- The Sainsbury Laboratory, University of East Anglia, Norwich Research Park, Norwich, United Kingdom
Calcium is an essential element needed for growth and development of plants under both non-stressed and stress conditions. It thereby fulfills a dual function, being not only an important factor for cell wall and membrane stability, but also serving as a second messenger in many developmental and physiological processes, including the response of plants to biotic stress. The perception of non-self hereby induces an influx of calcium ions (Ca2+) into the cytosol, which is decoded into downstream responses ultimately leading to defense. Maintaining intracellular Ca2+ homeostasis is crucial for the ability to generate this signal. This review will describe the current knowledge of the mechanisms involved in uptake and transport of calcium as well as cellular homeostasis and signal generation, describing known genes involved and discussing possible implications the plant’s nutritional status with regard to calcium might have on immunity.
Introduction
Calcium is an essential macronutrient in plants, with concentrations in the shoot ranging from 0.1 to over 5% of dry wt (Marschner, 1995; White and Broadley, 2003). It thereby exhibits a dual function, both as a structural component of cell walls and membranes and as intracellular second messenger. The uptake, distribution, and storage in the plant therefore need to be tightly regulated in order to comply with both tasks.
To fulfill the structural role, calcium has to be available for the plant in sufficient amounts. In general, calcium deficiency as a result of low soil availability is not very common (White and Broadley, 2003). Deficiency symptoms occur however more often in developing tissue such as young leaves and fruits, due to low remobilization from old to young tissue via the phloem. This leads to a strong dependency on supply via the xylem and thus on transpiration, which in young tissues is not very high. Resulting diseases are for example tipburn in lettuce or blossom end rot in tomato (White and Broadley, 2003; Hirschi, 2004).
Besides its structural role, the main function of calcium lies in its ability to serve as a second messenger in a variety of processes ranging from root or pollen tube growth and fertilization (Michard et al., 2011; Monshausen et al., 2011; Ortiz-Ramírez et al., 2017; Zhang et al., 2017) to responses to abiotic (Knight et al., 1991, 1997) as well as biotic stress (Blume et al., 2000; Lecourieux et al., 2002, 2005). Transient, sustained, or oscillatory rises in the cytosolic Ca2+ concentration thereby serve as a signal, which is decoded into downstream responses (McAinsh and Pittman, 2009; Dodd et al., 2010; Thor and Peiter, 2014). To exert this function, free Ca2+ levels in the cytosol under non-stimulated conditions need to be kept at a low level of around 0.1 μM. This is achieved by the action of biochemical buffers (Sanders et al., 1999) as well as H+/Ca2+ antiporters and Ca2+-ATPases, which actively deliver Ca2+ into the apoplast or intracellular stores (Dodd et al., 2010; Kudla et al., 2010, 2018). To generate a Ca2+ signal, the ion thus can move down the concentration gradient into the cytosol through channel proteins in the plasma or internal membranes (Figure 1).
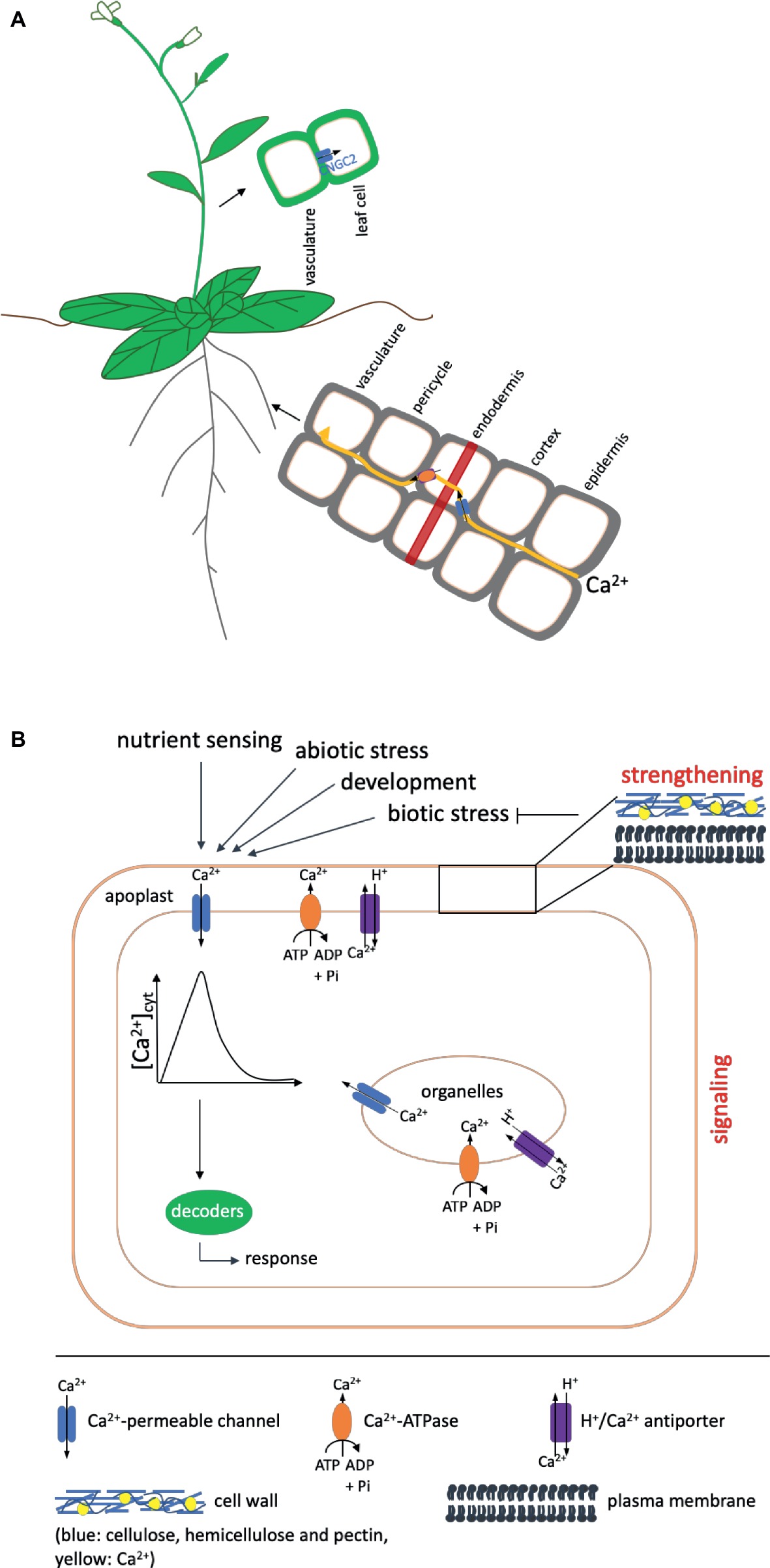
Figure 1. Overview of Ca2+ uptake and the functions it fulfills in the plant. Shown are only components mentioned in the text. (A) Ca2+ is taken up by the root and transported to the shoot in a mainly apoplastic way to avoid interference with its function as second messenger. To circumvent the casparian strip (indicated as red band), it has to enter the cytosol of the endodermal cells via channel proteins (shown in blue) and subsequently be exported into the apoplast via Ca2+-ATPases or Ca2+/H+ antiporters (shown in orange) (Clarkson, 1993). Influx into leaf cells after unloading from the vasculature in Arabidopsis has been proposed to occur via CNGC2. (B) Ca2+ fulfills two functions in the plant: It has a strengthening effect on cell walls and membranes and acts as a second messenger in signaling events during development and in response to environmental cues. A strengthened cell wall protects against pathogens (indicated by a blunt arrow). In addition, different kinds of biotic stress also induce signaling cascades, in which calcium serves as second messenger. In such signaling cascades, like in those induced by other events, Ca2+ enters the cytosol from compartments of higher concentration (apoplast, organelles) via channel proteins (blue) to induce an increase in the cytosolic calcium concentration [Ca2+]cyt, the Ca2+ signal, which is decoded by downstream components into an appropriate response. The signal is terminated by transport of Ca2+ out of the cytosol via Ca2+-ATPases (shown in orange) or H+/Ca2+ antiporters (purple) in the plasma or organeller membranes. The presence of these export proteins differs in different organelles. Details of the proteins and genes involved are given in the text. Arrows on top indicate events that induce Ca2+ signals. One such event for example also is the sensing of other nutrients.
Plants are exposed to a variety of pathogens, ranging from bacteria to fungi and oomycetes, and are also attacked by insects. To protect themselves against this biotic stress, they have evolved a two-layered immune signaling network providing defense. The first layer is termed PAMP-triggered immunity or PTI and leads to basal defense. Conserved microbial patterns (pathogen-associated molecular patterns or PAMPs) or host-derived damage-associated molecular patterns (DAMPs) are recognized by surface-localized pattern-recognition receptors (PRRs), initiating a signaling cascade leading to induction of downstream responses such as defense gene expression, stomatal closure, and callose deposition at the infection site. The Ca2+ signal is one of the earliest responses in this cascade (Blume et al., 2000; Dodds and Rathjen, 2010). To evade this defense, pathogens have evolved effector molecules, which are delivered into the host cell and target defense-related components. These effectors in turn are recognized by intracellular receptors [nucleotide-binding (NB)-leucin-rich repeat (LRR) receptors or NLRs] inducing the second layer of defense called effector-triggered immunity or ETI, which often is characterized by the occurrence of programmed cell death at the site of infection, the so-called hypersensitive response or HR. Ca2+ signals also play a role in ETI (Grant et al., 2000).
This review summarizes our current knowledge of the role calcium plays in the plant, with a focus on structural aspects, nutrition, and immunity. It describes known genes involved in its uptake and in the generation of Ca2+ signals and discusses possible links.
Calcium as a Structural Component
It has been known for many years that calcium determines the rigidity of the cell wall. Ca2+ thereby is cross-linking negatively charged carboxyl groups of de-esterified pectin in the middle lamella. In addition to cell walls, Ca2+ also stabilizes cell membranes through the interaction with phospholipids (Marschner, 1995; Hepler, 2005). A low calcium content therefore weakens the cell wall, which for example in the case of pollen tubes or root hairs is necessary to allow expansion during tip growth (Bascom et al., 2018). Interestingly, the application of EGTA to growing root hairs leads to bursting of the root hairs, which however does not seem to be a consequence of the cell wall rigidity itself but rather due to the disruption of the regulatory oscillating cytosolic tip Ca2+ gradient, which restricts growth after every cell expansion phase (Monshausen et al., 2008). The FERONIA (FER) receptor-like kinase has been implicated in root hair development (Duan et al., 2010) and a recent publication showed that salinity stress softens the cell wall most likely due to disturbance of ionic interactions by Na+, which in fer mutants leads to defects in restoring root growth under salt stress. These defects can be rescued by treatment with calcium and borate. FER interacts with pectin, and it is suggested that it senses the wall damage and induces a Ca2+ signal to induce restoration of cell wall integrity (Feng et al., 2018). It was also reported that periplasmic arabinogalactan glycoproteins (AGPs) can bind Ca2+ and therefore be a source for cytosolic Ca2+ elevations during tip growth (Lamport and Várnai, 2013).
The external application of calcium has been reported to be beneficial for resistance of plants against pathogens. Tomato plants cultivated in nutrient solution with high calcium content show increased resistance against infection by Ralstonia solanacearum (Yamazaki et al., 2000) and increasing calcium supply to soybean plants grown in the field reduced Phytophthora stem rot (Sugimoto et al., 2010). In accordance with this, early studies reported a correlation of a low plant calcium tissue content with a higher fungal infection rate, suggesting that due to the lower membrane stability, the efflux of compounds such as sugars into the apoplast could be increased (Marschner, 1995). Another early study revealed the correlation between high calcium supply and the induction of defense gene expression, suggesting that this would be due to Ca2+ acting as second messenger (Raz and Fluhr, 1992). In addition, calcium was reported to inhibit cell wall-degrading enzymes produced by fungi and bacteria, thus a lower calcium content could lead to higher degradation and infection (Marschner, 1995). After the cuticle, the cell wall constitutes the major barrier for invading pathogens. Nowadays, the understanding of the events happening at the cell wall during pathogen attack is much more detailed. We now know that cell wall-degrading enzymes and cell wall fragments can induce signaling pathways, in which Ca2+ serves as second messenger. Thus, it is not only a constituent of the cell wall itself but also transduces information on cell wall damage during pathogen attack.
Uptake and Distribution of Calcium in the Plant
Calcium is taken up from the soil solution through plasma membrane channels expressed in roots (White et al., 2002). While earlier, these channels were described based on their electrophysiological characteristics (Demidchik et al., 2002; White et al., 2002; Miedema et al., 2008), advances in the sequencing of genomes have led to the identification of different channel families and the functional characterization of specific members thereof. According to their electrophysiological properties, Ca2+-permeable channels in root cells were first classified into depolarization-activated channels (DACCs) and hyperpolarization-activated channels (HACCs) (Miedema et al., 2001, 2008). In addition, voltage-independent channels (VICs) have been described. Based on tissue expression, plasma membrane localization in root cells, and electrophysiological characteristics, annexins have been proposed to encode the HACCs, while members of the cyclic nucleotide-gated (CNGC) family of proteins and glutamate receptor homologs (GLRs) have been suggested to account for VIC-induced currents (Demidchik et al., 2002; White et al., 2002). However, most of these channels have been studied in the context of a potential role in signaling rather than nutrition.
As an important nutrient, calcium has to be taken up and distributed within the plant; however, its function as messenger affects the way it can be taken from the root surface to the xylem, in which it is transported to the shoot. In principle, both the apoplastic and the symplastic pathway could be available for this movement, but, as discussed earlier, Ca2+ concentrations in the cytosol need to be in the submicromolar range to ensure the ability of the cell to generate a Ca2+ signal. Symplastic movement therefore would interfere with the signaling function of calcium. A common model suggests that Ca2+ moves apoplastically from the epidermis through the cortex until it reaches the casparian strip of the endodermis (White, 2001). The casparian strip forms a barrier around endodermal cells, which consists mainly of suberin and lignin and prevents movement of water and solutes in order to inhibit the uptake of unwanted or toxic substances (Schreiber et al., 1999). Thus, reaching this cell layer, Ca2+ will have to enter the cytosol of the endodermal cells via channel proteins and be exported into the stelar apoplast via Ca2+-ATPases or Ca2+/H+ antiporters to finally be loaded into the xylem (Figure 1). The identities of the channels mediating the influx into endodermal cells are currently unknown. In addition to this pathway, which involves the symplast to a certain extent, a purely apoplastic pathway has been proposed (White, 2001). This could occur in regions of the root where the casparian strip of the endodermis is not yet fully developed or interrupted, such as the root apex or at the sites of lateral root emergence. An interplay between the two pathways would saturate the need of both, nutrition of and signaling in root cells as well as transport of Ca2+ to the xylem for distribution to the shoot (White, 2001).
Once loaded into the xylem, calcium is transported to the shoot, where it finally has to be unloaded into and distributed within the leaf cells (White and Broadley, 2003). Recently, CNGC2 (CYCLIC NUCLEOTIDE-GATED CHANNEL 2) from Arabidopsis has been identified as Ca2+ channel responsible for influx of Ca2+ into the leaf cells after unloading from the vasculature (Wang et al., 2017).
Calcium as Second Messenger in Signaling Networks
While early work concentrated on the role of calcium as a nutrient and structural component, it has become more and more clear now that the main function of calcium lies in its ability to serve as a second messenger in a vast variety of physiological, developmental, and stress-related processes, and indeed some of the observed effects of calcium application might also be connected to this function rather than being purely structural.
The generation of a Ca2+ signal is the result of a complex interplay between influx channels and exporters as well as pumps. As calcium signals are involved in a diverse range of processes, it is assumed that the spatiotemporal pattern of the calcium rise, the so-called Ca2+ signature, determines the specificity of the corresponding response (McAinsh and Pittman, 2009; Dodd et al., 2010). Different sets of proteins may determine the signature in response to different cues, although some components might be involved in several responses. As important as the players generating the signal are those that decode it into (specific) downstream responses. These are calmodulin (CaM), CaM-like proteins (CMLs), calcineurin B-like proteins (CBLs), CBL interacting protein kinases (CIPKs) as well as Ca2+-dependent kinases (CDPKs or CPKs in Arabidopsis). While CaM, CMLs, CBLs, and CDPKs contain EF-hand Ca2+-binding motifs, CIPKs are activated by the interaction with CBLs (Kudla et al., 2018). Activation of downstream targets such as transcription factors, transporters, or channels then further relays the signal into outputs such as gene expression or stomatal closure.
In the context of this review, the focus will be on two processes, nutrient and immunity signaling.
Nutrient Signaling
Calcium not only is a nutrient itself, interestingly in its function as second messenger, it is involved in signaling nutrient availability and changes thereof. This has been reported with relation to potassium, nitrate, iron, ammonium, and boron (Wilkins et al., 2016; Kudla et al., 2018) and also suggested for magnesium (Tang and Luan, 2017). Arabidopsis roots exhibit Ca2+ signals in response to K+ deficiency. The transport proteins responsible for the uptake of K+, AKT1 (Arabidopsis K+ transporter 1), and HAK5 (high-affinity K+ transporter 5) in Arabidopsis are both regulated via the same Ca2+ decoding complex consisting of CBL1/9 and CIPK23 (Xu et al., 2006; Ragel et al., 2015; Behera et al., 2017). The channels involved in the generation of the Ca2+ signal are still unknown. CIPK23 also regulates the activity of the transceptor IRT1 (iron-regulated transporter 1), which transports iron, but also zinc, manganese, cobalt, and cadmium. CIPK23 in this case has been shown to phosphorylate IRT1 upon binding of non-iron metals, which subsequently leads to its degradation and thus prevents unfavorable concentrations of other metals in the cytosol (Dubeaux et al., 2018). The involvement of CIPK23 thus also suggests the involvement of a Ca2+ signal in this process. Another nutrient transporter which is phosphorylated and thereby regulated by CIPK23 is NRT1.1 (nitrate transporter 1.1, also CHL1) (Ho et al., 2009). This phosphorylation occurs under low-nitrate conditions and turns the protein from a low- into a high-affinity transporter and downregulates primary nitrate responses (Ho et al., 2009). Despite the involvement of CIPK23 in all these processes, Ho et al. could show that there is no cross talk between potassium and nitrate sensing. In a later study, Riveras et al. (2015) could finally show a nitrate-induced, NRT1.1- and phospholipase C-dependent Ca2+ signal.
Plant Immunity Signaling
In immunity signaling, the recognition of a ligand by its receptor triggers a cascade of events involving influx of Ca2+ into the cytosol, the production of reactive oxygen species (ROS), MAPK signaling, and expression of defense genes as well as late responses such as callose deposition and stomatal closure. Prominent examples for PAMPs or DAMPs are the bacterial flagellin, or its synthetic analog flg22, which in Arabidopsis is recognized by the receptor FLS2 (FLAGELLIN SENSING 2) (Zipfel et al., 2004) or the endogenous peptide signal AtPep1 (Huffaker et al., 2006), which is recognized by PEPR1 and PEPR2 (Yamaguchi et al., 2006, 2010). The activity of pathogenic enzymes also leads to the production of cell wall fragments, such as oligogalacturonides (OGs). OGs result from the breakdown of pectin by polygalacturonases and serve as DAMPs to induce defense signaling. In Arabidopsis, they are recognized by the receptor Wall Associated Kinase 1 (WAK1) (He et al., 1996; Brutus et al., 2010). Cell wall-degrading enzymes can also serve as PAMPs themselves.
The influx of Ca2+ constitutes one of the earliest responses in the induced cascades. The channels mediating the Ca2+ influx are so far mostly unknown. Mainly two families of channels have been investigated in this context, CNGCs and GLRs. For example, mutants in the AtCNGC2 locus were entitled “defense, no death” (dnd1) according to their phenotype, which showed no HR but still resistance to avirulent Pseudomonas syringae as well as a range of virulent pathogens (Yu et al., 1998). dnd1 mutants exhibit an autoimmune phenotype, being dwarf and showing constitutively elevated levels of salicylic acid (SA) as well as defense gene expression such as PR1 (Yu et al., 1998; Clough et al., 2000). Wang et al. (2017) recently found that the dnd1 phenotype is dependent on the calcium supply in the medium. Consistent with a previous report by Chan et al. (2003), they found that the dwarf appearance of the mutant was dependent on high calcium supply, as was the suppression of HR. It was thus suggested that in the mutant, the CNGC2-mediated unloading of Ca2+ into the leaf cells is interrupted, leading to higher apoplastic calcium which then is strengthening the cell wall, thereby preventing HR. The establishment of the hypersensitive response as part of the plant defense therefore in this case seems to be depending on the nutritional status of the plant, i.e., the nutritional status of the plant with regard to calcium is influencing its immunity. CNGC2 has also been implicated in lipopolysaccharide-induced induction of nitric oxide (NO) production required for HR (Ali et al., 2007) as well as signaling upon perception of Pep3, but not flg22 (Ma et al., 2012). atglr3.3 mutants showed higher susceptibility to Hyaloperonospora arabidopsidis and were impaired in OG-induced NO and ROS production as well as gene expression; however, a reduction of the Ca2+ signal in these plants could not be shown (Manzoor et al., 2013). Recently, GLR3.3 and GLR3.6 together with TPC1 (two-pore channel1) have been shown to be involved in the generation of local cytosolic Ca2+ elevations at aphid feeding sites. These elevations were also shown to be dependent on the coreceptor BAK1 with the corresponding receptor perceiving the herbivore-associated molecular pattern still awaiting identification (Vincent et al., 2017).
On the decoding side, several CDPKs have been shown to be part of plant immunity signaling. Silencing of NtCDPK2 resulted in impairment of gene-for-gene-dependent HR (Romeis et al., 2001). Multiple knockout mutants of CPK4, CPK5, CPK6, and CPK11 are compromised in flg22-induced production of ROS, resistance to Pst DC3000, and show reduced levels of expression of a subset of flg22-induced genes in PTI signaling (Boudsocq et al., 2010). CPK5 has also been shown to phosphorylate respiratory burst oxidase homolog protein D (RBOHD) and participate in long-distance signaling of defense responses (Dubiella et al., 2013). In addition, cpk5/6/11 mutants are more susceptible to infection with Botrytis cinerea due to defects in ethylene production (Gravino et al., 2015). In NLR-mediated immunity, CPK4/5/6/11 phosphorylate a specific set of WRKY transcription factors to induce immune gene activation (Gao et al., 2013). CPK5 has recently been assigned a unique role in plant immunity in that, in contrast to CPK4, 6, or 11, it contributes to exo70B1-activated autoimmune responses via association with the truncated NLR protein TIR-NBS2 (TN2) (Liu et al., 2017). Another Ca2+-dependent kinase, CPK28, has been shown to be a negative regulator of PTI signaling (Monaghan et al., 2014).
Perspective
Calcium is an essential element in plants. It serves as a constituent of cell walls and membranes and thus contributes to the structure of cells and the upholding of physical barriers against pathogens. In accordance with this structural role, plants deficient in calcium have been shown to be more susceptible to pathogens and exogenous calcium supply in turn has been shown to improve the plant’s resistance. However, in recent years, it has emerged that the main function of calcium is to serve as a second messenger. It does so in signaling events connected to a vast variety of physiological, developmental, and environmental cues, among them the signaling of other nutrients as well as pathogen attack. Research is now concentrating on identifying the proteins mediating influx of Ca2+ in as well as export out of the cell and those that decode Ca2+ signals into downstream responses. Some genes have already been identified and their role in specific processes described; however, most of the proteins mediating Ca2+ uptake and distribution as well as signal generation and decoding are still unknown. Also, despite few examples, surprisingly little is known about how the involvement of calcium in one plant aspect is affecting another and whether the same or different genes are involved in two processes, as, for example, those taking up Ca2+ as a nutrient in roots and those responsible for influx during signal generation. Future research will shed more light on the different functions of this important nutrient and how they are interconnected.
Author Contributions
The author confirms being the sole contributor of this work and has approved it for publication.
Conflict of Interest Statement
The author declares that the research was conducted in the absence of any commercial or financial relationships that could be construed as a potential conflict of interest.
References
Ali, R., Ma, W., Lemtiri-Chlieh, F., Tsaltas, D., Leng, Q., von Bodman, S., et al. (2007). Death don’t have no mercy and neither does calcium: Arabidopsis CYCLIC NUCLEOTIDE GATED CHANNEL2 and innate immunity. Plant Cell 19, 1081–1095. doi: 10.1105/tpc.106.045096
Bascom, C. S., Hepler, P. K., and Bezanilla, M. (2018). Interplay between ions, the cytoskeleton, and cell wall properties during tip growth. Plant Physiol. 176, 28–40. doi: 10.1104/pp.17.01466
Behera, S., Long, Y., Schmitz-Thom, I., Wang, X.-P., Zhang, C., Li, H., et al. (2017). Two spatially and temporally distinct Ca2+ signals convey Arabidopsis thaliana responses to K+ deficiency. New Phytol. 213, 739–750. doi: 10.1111/nph.14145
Blume, B., Nürnberger, T., Nass, N., and Scheel, D. (2000). Receptor-mediated increase in cytoplasmic free calcium required for activation of pathogen defense in parsley. Plant Cell 12, 1425–1440. doi: 10.1105/tpc.12.8.1425
Boudsocq, M., Willmann, M. R., McCormack, M., Lee, H., Shan, L., He, P., et al. (2010). Differential innate immune signalling via Ca2+ sensor protein kinases. Nature 464, 418–423. doi: 10.1038/nature08794
Brutus, A., Sicilia, F., Macone, A., Cervone, F., and De Lorenzo, G. (2010). A domain swap approach reveals a role of the plant wall-associated kinase 1 (WAK1) as a receptor of oligogalacturonides. Proc. Natl. Acad. Sci. USA 107, 9452–9457. doi: 10.1073/pnas.1000675107
Chan, C. W. M., Schorrak, L. M., Smith, R. K., Bent, A. F., and Sussman, M. R. (2003). A cyclic nucleotide-gated ion channel, CNGC2, is crucial for plant development and adaptation to calcium stress. Plant Physiol. 132, 728–731. doi: 10.1104/pp.102.019216
Clarkson, D. T. (1993). Roots and the delivery of solutes to the xylem. Philos. Trans. R. Soc. Lond. B 341, 5–7. doi: 10.1098/rstb.1993.0086
Clough, S. J., Fengler, K. A., Yu, I.-C., Lippok, B., Smith, R. K., and Bent, A. F. (2000). The Arabidopsis dnd1 “defense, no death” gene encodes a mutated cyclic nucleotide-gated ion channel. Proc. Natl. Acad. Sci. USA 97, 9323–9328. doi: 10.1073/pnas.150005697
Demidchik, V., Bowen, H. C., Maathuis, F. J. M., Shabala, S. N., Tester, M. A., White, P. J., et al. (2002). Arabidopsis thaliana root non-selective cation channels mediate calcium uptake and are involved in growth. Plant J. 32, 799–808. doi: 10.1046/j.1365-313X.2002.01467.x
Dodd, A. N., Kudla, J., and Sanders, D. (2010). The language of calcium signaling. Annu. Rev. Plant Biol. 61, 593–620. doi: 10.1146/annurev-arplant-070109-104628
Dodds, P. N., and Rathjen, J. P. (2010). Plant immunity: towards an integrated view of plant–pathogen interactions. Nat. Rev. Genet. 11, 539–548. doi: 10.1038/nrg2812
Duan, Q., Kita, D., Li, C., Cheung, A. Y., and Wu, H.-M. (2010). FERONIA receptor-like kinase regulates RHO GTpase signaling of root hair development. Proc. Natl. Acad. Sci. USA 107, 17821–17826. doi: 10.1073/pnas.1005366107
Dubeaux, G., Neveu, J., Zelazny, E., and Vert, G. (2018). Metal sensing by the ITR1 transporter-receptor orchestrates its own degradation and plant metal nutrition. Mol. Cell 69, 953–964. doi: 10.1016/j.molcel.2018.02.009
Dubiella, U., Seybold, H., Durian, G., Komander, E., Lassig, R., Witte, C.-P., et al. (2013). Calcium-dependent protein kinase/NADPH oxidase activation circuit is required for rapid defense signal propagation. Proc. Natl. Acad. Sci. USA 110, 8744–8749. doi: 10.1073/pnas.1221294110
Feng, W., Kita, D., Peaucelle, A., Cartwright, H. N., Doan, V., Duan, Q., et al. (2018). The FERONIA receptor kinase maintains cell-wall integrity during salt stress through Ca2+ signaling. Curr. Biol. 28, 666–675. doi: 10.1016/j.cub.2018.01.023
Gao, X., Chen, X., Lin, W., Chen, S., Lu, D., Niu, Y., et al. (2013). Bifurcation of Arabidopsis NLR immune signaling via Ca2+-dependent protein kinases. PLoS Pathog. 9:e1003127. doi: 10.1371/journal.ppat.1003127
Grant, M., Brown, I., Adams, S., Knight, M., Ainslie, A., and Mansfield, J. (2000). The RPM1 plant disease resistance gene facilitates a rapid and sustained increase in cytosolic calcium that is necessary for the oxidative burst and hypersensitive cell death. Plant J. 23, 441–450. doi: 10.1046/j.1365-313x.2000.00804.x
Gravino, M., Savatin, D. V., Macone, A., and De Lorenzo, G. (2015). Ethylene production in Botrytis cinerea- and oligogalacturonide-induced immunity requires calcium-dependent protein kinases. Plant J. 84, 1073–1086. doi: 10.1111/tpj.13057
He, Z.-H., Fujiki, M., and Kohorn, B. D. (1996). A cell wall-associated, receptor-like protein kinase. J. Biol. Chem. 271, 19789–19793.
Hepler, P. K. (2005). Calcium: a central regulator of plant growth and development. Plant Cell 17, 2142–2155. doi: 10.1105/tpc.105.032508
Hirschi, K. D. (2004). The calcium conundrum. Both versatile nutrient and specific signal. Plant Physiol. 136, 2438–2442. doi: 10.1104/pp.104.046490
Ho, C.-H., Lin, S.-H., Hu, H.-C., and Tsay, Y.-F. (2009). CHL1 functions as a nitrate sensor in plants. Cell 138, 1184–1194. doi: 10.1016/j.cell.2009.07.004
Huffaker, A., Pearce, G., and Ryan, C. A. (2006). An endogenous peptide signal in Arabidopsis activates components of the innate immune response. Proc. Natl. Acad. Sci. USA 103, 10098–10103. doi: 10.1073/pnas.0603727103
Knight, M. R., Campbell, A. K., Smith, S. M., and Trewavas, A. J. (1991). Transgenic plant aequorin reports the effects of touch and cold-shock and elicitors on cytoplasmic calcium. Nature 352, 524–526. doi: 10.1038/352524a0
Knight, H., Trewavas, A. J., and Knight, M. R. (1997). Calcium signalling in Arabidopsis thaliana responding to drought and salinity. Plant J. 12, 1067–1078. doi: 10.1046/j.1365-313X.1997.12051067.x
Kudla, J., Batistič, O., and Hashimoto, K. (2010). Calcium signals: the lead currency of plant information processing. Plant Cell 22, 541–563. doi: 10.1105/tpc.109.072686
Kudla, J., Becker, D., Grill, E., Hedrich, R., Hippler, M., Kummer, U., et al. (2018). Advances and current challenges in calcium signaling. New Phytol. 218, 414–431. doi: 10.1111/nph.14966
Lamport, D. T. A., and Várnai, P. (2013). Periplasmic arabinogalactan glycoproteins act as a calcium capacitor that regulates plant growth and development. New Phytol. 197, 58–64. doi: 10.1111/nph.12005
Lecourieux, D., Lamotte, O., Bourque, S., Wendehenne, D., Mazars, C., Ranjeva, R., et al. (2005). Proteinaceous and oligosaccharidic elicitors induce different calcium signatures in the nucleus of tobacco cells. Cell Calcium 38, 527–538. doi: 10.1016/j.ceca.2005.06.036
Lecourieux, D., Mazars, C., Pauly, N., Ranjeva, R., and Pugin, A. (2002). Analysis and effects of cytosolic free calcium increases in response to elicitors in Nicotiana plumbaginifolia cells. Plant Cell 14, 2627–2641. doi: 10.1105/tpc.005579
Liu, N., Hake, K., Wang, W., Zhao, T., Romeis, T., and Tang, D. (2017). CALCIUM-DEPENDENT PROTEIN KINASE5 associates with the truncated NLR protein TIR-NBS2 to contribute to exo70b1-mediated immunity. Plant Cell 29, 746–759. doi: 10.1105/tpc.16.00822
Ma, Y., Walker, R. K., Zhao, Y., and Berkowitz, G. A. (2012). Linking ligand perception by PEPR pattern recognition receptors to cytosolic Ca2+ elevation and downstream immune signaling in plants. Proc. Natl. Acad. Sci. USA 109, 19852–19857. doi: 10.1073/pnas.1205448109
Manzoor, H., Kelloniemi, J., Chiltz, A., Wendehenne, D., Pugin, A., Poinssot, B., et al. (2013). Involvement of the glutamate receptor AtGLR3.3 in plant defense signaling and resistance to Hyaloperonospora arabidopsidis. Plant J. 76, 466–480. doi: 10.1111/tpj.12311
McAinsh, M. R., and Pittman, J. K. (2009). Shaping the calcium signature. New Phytol. 181, 275–294. doi: 10.1111/j.1469-8137.2008.02682.x
Michard, E., Lima, P. T., Borges, F., Silva, A. C., Portes, M. T., Carvalho, J. E., et al. (2011). Glutamate receptor–like genes form Ca2+ channels in pollen tubes and are regulated by pistil D-serine. Science 332, 434–437. doi: 10.1126/science.1201101
Miedema, H., Bothwell, J. H. F., Brownlee, C., and Davies, J. M. (2001). Calcium uptake by plant cells—channels and pumps acting in concert. Trends Plant Sci. 6, 514–519. doi: 10.1016/S1360-1385(01)02124-0
Miedema, H., Demidchik, V., Véry, A.-A., Bothwell, J. H. F., Brownlee, C., and Davies, J. M. (2008). Two voltage-dependent calcium channels co-exist in the apical plasma membrane of Arabidopsis thaliana root hairs. New Phytol. 179, 378–385. doi: 10.1111/j.1469-8137.2008.02465.x
Monaghan, J., Matschi, S., Shorinola, O., Rovenich, H., Matei, A., Segonzac, C., et al. (2014). The calcium-dependent protein kinase CPK28 buffers plant immunity and regulates BIK1 turnover. Cell Host Microbe 16, 605–615. doi: 10.1016/j.chom.2014.10.007
Monshausen, G. B., Messerli, M. A., and Gilroy, S. (2008). Imaging of the Yellow Cameleon 3.6 indicator reveals that elevations in cytosolic Ca2+ follow oscillating increases in growth in root hairs of Arabidopsis. Plant Physiol. 147, 1690–1698. doi: 10.1104/pp.108.123638
Monshausen, G. B., Miller, N. D., Murphy, A. S., and Gilroy, S. (2011). Dynamics of auxin-dependent Ca2+ and pH signaling in root growth revealed by integrating high-resolution imaging with automated computer vision-based analysis. Plant J. 65, 309–318. doi: 10.1111/j.1365-313X.2010.04423.x
Ortiz-Ramírez, C., Michard, E., Simon, A. A., Damineli, D. S. C., Hernández-Coronado, M., Becker, J. D., et al. (2017). Glutamate receptor-like channels are essential for chemotaxis and reproduction in mosses. Nature 549, 91–95. doi: 10.1038/nature23478
Ragel, P., Ródenas, R., García-Martín, E., Andrés, Z., Villalta, I., Nieves-Cordones, M., et al. (2015). The CBL-interacting protein kinase CIPK23 regulates HAK5-mediated high-affinity K+ uptake in Arabidopsis roots. Plant Physiol. 169, 2863–2873. doi: 10.1104/pp.15.01401
Raz, V., and Fluhr, R. (1992). Calcium requirement for ethylene-dependent responses. Plant Cell 4, 1123–1130. doi: 10.1105/tpc.4.9.1123
Riveras, E., Alvarez, J. M., Vidal, E. A., Oses, C., Vega, A., and Gutiérrez, R. A. (2015). The calcium ion is a second messenger in the nitrate signaling pathway of Arabidopsis. Plant Physiol. 169, 1397–1404. doi: 10.1104/pp.15.00961
Romeis, T., Ludwig, A. A., Martin, R., and Jones, J. D. (2001). Calcium-dependent protein kinases play an essential role in a plant defence response. EMBO J. 20, 5556–5567. doi: 10.1093/emboj/20.20.5556
Sanders, D., Brownlee, C., and Harper, J. F. (1999). Communicating with calcium. Plant Cell 11, 691–706. doi: 10.1105/tpc.11.4.691
Schreiber, L., Hartmann, K., Skrabs, M., and Zeier, J. (1999). Apoplastic barriers in roots: chemical composition of endodermal and hypodermal cell walls. J. Exp. Bot. 50, 1267–1280.
Sugimoto, T., Watanabe, K., Yoshida, S., Aino, M., Furiki, M., Shiono, M., et al. (2010). Field application of calcium to reduce phytophthora stem rot of soybean, and calcium distribution in plants. Plant Dis. 94, 812–819. doi: 10.1094/PDIS-94-7-0812
Tang, R.-J., and Luan, S. (2017). Regulation of calcium and magnesium homeostasis in plants: from transporters to signaling network. Curr. Opin. Plant Biol. 39, 97–105. doi: 10.1016/j.pbi.2017.06.009
Thor, K., and Peiter, E. (2014). Cytosolic calcium signals elicited by the pathogen-associated molecular pattern flg22 in stomatal guard cells are of an oscillatory nature. New Phytol. 204, 873–881. doi: 10.1111/nph.13064
Vincent, T. R., Avramova, M., Canham, J., Higgins, P., Bilkey, N., Mugford, S. T., et al. (2017). Interplay of plasma membrane and vacuolar ion channels, together with BAK1, elicits rapid cytosolic calcium elevations in Arabidopsis during aphid feeding. Plant Cell 29, 1460–1479. doi: 10.1105/tpc.17.00136
Wang, Y., Kang, Y., Ma, C., Miao, R., Wu, C., Long, Y., et al. (2017). CNGC2 is a Ca2+ influx channel that prevents accumulation of apoplastic Ca2+ in the leaf. Plant Physiol. 173, 1342–1354. doi: 10.1104/pp.16.01222
White, P. J. (2001). The pathways of calcium movement to the xylem. J. Exp. Bot. 52, 891–899. doi: 10.1093/jexbot/52.358.891
White, P. J., Bowen, H. C., Demidchik, V., Nichols, C., and Davies, J. M. (2002). Genes for calcium-permeable channels in the plasma membrane of plant root cells. Biochim. Biophys. Acta Biomembr. 1564, 299–309. doi: 10.1016/S0005-2736(02)00509-6
White, P. J., and Broadley, M. R. (2003). Calcium in plants. Ann. Bot. 92, 487–511. doi: 10.1093/aob/mcg164
Wilkins, K. A., Matthus, E., Swarbreck, S. M., and Davies, J. M. (2016). Calcium-mediated abiotic stress signaling in roots. Front. Plant Sci. 7:1296. doi: 10.3389/fpls.2016.01296
Xu, J., Li, H.-D., Chen, L.-Q., Wang, Y., Liu, L.-L., He, L., et al. (2006). A protein kinase, interacting with two calcineurin B-like proteins, regulates K+ transporter AKT1 in Arabidopsis. Cell 125, 1347–1360. doi: 10.1016/j.cell.2006.06.011
Yamaguchi, Y., Huffaker, A., Bryan, A. C., Tax, F. E., and Ryan, C. A. (2010). PEPR2 is a second receptor for the Pep1 and Pep2 peptides and contributes to defense responses in Arabidopsis. Plant Cell 22, 508–522. doi: 10.1105/tpc.109.068874
Yamaguchi, Y., Pearce, G., and Ryan, C. A. (2006). The cell surface leucine-rich repeat receptor for AtPep1, an endogenous peptide elicitor in Arabidopsis, is functional in transgenic tobacco cells. Proc. Natl. Acad. Sci. USA 103, 10104–10109. doi: 10.1073/pnas.0603729103
Yamazaki, H., Kikuchi, S., Hoshina, T., and Kimura, T. (2000). Effect of calcium concentration in nutrient solution on development of bacterial wilt and population of its pathogen Ralstonia solanacearum in grafted tomato seedlings. Soil Sci. Plant Nutr. 46, 535–539.
Yu, I.-c., Parker, J., and Bent, A. F. (1998). Gene-for-gene disease resistance without the hypersensitive response in Arabidopsis dnd1 mutant. Proc. Natl. Acad. Sci. USA 95, 7819–7824. doi: 10.1073/pnas.95.13.7819
Zhang, S., Pan, Y., Tian, W., Dong, M., Zhu, H., Luan, S., et al. (2017). Arabidopsis CNGC14 mediates calcium influx required for tip growth in root hairs. Mol. Plant 10, 1004–1006. doi: 10.1016/j.molp.2017.02.007
Keywords: calcium, cell wall, immunity, nutrient signaling, roots, uptake, transporters, kinases
Citation: Thor K (2019) Calcium—Nutrient and Messenger. Front. Plant Sci. 10:440. doi: 10.3389/fpls.2019.00440
Edited by:
Angélique Besson-Bard, Université de Bourgogne, FranceReviewed by:
Christian Mazars, UMR5546 Laboratoire de Recherche en Sciences Vegetales (LRSV), FranceStéphane Bourque, Université de Bourgogne, France
Copyright © 2019 Thor. This is an open-access article distributed under the terms of the Creative Commons Attribution License (CC BY). The use, distribution or reproduction in other forums is permitted, provided the original author(s) and the copyright owner(s) are credited and that the original publication in this journal is cited, in accordance with accepted academic practice. No use, distribution or reproduction is permitted which does not comply with these terms.
*Correspondence: Kathrin Thor, a2F0aHJpbi50aG9yQHRzbC5hYy51aw==