- 1Scientific and Technological Bioresource Nucleus BIOREN-UFRO, Universidad de La Frontera, Temuco, Chile
- 2Department of Soil Microbiology and Symbiotic Systems, Estación Experimental del Zaidín-Consejo Superior de Investigaciones Científicas, Granada, Spain
- 3Departamento de Ciencias Químicas y Recursos Naturales, Universidad de La Frontera, Temuco, Chile
- 4Section Ecology and Evolution, Biological Institute, University of Copenhagen, Copenhagen, Denmark
Wheat (Triticum aestivum L.) and barley (Hordeum vulgare L.) are major crops cultivated around the world, thus playing a crucial role on human diet. Remarkably, the growing human population requires a significant increase in agricultural production in order to feed everybody. In this context, phosphorus (P) management is a key factor as it is component of organic molecules such as nucleic acids, ATP and phospholipids, and it is the most abundant macronutrient in biomass after nitrogen (N), although being one of the scarcest elements in the lithosphere. In general, P fertilization has low efficiency, as only a fraction of the applied P is acquired by roots, leaving a substantial amount to be accumulated in soil as not readily available P. Breeding for P-efficient cultivars is a relatively low cost alternative and can be done through two mechanisms: i) improving P use efficiency (PUE), and/or ii) P acquisition efficiency (PAE). PUE is related to the internal allocation/mobilization of P, and is usually represented by the amount of P accumulated per biomass. PAE relies on roots ability to acquire P from the soil, and is commonly expressed as the relative difference of P acquired under low and high P availability conditions. In this review, plant adaptations related to improved PAE are described, with emphasis on arbuscular mycorrhizal (AM) symbiosis, which is generally accepted to enhance plant P acquisition. A state of the art (1980–2018) of AM growth responses and P uptake in wheat and barley is made to discuss about the commonly accepted growth promoting effect and P increased uptake by AM fungi and the contrasting evidence about the generally accepted lack of positive responses in both plant species. Finally, the mechanisms by which AM symbiosis can affect wheat and barley PAE are discussed, highlighting the importance of considering AM functional diversity on future studies and the necessity to improve PAE definition by considering the carbon trading between all the directly related PAE traits and its return to the host plant.
Introduction
Cereals have been cultivated for more than 10,000 years, playing a crucial role in the development of human civilization. Today, cereals are still important, being the principal crops harvested in the world with more than 2.8 Gt of combined grain production (FAO, 2013). Among major cereals, the widespread and closely related wheat (Triticum aestivum L.) and barley (Hordeum vulgare L.) represents 31% of global grain yield (El Rabey et al., 2015). Cereals are also the major component of human diet worldwide with more than 50% of daily caloric intake, with values exceeding 80% in the poorest countries (Awika, 2011). Agricultural practices and technology have greatly improved over the last decades to reduce problems associated with food scarcity and to provide cereals for the daily diet. However, risks and unprecedented challenges still remain considering that global food, and grain production must increase a 70% by the year 2050 as world population is expected to be reach 9 billion people (FAO, 2012). Meanwhile, the slight increase in crop yields observed since the 1980s and the scarcity of available land suitable for production make the focus on reducing crop losses empirical due to various kinds of biotic and abiotic stresses factors, such as pathogen attack, cold, heat, drought, salt, deficiency of nutrients as phosphorous (P), and phytotoxicity by heavy metal stresses (Ray et al., 2012; Bhardwaj et al., 2014).
P fertilizers are manufactured from rock phosphate found only in a few places in the world, being Morocco the owner of 85% of the known active mining reserves. As a non-renewable resource, rock phosphate, as well as other non-renewable resources such as oil and coal is expected to become scarce near the 2030s (Cordell et al., 2009), or more optimistically within two to three centuries (Sattari et al., 2012). The market and countries are already responding to this scenario, which is reflected in the fact that both USA and China (the biggest P producer in the world) have stopped exporting this resource (van de Wiel et al., 2016). In addition, P fertilizers may cause environmental problems associated with eutrophication (Gaxiola et al., 2001) and can contain heavy metals such as cadmium that may accumulate in arable soils as a result of the addition of rock phosphate (van de Wiel et al., 2016). Remarkably, usually only about 10–30% of the P fertilizer applied in the first year is taken up by the roots, with a substantial part accumulated in the soil as residual P not readily available for plants (Syers et al., 2008). In alkaline soils, P can be bound to calcium, and in acidic soils it can be readily complexed to charged Al and Fe oxides and groups hydroxyls on clay surfaces (Kochian et al., 2004; Seguel et al., 2013), limitations that can occur in ca. 30% of arable soils worldwide (Kochian, 2012). Moreover, organic material present in the soil (e.g., from manure or crop residues) can also bind phosphate ions as well as phytate (inositol compounds).
Ideally, P taken up by agricultural products should represent the same amount of applied P fertilizer, achieving a neutral balance (Helyar, 1998; Syers et al., 2008). However, this situation is often only achieved in low input, low production farming systems (e.g., Burkitt et al., 2007; McIvor et al., 2011), on intrinsically low P-buffering capacity soils in productive agriculture (e.g., sands), or where P-buffering capacity is low because sorption sites for P are close to saturation and soil P availability is relatively high (e.g., Syers et al., 2008). Elsewhere, P-balance is relatively low, which contributes to an inefficient P use (Richardson et al., 2011). Thus, P management must be improved in order to enhance plant uptake in soils, as well as using the less available and bound-P through a better understanding of the processes happening in the soil-plant systems.
Phosphorus in the Soil-Plant Continuum
In general, P is present in plants either as organic phosphate esters or as free inorganic orthophosphate forms, representing up to ca. 0.2% of plants dry weight, making it the most abundant macronutrient in plants after nitrogen (N). However, unlike N, the amount of P available for agriculture is finite (Bovill et al., 2013). When compared to other essential macronutrients, P is one of the less-abundant elements in the lithosphere (0.1% of the total). P is an important component of organic molecules such as nucleic acids, ATP and phospholipids; thus playing a crucial role in energy metabolism of both plants and animals (Abel et al., 2002; Vance et al., 2003). Phosphate is also involved in signal-transduction pathways via phosphorylation/dephosphorylation, hence regulating key enzyme reactions in general cellular metabolism, including N fixation on N-fixing plants (Theodorou and Plaxton, 1996; Schachtman et al., 1998; Marschner, 2012).
Plants acquire P from the soil solution predominately as inorganic phosphate (Pi) (H2/), having maximal uptake rates at pH 5-6 (Holford, 1997; Rae et al., 2003; Marschner, 2012). It acquisition occurs by a plasma membrane-localized phosphate transporter-mediated process, which has been suggested to operate as a H+ co-transporter (Rae et al., 2003; Raghothama, 2005). Phosphate transporters are classified into four families: Pht1, Pht2, Pht3, and Pht4, which are located on the plasma membrane, plastidial membrane, mitochondrial membrane, and Golgi-compartment, respectively (Lopez-Arredondo et al., 2014). Two different uptake systems have been proposed: one with high-affinity, which is regulated by Pi availability and activated mainly under P deficiency, with a Km of 3–7 μM; and the other is a low-affinity system constitutively expressed system with Km of 50–300 μM (Bucher et al., 2001; Preuss et al., 2010; Liu et al., 2011; Tian et al., 2017). Despite of having a high-affinity acquisition system, P has a low availability and poor mobility in the soil, being one of the most inaccessible elements for plants (Holford, 1997). Concentrations of available P in soil solution are extremely low, being generally lower than 10 μM (Holford, 1997; do Nascimento et al., 2016), whereas in wheat leaves and stems concentrations of over 100 mM can be achieved (Bauer et al., 1987; Seguel et al., 2017). Therefore, as plants normally take up P faster than it is supplied by diffusion a depletion zone around the root system is quickly created, inducing P deprivation (Figure 1A; Hinsinger, 2001).
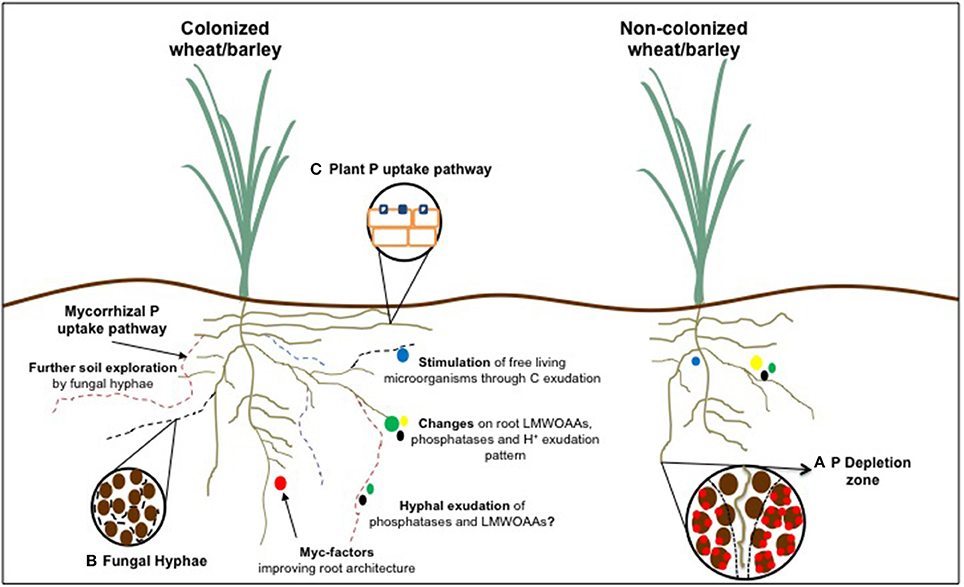
Figure 1. Phosphorus acquisition efficiency related traits of wheat and barley roots affected by arbuscular mycorrhizal symbiosis in comparison to a non-colonized counterpart. (A) Representation of P depletion zone around the rhizosphere; (B) Access to smaller soil pores by AM fungal hyphae; and (C) Modulation of plant P transporters following colonization.
The rhizosphere encompasses the first millimeters of the soil surrounding plant roots, where biological and ecological complex processes occur. This is the critical zone for P dynamics as plants roots are capable of modifying this environment through their physiological activities, especially by exudation of organic acid anions, enzymes, secondary metabolites and sugars (Bais et al., 2006; Giles et al., 2017). These processes not only determine solubilization/mineralization, acquisition of soil nutrients and microbial dynamics, but also control the efficiency of nutrient use by plants and crops, therefore influencing productivity and sustainability of the agroecosystems (Hinsinger et al., 2009; Zhang et al., 2010).
Phosphorus Efficiency
Great efforts have been made in the last decade concerning P efficiency. In this sense, agronomic strategies for increasing P fertilizer availability to crops has been developed, for example, by applying liquid fertilizers (Holloway et al., 2001) or by localized fertilizer placement (Ma et al., 2009). However, those techniques require modern technologies and increase operational costs. On the other hand, breeding for P-efficient crop cultivars has been advocated due to its relatively low cost, providing benefits to both high and low-input systems (Rose et al., 2010).
Despite the growing knowledge in the field, there is still controversy in the concept and measurement of efficiency, as it has many definitions, and even different terms are often used although they are calculated in the same way (Bovill et al., 2013). Nowadays, P efficiency is understood as two different mechanisms: i) the internal efficiency of allocation/mobilization of P in order to produce higher biomass with lower input, and ii) plant ability to acquire P from the soil, also known as P acquisition efficiency (Wang et al., 2010; Rose and Wissuwa, 2012; Sandaña and Pinochet, 2016).
The internal use efficiency or P use efficiency (PUE) is here defined as the amount of P accumulated in the tissue per biomass unit (shoot and/or root) or grain produced (Rose and Wissuwa, 2012). It is related to a range of metabolic modifications that can occur for reducing P demand during plant development (Hammond et al., 2009; Vaneklaas et al., 2012). Improving internal PUE will lead to a more resource-efficient use of P rather than increasing uptake of potentially scarce P forms, as in theory less P will be acquired by crops, minimizing P fertilizer requirement and removal from fields. However, to date no crop species or genotypes within species are known to be capable of reducing its net P uptake if the demand is reduced (Rose and Wissuwa, 2012). This is operating in sandy or low P-soption capacity soils. On the contrary, in soils rich in sorbed P, which are observed in the majority of acid soils, breeding programs focused on the optimization of P scavenging mechanisms would be a key role to improve P efficiency. Consenquently, this review has been mainly focused on P acquisition efficiency.
Phosphorus Acquisition Efficiency
While PUE aims to produce more biomass with lesser P costs, P acquisition efficiency (PAE) is related to enhancing its acquisition from soil, especially from unavailable forms, and for this purpose root traits are a key factor. PAE is commonly expressed in the literature as the relative difference of P taken up in low and high P availability conditions (Vandamme et al., 2013; Seguel et al., 2015, 2017). However, this definition does not take into account the root traits involved. In this sense, Liao et al. (2008) made a more realistic approximation by integrating root length and root biomass. Nevertherless, other traits related to root architecture and physiolgy must be integrated in the PAE definition due to their key role in uptake as discussed below (Figure 2).
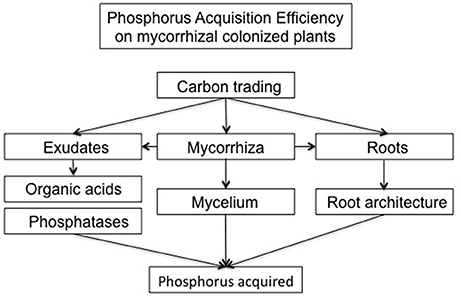
Figure 2. General scheme showing the proposed PAE determination based on carbon trading between all directly related P acquisition traits in AM colonized plants.
Root Architecture
P status is a major factor modulating root architecture, being a higher root-to-shoot ratio the most evident change in the majority of plants experiencing P deprivation (Wissuwa et al., 2005; Gruber et al., 2013). Phosphate, the available form of P, presents a heterogeneous distribution (patches) given its high affinity for the soil matrix. Root P gathering implies a continuous root growth due to the quickly depletion of rhizosphere P and the need of looking for new hotspots in soils (Figure 1A).
The upper soil layer (0–10 cm)—known as topsoil—is the zone where P availability for plants and microorganisms is generally higher, mainly due fertilizers input in the surface and its poor mobility throught soil profile. Important adaptations of plants to access this richer environment are the development of axial roots with shallower angle, enhancing adventitious rooting, and greater density and dispersion of lateral roots and root hairs (Wang et al., 2004; Lynch, 2007). These traits, together with root length, diameter and surface area comprise the most important inter- and intra-specifically functional variations of plant root adaptations for PAE for most plant species. During their screening for traits directly related to PAE, Manske et al. (2000) found that higher root length density in top soil of wheat crops was the most important root trait for P uptake, which was positively correlated with enhanced recovery of fertilized P. Basal roots in some legumes (as bean and soybean) appear in distinct nodes or “whorls,” which affect root growth angles and therefore top soil exploration. Differences of up to 100% of improved P acquisition can be found in common bean cultivars as basal root whorl number varies among genotypes (Lynch, 2007; Miguel, 2011). However, a certain tradeoff occurs between P and water uptake since plants with higher density of roots in top soil and shallower angles have lower water use efficiency, as water is usually more abundant in deeper layers under drought conditions (Ho et al., 2005). Another obstacle in improving root density is the associated carbon cost of producing root hairs, that have to be compensated by producing either smaller or thinner hairs and/or increased proportion of aerenchyma in the cortex and less secondary growth of the stele (Lynch and Ho, 2005; Zhu et al., 2010). Plants would otherwise spare carbon allocated in developing “productive” parts.
Modeling root traits are clearly advantageous strategy for enhancing PAE. However, screening and phenotyping for these traits remain a complex challenge as soil-based study systems are high technology based, and hydroponic/aeroponic systems cannot totally emulate the complexity of the processes occurring in the soil. Therefore, genotypes selected in this way do not always show their superiority in field trials (van de Wiel et al., 2016).
Root Exudates
If P is present on fixed sources and/or unavailable forms, plants having larger and/or more branched root architecture do not significantly improve P acquisition. In this case, root physiology and biochemical responses play a major role on accessing P from sparingly available pools in soil. Hence, the exudation of low molecular weight organic acids (LMWOAAs), proton extrusion, phosphatase exudation and/or association with symbiotic and non-symbiotic microorganisms present in the rhizosphere are the most important adaptations developed by plants (Figure 1).
As inorganic P forms availability and enzymatic activity are strongly affected by soil pH (Hinsinger, 2001), P solubility can be increased by root-induced acidification in alkaline soils or by pH increase of the rhizosphere in acidic and deeply weathered soils (Gahoonia et al., 1992; Jones and Oburger, 2011). This process occurs mainly because changes in pH in the rhizosphere can influence surface charges on soil particles and therefore Pi availability (Geelhoed et al., 1999). Plants have the ability to either increase or decrease rhizospheric pH up to 2–3 pH units, mainly by absorption or release of protons in order to equilibrate cation/ anion balance (Hinsinger et al., 2003). In the specific case of the cereals wheat and barley, Gahoonia and Nielsen (1996) observed that when rhizospheric pH was invariable, the plants displayed significant genotypic variation in terms of PAE, indicating that other mechanisms should also be involved in causing variation on P acquisition.
Carboxylates and the corresponding carboxylic acids, also known as LMWOAAs, constitute the major fraction of root exudates during P deficiency (Figure 1). Usually, the most common organic acid anions found in rhizosphere are lactate, acetate, oxalate, succinate, fumarate, malate, citrate, isocitrate, and aconitate (Jones, 1998). They have distinct functions on energetic cell metabolism, maintaining charge balance or osmotic potential. It has been widely suggested that LMWOAAs can improve P availability by mobilizing sparingly available P forms in the soil solution. This occurs by chelating metals ions like Al, Fe or Ca involved in P sorption and occupying sorption sites on minerals (Jones, 1998). P mobilizing activity through LMWOAAs is based on their variable negative charge, which would allow the complexation of metal cations and the displacement of anions from the soil matrix. The above is supported by several studies reporting an increase of organic acids exudation by roots in response to P deprivation, especially in plants from Proteacea family that possess cluster roots (Jones, 1998; Vance et al., 2003; Delgado et al., 2013). In addition, the presence of LMWOAAs in solution has been seen to increased P availability compared to water treatments (Gerke, 1992; Khademi et al., 2009, 2010). The efficiency in mobilizing P differs across LMWOAAs as follows: citrate > oxalate > malate > acetate. However, organic acid anion-induced P release depends on many factors, such as pH, soil mineralogy and anion concentration (>100 mM for citrate, >1 mM for oxalate, malate and tartrate) (Bolan et al., 1994; Jones and Darrah, 1994; Lan et al., 1995). Indeed, the rates to which Pi and organic anions are replaced in soil solution make predictions of the real effect difficult. Organic acid anions have a fast turnover as they can be quickly adsorbed in acidic soils and rapidly degraded in alkaline counterparts, with half-lives of several hours (Wang et al., 2010). Contrasting evidence found that, despite exuding citrate, pea genotypes were not capable of mobilizing P from Al-P and Fe-P complexes (Pearse et al., 2007). Nevertheless, organic acid production constitutes an important carbon cost in plant metabolism, with 5–25% of total fixed carbon by photosynthesis being used to sustain exudation. However, this does not seem to significantly affect net biomass production as P deficiency can reduce growth to an even greater extent (Johnson et al., 1996; Keerthisinghe et al., 1998).
Sparingly available organic P forms represent between 30% and 90% of total P in some soils (Borie et al., 1989; Jones and Oburger, 2011). Substantial flows of P occur between inorganic and organic P pools in soil through immobilization and mineralization, being both processes mediated predominantly by soil microorganisms (Oberson and Joner, 2005; Richardson and Simpson, 2011). In order to utilize this P source, organic compounds have to be mineralized; that is, organic P substrates must be hydrolyzed by enzymatic activity of phosphatases to release Pi. This activity seems to be more pronounced in the rhizosphere and it is associated with a depletion of soil organic P (Gahoonia and Nielsen, 1992; Chen et al., 2002; Spohn and Kuzyakov, 2013). Phosphatases are enzymes responsible for catalyzing the hydrolysis of phosphoric acid anhydrides and esters (Schmidt and Laskowski, 1961). These are classified by the Nomenclature Committee of the International Union of Biochemistry and Molecular Biology into 5 groups: phosphomonoesterases (EC 3.1.3), phosphodiesterases (EC 3.1.4), triphosphoric monoester hydrolases (EC 3.1.5), enzymes acting on phosphoryl-containing anhydrides (EC 3.6.1) and on P–N bonds (EC 3.9) (Nannipieri et al., 2011). Phosphomonoesterases are the most abundant enzymes in soils and include acid and alkaline forms and phytases, among others. To date, there is no evidence that any plants produce alkaline phosphomonoesterases.
There is an increasing interest on phytases due to the fact that they hydrolyze inositol phosphates (isomers and lower order derivatives of inositol hexakisphosphate) which generally constitute a major component of soil total organic P. Ranging from 4 to 40% of total P in soils (Borie et al., 1989; Smernik and Dougherty, 2007; Turner, 2007), inositol phosphates are readily adsorbed to soil particles and can react with cations (Fe and Al in acidic soils and Ca in alkaline ones) depending on pH to form poorly soluble precipitates (Shang et al., 1992; Celi and Barberis, 2005). However, in most plant species phytase activity has limited capability to mineralize inositol phosphate due to its low production and exudation from roots and the poor availability of the substrate in solution (Richardson et al., 2001; George et al., 2007). Attempts to creating transgenic plants overexpressing phytases and/or other phosphatases have been achieved (Lung et al., 2005; Wasaki et al., 2009) with little successes under natural soil conditions, where substrate availability is restricted (Lung and Lim, 2006; Wang et al., 2009). Interestingly, phosphatase activities are higher near the rhizosphere, with maximum activities found from 2 to 3.1 mm to the root surface for acid and 1.2 to 1.6 mm for alkaline phosphomonoesterases, showing a negative correlation with rhizospheric organic P content in wheat plants (Nannipieri et al., 2011). Phosphatase activity is also regulated by other factors, such as soil mineralogy, organic matter content, P availability and bacterial communities present in the rhizosphere (Joner and Jakobsen, 1995; Snajdr et al., 2008; Stursova and Baldrian, 2011).
Microorganisms
Non-symbiotic soil microorganisms play a key role on organic P ecosystem dynamics (Figure 1; Harvey et al., 2009; Khan et al., 2010). It has been proposed that all alkaline phosphomonoesterases found in soil have a microbial origin, mainly bacterial (Tabatabai, 1994; Yadav and Tarafdar, 2003). Additionally, the majority of Pi mineralized from phytase activity is mediated by free-living bacteria and fungi (Unno et al., 2005; Richardson and Simpson, 2011). Spohn et al. (2013) using the 33P isotopic approach found that the release of root exudates could be a plant strategy to increase P mineralization by enhancing microbial activity.
Free-living soil microorganisms are believed to be more efficient than plants in absorbing and incorporating P into their biomass. Therefore, microbial P represents an important soil sink (Xu et al., 2013) and a potential source of available P for most plants as microbial P is located in more labile intracellular compounds with a fast turnover (Oberson and Joner, 2005; Bünemann et al., 2013; Hinsinger et al., 2015). Despite having an important role in organic P dynamics, most research related to free-living soil microorganisms to enhance PAE has been focused on microorganisms capable of solubilizing sparingly available P (Wakelin et al., 2004; Leggett et al., 2007). Microorganisms can release protons, LMWOAAs, and other secondary organic metabolites that may contribute to P solubilization from minerals (Jones and Oburger, 2011). Indeed, between 1-50% of soil bacteria and about 0.5-0.1% of soil fungi can be classified as P-solubilizing microorganisms (Kucey et al., 1989; Gyaneshwar et al., 2002). Fungal isolates (particularly the Genus Penicillium) have been largely studied due to their great capacity for solubilizing Pi in both solid and liquid media (Gyaneshwar et al., 2002; Leggett et al., 2007; Morales et al., 2011). A group of bacteria, usually denominated as plant-growth-promoting rhizobacteria (PGPR), are widely found in the rhizosphere of cropping and wild species and have the potential of enhancing PAE mainly through influencing nutrient availability, such as P, or via the indirect production of phytohormones, or plant growth regulators (Richardson et al., 2009). Among the latter, classical phytohormones as auxin, cytokinin, ethylene, gibberellin, and abscisic acid are included. These regulators influence root architecture and other features related to plant development (Peleg and Blumwald, 2011; Vacheron et al., 2013). Although the benefits of using PAE enhancing microorganisms have been evidenced in laboratory and glasshouse conditions, inconsistent results have been observed in field trials (Goos et al., 1994; Karamanos et al., 2010), with the exception of arbuscular mycorrhizal symbiosis established with certain soil fungi.
Arbuscular Mycorrhizal Symbiosis
Mycorrhizal symbiosis is an association between plant and some fungal species that generally colonize root or rhizoids, and is beneficial to both partners, at least under some circumstances (Jansa et al., 2011). Arbuscular mycorrhizal (AM) is the most common and widespread type of mycorrhizal symbiosis (Trappe, 1987; Wang and Qiu, 2006; Smith and Read, 2008), found in ca. 80% of plant species among all major plants lineages (Wang and Qiu, 2006; Brundrett, 2009) and in most of agricultural species (exceptions include Brassica spp., and Lupinus spp.). Although AM symbiosis is facultative for many plant species, fossil evidence indicates that the symbiosis matches with the first appearance of land plants, more than 400 million years ago, playing a crucial role in the development of terrestrial plants (Bonfante and Genre, 2008; Brundrett, 2009). AM fungi (subphylum Glomeromycotina) are obligate biotrophs that when associated with plant roots can provide an enhanced foraging system in order to improve acquisition of soil water and nutrients, particularly P, and to improve resistance to biotic and abiotic stresses in exchange of energy (using carbohydrates as trade) for fungal growth and reproduction (Jansa et al., 2003a; Smith and Read, 2008; Jung et al., 2012; Pozo et al., 2015; Armada et al., 2016; Santander et al., 2017). P appears to be one of major regulators of AM symbiosis establishment and efficiency, as root colonization, P uptake through fungal pathway (Figure 1) (See section Changes in P Transporters) and growth responses diminish with increasing soil P availability (Smith and Read, 2008; Richardson et al., 2011; Smith et al., 2011). However, plants can also modulate the symbiosis, by stimulating fungal metabolic activity and hyphal branching among other effects (Bücking and Shachar-Hill, 2005; Besserer et al., 2006), through the exudation of strigolactones (Akiyama et al., 2005; Parniske, 2006; López-Ráez et al., 2017) Accordingly, the production of these strigolactones is promoted by P deprivation, although in wheat can be also promoted in a small fraction by N deficiency (Yoneyama et al., 2007, 2012; López-Ráez et al., 2008).
Despite its broad host range and that its cosmopolitan distribution, AM diversity involves only ~250 morphologically and 350 to 1000 molecularly defined AM fungi (Kivlin et al., 2011; Öpik et al., 2014), with low endemism patterns at global scale (Davison et al., 2015). The absence of AM fungal colonization is rare in natural conditions in plants able to perform the symbiosis, only being achieved in soils lacking AM fungal propagules or in non-mycorrhizal (NM) plant species (Smith et al., 2011). Commonly, the difference in plant growth in presence and absence of mycorrhizal fungal partners is defined as mycorrhizal growth responses (MGR) and vary widely from positive to negative depending on plant/fungi species and growth conditions (Johnson et al., 1997; Klironomos, 2003). When compared to MGR observed from other cereal crops [positives responses in maize (Sylvia et al., 1993; Karasawa et al., 2001) and rye (Baon et al., 1994a); and excluding rice, which is often not colonized or poorly colonized under continuous submersion (Vallino et al., 2009)], wheat and barley plants present a high variable response to AM colonization, being generally considered as low and sometimes showing even negative effects in plant growth (Hetrick et al., 1996; Grace et al., 2009). However, positive responses can also be found when applying different experimental conditions or analyzing at different growth stages, which indicates that AM fungal inoculation under appropiated circumstances can be an effective agronomic practice also in these crops (Borie and Rubio, 1999; Seguel et al., 2016a,b, 2017).
Interestingly, a recent meta-analysis by Pellegrino et al. (2015) looking at wheat responses to AM symbiosis inoculation under field conditions found out that although straw biomass was weakly correlated with root AM fungal colonization rate, grain yield and P accumulation correlated positively. A review of the main mycorrhizal growth responses and P uptake from mycorrhizal and NM treatments in wheat (Table 1A) and barley (Table 1B) are presented below highlighting the idea that growth responses associated to AM symbiosis are not directly related to P acquisition. Growth depletions upon AM fungal colonization are normally attributed to an excess of photosynthates shared with the fungal partner, which are estimated to be up to 20% of the C fixed by the host plant (Jakobsen, 1995; Ortas et al., 2002; Li et al., 2005; Morgan et al., 2005). However, some studies indicated that growth depletion resulting from C drain to the fungal symbiont do not apply in all cases. Hetrick et al. (1992) and Grace et al. (2009) reported that growth reductions in wheat and barley did not vary when associated with two different AM fungal partners with contrasting capacity to colonize their roots (e.g., 61 and 5%, respectively), and therefore, hypothetically different C demand from the host plant (Hetrick et al., 1992). Even so, cereals benefits from AM symbiosis despite growth and/or nutritional benefits (such as net P uptake) are not apparent. Special techniques such as isotopic labeling are necessary to demonstrate symbiosis functioning (nutrient, water and carbohydrate exchange) in these cases (Smith et al., 2004, 2009; Grace et al., 2009).
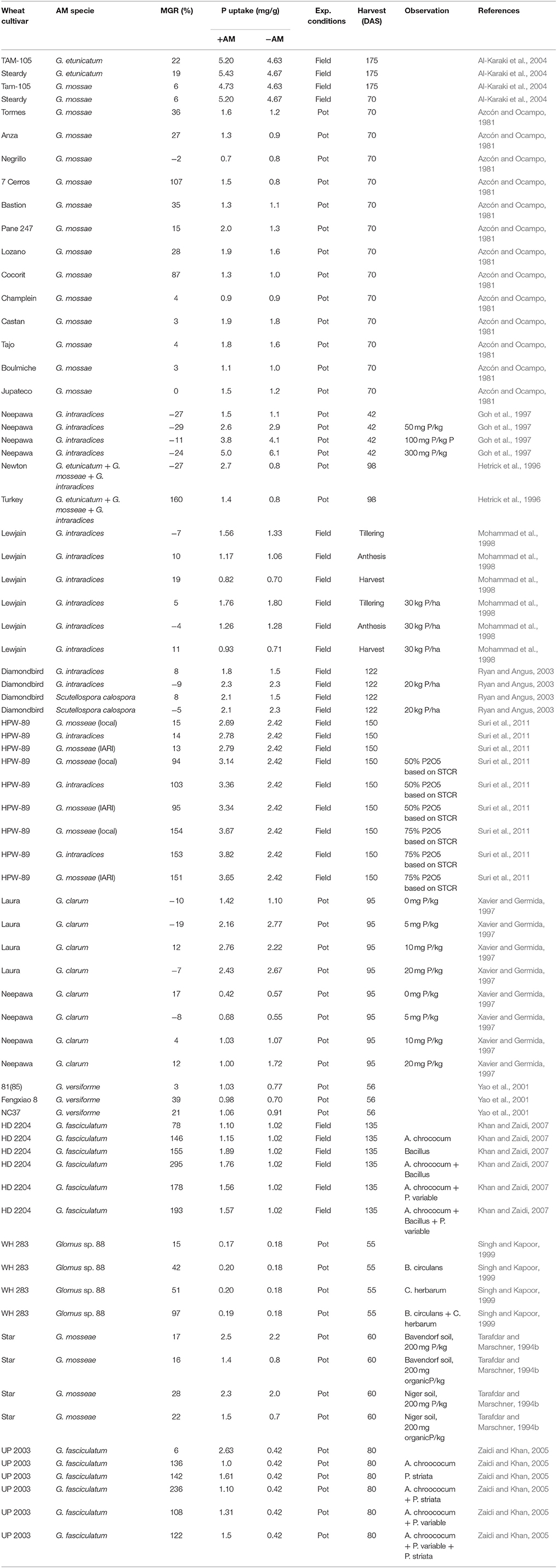
Table 1A. Mycorrhizal growth responses (MGR) and P uptake on mycorrhizal (+AM) and non-colonized (–AM) wheat (T. aestivum L.) cultivars under greenhouse or field conditions and at different days after sowing (DAS).
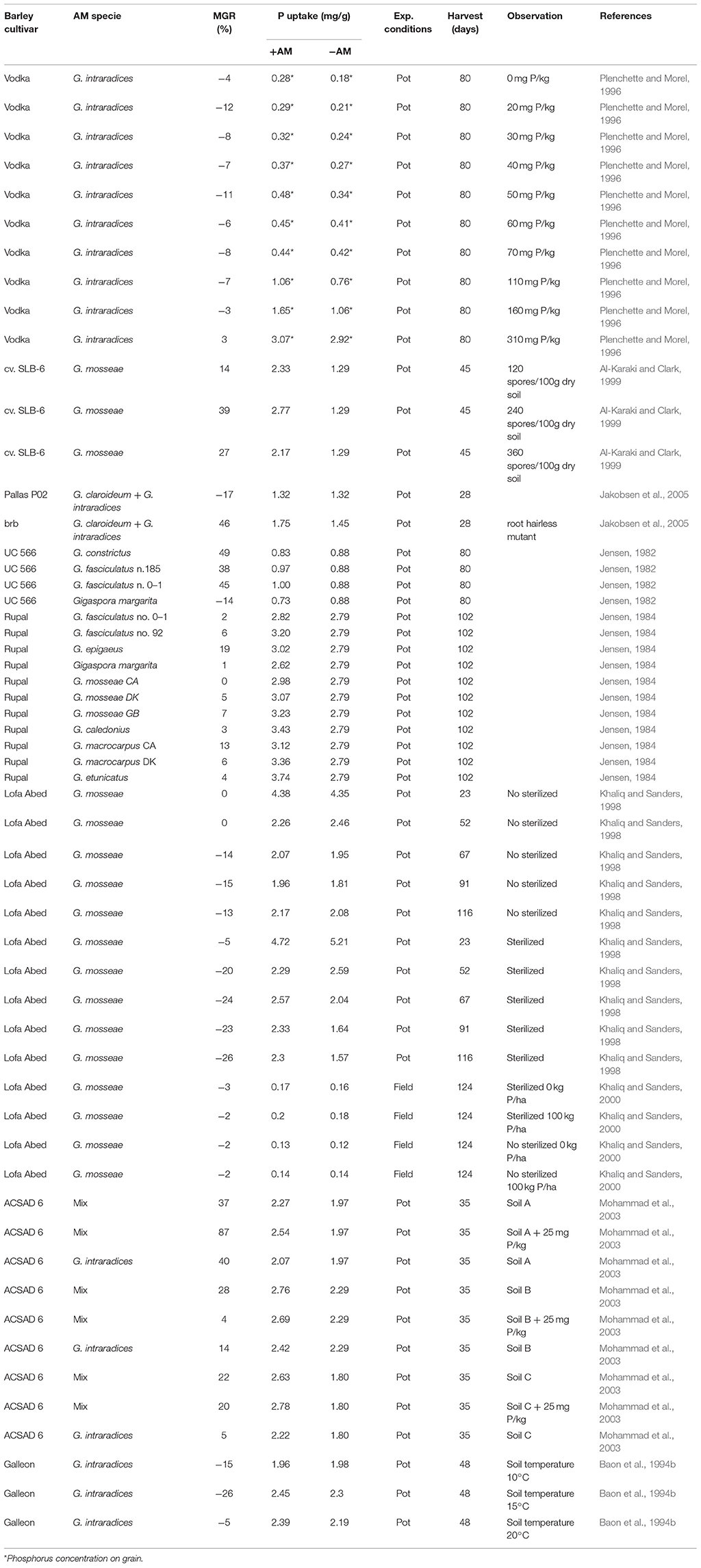
Table 1B. Mycorrhizal growth responses (MGR) and P uptake on mycorrhizal (+AM) and non-colonized (–AM) barley (H. vulgare L.) cultivars under greenhouse or field conditions and at different days after sowing (DAS).
Mycorrhizal Influence on PAE Traits of Wheat and Barley
Root Architecture and Surface Area
The root systems of grain cereals as wheat and barley consist of two types of roots. The first type is known as primary or seminal roots, and comprises between three to seven roots growing from the seedling. They have 0.2–0.4 mm diameter, occupying 5–10% of total root volume in mature plants. The second type is the secondary roots, also called nodal, crown, or adventitious roots. These roots emerge from nodes at the base of main stem and tillers 1–3 months after germination, having a larger diameter (0.3–0.7 mm) than primary roots (Hoad et al., 2001). Significant genetic variation for root architectural traits has been found among cereal cultivars (Kujira et al., 1994; Marschener, 1998). Interestingly, it was found out that the number of tillers positively correlated with root length density and grain yield of semidwarf bread wheat cultivars grown under P deficiency (Manske et al., 2000). In addition, Gahoonia et al. (1997) showed that the presence of root hairs increased the total root surface of winter wheat by 95–341% and by up to 112–245% for barley.
Perhaps the main mycorrhizal-associated mechanism enhancing plant PAE is the increase of explored soil volume by the AM fungal hyphae, which can extend plant access from millimeters to centimeters from root surface. Fungal hyphae can also access soil pores that root hairs cannot due to their smaller diameter (20–50 um) (Figure 1B). Moreover, AM roots can improve water and nutrients uptake efficiency compared to non-colonized roots due to a lower C cost per unit of hyphal surface related to the root surface (Jansa et al., 2003a; Jakobsen et al., 2005; Gregory, 2006; Schnepf et al., 2008).
There is a complex interplay between root architecture and AM fungi and, as expected, root traits can influence how plants respond to mycorrhizal colonization (Newsham et al., 1995; Smith and Read, 2008). It is suggested that species with root systems characterized by low root hair length and density, and roots with relatively large diameters would display the greatest growth benefits from the symbiosis (Brundrett, 2002; Fitter, 2004; Smith and Read, 2008), especially under P-limiting conditions. Several studies have corroborated this assumption by making this comparison between wild and agricultural species, reporting associations between root traits and MGR (Baon et al., 1994a; Declerck et al., 1995; Schweiger et al., 1995; Jakobsen et al., 2005). However, a recent meta-analysis carried out by Maherali (2014) does not support this hypothesis.
Usually, root system architecture is also frequently modified before and following the establishment AM symbiosis (Scannerini et al., 2001; Hodge et al., 2009), especially through some fungal exudates, known as Myc-factors (Figure 1; Maillet et al., 2011; Mukherjee and Ané, 2011). These signal molecules are exuded even in the absence of a host plant and are involved not only in symbiotic signaling stimulating colonization, but also acting as plant growth regulators by modifying root development in some plant species (Maillet et al., 2011; Mukherjee and Ané, 2011). The formation of lateral roots has been found to be the most affected trait, making roots progressively more branched, probably to increase the number of suitable sites for colonization (Harrison, 2005). However, mycorrhizal-induced modifications on root traits are still poorly understood and seem to vary according to specific plant-fungal combinations, (Schellenbaum et al., 1991; Berta et al., 2005; Fusconi, 2014). In the case of wheat and barley, evidences are controversial as well. Behl et al. (2003) found a significant increase of total root length in wheat colonized by G. fasciculatum, being up to 90% higher than control plants when co-inoculated with Azotobacter. The same pattern was found by Al-Karaki and Al-Raddad (1997), who studied the response of two durum wheat genotypes to AM colonization, detecting an increase of 25 and 20% in root length. On the other hand, AM fungal inoculation decreased wheat root length and surface area under high rates of P application in a calcareous soil (Mohammad and Malkawi, 2004).
Organic Acid Anion and Phosphatase Exudation
It has been suggested that AM fungi may have biochemical and physiological capacities to increase plant PAE through the uptake of P from sparingly available forms in soil, being the exudation of protons, phosphatases and LMWOAAs the suggested mechanisms involved in these processes (Figure 1; Tarafdar and Marschner, 1994a; Koide and Kabir, 2000; Klugh and Cumming, 2007).
AM fungi possess many genes encoding acid phosphatases (EC 3.1.3.2, ACP) in their genomes, with at least seven genes expressed in Rhizophagus clarus (Sato et al., 2015). However, exudation of phosphatases was mostly associated with the cell wall (Olsson et al., 2002) and their presence in the rhizosphere has been demonstrated only in limited cases (Tarafdar and Marschner, 1994a; Koide and Kabir, 2000). The magnitude of these processes is questioned as it is difficult to isolate the effects of plants, fungi and others microorganisms present in the experiments under unsterile conditions (Joner and Jakobsen, 1995; Joner et al., 2000). However, Sato et al. (2015) in an experiment with separated compartments for hyphal growth, collected exudates from soil solution, sand culture and in vitro monoxenic culture, providing strong evidence that the corresponding acid phosphatase activity was originated from R. clarus. Little information is available about the relationship between AM symbiosis and changes in enzymatic exudation and activity patterns in wheat and barley. Rubio et al. (1990) found out a positive correlation between wheat colonization by AM fungi and acid phosphatase activity in roots and soil, mainly under P-limiting conditions. Using a different experimental approach with separated compartments for hyphal growth, Tarafdar and Marschner (1994a,b) observed depletion in organic P content with a concomitant increase of phosphatase activity when wheat was colonized by Glomus mosseae (Nicol & Gerd) Gerd & Trappe. The same trend was found for barley in a 10 years' field trial where P-deprived plants presented higher colonization by AM fungi and higher phosphatase activity than fertilized treatments (Goicoechea et al., 2004). In this sense, Ye et al. (2018) in a recent report show the importance of phosphatase activity in P acquisition by non-AM colonized barley efficient genotypes through direct changes of rhizosphere P fractions. Nevertheless, the interaction of AM association with the phosphatase activity and the subsequent P acquisition by efficient genotypes is still unclear.
The phosphate-solubilizing activities of AM fungi are still controversial although AM plants have generally been shown to increase the uptake of insoluble Pi (Yao et al., 2001; Tawaraya et al., 2006; Klugh-Stewart and Cumming, 2009). In many studies, mycorrhizal inoculants proved to alter the composition and/or amount of total LMWOAAs exuded by Liriodendron tulipifera and Andropogon virginicus, respectively (Figure 1; Klugh and Cumming, 2007; Klugh-Stewart and Cumming, 2009). However, direct evidence for solubilization of P by AM fungi has not been obtained so far. Despite that AM fungi might not exude LMWOAAs by themselves, they can, however, improve P solubilization and/or mineralization indirectly by stimulating the surrounding soil microbes via the exudation of labile C, thus increasing local nutrient availability in the hyphosphere and in soil patches beyond the root hairs (Hodge et al., 2010; Cheng et al., 2012; Jansa et al., 2013). Recently, Kaiser et al. (2015) using nanoscale secondary ion mass spectrometry imaging and 13C-phospho and neutral lipid fatty acids, traced the flow of recently photoassimilated C and found out that a significant and exclusive proportion of photosynthates was delivered through AM pathway and used by different microbial groups compared to C directly released by the roots.
The interaction between phosphate-solubilizing microorganisms with AM wheat and barley plants has been assessed by some researchers, with positive responses on growth and P uptake. Omar (1998) observed that the interaction between Funneliformis constrictum and the rock-phosphate-solubilizing Aspergillus niger and Penicillium citrinum fungi significantly increased biomass production of wheat plants under all experimental conditions tested. The effect was more evident in non-sterilized conditions. Bacteria from the Azotobacter and Pseudomonas genera also improved AM wheat growth under field and pot conditions, with positive correlation between AM colonization and Azotobacter survival in the rhizosphere (Kucey, 1987; Behl et al., 2003; Zaidi and Khan, 2005; Yousefi et al., 2011). Singh and Kapoor (1999) analyzed the effect of Bacillus circulans, Cladosporium herbarum and an isolated AM fungus in wheat where larger populations of phosphate-solubilizing microorganisms in the rhizosphere of mycorrhizal roots and an enhanced P acquisition in combined inoculation were found. Similarly, the inoculation with Penicillium variable alone negatively affected the biomass production of wheat. However, when applied in combination with Azotobacter chroococcum, Pseudomonas striata and the AM fungus G. fasciculatum, grain yield significantly increased compared with the other treatments (Zaidi and Khan, 2005).
In another study, wheat grain yield was enhanced by 92.8% in the presence of the rhizobacteria Pseudomonas fluorescens and Burkholderia cepacia and the AM fungus Claroideoglomus etunicatum (Saxena et al., 2013). The synergistic effect of combined inoculation with plant growth-promoting rhizobacteria and AM fungi on wheat was also proved to be effective under field conditions. It was shown that the combination of A. chroococcum and Bacillus sp. with G. fasciculatum significantly increased the dry matter by 2.6-fold and grain yield by 2-fold when compared to the control (Khan and Zaidi, 2007). In another field study, Mehrvarz et al. (2008) found that although bacterial inoculation alone achievied the maximum biological yield, its application combined with AM fungi produced grains with higher weight.
Changes in P Transporters
In general, AM plants have two different pathways for P uptake from the soil (Figure 1C) with different P transporters involved in both of them. The direct P uptake is the plant endogenous pathway, which occurs via root epidermis and root hairs, while in the AM pathway the external hyphae is the responsible for acquiring P from the medium and transport to intracellular symbiotic interfaces where it finally goes to the plant (Grace et al., 2009; Smith et al., 2011). According to their function, plant transporters involved in the direct pathway are expressed mostly in the root apex and root hairs (Gordon-Weeks et al., 2003) and down-regulated in more mature regions. However, up-regulation of genes encoding phosphate transporters proved to have little influence on P acquisition. Rae et al. (2004) studying transgenic barley plants over-expressing a gene encoding for a phosphate transporter found no improvement on P uptake under any of the tested conditions, suggesting that post-transcriptional mechanisms could be involved affecting the activity of these transporters. AM transporters are less known due to their obligate biotrophic nature, coupled with the fact that they are multinuclear and heterocaryotic organisms (Sanders, 1999), which make the use of traditional genetic approaches difficult (Maldonado-Mendoza et al., 2001). These authors observed that the expression of a phosphate transporter gene from the extra-radical mycelium of Rhizofagus intraradices was regulated in response to P concentrations in the environment surrounding the extra-radical hyphae and that it was modulated by the overall phosphate status of the AM fungus rather than the host plant (Maldonado-Mendoza et al., 2001). Another important aspect of the AM pathway is the presence of AM-inducible plant P transporters, which are generally present at much higher levels in AM roots than other P transporters (Javot et al., 2007). These transporters are responsible for the exchange of P between the fungal hyphae and plant cell. They have been found in all AM plants investigated, regardless their growth response to colonization, and are mainly expressed in the colonized cortical cells, specifically in the arbusculated cells which is the place where the nutrient exchange takes place (Bucher, 2007; Javot et al., 2007). Genes encoding for AM-inducible transporters have been described in cereals and include the HvPHT1.11 and HvPHT1.8 for barley and TaPHT1.8, TaPHT1.11, TaPHT1.12, and TaPHT1.14 for wheat (Teng et al., 2017).
The two P pathways were believed to be additive in their contribution to plant nutrient uptake, and it was assumed that direct pathway made a constant contribution to the total P uptake, while the AM pathway participated as an extra contribution in plants with positive growth responses (Pearson and Jakobsen, 1993). However, further investigations proved that AM colonization could reduce the direct uptake pathway in some species (even in plants that respond positively to the symbiosis as in Medicago truncatula), and deactivate completely in others (Liu et al., 1998; Smith et al., 2004). Therefore, in order to not become P deficient AM pathway should compensate the reduced contribution of direct pathway (Smith et al., 2011). Recent studies using radioactive P isotopes has shown that AM pathway contributed significantly to total P uptake on wheat and barley. In this sense, Smith et al. (2015) clearly demonstrated that indigenous AM fungi contribute to wheat P uptake in 6.5–21% of total plant P in field conditions and 3–40% when grown in pots. However, mycorrhizal wheat plants acquired less P and produced less biomass when compared to their non-mycorrhizal counterpart (Li et al., 2006; Grace et al., 2009). It was suggested that negative growth responses could be generated by suppression of the direct pathway in these species, especially in the plants with very low colonization. Conversely, Grace et al. (2009) found out that the magnitude of the negative responses of barley was independent of contrasting colonization by two AM fungal species (R. intraradices and F. geosporum). In addition, the expression of P transporters belonging to direct pathway in barley was not affected by the symbiosis as expected. Again, this indicated that possible post-translational modifications of regulatory components could be involved in the plant response.
AM Functional Diversity
It is a general consensus that there is little specificity between AM fungal and host plant species, and that AM plants can be colonized by several AM fungal species at the same time (Merryweather and Fitter, 1998; Jansa et al., 2003b; Smith et al., 2011). However, the existence of different colonization patterns could imply certain preferences for specific AM fungal species, functional groups or the co-evolution strategies between specific plant-fungus associations (Smith et al., 2009; Chagnon et al., 2013; López-García et al., 2017). For instance, Mao et al. (2014) showed that these preferences can exist even across wheat cultivars as they found a variation in AM fungal community composition, displaying a complex pattern of cultivar-AM fungal interaction under experimental field conditions. Despite of the projection of this work, the study of the AM fungal diversity associated to wheat and barley is overall scarce. Considering the wide distribution and economic importance of these two species, only 131 and five AM fungal sequences in MaarjAM database, the most complete sequence database of Glomeromycota (Öpik et al., 2010), are associated to wheat (Triticum sp.) and barley (Hordeum sp.) respectively, out of 5,296 sequences belonging to Poaceae in the database. The few studies covering molecular diversity in roots of wheat have shown differences between in community composition associated to wheat and N-fixing crops (Bainard et al., 2014; Higo et al., 2016). Communities associated to wheat have also been found to vary during the growing season and depend on P fluxes and degree of fertilization (Wu et al., 2011; Bainard et al., 2014; Qin et al., 2015). The diversity of AM fungal communities associated directly with roots of wheat is overall high, including members of different taxonomic families (e.g., Manoharan et al., 2017), but being predominatly associated with Funneliformis spp., in conventional cropping, and Claroideoglomus spp., in organically managed systems (Dai et al., 2014). In agreement, with this result, one of the few studies analyzing AM fungi in roots if barley, found that the abundance of Funneliformis spp. were associated with high levels of P in soil, meanwhile Claroideoglomus spp. with lower levels of P (Cruz-Paredes et al., 2017), but harboring a high phylogenetic diversity as well (Manoharan et al., 2017). N fertilization has been seen another affecting AM fungal community composition in barley and interacting with the plant-fungus P trade, as tends to decrease the efficiency in the interexchange (Williams et al., 2017).
The lack of information on molecular diversity has been in some manner compensated with morphological studies of spore communities. In this context, a high taxonomic diversity has been found. Aguilera et al. (2014, 2017) analyzing spore morphology on acidic soils under continuous wheat cropping, found 24 AM fungal species, being Acaulospora and Scutellospora the dominant genera. In another study under similar conditions in acidic soils, Castillo et al. (2016b) described 26 fungal species with a prevalence of Acaulospora and Claroideoglomus. This dominance of Acaulospora spores in soils cropped with wheat was also observed by Hu et al. (2015) in North China and by Nadji et al. (2017) in Algeria, however in the last study Glomeraceae species was also detected as highly abundant.
The mycorrhizal growth response of a single host plant species can differ across AM fungal species, and in the same way, colonization by the same AM fungal isolated can result in different growth responses in different plant species or genotypes (Feddermann et al., 2010; Smith et al., 2011; Castillo et al., 2016a). Indeed, previous studies have demonstrated a high variability in the symbiotic response of different combinations of host plant and AM fungi (e.g., Smith et al., 2004; Avio et al., 2006; Jansa et al., 2008). Variations in MGR have also been revealed across wheat cultivars, which can range from −2% to 107% in different genotypes (Azcón and Ocampo, 1981). On the other hand, Graham and Abbott (2000) showed a huge variation in MGR when testing several AM fungal isolates in symbiosis with wheat, being Scutellospora calospora the only one promoting higher plant biomass. In a study in wheat showed that MGR by different AM fungal species and their combination or with F. mosseae alone resulted in negative growth responses, while positive responses were reported when inoculated with R. clarum (Talukdar and Germida, 1994). This variability in mycorrhizal response comes from the fact that AM fungi are functionally diverse both inter- and intraspecifically (see for example Koch et al., 2004, 2017; Antunes et al., 2011). Differences among AM fungal species have been suggested to exist in the colonization rates in roots and soils depending on the AM fungal colonization pattern (Hart and Reader, 2002; Powell et al., 2009). Perhaps, although morphological traits seem to be well-conserved across AM fungal phylogeny, i.e. morphological traits into the same species and related clades are similar, most of variation in plant growth promotion and P uptake occurs indeed intraspecifically (Munkvold et al., 2004; Koch et al., 2017). In general, it had been assumed that morphological traits, such as the hyphal lenght in soil, could be good predictors of P uptake. However, the above mentioned results on huge variabilities in plant P uptake on morphological and phylogenetically similar fungal isolates redirects the question toward which fungal functional trait have to be measured to understand soil-plant P dynamics in agricultural systems. Therefore, functional diversity among AM fungal species and genotypes need to be considered.
Future Perspectives
Despite displaying negative responses in some studies and being considered as non-responsive by many authors, wheat and barley plants presented positive growth and P responses by performing AM symbiosis (Tables 1A,B respectively). There could be factors involved in this large PAE variation and the processes affecting both AM function and its benefits are still unknown. The question is complex due to the many factors are involved: plant genotype and fungal functional diversity, as well as their mutual compatibility, soil variable conditions or agricultural management needs to be studied. Indeed, the fact is that a major part of the research carried out in the interaction between crop cereals and AM fungi has only involved a handful of AM fungal isolates. In addition, there is little information available regarding the effect of different -or combined- AM fungal taxa colonization and different genotypes of wheat and barley on root morphology, development, exudation pattern, interaction with PGPR and/or P-solubilizing fungi, and the interplay between the two pathways of P uptake.
It is widely accepted that AM plants access to poorly available sources more effectively than non-colonized plants, but the mechanisms by which they are operating at field are not well understood (Smith et al., 2015). Studies using more than one crop cultivar and multiple AM species and genotypes should be carried out in order to analyze the effect of fungal diversity on PAE related traits as root length, root hair angles, changes on root-mycorrhiza exudation patterns and degree of inhibition (or not) of plant P transporters. In addition, these studies should be traced along different stages of development, until grain production, as it was found that although mycorrhization could hamper biomass production, it enhanced P acquisition and final grain production (Pellegrino et al., 2015). Isotopic, spectroscopic and molecular techniques coupled to new experimental designs could help identify some of the mechanisms mentioned above and the genetic background behind the different responses. In this sense, we suggest an inclusion of the Carbon costs related to all P acquisition traits (not only root architeture), specially those involved and altered by mycorrhizal colonization, in order to support accurate phenotyping for breeding programs focused on lowering P fertilizer inputs (Figure 2).
Author Contributions
PCa wrote the manuscript. FB was the main advisor and revisor of the document. PCo contributed on wheat and barley agronomical aspects and microorganisms effects on PAE. AS contributed to the construction of the document and the figures, and on all features related to phosphorus. JL-R provided insights on mycorrhizal signaling and the physiological effects upon colonization. AL-G also contributed on mycorrhizal aspects, highlighting the importance of functional diversity.
Conflict of Interest Statement
The authors declare that the research was conducted in the absence of any commercial or financial relationships that could be construed as a potential conflict of interest.
Acknowledgments
We fully acknowledge the financial support of the FONDECYT 11160385 (AS) and FONDECYT 1170264 (PCo) grants from Comisión Nacional de Investigación Científica y Tecnológica (CONICYT-Chile) and the support granted by CONICYT scholarship 21161474 (PCa). JL-R is supported by the grant AGL2015-64990-C2-1R (MINECO-Spain). AL-G is supported by the grant 708530–DISPMIC (European Union's Horizon 2020 Marie Curie Individual Fellowship).
References
Abel, S., Ticconi, C. A., and Delatorre, C. A. (2002). Phosphate sensing in higher plants. Physiol. Plant. 115, 1–8. doi: 10.1034/j.1399-3054.2002.1150101.x
Aguilera, P., Cornejo, P., Borie, F., Barea, J. M., von Baer, E., and Oehl, F. (2014). Diversity of arbuscular mycorrhizal fungi associated with Triticum aestivum L. plants growing in an Andosol with high aluminum level. Agric. Ecosyst. Environ. 186, 178–184. doi: 10.1016/j.agee.2014.01.029
Aguilera, P., Marín, C., Oehl, F., Godoy, R., Borie, F., and Cornejo, P. (2017). Selection of aluminum tolerant cereal genotypes strongly influences the arbuscular mycorrhizal fungal communities in an acidic Andosol. Agric. Ecosyst. Environ. 246, 86–93. doi: 10.1016/j.agee.2017.05.031
Akiyama, K., Matsuzaki, K., and Hayashi, H. (2005). Plant sesquiterpenes induce hyphal branching in arbuscular mycorrhizal fungi. Nature 435, 824–827. doi: 10.1038/nature03608
Al-Karaki, G., and Al-Raddad, A. (1997). Effects of arbuscular mycorrhizal fungi and drought stress on growth and nutrient uptake of two wheat genotypes differing in drought resistance. Mycorrhiza 7, 83–88. doi: 10.1007/s005720050166
Al-Karaki, G., McMichael, B., and Zak, J. (2004). Field response of wheat to arbuscular mycorrhizal fungi and drought stress. Mycorrhiza 14, 263–269. doi: 10.1007/s00572-003-0265-2
Al-Karaki, G. N., and Clark, R. B. (1999). Varied rates of mycorrhizal inoculum on growth and nutrient acquisition by barley grown with drought stress. J. Plant Nutr. 22, 1775–1784. doi: 10.1080/01904169909365753
Antunes, P. M., Koch, A. M., Morton, J. B., Rillig, M. C., and Klironomos, J. N. (2011). Evidence for functional divergence in arbuscular mycorrhizal fungi from contrasting climatic origins. New Phytol. 189, 507–514. doi: 10.1111/j.1469-8137.2010.03480.x
Armada, E., López-Castillo, O., Roldán, A., and Azcón, R. (2016). Potential of mycorrhizal inocula to improve growth, nutrition and enzymatic activities in Retama sphaerocarpa compared with chemical fertilization under drought conditions. J. Soil Sci. Plant Nutr. 16, 380–399. doi: 10.4067/S0718-95162016005000035
Avio, L., Pellegrino, E., Bonari, E., and Giovannetti, M. (2006). Functional diversity of arbuscular mycorrhizal fungal isolates in relation to extraradical mycelial networks. New Phytol. 172, 347–357. doi: 10.1111/j.1469-8137.2006.01839.x
Awika, J. M. (2011). “Major cereal grains production and use around the world,” in Advances in Cereal Science: Implications to Food Processing and Health Promotion, J. M. Awika, V. Piironen, and S. Bean (Washington, DC: ACS Symposium Series), 2–13
Azcón, R., and Ocampo, J. A. (1981). Factors affecting the vesicular-arbuscular infection and mycorrhizal dependency of thirteen wheat cultivars. New Phytol. 87, 677–685. doi: 10.1111/j.1469-8137.1981.tb01702.x
Bainard, L. D., Bainard, J. D., Hamel, C., and Gan, Y. (2014). Spatial and temporal structuring of arbuscular mycorrhizal communities is differentially influenced by abiotic factors and host crop in a semi-arid prairie agroecosystem. FEMS Microbiol. Ecol. 88, 333–344. doi: 10.1111/1574-6941.12300
Bais, H. P., Weir, T. L., Perry, L. G., Gilroy, S., and Vivanco, J. M. (2006). The role of root exudates in rhizosphere interactions with plants and other organisms. Annu. Rev. Plant Biol. 57, 233–266. doi: 10.1146/annurev.arplant.57.032905.105159
Baon, J. B., Smith, S. E., and Alston, A. M. (1994a). Growth response and phosphorus uptake of rye with long and short root hairs: interactions with mycorrhizal infection. Plant Soil 167, 247–254. doi: 10.1007/BF00007951
Baon, J. B., Smith, S. E., and Alston, A. M. (1994b). Phosphorus uptake and growth of barley as affected by soil temperature and mycorrhizal infection. J. Plant Nutr. 17, 479–492. doi: 10.1080/01904169409364742
Bauer, A., Frank, A. B., and Black, A. L. (1987). Aerial parts of hard red spring Wheat. II. Nitrogen and phosphorus concentration and content by plant development stage 1. Agron. J. 79, 852–858. doi: 10.2134/agronj1987.00021962007900050020x
Behl, R. K., Sharman, H., Kumar, V., and Narula, N. (2003). Interactions amongst Mycorrhiza, Azotobacter chroococcum and root characteristics of wheat varieties. J. Agron. Crop Sci. 189, 151–155. doi: 10.1046/j.1439-037X.2003.00026.x
Berta, G., Sampò, S., Gamalero, E., Massa, N., and Lemanceau, P. (2005). Suppression of Rhizoctonia root-rot of tomato by Glomus mosseae BEG12 and Pseudomonas fluorescens A6RI is associated with their effect on the pathogen growth and on the root morphogenesis. Eur. J. Plant Pathol. 111, 279–288. doi: 10.1007/s10658-004-4585-7
Besserer, A., Puech-Pagès, V., Kiefer, P., Gomez-Roldan, V., Jauneau, A., Roy, S., et al. (2006). Strigolactones stimulate arbuscular mycorrhizal fungi by activating mitochondria. PLoS Biol. 4:e226. doi: 10.1371/journal.pbio.0040226
Bhardwaj, D., Ansari, M. W., Sahoo, R. K., and Tuteja, N. (2014). Biofertilizers function as key player in sustainable agriculture by improving soil fertility, plant tolerance and crop productivity. Microb Cell Facts. 13, 66–77. doi: 10.1186/1475-2859-13-66
Bolan, N. S., Naidu, R., Mahimairaja, S., and Baskaran, S. (1994). Influence of low-molecular-weight organic-acids on the solubilization of phosphates. Biol. Fert. Soils 18, 311–319. doi: 10.1007/BF00570634
Bonfante, P., and Genre, A. (2008). Plants and arbuscular mycorrhizal fungi: an evolutionary-developmental perspective. Trends Plant Sci. 13, 492–498. doi: 10.1016/j.tplants.2008.07.001
Borie, F., and Rubio, R. (1999). Effects of arbuscular mycorrhizae and liming on growth and mineral acquisition of aluminum tolerant and aluminum sensitive barley cultivars. J. Plant Nutr. 22, 121–137. doi: 10.1080/01904169909365612
Borie, F., Zunino, H., and Martinez, L. (1989). Macromolecule-p associations and inositol phosphates in some chilean volcanic soils of temperate regions. Commun. Soil Sci. Plant Anal. 20, 1881–1894. doi: 10.1080/00103628909368190
Bovill, W. D., Huang, C. Y., and McDonald, G. K. (2013). Genetic approaches to enhancing phosphorus-use efficiency (PUE) in crops: challenges and directions. Crop Pasture Sci. 64, 179–198. doi: 10.1071/CP13135
Brundrett, M. C. (2002). Coevolution of roots and mycorrhizas of land plants. New Phytol. 154, 275–304. doi: 10.1046/j.1469-8137.2002.00397.x
Brundrett, M. C. (2009). Mycorrhizal associations and other means of nutrition of vascular plants: understanding the global diversity of host plants by resolving conflicting information and developing reliable means of diagnosis. Plant Soil 320, 37–77. doi: 10.1007/s11104-008-9877-9
Bucher, M. (2007). Functional biology of plant phosphate uptake at root and mycorrhiza interfaces. New Phytol. 173, 11–26. doi: 10.1111/j.1469-8137.2006.01935.x
Bucher, M., Rausch, C., and Daram, P. (2001). Molecular and biochemical mechanisms of phosphate uptake into plants. J. Plant Nutr. Soil Sci. 164:209–221. doi: 10.1002/1522-2624(200104)164:<209::AID-JPLN209>3.0.CO;2-F
Bücking, H., and Shachar-Hill, Y. (2005). Phosphate uptake, transport and transfer by the arbuscular mycorrhizal fungus Glomus intraradices is stimulated by increased carbohydrate availability. New Phytol. 165, 899–911. doi: 10.1111/j.1469-8137.2004.01274.x
Bünemann, E. K., Keller, B., Hoop, D., Jud, K., Boivin, P., and Frossard, E. (2013). Increased availability of phosphorus after drying and rewetting of a grassland soil: processes and plant use. Plant Soil 370, 511–526. doi: 10.1007/s11104-013-1651-y
Burkitt, L. L., Small, D. R., McDonald, J. W., Wales, W. J., and Jenkin, M. L. (2007). Comparing irrigated, biodynamic and conventionally managed dairy farms. 1. Soil and pasture properties. Aust. J. Experim. Agric. 47, 479–488. doi: 10.1071/EA05196
Castillo, C. G., Borie, F., Oehl, F., and Sieverding, E. (2016a). Arbuscular mycorrhizal fungi biodiversity: prospecting in Southern-Central zone of Chile. A review. J. Soil Sci. Plant Nutr. 16, 400–422. doi: 10.4067/S0718-95162016005000036
Castillo, C. G., Oehl, F., and Sieverding, E. (2016b). Arbuscular mycorrhizal fungal diversity in wheat agro-ecosystems in Southern Chile and effects of seed treatment with natural products. J. Soil Sci. Plant Nutr. 16, 967–978. doi: 10.4067/S0718-95162016005000069
Celi, L., and Barberis, E. (2005). “Abiotic stabilization of organic phosphorus in the environment,” in Organic Phosphorus in the Environment, eds B. L. Turner, E. Frossard, and D. Baldwin (Wallingford: CABI Publishing) 113–132.
Chagnon, P. L., Bradley, R. L., Maherali, H., and Klironomos, J. N. (2013). A trait-based framework to understand life history of mycorrhizal fungi. Trends Plant Sci. 18, 484–491. doi: 10.1016/j.tplants.2013.05.001
Chen, C. R., Condron, L. M., Davis, M. R., and Sherlock, R. R. (2002). Phosphorus dynamics in the rhizosphere of perennial ryegrass (Lolium perenne L.) and radiata pine (Pinus radiata D.Don). Soil Biol. Biochem. 34, 487–499. doi: 10.1016/s0038-0717(01)00207-3
Cheng, L., Booker, F., Tu, C., Burkey, K. O., Zhou, L., Shew, H. D., et al. (2012). Arbuscular mycorrhizal fungi increase organic carbon decomposition under elevated CO2. Science 2, 1084–1087. doi: 10.1126/science.1224304
Cordell, D., Drangert, J. O., and White, S. (2009). The story of phosphorus: global food security and food for thought. Glob. Environ. Change 19, 292–305. doi: 10.1016/j.gloenvcha.2008.10.009
Cruz-Paredes, C., López-García, Á., Rubæk, G. H., Hovmand, M. F., Sørensen, P., and Kjøller, R. (2017). Risk assessment of replacing conventional P fertilizers with biomass ash: residual effects on plant yield, nutrition, cadmium accumulation and mycorrhizal status. Sci. Total Environ. 575, 1168–1176. doi: 10.1016/j.scitotenv.2016.09.194
Dai, M., Hamel, C., Bainard, L. D., Arnaud, M. S., Grant, C. A., Lupwayi, N. Z., et al. (2014). Negative and positive contributions of arbuscular mycorrhizal fungal taxa to wheat production and nutrient uptake efficiency in organic and conventional systems in the Canadian prairie. Soil Biol. Biochem. 74, 156–166. doi: 10.1016/j.soilbio.2014.03.016
Davison, J., Moora, M., Öpik, M., Adholeya, A., Ainsaar, L., Bâ, A., et al. (2015). Global assessment of arbuscular mycorrhizal fungus diversity reveals very low endemism. Nature 349, 970–973. doi: 10.1126/science.aab1161
Declerck, S., Plenchette, C., and Strullu, D. G. (1995). Mycorrhizal dependency of banana (Musa acuminata, AAA group) cultivar. Plant Soil 176, 183–187. doi: 10.1007/BF00017688
Delgado, M., Zúñiga-Feest, A., Alvear, M., and Borie, F. (2013). The effect of phosphorus on cluster-root formation and functioning of Embothrium coccineum (R. et J. Forst.). Plant Soil 373, 765–773. doi: 10.1007/s11104-013-1829-3
do Nascimento, C. A., Pagliari, P. H., Schmitt, D., He, Z., and Waldrip, H. (2016). Phosphorus concentrations in sequentially fractionated soil samples as affected by digestion methods. Sci. Rep. 5:17967. doi: 10.1038/srep17967
El Rabey, H. A., Alshubaily, F., and Al-Otaibi, K. M. (2015). Phylogenetic relationships of some economically important cereal plants based on genome characterization using molecular markers. Caryologia 68, 225–232. doi: 10.1080/00087114.2015.1032612
FAO (2012). World Agriculture: Towards 2030/2050. The 2012 Revision. Available online at: http://www.fao.org/docrep/016/ap106e/ap106e.pdf (Accessed Jun 01, 2016).
FAO (2013). World Agriculture Statistics. Available online at: http://faostat3.fao.org/home/E (Accessed: June 01, 2016).
Feddermann, N., Finlay, R., Boller, T., and Elfstrand, M. (2010). Functional diversity in arbuscular mycorrhiza–the role of gene expression, phosphorous nutrition and symbiotic efficiency. Fungal Ecol. 3, 1–8. doi: 10.1016/j.funeco.2009.07.003
Fitter, A. H. (2004). Magnolioid roots–hairs, architecture and mycorrhizal dependency. New Phytol. 164, 15–16. doi: 10.1111/j.1469-8137.2004.01193.x
Fusconi, A. (2014). Regulation of root morphogenesis in arbuscular mycorrhizae: what role do fungal exudates, phosphate, sugars and hormones play in lateral root formation? Ann. Bot-London 113, 19–33. doi: 10.1093/aob/mct258
Gahoonia, T. S., Care, D., and Nielsen, N. E. (1997). Root hairs and phosphorus acquisition of wheat and barley cultivars. Plant Soil 191, 181–188. doi: 10.1023/A:1004270201418
Gahoonia, T. S., Claassen, N., and Jungk, A. (1992). Mobilization of phosphate in different soils by ryegrass supplied with ammonium or nitrate. Plant Soil 140, 241–248. doi: 10.1007/BF00010600
Gahoonia, T. S., and Nielsen, N. E. (1992). The effect of root induced pH changes on the depletion of inorganic and organic phosphorus in the rhizosphere. Plant Soil 143, 185–191. doi: 10.1007/BF00007872
Gahoonia, T. S., and Nielsen, N. E. (1996). Variation in acquisition of soil phosphorus among wheat and barley genotypes. Plant Soil 178, 223–230. doi: 10.1007/BF00011587
Gaxiola, R. A., Edwards, M., and Elser, J. J. (2001). A transgenic approach to enhance phosphorus use efficiency in crops as part of a comprehensive strategy for sustainable agriculture. Chemosphere 84, 840–845. doi: 10.1016/j.chemosphere.2011.01.062
Geelhoed, J. S., van Riemsdijk, W. H., and Findenegg, G. R. (1999). Simulation of the effect of citrate exudation from roots on the plant availability of phosphate adsorbed on goethite. Eur. J. Soil Sci. 50, 379–390. doi: 10.1046/j.1365-2389.1999.00251.x
George, T. S., Gregory, P. J., Simpson, R. J., and Richardson, A. E. (2007). Differential interactions of Aspergillus niger and Peniophora lycii phytases with soil particles affects the hydrolysis of inositol phosphates. Soil Biol. Biochem. 39, 793–803. doi: 10.1016/j.soilbio.2006.09.029
Gerke, J. (1992). Phosphate, aluminium and iron in the soil solution of three different soils in relation to varying concentrations of citric acid. J. Plant Nut. Soil Sci. 155, 339–343. doi: 10.1002/jpln.19921550417
Giles, C. D., Brown, L. K., Adu, M. O., Mezeli, M. M., Sandral, G. A., Simpson, R. J., et al. (2017). Response-based selection of barley cultivars and legume species for complementarity: Root morphology and exudation in relation to nutrient source. Plant Sci. 255, 12–28. doi: 10.1016/j.plantsci.2016.11.002
Goh, T. B., Banerjee, M. R., Tu, S., and Burton, D. L. (1997). Vesicular arbuscular mycorrhizae-mediated uptake and translocation of P and Zn by wheat in a calcareous soil. Can. J. Plant Sci. 339–346. doi: 10.4141/P95-079
Goicoechea, N., Sánchez-Diaz, M., Sáez, R., and Irañeta, J. (2004). The Association of Barley with AM fungi can result in similar yield and grain quality as a long term application of P or P-K fertilizers by enhancing root phosphatase activity and sugars in leaves at tillering. Biol. Agric. Hortic. 22, 69–80. doi: 10.1080/01448765.2004.9754989
Goos, R. J., Johnson, B. E., and Stack, R. W. (1994). Penicillium bilaji and phosphorus fertilization effects on the growth, development yield and common root rot severity of spring wheat. Fert. Res. 39, 97–103. doi: 10.1007/BF00750908
Gordon-Weeks, R., Tong, Y., Davies, T. G., and Leggewie, G. (2003). Restricted spatial expression of a high-affinity phosphate transporter in potato roots. J. Cell Sci. 116, 3135–3144. doi: 10.1242/jcs.00615
Grace, E. J., Cotsaftis, O., Tester, M., Smith, F. A., and Smith, S. E. (2009). Arbuscular mycorrhizal inhibition of growth in barley cannot be attributed to extent of colonization, fungal phosphorus uptake or effects on expression of plant phosphate transporter genes. New Phytol. 181, 938–949. doi: 10.1111/j.1469-8137.2008.02720.x
Graham, J. H., and Abbott, L. K. (2000). Wheat responses to aggressive and non-aggressive arbuscular mycorrhizal fungi. Plant Soil 220, 207–218. doi: 10.1023/a:1004709209009
Gruber, B. D., Giehl, R. F., Friedel, S., and von Wirén, N. (2013). Plasticity of the Arabidopsis root system under nutrient deficiencies. Plant Physiol. 163, 161–179. doi: 10.1104/pp.113.218453
Gyaneshwar, P., Naresh Kumar, G., Parekh, L. J., and Poole, P. S. (2002). Role of soil microorganisms in improving P nutrition of plants. Plant Soil 245, 83–93. doi: 10.1023/A:1020663916259
Hammond, J. P., Broadley, M. R., White, P. J., King, G. J., Bowen, H. C., Hayden, R., et al. (2009). Shoot yield drives phosphorus use efficiency in Brassica oleracea and correlates with root architecture traits. J. Exp. Bot. 60, 1953–1968. doi: 10.1093/jxb/erp083
Harrison, M. J. (2005). Signaling in the arbuscular mycorrhizal symbiosis. Annu. Rev. Microbiol. 59, 19–42. doi: 10.1146/annurev.micro.58.030603.123749
Hart, M. M., and Reader, R. J. (2002). Taxonomic basis for variation in the colonization strategy of arbuscular mycorrhizal fungi. New Phytol. 153, 335–344. doi: 10.1046/j.0028-646X.2001.00312.x
Harvey, P. R., Warren, R. A., and Wakelin, S. A. (2009). Potential to improve root access to phosphorus: the role of nonsymbiotic microbial inoculants in the rhizosphere. Crop Pasture Sci. 60, 144–151. doi: 10.1071/CP08084
Helyar, K. R. (1998). Efficiency of nutrient utilization and sustaining soil fertility with particular reference to phosphorus. Field Crops Res. 56, 187–195. doi: 10.1016/S0378-4290(97)00129-9
Hetrick, B. A. D., Wilson, G. W. T., and Todd, T. C. (1996). Mycorrhizal response in wheat cultivars: relationship to phosphorus. Can. J. Bot. 74, 19–25. doi: 10.1139/b96-003
Hetrick, B., Wilson, G., and Cox, T. (1992). Mycorrhizal dependece of modern wheat varieties, landraces, and ancestors. Can. J. Bot. 70, 2032–2040. doi: 10.1139/b92-253
Higo, M., Isobe, K., Miyazawa, Y., Matsuda, Y., Drijber, R. A., and Torigoe, Y. (2016). Molecular diversity and distribution of indigenous arbuscular mycorrhizal communities colonizing roots of two different winter cover crops in response to their root proliferation. J. Microbiol. 54, 86–97. doi: 10.1007/s12275-016-5379-2
Hinsinger, P. (2001). Bioavailability of soil inorganic P in the rhizosphere as affected by root-induced chemical changes: a review. Plant Soil 237, 173–195. doi: 10.1023/A:1013351617532
Hinsinger, P., Bengough, A. G., Vetterlein, D., and Young, I. M. (2009). Rhizosphere: biophysics, biogeochemistry and ecological relevance. Plant Soil 321, 117–152. doi: 10.1007/s11104-008-9885-9
Hinsinger, P., Herrmann, L., Lesueur, A. R., Robin, A., Trap, J., Waithaisong, K., et al. (2015). Impact of roots, microorganisms and microfauna on the fate of soil phosphorus in the rhizosphere. Annu. Plant Rev. 48, 77–408. doi: 10.1002/9781118958841.ch13
Hinsinger, P., Plassard, C., Tang, C., and Jaillard, B. (2003). Origins of root-mediated pH changes in the rhizosphere and their responses to environmental constraints: A review. Plant Soil 248, 43–59. doi: 10.1023/A:1022371130939
Ho, M. D., Rosas, J. C., Brown, K. M., and Lynch, J. P. (2005). Root architectural tradeoffs for water and phosphorus acquisition. Funct. Plant Biol. 32, 737–748. doi: 10.1071/FP05043
Hoad, S. P., Russell, G., Lucas, M. E., and Bingham, I. J. (2001). The management of wheat, barley, and oat root systems. Adv. Agron. 74, 193–246. doi: 10.1016/S0065-2113(01)74034-5
Hodge, A., Berta, G., Doussan, C., Merchan, F., and Crespi, M. (2009). Plant root growth, architecture and function. Plant Soil 321, 153–187. doi: 10.1007/s11104-009-9929-9
Hodge, A., Helgason, T., and Fitter, A. H. (2010). Nutritional ecology of arbuscular mycorrhizal fungi. Fung. Ecol. 3, 267–273. doi: 10.1016/j.funeco.2010.02.002
Holford, I. C. R. (1997). Soil phosphorus: its measurement, and its uptake by plants. Aust. J. Soil. Res. 35, 227–239. doi: 10.1071/S96047
Holloway, R. E., Bertrand, I., Frischke, A. J., Brace, D. M., McLaughlin, M. J., and Shepperd, W. (2001). Improving fertiliser efficiency on calcareous and alkaline soils with fluid sources of P, N and Zn. Plant Soil 236, 209–219. doi: 10.1023/A:1012720909293
Hu, J., Yang, A., Zhu, A., Wang, J., Dai, J., Wong, M. H., et al. (2015). Arbuscular mycorrhizal fungal diversity, root colonization, and soil alkaline phosphatase activity in response to maize-wheat rotation and no-tillage in North China. J. Microbiol. 53, 454–461. doi: 10.1007/s12275-015-5108-2
Jakobsen, I. (1995). “Transport of phosphorus and carbon in VA mycorrhizas,” in Mycorrhiza: Structure, Function, Molecular Biology and Biotechnology, eds A. Varma and B. Hock (Berlin: Springer), 297–324.
Jakobsen, I., Leggett, M. E., and Richardson, A. E. (2005). “Rhizosphere microorganisms and plant phosphorus uptake,” in Phosphorus, Agriculture and the Environment, eds J.T. Sims and A. N. Sharpley (Madison, WI: American Society for Agronomy), 437–494.
Jansa, J., Bukovská, P., and Gryndler, M. (2013). Mycorrhizal hyphae as ecological niche for highly specialized hypersymbionts–or just soil free-riders? Front. Plant Sci. 4:134 doi: 10.3389/fpls.2013.00134
Jansa, J., Finlay, R., Wallander, H., Smith, F. A., and Smith, S. E. (2011). “Role of mycorrhizal symbiosis in phosphorus cycling,” in Phosphorus in Action. Biological Processes in Soil Phosphorus Cycling, eds E. K. Bunemann, A. Oberson, and E. Frossard (Berlin; Heidelberg: Springer-Verlag), 169–198.
Jansa, J., Mozafar, A., and Frossard, E. (2003a). Long-distance transport of P and Zn through the hyphae of an arbuscular mycorrhizal fungus in symbiosis with maize. Agronomie 23, 481–488. doi: 10.1051/agro:2003013
Jansa, J., Mozafar, A., Kuhn, G., Anken, T., Ruh, R., Sanders, I. R., et al. (2003b). Soil tillage affects the community structure of mycorrhizal fungi inmaize roots. Ecol. Appl. 13, 1164–1176. doi: 10.1890/1051-0761(2003)13[1164:STATCS]2.0.CO;2
Jansa, J., Smith, F. A., and Smith, S. E. (2008). Are there benefits of simultaneous root colonization by diferent arbuscular mycorrhizal fungi? New Phytol. 177, 779–789. doi: 10.1111/j.1469-8137.2007.02294.x
Javot, H., Pumplin, N., and Harrison, M. J. (2007). Phosphate in the arbuscular mycorrhizal symbiosis: transport properties and regulatory roles. Plant Cell Environ. 30, 310–322. doi: 10.1111/j.1365-3040.2006.01617.x
Jensen, A. (1982). Influence of four vesicular-arbuscular mycorrhizal fungi on nutrient uptake and growth in Barley (Hordeum Vulgare). New Phytol. 90, 45–50. doi: 10.1111/j.1469-8137.1982.tb03239.x
Jensen, A. (1984). Responses of barley, pea, and maize to inoculation with different vesicular-arbuscular mycorrhizal fungi in irradiated soil. Plant Soil 78, 315–323. doi: 10.1007/BF02450365
Johnson, J. F., Allan, D. L., Vance, C. P., and Weiblen, G. (1996). Root carbon dioxide fixation by phosphorus-deficient Lupinus albus: contribution to organic-acid exudation by proteoid roots. Plant Physiol. 112, 19–30. doi: 10.1104/pp.112.1.19
Johnson, N. C., Graham, J. H., and Smith, F. A. (1997). Functioning of mycorrhizal associations along the mutualism–parasitism continuum. New Phytol. 135, 575–586. doi: 10.1046/j.1469-8137.1997.00729.x
Joner, E. J., and Jakobsen, I. (1995). Growth and extracellular phosphatase activity of arbuscular mycorrhizal hyphae as influenced by soil organic matter. Soil Biol. Biochem. 27, 1153–1159. doi: 10.1016/0038-0717(95)00047-I
Joner, E. J., Van Aarle, I. M., and Vosatka, M. (2000). Phosphatase activity of extra-radical arbuscular mycorrhizal hyphae: a review. Plant Soil 226, 199–210. doi: 10.1023/A:1026582207192
Jones, D. L. (1998). Organic acids in the rhizosphere–a critical review. Plant Soil 205, 25–44. doi: 10.1023/A:1004356007312
Jones, D. L., and Darrah, P. R. (1994). Amino-acid influx at the soil-root interface of Zea mays L. and its implications in the rhizosphere. Plant Soil 163, 1–12. doi: 10.1007/BF00033935
Jones, D. L., and Oburger, E. (2011). “Solubilization of phosphorus by soil microorganisms,” in Phosphorus in Action. Biological Processes in Soil Phosphorus Cycling, eds E. K. Bunemann, A. Oberson, and E. Frossard (Berlin; Heidelberg: Springer-Verlag), 169–198.
Jung, S. C., Martinez-Medina, A., Lopez-Raez, J. A., and Pozo, M. J. (2012). Mycorrhiza-induced resistance and priming of plant defenses. J. Chem. Ecol. 38, 651–664. doi: 10.1007/s10886-012-0134-6
Kaiser, C., Kilburn, M. R., Clode, P. L., Fuchslueger, L., Koranda, M., Cliff, J. B., et al. (2015). Exploring the transfer of recent plant photosynthates to soil microbes: mycorrhizal pathway vs direct root exudation. New Phytol. 205, 1537–1551. doi: 10.1111/nph.13138
Karamanos, R. E., Flore, N. A., and Harapiak, J. T. (2010). Re-visiting use of Penicillium bilaii with phosphorus fertilization of hard red spring wheat. Can. J. Plant Sci. 90, 265–277. doi: 10.4141/CJPS09123
Karasawa, T., Kasahara, Y., and Takebe, M. (2001). Variable response of growth and arbuscular mycorrhizal colonization of maize plants to preceding crops in various types of soils. Biol. Fertil. Soils 33, 286–293. doi: 10.1007/s003740000321
Keerthisinghe, G., Hocking, P. J., Ryan, P. R., and Delhaize, E. (1998). Effect of phosphorus supply on the formation and function of proteoid roots of white lupin (Lupinus albus L.). Plant Cell Environ. 21, 467–478. doi: 10.1046/j.1365-3040.1998.00300.x
Khademi, Z., Jones, D. L., Malakouti, M. J., and Asadi, F. (2010). Organic acids differ in enhancing phosphorus uptake by Triticum aestivum L.-effects of rhizosphere concentration and counterion. Plant Soil 334, 151–159. doi: 10.1007/s11104-009-0215-7
Khademi, Z., Jones, D. L., Malakouti, M. J., Asadi, F., and Ardebili, M. (2009). Organic acid mediated nutrient extraction efficiency in three calcareous soils. Aust. J. Soil Res. 47, 213–220. doi: 10.1071/SR07179
Khaliq, A., and Sanders, F. E. (1998). Effects of vesicular arbuscular mycorrhizal inoculation on growth and phosphorus nutrition of barley in natural or methyl bromide-treated soil. J. Plant Nutr. 21, 2163–2177. doi: 10.1080/01904169809365552
Khaliq, A., and Sanders, F. E. (2000). Effects of vesicular-arbuscular mycorrhizal inoculation on the yield and phosphorus uptake of field-grown barley. Soil Biol. Biochem. 32, 1691–1696. doi: 10.1016/S0038-0717(00)00086-9
Khan, M. S., and Zaidi, A. (2007). Synergistic effects of the inoculation with plant growth-promoting rhizobacteria and an arbuscular mycorrhizal fungus on the performance of wheat. Turkish J. Agric. For. 31, 355–362. doi: 10.1002/jpln.200620602
Khan, M. S., Zaidi, A., Ahemad, M., Oves, M., and Wani, P. A. (2010). Plant growth promotion by phosphate solubilizing fungi—current perspective. Arch. Agron. Soil Sci. 56, 73–98. doi: 10.1080/03650340902806469
Kivlin, S. N., Hawkes, C. V., and Treseder, K. K. (2011). Global diversity and distribution of arbuscular mycorrhizal fungi. Soil Biol. Biochem. 43, 2294–2303. doi: 10.1016/j.soilbio.2011.07.012
Klironomos, J. N. (2003). Variation in plant response to native and exotic arbuscular mycorrhizal fungi. Ecology 84, 2292–2301. doi: 10.1890/02-0413
Klugh, K. R., and Cumming, J. R. (2007). Variations in organic acid exudation and aluminum resistance among arbuscular mycorrhizal species colonizing Liriodendron tulipifera. Tree Physiol. 27, 1103–1112. doi: 10.1093/treephys/27.8.1103
Klugh-Stewart, K., and Cumming, J. R. (2009). Organic acid exudation by mycorrhizal Andropogon virginicus L. (broomsedge) roots in response to aluminum. Soil Biol. Biochem. 41, 367–373. doi: 10.1016/j.soilbio.2008.11.013
Koch, A. M., Antunes, P. M., Maherali, H., and Klironomos, J. N. (2017). Evolutionary asymmetry in the mycorrhizal symbiosis: I. Conservatism in Glomeromycota fungal morphology does not predict host plant growth response. New Phytol. 214, 1330–1337. doi: 10.1111/nph.14465
Koch, A. M., Kuhn, G., Fontanillas, P., Fumagalli, L., Goudet, J., and Sanders, I. R. (2004). High genetic variability and low local diversity in a population of arbuscular mycorrhizal fungi. Proc. Natl. Acad. Sci. U.S.A. 101, 2369–2374. doi: 10.1073/pnas.0306441101
Kochian, L., Hoekenga, O., and Pineros, M. A. (2004). How do crop plants tolerate acid soils? Mechanisms of aluminum tolerance and phosphorus efficiency. Annu. Rev. Plant Biol. 55, 459–493. doi: 10.1146/annurev.arplant.55.031903.141655
Koide, R. T., and Kabir, Z. (2000). Extraradical hyphae of the mycorrhizal fungus Glomus intraradices can hydrolyse inorganic phosphate. New Phytol. 148, 511–517. doi: 10.1046/j.1469-8137.2000.00776.x
Kucey, R. M. (1987). Increased phosphorus uptake by wheat and field beans inoculated with a phosphorus-solubilizing penicillium bilaji strain and with vesicular-arbuscular mycorrhizal fungi. Appl. Environ. Microbiol. 53, 2699–2703.
Kucey, R. M. N., Jenzen, H. H., and Leggett, M. E. (1989). Microbially mediated increases in plant available phosphorus. Adv. Agron. 42, 199–228. doi: 10.1016/S0065-2113(08)60525-8
Kujira, Y., Grove, J. H., and Ronzelli, P. (1994). Varietal differences of root systems in winter-wheat seedlings. Jap. J. Crop Sci. 63, 524–530. doi: 10.1626/jcs.63.524
Lan, M., Comerford, N. B., and Fox, T. R. (1995). Organic anions effect on phosphorus release from spodic horizons. Soil Sci. Soc. Am. J. 59, 1745–1749. doi: 10.2136/sssaj1995.03615995005900060034x
Leggett, M., Cross, J., Hnatowich, G., and Holloway, G. (2007). “Challenges in commercializing a phosphate-solubilizing microorganisms: Penicillium bilaiae, a case history,” in First International Meeting on Microbial Phosphate Solubilization, eds E. Velázquez, C. Rodríguez-Barrueco, E. Velázquez, and C. Rodríguez-Barrueco (Dordrecht: Springer), 215–222.
Li, H., Smith, S. E., Holloway, R. E., Zhu, Y., and Smith, F. A. (2006). Arbuscular mycorrhizal fungi contribute to phosphorus uptake by wheat grown in a phosphorus-fixing soil even in the absence of positive growth responses. New Phytol. 172, 536–543. doi: 10.1111/j.1469-8137.2006.01846.x
Li, H. Y., Zhu, Y. G., Marschner, P., Smith, F. A., and Smith, S. E. (2005). Wheat responses to arbuscular mycorrhizal fungi in a highly calcareous soil differ from those of clover, and change with plant development and P supply. Plant Soil 277, 221–232. doi: 10.1007/s11104-005-7082-7
Liao, M., Hocking, P. J., Dong, B., Delhaize, E., Richardson, A. E., and Ryan, P. R. (2008). Variation in early phosphorus-uptake efficiency among wheat genotypes grown on two contrasting Australian soils. Aust. J. Agri. Res. 59, 157–166. doi: 10.1071/AR06311
Liu, C., Muchhal, U. S., Uthappa, M., Kononowicz, A. K., and Raghothama, K. G. (1998). Tomato phosphate transporter genes are differentially regulated in plant tissues by phosphorus. Plant Physiol. 116, 91–99. doi: 10.1104/pp.116.1.91
Liu, F., Chang, X.-J., Ye, Y., Xie, W.-B., Wu, P., and Lian, X.-M. (2011). Comprehensive sequence and whole-life-cycle expression profile analysis of the phosphate transporter gene family in rice. Mol. Plant 4, 1105–1122. doi: 10.1093/mp/ssr058
López-Arredondo, D. L., Leyva-González, M. A., González-Morales, S. I., López-Bucio, J., and Herrera-Estrella, L. (2014). Phosphate nutrition: improving low-phosphate tolerance in crops. Annu. Rev. Plant Biol. 65, 95–123. doi: 10.1146/annurev-arplant-050213-035949
López-García, Á., Varela-Cervero, S., Vasar, M., Öpik, M., Barea, J. M., and Azcón-Aguilar, C. (2017). Plant traits determine the phylogenetic structure of arbuscular mycorrhizal fungal communities. Mol. Ecol. 26, 6948–6959. doi: 10.1111/mec.14403
López-Ráez, J. A., Charnikhova, T., Gómez-Roldán, V., Matusova, R., Kohlen, W., de Vos, R., et al. (2008). Tomato strigolactones are derived from carotenoids and their biosynthesis is promoted by phosphate starvation. New Phytol. 178, 863–874. doi: 10.1111/j.1469-8137.2008.02406.x
López-Ráez, J. A., Shirasu, K., and Foo, E. (2017). Strigolactones in plant interactions with beneficial and detrimental organisms: the Yin and Yang. Trends Plant Sci. 22, 527–537. doi: 10.1016/j.tplants.2017.03.011
Lung, S.-C., Chan, W.-L., Yip, W., Wang, L., Yeung, E. C., and Lim, B. L. (2005). Secretion of beta-propeller phytase from tobacco and Arabidopsis roots enhances phosphorus utilization. Plant Sci. 169, 341–349. doi: 10.1016/j.plantsci.2005.03.00
Lung, S.-C., and Lim, B. L. (2006). Assimilation of phytate-phosphorus by the extracellular phytase activity of tobacco (Nicotiana tabaccum) is affected by the availability of soluble phytate. Plant Soil 279, 187–199. doi: 10.1007/s11104-005-1009-1
Lynch, J. P. (2007). Roots of the second green revolution. Aust. J. Bot. 55, 1–20. doi: 10.1071/BT06118
Lynch, J. P., and Ho, M. D. (2005). Rhizoeconomics: Carbon costs of phosphorus acquisition. Plant Soil 269, 45–56. doi: 10.1007/s11104-004-1096-4
Ma, Q., Rengel, Z., and Rose, T. J. (2009). The effectiveness of deep placement of fertilisers is determined by crop species and edaphic conditions in Mediterranean-type environments: a review. Aust. J. Soil Res. 47, 19–32. doi: 10.1071/SR08105
Maherali, H. (2014). Is there an association between root architecture and mycorrhizal growth response? New Phytol. 204, 192–200. doi: 10.1111/nph.12927
Maillet, F., Poinsot, V., André, O., Puech-Pagès, V., Haouy, A., Gueunier, M., et al. (2011). Fungal lipochitooligosaccharide symbiotic signals in arbuscular mycorrhiza. Nature 469, 58–63. doi: 10.1038/nature09622
Maldonado-Mendoza, I. E., Dewbre, G. R., and Harrison, M. J. (2001). A phosphate transporter gene from the extra-radical mycelium of an arbuscular mycorrhizal fungus Glomus intraradices is regulated in response to phosphate in the environment. Mol. Plant Microbe Interact. 14, 1140–1148. doi: 10.1094/MPMI.2001.14.10.1140
Manoharan, L., Rosenstock, N. P., Williams, A., and Hedlund, K. (2017). Agricultural management practices influence AMF diversity and community composition with cascading effects on plant productivity. Agric. Ecosyst. Environ,. Appl. Soil Ecol. 115, 53–59. doi: 10.1016/j.apsoil.2017.03.012
Manske, G. G. B., Ortiz-Monasterio, J. I., Van Ginkel, M., González, R. M., Rajaram, S., Molina, E., et al. (2000). Traits associated with improved P-uptake efficiency in CIMMYT's semidwarf spring bread wheat grown on an acid Andisol in Mexico. Plant Soil 221, 189–204. doi: 10.1023/A:1004727201568
Mao, L., Liu, Y., Shi, G., Jiang, S., Cheng, G., Li, X., et al. (2014). Wheat cultivars form distinctive communities of root-associated arbuscular mycorrhiza in a conventional agroecosystem. Plant Soil 374, 949–961. doi: 10.1007/s11104-013-1943-2
Marschener, H. (1998). Role of root growth, arbuscular mycorrhiza, and root exudates for the efficiency in nutrient acquisition. F. Crop. Res. 56, 203–207. doi: 10.1016/S0378-4290(97)00131-7
Marschner, P. (ed.). (2012). “Phosphorus,” in Marschner's Mineral Nutrition of Higher Plant, 3rd Edn (London: Academic Press; Elsevier Ltd.), 265–277.
McIvor, J. G., Guppy, C., and Probert, M. E. (2011). Phosphorus requirements of tropical grazing systems: the northern Australian experience. Plant Soil. 349, 55–67. doi: 10.1007/s11104-011-0906-8
Mehrvarz, S., Chaichi, M. R., and Alikhani, H. A. (2008). Effects of phosphate solubilizing microorganisms and phosphorus chemical fertilizer on yield and yield components of barley (Hordeum vulgare L.). Am. Eur. J. Agric. Env. Sci. 3, 822–828.
Merryweather, J., and Fitter, A. (1998). The arbuscular mycorrhizal fungi of Hyacinthoides non-scripta I. Diversity of fungal taxa. New Phytol. 138, 117–129. doi: 10.1046/j.1469-8137.1998.00888.x
Miguel, M. A. (2011). Functional Role and Synergistic Effect of Root Traits for Phosphorus Acquisition Efficiency and Their Genetic Basis in Common Bean (Phaseolus vulgaris L.). Ph.D. thesis, Pennsylvania State University, University Park.
Mohammad, M. J., and Malkawi, H. I. (2004). Root, shoot and nutrient acquisition responses of mycorrhizal and nonmycorrhizal wheat to phosphorus application to highly calcareous soils. Asian J. Plant Sci. 3, 363–369. doi: 10.3923/ajps.2004.363.369
Mohammad, M. J., Malkawi, H. I., and Shibli, R. (2003). Effects of arbuscular mycorrhizal fungi and phosphorus fertilization on growth and nutrient uptake of barley grown on soils with different levels of salts. J. Plant Nutr. 26, 125–137. doi: 10.1081/PLN-120016500
Mohammad, M. J., Pan, W. L., and Kennedy, A. C. (1998). Seasonal mycorrhizal colonisation of winter wheat and its effect on wheat growth under dryland field conditions. Mycorrhiza 8, 139–144. doi: 10.1007/s005720050226
Morales, A., Alvear, M., Valenzuela, E., Castillo, C. E., and Borie, F. (2011). Screening, evaluation and selection of phosphate-solubilising fungi as potential biofertiliser. J. Soil Sci. Plant Nut. 11, 89–103. doi: 10.4067/S0718-95162011000400007
Morgan, J. A., Bending, G. D., and White, P. J. (2005). Biological costs and benefits to plant–microbe interactions in the rhizosphere. J. Exp. Bot. 56, 1729–1739. doi: 10.1093/jxb/eri205
Mukherjee, A., and Ané, J. M. (2011). Germinating spore exudates from arbuscular mycorrhizal fungi: molecular and developmental responses in plants and their regulation by ethylene. Mol. Plant Microbe Interact. 24, 260–270. doi: 10.1094/MPMI-06-10-0146
Munkvold, L., Kjøller, R., Vestberg, M., Rosendahl, S., and Jakobsen, I. (2004). High functional diversity within species of arbuscular mycorrhizal fungi. New Phytol. 164, 357–364. doi: 10.1111/j.1469-8137.2004.01169.x
Nadji, W., Belbekri, N., Ykhlef, N., and Djekoun, A. (2017). Diversity of arbuscular mycorrhizal fungi of durum Wheat (Triticum durum Desf.) fields of the East of Algeria. J. Agric. Sci. 9:117. doi: 10.5539/jas.v9n3p117
Nannipieri, P., Giagnoni, L., Landi, L., and Renella, G. (2011). “Role of phosphatase enzymes in soil,” in Phosphorus in Action. Biological Processes in Soil Phosphorus Cycling, eds E. K. Bünemann, A. Oberson, and E. Frossard (Berlin; Heidelberg: Springer-Verlag), 169–198.
Newsham, K. K., Fitter, A. H., and Watkinson, A. R. (1995). Multi-functionality and biodiversity in arbuscular mycorrhizas. Trends Ecol. Evol. 10, 407–411. doi: 10.1016/S0169-5347(00)89157-0
Oberson, A., and Joner, E. J. (2005). “Microbial turnover of phosphorus in soil,” in Organic Phosphorus in the Environment, eds B. L. Turner, E. Frossard, and D. S. Baldwin (Wallingford: CABI), 133–164.
Olsson, P. A., van Aarle, I. M., Allaway, W. G., Ashford, A. E., and Rouhier, H. (2002). Phosphorus effects on metabolic processes in monoxenic arbuscular mycorrhiza cultures. Plant Physiol. 130, 1162–1171. doi: 10.1104/pp.009639
Omar, S. A. (1998). The role of rock-phosphate-solubilizing fungi and vesicular-arbusular-mycorrhiza (VAM) in growth of wheat plants fertilized with rock phosphate. World J. Microbiol. Biotechnol. 14, 211–218. doi: 10.1023/A:1008830129262
Öpik, M., Davison, J., Moora, M., and Zobel, M. (2014). DNA-based detection and identification of Glomeromycota: the virtual taxonomy of environmental sequences. Botany 92, 135–147. doi: 10.1139/cjb-2013-0110
Öpik, M., Vanatoa, A., Vanatoa, E., Moora, M., Davison, J., Kalwij, J. M., Zobel, M., et al. (2010). The online database MaarjAM reveals global and ecosystemic distribution patterns in arbuscular mycorrhizal fungi (Glomeromycota). New Phytol. 188, 223–241. doi: 10.1111/j.1469-8137.2010.03334.x
Ortas, I., Ortakci, D., Kaya, Z., Cinar, A., and Onelge, N. (2002). Mycorrhizal dependency of sour orange in relation to phosphorus and zinc nutrition. J. Plant Nutr. 25, 1263–1279. doi: 10.1081/PLN-120004387
Parniske, M. (2006). Plant–fungal associations: Cue for the branching connection. Nature 435, 750–751. doi: 10.1038/435750a
Pearse, S. J., Veneklaas, E. J., Cawthray, G., Bolland, M. D., and Lambers, H. (2007). Carboxylate composition of root exudates does not relate consistently to a crop species' ability to use phosphorus from aluminium, iron or calcium phosphate sources. New Phytol. 173, 181–190. doi: 10.1111/j.1469-8137.2006.01897.x
Pearson, J. N., and Jakobsen, I. (1993). The relative contribution of hyphae and roots to phosphorus uptake by arbuscular mycorrhizal plants, measured by dual labelling with 32P and 33P. New Phytol. 124, 489–494. doi: 10.1111/j.1469-8137.1993.tb03840.x
Peleg, Z., and Blumwald, E. (2011). Hormone balance and abiotic stress tolerance in crop plants. Curr. Opin. Plant Biol. 14, 290–295. doi: 10.1016/j.pbi.2011.02.001
Pellegrino, E., Öpik, M., Bonari, E., and Ercoli, L. (2015). Responses of wheat to arbuscular mycorrhizal fungi: a meta-analysis of field studies from 1975 to 2013. Soil Biol. Biochem. 84, 210–217. doi: 10.1016/j.soilbio.2015.02.020
Plenchette, C., and Morel, C. (1996). External phosphorus requirement of mycorrhizal and non- mycorrhizal barley and soybean plants. Biol. Fertil. Soils 21, 303–308. doi: 10.1007/s003740050064
Powell, J. R., Parrent, J. L., Hart, M. M., Klironomos, J. N., Rillig, M. C., and Maherali, H. (2009). Phylogenetic trait conservatism and the evolution of functional trade-offs in arbuscular mycorrhizal fungi. Proc. R. Soc. B Biol. Sci. 276, 4237–4245. doi: 10.1098/rspb.2009.1015
Pozo, M. J., López-Ráez, J. A., Azcón-Aguilar, C., and García-Garrido, J. M. (2015). Phytohormones as integrators of environmental signals in the regulation of mycorrhizal symbioses. New Phytol. 205, 1431–1436. doi: 10.1111/nph.13252
Preuss, C. P., Huang, C. Y., Gilliham, M., and Tyerman, S. D. (2010). Channel-like characteristics of the low-affinity barley phosphate transporter Pht1;6 when expressed in xenopus oocytes. Plant Physiol. 152, 1431–1441. doi: 10.1104/pp.109.152009
Qin, H., Lu, K., Strong, P. J., Xu, Q., Wu, Q., Xu, Z., et al. (2015). Long-term fertilizer application effects on the soil, root arbuscular mycorrhizal fungi and community composition in rotation agriculture. Agric. Ecosyst. Environ. Appl. Soil Ecol. 89, 35–43. doi: 10.1016/j.apsoil.2015.01.008
Rae, A. L., Cybinski, D. H., Jarmey, J. M., and Smith, F. W. (2003). Characterization of two phosphate transporters from barley; evidence for diverse function and kinetic properties among members of the Pht1 family. Plant Mol. Biol. 53, 27–36. doi: 10.1023/B:PLAN.0000009259.75314.15
Rae, A. L., Jarmey, J. M., Mudge, S. R., and Smith, F. W. (2004). Over-expression of a high-affinity phosphate transporter in transgenic barley plants does not enhance phosphate uptake rates. Funct. Plant Biol. 31, 141–148. doi: 10.1007/s11104-007-9222-8
Raghothama, K. G. (2005). “Phosphorous,” in Plant Nutritional Genomics, eds M. R. Broadley and P. J. White (Oxford: Blackwell Publishing Ltd.,), 112–126.
Ray, D. K., Ramankutty, N., Mueller, N. D., West, P. C., and Foley, J. A. (2012). Recent patterns of crop yield growth and stagnation. Nat. Commun. 3, 1293–1299. doi: 10.1038/ncomms2296
Richardson, A. E., Barea, J. M., McNeill, A. M., and Prigent-Combaret, C. (2009). Acquisition of phosphorus and nitrogen in the rhizosphere and plant growth promotion by microorganisms. Plant Soil 321, 305–339. doi: 10.1007/s11104-009-9895-2
Richardson, A. E., Hadobas, P. A., and Hayes, J. E. (2001). Extracellular secretion of Aspergillus phytase from Arabidopsis roots enables plants to obtain phosphorus from phytate. Plant J. 25, 641–649. doi: 10.1046/j.1365-313x.2001.00998.x
Richardson, A. E., Lynch, J. P., Ryan, P. R., Delhaize, E., Smith, F. A., Smith, S. E., et al. (2011). Plant and microbial strategies to improve the phosphorus efficiency of agriculture. Plant Soil 349, 121–156. doi: 10.1007/s11104-011-0950-4
Richardson, A. E., and Simpson, R. J. (2011). Soil microorganisms mediating phosphorus availability. Plant Physiol. 156, 989–996. doi: 10.1104/pp.111.175448
Rose, T. J., Pariasca-Tanaka, J., Rose, M. T., Fukuta, Y., and Wissuwa, M. (2010). Genotypic variation in grain phosphorus concentration; and opportunities to improve P-use efficiency in rice. Field Crop Res. 119, 154–160. doi: 10.1016/j.fcr.2010.07.004
Rose, T. J., and Wissuwa, M. (2012). Rethinking Internal Phosphorus Utilization Efficiency. A New Approach Is Needed to Improve PUE in Grain Crops. Burlington: 1st Edn. Elsevier Inc.
Rubio, R., Moraga, E., and Borie, F. (1990). Acid phosphatase activity and vesicular-arbuscular mycorrhizal infection associated with roots of four wheat cultivars. J. Plant Nutr. 13, 585–598. doi: 10.1080/01904169009364102
Ryan, M. H., and Angus, J. F. (2003). Arbuscular mycorrhizae in wheat and field pea crops on a low P soil: Increased Zn-uptake but no increase in P-uptake or yield. Plant Soil 250, 225–239. doi: 10.1023/A:1022839930134
Sandaña, P., and Pinochet, D. (2016). Phosphorus acquisition of wheat, pea and narrow-leafed lupin under different P supplies. J. Soil Sci. Plant Nut. 16, 537–549. doi: 10.4067/S0718-95162016005000044
Sanders, I. R. (1999). Evolutionary genetics: no sex please, we're fungi. Nature 399, 737–739. doi: 10.1038/21544
Santander, C., Aroca, R., Ruiz-Lozano, J. M., Olave, J., Cartes, P., and Borie, F. (2017). Arbuscular mycorrhiza effects on plant performance under osmotic stress. Mycorrhiza 27:639–657. doi: 10.1007/s00572-017-0784-x
Sato, T., Ezawa, T., Cheng, W., and Tawaraya, K. (2015). Release of acid phosphatase from extraradical hyphae of arbuscular mycorrhizal fungus Rhizophagus clarus. Soil Sci. Plant Nutr. 61, 269–274. doi: 10.1080/00380768.2014.993298
Sattari, S. Z., Bouwman, A. F., Giller, K. E., and van Ittersum, M. K. (2012). Residual soil phosphorus as the missing piece in the global phosphorus crisis puzzle. Proc. Natl. Acad. Sci. U.S.A. 109, 6348–6353. doi: 10.1073/pnas.1113675109
Saxena, J., Chandra, S., and Nain, L. (2013). Synergistic effect of phosphate solubilizing rhizobacteria and arbuscular mycorrhiza on growth and yield of wheat plants. J. Soil Sci. Plant Nutr. 13, 511–525. doi: 10.4067/S0718-95162013005000040
Scannerini, S., Fusconi, A., and Mucciarelli, M. (2001). “The effect of endophytic fungi on host plant morphogenesis,” in Symbiosis: Organisms and Model Systems, ed J. Seckback (Kluwer: Dordrecht), 427–447.
Schachtman, D. P., Reid, R. J., and Ayling, S. M. (1998). Phosphorus uptake by plants: from soil to cell. Plant Physiol. 116, 447–453. doi: 10.1104/pp.116.2.447
Schellenbaum, L., Berta, G., Raviolanirina, F., Tisserant, B., Gianinazzi, S., and Fitter, H. (1991). Influence of endomycorrhizal infection on root morphology in a micropropagated woody plant species (Vitis vinifera L.). Ann. Bot. Lond. 68, 135–141. doi: 10.1093/oxfordjournals.aob.a088231
Schmidt, G., and Laskowski, M. S. (1961). “Phosphatase ester cleavage (survey),” in The enzymes, 2nd Edn., eds P. D. Boyer, H. Lardy, and K. Myrback (New York, NY: Academic), 3–35.
Schnepf, A., Roose, T., and Schweiger, P. (2008). Impact of growth and uptake patterns of arbuscular mycorrhizal fungi on plant phosphorus uptake–a modelling study. Plant Soil 312, 85–99. doi: 10.1007/s11104-008-9749-3
Schweiger, P. F., Robson, A. D., and Barrow, N. J. (1995). Root hair length determines beneficial effect of a Glomus species on shoot growth of some pasture species. New Phytol. 131, 247–254. doi: 10.1111/j.1469-8137.1995.tb05726.x
Seguel, A., Barea, J. M., Cornejo, P., and Borie, F. (2015). Role of arbuscular mycorrhizal symbiosis in phosphorus-uptake efficiency and aluminium tolerance in barley growing in acid soils. Crop Pasture Sci. 66, 696–705. doi: 10.1071/CP14305
Seguel, A., Cornejo, P., Ramos, A., Von Baer, E., Cumming, J., and Borie, F. (2017). Phosphorus acquisition by three wheat cultivars contrasting in aluminium tolerance growing in an aluminium-rich volcanic soil. Crop Pasture Sci. 68, 305–316. doi: 10.1071/CP16224
Seguel, A., Cumming, J., Cornejo, P., and Borie, F. (2016a). Aluminum tolerance of wheat cultivars and relation to arbuscular mycorrhizal colonization in a non-limed and limed Andisol. Agric. Ecosyst. Environ. Appl. Soil Ecol. 108, 228–237. doi: 10.1016/j.apsoil.2016.08.014
Seguel, A., Castillo, C., Morales, A., Campos, P., Cornejo, P., and Borie, F. (2016b). Arbuscular Mycorrhizal symbiosis in four Al-tolerant wheat genotypes grown in an acidic Andisol. J. Soil Sci. Plant Nutr. 16, 164–173. doi: 10.4067/S0718-95162016005000013
Seguel, A., Cumming, J. R., Klugh-Stewart, K., Cornejo, P., and Borie, F. (2013). The role of arbuscular mycorrhizas in decreasing aluminium phytotoxicity in acidic soils: a review. Mycorrhiza 23, 167–183. doi: 10.1007/s00572-013-0479-x
Shang, C., Stewart, J. W. B., and Huang, P. M. (1992). pH effect on kinetics of adsorption of organic and inorganic phophates by short-range ordered aluminium and iron precipitates. Geoderma 53, 1–14. doi: 10.1016/0016-7061(92)90017-2
Singh, S., and Kapoor, K. K. (1999). Inoculation with phosphate-solubilizing microorganisms and a vesicular-arbuscular mycorrhizal fungus improves dry matter yield and nutrient uptake by wheat grown in a sandy soil. Biol. Fertil. Soils 28, 139–144. doi: 10.1007/s003740050475
Smernik, R. J., and Dougherty, W. J. (2007). Identification of phytate in phosphorus-31 nuclear magnetic resonance spectra: the need for spiking. Soil Sci. Soc. Am. J. 71, 1045–1050. doi: 10.2136/sssaj2006.0295
Smith, F. A., Grace, E. J., and Smith, S. E. (2009). More than a carbon economy: nutrient trade and ecological sustainability in facultative arbuscular mycorrhizal symbioses. New Phytol. 182, 347–358. doi: 10.1111/j.1469-8137.2008.02753.x
Smith, S. E., Jakobsen, I., Grønlund, M., and Smith, F. A. (2011). Roles of arbuscular mycorrhizas in plant phosphorus nutrition: interactions between pathways of phosphorus uptake in arbuscular mycorrhizal roots have important implications for understanding and manipulating plant phosphorus acquisition. Plant Physiol. 156, 1050–1057. doi: 10.1104/pp.111.174581
Smith, S. E., Manjarrez, M., Stonor, R., McNeill, A., and Smith, F. A. (2015). Indigenous arbuscular mycorrhizal (AM) fungi contribute to wheat phosphate uptake in a semi-arid field environment, shown by tracking with radioactive phosphorus. Agric. Ecosyst. Environ. Appl. Soil Ecol. 96, 68–74. doi: 10.1016/j.apsoil.2015.07.002
Smith, S. E., Smith, F. A., and Jakobsen, I. (2004). Functional diversity in arbuscular mycorrhizal (AM) symbioses: the contribution of the mycorrhizal P uptake pathway is not correlated with mycorrhizal responses in growth or total P uptake. New Phytol. 162, 511–524. doi: 10.1111/j.1469-8137.2004.01039.x
Snajdr, J., Valaskova, V., Merhautova, V., Herinková, J., Cajthaml, T., and Baldrian, P. (2008). Spatial variability of enzyme activities and microbial biomass in the upper layers of Quercus petraea forest soil. Soil Biol. Biochem. 40, 2068–2075. doi: 10.1016/j.soilbio.2008.01.015
Spohn, M., Ermak, A., and Kuzyakov, Y. (2013). Microbial gross organic-Phosphorus mineralization can be stimulated by root exudates a 33P isotopic dilution study. Soil Biol. Biochem. 65, 254–263. doi: 10.1016/j.soilbio.2013.05.028
Spohn, M., and Kuzyakov, Y. (2013). Distribution of microbial- and root-derived phosphatase activities in the rhizosphere depending on P availability and C allocation-Coupling soil zymography with 14C imaging. Soil Biol. Biochem. 67, 106–113. doi: 10.1016/j.soilbio.2013.08.015
Stursova, M., and Baldrian, P. (2011). Effects of soil properties and management on the activity of soil organic matter transforming enzymes and the quantification of soilbound and free activity. Plant Soil 338, 99–110. doi: 10.1007/s11104-010-0296-3
Suri, V. K., Choudhary, A. K., Chander, G., and Verma, T. S. (2011). Influence of vesicular arbuscular mycorrhizal fungi and applied phosphorus on root colonization in wheat and plant nutrient dynamics in a phosphorus-deficient acid alfisol of Western Himalayas. Commun. Soil Sci. Plant Anal. 42, 1177–1186. doi: 10.1080/00103624.2011.566962
Syers, J. K., Johnston, A. E., and Curtin, D. (2008). Efficiency of Soil and Fertilizer Phosphorus Use—Reconciling Changing Concepts of Soil Phosphorus Behaviour With Agronomic Information. FAO Fertilizer and Plant Nutrition Bulletin 18. Rome: United Nations. Available online at: http://www.fao.org/docrep/010/a1595e/a1595e00.htm (Accessed July 02, 2016).
Sylvia, D. M., Hammond, L. C., Bennett, J. M., Haas, J. H., and Linda, S. B. (1993). Field response of maize to a VAM fungus and water management. Agron. J. 85, 193–198. doi: 10.2134/agronj1993.00021962008500020006x
Tabatabai, M. A. (1994). “Soil enzymes,” in Methods of Soil Analysis. Part 2. Microbiological and Biochemical Properties, eds R. W. Weaver, S. Angle, P. Bottomley, D. Bezdicek, S. Smith, A. Tabatabai, and A. Wollum (Madison, WI: Soil Science Society of America Journal), 775–833.
Talukdar, N. C., and Germida, J. J. (1994). Growth and yield of lentil and wheat inoculated with three Glomus isolates from Saskatchewan soils. Mycorrhiza 5, 145–152. doi: 10.1007/BF00202347
Tarafdar, J. C., and Marschner, H. (1994a). Phosphatase activity in the rhizosphere and hyphosphere of VA mycorrhizal wheat supplied with inorganic and organic phosphorus. Soil Biol. Biochem. 26, 387–395. doi: 10.1016/0038-0717(94)90288-7
Tarafdar, J. C., and Marschner, H. (1994b). Efficiency of vam hyphae in utilisation of organic phosphorus by wheat plants. Soil Sci. Plant Nutr. 40, 593–600. doi: 10.1080/00380768.1994.10414298
Tawaraya, K., Naito, M., and Wagatsuma, T. (2006). Solubilization of insoluble inorganic phosphate by hyphal exudates of arbuscular mycorrhizal fungi. J. Plant Nutr. 29, 657–665. doi: 10.1080/01904160600564428
Teng, W., Zhao, Y.-Y., Zhao, X.-Q., He, X., Ma, W.-Y., Deng, Y., et al. (2017). Genome-wide identification, characterization, and expression analysis of PHT1 phosphate transporters in wheat. Front. Plant Sci. 8, 1–14. doi: 10.3389/fpls.2017.00543
Theodorou, M. E., and Plaxton, W. C. (1996). Purification and characterization of pyrophosphate-dependent phosphofructokinase from phosphate-starved Brassica nigra suspension cells. Plant Physiol. 112, 343–351.
Tian, H., Yuan, X., Duan, J., Li, W., Zhai, B., and Gao, Y. (2017). Influence of nutrient signals and carbon allocation on the expression of phosphate and nitrogen transporter genes in winter wheat (Triticum aestivum L.) roots colonized by arbuscular mycorrhizal fungi. PLoS ONE 12:e0172154. doi: 10.1371/journal.pone.0172154
Trappe, J. M. (1987). “Phylogenetic and ecologic aspects of mycotrophy in the angiosperms from an evolutionary standpoint,” in Ecophysiology of VA Mycorrhizal Plants, ed G. R. Safir (Boca Raton, FL: CRC), 5–25.
Turner, B. L. (2007). “Inositol phosphates in soil: amounts, forms and significance of the phosphorylated inositol stereoisomers,” in Inositol Phosphates: Linking Agriculture and the Environment, eds B. L. Turner, A. E. Richardson and E. J. Mullaney (Wallingford: CABI Publishing), 186–206.
Unno, Y., Okubo, K., Wasaki, J., Shinano, T., and Osaki, M. (2005). Plant growth promotion abilities and microscale bacterial dynamics in the rhizosphere of Lupin analysed by phytate utilization ability. Environ. Microbiol. 7, 396–404. doi: 10.1111/j.1462-2920.2004.00701.x
Vacheron, J., Desbrosses, G., Bouffaud, M. L., Touraine, B., Moënne-Loccoz, Y., Muller, D., et al. (2013). Plant growth-promoting rhizobacteria and root system functioning. Front. Plant Sci. 4:356. doi: 10.3389/fpls.2013.00356
Vallino, M., Greppi, D., Novero, M., Bonfante, P., and Lupotto, E. (2009). Rice root colonisation by mycorrhizal and endophytic fungi in aerobic soil. Ann. Appl. Biol. 154, 195–204. doi: 10.1111/j.1744-7348.2008.00286.x
Vance, C. P., Uhde-Stone, C., and Allan, D. L. (2003). Phosphorus acquisition and use: Critical adaptations by plants for securing a nonrenewable resource. New Phytol. 157, 423–447. doi: 10.1046/j.1469-8137.2003.00695.x
Vandamme, E., Renkens, M., Pypers, P., Smolders, E., Vanlauwe, B., and Merckx, R. (2013). Root hairs explain P uptake efficiency of soybean genotypes grown in a P-deficient Ferralsol. Plant Soil 369, 269–282. doi: 10.1007/s11104-012-1571-2
van de Wiel, C. C. M., van der Linden, C. G., and Scholten, O. E. (2016). Improving phosphorus use efficiency in agriculture: opportunities for breeding. Euphytica 207, 1–22. doi: 10.1007/s10681-015-1572-3
Vaneklaas, E. J., Lambers, H., Bragg, J., Finnegan, P. M., Lovelock, C. E., Plaxton, W. C., et al. (2012). Opportunities for improving phosphorus-use efficiency in crop plants. New Phytol. 195, 306–320. doi: 10.1111/j.1469-8137.2012.04190.x
Wakelin, S. A., Warren, R. A., Harvey, P. R., and Ryder, M. H. (2004). Phosphate solubilization by Penicillium spp. Closely associated with wheat roots. Biol. Fert. Soils 40, 36–43. doi: 10.1007/s00374-004-0750-6
Wang, B., and Qiu, Y. L. (2006). Phylogenetic distribution and evolution of mycorrhizas in land plants. Mycorrhiza 16, 299–363. doi: 10.1007/s00572-005-0033-6
Wang, L., Liao, H., Yan, X., Zhuang, B., and Dong, Y. (2004). Genetic variability for root hair traits as related to phosphorus status in soybean. Plant Soil 261, 77–84. doi: 10.1023/B:PLSO.0000035552.94249.6a
Wang, X., Shen, J., and Liao, H. (2010). Acquisition or utilization, which is more critical for enhancing phosphorus efficiency in modern crops? Plant Sci. 179, 302–306. doi: 10.1016/j.plantsci.2010.06.007
Wang, X., Wang, Y., Tian, J., Lim, B. L., Yan, X., and Liao, H. (2009). Overexpressing AtPAP15 enhances phosphorus efficiency in soybean. Plant Physiol. 151, 233–240. doi: 10.1104/pp.109.138891
Wasaki, J., Maruyama, H., Tanaka, M., Yamamura, T., Dateki, H., Shinano, T., et al. (2009). Overexpression of the LaSAP2 gene for secretory acid phosphatase in white lupin improves the phosphorus uptake and growth of tobacco plants. Soil Sci. Plant Nut. 55, 107–113. doi: 10.1111/j.1747-0765.2008.00329.x
Williams, A., Manoharan, L., Rosenstock, N. P., Olsson, P. A., and Hedlund, K. (2017). Long-term agricultural fertilization alters arbuscular mycorrhizal fungal community composition and barley (Hordeum vulgare) mycorrhizal carbon and phosphorus exchange. New Phytol. 213, 874–885. doi: 10.1111/nph.14196
Wissuwa, M., Gamat, G., and Ismail, A. M. (2005). Is root growth under phosphorus deficiency affected by source or sink limitations? J. Exp. Bot. 417, 1943–1950. doi: 10.1093/jxb/eri189
Wu, F., Dong, M., Liu, Y., Ma, X., An, L., Young, J. P. W., et al. (2011). Effects of long-term fertilization on AM fungal community structure and Glomalin-related soil protein in the Loess Plateau of China. Plant Soil 342, 233–247. doi: 10.1007/s11104-010-0688-4
Xavier, L. J. C., and Germida, J. J. (1997). Growth response of lentil and wheat to Glomus clarum NT4 over a range of P levels in a Saskatchewan soil containing indigenous AM fungi. Mycorrhiza 7, 3–8. doi: 10.1007/s005720050156
Xu, X., Thornton, P. E., and Post, W. M. (2013). A global analysis of soil microbial biomass carbon, nitrogen and phosphorus in terrestrial ecosystems. Global Ecol. Biogeogr. 22, 737–749. doi: 10.1111/geb.12029
Yadav, R. S., and Tarafdar, J. C. (2003). Phytase of fungi in arid and semi-arid soils and their efficiency in hydrolysing different organic P compounds. Soil Biol. Biochem. 35, 1–7. doi: 10.1016/S0038-0717(03)00089-0
Yao, Q., Li, X. L., Feng, G., and Christie, P. (2001). Mobilization of sparingly soluble inorganic phosphates by the external mycelium of an arbuscular mycorrhizal fungus. Plant Soil 230, 279–285. doi: 10.1023/A:1010367501363
Ye, D., Zhang, X., Li, T., Xu, J., and Chen, G. (2018). Phosphorus-acquisition characteristics and rhizosphere properties of wild barley in relation to genotypic differences as dependent on soil phosphorus availability. Plant Soil 423, 503–516. doi: 10.1007/s11104-017-3530-4
Yoneyama, K., Xie, X., Kim, H. I., Kisugi, T., Nomura, T., Sekimoto, H., et al. (2012). How do nitrogen and phosphorus deficiencies affect strigolactone production and exudation? Planta 235, 1197–1207. doi: 10.1007/s00425-011-1568-8
Yoneyama, K., Xie, X., Kusumoto, D., Sekimoto, H., Sugimoto, Y., Takeuchi, Y., et al. (2007). Nitrogen deficiency as well as phosphorus deficiency in sorghum promotes the production and exudation of 5-deoxystrigol, the host recognition signal for arbuscular mycorrhizal fungi and root parasites. Planta 227, 125–132. doi: 10.1007/s00425-007-0600-5
Yousefi, A. A., Khavazi, K., Moezi, A. A., Rejali, F., and Nadian, H. A. (2011). Phosphate solubilizing bacteria and arbuscular mycorrhizal fungi impacts on inorganic phosphorus fractions and wheat growth. World Appl. Sci. J. 15, 1310–1318.
Zaidi, A., and Khan, M. S. (2005). Interactive effect of rhizotrophic microorganisms on growth, yield, and nutrient uptake of wheat. J. Plant Nutr. 28, 2079–2092. doi: 10.1080/01904160500320897
Zhang, Q., Blaylock, L. A., and Harrison, M. J. (2010). Two Medicago truncatula half-ABC transporters are essential for arbuscules development in arbuscular mycorrhizal symbiosis. Plant Cell 22, 1483–1497. doi: 10.1105/tpc.110.074955
Keywords: cereal, phosphorus, fungal diversity, mycorrhizae, nutrient uptake, PAE, root traits
Citation: Campos P, Borie F, Cornejo P, López-Ráez JA, López-García Á and Seguel A (2018) Phosphorus Acquisition Efficiency Related to Root Traits: Is Mycorrhizal Symbiosis a Key Factor to Wheat and Barley Cropping? Front. Plant Sci. 9:752. doi: 10.3389/fpls.2018.00752
Received: 12 January 2018; Accepted: 16 May 2018;
Published: 05 June 2018.
Edited by:
Jose M. Garcia-Mina, Universidad de Navarra, SpainReviewed by:
Raffaella Balestrini, Consiglio Nazionale Delle Ricerche (CNR), ItalyMonica Calvo-Polanco, Montpellier SupAgro, France
Copyright © 2018 Campos, Borie, Cornejo, López-Ráez, López-García and Seguel. This is an open-access article distributed under the terms of the Creative Commons Attribution License (CC BY). The use, distribution or reproduction in other forums is permitted, provided the original author(s) and the copyright owner are credited and that the original publication in this journal is cited, in accordance with accepted academic practice. No use, distribution or reproduction is permitted which does not comply with these terms.
*Correspondence: Juan A. López-Ráez, anVhbi5sb3BlenJhZXpAZWV6LmNzaWMuZXM=
Alex Seguel, YWxleC5zZWd1ZWxAdWZyb250ZXJhLmNs