- College of Horticulture, China Agricultural University, Beijing, China
Ethylene has long been known to be a critical signal controlling the ripening of climacteric fruits; however, the signaling mechanism underlying ethylene production during fruit development is unknown. Here, we report that two FERONIA-like receptor kinases (FERLs) regulate fruit ripening by modulating ethylene production in the climacteric fruit, apple (Malus×domestica). Bioinformatic analysis indicated that the apple genome contains 14 members of the FER family (MdFERL1–17), of these 17 FERLs, MdFERL6 was expressed at the highest level in fruit. Heterologous expression of MdFERL6 or MdFERL1, the apple homolog of Arabidopsis FER, in another climacteric fruit, tomato (Solanum lycopersicum) fruit delayed ripening and suppressed ethylene production. Overexpression and antisense expression of MdFERL6 in apple fruit calli inhibited and promoted ethylene production, respectively. Additionally, virus-induced gene silencing (VIGS) of SlFERL1, the tomato homolog of FER, promoted tomato fruit ripening and ethylene production. Both MdFERL6 and MdFERL1 physically interacted with MdSAMS (S-adenosylmethionine synthase), a key enzyme in the ethylene biosynthesis pathway. MdFERL6 was expressed at high levels during early fruit development, but dramatically declined when fruit ripening commenced, implying that MdFERL6 might limit ethylene production prior to fruit development and the ethylene production burst during fruit ripening. These results indicate that FERLs regulate apple and tomato fruit ripening, shedding light on the molecular mechanisms underlying ripening in climacteric fruit.
Introduction
Fleshy fruits are physiologically classified as climacteric or non-climacteric. Climacteric fruits exhibit an increase in respiration at the onset of ripening (Nitsch, 1953; Coombe, 1976; Brady, 1987). Studies of the mechanisms regulating fruit ripening began in the 1920s (Brady, 1987) and a major focus has been identifying the critical internal factors or signals governing this process. Ethylene has long been known to be a critical signal controlling the ripening of climacteric fruit (Biale, 1964; Burg and Burg, 1965; Alexander and Grierson, 2002), which exhibit a large increase in ethylene production at the onset of ripening. Exposure to exogenous ethylene can initiate the ripening of climacteric fruits (Seymour et al., 2013), and its effect is so great that limiting ethylene production in fruits or ethylene exposure for harvested fruit has become a major concern in the commercial cultivation industry (Brady, 1987).
The ripening of fleshy fruits is a complex process that involves dramatic changes in physiological and biochemical metabolism (Giovannoni, 2001; Seymour et al., 2013). While early studies focused on these changing patterns, more recent research has aimed to establish the molecular basis for the regulation of fruit development and ripening (Seymour et al., 2013). Several genes associated with the regulation of fruit development and ripening have been identified, but as most encode enzymes or transcription factors, little is known about the signaling mechanisms that underlie these processes to control the changes in physiological and biochemical metabolism (Fischer and Bennett, 1991; Giovannoni, 2001; Klee and Giovannoni, 2011; Seymour et al., 2013).
Most studies of fruit development-associated signaling have focused on the molecular perception of and response to ethylene in the model plant, tomato (Solanum lycopersicum; Felix et al., 1991; Wang et al., 2002; Bram et al., 2015). The mechanisms controlling the increase in ethylene production are essential for its function as a signal regulating fruit development and ripening. Ethylene production is primarily controlled by the activities of the key enzymes in its biosynthesis pathway. In the context of cellular signaling, enzyme activity may be regulated at the transcriptional or post-transcriptional level by upstream signaling cascades. While ethylene biosynthesis and its downstream signaling pathways have been studied extensively, little is known about the signaling cascades upstream of ethylene biosynthesis. However, it was shown that ethylene biosynthesis is induced by both cell wall fragments and wall-lysing enzymes. Wall fragments were implicated as possible regulators of ethylene biosynthesis in ripening citrus fruit (Citrus sp.; Baldwin and Biggs, 1988), tomato fruit (Baldwin and Pressey, 1988; Brecht and Huber, 1988), and cultured pear (Pyrus sp.) cells (Tong et al., 1986), and the wall-lysing enzymes polygalacturonase and endoxylanase were reported to induce ethylene biosynthesis in tomato fruit (Baldwin and Pressey, 1988) and tobacco (Nicotiana tabacum) leaves (Fuchs et al., 1989), respectively. Although the precise mechanism by which cell wall degradation induces ethylene biosynthesis is not known, these related studies suggest that receptors or sensors that initiate the signaling cascade upstream of ethylene biosynthesis exist in the membrane–wall complex.
Plant receptor-like kinases (RLKs) are transmembrane proteins with putative amino-terminal extracellular domains and carboxyl-terminal intracellular kinase domains (Shiu and Bleecker, 2001a; Humphrey et al., 2007; Boisson-Dernier et al., 2011; Cheung and Wu, 2011; Lindner et al., 2012). The Arabidopsis thaliana genome contains more than 600 RLK homologs with diverse functions (Shiu and Bleecker, 2001a,b, 2003). We hypothesize that RLKs function as membrane–wall complex-anchored receptors that regulate ethylene biosynthesis during fruit development and ripening. The RLK superfamily contains a subfamily with extracellular malectin or malectin-like domains that putatively bind oligosaccharides (Verica and He, 2002; Annabelle et al., 2006; Alexandre et al., 2010; Schallus et al., 2010). Oligosaccharides have increasingly been demonstrated to function as signals that activate either defensive or developmental processes in plants (Clarence and Farmer, 1991; Schallus et al., 2008), and therefore elucidating whether malectin or maletin domain-containing RLKs are implicated in the regulation of fruit development and ripening is a key research target. In a previous study, we found that the strawberry genome (Fragaria vesca) contains 62 RLK members (designated FvMRLKs) that contain malectin or malectin-like domains, and a preliminary analysis suggested that more than half of the FvMRLKs could be implicated in strawberry fruit development and ripening (Zhang et al., 2016).
Recently, several malectin domain-containing RLKs have been demonstrated to play important roles in Arabidopsis growth and development (Nissen et al., 2016); among these genes, FERONIA (FER) is of particular interest because of its diverse roles in the regulation of a series of crucial biological processes. FER was first identified for its role in fertilization (Huck et al., 2003). In Arabidopsis, FER directly interacts with Rho of plants guanine nucleotide exchange factors (RopGEFs), activating the ROP GTPase and mediating the production of reactive oxygen species (ROS) at the entrance of the female gametophyte, thereby inducing pollen tube rupture and sperm release (Kessler et al., 2010; Duan et al., 2014; Ngo et al., 2014). FER is believed to function in a variety of other important processes, such as root hair elongation (Duan et al., 2010; Huang et al., 2013), starch accumulation (Yang et al., 2015), sugar utilization (Pu et al., 2017), seed development (Yu et al., 2014), pathogen resistance (Keinath et al., 2010; Kessler et al., 2010), and vegetative growth (Guo et al., 2009; Deslauriers and Larsen, 2010). Notably, FER has been demonstrated to play a pivotal role in the cross-talk signaling among the phytohormones that play crucial roles in Arabidopsis, including abscisic acid (ABA; Yu et al., 2012; Chen et al., 2016), auxin (Duan et al., 2010), brassinosteroids (BR), and ethylene (Guo et al., 2009; Deslauriers and Larsen, 2010). Importantly, a recent study by Mao et al. (2015) reported that FER was able to physically interact with S-adenosylmethionine synthetase (SAMS) and thereby suppress ethylene biosynthesis in Arabidopsis.
Given the involvement of FER in a variety of important processes, especially in the cross-talk between phytohormones and in ethylene biosynthesis in Arabidopsis, we were particularly interested to determining whether some FER-like RLKs regulate fruit development and ripening. Apple (Malus × domestica) is one of the most popular and important fruit crops worldwide; however, due to difficulties in molecular research using apple fruit, basic research into fruit development and ripening has commonly been conducted using tomato as a model plant. In the present study, we searched the apple genome database and identified 14 FER-like RLKs. We demonstrated that two of these proteins are important regulators of fruit development and ripening. Interestingly, two of the FER-like RLKs were found to be regulators of ethylene production in fruits. This work sheds light on the mechanisms underlying climacteric fruit development and ripening, by suggesting that Feronia-like RLKs represent upstream signaling components that regulate ethylene production during ripening.
Materials and Methods
Plant Materials and Growth Conditions
Tomatoes (Solanum lycopersicum L. cv. Micro Tom) were planted in pots (diameter, 20 cm; depth, 20 cm) containing a mixture of nutrient soil, vermiculite, and organic fertilizer (4:2:1, v/v/v). Tomato seedlings were grown under standard conditions: 60% relative humidity and 25°C/18°C (day/night) under a 12 h/12 h light/dark cycle. Plants were watered daily to the drip point. Apple (Malus × domestica) callus tissue derived from the “Golden Delicious” cultivar was grown on Murashige and Skoog (MS) medium at 27°C ± 1°C in darkness, and subcultured at 10-day intervals before being used for gene transformation.
Gene Isolation and Sequence Analysis
The cDNA sequences of full-length Arabidopsis CrRLK1Ls were obtained from TAIR (http://www.arabidopsis.org). To identify FER-like (FERL) genes in apple or rice, the coding sequence of FER (At3g51550) was used as a query to BLAST the apple genome database (http://genomics.research.iasma.it/) or NCBI (https://www.ncbi.nlm.nih.gov/). Phylogenetic trees were constructed using the Neighbor-Joining (NJ) method in MEGA 4.0.2 software (Tamura et al., 2007), with 1000 bootstrap replicates performed to evaluate the reliability of the different phylogenetic groups. The deduced amino acid sequences of the MdFERLs were aligned using ClustalX 2.0.12 (Larkin et al., 2007) with default settings. The alignments were edited and marked using GeneDoc (Nicholas et al., 1997).
To isolate MdFERL1 and MdFERL6, total RNA was extracted from the fruit using an EZNATotal RNA Kit (Omega Biotek), according to the manufacturer's instructions. First-strand cDNA synthesis was conducted from 1 μg of total RNA. Full-length MdFERL1 and MdFERL6 were cloned from cDNA by PCR using Q5 High-Fidelity DNA Polymerase (New England Biolabs) and the following conditions: 98°C for 30 s (one cycle); 98°C for 30 s, 59°C for 25 s, and 72°C for 5 min (35 cycles); with a final extension of 72°C for 2 min. The PCR products were subcloned into the pCambia1304 vector and used to produce positive colonies. The primer sequences and GenBank accession numbers are shown in Supplemental Table S1.
Quantitative Reverse Transcription PCR (RT-qPCR)
RT-qPCR was performed using SYBR Premix Ex TaqTM (Takara Bio) in a 7500 Real-Time PCR System (Applied Biosystems). The expression level of each gene was analyzed in three pools of five fruits, with three measurement replicates per pool. ACTIN was used as an internal control. The 2−ΔΔCT method was used to determine transcription levels, where ΔCT represents the difference between the cycle threshold values of the target and reference genes (Schmittgen and Livak, 2008).
Spatio-Temporal Expression of MdFERLs and Ripening Marker Genes
The whole process from fruit set to ripening was classified into five stages based on days post-anthesis (DPA), namely 60DPA, 85DPA, 105DPA, 130DPA, and 155DPA. The fruit was frozen in liquid nitrogen, and kept at –80°C until required for gene expression analysis. The expression of MdFERL and ripening marker genes was assessed by RT-qPCR analysis, using primers designed in Primer3 Plus (http://www.primer3plus.com/cgi-bin/dev/primer3plus.cgi). Primer sequences are shown in Supplemental Tables S2, S3, S5. The data from five fruits were combined as an individual sample and each sample was analyzed in triplicate.
Analysis of MdFERL Expression Changes in Response to 1-MCP and ACC Treatment
For the apple fruit treatment, 5 g of fruit disks (10 mm in diameter and 1 mm in thickness) was prepared and combined from six fruits at the 105 DPA stage. The disk samples were vacuum infiltrated for 30 min in equilibration buffer (10 mM MgCl2, 50 mM MES-Tris (pH 5.5), 5 mM CaCl2, 10 mM EDTA, 200 mM mannitol, and 5 mM vitamin C) (Archbold, 1988). The samples were then shaken for 6 h at 25°C in equilibration buffer containing 1 mM ACC or 3 μM 1-MCP. After incubation in the dark for 6 h, the samples were washed and frozen immediately in liquid nitrogen, and maintained at –80°C until required. Each individual analysis was conducted with three sample replicates. Primers used for the RT-qPCR analysis of ripening-related genes are provided in Supplemental Table S2.
To treat apple calli with 1-MCP, the calli were transfected with MdFERL6-AS and empty pCambia1304 vector, and then cultivated on MS medium at 27°C in darkness. Three days after the transfection, calli were treated with 3 μM 1-MCP for 6 h and expression of the selected genes was analyzed as described above.
Transfection of Tomato Fruit and Apple Callus by Agroinfiltration
To construct vectors for the overexpression (OE) of MdFERL1 (MDP0000445374) and MdFERL6 (MDP0000465341) (abbreviated hereafter as MdFERL1-OE and MdFERL6-OE), full-length MdFERL1 and MdFERL6 were respectively cloned into the plant expression vector pCambia1304 using the KpnI and EcoRI restriction sites. The empty pCambia1304 vector and the overexpression vectors MdFERL1-OE and MdFERL6-OE were transformed individually into the Agrobacterium tumefaciens strain EHA105 (Lazo et al., 1991). For the MdFERL6-antisense (as) vector, a 621-bp fragment near the 5′ end of MdFERL6 cDNA was amplified by PCR using the primer pair 5′-GAATTCGTCCACGAACGTGTCTC -3′ (with an EcoRI restriction site; forward) and 5′- GGTACCGGATCGTTGATCTCAGAG -3′ (with a KpnI restriction site; reverse). The obtained fragment was sequenced and forward-cloned into the EcoRI and KpnI restriction sites of pCambia1304. To generate the TRV-VIGS (virus-induced gene silencing) vectors, a 595-bp fragment of SlFERL1 (GenBank accession number KY435602) was PCR-amplified from tomato cDNA. The resulting product was cloned into the vector pTRV2 to generate pTRV2-SlFERL1. The A. tumefaciens strain GV3101 containing vectors pTRV1 or pTRV2 and their derivatives was used for the VIGS experiments.
The transformed strains were grown at 28°C in Luria-Bertani liquid medium containing 10 mM MES, 20 μM acetosyringone, and the appropriate antibiotics. When the culture reached an optical density at 600 nm of approximately 0.8, the A. tumefaciens cells were harvested, resuspended in infection buffer (10 mM MgCl2, 10 mM MES (pH 5.6), and 200 mM acetosyringone), and shaken for 2 h at room temperature before being used for infiltration. For VIGS infiltration, each A. tumefaciens strain containing pTRV1 and pTRV2 or their derivative vectors was mixed at a ratio of 1:1 and infiltrated into the carpopodium of tomato fruits attached to the plant about 18 days after pollination, using a 1-mL needle-less syringe. Pairs of fruit at the same developmental stage and with similar phenotypes were selected; for each pair, one fruit was transfected with MdFERL1-OE, MdFERL6-OE, or SlFERL1-VIGS, while the other was transfected with an empty vector as the control. For each gene, 25 pairs of fruit were injected. To examine the effect of MdFERL1-OE, MdFERL6-OE, and SlFERL1-VIGS on the expression of ripening-related genes, the fruits were collected 10 dayd after transfection for MdFERL1-OE and MdFERL6-OE, and 12 days after transfection for SlFERL1-VIGS.
Callus tissues were subcultured three times at 15-day intervals before being used for gene transformation. The fresh calli were soaked for 20 min in an A. tumefaciens solution containing the MdFERL6-OE or MdFERL6-AS constructs, or the empty vector. The calluses were co-cultured on MS medium at 25°C.
Determination of Fruit Ripening-Associated Physiological Parameters
Flesh firmness was measured after skin had been removed on opposite sides of the fruit using a GY-4 fruit hardness tester (Zhejiang Top Instrument). The contents of fruit pigment, flavonoid, and total phenol were determined as described (Fuleki and Francis, 1968; Lees and Francis, 1971). The soluble sugar content was determined as described by Jia et al. (2011). Titratable acidity was calculated as malic acid. The total titratable acidity was determined using the acid-base titration method (Kafkas et al., 2007). Fruit aroma production was characterized by performing a headspace solid-phase microextraction and gas chromatography-mass spectrometry, as described by Dong et al. (2013).
Measurements of Ethylene Production
To measure ethylene production, intact fruits were enclosed in a gas-tight container (0.86 L, 24°C) equipped with septa, and 1 mL of headspace gas was sampled using a gas syringe. The ethylene concentration was measured using a gas chromatograph (GC-17A, Shimadzu). Six fruits were assessed as one sample, and three replicates were performed.
Bimolecular Fluorescence Complementation (BiFC) and Subcellular Localization Assays
For BiFC assays, the full-length coding sequences of MdFERL1, MdFERL6, MdSAMS, MdETR2, MdETR5, MdACS3, and MdACS5 were amplified and individually cloned into pCambia1300-YFP(n/c) vectors. To determine the subcellular localization of MdFERL6, the full-length cDNA sequence was amplified and fused to green fluorescent protein (GFP) in the pMDC83 plant expression vector. A coexpression analysis was conducted in tobacco (Nicotiana tabacum) leaves as described by Schütze et al. (2009). Fluorescence was observed 3 days after transformation using a confocal laser-scanning microscope (Fluoview FV1000, Olympus). Maize (Zea mays) protoplast isolation and transformation were carried out according to a protocol described by Sheen et al. (1995), with minor modifications (Li et al., 2012). The primers used are listed in Supplemental Table S4.
Co-Immunoprecipitation (CO-IP) Assay
For CoIP assays, the vector pMDC83:His-MdFERL6-GFP was used to generate the fusion protein His-MdFERL6-GFP, and the vector pCambia1300:Myc-MdSAMS-YFPn was used to generate the fusion protein Myc-MdSAMS-YFPn. The primers used are listed in Supplemental Table S4. His-MdFERL6-GFP and Myc-MdSAMS-YFPn were co-transformed into apple calli, using co-transformation of His-MdFERL6-GFP and pCambia1300-Myc-YFPn or Myc-MdSAMS-YFPn and pMDC83-GFP as control. The total proteins were extracted 3 days after transformation using extraction buffer (25 mM Tris–HCl, 1 mM EDTA, 10% glycerin, 0.5% Triton X-100, and 1 mM DTT). Protein A+G-Sepharose beads (100 μL, ComWin Biotech) were incubated with 10 μL polyclonal anti-Myc or anti-His antibody (ComWin Biotech) for 2 h at 4°C. An equal amount of anti-Myc antibody-coupled Protein A+G-Sepharose beads was added to total protein samples expressing Myc-MdSAMS-YFPn/His-MdFERL6-GFP and Myc-MdSAMS-YFPn/pMDC83-GFP, and detected with anti-His antibody. An equal amount of anti-His antibody coupled Protein A+G-Sepharose beads was added to total protein samples expressing Myc-MdSAMS-YFPn/His-MdFERL6-GFP and His-MdFERL6-GFP/ pCambia1300-Myc-YFPn and detected with anti-Myc antibody.
Ethionine Sensitivity Assay
Apple calli were transfected with MdFERL6-OE, MdFERL6-AS, and empty vector, and then cultivated on MS medium containing 0, 100, and 200 μM L-ethionine. The growth status of calli was used to reflect the activity of SAM synthase. After a 7-days incubation at 25°C, the weights of the calli were measured and MdFERL6 and MdSAMS expression were determined by qRT-PCR.
Statistical Analysis
Samples were analyzed in triplicate, and the data were noted as the mean + SD. Data were analyzed by Student's t-test using SAS software (version 8.1, USA), and the least significant difference at a 0.05 level of probability was used to explore the effect of P input on parameters. P < 0.05 was considered as significantly different, P < 0.01 was considered as highly significantly different. The statistical significance of differences between samples in Figure 6C was tested with ANOVA using the SAS software package 8.02 and the least significant difference (LSD) was used to compare the means.
Results
Genome-Wide Identification of Apple FER-Like Genes
The FER-like receptor kinases (FERLs) belong to the CrRLK1L family. To identify FER-like genes in apple, we first conducted a genome-wide screen for members of the MdCrRLK1L family. The MdCrRLK1L family consists of 34 members, which can be divided into six clades (Figure 1). A comparison of the MdCrRLK1L family with the AtCrRLK1Ls of Arabidopsis thaliana and the OsCrRLK1Ls of rice (Oryza sativa) indicated that the functionally characterized representative members of AtCrRLK1L, i.e., FERONIA, NAXUR, HERK, and THESEUS, were distributed in different clades, with FER being located in clade II. Interestingly, all members of clade I were apple CrRLK1Ls, suggesting that this group of CrRLK1Ls could be specific to the apple genome. Compared to other genes, the proteins encoded by members of clades I and clade II showed a relatively higher amino acid sequence identity with FER, and hence were designated as FERLs (FERONIA-Like receptor kinase). There were 17 MdFERL members, designated as MdFERL1–17. Clades I and II contained 12 and 5 MdFERLs, respectively. When the MdFERLs were aligned, all members were found to have an extracellular domain containing a malectin domain, which is predicted to bind carbohydrates.
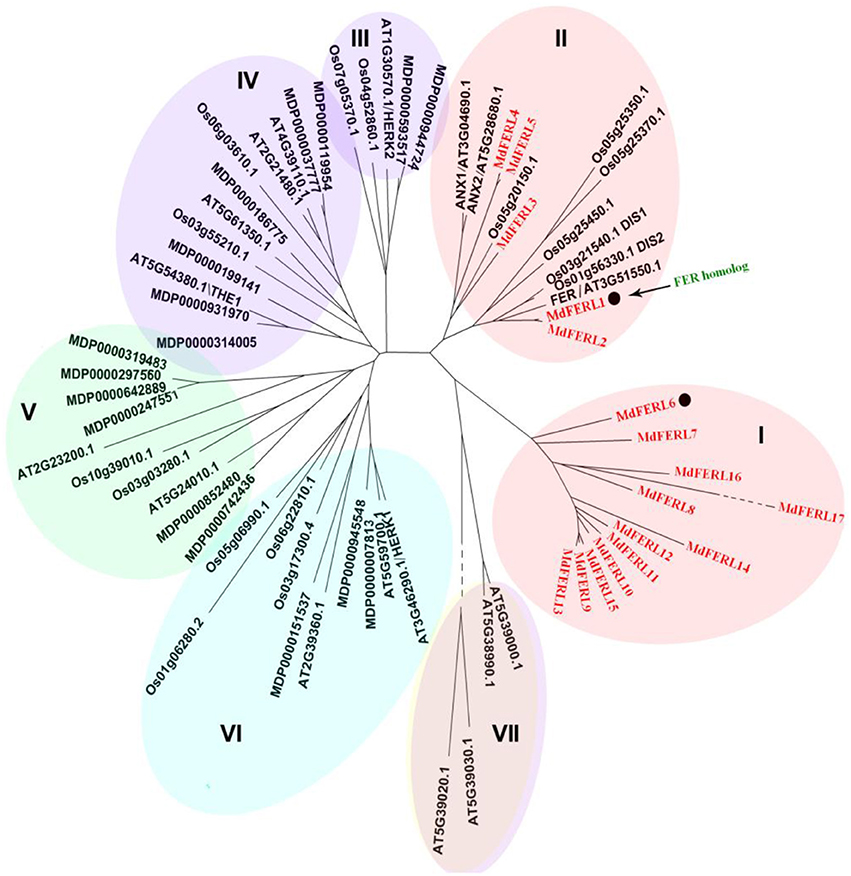
Figure 1. Phylogenetic tree of deduced CrRLK1L amino acid sequences. Phylogenetic analysis of CrRLK1L homologs from various plant species. The phylogenetic tree was constructed using the Neighbor-Joining method in MEGA4.0.2 using 1,000 bootstrap replicates. The numbers at nodes represent bootstrap values. Species name abbreviations are as follows: Md, Malus × domestica; At, Arabidopsis thaliana; Os, Oryza sativa.
Expression of MdFERLs and Ripening-Related Marker Genes during Apple Fruit Development and Ripening
The expression patterns of FERLs during fruit development and ripening are commonly correlated with their corresponding functions. To investigate the potential roles of MdFERLs in apple, we first examined their expression patterns in relation to fruit development and ripening. Apple fruit development and ripening occur between anthesis and the time when fruits are ready to be harvested (i.e., during a period of about 160 days) (Figure 2A). A preliminary examination by RT-PCR detected only six MdFERL members with a relatively high transcript level in the fruits (Supplemental Figure S1A); therefore, these six genes were further analyzed by RT-qPCR. Two of these genes, MdFERL1 and MdFERL6, were expressed more than 10- to 1,000-fold higher than the others. Strikingly, unlike the expression patterns for the other MdFERL members examined, the transcript level of MdFERL6 was high at the early stages of fruit development, but quickly declined after about 105 DPA (Figure 2B). It has been reported that ethylene production in “golden” apple fruit peaks at around 105 DAF, then remains high during the fruit development and ripening stages (Li et al., 2015). Accordingly, we found that most ethylene biosynthesis and signaling transduction genes, such as MdACO1, MdACO2, MdACS1, MdACS3, MdERF2, and MdERF3, were significantly upregulated at around 105 DPA and remained high from then onwards (Figure 2C). Comprehensive analysis of the unique expression pattern of MdFERL6 and of ethylene evolution in apple fruit, suggests that MdFERL6 regulates ethylene production in apple fruit.
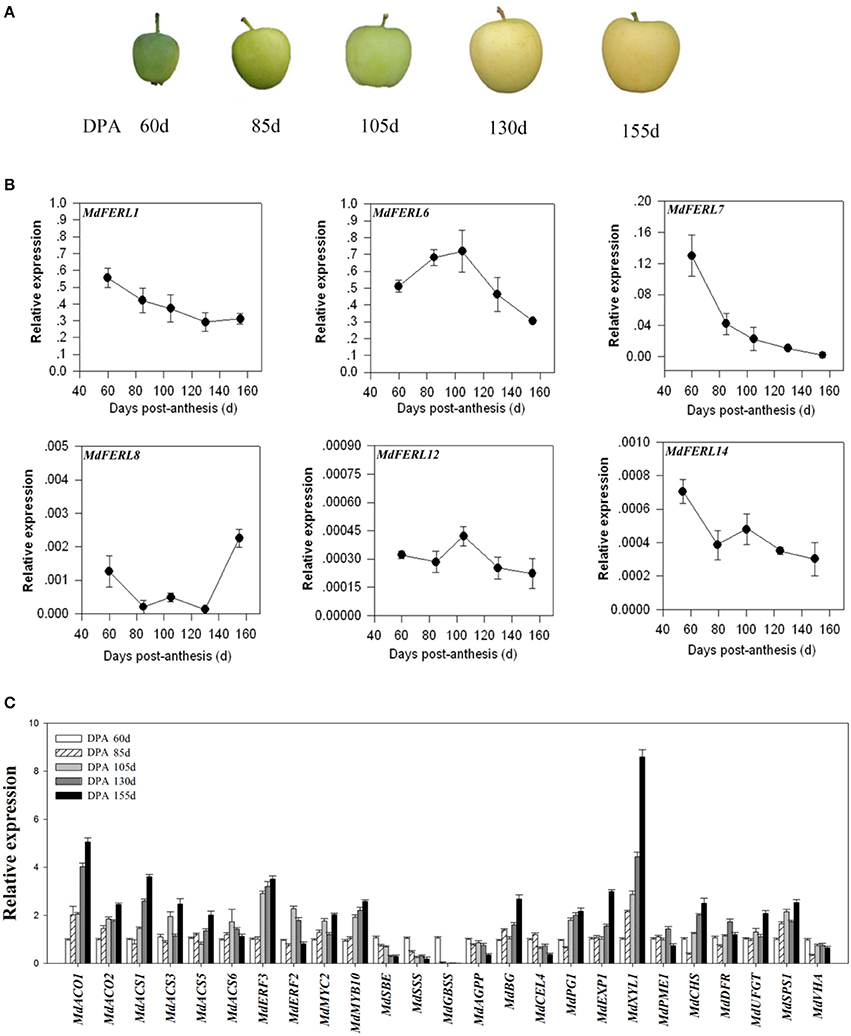
Figure 2. Quantitative reverse transcription (RT-qPCR) analysis of gene expression in apple fruit at different developmental stages. (A) Phenotypes of apple fruit at different developmental stages: days post-anthesis (DPA) 60, 85, 105, 130, and 155 days. (B) Quantitative reverse transcription (RT-qPCR) analysis of MdFERL expression at different developmental stages. (C) Quantitative reverse transcription (RT-qPCR) analysis of the expression of ripening-related genes at different developmental stages. MdACTIN was used as an internal control. Values are means + SD of three biological replicates.
To further characterize the onset of ripening in apple fruit, we evaluated the expression of ripening-related genes such as key transcription factor genes and structural genes involved in pigment accumulation, starch degradation, sugar metabolism, acid metabolism, and fruit softening (Figure 2C). Most ripening-related genes examined, including the softening genes MdBG, MdEXP1, and MdXYL1, pigment metabolism genes MdMYB10, MdCHS, MdDFR, and MdUFGT, and the sugar metabolism gene MdSPS1, were strongly up-regulated after 105 DPA. Starch biosynthesis genes did not follow this pattern, and were down-regulated at this time point. Collectively, these results suggest that MdFERL6 is a regulator of ethylene-mediated fruit ripening.
Expression of MdFERLs in Response to 1-MCP and ACC Treatment
To further explore the relationship between FERLs and ethylene in apple fruit, we examined the effects of 1-MCP, an inhibitor of ethylene perception, and ACC (1-aminocyclopropanecarboxylic acid), a precursor of ethylene, on the expression of the six MdFERLs evaluated earlier. As shown in Figure 3, MdFERL1, MdFERL6, MdFERL8, and MdFERL12 were upregulated by 1-MCP and suppressed by ACC treatment; however, MdFERL7 could only be induced by 1-MCP and MdFERL14 was only suppressed by ACC (Figure 3).
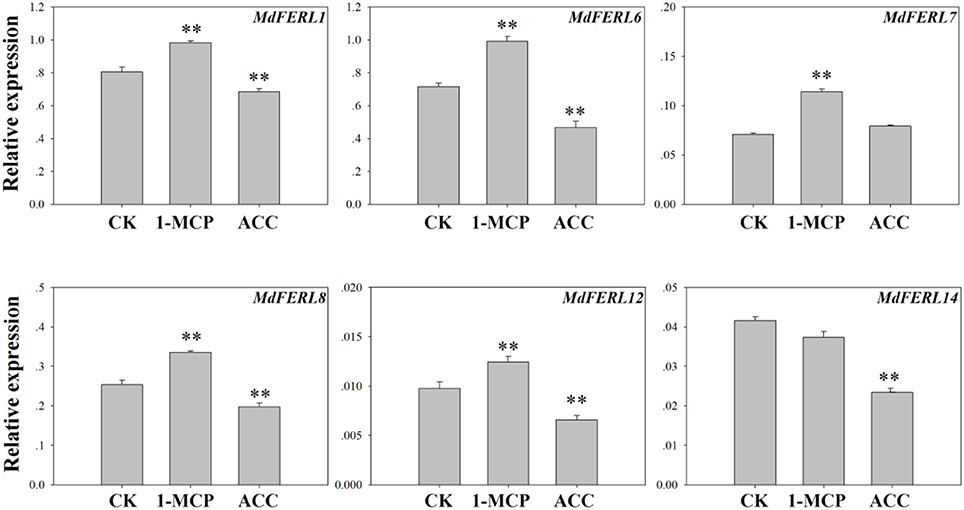
Figure 3. RT-qPCR analysis of MdFERL expression in response to 1-MCP and ACC. RT-qPCR was conducted using MdACTIN as an internal control. Values are means + SD of three biological replicates. **P < 0.01 (Student's t-test), when compared with control values.
Effect of MdFERL1 and MdFERL6 Manipulation on Physiological Parameters and Molecular Events Associated with Fruit Ripening and Quality
As mentioned above, MdFERL1 and MdFERL6 have the highest transcript levels in apple fruit among the FERLs examined. MdFERL1 has the highest level of amino acid identity with Arabidopsis FER, while MdFERL6 belongs to the group of CrRLK1Ls that appears to be specific to the apple genome. We thus examined the potential roles of MdFERL1 and MdFERL6 in fruit ripening and development. Our attempt to manipulate the expression of these genes in a variety of apple species under different conditions failed. However, methods of transgenic manipulation are well established in tomato; therefore, we heterologously expressed MdFERL1 and MdFERL6 in tomato fruit. Expressing either MdFERL1 or MdFERL6 in tomato fruit delayed ripening in comparison with the control fruit transformed with empty vector (Figure 4A). Since a dramatic increase in ethylene biosynthesis is a critical signal controlling apple and tomato fruit development and ripening, we examined the effects of MdFERL1 and MdFERL6 on ethylene production. The heterologous expression of MdFERL1 or MdFERL6 both resulted in a significant reduction in ethylene production in comparison with the control (Figure 4B). Notably, the regulatory effect of MdFERL6 on both fruit ripening (Figure 4A) and ethylene production (Figure 4B) was much stronger than that of MdFERL1. These results, in combination with the finding that MdFERL6 was potentially specific to the apple genome and that the expression pattern of MdFERL6 was most tightly associated with fruit ripening, prompted us to select this gene for further studies using overexpression and antisense manipulation in apple fruit calli. We found that MdFERL6 overexpression greatly suppressed ethylene production, and conversely, that antisense expression greatly promoted ethylene production (Figure 4C).
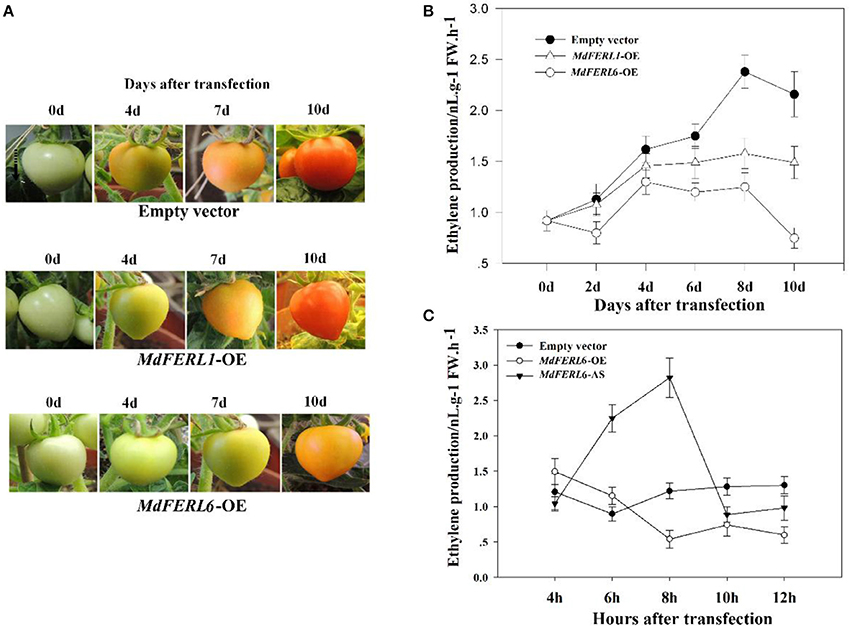
Figure 4. Effect of MdFERL1 and MdFERL6 overexpression (OE) and SlFERL1-VIGS (virus-induced gene silencing) on transgenic tomato fruit ripening. (A) The influence of MdFERL1-OE and MdFERL6-OE on the time course of tomato fruit development and ripening. (B) The effect of MdFERL1-OE and MdFERL6-OE on tomato fruit ethylene production. (C) The effect of MdFERL6-OE and MdFERL6-AS on apple (Malus × domestica) callus ethylene production.
Since the heterologous expression of MdFERL1/6 in tomato fruits in combination with the overexpression and antisense expression of MdFERL6 in apple fruit calli strongly implicated FERLs in fruit ripening and ethylene production, we investigated the effect of FERL downregulation on fruit ripening. Using VIGS, we investigated the effect of downregulating tomato FERL expression on fruit ripening. A search of the tomato genome identified a total of six FERLs, designated SlFERL1–6, which contained many relatively conserved domains (Supplemental Figure S2). SlFERL1 shared 55.3% amino acid sequence identity with FER, and could be considered a FER homolog; therefore, we selected SlFERL1 for VIGS manipulation. Unexpectedly, VIGS of SlFERL1 caused a great decrease in the transcript levels of not only SlFERL1, but also of the other five SlFERLs (Figure 5A). Furthermore, VIGS of SlFERL1 greatly promoted fruit ripening (Figure 5B) and ethylene production (Figure 5C).
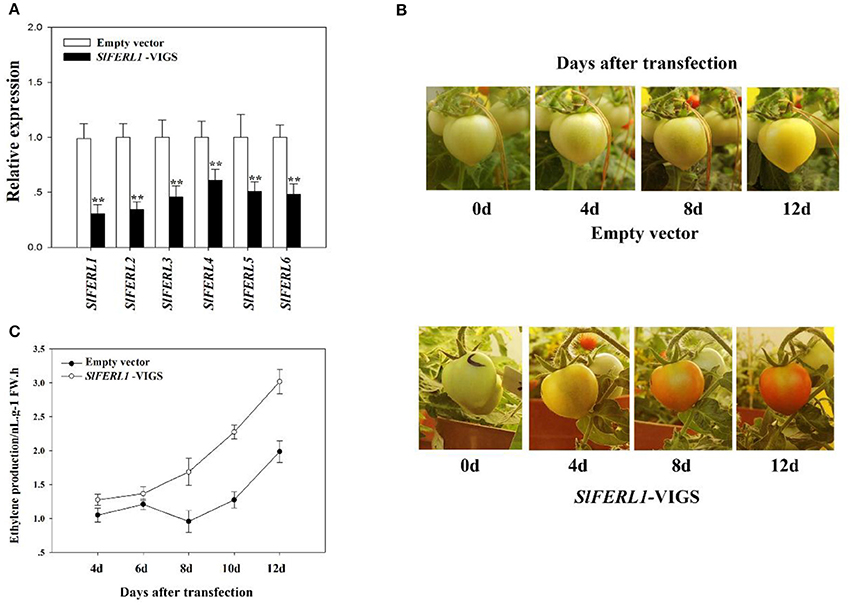
Figure 5. Effect of SlFERL1-VIGS (virus-induced gene silencing) on tomato fruit development and ripening. (A) The influence of SlFERL1-VIGS on the expression of the other SlFERLs. RT-qPCR was conducted using SlACTIN as an internal control. Values are means + SD of three biological replicates. Double asterisks denote a significant difference at P < 0.01 using Student's t-test. (B) The influence of SlFERL1-VIGS on the time course of tomato fruit development and ripening. (C) Effect of SlFERL1-VIGS on tomato fruit ethylene production.
Fruit ripening involves dramatic changes in a series of physiological parameters, such as pigment content, sugar/acid content, aroma, flavor, and texture. The effect of FERL manipulation on fruit ripening described above is reflected by a change in pigment accumulation. To decipher the roles of FERLs in fruit development and ripening, we examined their effects on some related physiological parameters. Given that VIGS-mediated silencing of SlFERL1 downregulated the expression of several other FERL members, and importantly, that it affected fruit ripening more strongly than OE, we analyzed various physiological parameters of the VIGS line. We found that SlFERL1-VIGS affected several major physiological parameters associated with fruit ripening and quality (Table 1). Strikingly, while SlFERL1-VIGS had only a small effect on fructose content, it had a major impact on both sucrose and glucose contents.
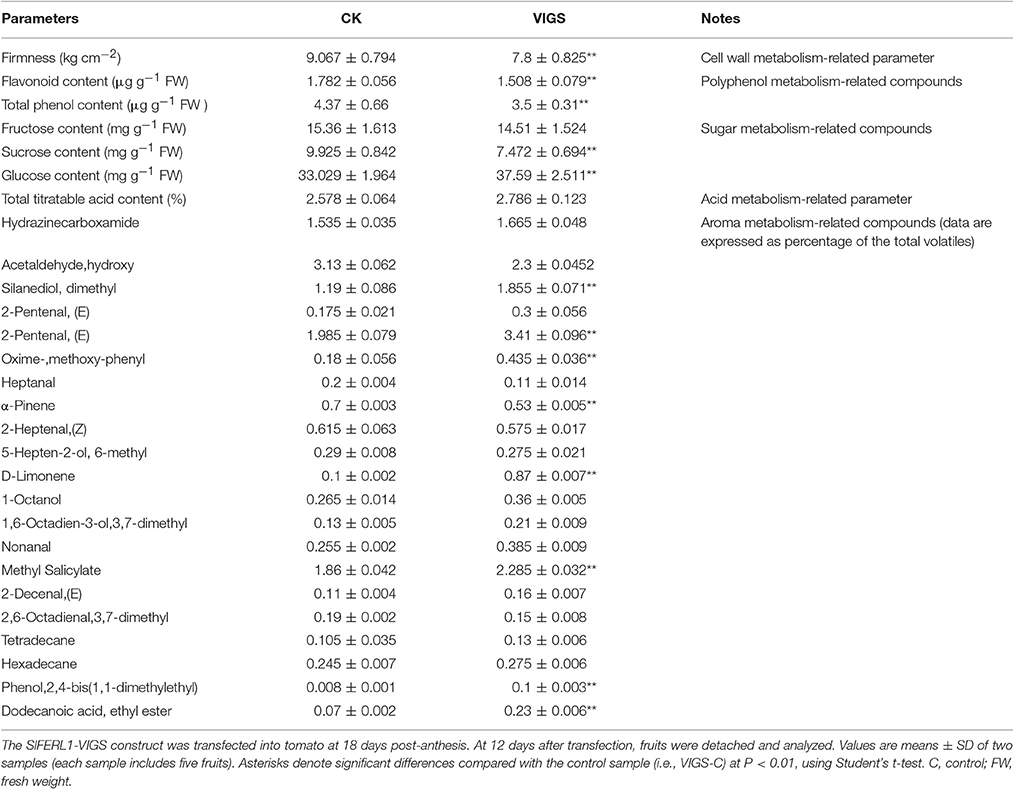
Table 1. Effects of SlFERL1-VIGS (virus-induced gene silencing) on major fruit ripening-related parameters.
To further probe the mechanisms by which MdFERLs influence fruit development and ripening, we examined the altered expression of important fruit development genes following the manipulation of the MdFERLs. Given that ethylene production was greatly affected by the changes in the expression of the MdFERLs, we focused on genes associated with ethylene biosynthesis and signaling responses, such as ACO, ACS, ERF, E4, and E8. We also examined the expression of several marker genes of fruit ripening and quality, such as CHS, F3H, ANS, and PSY, which function in pigment metabolism, SS and SPS, which are involved in fruit sugar metabolism (fruit quality), PG, PME, XYL, and EXPs, which function in cell wall metabolism (fruit texture), and RIN, CNR, and HB1, which encode ripening-related transcription factors in tomato. Heterologous expression of MdFERL1 and MdFERL6 in tomato fruits significantly suppressed the expression of most of the genes examined, except for SPS1 and SS, which were up-regulated rather than suppressed, and EXP1, which was not altered (Figure 6A). In accordance with these observations, VIGS of SlFERL1 resulted in an increase in the transcript levels of most of the genes examined, particularly ACS, E4, and E8, but the expression of SS and SPS1 decreased, while no change was observed for ACO1 or ACO2 (Figure 6B). To further reveal how MdFERL6 regulates fruit ripening and to identify its precise role in ethylene-mediated fruit ripening, we examined the expression of various genes involved in the regulation of ethylene biosynthesis, ethylene signaling transduction, and fruit ripening in apple fruit calli in which the levels of MdFERL6 had been manipulated. Overexpression of MdFERL6 caused a dramatic decrease in the expression of the ethylene biosynthesis genes MdACO1, MdACS1, MdACS5; ethylene signal transduction genes MdCTR1, MdETR2, and MdETR5; and fruit ripening-related genes MdCEL4, MdPG1, and MdXYL1 (Figure 6C). By contrast, antisense expression of MdFERL6 caused a great increase in the expression of these genes (Figure 6D). Furthermore, the expression of most of the analyzed genes was suppressed by 1-MCP treatment. Interestingly, MdACO2, MdACS5, MdETR5, MdERF1, MdCEL4, MdPG1, MdEXP1, and MdPME1 expression was less sensitive to 1-MCP treatment in apple calli harboring the MdFERL6 antisense construct (MdFERL6-AS) than in non-transgenic control calli treated with 1-MCP, while the expression of MdSS1 and MdSPS1 was more sensitive to 1-MCP treatment, suggesting that all of these genes are regulated by ethylene through MdFERL6-mediated pathways. However, compared to non-treated MdFERL6-AS calli, the expression of MdACS1, MdACS3, MdETR2, MdCTR1, MdERF2, MdXYL1, and MdEXP1 was not significantly altered by 1-MCP, suggesting that MdFERL6 also regulates fruit ripening via an ethylene-independent mechanism or that MdFERL6 functions downstream of the examined signaling proteins (e.g., MdCTR1 and MdERF2; Figure 6D). The intricate roles of MdFERL6 in the regulation of fruit ripening and ethylene signal transduction merit further investigation.
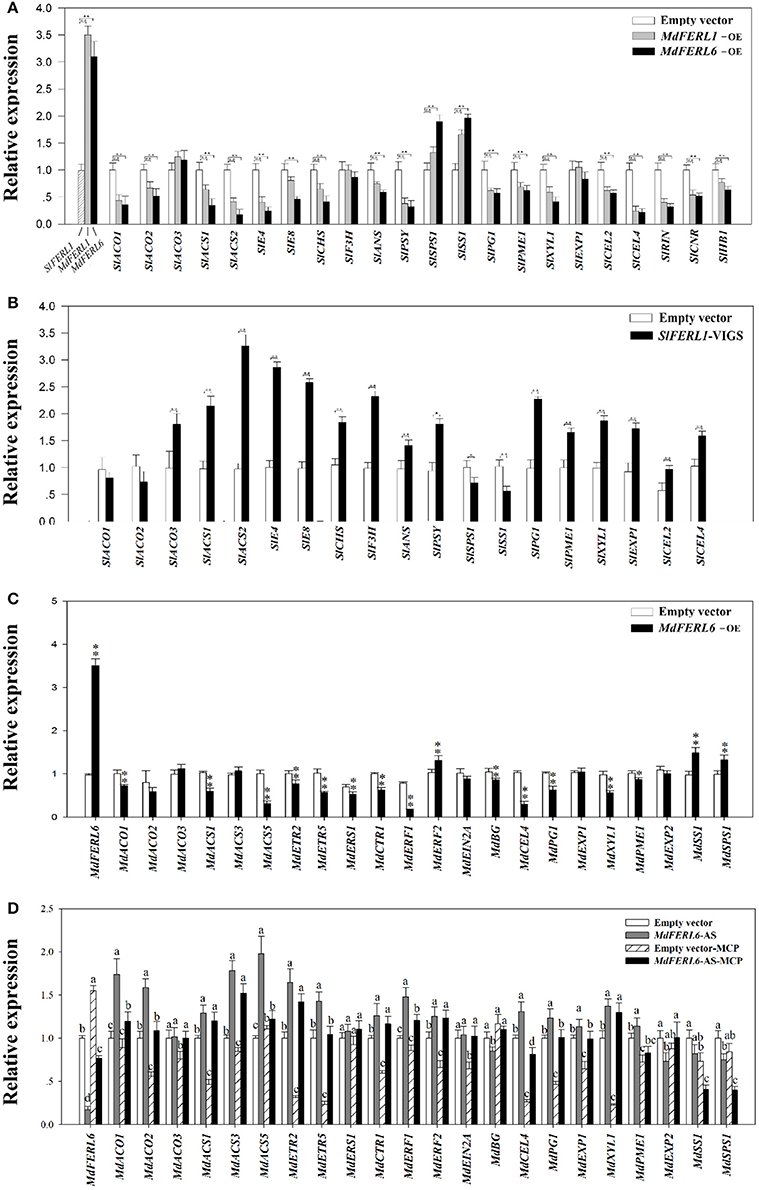
Figure 6. Effect of MdFERL1, SlFERL1, and MdFERL6 on the expression of ripening-related genes. (A) Effect of MdFERL1 and MdFERL6 on the expression of tomato ripening-related genes. The MdFERL1-OE (overexpression) and MdFERL6-OE constructs were injected into tomato fruits at 18 DPA and gene expression was analyzed 10 days after transfection. Control samples were transfected with the empty vector (pCambia1304). RT-qPCR was conducted using SlACTIN as an internal control. Values are means + SD of three biological replicates. Double asterisks denote a significant difference at **P < 0.01 and *P < 0.05 using Student's t-test. (B) Effect of SlFERL1-VIGS (virus-induced gene silencing) on the expression of tomato ripening-related genes. The VIGS constructs were injected into fruits at 18 DPA, and gene expression was examined 12 days after transfection, using RT-qPCR. SlACTIN was used as the internal control. Values are means + SD of three biological replicates. Double asterisks denote a significant difference at **P < 0.01 and *P < 0.05 using Student's t-test. (C) Effect of MdFERL6-OE on the expression of ripening-related genes in apple calli. RT-qPCR was conducted using MdACTIN as an internal control. Values are means + SD of three biological replicates; different lowercase letters represent significant differences based on ANOVA (P < 0.05). (D) Effect of MdFERL6-AS and MdFERL6-AS combined with 1-MCP on the expression of ripening-related genes in apple calli. RT-qPCR was conducted using MdACTIN as an internal control. Values are means + SD of three biological replicates; different lowercase letters represent significant differences based on ANOVA (P < 0.05).
MdFERl1 and MdFERL6 Physically Interact with MdSAMS
Given that MdFERL1 and MdFERL6 were shown to regulate ethylene production as well as ethylene responses, we further tested the possibility that these enzymes physically interact with key enzymes in the ethylene biosynthesis pathway and the signaling proteins involved in the ethylene response. The ethylene receptor ETRs are central proteins in ethylene signal transduction, and are localized to the endoplasmic reticulum. Interestingly, in addition to its localization to the membrane, MdFERL6 was also found to localize to the ER (Figure 7A). We thus tested the possibility that MdFERL6 interacts with ethylene receptors, using a BiFC assay. We also tested whether three well-characterized key enzymes in the ethylene biosynthesis pathway, SAMS, ACS, and ACO, could interact with MdFERL1 and MdFERL6. While fluorescence was not observed in the control (combination of empty vectors) or in the combinations of MdFERL6 and MdACS and of MdFERL6 and MdACO, strong fluorescence appeared when MdSAMS-YFPn was combined with MdFERL1 and MdFERL6 (Figures 7B,C). Furthermore, MdFERL6 did not appear to interact with the ethylene receptor MdETRs. The interaction between MdFERL1/6and SAMS further indicate that MdFERL1 and MdFERL6 modulate ethylene production during fruit development and ripening. To test whether MdFERL6 could interact with MdSAMS in apple calli, we conducted a co-immunoprecipitation (Co-IP) assay. As shown in Figure 7D, the Myc-MdSAMS protein complex (43 kD) was co-immunoprecipitated by His-MdFERL6 with anti-His antibody, and the His-MdFERL6-GFP complex (98 kD) was co-immunoprecipitated by Myc-MdSAMS with anti-Myc antibody. These results suggest that MdFERL1 and MdFERL6 may interact in vivo.
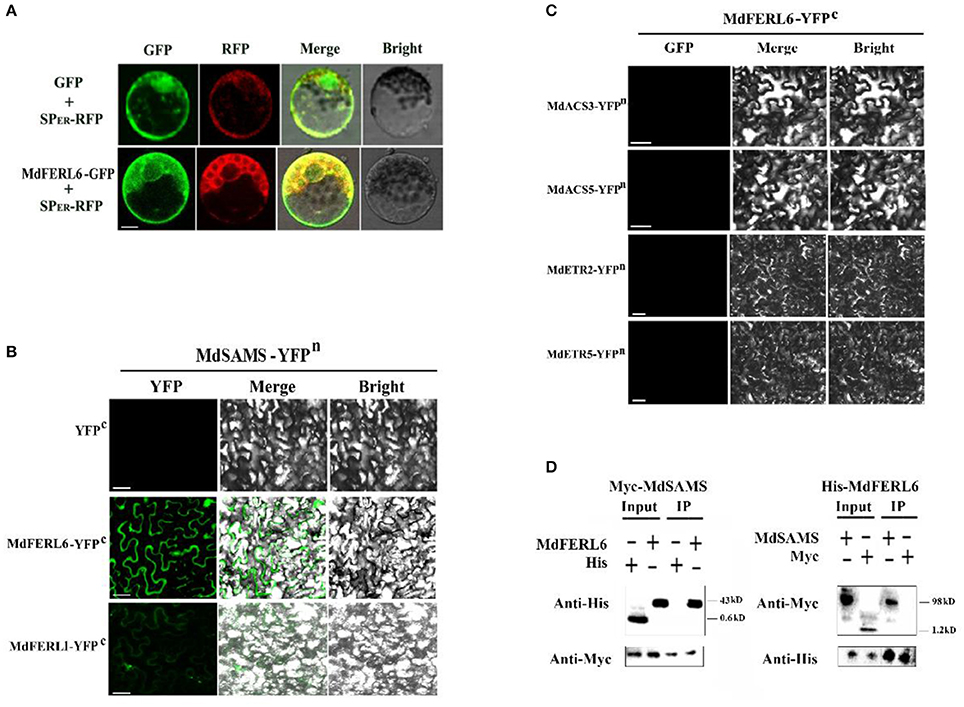
Figure 7. Subcellular localization of MdFERL6 and its physical interaction with proteins involved in ethylene production and signal transduction. (A) Subcellular localization of MdFERL6. pMDC83-MdFERL6 was transformed into maize (Zea mays) protoplasts, and fluorescence was observed by confocal microscopy. Bars = 10 μm. (B) Bimolecular fluorescence complementation (BiFC) analysis of the physical interaction between MdFERL1 or MdFERL6 and MdSAMS, fused with the C-, C-, and N-terminus, respectively, of yellow fluorescent protein (YFP; designated as YFPc or YFPn, respectively). Different combinations of the fused constructs were co-transformed into tobacco (Nicotiana tabacum) cells, and visualized using confocal microscopy. YFP and bright-field were excited at 488 nm and 543 nm, respectively. Bars = 20 μm. (C) BiFC analysis of the physical interaction between MdFERL6 and MdACS3, MdACS5, MdETR2, and MdETR5. MdFERL6 was fused to the C-terminus of YFP, while the other proteins were fused to the N-terminus of YFP. (D) Co-immunoprecipitation (Co-IP) assay of the interaction between MdFERL6 and MdSAMS in apple calli. MdFERL6 was fused with GFP/His using the pMDC83 vector, and MdSAMS was fused with Myc using the pCambia1300 vector. (The molecular weight of Myc-MdSAMS is 43 kD, Myc is 1.2 kD, His-MdFERL6 is 98 kD, and 6 × His is 0.6 kD).
Ethionine Activity Assay in MdFERL6-OE and MdFERL6-AS Apple Calli
Having demonstrated that MdFER6 physically interacts with MdSAMS in apple, we further examined the roles of the MdFERL-SAMS protein complex in ethylene production by performing an ethionine activity assay. Ethionine is a toxic analog of methionine (Met). When cultivated with ethionine, plants with higher SAMS activity absorb more ethionine, resulting in toxic symptoms. Thus, plant growth status can be used as an indicator of SAMS activity (Mao et al., 2015). As shown in Figure 8, when cultivated in the presence of ethionine, the growth status of MdFERL6-OE and MdFERL6-AS transgenic calli was altered. MdFERL6-AS transgenic calli were more sensitive than control calli to ethionine treatment. After 7 days of cultivation, MdFERL6-AS transgenic calli had become pale and gained less weight than control calli, suggesting that SMAS has higher activity in MdFERL6-AS transgenic calli than in the control. Furthermore, after 7 days of cultivation, MdFERL6-OE transgenic calli had become dark yellow and, for the 200 μM treatment, had gained more weight than the control, indicating that overexpression of MdFERL6 suppresses SAMS activity (Figures 8A,B). Furthermore, the expression of MdSAMS was increased in MdFERL6-AS and repressed in MdFERL6-OE, suggesting that MdFERL6 affects SAM synthesis through both activity level and transcription level (Figure 8C).
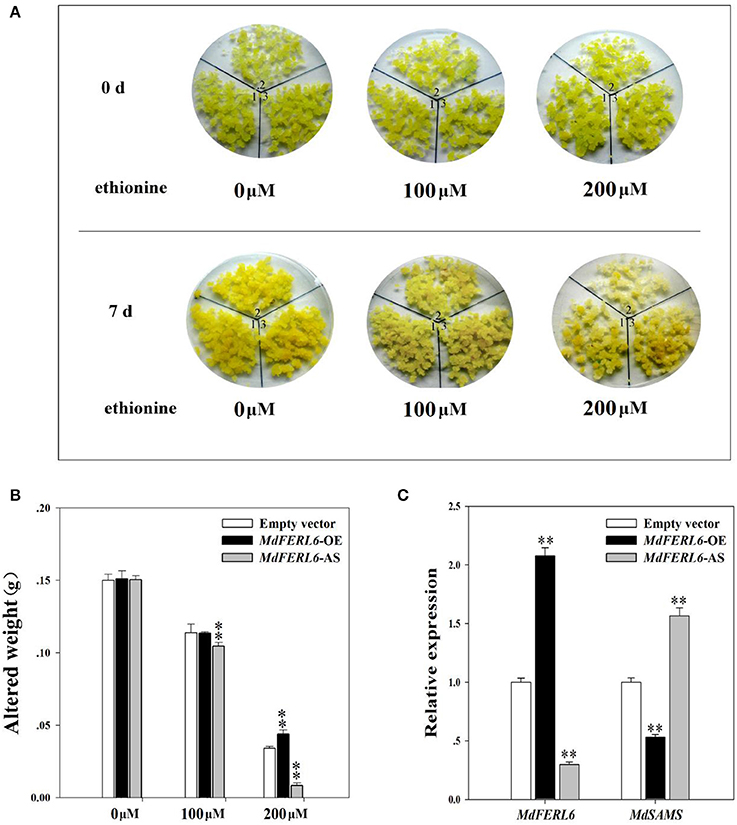
Figure 8. Ethionine activity assay. (A) Ethionine altered the growth status of apple calli. Apple calli transfected with empty vector (1); MdFERL6-AS (2); and MdFERL6-OE (3). (B) Measurement of calli weight. Transfected apple calli were weighed before treatment (0 day, and were then cultivated with ethionine for 7 days. The increase in weight between 0 and 7 days is indicated on the y-axis. Values are means + SD of three biological replicates. Single asterisks denote a significant difference at P < 0.05 using Student's t-test; double asterisks denote a significant difference at P < 0.01 using Student's t-test. (C) Gene expression of MdFERL6 and MdSAMS were detected in apple calli cultivated in 200 μM ethionine for 7 days. Values are means + SD of three biological replicates. Single asterisks denote a significant difference at P < 0.05 using Student's t-test; double asterisks denote a significant difference at P < 0.01 using Student's t-test.
Discussion
The first receptor-like protein kinase with a carbohydrate-binding domain, malectin, was identified from Catharanthus roseus, and thus named CrRLK1 (Schulze-Muth et al., 1996). Since then, CrRLK1-like receptor kinases (CrRLK1Ls) have increasingly attracted the interest of plant biologists. The genome size of rice is nearly four times that of Arabidopsis thaliana (Hong et al., 1997; Arabidopsis Genome Initiative, 2000), yet the number of CrRLK1Ls in rice (20 genes) is only slightly larger than that in Arabidopsis (17 genes; Kessler et al., 2010; Haruta et al., 2014). By contrast, the genome size of apple is only slightly larger than that of rice (Hong et al., 1997; Velasco et al., 2010), yet in the present study, we found that the CrRLK1L family in apple contains 34 members, and is therefore much larger than its counterpart in Arabidopsis and rice. In the context of plant morphology and anatomy, one of the major differences between apple and Arabidopsis or rice is the production of fleshy fruit, and accordingly, we were interested in determining whether the large difference in CrRLK1L family size between these species is correlated with fruit development. Our phylogenetic tree of CrRLK1L members from three species contained seven clades, five of which contained members from all three species. Clade I was not only specific to apple, but also contained more MdRLKs (12 members) than the other clades, while clade II contained the AtFER homolog, MdFERL1. We thus focused on MdFERLs in clades I and II in the present study.
Apple is one of the most popular and economically important fruit crops worldwide, and knowledge of the molecular mechanisms underlying apple fruit development and ripening could have beneficial applications in the apple industry. Difficulties in apple transgenesis, such as the long time required to obtain transgenic fruit and the lack of an efficient transformation system, have meant that molecular studies of apple fruit development and ripening have been limited, with most studies being performed in a tomato model. Despite extensive efforts, we failed to develop a transient transgenic system that could be successfully used for gene manipulation in apple fruits. In the present study, we examined apple fruit development and ripening using three approaches: (1) the heterologous expression of MdFERL1 and MdFERL6 in tomato fruit, (2) VIGS-mediated manipulation of SlFERL1 in tomato fruit, and (3) overexpression and antisense transformation of the apple genes in apple fruit calli. Heterologous expression of MdFERL1 and MdFERL6 in tomato fruits delayed fruit ripening, while downregulation of SlFERL1 expression dramatically promoted tomato fruit ripening. The two systems corroborate each other, collectively demonstrating the pivotal roles of MdFERL1 and MdFERL6 in fruit development and ripening. Strikingly, when compared with the heterologous expression experiment, the effect of SlFERL1 VIGS on fruit ripening was much stronger. To elucidate this response, we examined the effect of SlFERL1 VIGS on the transcript levels of the other SlFERLs we identified in the tomato genome, and found it to cause a significant decrease in the expression of all five related genes. This implies that multiple SlFERL genes play important roles in fruit development and ripening. In this study, we identified 17 MdFERLs in the apple genome, and demonstrated that two of these members, MdFERL1 and MdFERL6, regulate fruit development and ripening. To fully understand the roles of MdFERLs in these processes, the contribution of the remaining MdFERLs should be examined.
Since its identification as an essential regulator of female fertility (Huck et al., 2003; Rotman et al., 2003), FER has been found to control a series of different developmental and biological processes. Besides its critical roles in fertilization (Escobar-Restrepo et al., 2007; Duan et al., 2014; Ngo et al., 2014; Liu et al., 2016), it has also been implicated in the regulation of cell elongation (Guo et al., 2009), root hair growth (Duan et al., 2010), seed size (Yu et al., 2014), plant defense (Keinath et al., 2010; Kessler et al., 2010), and mechanosensing and calcium signaling (Shih et al., 2014). In the context of metabolism, FER was found to be a multifunctional regulator of starch accumulation (Yang et al., 2015) and sugar metabolism (Yeats et al., 2016; Pu et al., 2017). Recently, FER has been implicated in the cross-talk signaling of various phytohormones, including in ABA (Yu et al., 2012; Chen et al., 2016), auxin (Duan et al., 2010), BR, and ethylene (Guo et al., 2009; Deslauriers and Larsen, 2010) signaling. FER may also interact with SAM/SAMS, thereby suppressing ethylene production in Arabidopsis (Mao et al., 2015). We found that MdFERL6 could physically interact with MdSAMS, leading to changes in MdSAMS expression and MdSAMS activity in apple (Figures 7, 8). Phosphorylation site analysis identified five sites in MdSAMS, 134T, 153T, 250T, 271S, and 284Y, that can be potentially be phosphorylated by kinases (http://kinasephos.mbc.nctu.edu.tw/index.php). However, the mechanism by which MdFERL6 and MdSAMS interact, including the involvement of phosphorylation, remains to be elucidated. A recent study showed that a FERONIA-like kinase (MDP0000493959), which was identified as MdFERL12 in our study, was transcriptionally downregulated by ethylene during postharvest ripening and senescence of apple fruit (Zermiani et al., 2015). We found that MdFERL12 was expressed at lower levels than MdFERL1 and MdFERL6 in apple fruit (Figure 3, Supplemental Figure S1B); however, MdFERL12 could be induced by 1-MCP and other ripening-related signals such as mannitol, PEG, and high temperature (Supplemental Figure S3), suggesting that FERLs have diverse functions in regulating ethylene production and signal transduction, and that their mechanisms need to be further revealed.
Although FER has been extensively studied as described above, a potential role of FERLs in fruit development has not been reported. The present study demonstrates that FERLs are critical regulators of fruit development and ripening, further indicating the importance of FERLs as universal regulators of plant growth and development. In contrast to Arabidopsis, which has just one FER gene, the apple genome contains 14 FERLs. If each individual MdFERL member controls multiple developmental or biological processes, the mechanisms by which these proteins collectively or synergistically regulate apple plant growth and development may be of great significance, and should be thoroughly investigated.
Ethylene has long been known to be the critical signal controlling climacteric fruit ripening (Biale, 1964; Burg and Burg, 1965; Alexander and Grierson, 2002; Bram et al., 2015). One of the important features of ethylene signaling is its large increase in production during fruit ripening; therefore, any cellular internal factor controlling ethylene production should be investigated as a critical regulator of climacteric fruit development and ripening. It has been well established that there are three key enzymes in the ethylene biosynthesis pathway; SAM synthase, ACC Synthase (ACS), and ACC oxidase (ACO). SAM synthase catalyzes the first step in the ethylene biosynthesis pathway, namely the formation of S-adenosylmethionine from methionine and ATP. ACS catalyzes the formation of ACC from S-adenosylmethionine. In the present study, we revealed that both MdFERL1 and MdFERL6 physically interact with SAMS, but not with ACS or ACO. The present study suggests that multiple FER-like protein kinases physically interact with SAMS; such a variety of interactions is not possible in Arabidopsis, as it contains just one member of this family. The finding that different MdFERLs jointly regulate the same biological process reflects the significance of the existence of so many FERLs in the apple genome. As ACC is the immediate precursor of ethylene, the rate-limiting step of ethylene biosynthesis is, under most conditions, considered to be the conversion of S-adenosylmethionine to ACC by ACS (Wang et al., 2002). Thus, the regulation of ethylene synthesis via SAMS rather than ACS or ACO suggests that other rate-limiting steps exist in this pathway. In a previous study, we found that the amount of ABA that accumulated under drought stress was controlled by enzymes that function in the early stages of ABA biosynthesis, rather than by 9-cis-epoxycarotenoid dioxygenase (NCED), an enzyme that catalyzes the production of xanthin, the immediate precursor of ABA (Ren et al., 2007). Once ABA starts to accumulate, its immediate precursors are rapidly exhausted, to the extent that the early stages in the pathway become the rate-limiting steps that determine how much ABA accumulates, rather than the NCED-catalyzed step. A similar mechanism operates in ethylene production during climacteric fruit ripening; the regulation of ethylene production by FER-like protein kinases occurs at an early stage of ethylene biosynthesis (at the step catalyzed by SAMS), rather than at the downstream enzymes ACS and ACO.
In climacteric fruits, ethylene production occurs via two systems, the origins of which are key to our understanding of the mechanisms regulating fruit development and ripening. The first system is basal low rate of ethylene production, which occurs until fruit ripening commences, while the second system generates the large increase in ethylene production during fruit ripening (McMurchie et al., 1972; Hoffman and Yang, 1980; Brady, 1987). Given that both MdFERL1 and MdFERL6 suppress ethylene production, it is reasonable to propose that MdFERL1 and MdFERL6 are implicated in the first system, acting to inhibit ethylene biosynthesis before fruit ripening occurs. Nevertheless, the transcript levels of both MdFERL1 and MdFERL6 declined as apple fruit growth and ripening progressed, suggesting that MdFERL1 and MdFERL6 are likely associated with the regulation of the ethylene production spike during ripening. Notably, in contrast to the continual decline in MdFERL1 expression throughout the process of fruit set to ripening, the transcript level of MdFERL6 remained high until about 100 DPA, after which it decreased dramatically. Compared with MdFERL1, MdFERL6 had a stronger effect on fruit ripening in transgenic tomatoes, suggesting that it is the decline of the MdFERL transcripts at the later development stages that is most important for the regulation of fruit ripening. On the other hand, the different patterns of MdFERL1 and MdFERL6 expression implies that ethylene production is tightly modulated throughout the process, from fruit set to ripening; individual MdFERLs may play different roles in the two phases of ethylene production, and they collectively or synergistically determine the spatio-temporal changes in ethylene content.
In summary, we found that FERLs in apple can be categorized into two groups; some share a relatively high level of amino acid sequence identity with the FERLs in both Arabidopsis and rice, while others are less similar and appear to be specific to the apple genome. MdFERL1 and MdFERL6 belong to the first and second of these groups, respectively, and were the most highly expressed of all the MdFERLs in developing fruit. The following four findings support the notion that MdFERL1 and MdFERL6 regulate fruit ripening: (1) heterologous expression of MdFERL1 and MdFERL6 delayed fruit ripening and suppressed ethylene production in tomato; (2) overexpression and antisense silencing of MdFERL6 suppressed and promoted ethylene production, respectively; (3) VIGS ofSlFERL1 promoted fruit ripening and ethylene production; and (4) MdFERL1 and MdFERL6 were capable of physically interacting with the key ethylene biosynthesis enzyme, SAMS. As MdFERL6 and MdFERL1 suppress ethylene production during fruit development and ripening, their high transcription levels in the early stages of fruit growth followed by their dramatic decreases (especially for MdFERL6) during fruit ripening imply that they contribute to the regulation of ethylene production throughout development; i.e., they maintain ethylene at relatively low levels during the early developmental stages, and boost ethylene levels when fruit ripening commences. MdFERL1 and MdFERL6 therefore function as negative regulators of climacteric fruit ripening. This work has, for the first time, demonstrated that FER-like protein kinases are implicated in fleshy fruit development and ripening, providing new insights into the molecular basis of these processes. Nevertheless, this is a preliminary study; more research is required to elucidate the molecular recognition and signaling cascades of MdFERLs during fruit development and ripening.
Author Contributions
MJ and PD, performed most of the experiments; ND, QZ, and SX, contributed to some of the experiments; LW, YZ, WM, and JL, provided technical assistance; BL, designed the experiments and contributed to the date analysis; WJ, conceived the project, supervised the experiments, and complemented the writing.
Funding
National Natural Science Foundation of China.
Conflict of Interest Statement
The authors declare that the research was conducted in the absence of any commercial or financial relationships that could be construed as a potential conflict of interest.
Acknowledgments
This work was supported by the National Natural Science Foundation of China (Grant No. 31471851, 31672133, 31572104), Fok Ying-Tong Education Foundation, China (Grant No. 151027), the 111 Project (Grant No. B17043), and Beijing Natural Science Foundation (Grant No. 6171001).
Supplementary Material
The Supplementary Material for this article can be found online at: http://journal.frontiersin.org/article/10.3389/fpls.2017.01406/full#supplementary-material
References
Alexander, L., and Grierson, D. (2002). Ethylene biosynthesis and action in tomato: a model for climacteric fruit ripening. J. Exp. Bot. 53, 2039–2055. doi: 10.1093/jxb/erf072
Alexandre, B., Francesca, S., Alberto, M., Felice, C., and Giulia, D. L. (2010). A domain swap approach reveals a role of the plant wall-associated kinase 1 (WAK1) as a receptor of oligogalacturonides. Proc. Natl. Acad. Sci. U.S.A. 107, 9452–9457. doi: 10.1073/pnas.1000675107
Annabelle, D., Annick, T., Benot, S., Robert, B., Pierre, V. C., and Johan, M. (2006). In vitro characterization of the homogalacturonan-binding domain of the wall-associated kinase WAK1 using site-directed mutagenesis. Phytochemistry 67, 1068–1079. doi: 10.1016/j.phytochem.2006.03.009
Arabidopsis Genome Initiative (2000). Analysis of the genome sequence of the flowering plantArabidopsis thaliana. Nature 408, 796–815. doi: 10.1038/35048692
Archbold, D. D. (1988). Abscisic acid facilitates sucrose import by strawberry fruit explants and cortex disks in vitro. HortScience 23, 880–881.
Baldwin, E. A., and Biggs, R. H. (1988). Cell-wall lysing enzymes and products of cell-wall digestion elicit ethylene in citrus. Plant Physiol. 73, 58–64. doi: 10.1111/1399-3054.ep12975695
Baldwin, E. A., and Pressey, R. (1988). Tomato polygalacturonase elicits ethylene production in tomato fruit. J. Am. Soc. Hortie. Sci. 113, 92–95.
Biale, J. B. (1964). Growth, maturation, and senescence in fruits. Science 146, 880–888. doi: 10.1126/science.146.3646.880
Boisson-Dernier, A., Kessler, S. A., and Grossniklaus, U. (2011). The walls have ears: the role of plant CrRLK1Ls in sensing and transducing extracellular signals. J. Exp. Bot. 62, 1581–1591. doi: 10.1093/jxb/erq445
Brady, C. J. (1987). Fruit ripening. Annu. Rev. Plant Physiol. 38, 155–178. doi: 10.1146/annurev.pp.38.060187.001103
Bram, V., Dajo, S., and Dominique, V. (2015). Ethylene and hormonal cross talk in vegetative growth and development. Plant Physiol. 169, 61–72. doi: 10.1104/pp.15.00724
Brecht, J. K., and Huber, D. J. (1988). Products released from enzymically active cell wall stimulate ethylene production and ripening in precJimacteric tomato (Lycopersicon esculentum Mill.) fruit. Plant Physiol. 88, 1037–1041. doi: 10.1104/pp.88.4.1037
Burg, S. P., and Burg, E. A. (1965). Ethylene action and the ripening of fruits. Science 148, 1190–1196. doi: 10.1126/science.148.3674.1190
Chen, J., Yu, F., Liu, Y., Du, C., Li, X., Zhu, S., et al. (2016). FERONIA interacts with ABI2-type phosphatases to facilitate signaling cross-talk between abscisic acid and RALF peptide in Arabidopsis. Proc. Natl. Acad. Sci. U.S.A. 113, E5519–E5527. doi: 10.1073/pnas.1608449113
Cheung, A. Y., and Wu, H. M. (2011). THESEUS 1, FERONIA and relatives: a family of cell wall-sensing receptor kinases? Curr. Opin. Plant Biol. 14, 632–641. doi: 10.1016/j.pbi.2011.09.001
Clarence, A. R., and Farmer, E. E. (1991). Oligosaccharide signals in plants: a current assessment. Annu. Rev. Plam Physiol. Mol. Biol. 42, 651–674. doi: 10.1146/annurev.pp.42.060191.003251
Coombe, B. G. (1976). The development of fleshy fruits. Annu. Rev. Plant Physiol. 27, 207–228. doi: 10.1146/annurev.pp.27.060176.001231
Deslauriers, S. D., and Larsen, P. B. (2010). FERONIA is a key modulator of brassinosteroid and ethylene responsiveness in Arabidopsis hypocotyls. Mol. Plant 3, 626–640. doi: 10.1093/mp/ssq015
Dong, J., Zhang, Y., Tang, X., Jinb, W., and Hana, Z. (2013). Differences in volatile ester composition between Fragaria × ananassa and F. vesca and implications for strawberry aroma patterns. Sci Hortic. 150, 47–53. doi: 10.1016/j.scienta.2012.11.001
Duan, Q., Kita, D., Johnson, E. A., Aggarwal, M., Gates, L., Wu, H. M., et al. (2014). Reactive oxygen species mediate pollen tube rupture to release sperm for fertilization in Arabidopsis. Nat. Commun. 5:3129. doi: 10.1038/ncomms4129
Duan, Q., Kita, D., Li, C., Cheung, A. Y., and Wu, H. M. (2010). FERONIA receptor-like kinase regulates RHO GTPase signaling of root hair development. Proc. Natl. Acad. Sci. U.S.A. 107, 17821–17826. doi: 10.1073/pnas.1005366107
Escobar-Restrepo, J. M., Huck, N., Kessler, S., Gagliardini, V., Gheyselinck, J., Yang, W. C., et al. (2007). The FERONIA receptor-like kinase mediates male-female interactions during pollen tube reception. Science 317, 656–660. doi: 10.1126/science.1143562
Felix, G., Grosskopf, D. G., Regenass, M., Basse, C. W., and Boller, T. (1991). Elicitor-induced ethylene biosynthesis in tomato cells. Plant Physiol. 97, 19–25. doi: 10.1104/pp.97.1.19
Fischer, R. L., and Bennett, A. B. (1991). Role of cell wall hydrolases in fruit ripening. Annu. Rev. Plant Physiol. Plant Mol. Biol. 42, 675–703. doi: 10.1146/annurev.pp.42.060191.003331
Fuchs, Y., Saxhena, A., Gamble, H. R., and Anderson, J. D. (1989). Ethylene biosynthesis-inducing protein from cellulysin is an endoxylanase. Plant Physiol. 89, 138–143. doi: 10.1104/pp.89.1.138
Fuleki, T., and Francis, F. J. (1968). Quantitative methods for anthocyanins. J. Food Sci. 33, 266–274. doi: 10.1111/j.1365-2621.1968.tb01365.x
Giovannoni, J. (2001). Molecular biology of fruit maturation and ripening. Annu. Rev. Plant Biol. 52, 725–749. doi: 10.1146/annurev.arplant.52.1.725
Guo, H., Li, L., Ye, H., Yu, X., Algreen, A., and Yin, Y. (2009). Three related receptor-like kinases are required for optimal cell elongation in Arabidopsis thaliana. Proc. Natl. Acad. Sci. U.S.A. 106, 7648–7653. doi: 10.1073/pnas.0812346106
Haruta, M., Sabat, G., Stecker, K., Minkoff, B. B., and Sussman, M. R. (2014). A peptide hormone and its receptor protein kinase regulate plant cell expansion. Science 343, 408–411. doi: 10.1126/science.1244454
Hoffman, N. E., and Yang, S. F. (1980). Changes in l-amino-cyclopropane- l-carboxylic acid content in rpening fruits in relation to their ethylene productionrates. J. Am. Soc. Hortie. Sci. 105, 492–495.
Hong, G., Qian, Y., Yu, S., Hu, X., and Zhu, J. (1997). A120 kilobase resolution contig map of the rice genome. DNA Sequence 7, 319–335. doi: 10.3109/10425179709034052
Huang, G. Q., Li, E., Ge, F. R., Li, S., Wang, Q., Zhang, C. Q., et al. (2013). Arabidopsis RopGEF4 and RopGEF10 are important for FERONIA-mediated developmental but not environmental regulation of root hair growth. New Phytol. 200, 1089–1101. doi: 10.1111/nph.12432
Huck, N., Moore, J. M., Federer, M., and Grossniklaus, U. (2003). The Arabidopsis mutant feronia disrupts the female gametophytic control of pollen tube reception. Development 130, 2149–2159. doi: 10.1242/dev.00458
Humphrey, T. V., Bonetta, D. T., and Goring, D. R. (2007). Sentinels at the wall; cell wall receptors and sensors. New Phytol. 176, 7–21. doi: 10.1111/j.1469-8137.2007.02192.x
Jia, H. F., Chai, Y. M., Li, C. L., Lu, D., Luo, J. J., Qin, L., et al. (2011). Abscisic acid plays an important role in the regulation of strawberry fruit ripening. Plant Physiol. 157, 188–199. doi: 10.1104/pp.111.177311
Kafkas, E., Koşar, M., Paydaş, S., Kafkas, S., and Başer, K. H. C. (2007). Quality characteristics of strawberry genotypes at different maturation stages. Food Chem. 100, 1229–1236. doi: 10.1016/j.foodchem.2005.12.005
Keinath, N. F., Kierszniowska, S., Lorek, J., Bourdais, G., Kessler, S. A., Shimosato-Asano, H., et al. (2010). PAMP (pathogen-associated molecular pattern)-induced changes in plasma membrane compartmentalization reveal novel components of plant immunity. J. Biol. Chem. 285, 39140–39149. doi: 10.1074/jbc.M110.160531
Kessler, S. A., Shimosato-Asano, H., Keinath, N. F., Wuest, S. E., Ingram, G., Panstruga, R., et al. (2010). Conserved molecular components for pollen tube reception and fungal invasion. Science 330, 968–971. doi: 10.1126/science.1195211
Klee, H. J., and Giovannoni, J. J. (2011). Genetics and control of tomato fruit ripening and quality attributes. Annu. Rev. Genet. 45, 41–59. doi: 10.1146/annurev-genet-110410-132507
Larkin, M. A., Blackshields, G., Brown, N. P., Chenna, R., McGettigan, P. A., McWilliam, H., et al. (2007). Clustal W and Clustal X version 2.0. Bioinformatics 23, 2947–2948. doi: 10.1093/bioinformatics/btm404
Lazo, G. R., Stein, P. A., and Ludwig, R. A. (1991). A DNA transformation-competent Arabidopsis genomic library in Agrobacterium. Nat. Biotechnol. 9, 963–967. doi: 10.1038/nbt1091-963
Lees, D. H., and Francis, F. J. (1971). Quantitative methods for anthocyanins. J. Food Sci. 36, 1056–1060. doi: 10.1111/j.1365-2621.1971.tb03345.x
Li, B., Zhao, Y., Liang, L., Ren, H., Xing, Y., Chen, L., et al. (2012). Purification and characterization of ZmRIP1, a novel reductant-inhibited protein tyrosine phosphatase from maize. Plant Physiol. 159, 671–681. doi: 10.1104/pp.111.191510
Li, T., Tan, D., Liu, Z., Jiang, Z., Wei, Y., Zhang, L., et al. (2015). Apple MdACS6 regulates ethylene biosynthesis during fruit development involving ethylene-responsive factor. Plant Cell Physiol. 6, 1909–1917. doi: 10.1093/pcp/pcv111
Lindner, H., Muller, L. M., Boisson-Dernier, A., and Grossniklaus, U. (2012). CrRLK1L receptor-like kinases: not just another brick in the wall. Curr. Opin. Plant Biol. 15, 659–669. doi: 10.1016/j.pbi.2012.07.003
Liu, X., Claudia, C., Wang, Y., Noble, J., Ponvert, N., Bundy, M., et al. (2016). The role of LORELEI in pollen tube reception at the interface of the synergid cell and pollen tube requires the modified eight-cyste ine motif and the receptor-like kinase FERONIA. Plant Cell. 28, 1035–1052. doi: 10.1105/tpc.15.00703
Mao, D., Yu, F., Li, J., Van de Poel, B., Tan, D., Li, J., et al. (2015). FERONIA receptor kinase interacts with S-adenosylmethioninesynthetase and suppresses S-adenosylmethionine production and ethylene biosynthesis in Arabidopsis. Plant Cell Environ. 38, 2566–2574. doi: 10.1111/pce.12570
McMurchie, E. J., McGlasson, W. B., and Eaks, I. L. (1972). Treatment of fruit with propylene gives information about the biogenesis of ethylene. Nature 237, 235–236. doi: 10.1038/237235a0
Ngo, Q. A., Vogler, H., Lituiev, D. S., Nestorova, A., and Grossniklaus, U. (2014). A calcium dialog mediated by the FERONIA signal transduction pathway controls plant sperm delivery. Dev. Cell. 29, 491–500. doi: 10.1016/j.devcel.2014.04.008
Nicholas, K. B., Nicholas, H. B. Jr., and Deerfield, D. W. (1997). GeneDoc: analysis and visualization of genetic variation. Embnew. News 4:14.
Nissen, K. S., Willats, W. G. T., and Malinovsky, F. G. (2016). Understanding CrRLK1L function: cell walls and growth control. Trends Plant Sci. 21, 516–527. doi: 10.1016/j.tplants.2015.12.004
Nitsch, J. P. (1953). The physiology of fruit growth. Annu. Rev. Plant Physiol. 4, 199–236. doi: 10.1146/annurev.pp.04.060153.001215
Pu, C., Han, Y., Zhu, S., Song, F., and Zhao, Y. (2017). The rice receptor-like kinases DWARF and RUNTISH SPIKELET1 and 2 repress cell death and affect sugar utilization during reproductive development. Plant Cell 29, 70–89. doi: 10.1105/tpc.16.00218
Ren, H., Gao, Z., Chen, L., Wei, K., Liu, J., Fan, Y., et al. (2007). Dynamic analysis of ABA accumulation in relation to the rate of ABA catabolism in maize tissues under water deficit. J. Exp. Bot. 58, 211–219. doi: 10.1093/jxb/erl117
Rotman, N., Rozier, F., Boavida, L., Dumas, C., Berger, F., and Faure, J. E. (2003). Female control of male gamete delivery during fertilization in Arabidopsis thaliana. Curr. Biol. 13, 432–436. doi: 10.1016/S0960-9822(03)00093-9
Schallus, T., Feher, K., Sternberg, U., Rybin, V., and Muhle, C. (2010). Analysis of the specific interactions between the lectin domain of malectin and diglucosides. Glycobiology 20, 1010–1020. doi: 10.1093/glycob/cwq059
Schallus, T., Jaeckh, C., Feher, K., Palma, A. S., Liu, Y., Simpson, J. C., et al. (2008). Malectin: a novel carbohydrate-binding protein of the endoplasmic reticulum and a candidate player in the early steps of protein N-glycosylation. Mol. Biol. Cell. 19, 3404–3414. doi: 10.1091/mbc.E08-04-0354
Schmittgen, T. D., and Livak, K. J. (2008). Analyzing real-time PCR data by the comparative CT method. Nat. Protoc. 3, 1101–1108. doi: 10.1038/nprot.2008.73
Schulze-Muth, P., Irmler, S., Schröder, G., and Schröder, J. (1996). Novel type of receptor-like protein kinase from a higher plant (Catharanthus roseus). cDNA, gene, intramolecular autophosphorylation, and identification of a threonine important for auto- and substrate phosphorylation. J. Biol. Chem. 271, 26684–26689. doi: 10.1074/jbc.271.43.26684
Schütze, K., Harter, K., and Chaban, C. (2009). Bimolecular fluorescence complementation (BiFC) to study protein-protein interactions in living plant cells. Methods Mol. Biol. 479, 189–202. doi: 10.1007/978-1-59745-289-2_12
Seymour, G. B., Østergaard, L., Chapman, N. H., Knapp, S., and Martin, C. (2013). Fruit development and ripening. Annu. Rev. Plant Physiol. 64, 219–241. doi: 10.1146/annurev-arplant-050312-120057
Sheen, J., Hwang, S., Niwa, Y., Kobayashi, H., and Galbraith, D. W. (1995). Green-fluorescent protein as a new vital marker in plant cells. Plant J. 8, 777–784. doi: 10.1046/j.1365-313X.1995.08050777.x
Shih, H. W., Miller, N. D., Dai, C., Spalding, E. P., and Monshausen, G. B. (2014). The receptor-like kinase FERONIA is required for mechanical signal transduction in Arabidopsis seedlings. Curr. Biol. 24, 1887–1892. doi: 10.1016/j.cub.2014.06.064
Shiu, S. H., and Bleecker, A. B. (2001a). Plant receptor-like kinase gene family: diversity, function, and signaling. Sci. STKE 113, 1–13. doi: 10.1126/stke.2001.113.re22
Shiu, S. H., and Bleecker, A. B. (2001b). Receptor-like kinases from Arabidopsis form a monophyletic gene family related to animal receptor kinases. Proc. Natl. Acad. Sci. U.S.A. 98, 10763–10768. doi: 10.1073/pnas.181141598
Shiu, S. H., and Bleecker, A. B. (2003). Expansion of the receptor-like kinase/Pelle gene family and receptor-like proteins in Arabidopsis. Plant Physiol. 132, 530–543. doi: 10.1104/pp.103.021964
Tamura, K., Dudley, J., Nei, M., and Kumar, S. (2007). MEGA4: molecular evolutionary genetics analysis (MEGA) software version 4.0. Mol. Biol. Evol. 24, 1596–1599. doi: 10.1093/molbev/msm092
Tong, C. B., Labavitch, J. M., and Yang, S. F. (1986). The induction of ethylene production from pear cell culture by cell wall fragments. Plant Physiol. 81, 929–930. doi: 10.1104/pp.81.3.929
Velasco, R., Zharkikh, A., Affourtit, J., Dhingra, A., and Cestaro, A. (2010). The genome of the domesticated apple (Malus × domestica Borkh.). Nat. Genet. 42, 833–839. doi: 10.1038/ng.654
Verica, J. A., and He, Z.-H. (2002). The Cell Wall-Associated Kinase (WAK) and WAK-Like Kinase Gene Family. Plant Physiol. 129, 455–459. doi: 10.1104/pp.011028
Wang, K., Li, H., and Ecker, J. R. (2002). Ethylene Biosynthesis and Signaling Networks. Plant Cell 14, S131–S151. doi: 10.1105/tpc.001768
Yang, T., Wang, L., Li, C., Liu, Y., Zhu, S., Qi, Y., et al. (2015). Receptor protein kinase FERONIA controls leaf starch accumulation by interacting with glyceraldehyde-3-phosphate dehydrogenase. Biochem. Biophys. Res. Commun. 465, 77–82. doi: 10.1016/j.bbrc.2015.07.132
Yeats, T. H., Sorek, H., Wemmer, D. E., and Somerville, C. R. (2016). Cellulose deficiency is enhanced on hyper accumulation of sucrose by a H+-coupled Sucrose symporter. Plant Physiol. 171, 110–124. doi: 10.1104/pp.16.00302
Yu, F., Li, J., Huang, Y., Liu, L., Li, D., Chen, L., et al. (2014). FERONIA receptor kinase controls seed size in Arabidopsis thaliana. Mol. Plant 7, 920–922. doi: 10.1093/mp/ssu010
Yu, F., Qian, L., Nibau, C., Duan, Q., Kita, D., Levasseur, K., et al. (2012). FERONIA receptor kinase pathway suppresses abscisic acid signaling in Arabidopsis by activating ABI2 phosphatase. Proc. Natl. Acad. Sci. U.S.A. 109, 14693–14698. doi: 10.1073/pnas.1212547109
Zermiani, M., Zonin, E., Nonis, A., Begheldo, M., Ceccato, L., Vezzaro, A., et al. (2015). Ethylene negatively regulates transcript abundance of ROP-GAP rheostat-encoding genes and affects apoplastic reactive oxygen species homeostasis in epicarps of cold stored apple fruits. J. Exp. Bot. 66, 7255–7270. doi: 10.1093/jxb/erv422
Keywords: apple, ethylene production, fruit ripening, FERONIA-like receptor kinase, tomato, VIGS
Citation: Jia M, Du P, Ding N, Zhang Q, Xing S, Wei L, Zhao Y, Mao W, Li J, Li B and Jia W (2017) Two FERONIA-Like Receptor Kinases Regulate Apple Fruit Ripening by Modulating Ethylene Production. Front. Plant Sci. 8:1406. doi: 10.3389/fpls.2017.01406
Received: 13 March 2017; Accepted: 28 July 2017;
Published: 10 August 2017.
Edited by:
Yong-Ling Ruan, University of Newcastle, AustraliaReviewed by:
Hiroshi Ezura, University of Tsukuba, JapanBenedetto Ruperti, University of Padua, Italy
Shaohua Zeng, South China Institute of Botany (CAS), China
Copyright © 2017 Jia, Du, Ding, Zhang, Xing, Wei, Zhao, Mao, Li, Li and Jia. This is an open-access article distributed under the terms of the Creative Commons Attribution License (CC BY). The use, distribution or reproduction in other forums is permitted, provided the original author(s) or licensor are credited and that the original publication in this journal is cited, in accordance with accepted academic practice. No use, distribution or reproduction is permitted which does not comply with these terms.
*Correspondence: Bingbing Li, bGliaW5nYmluZ0BjYXUuZWR1LmNu
Wensuo Jia, amlhd3NAY2F1LmVkdS5jbg==
†These authors have contributed equally to this work.