physiological responses of bermudagrass (Cynodon dactylon) to submergence. Acta Physiol. Plant. 32, 133–140. doi: 10.1007/s11738-009-0388-y
Van Veen, H., Mustroph, A., Barding, G. A., Vergeer-van Eijk, M., Welschen-Evertman, R. A., Pedersen, O., et al. (2013). Two Rumex species from contrasting hydrological niches regulate flooding tolerance through distinct mechanisms. Plant Cell 25, 4691–4707. doi: 10.1105/tpc.113.119016
Van Veen, H., Vashisht, D., Akman, M., Girke, T., Mustroph, A., Reinen, E., et al. (2016). Transcriptomes of eight Arabidopsis thaliana accessions reveal core conserved, genotype- and organ-specific responses to flooding stress. Plant Physiol. 172, 668–689.
Vashisht, D., Hesselink, A., Pierik, R., Ammerlaan, J. M., Bailey-Serres, J., Visser, E. J., et al. (2011). Natural variation of submergence tolerance among Arabidopsis thaliana accessions. New Phytol. 190, 299–310. doi: 10.1111/j.1469-8137.2010.03552.x
Vashisht, D., van Veen, H., Akman, M., and Sasidharan, R. (2016). Variation in Arabidopsis flooding responses identifies numerous putative “tolerance genes”. Plant Signal. Behav. 11:e1249083. doi: 10.1080/15592324.2016.1249083
Voesenek, L., Banga, M., Thier, R. H., Mudde, C. M., Harren, F., Barendse, G., et al. (1993). Submergence-induced ethylene synthesis, entrapment, and growth in two plant species with contrasting flooding resistances. Plant Physiol. 103, 783–791.
Voesenek, L. A., Benschop, J. J., Bou, J., Cox, M. C., Groeneveld, H. W., Millenaar, F. F., et al. (2003). Interactions between plant hormones regulate submergence-induced shoot elongation in the flooding-tolerant dicot Rumex palustris. Ann. Bot. 91, 205–211. doi: 10.1093/aob/mcf116
Vriezen, W. H., Hulzink, R., Mariani, C., and Voesenek, L. A. (1999). 1-Aminocyclopropane-1-carboxylate oxidase activity limits ethylene biosynthesis in Rumex palustris during submergence. Plant Physiol. 121, 189–196.
Weckx, S., Del-Favero, J., Rademakers, R., Claes, L., Cruts, M., De Jonghe, P., et al. (2005). NovoSNP, a novel computational tool for sequence variation discovery. Genome Res. 15, 436–442. doi: 10.1101/gr.2754005
Yang, S. F., and Hoffman, N. E. (1984). Ethylene biosynthesis and its regulation in higher plants. Annu. Rev. Plant Physiol. 35, 155–189. doi: 10.1146/annurev.pp.35.060184.001103
Ye, X. Q., Meng, J. L., Zeng, B., Wu, M., Zhang, Y. Y., and Zhang, X. P. (2016). Submergence causes similar carbohydrate starvation but faster post-stress recovery than darkness in Alternanthera philoxeroides plants. PLoS ONE 11:e0165193. doi: 10.1371/journal.pone.0165193
Yu, X., Bai, G., Liu, S., Luo, N., Wang, Y., Richmond, D. S., et al. (2013). Association of candidate genes with drought tolerance traits in diverse perennial ryegrass accessions. J. Exp. Bot. 64, 1537–1551. doi: 10.1093/jxb/ert018
Yu, X., Bai, G., Luo, N., Chen, Z., Liu, S., Liu, J., et al. (2011). Association of simple sequence repeat (SSR) markers with submergence tolerance in diverse populations of perennial ryegrass. Plant Sci. 180, 391–398. doi: 10.1016/j.plantsci.2010.10.013
Yu, X., Luo, N., Yan, J., Tang, J., Liu, S., and Jiang, Y. (2012). Differential growth response and carbohydrate metabolism of global collection of perennial ryegrass accessions to submergence and recovery following de-submergence. J. Plant Physiol. 169, 1040–1049. doi: 10.1016/j.jplph.2012.03.001
Yu, X., Pijut, P. M., Byrne, S., Asp, T., Bai, G., and Jiang, Y. (2015). Candidate gene association mapping for winter survival and spring regrowth in perennial ryegrass. Plant Sci. 235, 37–45. doi: 10.1016/j.plantsci.2015.03.003
Yuan, L. B., Dai, Y. S., Xie, L. J., Yu, L. J., Zhou, Y., Lai, Y. X., et al. (2017). Jasmonate regulates plant responses to postsubmergence reoxygenation through transcriptional activation of antioxidant synthesis. Plant Physiol. 173, 1864–1880. doi: 10.1104/pp.16.01803
Zhang, Z., Zhang, D., and Zheng, Y. (2009). Transcriptional and post-transcriptional regulation of gene expression in submerged root cells of maize. Plant Signal. Behav. 4, 132–135.
Zhou, M., Xu, M., Wu, L., Shen, C., Ma, H., and Lin, J. (2014). CbCBF from Capsella bursa-pastoris enhances cold tolerance and restrains growth in Nicotiana tabacum by antagonizing with gibberellin and affecting cell cycle signaling. Plant Mol. Biol. 85, 259–275. doi: 10.1007/s11103-014-0181-1
Keywords: association mapping, candidate gene, Lolium perenne, recovery, submergence
Citation: Wang X, Jiang Y, Zhao X, Song X, Xiao X, Pei Z and Liu H (2017) Association of Candidate Genes With Submergence Response in Perennial Ryegrass. Front. Plant Sci. 8:791. doi: 10.3389/fpls.2017.00791
Received: 25 March 2017; Accepted: 27 April 2017;
Published: 16 May 2017.
Edited by:
Rosa M. Rivero, Centro de Edafología y Biología Aplicada del Segura (CSIC), SpainReviewed by:
Hailin Guo, Institute of Botany, Jiangsu Province and Chinese Academy of Sciences, ChinaYan Xu, Ramapo College, USA
Copyright © 2017 Wang, Jiang, Zhao, Song, Xiao, Pei and Liu. This is an open-access article distributed under the terms of the Creative Commons Attribution License (CC BY). The use, distribution or reproduction in other forums is permitted, provided the original author(s) or licensor are credited and that the original publication in this journal is cited, in accordance with accepted academic practice. No use, distribution or reproduction is permitted which does not comply with these terms.
*Correspondence: Yiwei Jiang, eWppYW5nQHB1cmR1ZS5lZHU=
†These authors have contributed equally to this work.