- Instituto de Bioquímica Vegetal y Fotosíntesis, Consejo Superior de Investigaciones Científicas, Universidad de Sevilla, Seville, Spain
Light is probably the most important environmental stimulus for plant development. As sessile organisms, plants have developed regulatory mechanisms that allow the rapid adaptation of their metabolism to changes in light availability. Redox regulation based on disulfide-dithiol exchange constitutes a rapid and reversible post-translational modification, which affects protein conformation and activity. This regulatory mechanism was initially discovered in chloroplasts when it was identified that enzymes of the Calvin-Benson cycle (CBC) are reduced and active during the day and become rapidly inactivated by oxidation in the dark. At present, the large number of redox-sensitive proteins identified in chloroplasts extend redox regulation far beyond the CBC. The classic pathway of redox regulation in chloroplasts establishes that ferredoxin (Fdx) reduced by the photosynthetic electron transport chain fuels reducing equivalents to the large set of thioredoxins (Trxs) of this organelle via the activity of a Fdx-dependent Trx reductase (FTR), hence linking redox regulation to light. In addition, chloroplasts harbor an NADPH-dependent Trx reductase with a joint Trx domain, termed NTRC. The presence in chloroplasts of this NADPH-dependent redox system raises the question of the functional relationship between NTRC and the Fdx-FTR-Trx pathways. Here, we update the current knowledge of these two redox systems focusing on recent evidence showing their functional interrelationship through the action of the thiol-dependent peroxidase, 2-Cys peroxiredoxin (2-Cys Prx). The relevant role of 2-Cys Prxs in chloroplast redox homeostasis suggests that hydrogen peroxide may exert a key function to control the redox state of stromal enzymes. Indeed, recent reports have shown the participation of 2-Cys Prxs in enzyme oxidation in the dark, thus providing an explanation for the long-lasting question of photosynthesis deactivation during the light-dark transition.
Introduction
Photosynthesis is the process that allows the use of light energy for biomass production using water as source of reducing power; hence, chloroplasts have the function of providing the metabolic intermediates that support plant growth. These intermediates include molecules with signaling function such as hormones and hormone precursors, so that chloroplast performance also plays an important role in the harmonization of the growth of the different organs during all stages of plant development. Therefore, light is a key environmental factor for plant growth and development. Some of the changes in light availability, i.e., intensity and quality, vary in a predictable manner and plants, like other organisms, are able to anticipate these changes by the circadian clock. However, in nature, light availability changes continuously and, consequently, chloroplast performance needs to be rapidly adapted to these unpredictable conditions.
Central for the ability of chloroplast metabolism to rapidly respond to changes in light intensity is thiol-based redox regulation, which relies on the extraordinary properties of the thiol group of cysteines (Cremers and Jakob, 2013). Considering random distribution of amino acids in proteins, cysteine is underrepresented in all organisms though its presence appears to correlate with complexity ranging between 2.26% in mammals to 0.5% in some archaebacteria (Miseta and Csutora, 2000). The thiol group of cysteines is very sensitive to oxidant conditions, being able to react with hydrogen peroxide so that it may be oxidized as sulfenic (-SOH), sulfinic (-SO2H), and even sulfonic acid (-SO3H) (Cremers and Jakob, 2013). In its reduced form, cysteine may react with another cysteine forming a disulfide bridge. Cysteine residues involved in dithiol-disulfide redox exchange usually show a high degree of conservation in redox-sensitive proteins, the conformation and activity of which is deeply affected by the redox state of these pairs of cysteines.
Dithiol-disulfide exchange constitutes a universal regulatory mechanism present in all types of organisms from bacteria and fungi to plants and animals. The reduction of disulfide bridges in redox-regulated proteins relies on the protein disulfide reductase activity of thioredoxins (Trxs) and glutaredoxins (Grxs) (Meyer et al., 2012). However, most studies in different types of organisms have focused on the regulatory properties of Trxs, small polypeptides of 12–14 kDa with a well-conserved active site (WCGPC), which were initially described as cofactors of ribonucleotide reductase from Escherichia coli (Laurent et al., 1964). In heterotrophic organisms, the reducing power required for Trx activity is provided by NADPH via the action of an NADPH-dependent Trx reductase (NTR) (Jacquot et al., 2009; Figure 1). Interestingly, despite the large number of proteins that undergo redox regulation in heterotrophic organisms, the gene families encoding NTRs and Trxs is rather low, having typically two, at most three, members (Meyer et al., 2012). Redox regulation is also very important in plant chloroplasts; however, in these organelles, this regulatory mechanism shows remarkable differences as compared to heterotrophic organisms. In this review, we discuss the complex redox regulatory network of plant chloroplasts, focusing on the relevance of redox regulation for the rapid adaptation of chloroplast metabolism to light availability.
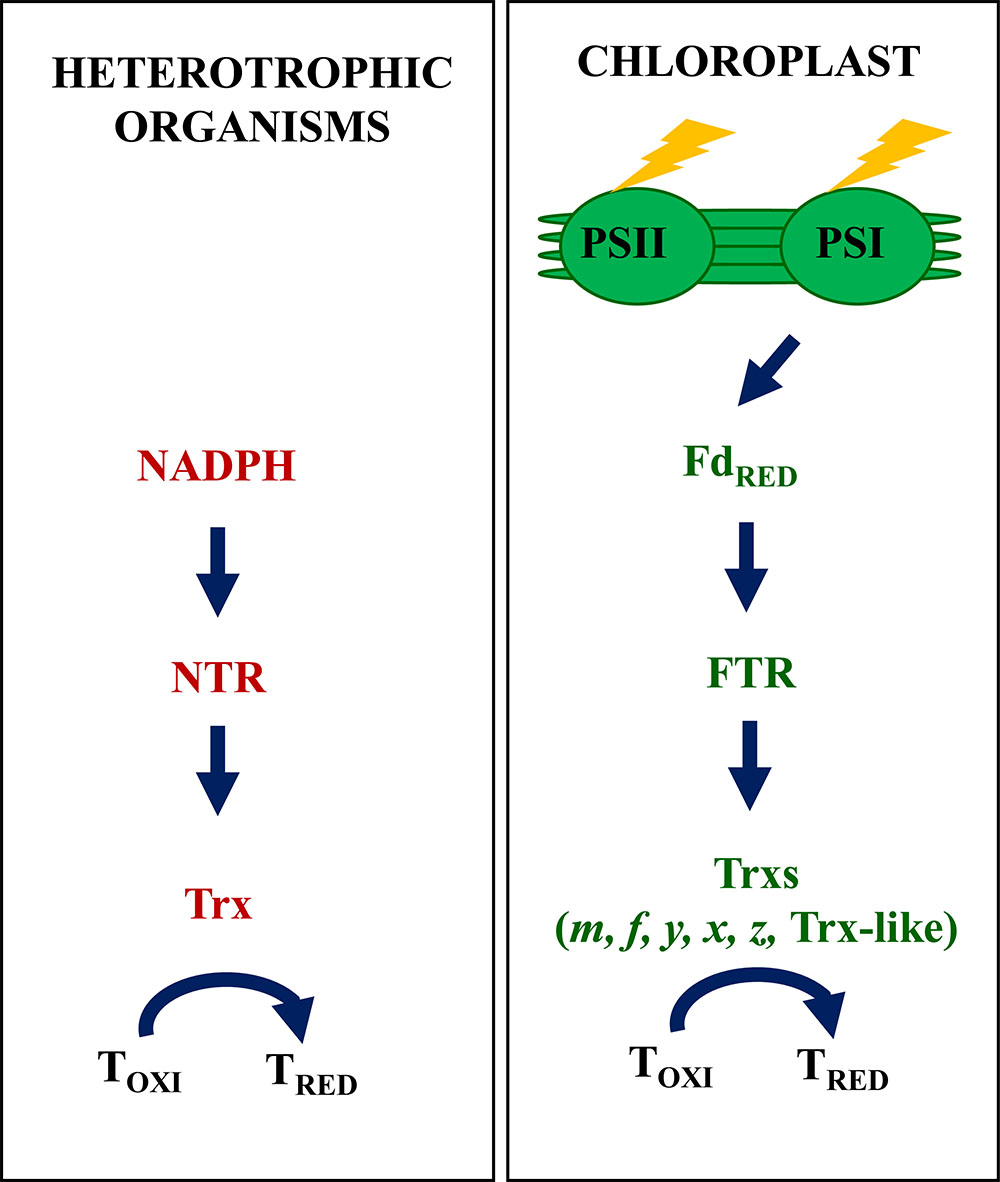
Figure 1. The classic view of redox regulatory pathways in heterotrophic organisms and plant chloroplasts. Regulation of enzyme activity based on the disulfide-dithiol exchange is a universal mechanism present in any type of organisms from bacteria and fungi to animals and plants. In heterotrophic organisms, this regulation is largely based in a two-component system. The disulfide reductase activity of Trx catalyzes the reduction of regulatory disulfides in target proteins (T), the required reducing power being provided by NADPH via the participation of NTR. In plant chloroplasts redox regulation is essential for the rapid adaptation of metabolism to ever changing light availability. Chloroplasts harbor a complex set of Trxs and Trx-like proteins, which rely on photo-reduced Fdx via the participation of FTR, thus linking redox regulation of enzyme activity to light. In addition, chloroplasts contain an additional redox system, NTRC, which relies on NADPH (see below, Figure 2).
Thiol-Dependent Redox Regulation in Plant Chloroplasts
Redox regulation of enzyme activity was first discovered by the seminal work of Prof. Buchanan at Berkeley (Buchanan, 2016). The initial key finding was that photo-reduced Fdx is required for the activation of the Calvin-Benson cycle (CBC) enzyme fructose bisphosphatase (FBPase) (Buchanan et al., 1967). Further analyses uncovered the participation of two components, Trx and an Fdx-dependent Trx reductase (FTR), which fuel reducing equivalents from photo-reduced Fdx for the reduction of a regulatory disulfide of FBPase, which rendered a more active form of the enzyme (Buchanan et al., 1971; Wolosiuk and Buchanan, 1977). Different approaches based on biochemical analyses soon identified two isoforms of Trxs in chloroplasts, f and m, named on their ability to preferentially activate FBPase and NADP-malate dehydrogenase, respectively (Buchanan, 2016). More recently, the enormous availability of genome sequence data from a large number of plant species has shown that chloroplasts harbor a complex set of up to twenty Trxs and Trx-like proteins (Buchanan et al., 2012; Meyer et al., 2012; Balsera et al., 2014; Geigenberger et al., 2017). In addition, mass spectrometry analyses in conjunction with techniques for trapping proteins interacting with Trxs have allowed the identification of a large number of putative Trx targets from chloroplasts (Montrichard et al., 2009). Although the redox regulation of many of these proteins awaits in vivo experimental confirmation, the number of putative Trx-interacting enzymes so far identified suggests that redox regulation may virtually affect any process occurring in the chloroplast, being thus a regulatory mechanism essential for the rapid adaptation of chloroplast performance to ever changing light conditions.
The classical view of redox regulation in chloroplasts, based on the FTR-Trx system, relies on Fdx reduced by the photosynthetic electron transport chain, which thus directly links redox regulation in the organelle to light (Schürmann and Buchanan, 2008). This is in clear contrast with heterotrophic organisms, where redox regulation relies on NADPH and the NTR-Trx redox system (Buchanan, 2017). Therefore, a major difference in redox regulation between chloroplasts and heterotrophic organisms is the source of reducing power used for this regulatory mechanism (Figure 1). This notion was modified by the discovery of NTRC, a novel NTR with a joint Trx domain at the C-terminus (Serrato et al., 2002, 2004). NTRC is exclusively found in organisms that perform oxygenic photosynthesis, namely plants, in which it is encoded by one or two genes, algae and some, but not all, cyanobacteria (Pascual et al., 2010; Nájera et al., 2017). In plants, NTRC shows localization in all types of plastids, either from photosynthetic or non-photosynthetic tissues (Kirchsteiger et al., 2012); however, it is a relatively abundant protein in chloroplasts where it shows stromal localization (Serrato et al., 2004; Moon et al., 2006; Pérez-Ruiz et al., 2009). Initial biochemical analyses of NTRC, based on the study of truncated polypeptides containing the NTR or the Trx domain of the enzyme, confirmed the NTR and Trx activities, respectively, of these domains (Serrato et al., 2004). Thus, based on these results, we suggested that NTRC is a bi-functional enzyme, which might function either as NTR or as Trx in the chloroplast. Soon afterward, it was reported that NTRC is able to efficiently reduce 2-Cys peroxiredoxins (2-Cys Prxs) (Moon et al., 2006; Pérez-Ruiz et al., 2006; Alkhalfioui et al., 2007). Indeed, the incubation of NTRC and 2-Cys Prx allows the rapid reduction of hydrogen peroxide using NADPH as source of reducing power, hence showing that the enzyme is able to conjugate both NTR and Trx activities for the efficient reduction of these thiol-peroxidases. Further biochemical analyses using mutated versions of the enzyme suggested that the catalytic active form of NTRC is a dimer arranged in a head-to-tail conformation, which interacts with 2-Cys Prxs through the Trx domain (Pérez-Ruiz and Cejudo, 2009; Bernal-Bayard et al., 2012). Overall, these evidences uncover the use of NADPH to support the antioxidant function of 2-Cys Prxs in chloroplasts (Pérez-Ruiz et al., 2006). It is known that 2-Cys Prxs, which are among the most abundant proteins of the chloroplast stroma (Peltier et al., 2006), show a very efficient hydrogen peroxide scavenging activity (Dietz, 2011; Perkins et al., 2015; Liebthal et al., 2018). In addition to NTRC, chloroplast Trxs and Trx-like proteins also show capacity of 2-Cys Prx reduction in vitro (Broin et al., 2002; Collin et al., 2003, 2004; Dangoor et al., 2012; Eliyahu et al., 2015; Hochmal et al., 2016; Vaseghi et al., 2018; Yoshida et al., 2018). In vitro assays comparing several chloroplast Trxs indicated that type-x Trx is the most efficient 2-Cys Prx reductant (Collin et al., 2003). The comparison of the in vivo redox state of 2-Cys Prxs in NTRC and Trx x Arabidopsis knock out mutants showed a severe impairment of the redox state of the 2-Cys Prxs in the ntrc mutant, whereas in the trxx mutant it was indistinguishable of the wild type (Pulido et al., 2010). Based on these results, it was proposed that NTRC is the most relevant reductant of 2-Cys Prxs in vivo. Because NTRC interacts with 2-Cys Prxs by the Trx domain, it could be considered that NTRC is a Trx that incorporates its own reductase, which would explain the high catalytic efficiency of this enzyme (Cejudo et al., 2012). However, the presence of an NTR domain in NTRC raises the question of whether this enzyme also enables the transfer of reducing equivalents from NADPH to plastidial Trxs. The overexpression of the NTR domain of NTRC in the Arabidopsis ntrc mutant partially recovered the wild type phenotype, suggesting that NTRC displays NTR activity and might interact with chloroplast Trxs, in particular with Trx f (Toivola et al., 2013). This notion, however, is questioned by the fact that NTRC is unable to reduce neither of the chloroplast Trxs in vitro (Bohrer et al., 2012). Therefore, whether NTRC interacts with its targets exclusively through the Trx domain, as it does with 2-Cys Prxs, or through the NTR domain, as it was proposed for Trx f, remains an open issue.
The Chloroplast Redox Systems NTRC and Fdx-FTR-Trx Act Concertedly
The identification of NTRC as an NADPH-dependent redox system established the presence of two redox pathways in chloroplasts, hence raising the question of the functional relationship between them. This issue is being addressed through reverse genetic approaches in Arabidopsis thaliana. The deficiency of different chloroplast Trxs results in a surprising low effect on plant growth. This is the case of knock out mutants for Trx x (Pulido et al., 2010) or Trxs y (Laugier et al., 2013), which show growth phenotypes very similar to the wild type. More intriguingly, despite the major role proposed for Trxs f in light-dependent redox regulation of CBC enzymes (Michelet et al., 2013), the double knockout mutant of Arabidopsis lacking Trxs f1 and f2 show almost wild type growth phenotype (Yoshida et al., 2015; Naranjo et al., 2016a). An in-depth analysis of the in vivo redox state of FBPase in this mutant revealed that the light-dependent redox regulation of the enzyme was only partially affected (Naranjo et al., 2016a), indicating that other chloroplast Trxs contribute to the redox regulation of FBPase. In this regard, it was shown that m-type Trxs have a relevant function in the light-dependent redox regulation of CBC enzymes (Okegawa and Motohashi, 2015). There are four isoforms of type m Trxs in Arabidopsis (Okegawa and Motohashi, 2015). Single mutants deficient in Trxs m1 and m4 show growth phenotypes similar to the wild type (Courteille et al., 2013; Laugier et al., 2013), though the deficiency of Trx m4 causes up-regulation of the NADH dehydrogenase-like complex-dependent plastoquinone reduction pathway of photosynthetic electron transport (Courteille et al., 2013). Notably, mutant plants devoid of Trx m3, the less abundant m-type Trx (Okegawa and Motohashi, 2015) showed unaffected chloroplast performance but impaired symplastic trafficking (Benítez-Alfonso et al., 2009). To our knowledge, no mutants completely devoid of the four m-type Trxs have been reported, however, approaches based on gene silencing generated Arabidopsis plants with very decreased contents of Trxs m1, m2 and m4, which allowed to propose a function of these m-type Trxs in photosystem II (PSII) biogenesis (Wang et al., 2013). Plants impaired in the expression of the variable subunit of FTR, which provides electrons to plastidial Trxs, display marked phenotype traits such as sensitivity to oxidative stress, impaired light-dependent reduction of Trx-regulated enzymes and increased contents of 2-Cys Prxs (Keryer et al., 2004). The latter trait giving further support to the tight relationship between FTR and NTRC redox systems. Finally, it should be mentioned that the deficiency of Trx z affects chloroplast transcription, thus compromising chloroplast biogenesis (Arsova et al., 2010). However, it is not clear whether the role of Trx z in the expression of plastid-encoded genes is redox-dependent (Wimmelbacher and Bornke, 2014).
In clear contrast, the Arabidopsis NTRC knockout mutant, ntrc, shows a characteristic phenotype consisting in retarded growth and pale green leaves, with about 70–75 % of the chlorophyll contents of the wild type plants (Serrato et al., 2004; Lepistö et al., 2009). Interestingly, the growth phenotype of the ntrc mutant is highly dependent on light availability; i.e., growth retard is aggravated when plants are grown under short-day conditions, as compared with long-day conditions (Pérez-Ruiz et al., 2006; Lepistö et al., 2009), and fluctuating light intensities (Thormählen et al., 2017). In addition, the Arabidopsis ntrc mutant shows high sensitivity to different abiotic stresses including high salt (Serrato et al., 2004), prolonged darkness (Pérez-Ruiz et al., 2006), and high temperature (Chae et al., 2013), as well as to biotic stress (Ishiga et al., 2012, 2016). As 2-Cys Prxs are very efficient hydrogen peroxide scavengers and NTRC is the most efficient reductant of these enzymes, the increased sensitivity of the ntrc mutant to biotic and abiotic stresses might be in agreement with the antioxidant function proposed for the enzyme. Therefore, the antioxidant function proposed for NTRC implies that NADPH serves as source of reducing power in chloroplasts, at least to support the hydrogen peroxide scavenging activity of 2-Cys Prxs (Spínola et al., 2008). This notion not only suggested a relevant role for NADPH in chloroplast redox homeostasis, it changes the paradigm of redox regulation linked to light, as NADPH is produced in chloroplasts from photo-reduced Fdx but also from sugars by the oxidative pentose phosphate pathway during darkness.
In summary, the approaches based on the analysis of Arabidopsis mutants show that while the deficiency of NTRC causes a severe effect on plant growth and development, the deficiency of different types of chloroplast Trxs, with the exception of Trx z, has low or no effect on plant growth, indicating the functional redundancy of these enzymes. Moreover, the fact that the absence of NTRC has such severe phenotype suggests the participation of this enzyme in different aspects of chloroplast redox homeostasis, besides its proposed antioxidant function as electron donor to 2-Cys Prxs. Key chloroplast metabolic pathways, such as chlorophyll and starch biosynthesis, and light energy utilization, which were known to be regulated by the canonical FTR/Trx pathway, are also affected by NTRC. A first indication of the effect of NTRC on redox-regulated processes was the finding that the pathway of chlorophyll biosynthesis is impaired in the ntrc mutant (Stenbaek et al., 2008). Initially, it was proposed that the positive effect of NTRC is exerted by the reduction of 2-Cys Prxs, which protects the aerobic cyclase activity of oxidant conditions. Further analyses revealed a direct role of NTRC on the redox regulation of the chlorophyll biosynthesis enzyme MgP methyltransferase (CHLM) and direct interaction of NTRC with CHLM and glutamyl-transfer RNA reductase1 (GluTR1) (Richter et al., 2013). Moreover, the content of both CHLM and GluTR enzymes were decreased in the ntrc mutant, suggesting that NTRC also affects the stability of these enzymes (Richter et al., 2013). In line with this observation, the I subunit (CHLI) of Mg chelatase, a redox-regulated enzyme that catalyzes the first dedicated step of chlorophyll biosynthesis (Ikegami et al., 2007), was shown to be regulated by NTRC (Pérez-Ruiz et al., 2014). Taken together, these results strongly suggest the participation of NTRC in the redox regulation of the chlorophyll biosynthesis pathway. In addition, NTRC participates in the redox regulation of starch biosynthesis (Michalska et al., 2009). A key regulatory step of this pathway is catalyzed by ADP glucose pyrophosphorylase (AGPase), a heterotetrameric enzyme formed by two small and two large subunits. Under illumination, AGPase is activated by the reduction of a disulfide bridge that links the two small subunits, a process that was described to be regulated by Trxs (Geigenberger, 2011). Indeed, Arabidopsis mutant plants deficient in Trx f1 show decreased light-dependent activation of AGPase (Thormählen et al., 2013). Interestingly, the Arabidopsis ntrc mutant shows decreased starch contents in leaves, and impaired reduction of AGPase in response to light (Michalska et al., 2009). Moreover, NTRC interacts and triggers the reduction of the enzyme in vitro, thus indicating the participation of NTRC in the light-dependent reductive activation of AGPase (Michalska et al., 2009), a notion further confirmed by the analysis of the regulation of starch metabolism in the ntrc mutant grown under short-day conditions (Lepistö et al., 2013). Altogether, these studies show that redox regulation of chlorophyll and starch biosynthesis, two key metabolic pathways previously shown to be regulated by Trxs, are also regulated by NTRC, indicating overlapping functions of the FTR-Trxs and NTRC redox systems in plant chloroplasts. Finally, the analysis of photochemical parameters revealed that mutant plants lacking NTRC show high non-photochemical quenching (NPQ) (Thormählen et al., 2015; Carrillo et al., 2016; Naranjo et al., 2016b). Although the ntrc mutant shows altered xanthophyll cycle (Naranjo et al., 2016b) and impaired reduction of the γ subunit ATP synthase under low irradiance, which causes higher lumen acidification and lower electron transport rate (Carrillo et al., 2016), the ultimate reason to explain how NTRC affects the efficiency of light energy utilization remains unknown. Nevertheless, these results reveal that NTRC does not only participate in the light-dependent redox regulation of metabolic pathways including the biosynthesis of starch and chlorophyll, it does also participate in the regulation of photochemical reactions such as NPQ. Therefore, the action of NTRC in chloroplast performance is exerted by the antioxidant function of the enzyme due to its capacity to reduce 2-Cys Prxs, but also on upstream photochemical reactions and on downstream redox-regulated targets (Figure 2).
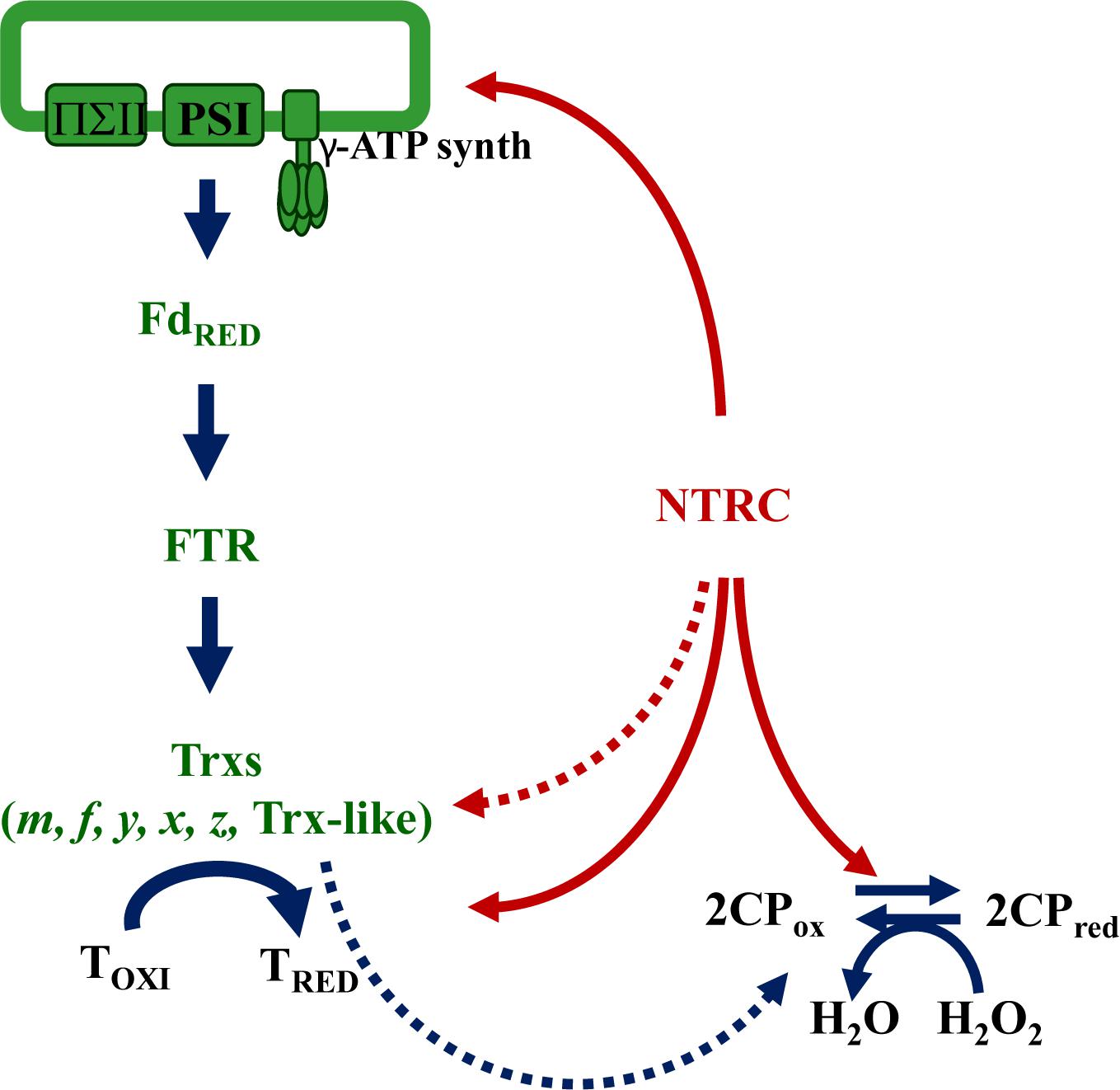
Figure 2. NTRC allows the use of NADPH in chloroplast redox homeostasis. NTRC is an efficient reductant of 2-Cys Prxs and, thus, allows the use of reducing equivalents from NADPH to support the hydrogen peroxide scavenging activity of these thiol-dependent peroxidases. Though at lower efficiency, chloroplast Trxs are also able to reduce 2-Cys Prxs. Different studies have shown the participation of NTRC in the upstream photochemical reactions of photosynthesis and the redox regulation of the γ subunit of ATP synthase (γ), and of downstream targets including enzymes of the Calvin-Benson cycle, starch and chlorophyll biosynthesis. Moreover, NTRC might display NTR activity reducing chloroplast Trxs such as Trx f.
A useful approach for understanding the functional relationship between the FTR-Trxs and NTRC redox systems was the analysis of Arabidopsis mutants simultaneously deficient in both pathways. While mutant plants knock out for Trx f1 (Thormählen et al., 2013), or Trxsf1 and f2 (Yoshida et al., 2015; Naranjo et al., 2016a) showed slight growth phenotype effects, the combined deficiencies of Trxs f and NTRC, the ntrc-trxf1f2 mutant, produced a very severe growth inhibition phenotype (Thormählen et al., 2015; Ojeda et al., 2017). The dramatic growth inhibition phenotype of these mutants is in agreement with the severe decrease of light energy utilization efficiency (Ojeda et al., 2017). Similarly, the lack of Trx x has almost no effect on plant growth (Pulido et al., 2010), whereas the simultaneous deficiency of Trx x and NTRC causes as well a dramatic effect of plant growth, much more severe than that of the trxx or ntrc single mutants (Ojeda et al., 2017). Interestingly, a remarkable feature of the ntrc-trxf1f2 and the ntrc-trxx mutants is the high mortality at the seedling stage (Ojeda et al., 2017), suggesting that chloroplast redox regulatory mechanisms are essential at the cotyledon-to-true leaf transition, which is a critical stage of plant development. The fact that the lack of chloroplast Trxs causes such a dramatic effect when combined with the lack of NTRC was further supported by the finding that mutant plants simultaneously devoid of NTRC and the catalytic subunit of FTR are inviable (Yoshida and Hisabori, 2016).
It has been reported that NTRC interacts with well-established Trx targets such as phosphoribulokinase (PRK) and FBPase (Nikkanen et al., 2016). Moreover, the analysis of the redox state of FBPase, a well-known redox-regulated enzyme of the CBC, showed decreased level of light-dependent reduction in the ntrc (Ojeda et al., 2017), and the trxf1f2 (Naranjo et al., 2016a) mutants. Intriguingly, the level of light-dependent reduction of FBPase in the trxx and the trxf1f2 mutants was very similar (Ojeda et al., 2017), despite the fact that Trx x is considered not involved in the redox regulation of enzymes of the CBC (Collin et al., 2003). The light-dependent redox reduction of FBPase is even more affected in the ntrc mutant, and essentially undetectable in mutant plants simultaneously lacking Trxs f or x and NTRC (Ojeda et al., 2017). Thus, these results support the notion that both Trxs (f and x) and NTRC concertedly participate in the redox regulation of FBPase. A possibility to explain the concerted action of the NTRC and the FTR-Trxs redox systems is that both have overlapping regulatory activity on the different redox regulated targets. Remarkably, in vitro assays with the purified proteins confirmed the high efficiency of Trxs f1 and f2, as compared with Trx x, in FBPase reduction, but revealed that NTRC is unable to reduce the enzyme, despite the fact that the light-dependent reduction of FBPase is severely affected in the ntrc mutant (Ojeda et al., 2017). Therefore, these studies show that the activity of NTRC is needed for the function of different chloroplast Trxs; though for the redox regulation of FBPase the effect of NTRC seems to be exerted indirectly.
The Central Role of 2-Cys Prxs: Integrating Antioxidant and Redox Regulatory Mechanisms
The puzzling question arising is how NTRC exerts such pleiotropic effects on different chloroplast redox regulated processes while, at least for the light-dependent redox regulation of FBPase, the effect is exerted without the direct interaction of both enzymes. The clarification of this conundrum came from the analysis of Arabidopsis lines combining NTRC and 2-Cys Prxs mutations. Although these plants are devoid of the redox regulatory function of NTRC, the phenotype of these mutants resembles that of the wild type (Pérez-Ruiz et al., 2017). This counterintuitive result indicated that the deficiency of 2-Cys Prxs exerts a suppressor effect of the ntrc mutant phenotype. Moreover, the overexpression of 2-Cys Prxs in the ntrc mutant background provoked the aggravation of the growth inhibition phenotype of these transgenic plants while the overexpression of the 2-Cys Prxs in the wild type background exerted no or low effect (Pérez-Ruiz et al., 2017). These results indicated that the increase of the content of 2-Cys Prxs becomes toxic for plant growth only when NTRC is not present. Although the absence of NTRC is expected to severely impair the antioxidant capacity of 2-Cys Prxs, it is important to take into account that, as stated above, different chloroplast Trxs are able to transfer reducing equivalents to 2-Cys Prxs, albeit much less efficiently than NTRC (Broin et al., 2002; Collin et al., 2003, 2004; Dangoor et al., 2012; Eliyahu et al., 2015; Hochmal et al., 2016; Vaseghi et al., 2018; Yoshida et al., 2018). Indeed, the light-dependent reduction of 2-Cys Prxs observed in the ntrc mutant (Pérez-Ruiz et al., 2017) suggests that these enzymes deplete reducing power from the pool of Trxs. Based on these results, it was hypothesized that in wild type plants the redox state of 2-Cys Prxs is maintained by NTRC, which is the most efficient reductant of the enzyme. Thus, the drainage of reducing equivalents from the other chloroplast Trxs would be very low and, consequently, the redox state of the pool of Trxs allows the light-dependent redox regulation of downstream targets (Figure 3A). In contrast, the ntrc mutant lacks the major source of reducing equivalents for 2-Cys Prxs, hence causing the accumulation of the oxidized form of these enzymes. Despite the lower efficiency of electron transfer of Trxs, the increased level of oxidized 2-Cys Prxs in plants lacking NTRC would provoke higher drainage of reducing equivalents from the pool of Trxs, compromising the light-dependent reduction of downstream targets (Figure 3B). Finally, in plants combining NTRC and 2-Cys Prx mutations, the decreased levels of 2-Cys Prxs would alleviate the drainage of reducing equivalents from the pool of Trxs, hence keeping sufficiently reduced the stromal Trxs for the light-dependent reduction of downstream targets, which would be thus the molecular basis of the suppression of the ntrc phenotype (Figure 3C). The analysis of the redox state of Trxs f, FBPase and PRK in the suppressed line fully confirmed this hypothesis hence uncovering the central function of 2-Cys Prxs in chloroplast redox regulation (Pérez-Ruiz et al., 2017). Moreover, the suppressed line also recovered wild type levels of chlorophyll biosynthesis enzymes (Richter et al., 2018), indicating that the suppressor effect caused by decreased levels of 2-Cys Prxs is exerted beyond the enzymes of the CBC. Based on these results, we proposed a model according to which the redox balance of 2-Cys Prxs, which is maintained by NTRC and NADPH, modulates the light-dependent reduction of redox regulated enzymes based on Fdx reduced by the photosynthetic electron transport chain, which is fueled to stromal Trxs via the action of FTR (Pérez-Ruiz et al., 2017). This model integrates the redox exchange of Trx and redox-regulated targets with hydrogen peroxide via the action of 2-Cys Prxs.
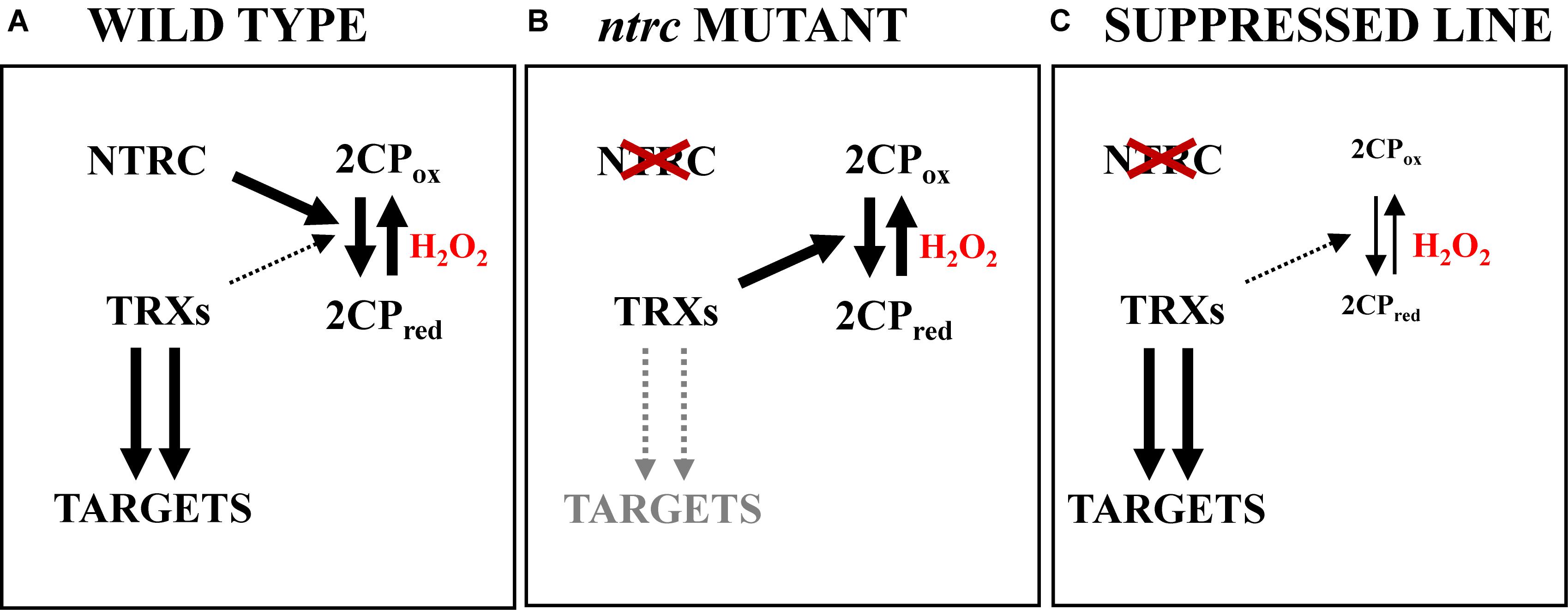
Figure 3. Low contents of 2-Cys Prxs exert a suppressor effect of the ntrc phenotype. (A) In chloroplasts from wild type plants, NTRC, which is the most efficient reductant of 2-Cys Prxs, maintains the redox state of these enzymes. Consequently, drainage of electron equivalents from the pool of Trxs is negligible and, thus, the redox state of Trxs is appropriate for the light-dependent reduction of redox-regulated targets. (B) In the ntrc mutant, the lack of the most efficient reductant of 2-Cys Prxs causes the imbalance of the redox state of these enzymes, which accumulate in oxidized form. Though Trxs transfer electrons to 2-Cys Prxs less efficiently than NTRC, the accumulation of the oxidized form of 2-Cys Prxs provokes a significant drainage of reducing equivalents from the pool of Trxs, thereby affecting the light-dependent reduction of downstream targets. (C) The suppressor effect is produced by decreased levels of 2-Cys Prxs. In the absence of NTRC, 2-Cys Prxs accumulate in oxidized form, however, as the amount of 2-Cys Prxs is low, the drainage of reducing equivalents from the pool of Trxs is also low, and hence the redox state of the pool of Trxs is appropriate for the reduction of downstream targets.
As mentioned above, the simultaneous lack of NTRC and Trx x or Trxs f cause dramatic loss of photosynthetic efficiency, light-dependent redox regulation of CBC enzymes and, consequently, severe growth inhibition phenotypes (Ojeda et al., 2017). Thus, to test the robustness of the function of the couple NTRC-2-Cys Prxs in chloroplast redox regulation, mutants plants deficient in NTRC, and Trxs x or f were combined with decreased contents of 2-Cys Prxs (Pérez-Ruiz et al., 2017; Ojeda et al., 2018b). Decreased contents of 2-Cys Prxs recovered growth phenotypes in plants lacking NTRC and Trx x (Ojeda et al., 2018b) or NTRC and Trxs f (Pérez-Ruiz et al., 2017), thus extending the suppressor effect of the deficiency of 2-Cys Prxs to the dramatic growth inhibition phenotypes caused by the simultaneous lack of NTRC and Trxs. Altogether, these results confirm the essential function of the NTRC-2-Cys Prxs redox couple in chloroplast performance and plant growth.
2-Cys Prxs Participate in Chloroplast Enzyme Oxidation in the Dark
The redox regulation of chloroplast enzymes in response to light was discovered in the sixties of the past century; since then, most studies have focused on the light-dependent enzyme activation by reduction, achieving a comprehensive knowledge of this regulatory mechanism (Buchanan, 2016). At the same time, it became clear that redox regulated enzymes of the CBC such as FBPase (Leegood and Walker, 1980) and glyceraldehyde phosphate dehydrogenase (GAPDH) (Sparla et al., 2002) were rapidly oxidized in the dark; however, the mechanism of enzyme oxidation has remained unknown. Our model of chloroplast redox regulation proposes a central role of 2-Cys Prxs in maintaining the redox state of the pool of Trxs that participate in the light-dependent redox regulation of chloroplast metabolic pathways including enzymes of the CBC (Pérez-Ruiz et al., 2017; Ojeda et al., 2018b) and the chlorophyll biosynthesis pathway (Richter et al., 2018). An important consequence of this model is that hydrogen peroxide acts as sink of reducing equivalents and, thus, could be an effective way of relieving reducing equivalents of the pool of Trxs in the dark, hence providing a possible explanation for the long-standing question of how chloroplast enzymes become oxidized in the night. Our group addressed this possibility by analyzing the redox state of well-studied redox-regulated enzymes, FBPase, GAPDH and the γ subunit of ATPase in the Arabidopsis 2cpab mutant, which is knock out for 2-Cys Prxs A and B (Ojeda et al., 2018a). The enzymes under analysis were detected almost fully reduced in both wild type and 2cpab mutant plants adapted to light. While in the wild type these enzymes were rapidly oxidized in the dark, oxidation was significantly delayed in the 2cpab mutant, indicating the participation of 2-Cys Prxs in the short-term oxidation of chloroplast enzymes in the dark (Ojeda et al., 2018a). Independently, Vaseghi et al. (2018) reported the participation of 2-Cys Prxs in the oxidation of reductively activated chloroplast enzymes FBPase, PRK, and NADPH-dependent malate dehydrogenase (MDH) and showed that oxidation was compromised in Arabidopsis mutant plants devoid of 2-Cys Prxs. The analysis of the oxidative activity of the Trx-like2 (Trx-L2) protein in vitro, in conjunction with the use of a severe 2-Cys Prxs knock down mutant of Arabidopsis led Hisabori’s group to propose the participation of an oxidant pathway formed by Trx-L2/2-Cys Prxs in enzyme oxidation in the dark (Yoshida et al., 2018). The identification of the function of 2-Cys Prxs in the oxidation of thiol groups is in line with the previous proposal of the oxidizing activity of this enzyme in response to moderate light intensity (Dangoor et al., 2012) and for oxidation of the small subunit of AGPase (Eliyahu et al., 2015).
Therefore, after decades of research focused on the mechanisms leading to enzyme activation in the light, three independent studies (Ojeda et al., 2018a; Vaseghi et al., 2018; Yoshida et al., 2018) identified the participation of 2-Cys Prxs in enzyme oxidation in the dark. The characterization of the 2-Cys Prx interactome revealed the interaction of 2-Cys Prxs with a large number of chloroplast proteins including FBPase (Cerveau et al., 2016). Therefore, a possibility to be taken into account is that FBPase oxidation occurs by the direct transfer of electrons from the enzyme to 2-Cys Prxs. However, studies performed in vitro with recombinant enzymes (Ojeda et al., 2018a; Yoshida et al., 2018) or with extracts from chloroplast stroma (Vaseghi et al., 2018) showed that reduced FBPase was not directly oxidized in the presence of 2-Cys Prx or Trxs. In contrast, the addition of 2-Cys Prxs and Trxs resulted in FBPase oxidation (Ojeda et al., 2018a), indicating the participation of these Trxs in the process of oxidation. This notion was further supported by Vaseghi et al. (2018), who determined the MDH oxidation efficiency by different Trxs, including canonical m- f- and x-types and Trx-like proteins such as chloroplast drought-induced stress protein of 32 kDa (CDSP32). Finally, it was shown that Trx-L2, which has the less negative reducing potential and is unable to reduce FBPase, is the most efficient chloroplast Trx for transfer of reducing equivalents of reduced enzymes to 2-Cys Prxs and H2O2 (Yoshida et al., 2018). Altogether, these studies proposed a pathway of oxidation involving Trxs, which would act as intermediates between the reduced targets and 2-Cys Prxs (Figure 4). This finding is an important advance in the current understanding of the control of chloroplast photosynthetic metabolism by light and darkness. However, it should be noted that, albeit delayed, enzyme oxidation still takes place in mutant plants lacking 2-Cys Prxs, indicating the participation of additional mechanism(s) in the process of enzyme oxidation in the dark. In search of these additional mechanisms, our group tested the participation of Prx Q and Prx IIE, monomeric Prxs present in Arabidopsis chloroplasts (Dietz, 2011). However, Arabidopsis triple mutants combining the lack of 2-Cys Prxs with severely decreased levels of either Prx Q or Prx IIE showed similar growth phenotype and rates of enzyme oxidation in the dark than the 2cpab mutant, suggesting that these Prxs have no relevant function in enzyme oxidation (Ojeda et al., 2018a).
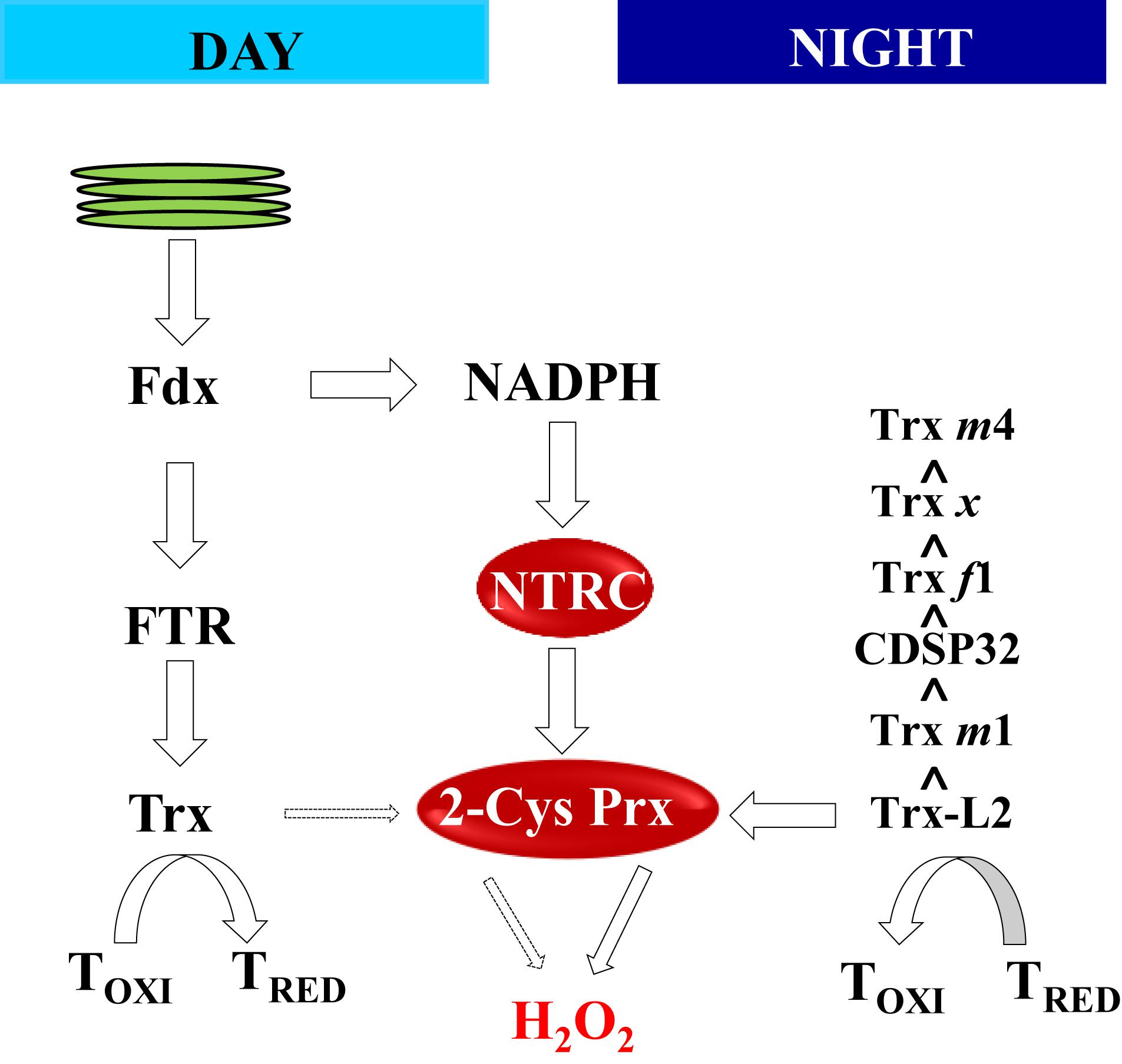
Figure 4. The NTRC-2-Cys Prx couple controls the reduction/oxidation balance of redox-regulated targets in the day and in the night. During the day, photo-reduced Fdx fuels reducing equivalents via FTR and Trxs for the reduction (activation) of redox-regulated enzymes. The redox state of the pool of Trxs is maintained by the couple NTRC-2-Cys Prxs, which relies on NADPH as source of reducing power. In the dark, the input of reducing equivalents via reduced Fdx ceases and Trxs mediate the oxidation of reduced targets transferring electrons through the activity of 2-Cys Prxs to hydrogen peroxide, which acts as final sink of electrons. The oxidant efficiency of different Trxs is presented as proposed by Yoshida et al. (2018) and Vaseghi et al. (2018).
Concluding Remarks and Future Prospect
While redox regulation in heterotrophic organisms involves a low number of Trxs and NTRs, and depends on NADPH, redox regulation in chloroplasts involves two redox systems: NTRC, which relies on NADPH, and the FTR-Trxs system, in which a large set of Trxs relies on photo-reduced Fdx. Therefore, redox regulation in chloroplasts is much more complex than in heterotrophic organisms, probably reflecting the sessile life style of plants and their necessity to rapidly respond to natural fluctuations in light. A recent report has identified the central role of 2-Cys Prxs integrating disulfide-dithiol exchange of redox-regulated enzymes and hydrogen peroxide detoxification (Pérez-Ruiz et al., 2017). Moreover, the participation of 2-Cys Prxs in the short-term oxidation of chloroplast enzymes in the dark has been recently reported (Ojeda et al., 2018a; Vaseghi et al., 2018; Yoshida et al., 2018), thus starting to solve the long-lasting question of the mechanism that allows the rapid oxidation of chloroplast enzymes in the night (Jacquot, 2018). Nevertheless, the participation of additional mechanism(s), yet to be identified, as well as the role of chloroplast Trxs in the oxidation process need to be clarified. Finally, the function of NTRC could be exclusively the maintenance of the redox balance of 2-Cys Prxs for proper chloroplast function, however whether this enzyme hase additional regulatory functions deserves further attention in next few years.
Author Contributions
FJC designed and wrote the initial version of the manuscript. VO, VD-R, MG, and JMP-R contributed to the final version.
Funding
Work in our laboratory was supported by European Regional Development Fund-co-financed grant (BIO2017-85195-C2-1-P) from the Spanish Ministry of Economy, Industry, and Competiveness (MINECO) and BIO-182 from Junta de Andalucía, Spain.
Conflict of Interest Statement
The authors declare that the research was conducted in the absence of any commercial or financial relationships that could be construed as a potential conflict of interest.
References
Alkhalfioui, F., Renard, M., and Montrichard, F. (2007). Unique properties of NADP-thioredoxin reductase C in legumes. J. Exp. Bot. 58, 969–978. doi: 10.1093/jxb/erl248
Arsova, B., Hoja, U., Wimmelbacher, M., Greiner, E., Ustun, S., Melzer, M., et al. (2010). Plastidial thioredoxin z interacts with two fructokinase-like proteins in a thiol-dependent manner: evidence for an essential role in chloroplast development in Arabidopsis and Nicotiana benthamiana. Plant Cell 22, 1498–1515. doi: 10.1105/tpc.109.071001
Balsera, M., Uberegui, E., Schürmann, P., and Buchanan, B. B. (2014). Evolutionary development of redox regulation in chloroplasts. Antioxid. Redox Signal. 21, 1327–1355. doi: 10.1089/ars.2013.5817
Benítez-Alfonso, Y., Cilia, M., San Roman, A., Thomas, C., Maule, A., Hearn, S., et al. (2009). Control of Arabidopsis meristem development by thioredoxin-dependent regulation of intercellular transport. Proc. Natl. Acad. Sci. U.S.A. 106, 3615–3620. doi: 10.1073/pnas.0808717106
Bernal-Bayard, P., Hervás, M., Cejudo, F. J., and Navarro, J. A. (2012). Electron transfer pathways and dynamics of chloroplast NADPH-dependent thioredoxin reductase C (NTRC). J. Biol. Chem. 287, 33865–33872. doi: 10.1074/jbc.M112.388991
Bohrer, A. S., Massot, V., Innocenti, G., Reichheld, J. P., Issakidis-Bourguet, E., and Vanacker, H. (2012). New insights into the reduction systems of plastidial thioredoxins point out the unique properties of thioredoxin z from Arabidopsis. J. Exp. Bot. 63, 6315–6323. doi: 10.1093/jxb/ers283
Broin, M., Cuine, S., Eymery, F., and Rey, P. (2002). The plastidic 2-cysteine peroxiredoxin is a target for a thioredoxin involved in the protection of the photosynthetic apparatus against oxidative damage. Plant Cell 14, 1417–1432. doi: 10.1105/tpc.001644
Buchanan, B. B. (2016). The path to thioredoxin and redox regulation in chloroplasts. Annu. Rev. Plant Biol. 67, 1–24. doi: 10.1146/annurev-arplant-043015-111949
Buchanan, B. B. (2017). The path to thioredoxin and redox regulation beyond chloroplasts. Plant Cell Physiol. 58, 1826–1832. doi: 10.1093/pcp/pcx119
Buchanan, B. B., Holmgren, A., Jacquot, J. P., and Scheibe, R. (2012). Fifty years in the thioredoxin field and a bountiful harvest. Biochim. Biophys. Acta 1820, 1822–1829. doi: 10.1016/j.bbagen.2012.07.006
Buchanan, B. B., Kalberer, P. P., and Arnon, D. I. (1967). Ferredoxin-activated fructose diphosphatase in isolated chloroplasts. Biochem. Biophys. Res. Commun. 29, 74–79. doi: 10.1016/0006-291X(67)90543-8
Buchanan, B. B., Schurmann, P., and Kalberer, P. P. (1971). Ferredoxin-activated fructose diphosphatase of spinach chloroplasts. Resolution of the system, properties of the alkaline fructose diphosphatase component, and physiological significance of the ferredoxin-linked activation. J. Biol. Chem. 246, 5952–5959.
Carrillo, L. R., Froehlich, J. E., Cruz, J. A., Savage, L., and Kramer, D. M. (2016). Multi-level regulation of the chloroplast ATP synthase: the chloroplast NADPH thioredoxin reductase C (NTRC) is required for redox modulation specifically under low irradiance. Plant J. 87, 654–663. doi: 10.1111/tpj.13226
Cejudo, F. J., Ferrández, J., Cano, B., Puerto-Galán, L., and Guinea, M. (2012). The function of the NADPH thioredoxin reductase C-2-Cys peroxiredoxin system in plastid redox regulation and signalling. FEBS Lett. 586, 2974–2980. doi: 10.1016/j.febslet.2012.07.003
Cerveau, D., Kraut, A., Stotz, H. U., Mueller, M. J., Couté, Y., and Rey, P. (2016). Characterization of the Arabidopsis thaliana 2-Cys peroxiredoxin interactome. Plant Sci. 252, 30–41. doi: 10.1016/j.plantsci.2016.07.003
Chae, H. B., Moon, J. C., Shin, M. R., Chi, Y. H., Jung, Y. J., Lee, S. Y., et al. (2013). Thioredoxin reductase type C (NTRC) orchestrates enhanced thermotolerance to Arabidopsis by its redox-dependent holdase chaperone function. Mol. Plant 6, 323–336. doi: 10.1093/mp/sss105
Collin, V., Issakidis-Bourguet, E., Marchand, C., Hirasawa, M., Lancelin, J. M., Knaff, D. B., et al. (2003). The Arabidopsis plastidial thioredoxins: new functions and new insights into specificity. J. Biol. Chem. 278, 23747–23752. doi: 10.1074/jbc.M302077200
Collin, V., Lamkemeyer, P., Miginiac-Maslow, M., Hirasawa, M., Knaff, D. B., Dietz, K. J., et al. (2004). Characterization of plastidial thioredoxins from Arabidopsis belonging to the new y-type. Plant Physiol. 136, 4088–4095. doi: 10.1104/pp.104.052233
Courteille, A., Vesa, S., Sanz-Barrio, R., Cazale, A. C., Becuwe-Linka, N., Farran, I., et al. (2013). Thioredoxin m4 controls photosynthetic alternative electron pathways in Arabidopsis. Plant Physiol. 161, 508–520. doi: 10.1104/pp.112.207019
Cremers, C. M., and Jakob, U. (2013). Oxidant sensing by reversible disulfide bond formation. J. Biol. Chem. 288, 26489–26496. doi: 10.1074/jbc.R113.462929
Dangoor, I., Peled-Zehavi, H., Wittenberg, G., and Danon, A. (2012). A chloroplast light-regulated oxidative sensor for moderate light intensity in Arabidopsis. Plant Cell 24, 1894–1906. doi: 10.1105/tpc.112.097139
Dietz, K. J. (2011). Peroxiredoxins in plants and cyanobacteria. Antioxid. Redox Signal. 15, 1129–1159. doi: 10.1089/ars.2010.3657
Eliyahu, E., Rog, I., Inbal, D., and Danon, A. (2015). ACHT4-driven oxidation of APS1 attenuates starch synthesis under low light intensity in Arabidopsis plants. Proc. Natl. Acad. Sci. U.S.A. 112, 12876–12881. doi: 10.1073/pnas.1515513112
Geigenberger, P. (2011). Regulation of starch biosynthesis in response to a fluctuating environment. Plant Physiol. 155, 1566–1577. doi: 10.1104/pp.110.170399
Geigenberger, P., Thormählen, I., Daloso, D. M., and Fernie, A. R. (2017). The unprecedented versatility of the plant thioredoxin system. Trends Plant Sci. 22, 249–262. doi: 10.1016/j.tplants.2016.12.008
Hochmal, A. K., Zinzius, K., Charoenwattanasatien, R., Gabelein, P., Mutoh, R., Tanaka, H., et al. (2016). Calredoxin represents a novel type of calcium-dependent sensor-responder connected to redox regulation in the chloroplast. Nat. Commun. 7:11847. doi: 10.1038/ncomms11847
Ikegami, A., Yoshimura, N., Motohashi, K., Takahashi, S., Romano, P. G., Hisabori, T., et al. (2007). The CHLI1 subunit of Arabidopsis thaliana magnesium chelatase is a target protein of the chloroplast thioredoxin. J. Biol. Chem. 282, 19282–19291. doi: 10.1074/jbc.M703324200
Ishiga, Y., Ishiga, T., Ikeda, Y., Matsuura, T., and Mysore, K. S. (2016). NADPH-dependent thioredoxin reductase C plays a role in nonhost disease resistance against Pseudomonas syringae pathogens by regulating chloroplast-generated reactive oxygen species. PeerJ 4:e1938. doi: 10.7717/peerj.1938
Ishiga, Y., Ishiga, T., Wangdi, T., Mysore, K. S., and Uppalapati, S. R. (2012). NTRC and chloroplast-generated reactive oxygen species regulate Pseudomonas syringae pv. tomato disease development in tomato and Arabidopsis. Mol. Plant Microbe Interact. 25, 294–306. doi: 10.1094/MPMI-05-11-0130
Jacquot, J. P. (2018). Dark deactivation of chloroplast enzymes finally comes to light. Proc. Natl. Acad. Sci. U.S.A. 115, 9334–9335. doi: 10.1073/pnas.1814182115
Jacquot, J. P., Eklund, H., Rouhier, N., and Schürmann, P. (2009). Structural and evolutionary aspects of thioredoxin reductases in photosynthetic organisms. Trends Plant Sci. 14, 336–343. doi: 10.1016/j.tplants.2009.03.005
Keryer, E., Collin, V., Lavergne, D., Lemaire, S., and Issaakidis-Bourguet, E. (2004). Characterization of Arabidopsis mutants for the variable subunit of ferredoxin:thioredoxin reductase. Photosynth. Res. 79, 265–274. doi: 10.1023/B:PRES.0000017173.46185.3e
Kirchsteiger, K., Ferrández, J., Pascual, M. B., González, M., and Cejudo, F. J. (2012). NADPH thioredoxin reductase C is localized in plastids of photosynthetic and nonphotosynthetic tissues and is involved in lateral root formation in Arabidopsis. Plant Cell 24, 1534–1548. doi: 10.1105/tpc.111.092304
Laugier, E., Tarrago, L., Courteille, A., Innocenti, G., Eymery, F., Rumeau, D., et al. (2013). Involvement of thioredoxin y2 in the preservation of leaf methionine sulfoxide reductase capacity and growth under high light. Plant Cell Environ. 36, 670–682. doi: 10.1111/pce.12005
Laurent, T. C., Moore, E. C., and Reichard, P. (1964). Enzymatic synthesis of deoxyribonucleotides. IV. Isolation and characterization of thioredoxin, the hydrogen donor from Escherichia coli B. J. Biol. Chem. 239, 3436–3444.
Leegood, R. C., and Walker, D. A. (1980). Regulation of fructose-1,6-bisphosphatase activity in intact chloroplasts. Studies of the mechanism of inactivation. Biochim. Biophys. Acta 593, 362–370. doi: 10.1016/0005-2728(80)90073-0
Lepistö, A., Kangasjarvi, S., Luomala, E. M., Brader, G., Sipari, N., Keranen, M., et al. (2009). Chloroplast NADPH-thioredoxin reductase interacts with photoperiodic development in Arabidopsis. Plant Physiol. 149, 1261–1276. doi: 10.1104/pp.108.133777
Lepistö, A., Pakula, E., Toivola, J., Krieger-Liszkay, A., Vignols, F., and Rintamaki, E. (2013). Deletion of chloroplast NADPH-dependent thioredoxin reductase results in inability to regulate starch synthesis and causes stunted growth under short-day photoperiods. J. Exp. Bot. 64, 3843–3854. doi: 10.1093/jxb/ert216
Liebthal, M., Maynard, D., and Dietz, K. J. (2018). Peroxiredoxins and redox signaling in plants. Antioxid. Redox Signal. 28, 609–624. doi: 10.1089/ars.2017.7164
Meyer, Y., Belin, C., Delorme-Hinoux, V., Reichheld, J. P., and Riondet, C. (2012). Thioredoxin and glutaredoxin systems in plants: molecular mechanisms, crosstalks, and functional significance. Antioxid. Redox Signal. 17, 1124–1160. doi: 10.1089/ars.2011.4327
Michalska, J., Zauber, H., Buchanan, B. B., Cejudo, F. J., and Geigenberger, P. (2009). NTRC links built-in thioredoxin to light and sucrose in regulating starch synthesis in chloroplasts and amyloplasts. Proc. Natl. Acad. Sci. U.S.A. 106, 9908–9913. doi: 10.1073/pnas.0903559106
Michelet, L., Zaffagnini, M., Morisse, S., Sparla, F., Pérez-Peérez, M. E., Francia, F., et al. (2013). Redox regulation of the Calvin-Benson cycle: something old, something new. Front. Plant Sci. 4:470. doi: 10.3389/fpls.2013.00470
Miseta, A., and Csutora, P. (2000). Relationship between the occurrence of cysteine in proteins and the complexity of organisms. Mol. Biol. Evol. 17, 1232–1239. doi: 10.1093/oxfordjournals.molbev.a026406
Montrichard, F., Alkhalfioui, F., Yano, H., Vensel, W. H., Hurkman, W. J., and Buchanan, B. B. (2009). Thioredoxin targets in plants: the first 30 years. J. Proteom. 72, 452–474. doi: 10.1016/j.jprot.2008.12.002
Moon, J. C., Jang, H. H., Chae, H. B., Lee, J. R., Lee, S. Y., Jung, Y. J., et al. (2006). The C-type Arabidopsis thioredoxin reductase ANTR-C acts as an electron donor to 2-Cys peroxiredoxins in chloroplasts. Biochem. Biophys. Res. Commun. 348, 478–484. doi: 10.1016/j.bbrc.2006.07.088
Nájera, V. A., González, M. C., Pérez-Ruiz, J. M., and Cejudo, F. J. (2017). An event of alternative splicing affects the expression of the NTRC gene, encoding NADPH-thioredoxin reductase C, in seed plants. Plant Sci. 258, 21–28. doi: 10.1016/j.plantsci.2017.02.001
Naranjo, B., Díaz-Espejo, A., Lindahl, M., and Cejudo, F. J. (2016a). Type-f thioredoxins have a role in the short-term activation of carbon metabolism and their loss affects growth under short-day conditions in Arabidopsis thaliana. J. Exp. Bot. 67, 1951–1964. doi: 10.1093/jxb/erw017
Naranjo, B., Mignee, C., Krieger-Liszkay, A., Hornero-Méndez, D., Gallardo-Guerrero, L., Cejudo, F. J., et al. (2016b). The chloroplast NADPH thioredoxin reductase C, NTRC, controls non-photochemical quenching of light energy and photosynthetic electron transport in Arabidopsis. Plant Cell Environ. 39, 804–822. doi: 10.1111/pce.12652
Nikkanen, L., Toivola, J., and Rintamaki, E. (2016). Crosstalk between chloroplast thioredoxin systems in regulation of photosynthesis. Plant Cell Environ. 39, 1691–1705. doi: 10.1111/pce.12718
Ojeda, V., Pérez-Ruiz, J. M., and Cejudo, F. J. (2018a). 2-Cys Peroxiredoxins participate in the oxidation of chloroplast enzymes in the dark. Mol. Plant 11, 1377–1388. doi: 10.1016/j.molp.2018.09.005
Ojeda, V., Pérez-Ruiz, J. M., and Cejudo, F. J. (2018b). The NADPH-dependent thioredoxin reductase C-2-Cys peroxiredoxin redox system modulates the activity of thioredoxin x in Arabidopsis chloroplasts. Plant Cell Physiol. 59, 2155–2164. doi: 10.1093/pcp/pcy134
Ojeda, V., Pérez-Ruiz, J. M., González, M. C., Nájera, V. A., Sahrawy, M., Serrato, A. J., et al. (2017). NADPH thioredoxin reductase C and thioredoxins act concertedly in seedling development. Plant Physiol. 174, 1436–1448. doi: 10.1104/pp.17.00481
Okegawa, Y., and Motohashi, K. (2015). Chloroplastic thioredoxin m functions as a major regulator of Calvin cycle enzymes during photosynthesis in vivo. Plant J. 84, 900–913. doi: 10.1111/tpj.13049
Pascual, M. B., Mata-Cabana, A., Florencio, F. J., Lindahl, M., and Cejudo, F. J. (2010). Overoxidation of 2-Cys peroxiredoxin in prokaryotes: cyanobacterial 2-Cys peroxiredoxins sensitive to oxidative stress. J. Biol. Chem. 285, 34485–34492. doi: 10.1074/jbc.M110.160465
Peltier, J. B., Cai, Y., Sun, Q., Zabrouskov, V., Giacomelli, L., Rudella, A., et al. (2006). The oligomeric stromal proteome of Arabidopsis thaliana chloroplasts. Mol. Cell. Proteomics 5, 114–133. doi: 10.1074/mcp.M500180-MCP200
Pérez-Ruiz, J. M., and Cejudo, F. J. (2009). A proposed reaction mechanism for rice NADPH thioredoxin reductase C, an enzyme with protein disulfide reductase activity. FEBS Lett. 583, 1399–1402. doi: 10.1016/j.febslet.2009.03.067
Pérez-Ruiz, J. M., González, M., Spínola, M. C., Sandalio, L. M., and Cejudo, F. J. (2009). The quaternary structure of NADPH thioredoxin reductase C is redox-sensitive. Mol. Plant 2, 457–467. doi: 10.1093/mp/ssp011
Pérez-Ruiz, J. M., Guinea, M., Puerto-Galán, L., and Cejudo, F. J. (2014). NADPH thioredoxin reductase C is involved in redox regulation of the Mg-chelatase I subunit in Arabidopsis thaliana chloroplasts. Mol. Plant 7, 1252–1255. doi: 10.1093/mp/ssu032
Pérez-Ruiz, J. M., Naranjo, B., Ojeda, V., Guinea, M., and Cejudo, F. J. (2017). NTRC-dependent redox balance of 2-Cys peroxiredoxins is needed for optimal function of the photosynthetic apparatus. Proc. Natl. Acad. Sci. U.S.A. 114, 12069–12074. doi: 10.1073/pnas.1706003114
Pérez-Ruiz, J. M., Spínola, M. C., Kirchsteiger, K., Moreno, J., Sahrawy, M., and Cejudo, F. J. (2006). Rice NTRC is a high-efficiency redox system for chloroplast protection against oxidative damage. Plant Cell 18, 2356–2368. doi: 10.1105/tpc.106.041541
Perkins, A., Nelson, K. J., Parsonage, D., Poole, L. B., and Karplus, P. A. (2015). Peroxiredoxins: guardians against oxidative stress and modulators of peroxide signaling. Trends Biochem. Sci. 40, 435–445. doi: 10.1016/j.tibs.2015.05.001
Pulido, P., Spínola, M. C., Kirchsteiger, K., Guinea, M., Pascual, M. B., Sahrawy, M., et al. (2010). Functional analysis of the pathways for 2-Cys peroxiredoxin reduction in Arabidopsis thaliana chloroplasts. J. Exp. Bot. 61, 4043–4054. doi: 10.1093/jxb/erq218
Richter, A. S., Pérez-Ruiz, J. M., Cejudo, F. J., and Grimm, B. (2018). Redox-control of chlorophyll biosynthesis mainly depends on thioredoxins. FEBS Lett. 592, 3111–3115. doi: 10.1002/1873-3468.13216
Richter, A. S., Peter, E., Rothbart, M., Schlicke, H., Toivola, J., Rintamaki, E., et al. (2013). Posttranslational influence of NADPH-dependent thioredoxin reductase C on enzymes in tetrapyrrole synthesis. Plant Physiol. 162, 63–73. doi: 10.1104/pp.113.217141
Schürmann, P., and Buchanan, B. B. (2008). The ferredoxin/thioredoxin system of oxygenic photosynthesis. Antioxid. Redox Signal. 10, 1235–1274. doi: 10.1089/ars.2007.1931
Serrato, A. J., Pérez-Ruiz, J. M., and Cejudo, F. J. (2002). Cloning of thioredoxin h reductase and characterization of the thioredoxin reductase-thioredoxin h system from wheat. Biochem. J. 367, 491–497. doi: 10.1042/bj20020103
Serrato, A. J., Pérez-Ruiz, J. M., Spínola, M. C., and Cejudo, F. J. (2004). A novel NADPH thioredoxin reductase, localized in the chloroplast, which deficiency causes hypersensitivity to abiotic stress in Arabidopsis thaliana. J. Biol. Chem. 279, 43821–43827. doi: 10.1074/jbc.M404696200
Sparla, F., Pupillo, P., and Trost, P. (2002). The C-terminal extension of glyceraldehyde-3-phosphate dehydrogenase subunit B acts as an autoinhibitory domain regulated by thioredoxins and nicotinamide adenine dinucleotide. J. Biol. Chem. 277, 44946–44952. doi: 10.1074/jbc.M206873200
Spínola, M. C., Pérez-Ruiz, J. M., Pulido, P., Kirchsteiger, K., Guinea, M., González, M., et al. (2008). NTRC new ways of using NADPH in the chloroplast. Physiol. Plant. 133, 516–524. doi: 10.1111/j.1399-3054.2008.01088.x
Stenbaek, A., Hansson, A., Wulff, R. P., Hansson, M., Dietz, K. J., and Jensen, P. E. (2008). NADPH-dependent thioredoxin reductase and 2-Cys peroxiredoxins are needed for the protection of Mg-protoporphyrin monomethyl ester cyclase. FEBS Lett. 582, 2773–2778. doi: 10.1016/j.febslet.2008.07.006
Thormählen, I., Meitzel, T., Groysman, J., Ochsner, A. B., von Roepenack-Lahaye, E., Naranjo, B., et al. (2015). Thioredoxin f1 and NADPH-dependent thioredoxin reductase C have overlapping functions in regulating photosynthetic metabolism and plant growth in response to varying light conditions. Plant Physiol. 169, 1766–1786. doi: 10.1104/pp.15.01122
Thormählen, I., Ruber, J., von Roepenack-Lahaye, E., Ehrlich, S. M., Massot, V., Hummer, C., et al. (2013). Inactivation of thioredoxin f1 leads to decreased light activation of ADP-glucose pyrophosphorylase and altered diurnal starch turnover in leaves of Arabidopsis plants. Plant Cell Environ. 36, 16–29. doi: 10.1111/j.1365-3040.2012.02549.x
Thormählen, I., Zupok, A., Rescher, J., Leger, J., Weissenberger, S., Groysman, J., et al. (2017). Thioredoxins play a crucial role in dynamic acclimation of photosynthesis in fluctuating light. Mol. Plant 10, 168–182. doi: 10.1016/j.molp.2016.11.012
Toivola, J., Nikkanen, L., Dahlstrom, K. M., Salminen, T. A., Lepisto, A., Vignols, H. F., et al. (2013). Overexpression of chloroplast NADPH-dependent thioredoxin reductase in Arabidopsis enhances leaf growth and elucidates in vivo function of reductase and thioredoxin domains. Front. Plant Sci. 4:389. doi: 10.3389/fpls.2013.00389
Vaseghi, M. J., Chibani, K., Telman, W., Liebthal, M. F., Gerken, M., Schnitzer, H., et al. (2018). The chloroplast 2-cysteine peroxiredoxin functions as thioredoxin oxidase in redox regulation of chloroplast metabolism. eLife 7:e38194. doi: 10.7554/eLife.38194
Wang, P., Liu, J., Liu, B., Feng, D., Da, Q., Shu, S., et al. (2013). Evidence for a role of chloroplastic m-type thioredoxins in the biogenesis of photosystem II in Arabidopsis. Plant Physiol. 163, 1710–1728. doi: 10.1104/pp.113.228353
Wimmelbacher, M., and Bornke, F. (2014). Redox activity of thioredoxin z and fructokinase-like protein 1 is dispensable for autotrophic growth of Arabidopsis thaliana. J. Exp. Bot. 65, 2405–2413. doi: 10.1093/jxb/eru122
Wolosiuk, R. A., and Buchanan, B. B. (1977). Thioredoxin and glutathione regulate photosynthesis in chloroplasts. Nature 266, 565–567. doi: 10.1038/266565a0
Yoshida, K., Hara, A., Sugiura, K., Fukaya, Y., and Hisabori, T. (2018). Thioredoxin-like2/2-Cys peroxiredoxin redox cascade supports oxidative thiol modulation in chloroplasts. Proc. Natl. Acad. Sci. U.S.A. 115, E8296–E8304. doi: 10.1073/pnas.1808284115
Yoshida, K., Hara, S., and Hisabori, T. (2015). Thioredoxin selectivity for thiol-based redox regulation of target proteins in chloroplasts. J. Biol. Chem. 290, 14278–14288. doi: 10.1074/jbc.M115.647545
Keywords: chloroplast, hydrogen peroxide, light, darkness, peroxiredoxin, photosynthesis, redox regulation, thioredoxin
Citation: Cejudo FJ, Ojeda V, Delgado-Requerey V, González M and Pérez-Ruiz JM (2019) Chloroplast Redox Regulatory Mechanisms in Plant Adaptation to Light and Darkness. Front. Plant Sci. 10:380. doi: 10.3389/fpls.2019.00380
Received: 05 December 2018; Accepted: 12 March 2019;
Published: 04 April 2019.
Edited by:
Sang Yeol Lee, Gyeongsang National University, South KoreaReviewed by:
David Kramer, Michigan State University, United StatesPascal Rey, Commissariat à l’Energie Atomique et aux Energies Alternatives (CEA), France
Copyright © 2019 Cejudo, Ojeda, Delgado-Requerey, González and Pérez-Ruiz. This is an open-access article distributed under the terms of the Creative Commons Attribution License (CC BY). The use, distribution or reproduction in other forums is permitted, provided the original author(s) and the copyright owner(s) are credited and that the original publication in this journal is cited, in accordance with accepted academic practice. No use, distribution or reproduction is permitted which does not comply with these terms.
*Correspondence: Francisco Javier Cejudo, ZmpjZWp1ZG9AdXMuZXM=