- 1Facultad de Ciencias Ambientales y Bioquímica, Universidad de Castilla-La Mancha, Toledo, Spain
- 2Departamento de Genética Molecular de Plantas, Centro Nacional de Biotecnología (CNB), Consejo Superior de Investigaciones Científicas (CSIC), Madrid, Spain
Stomatal abundance varies widely across natural populations of Arabidopsis thaliana, and presumably affects plant performance because it influences water and CO2 exchange with the atmosphere and thence photosynthesis and transpiration. In order to determine the genetic basis of this natural variation, we have analyzed a recombinant inbred line (RIL) population derived from the wild accession Ll-0 and the reference strain Landsberg erecta (Ler), which show low and high stomatal abundance, respectively. Quantitative trait locus (QTL) analyses of stomatal index, stomatal density, and pavement cell density measured in the adaxial cotyledon epidermis, identified five loci. Three of the genomic regions affect all traits and were named MID (Modulator of Cell Index and Density) 1 to 3. MID2 is a large-effect QTL overlapping with ERECTA (ER), the er-1 allele from Ler increasing all trait values. Additional analyses of natural and induced loss-of-function er mutations in different genetic backgrounds revealed that ER dysfunctions have differential and opposite effects on the stomatal index in adaxial and abaxial cotyledon epidermis and confirmed that ER is the gene underlying MID2. Ll-0 alleles at MID1 and MID3 displayed moderate and positive effects on the various traits. Furthermore, detailed developmental studies tracking primary and satellite stomatal lineages show that MID3-Ll-0 allele promotes the spacing divisions that initiate satellite lineages, while the ER allele limits them. Finally, expression analyses suggest that ER and MID3 modulate satellization through partly different regulatory pathways. Our characterization of MID3 indicates that genetic modulation of satellization contributes to the variation for stomatal abundance in natural populations, and subsequently that this trait might be involved in plant adaptation.
Introduction
Gas exchange of terrestrial plants with the atmosphere takes place mostly through stomata, i.e., the microscopic pores that punctuate their otherwise gas-impermeable epidermis (Raven, 2002). Stomatal pores are delimited by two guard cells, whose shape changes in response to physiological and environmental cues dynamically open or close the pore, making stomata behaving as effective valves that regulate gas exchange (Zeiger et al., 1987). The main gases moving through stomata are CO2 and H2O vapor, which diffuse passively between the leaf internal space and the adjacent atmosphere following concentration gradients. Thus, stomatal opening results in CO2 uptake and H2O loss or transpiration. This simultaneous gas exchange leads to a physiological conflict or trade-off, since capture of the CO2 needed for photosynthesis concurs in space and time with transpiration, making loss of internal water unavoidable (Hetherington and Woodward, 2003). Transpiration, on the other hand, drives water and nutrients uptake by the roots and their xylematic transport, and it refrigerates the plant surface through evaporative cooling (Raven, 2002). As water is often limiting, and the concentration of atmospheric CO2 is low, stomata often operate to maximize CO2 uptake and moderate water loss, balancing photosynthetic and transpiration rates to prevent desiccation and optimize water use efficiency and growth (Condon et al., 2004; Medrano et al., 2015). Gas exchange balances depend first on stomatal behavior, as guard cell physiology adjusts the degree of stomatal pore opening (Schroeder et al., 2001; Lawson and Blatt, 2014; Murata et al., 2015). However, they also depend on stomata numbers, spatial distribution, and size (reviewed by Bertolino et al., 2019).
Stomatal anatomical features are determined during organ growth, when stomata are gradually formed across the developing epidermis (Geisler and Sack, 2002; de Marcos et al., 2016) under the influence of internal and environmental factors (Casson and Hetherington, 2010; Qi and Torii, 2018). Stomatal development has been deeply studied in the model species Arabidopsis thaliana providing precise anatomical and molecular-genetic descriptions (reviewed by Zoulias et al., 2018; Lee and Bergmann, 2019). Three genes encoding related basic helix-loop-helix (bHLH)-type transcription factors drive this process, SPEECHLESS (SPCH), MUTE, and FAMA (Ohashi-Ito and Bergmann, 2006; MacAlister et al., 2007; Pillitteri et al., 2007). SPCH initiates the stomatal lineage from a protodermal cell termed meristemoid mother cell (MMC), which experiences an asymmetric division whose smaller product, the meristemoid, undergo repeated asymmetric divisions (MacAlister et al., 2007). The larger products of these asymmetric division are termed stomatal lineage ground cells (SLGCs) and differentiate into pavement cells that surround the stoma to ensure its proper function and occupy most of the mature epidermis (Bergmann and Sack, 2007). Alternatively, SLGCs can experience an asymmetric division termed spacing division and initiate a satellite stomatal lineage that form another stoma away from the primary one (Geisler and Sack, 2002). MUTE directs the late meristemoid to differentiate into a guard mother cell that divides symmetrically (Pillitteri et al., 2007), whereas FAMA drives the differentiation of the twin cell products into guard cells, thus forming the stoma and terminating the lineage (Ohashi-Ito and Bergmann, 2006). These three proteins act together with the bHLHs SCREAM1/ICE1 and SCREAM2 (Kanaoka et al., 2008). In addition, this positively driven process is under the control of complex phosphorylation networks through routes like YODA (YDA)-related MITOGEN ACTIVATED PROTEIN KINASE (MAPK) cascades and brassinosteroids-related BRASSINOSTEROID INSENSITIVE 2 (BIN2) (Bergmann et al., 2004; Gudesblat et al., 2012). Several signaling peptides of the EPIDERMAL PATTERNING FACTORS (EPFs; Torii, 2015) and CLAVATA3/EMBRYO SURROUNDING REGION RELATED (CLE; Qian et al., 2018) families regulate the phosphorylation cascades. These peptides interact, among others, with homo or heterodimers of the membrane receptor TOO MANY MOUTHS (TMM; Nadeau and Sack, 2002) and receptor-kinases of the ERECTA family (ERf; Shpak et al., 2005; Lee et al., 2012). Some of these components are cell-stage specific, short-lived, and act in a combinatorial fashion (Torii, 2012; Lau and Bergmann, 2012), resulting in the orchestrated development of lineages and the formation of precise stomata patterns and abundances.
Direct and specific roles on satellite lineage production has been demonstrated only for a few genes (AGL16, miR824, AGB1, and GPA1; Kutter et al., 2007; Zhang et al., 2008). It has been recently established that the SLGC potential for spacing division, impinging on satellite linage formation, is regulated by CLE9/CLE10 and ARR16/17 (Vatén et al., 2018). On the other hand, a number of stomatal development regulators, including TMM, ERf, and EPF1, reinforce correct stomatal patterns regulating stomatal fate acquisition in SLGCs and orienting their asymmetric divisions to place the new meristemoid away from the primary stoma preventing the formation of stomata in contact (Zoulias et al., 2018). However, although EPF1 and triple ERf mutants generate abundant stomata in clusters, their contribution to satellization has not been addressed in detail. Thus, both the number of satellite lineages and the correct stomata patterning during satellization are under the control of these genes. In addition, amplifying divisions contribute indirectly to satellite stomata initiation by regulating the population of SLGCs amenable to undergo spacing divisions and, therefore, regulators involved in amplifying divisions should indirectly impact on satellization.
Previous studies in model and crop plants have established consistent links between stomatal abundance or pattern, and physiological behaviors, which impact water use efficiency and yield in different ways (Bertolino et al., 2019). The latter are relevant target traits for breeding, whose genetic manipulation through determinants of stomatal development is now pursued. Such manipulations include transgenic approaches and induced mutations that modify stomatal numbers and alter physiological performance (reviewed by Bertolino et al., 2019; Endo and Torii, 2019). However, these studies have shown contrasting effects of stomatal density changes, as higher values do not necessarily correlate with increased stomatal conductance and vice versa (Dittberner et al., 2018; Bertolino et al., 2019).
In addition to artificial genetic modifications, substantial natural variation has been found for stomata related traits in model and crop plants, such as wheat, soybean, or cotton, which have identified genomic regions conferring advantageous growth through variations of stomatal conductance (reviewed by Faralli et al., 2019). In particular, several studies have taken advantage of the broad natural genetic variation described for anatomical stomatal traits in the model species A. thaliana (Woodward et al., 2002; Delgado et al., 2011; Dittberner et al., 2018). This natural variation presumably reflects adaptations to different environmental cues, enabling the identification of wild alleles that have been maintained in natural populations adapted to diverse habitats (Weigel and Nordborg, 2015). Analysis of a core collection of natural accessions revealed considerable genetic diversity not just for anatomical stomatal traits, but also for the developmental pathways underlying stomatal abundance and pattern in mature organs (Delgado et al., 2011). Furthermore, a recent analysis of 330 Arabidopsis accessions described significant correlations between anatomical stomatal traits and water use efficiency (Dittberner et al., 2018). Most of this natural variation is quantitative, which indicates that is determined by the simultaneous effect of multiple loci and the environment (reviewed by Alonso-Blanco and Méndez-Vigo, 2014). These loci have been classically addressed by quantitative trait locus (QTL) mapping using mainly recombinant inbred lines (RILs) and introgression lines (ILs) (Wijnen and Keurentjes, 2014; Bazakos et al., 2017; Cockram and Mackay, 2018). However, the recent availability of whole genome sequences for large number of accessions (Cao et al., 2011; The 1001 Genomes Consortium, 2016) has allowed genome-wide association (GWAS) analysis to determine the genetic architecture of complex Arabidopsis traits (Atwell et al., 2010; The 1001 Genomes Consortium, 2016; Bazakos et al., 2017; Dittberner et al., 2018). Thus, GWAS analysis of stomatal conductance together with anatomical stomatal traits has shown that natural variation in stomata size is an adaptive trait contributing to the optimization of water use efficiency (Dittberner et al., 2018). Nevertheless, the genetic bases of the natural variation for developmental processes, such as stomatal index or satellization (Delgado et al., 2011), remain unknown.
In this study, we have addressed the genetic bases of the natural variation for stomatal abundance and the underlying developmental processes in Arabidopsis. To this end, we have analyzed a RIL population derived from the wild accession Ll-0 and the reference strain Ler. QTL mapping of cotyledon stomatal index, and stomatal and pavement cell density, identified three loci affecting all traits. To characterize the major effect locus we analyzed in detail multiple natural and induced loss-of-function er mutations in different genetic backgrounds, revealing that ER dysfunctions have differential and opposite effects on the stomatal traits in adaxial and abaxial epidermis. Moreover, we validated the QTL identified in chromosome 3 [Modulator of Cell Index and Density (MID) 3], whose Ll-0 allele leads to a large increase in stomatal numbers. Through genetic and developmental studies, we show that MID3 and ER exhibit additive effects for stomatal abundance traits, and that they have allele-specific effects on the spacing divisions that initiate satellite lineages. Our MID3 results with indicate that Arabidopsis natural variation for stomatal abundance traits is partly determined by genetic modification of satellization, a highly specific event during stomatal lineage development.
Material and Methods
Plant Material and Growth Conditions
A. thaliana accessions and mutants were obtained from the Arabidopsis Biological Resource Center (ABRC), the Nottingham Arabidopsis Stock Center (NASC), or the National Institute of Versailles's Agronomic Research (INRA, France). The Landsberg strain carrying wild-type ERECTA (ER) allele (here designated as LER) was provided by Dr. M. Koornneef (Wageningen University, the Netherlands). The population of 139 RILs derived from a cross between Landsberg erecta (which carries er-1 mutation) and the Llagostera-0 wild accession (Ler x Ll-0 RILs) has been previously described (Sánchez-Bermejo et al., 2012). The loss-of-function mutant alleles used for ER were described previously: er-1 (Rédei, 1962; Torii et al., 1996), er-105 (Torii et al., 1996) and er-123 (Lease et al., 2001).
For the genetic validation of MID3, two near isogenic lines (NILs) carrying MID3-Ll-0 region were developed in LER (NIL1) or Ler (NIL2) backgrounds. These NILs were derived by crossing LLL90, which carries 30 and 70% Ll-0 and Ler genome proportions, with LER. The F2 (LLL90xLER) progeny was genotyped to select a plant heterozygous for the ER locus (ER/er-1), homozygous for MID3-Ll-0 region and with no other Ll-0 introgression. NIL1 and NIL2 were selected from the self-progeny of this plant, as homozygous for a single introgression around the MID3-Ll-0 region (between positions 1.8 and 7.9 Mb of chromosome 3) in the LER and Ler backgrounds, respectively. For MID3 fine-mapping, NIL1 was backcrossed with LER and the derived F2 was used to develop six ILs referred to as STAIR (ST) lines, which were homozygous for partially overlapping Ll-0 fragments of the MID3 region. Thereafter, the ST6 line, bearing a Ll-0 fragment between positions 6.35 and 7.5 Mb, was backcrossed again with LER to develop four additional homozygous ILs carrying smaller LL-0 introgression fragments and referred to as mini-ST lines.
Plants were grown in a greenhouse supplemented with lamps to provide a long‐day (LD) photoperiod (16 h light/8 h dark) at 18–23°C, as previously described (Sanchez-Bermejo et al., 2012) or in growth-chambers (Conviron MTR30) set-up at 21 ± 1°C, 60% relative humidity, and 150 ± 20 µmol m−2s−1 irradiance (Delgado et al., 2011). Seeds were sown in Petri dishes containing a filter paper soaked in water and stratified 4 days at 4°C in darkness. Thereafter, Petri dishes were transferred to a growth chamber for four additional days to allow germination, and seedlings were then planted on pots containing soil:vermiculite in proportions 3:1. Pots were moved to greenhouse or growth chambers depending on the experiments.
For the epidermal phenotyping of the RIL population, all RILs and parents were grown simultaneously in the greenhouse, in a single experiment. The MID3 NILs, ST, mini-ST lines, and related F1 plants were grown along their reference genotypes in several greenhouse and/or growth chamber experiments. All phenotyping experiments were organized in three complete randomized block designs, with one pot per line and block, and five to six plants per pot.
Quantitative Analysis of Epidermal Phenotypes and Stomatal Lineages
Stomatal and pavement cell densities (SD and PD) and stomatal index (SI) were scored in mature cotyledons, using the dental resin method as previously described (Delgado et al., 2011). Epidermal cell counts of each individual plant were an average from two 0·327-mm2 areas at the median region of the cotyledon. For evaluation of the RILs, three individuals per genotype were scored. In the rest of experiments, 10–20 plants of each genotype were used. SD and PD were calculated as number of stomata or pavement cells per area unit (cell number mm−2), respectively, and SI as percentage of epidermal cells that were stomata (number of stomata/total number of epidermal cells × 100).
Primary and satellite stomatal lineages were scored as in Delgado et al. (2011). In brief, cotyledons were collected at 3 or 5 dag and fixed in ethanol:acetic acid 9:1 (v/v), dehydrated through ethanol:water series, rehydrated, and mounted in chloral hydrate:glycerol:water (8:1:2, w/v/v) clearing solution. The adaxial epidermis was inspected with differential interference contrast (DIC) under a Nikon Eclipse 90i upright microscope and a DXM1200C camera for image acquisition. The different cell types were identified and scored. Lineage initiation was monitored by the primary lineage index (PLI) or proportion of primary stomata plus primary stoma precursors to total epidermal cells, and the satellite lineage index (SLI), or proportion of satellite stomata plus satellite stomata precursor. From this values, the total lineage index (TLI = PLI + SLI) and the percentage of satellite lineages (%SL = SLI/TLI) were calculated. Reiteration of satellite lineages was monitored as the proportion of primary lineages producing a satellite lineage (%PLS) and the proportion of satellite lineages reiterating satellization (%SLS). In addition, SI was determined in mature cotyledons of 10 plants simultaneously grown in same chambers.
Environmental interaction of MID3 effects on SI, SD, and PD were evaluated by a two-factor analysis of variance (ANOVA), with genotype (NIL1) and environment (green house vs. growth chamber) as fixed factors. Differences between mean trait values or stomatal lineage indices were tested by Student's t-tests. All statistical analyses were performed with the SPSS v. 24 package (SPSS Inc., Chicago, IL, USA).
Genotyping and Gene Sequencing
DNA for genotyping was prepared according to Edwards et al. (1991). Plants were genotyped with markers previously reported (Sánchez-Bermejo et al., 2012) or newly developed within MID3-region, or at specific alleles of ER (er-1, er-Van-0 and ER), FAMA, MUTE, and mitogen-activated protein kinase kinase 5 (MKK5). For new markers, public resources (Nordborg et al., 2005; Cao et al., 2011) were used to design INDELS, CAPS, or dCAPS markers (Table S1).
The coding region of MKK5 (1,046 bp) was sequenced in Ll-0, Ler, and LER using DNA extracted with the DNeasy Plant Mini Kit (Qiagen). The MKK5 region was amplified by polymerase chain reaction (PCR) with the HiFi PCR Kit (KapaBiosystems) using specific primers (Table S2). The PCR products were purified with the Illustra GFX PCR Purification Kit (GE Healthcare) and sequenced with BigDye technology.
QTL Anaylis
QTL mapping was carried out separately for each trait using mean RIL values that were previously loge transformed for cell density traits (SP and PD) or arcsin-root transformed for the SI. QTL were located by the multiple‐QTL‐model method (MQM) implemented in MapQTL v. 4.0 software (Van Ooijen, 2000). A logarithm of the odds (LOD) thresholds of 2.4 was used for QTL detection, corresponding to a genome‐wide significance α = 0.05 as estimated with MapQTL permutation test. The additive allele effect and the percentage of variance explained by each QTL, as well as the total variance explained by the additive effects of all QTL detected for each trait, were obtained from MQMs. Additive allele effects correspond to half the differences between the estimated means of the two RIL genotypic groups.
Two‐way genetic interactions were tested by two‐factor using the markers linked to detected QTL. The percentage of variance explained by significant interactions was estimated by type III variance components analysis. The total variances explained for each trait, including additive and interaction effects, were estimated from general linear models including all significant effects from the detected QTL. Broad sense heritabilities (h2b) were estimated as the variance component among RILs derived from type III ANOVAS. Statistical tests were performed with SPSS v. 24 package (SPSS Inc., Chicago, IL, USA).
RNA Extraction and qPCR Analysis
Cotyledons were collected at 3 dag and RNA was extracted from three independent biological replicates with TRIzol (Invitrogen), followed by column purification with the High Pure RNA Extraction Kit (Roche Diagnostics). cDNA was synthesized with the High-Capacity cDNA Reverse Transcription Kit (Applied Biosystems) according to the manufacturer's instructions. qPCR reactions were performed with the Maxima SYBR Green qPCR Master Mix (Thermo Scientific), and run in a LightCycler 480 Instrument (Roche Diagnostics). Relative expression changes were determined using the LightCycler® 480 Software Version 1.5 (Roche Diagnostics). ACT2 (At3g18780) and UBQ10 (At4g05320) were used as reference genes. The primer sets used are given in the Table S2.
Results
Genetic Variation for Stomatal Abundance Traits in Ler, Ll-0, F1 Hybrids, and the Ler x LI-0 RIL Population
The large quantitative variation previously described for stomatal numbers among natural A. thaliana accessions (Delgado et al., 2011) indicates that stomatal abundance is under multigenic control. To investigate the genetic bases underlying this variation we selected the Llagostera-0 accession (Ll-0) and the reference strain Landsberg erecta (Ler) since preliminary analyses identified substantial phenotypic differences and they are the parents of an existing RIL population (Sánchez-Bermejo et al, 2012). Phenotypic analyses of both lines were assessed in the adaxial epidermis of fully expanded cotyledons for three stomatal abundance related traits, namely stomatal index (SI), stomatal density (SD), and pavement cell density (PC). Values of the three stomatal abundance traits were strikingly lower in Ll-0 than in Ler (Figure 1), with a range of variation similar to that observed among extreme phenotypes in representative samples of other natural accessions (Delgado et al., 2011).
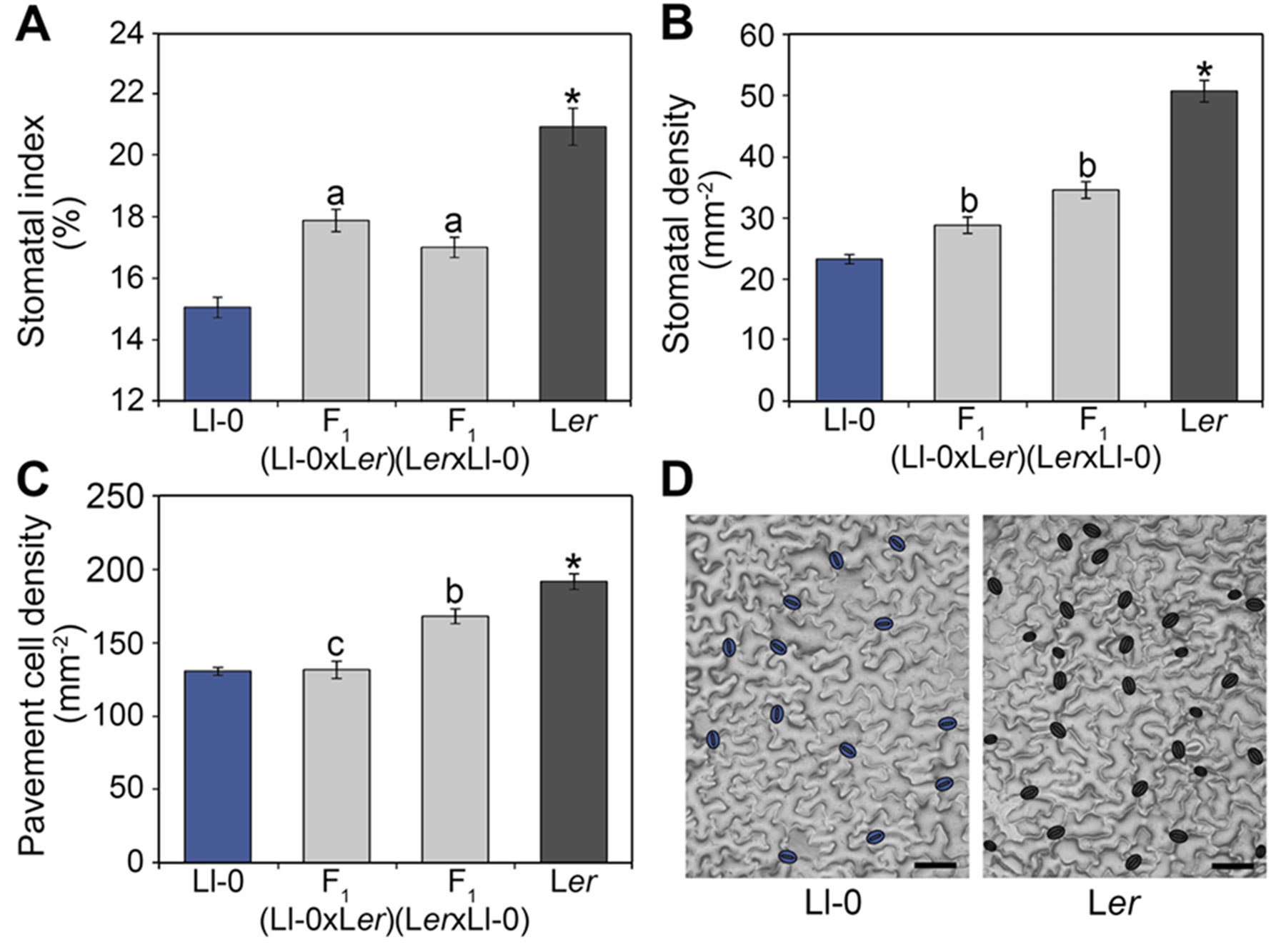
Figure 1 Stomatal abundance traits in Ll-0, Ler, and their reciprocal hybrids. Adaxial cotyledon epidermis was scored at maturity (21 dag) for stomatal index (A) and density (B) and for pavement cell density (C). Bars represent mean ± SE of 10 plants. Phenotypic differences were analyzed using a Tukey's test. Significant differences between Ll-0 and Ler are indicated with an asterisk. Letters over bars refer to phenotypic comparisons of each F1 hybrid with the remaining genotypes: "a" indicates significant differences from both parents; "b" indicates differences from all other genotypes; "c" indicates differences from male parent and reciprocal hybrid. (D) Representative mature adaxial cotyledon epidermis of Ll-0 and Ler, with stomata false-colored in blue. Micrographs were obtained with the dental resin method. Scale bars are 100 µm.
To determine the overall mode of inheritance and the dominance of stomatal phenotypes we also measured the abundances of the different epidermal cell-types in F1 hybrids derived from reciprocal crosses between Ll-0 and Ler (Figure 1). The F1 hybrids obtained using Ll-0 or Ler as mother plant showed similar SI, with intermediate values between both parental lines. Thus, the low Ll-0 SI appeared determined by the zygotic genotype and acts semidominantly. By contrast, the reciprocal F1 hybrids differed significantly for SD and PD, indicating an effect of the maternal genotype on these traits. Nevertheless, F1 hybrids displayed an intermediate phenotype between the two parental lines for SD, but a similar phenotype to mother plants for PD. Therefore, PD seems determined mainly by the maternal genotype whereas SD is under the control of both the zygote and mother genotypes.
To establish the genetic bases of the differences between Ll-0 and Ler in epidermal cell-type abundance we measured SI, SD, and PD in adaxial cotyledon epidermis in a Ler x Ll-0 RIL population of 139 lines (Table S3). Broad sense heritabilities varied between 78.4% for SD and 66.6% for SI, with substantial transgressive segregation beyond both parental values appearing for all traits (Figure 2). In addition, the three traits were highly and positively correlated within the RILs (Figure S1), as observed for natural accessions (Delgado et al., 2011). Cell-density traits (SD and PD) showed a stronger correlation (r = 0.96; P < 10−77) than stomatal abundance traits (SI and SD; r = 0.88; P < 10−45), while SI and PD had the lowest correlation strength (r = 0.72; P < 10−23). These results suggest that the three stomatal traits share a large portion of their genetic bases, which involve alleles increasing and decreasing them in both parental accessions.
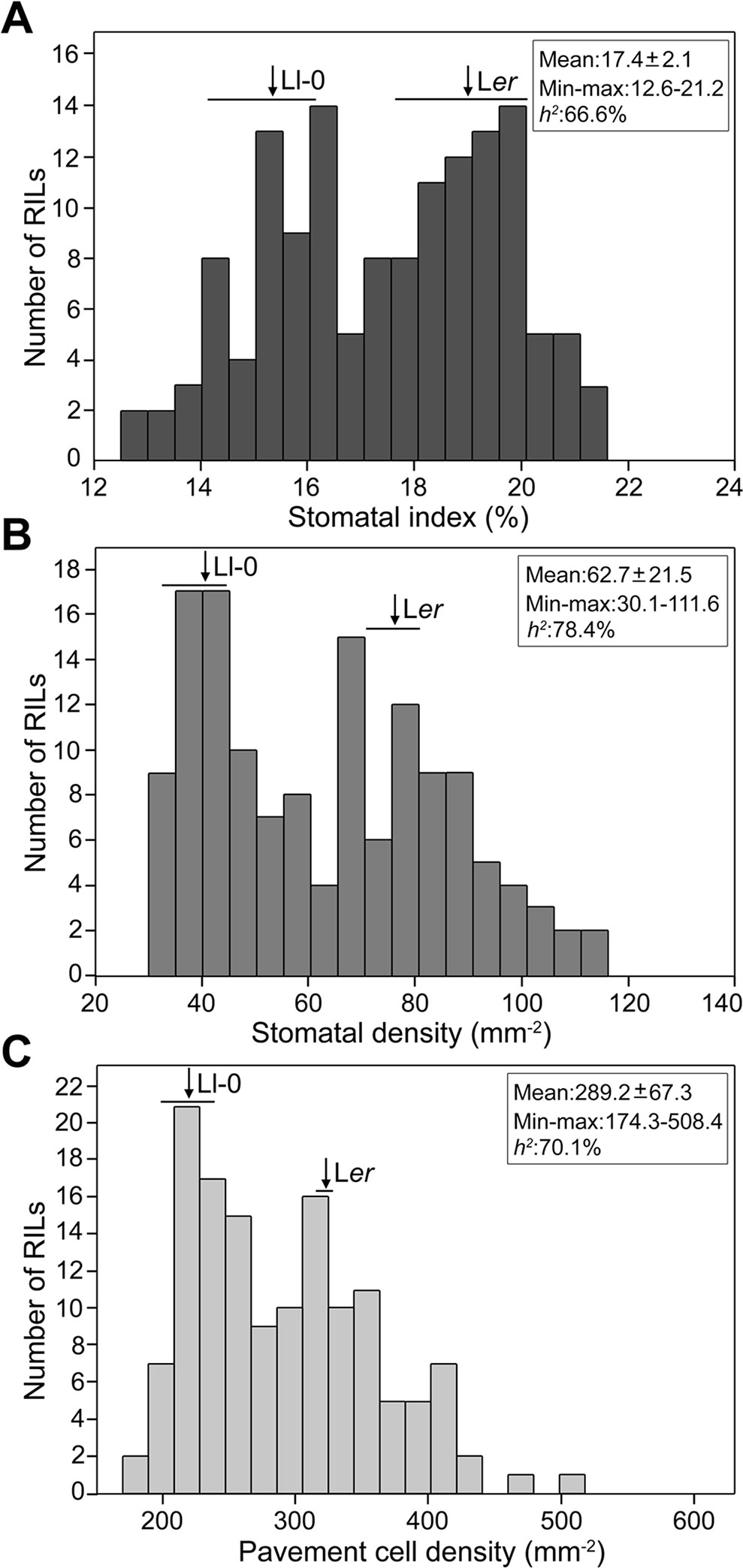
Figure 2 Frequency distributions of stomatal abundance traits in the Ler x Ll-0 (RIL)population. Stomatal index (A) and density (B) and pavement cell density (C) were scored in mature adaxial cotyledon epidermis of green-house grown plants. Arrows and horizontal bars mark mean ± SD of parental lines. The population mean, the minimum and maximum RIL means, and the broad sense heritability (h2) of traits are indicated inside each panel.
Quantitative Trait Locus Analysis in the Ler x Ll-0 RIL Population
To identify the loci that contribute to the described phenotypes, we performed QTL analyses for each trait. In total, five genomic regions affecting two or three of the traits were detected as accounting for 75.6%, 80.5%, and 71.4% of the variation for SI, SD, and PD, respectively (Figure 3 and Table S4). Three genomic regions located on chromosomes 1, 2, and 3 affected all traits suggesting pleiotropic effects of these three loci on cell-type proportion and density. Thus we named these regions MID (Modulator of Cell Index and Density) 1 to 3 according to their chromosomal location. The other two genomic regions, located on chromosomes 1 and 4, affected both SD and PD suggesting the presence of loci specifically regulating cell size processes, and were named as MCD (Modulator of Cell Density) 1 and 4.
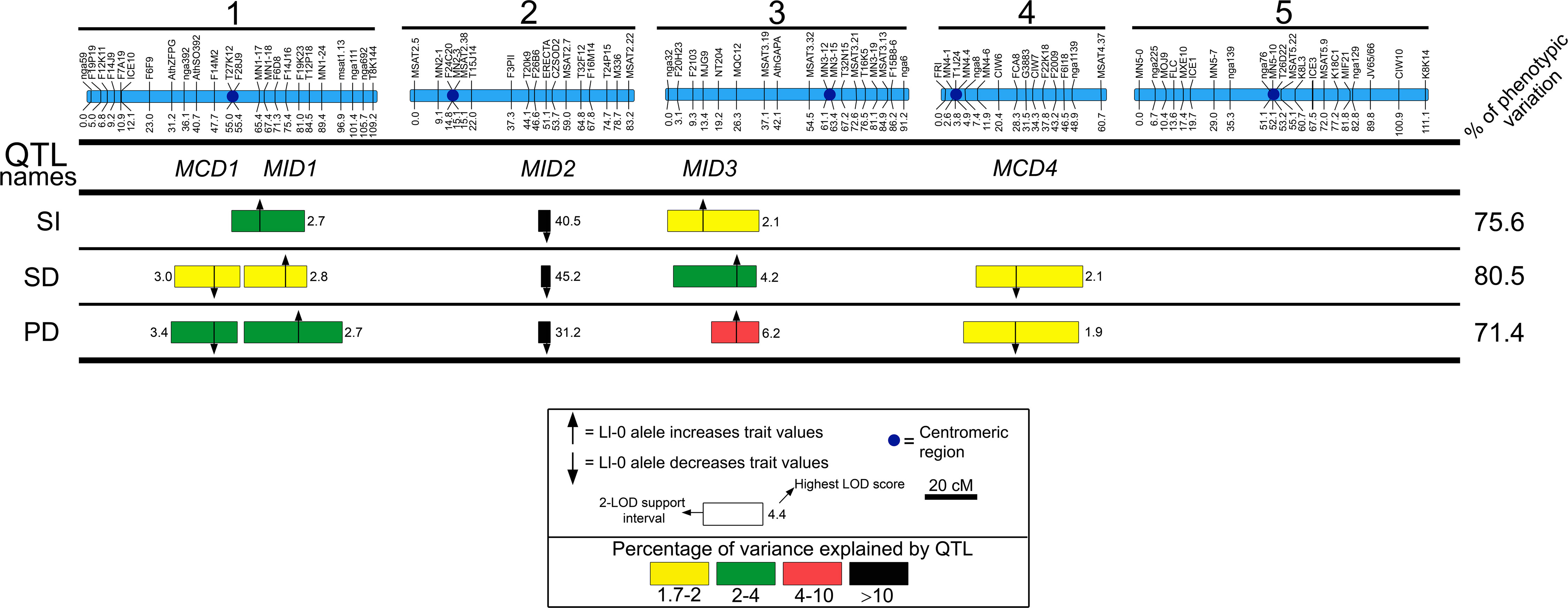
Figure 3 QTL mapping of stomatal abundance traits in the Ler/Ll-0 RIL population. Blue bars on the top represent the genetic maps of the five chromosomes, whereas QTL names are shown below: MID, Modulator of Cell Index and Density; MDC, Modulator of Cell Density. Horizontal lines separate traits analyzed: stomatal index (SI), stomatal density (SD), and pavement cell density (PD). Numbers in the right side show the percentage of phenotypic variance explained by the additive effects of all detected QTL. For each trait, the locations of QTL identified are shown as 2-LOD support intervals. Position of arrows and numbers inside boxes correspond to the highest LOD scores. Colors of QTL boxes depict the different ranges of QTL-explained variances as described in the inset. Arrows indicate that the additive effect of Ll-0 alleles increase (pointing up) or decrease (pointing down) the trait values in comparison with Ler alleles.
MID2 showed very large effects explaining between 51.7 and 67.6% of the phenotypic variation for all traits, with the Ll-0 allele reducing cell-type abundance values. The remaining regions had small relative effects (<5%); Ll-0 alleles at MID1 and MID3 displayed positive effects on the various traits, whereas at MCD1 and MCD4 Ll-0 alleles decreased cell densities. Hence, both parental genotypes carry alleles increasing and reducing each trait, in agreement with the transgressive segregation observed in the RIL population. Moreover, the co-location of QTL affecting the three traits in the various MID genomic regions explains the strong correlation among cell proportion and cell density traits in this population.
Finally, no significant two-way genetic interaction (P > 0.01) was found among these regions for any trait, supporting that differences in stomata and pavement cell abundance among Ler and Ll-0 are mainly determined by additive effects of a small number of loci.
Phenotypic Characterization and Candidate Gene for MID2
MID2 mapped centered on ER, which segregates in the Ler x Ll-0 mapping population because Ler parental carries the er-1 mutant allele while Ll-0 harbors an ER functional allele. Since it has been shown that erecta mutations in Col and Landsberg backgrounds strongly affect stomatal abundance traits in the abaxial epidermis of cotyledons and adult leaves (Masle et al., 2005; Shpak et al., 2005; Tisné et al., 2008) ER might underlie MID2. To test this hypothesis we evaluated the effects of er-1, er-105, and er-123 loss-of-function mutations in Ler, Col, and Ws-2 genetic backgrounds, respectively, on stomatal abundance traits in adaxial and abaxial cotyledon epidermis (Figure 4). The three er mutations largely increased adaxial SI, SD, and PD compared to their corresponding wild-type alleles (Figures 4A–C), thus indicating that ER underlies MID2. In agreement with earlier studies (Shpak et al., 2005), we also found that these er mutations lowered SI whereas they increased SD and PD in the abaxial epidermis of cotyledons (Figures 4D–F). The decrease in abaxial SI is partly due to the abundant small cell patches that result from arrested lineages, which did not produce stomata (see Discussion). Figure S2 shows representative adaxial and abaxial epidermis of er-105 cotyledons where the arrested lineages are patent in the abaxial side but absent in the adaxial epidermis. Therefore, ER dysfunction has differential and opposite effects on cell-type proportions in adaxial and abaxial cotyledon epidermis.
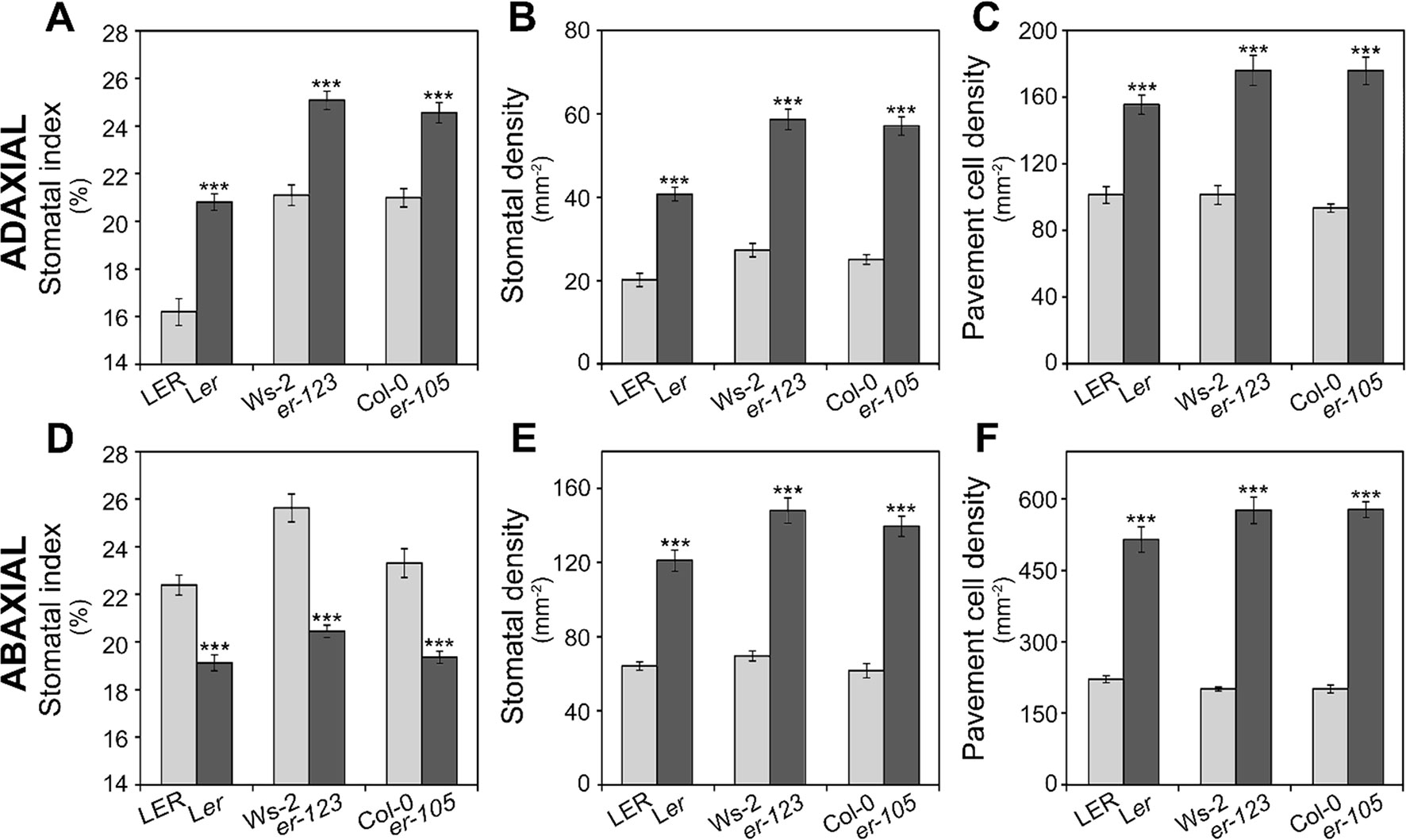
Figure 4 Effect of erecta alleles on stomatal abundance traits in both cotyledon epidermis. Stomatal index and density and pavement cell density were scored in adaxial (A–C) or abaxial (D–F) cotyledon epidermis of indicated genotypes. LER, Col-0, and Ws-2 carry wild-type ERECTA alleles, whereas Ler, er-105, and er-123 harbor dysfunctional erecta alleles. For each trait, the mean ± SE of 10–20 plants are presented. Asterisks indicate significant differences (P ≤ 0.001; Student's t-test) between a given er mutant and the corresponding wild type.
We further tested if the natural erecta null allele in the Vancouver-0 (Van-0) accession (van Zanten et al., 2009) is also involved in determining the very high SI, SD, and PD on adaxial cotyledon we previously described for this genotype (Delgado et al., 2011). An allelism test between Van-0 and er-1 was performed using Ler and LER as sources of mutant and wild-type ER alleles (Figure S3). Van-0 displayed significantly higher SI, SD, and PD than Ler (P ≤ 0.01 for all traits; Student's t-test). Reciprocal F1 hybrids derived from crosses between Van-0 and Ler showed similar low values to Ler for cell density traits, but high and similar to Van-0 for SI. By contrast, the reciprocal Van-0/LER F1 hybrids displayed low SD and PD values as LER, thought they had a SI phenotype intermediate between both parents (Figure S3). These results indicate an overall dominance of the ER functional allele for cell density traits but semidominance for cell-type proportion, although additional loci likely contribute to the LER/Ler/Van-0 variation.
Genetic Validation of MID3
In order to confirm the effects of the MID3 genomic region on epidermal cell traits, we developed two NILs carrying a single Ll-0 introgression fragment in the Landsberg genetic background (Figures 3 and 5A, B), but differing in the ER allele (Figure 5B). Analysis of these lines showed that NIL1 and NIL2 have significantly higher values for the three epidermal traits than their corresponding reference genotypes LER and Ler, in agreement with the MID3-Ll-0 allele effects estimated in the QTL mapping (Figures 5C–E). This result validates MID3 effects on adaxial cotyledon traits and narrows down its location to a genomic region of ∼6 Mb, between positions 1.8 and 7.9 of chromosome 3 (Figure 5A). It is worth noting, however, that the estimates of MID3 effects in both NILs were substantially higher for SI and lower for the cell-density traits than in the RIL population (see Figures 5C–E and Table S4). Moreover, comparison of the phenotypic effects in both NILs showed that MID3 and ER/MID2 acted additively for the tree traits (Figures 5C–E).
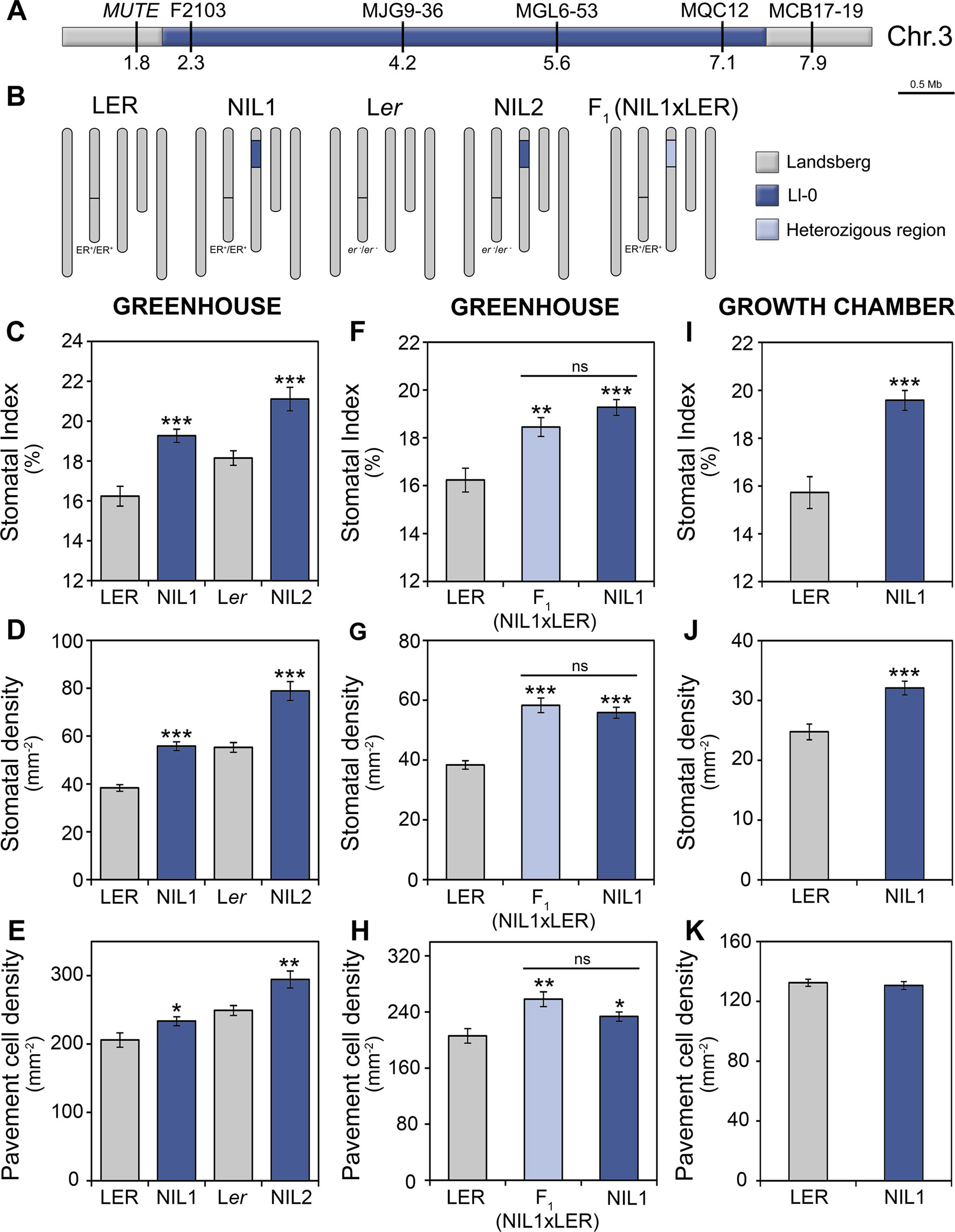
Figure 5 Characterization of stomatal abundance related phenotypes of MID3 in NILs. (A) Physical map of the MID3 region on chromosome 3, indicating marker names and positions in megabases (Mb). (B) Graphical genotypes of NILs, their reference strains and the F1 hybrids. NILs carried Ll-0 alleles at the MID3 region in LER (ER; NIL1) or Ler (er-1; NIL2) background. The color code indicates homozygous and heterozygous regions for Landsberg and Ll-0 alleles. (C–K) Stomatal abundance related traits in the adaxial cotyledon epidermis of 21 dag plants. Stomatal index (C, F, I), stomatal density (D, G, J), and pavement cell density (E, H, K) were scored in the genotypes and growth environments indicated. Each panel shows the mean ± SE of 10–20 plants. Significant differences (***P ≤ 0.001; **P ≤ 0.01; *P ≤ 0.05; Student's t-test) with respect to the reference strain (LER or Ler) are indicated by asterisks. ns, not significant.
To characterize MID3 we also analyzed the F1 (NIL1xLER) progeny. These F1 plants showed similar phenotypes than NIL1 for all traits (Figures 5F–H) indicating that Ll-0 alleles at MID3 are dominant over LER alleles. Furthermore, to determine if MID3 interacts with environmental factors, NIL1 was also assessed in growth chambers. In these conditions, the Ll-0 allele increased SI and SD (P < 0.001), as observed in greenhouse experiments, but it did not affect PD (Figures 5I–K). Therefore, MID3 showed significant genotype-by-environment interactions for PD (Table S5) indicating that MID3 cell-size effects are sensitive to environmental inputs.
Fine Mapping of MID3
To fine map MID3 we developed a mapping population based on a STAIRS design (Stepped Aligned Inbred Recombinant Strains; Koumproglou et al., 2002). This STAIRS (ST) population consisted of six homozygous lines that carry partially overlapping Ll-0 fragments of the MID3 region from NIL1, in a LER background (Figure 6A). All ST lines with Ll-0 alleles at marker MQC12 (ST4, ST5 and ST6) did not differ significantly from NIL1 in any trait examined, while lines with LER alleles at MQC12 (ST1, ST2, and ST3) were phenotypically similar to LER. These results located MID3 in a genomic region of ∼2.5 Mb, between positions 6.35 and 7.5 Mb (Figure 6A).
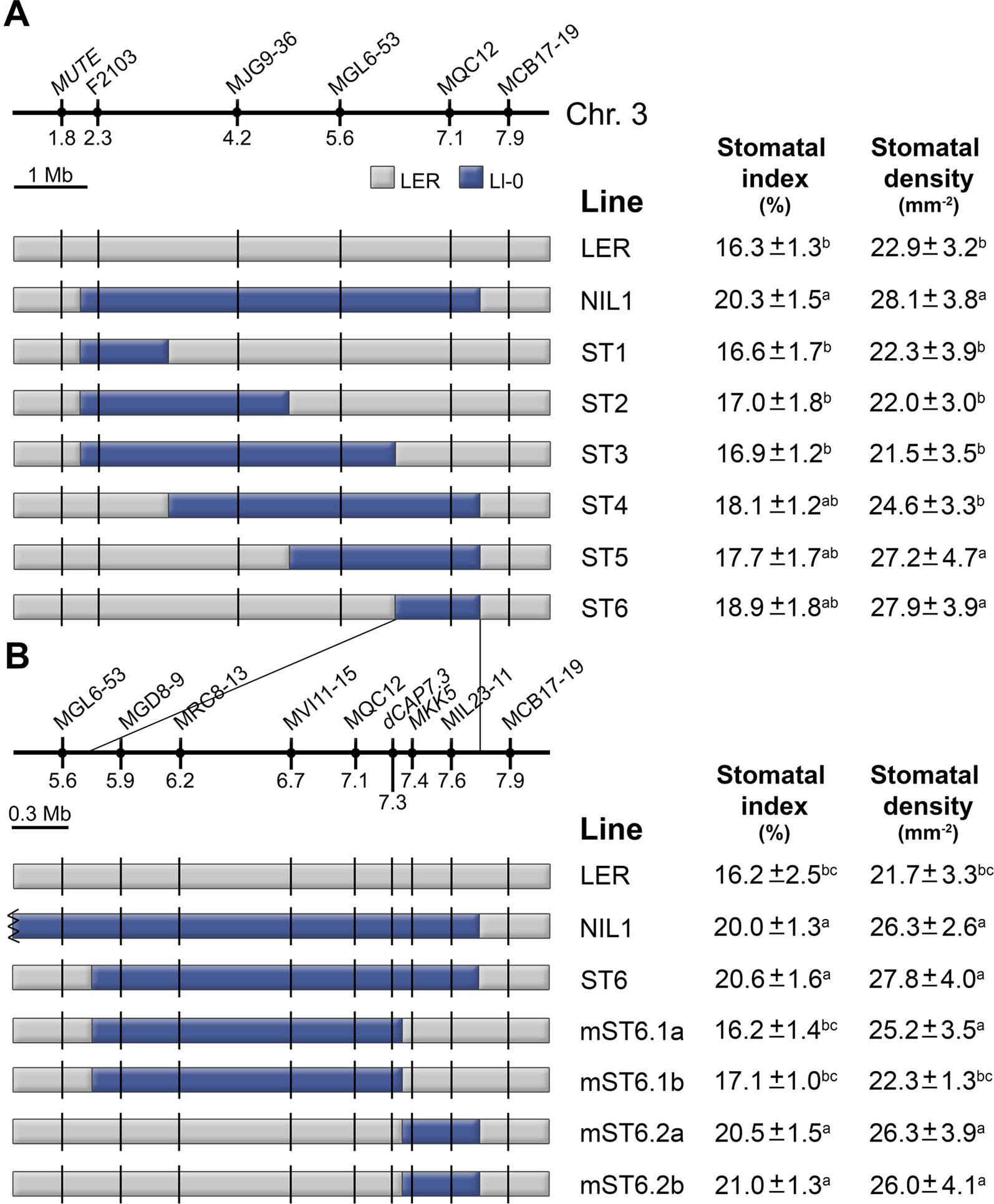
Figure 6 Fine mapping of MID3. (A) LER lines with Ll-0 overlapping introgression fragments derived from NIL1. (B) The region introgressed in ST6 is expanded to show the newly developed markers and the precise recombinant breakpoints for proximal and distal mini-STAIR (mST) lines. Names and position (Mb) of markers are shown above each panel. Color codes indicate Landsberg (gray) and Ll-0 (blue) alleles. Stomatal index and density were scored in the adaxial cotyledon epidermis at 21 dag for all lines in growth chamber experiments. Each panel shows the mean ± SE of 10–15 plants. Significant phenotypic differences (P ≤ 0.05; Tukey's test) are indicated with letters. a: differing from LER; b: differing from NIL1; c: differing from ST6.
To find candidate genes for MID3 we analyzed this genomic region searching for genes previously involved in stomata development. Interestingly, MUTE and FAMA (Pillitteri et al., 2007; Ohashi-Ito and Bergmann, 2006) were excluded because they are located in the chromosome three distal region outside the MID3 location. However, this region encompasses the MKK5 gene, which is known to negatively regulate stomatal numbers (Wang et al., 2007). Then, we used ST6 to develop a new set of NILs carrying recombination events around the MKK5 locus (Figure 6B). Homozygous lines carrying proximal or distal regions of the ST6 introgression were named as mini-STAIRS (mST). Phenotypic analyses showed that lines mST6.2a and mST6.2b carrying Ll-0 proximal introgressions that span MKK5 displayed high SI and SD values similar to ST6 and NIL1 (Figure 6B). Accordingly, lines mST6.1a and mST6.1b bearing the distal Ll-0 segment, i.e., MKK5-LER allele, displayed similar SI and SD to LER. Therefore, the MID3 gene(s) was mapped to a ∼600 kb genomic region overlapping with MKK5. Sequencing of the MKK5 coding region of Ll-0 and Ler identified two nucleotide polymorphisms, none of them resulting in amino acid substitutions (Table S6). Hence, if MKK5 underlies MID3, functional differences between Ll-0 and Ler alleles may arise from cis-acting regulatory polymorphisms but not from changes in protein structure.
We finally tested if the small mapping region of MID3 also affects stomata abundance traits in greenhouse conditions. In this environment, NIL1, ST6, and mST6.2a had similar SI, SD, and PD but significantly higher than those of LER (Figure S4), supporting the pleiotropic effects of MID3 on cell-type proportion and density.
Stomata Developmental Processes Regulated by ERECTA and MID3
Plants carrying the MID3-Ll-0 or the er-1 alleles display a SI increase in the mature adaxial cotyledon epidermis as compared to LER. These phenotypes could arise from changes in the occurrence of primary stomatal lineages initiated from the protodermis, or from their increased satellization at later stages of epidermal development. The two processes are under distinct genetic control as they present independent variation in natural Arabidopsis accessions (Delgado et al., 2011), and some genes have been reported to specifically control satellization in Arabidopsis. To examine whether ER and MID3 differentially regulate these initiation events we measured the relative contribution of primary lineages (PL) and satellite lineages (SL) to the total lineages (TL) formed at 3 dag in adaxial cotyledons of Ler (er-1) and the MID3-Ll-0 mini-ST line mST6.2b with respect to LER (Figures 7A–D and Table S7). At this developmental time, SL were already abundant and easily identified in all genotypes (see material and methods for identification criteria). While er-1 or MID3-Ll-0 alleles did not significantly affect the PL index (PLI), they both led to an increase of the SL index (SLI), particularly strong in the er-1 (Ler) mutant. Accordingly, SL contribution to total stomatal lineages (%SL) raised by about 30% in Ler and 10% in MID3-Ll-0 line with respect to LER (Table S7). Reiterated satellization was undetectable in LER and MID3-Ll-0 line, while Ler showed a small but significant proportion of reiterated satellization (above 4%). Despite Ler and the MID3-Ll-0 line had similarly higher SI than LER in mature organs, Ler showed a TL index (TLI) much higher than the MID3-Ll-0 line and LER at 3 dag, while MID3-Ll-0 differed only marginally from LER. Hence, we compared PL and SL abundance in MID3-Ll-0 and LER at a later developmental time (5 dag) (Table S8). In Ler, the over-proliferating epidermal cells characteristic of the er-1 mutation prevented a clear SL quantification at this stage. At 5 dag, TLI was already larger in the MID3-Ll-0 line than LER, consistent with their SI at maturity, and the overproduction of SL by MID3-Ll-0 was the only process contributing significantly to such increase. Taken together, these data indicate that ER and MID3 control the spacing divisions that initiate SL in opposite directions: the functional ER allele limits these divisions, while the dominant MID3-Ll-0 allele promotes them.
Effect of MID3 and ERECTA Alleles on the Expression Profile of Stomatal Genes
To test whether MID3 and ER might control spacing divisions by regulating the expression of distinct key stomatal regulators, we measured gene expression levels by qPCR in 3 dag seedlings of the MID3-Ll-0 line mST6.2b, Ler, and LER (Figure 7E). At this stage, most developing stomatal lineages in the seedling come from cotyledons and, as shown above, the three genotypes already differ in the relative proportions of PL and SL (Figures 7A–D and Table S7). The set of genes analyzed included positive (SPCH) and negative (ERL1, EPF2, TMM, and SDD1) general regulators of stomatal lineage initiation and/or progression, as well as specific regulators for SL formation. The latter include the satellization-promoting factor AGL16 and its down-regulator miR824, a pathway that enhances satellization, and also AGB1 and GPA, known to reduce the occurrence of satellite stomata (Kutter et al., 2007; Zhang et al., 2008; Yang et al., 2014). In addition, we evaluated the expression of the candidate gene for MID3, MKK5, and its functionally redundant paralog MKK4, both MKK genes acting as general negative regulators of stomatal numbers (Wang et al., 2007; Lampard et al., 2009). Transcript levels for the satellization factors (AGL16, miR824, AGB1, and GPA1) did not differ among the MID3-Ll-0 line, Ler, and LER. A weak decrease in MKK5 and MKK4 transcript levels was detected in the MID3-Ll-0 line compared to LER, though the changes were no statistical significant (P = 0.14). Therefore, these results do not clarify if MKK5 is the gene underlying MID3. The remaining general regulators of stomata development did not differ significantly between MID3-Ll-0 line and LER. However, expression levels of SPCH, EPF2, and particularly TMM, were much higher in Ler than LER or the MID3-Ll-0 line. Intriguingly, ER did not affect its own expression level or the SPCH-target genes ERL1 and SDD1, whose transcript levels have been previously correlated with the abundance of developing stomatal lineages in most genotypes and environments (Lau et al., 2014)
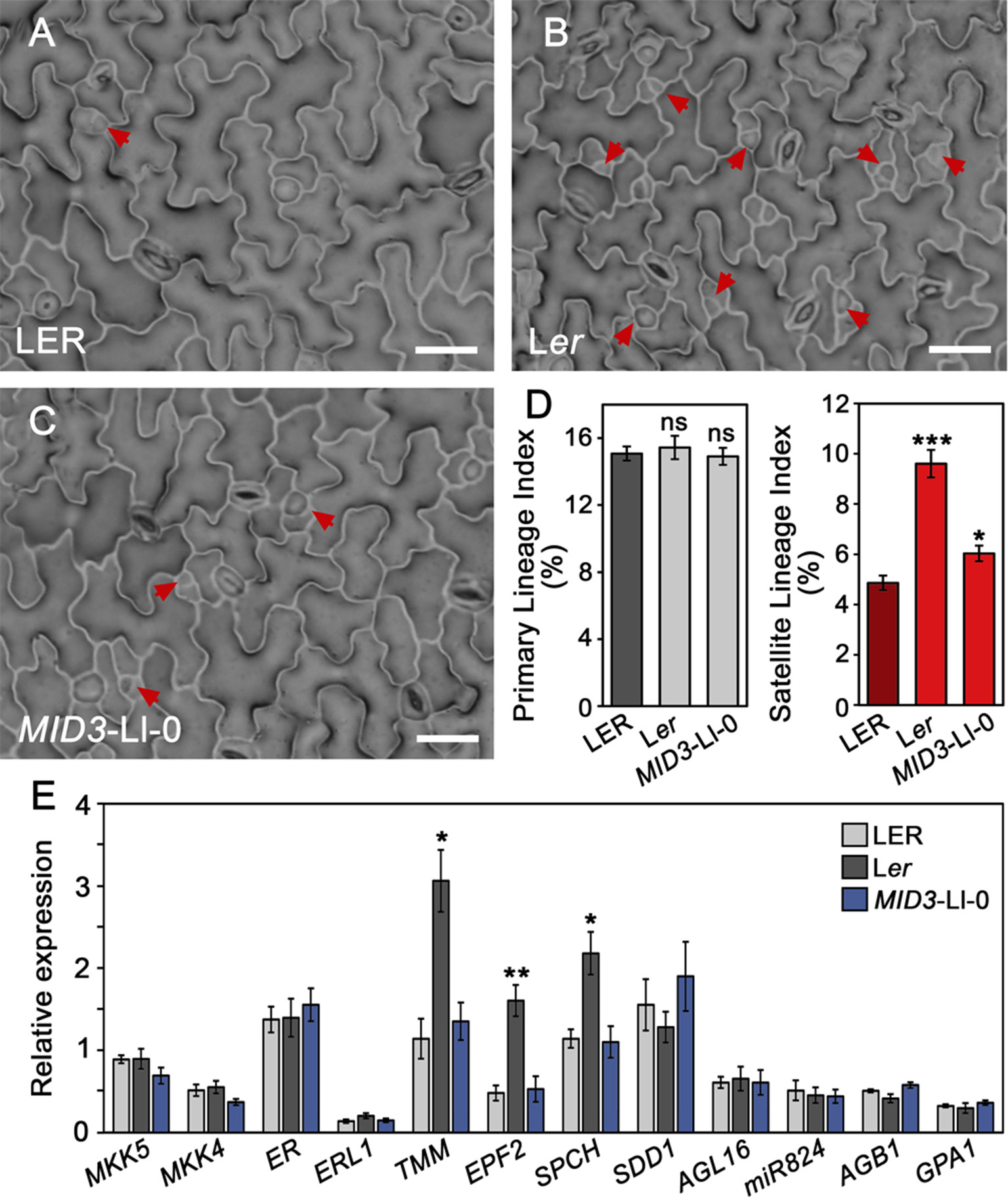
Figure 7 Differential regulation of stomatal processes and gene expression by MID3 and ER. (A–D) Indexes of primary and satellite stomatal lineages were scored in the adaxial cotyledon epidermis of 3 dag seedlings for the indicated genotypes. (A–C) show representative epidermis from the plants scored; red arrows indicate satellite lineages. (D) Mean ± SD of primary (gray) and satellite (red) lineage indexes obtained from 10 to 15 plants. Asterisks indicated significant differences (***P ≤ 0.001; *P ≤ 0.05; Student's t-test) with respect to LER. ns, not significant differences. (E) Relative gene expression determined by qPCR for the indicated genes in 3 dag seedlings of LER, Ler, and MID3-Ll-0 lines. Bars show the mean ± SE of three biological replicates. Asterisks indicated significant differences (**P ≤ 0.01; *P ≤ 0.05; Student's t-test) with respect to LER.
Discussion
Genetic Basis of the Ler/Ll-0 Variation for Stomatal Traits
Based on the analysis of cell-type proportion and density traits in the adaxial epidermis of cotyledons and first leaves, we have previously documented a wide natural genetic variation for stomatal abundance in A. thaliana (Delgado et al., 2011). Here, we dissected the genetic bases for the distinct stomatal phenotypes of Ll-0 and Ler, focusing on their adaxial cotyledon epidermis. The analysis of reciprocal F1 hybrids indicates different contributions from the zygotic and maternal genotypes on the various traits. While SI was controlled by the zygotic genotype, PD was under a strong maternal influence. Both effects combined for SD, as expected from the equal contribution to SD values of stomatal development and cell size (measured by SI and PD, respectively). Whether these maternal genetic effects persist in true leaves or they relate to the known maternal control of early seedling growth (Lemontey et al., 2000) remains to be determined.
Phenotypic variation in the Ler×Ll‐0 RIL population exhibited a robust genetic basis for all traits suggestive of a multigenic control, with opposite allelic effects from both parental accessions. Broad-sense heritability for SI was particularly high when compared with natural accessions (66.6 vs. 33–49%, respectively; see Delgado et al., 2011), suggesting that genes controlling stomatal developmental processes, and not just epidermal cell sizes (as measured by PD), underlie the stomatal differences between Ll-0 and Ler. Notably, some RILs displayed transgressive phenotypes with very high values for all traits, which, however, did not concur with aberrant stomatal patterns. This suggests that the genetic variants segregating in the RILs control stomatal production without interfering with cell-to-cell signaling mechanisms involved in proper stomatal spacing (reviewed by Zoulias et al., 2018; Lee and Bergmann, 2019).
Our QTL mapping in this RIL population identified five genomic regions affecting stomatal abundance. The three MID regions contain the identified QTL for SI, all them overlapping with QTL for SD and PD. Such phenotypic effects fit with those expected for loci modulating stomatal development and having an additional effect on pavement cell size. In the two MDC regions, only QTL for SD and PD co-located, suggesting that MDCs influence SD by regulating the size of pavement cells. Interestingly, such distinct MID and MDC effects agree with the effects described for genes controlling both initiation and cell-divisions in the stomatal lineages, the expansion of pavement cells, or both processes (Asl et al., 2011; Sablowski 2016; Fox et al., 2018). MID and MDC regions showed mostly small additive effects on the various traits, with the exception of MID2, which explained more than 50% of the phenotypic variance of all traits. Consistent with the correlations among traits, MID regions affect SI, SD, and PD values in the same direction, as MDC regions also do for SD and PD. Therefore, these allelic variants seem to allow for compensatory mechanisms that counterbalance cell size and cell numbers in the leaf epidermis, integrating the division activity of stomatal lineages (Geisler and Sack, 2002; Tsukaya, 2008). Taken together, these results indicate that Ll-0 and Ler differences in stomatal abundance traits are due to a small number of loci that likely regulate stomatal development processes and/or cell size by partly independent pathways.
Since natural variation in stomatal development has barely been studied, we focused in the characterization MID2 and MID3 by genetic, developmental, and molecular approaches. Our results confirmed that ER is the gene underlying MID2, as determined by the er-1 loss-of-function allele present in Ler parental accession (Torii et al., 1996). We also validated MID3 effects and mapped the underlying gene(s) to a ∼600 kb region. As discussed in the next sections, the MID2-Ler (er-1) and MID3-Ll-0 alleles lead to large increases in SI, SD, and PD that resulted from combining higher stomatal lineage initiation with reduction of pavement cell sizes. These findings demonstrate the value of our strategy to identify loci directly involved in stomata developmental processes. Moreover, MID3-Ll-0 provides the first description of a natural allele with a large impact on stomatal numbers demonstrated to regulate stomatal development. In fact, when isolated as a single introgression in a LER background, MID3-Ll-0 SI and SD values were nearly identical those produced by the MID2-Ler allele. Most likely, the under-estimation of MID3 effects in the RIL population is due to the segregation of multiple QTL in the RIL population, which increases the residual variance at each QTL under study (Keurentjes et al., 2007). Furthermore, both MID2 and MID3 loci differ in their genetic and environmental interactions. MID3-Ll-0 allele behaves as dominant, and shows significant genotype-by-environment interactions for PD. By contrast, MID2/ER, is semidominant for SI and not affected by the environmental factors in our conditions. Finally, MID3 and MID2/ER showed additive effects on the three traits. Therefore, MID3 and MID2/ER seem to control stomatal development and numbers by different regulatory pathways.
ERECTA Shows Differential Effects on Cell-Type Proportions and Densities of Both Cotyledon Epidermes
ER encodes a leucine-rich repeat receptor-like protein kinase with a complex functional redundancy in stomatal development with its paralogs ERL1 and ERL2 (Shpak et al., 2005). However, only ER displays a major role in restricting the entry divisions that initiate stomatal cell lineages (Shpak et al., 2005). Upon EPF2 peptide binding, ER activates the YDA-leaded MAPK cascade, which down-regulates SPCH activity inhibiting the entry divisions (Lampard et al., 2008; Lee et al., 2012; Lee et al., 2015). ER also promotes the growth of epidermal cells by increasing their ploidy levels through E2Fa regulation (Guo et al., 2019).
The phenotypic characterization carried out in this study shows that all three er loss-of-function mutant alleles have increased stomatal and pavement cell densities in both, adaxial and abaxial, epidermis of cotyledons. However, while the SI also increased in the adaxial side, it decreased in the abaxial epidermis. The lack of ER function has been previously reported to produce two developmental effects on the abaxial cotyledon, which impinge on SI in opposite ways. It increases stomatal initiation (which would rise both SLGCs and stomata numbers) but, since a substantial proportion of the lineages failed to differentiate into stomata, the net balance is a reduction of the number of stomata with respect to the SLGCs produced, and a decreased SI (Shpak et al., 2005). Our abaxial data confirm the presence of arrested linages and a decreased SI in all the er mutants studied. In the adaxial cotyledon epidermis, however, we did not observe arrested linages and, since stomatal initiation increases (see next section), SI increases. We cannot rule out that changes in amplifying divisions, which decrease SI but increase the pool of SLGCs amenable to undergo spacing divisions, contribute to the observed er phenotypes. In addition, the satellite lineages might undergo fewer amplifying divisions, perhaps because they take place at later developmental times, when the division competence of the lineages is limited (Geisler and Sack, 2002). Furthermore, our results show that ER is only necessary for lineage progression in the abaxial epidermis and suggest differences in developmental signals between the adaxial and abaxial domain. Interestingly, mutations in TMM, a stomatal receptor that works in complexes with ER, also lead to more severe phenotypes in the abaxial than the adaxial side (Geisler et al., 1998). Nevertheless, the opposite SI phenotypes found here for er cotyledons contrast with other reports in late rosette leaves, where no change in adaxial SI or an increase in abaxial SI was observed (Masle et al., 2005; Tisné et al., 2011; Jordá et al., 2016). Differences in the developmental contexts of cotyledons and leaves and/or in growth conditions may account for these disparities.
Despite de involvement of ER in many developmental and physiological processes that impact plant performance and survival, current knowledge on natural allelic variation in the ER locus is surprisingly limited. In fact, dysfunctional ER alleles have been reported only in the Van-0 and Hir (Hiroshima) accessions (van Zanten et al., 2009), and their effects have been studied for just a few processes. Our results show that ER loss-of-function contributes to the high stomatal values of Van-0 adaxial cotyledons. ER dosage affects SI but not PD values, indicating that stomatal developmental processes are sensitive to ER levels. This result agrees with the ER role in fine-modulating the accumulation of SPCH (Lee et al., 2012; Lee et al., 2015), which in turn drives stomatal production. However, it remains to be determined if effects of natural ER alleles on stomatal traits provide any adaptive advantage under natural environments, as suggested in wild beans, where some SNP haplotypes at an ER homologue appeared associated with drought tolerance (Blair et al., 2016).
MID3 and ERECTA Modulate Satellite Stomatal Lineages
We have shown that ER and MID3 influence stomatal production by modulating the incidence of satellite stomata, which implies that both loci regulate the competence of SLGCs to execute spacing divisions. MID3 effect appears to be restricted to first order SL, while ER showed an additional role in SL re-iteration. However, our data do not exclude that ER and MID3 may have an additional function on PL initiation, which can occur at later times and could be especially relevant for er-1, as this allele expands the time-window of cell division competence in the leaf epidermis (Tisné et al., 2011). Notably, the MID3-Ll-0 allele promotes spacing divisions in a dominant manner, in contrast with the ER repressive role. Multiple studies have addressed how ER negatively regulates SPCH abundance to restrict entry divisions (reviewed in Endo and Torii, 2019), but little is known about its involvement in other processes during stomatal developmental. Lineage arrest in er mutants supports the idea that ER is expressed in SLGCs to buffer the inhibitory activity of EPF1 in the neighboring meristemoid (Qi et al., 2017). Our results show that er-1 increased spacing divisions, providing a novel ER role in limiting the stomatal fate of SLGCs. This new role of ER fits well with the negative program that differentially inhibits both SPCH expression and division potential in SLGCs, needed to assign distinct cell fates after lineage asymmetric divisions (Robinson et al., 2011). Recent studies have addressed the mechanisms involved in this SLGC repression and how it could be counterbalanced to allow spacing divisions (Zhang et al., 2015; Zhang et al., 2016; Houbaert et al., 2018; Vatén et al., 2018). These reports evidenced a main role of the YDA-MAPK module in SLGC fate decision, associating high levels of MAPK activity with pavement cell differentiation, while low levels associated with spacing divisions. Interestingly, MID3 fine mapping identified the MKK5 gene, which encodes a component of this MAPK module, as a candidate for MID3. Sequencing of the MKK5 coding region ruled out functional differences between the MKK5 protein in Ll-0 and Ler. We then tested if MKK5 cis-regulatory polymorphisms could underlie MID3 locus, but we could not detect significant expression differences between MID3-Ll-0 line and LER. While these results do not excluded that MKK5 underlies the MID3-Ll-0 phenotype, they do not provide support for this possibility either. Therefore, the possible causal relationship between MID3 and MKK5 remains to be determined. Nevertheless, the effect of the natural MID3-Ll-0 allele illustrates the large impact that a single genetic factor can have on stomatal numbers by promoting SL. Indeed, MID3-Ll-0 increased SI by a 17%, and SD by about a 45%, while maintaining a proper stomatal pattern, with no observable pleiotropic effect on plant growth and reproduction.
Our genetic, developmental and expression analyses suggest that MID3 and ER act through different regulatory pathways to control satellite stomata frequency. First, they exhibited additive effects for SI, a trait that reflects the frequency of SL. Second, MID3 and ER differ in the timing of their developmental action because er-1 effects on satellite and total SI appears earlier during cotyledon development than MID3-Ll-0 effects. Third, they also show differential gene expression patterns since er-1 showed increased expression of general regulators of stomatal lineage progression (SPCH, EPF2, and TMM), while MID3-Ll-0 had no detectable change at 3 dag. Expression changes in these genes could simply represent the epidermal phenotype of Ler and MID3-L1-0, as increased number of the cell types (stomatal precursor cells) which express SPCH, EPF2, and TMM would increase the level of these transcripts. Interestingly, neither MID3 nor ER altered the expression of specific regulators of SL production (AGL16, miR824, AGB1, and GPA1; Kutter et al., 2007; Zhang et al., 2008). Thus, MID3 and ER might operate through pathways that do not involve such regulators. Recently, cytokinins have been shown to promote spacing divisions in SLGCs through re-activation of SPCH expression, which increases total stomata by 10% (Vatén et al., 2018). Therefore, MID3 and ER might also impinge on SL initiation through still undescribed pathways. It has been assumed that PL and SL contribute to the phenotypic plasticity of stomatal development in response to internal and environmental signals (Bergmann and Sack, 2007; Casson and Hetherington, 2010; Lampard, 2010). Supporting this view, recent work on the stomatal development responses (Haus et al., 2018) highlights the importance of SL in developmental plasticity in response to the atmospheric CO2 concentration.
Although satellization can contribute to as much as 35% of adaxial and 65% of abaxial stomata in Col-0 cotyledons, (Geisler and Sack, 2002), few studies have addressed this process known to result from highly specific asymmetric divisions that are different from the entry or amplification divisions. Our work demonstrates a direct role of ER in SL initiation. In Delgado et al. (2011)we reported that satellization underlies a relevant fraction of the natural variation observed in stomatal abundance among wild accessions. In fact, some accessions showing similar SI values differed in satellite index. In that work, we substantiated the existence of natural variation for both PL and SL initiation, and suggested that genetically independent pathways govern the two processes. Here, we confirmed the existence of natural variation for SL initiation, and identified a locus, MID3, directly involved in SL initiation through pathways partially different from ER. Thus, our findings on MID3 indicate that genetic modulation of satellization is a component of the variation for stomatal abundance among natural populations, which might contribute to plant adaptation.
Data Availability Statement
All datasets for this study are included in the article/Supplementary Material.
Author Contributions
DD, CA-B, and MM designed the experiment with contributions of CF. CA-B and MM supervised the work. DD performed most of the experimental work with contributions from ES-B in RIL experiments and QTL analysis, AM in qPCRs assays, and CM-J in obtaining MKK5 sequences. All authors analyzed the data. MM and CA-B wrote the manuscript with the contribution of CF and DD.
Funding
MM's and CF's lab has been funded by grants AGL2015-65053-R from the Spanish Government and PPII10-0194-4164 and SBPLY/17/180501/000394 from Junta de Comunidades de Castilla-la Mancha and by UCLM intramural grants. EU FEDER funds complemented all these grants. CA-B's lab has been funded by grant BIO2016-75754-P from the Agencia Estatal de Investigación (AEI) of Spain and the Fondo Europeo de Desarrollo Regional (FEDER, UE).
Conflict of Interest
The authors declare that the research was conducted in the absence of any commercial or financial relationships that could be construed as a potential conflict of interest.
Acknowledgments
We thank Ana Rapp for her technical support and the reviewers of the manuscript for their helpful criticisms.
Supplementary Material
The Supplementary Material for this article can be found online at: https://www.frontiersin.org/articles/10.3389/fpls.2019.01392/full#supplementary-material
References
Alonso-Blanco, C., Méndez-Vigo, B. (2014). Genetic architecture of naturally occurring quantitative traits in plants: an updated synthesis. Curr. Opin. Plant Biol. 18, 37–43. doi: 10.1016/j.pbi.2014.01.002
Asl, L. K., Dhondt, S., Boudolf, V., Beemster, G. T., Beeckman, T., Inzé, D., et al. (2011). Model-based analysis of Arabidopsis leaf epidermal cells reveals distinct division and expansion patterns for pavement and guard cells. Plant Physiol. 156(4), 2172–2183. doi: 10.1104/pp.111.181180
Atwell, S., Huang, Y. S., Vilhjálmsson, B. J., Willems, G., Horton, M., Li, Y., et al. (2010). Genome-wide association study of 107 phenotypes in Arabidopsis thaliana inbred lines. Nat. 465 (7298), 627–631. doi: 10.1038/nature08800
Bazakos, C., Hanemian, M., Trontin, C., Jiménez-Gómez, J. M., Loudet, O. (2017). New strategies and tools in quantitative genetics: how to go from the phenotype to the genotype. Annu. Rev. Plant Biol. 68, 435–455. doi: 10.1146/annurev-arplant-042916-040820
Bergmann, D. C., Lukowitz, W., Somerville, C. R. (2004). Stomatal development and pattern controlled by a MAPKK kinase. Sci. 304 (5676), 1494–1497. doi: 10.1126/science.1096014
Bergmann, D. C., Sack, F. D. (2007). Stomatal development. Annu. Rev. Plant Biol. 58, 163–181. doi: 10.1146/annurev.arplant.58.032806.104023
Bertolino, L. T., Caine, R. S., Gray, J. E. (2019). Impact of stomatal density and morphology on water-use efficiency in a changing world. Front Plant Sci. 10, 225. doi: 10.1146/annurev.arplant.58.032806.104023
Blair, M. W., Cortés, A. J., This, D. (2016). Identification of an ERECTA gene and its drought adaptation associations with wild and cultivated common bean. Plant Sci. 242, 250–259. doi: 10.1016/j.plantsci.2015.08.004
Cao, J., Schneeberger, K., Ossowski, S., Günther, T., Bender, S., Fitz, J., et al. (2011). Whole-genome sequencing of multiple Arabidopsis thaliana populations. Nat. Genet. 43 (10), 956–963. doi: 10.1038/ng.911
Casson, S. A., Hetherington, A. M. (2010). Environmental regulation of stomatal development. Curr. Opin. Plant. Biol. 13 (1), 90–95. doi: 10.1016/j.pbi.2009.08.005
Cockram, J., Mackay, I. (2018). Genetic mapping populations forconducting high-resolution tait mapping in plants. Adv. Biochem. Eng. Biotechnol. 164, 109–138. doi: 10.1007/10_2017_48
Condon, A. G., Richards, R. A., Rebetzke, G. J., Farquhar, G. D. (2004). Breeding for high water-use efficiency. J. Exp. Bot. 55 (407), 2447–2460. doi: 10.1093/jxb/erh277
de Marcos, A., Triviño, M., Fenoll, C., Mena, M. (2016). Too many faces for TOO MANY MOUTHS? New Phytol. 210 (3), 779–785. doi: 10.1111/nph.13827
Delgado, D., Alonso-Blanco, C., Fenoll, C., Mena, M. (2011). Natural variation in stomatal abundance of Arabidopsis thaliana includes cryptic diversity for different developmental processes. Ann. Bo. 107 (8), 1247–1258. doi: 10.1093/aob/mcr060
Dittberner, H., Korte, A., Mettler-Altmann, T., Weber, A. P. M., Monroe, G., de Meaux, J. (2018). Natural variation in stomata size contributes to the local adaptation of water-use efficiency in Arabidopsis thaliana. Mol. Ecol. 27 (20), 4052–4065. doi: 10.1111/mec.14838
Edwards, K., Johnstone, C., Thompson, C. (1991). A simple and rapid method for the preparation of plant genomic DNA for PCR analysis. Nucleic Acids Res. 19 (6), 1349. doi: 10.1093/nar/19.6.1349
Endo, H., Torii, K. U. (2019). Stomatal development and perspectives toward agricultural improvement. Cold Spring Harb Perspect. Biol. 11 (5), a034660. doi: 10.1101/cshperspect.a034660
Faralli, M., Matthews, J., Lawson, T. (2019). Exploiting natural variation and genetic manipulation of stomatal conductance for crop improvement. Curr. Opin. Plant. Biol. 49, 1–7. doi: 10.1016/j.pbi.2019.01.003
Fox, S., Southam, P., Pantin, F., Kennaway, R., Robinson, S., Castorina, G., et al. (2018). Spatiotemporal coordination of cell division and growth during organ morphogenesis. PLoS Biol. 16 (11), e2005952. doi: 10.1371/journal.pbio.2005952
Geisler, M., Yang, M., Sack, F. D. (1998). Divergent regulation of stomatal initiation and patterning in organ and suborgan regions of the Arabidopsis mutants too many mouths and four lips. Planta 205 (4), 522–530. doi: 10.1007/s004250050351
Geisler, M. J., Sack, F. D. (2002). Variable timing of developmental progression in the stomatal pathway in Arabidopsis cotyledons. New Phytol. 153, 469–476. doi: 10.1046/j.0028-646X.2001.00332.x
Gudesblat, G. E., Betti, C., Russinova, E. (2012). Brassinosteroids tailor stomatal production to different environments. Trends Plant Sci. 17 (12), 685–687. doi: 10.1016/j.tplants.2012.09.005
Guo, X. Y., Wang, Y., Zhao, P. X., Xu, P., Yu, G. H., Zhang, L. Y., et al. (2019). AtEDT1/HDG11 regulates stomatal density and water use efficiency via ERECTA and E2Fa. New Phytol. 223 (3), 1478–1488. doi: 10.1111/nph.15861
Haus, M. J., Li, M., Chitwood, D. H., Jacobs, T. W. (2018). Long-distance and trans-generational stomatal patterning by CO2 across Arabidopsis organs. Front Plant Sci. 9, 1714. doi: 10.3389/fpls.2018.01714
Hetherington, A. M., Woodward, F. I. (2003). The role of stomata in sensing and driving environmental change. Nat. 424 (6951), 901–908. doi: 10.1038/nature01843
Houbaert, A., Zhang, C., Tiwari, M., Wang, K., de Marcos Serrano, A., Savatin, D. V., et al. (2018). POLAR-guided signalling complex assembly and localization drive asymmetric cell division. Nat. 563 (7732), 574–578. doi: 10.1038/s41586-018-0714-x
Jordá, L., Sopeña-Torres, S., Escudero, V., Nuñez-Corcuera, B., Delgado-Cerezo, M., Torii, K. U., et al. (2016). ERECTA and BAK1 Receptor like Kinases interact to regulate immune responses in Arabidopsis. Front Plant Sci. 7, 897. doi: 10.3389/fpls.2016.00897
Kanaoka, M. M., Pillitteri, L. J., Fujii, H., Yoshida, Y., Bogenschutz, N. L., Takabayashi, J., et al. (2008). SCREAM/ICE1 and SCREAM2 specify three cell-state transitional steps leading to Arabidopsis stomatal differentiation. Plant Cell 20 (7), 1775–1785. doi: 10.1105/tpc.108.060848
Keurentjes, J. J., Bentsink, L., Alonso-Blanco, C., Hanhart, C. J., Blankestijn-De Vries, H., Effgen, S., et al. (2007). Development of a near-isogenic line population of Arabidopsis thaliana and comparison of mapping power with a recombinant inbred line population. Genetics 175 (2), 891–905. doi: 10.1534/genetics.106.066423
Koumproglou, R., Wilkes, T. M., Townson, P., Wang, X. Y., Beynon, J., Pooni, H. S., et al. (2002). STAIRS: a new genetic resource for functional genomic studies of Arabidopsis. Plant J. 31 (3), 355–364. doi: 10.1046/j.1365-313x.2002.01353.x
Kutter, C., Schöb, H., Stadler, M., Meins, F., Si-Ammour, A. (2007). MicroRNA-mediated regulation of stomatal development in Arabidopsis. Plant Cell 19 (8), 2417–2429. doi: 10.1105/tpc.107.050377
Lampard, G. R. (2010). Plasticity in stomatal development: what role does MAPK signaling play? Plant Signal Behav. 5 (5), 576–579. doi: 10.4161/psb.11494
Lampard, G. R., Lukowitz, W., Ellis, B. E., Bergmann, D. C. (2009). Novel and expanded roles for MAPK signaling in Arabidopsis stomatal cell fate revealed by cell type-specific manipulations. Plant Cell 21 (11), 3506–3517. doi: 10.1105/tpc.109.070110
Lampard, G. R., Macalister, C. A., Bergmann, D. C. (2008). Arabidopsis stomatal initiation is controlled by MAPK-mediated regulation of the bHLH SPEECHLESS. Sci. 322 (5904), 1113–1116. doi: 10.1126/science.1162263
Lau, O. S., Bergmann, D. C. (2012). Stomatal development: a plant's perspective on cell polarity, cell fate transitions and intercellular communication. Dev. 139 (20), 3683–3692. doi: 10.1242/dev.080523
Lau, O. S., Davies, K. A., Chang, J., Adrian, J., Rowe, M. H., Ballenger, C. E., et al. (2014). Direct roles of SPEECHLESS in the specification of stomatal self-renewing cells. Sci. 345 (6204), 1605–1609. doi: 10.1126/science.1256888
Lawson, T., Blatt, M. R. (2014). Stomatal size, speed, and responsiveness impact on photosynthesis and water use efficiency. Plant Physiol. 164 (4), 1556–1570. doi: 10.1104/pp.114.237107
Lease, K. A., Lau, N. Y., Schuster, R. A., Torii, K. U., Walker, J. C. (2001). Receptor serine/threonine protein kinases in signalling: analysis of the erecta receptor-like kinase of Arabidopsis thaliana. New Phytol. 151 (1), 133–143. doi: 10.1046/j.1469-8137.2001.00150.x
Lee, J. S., Hnilova, M., Maes, M., Lin, Y. C., Putarjunan, A., Han, S. K., et al. (2015). Competitive binding of antagonistic peptides fine-tunes stomatal patterning. Nat. 522 (7557), 439–443. doi: 10.1038/nature14561
Lee, J. S., Kuroha, T., Hnilova, M., Khatayevich, D., Kanaoka, M. M., McAbee, J. M., et al. (2012). Direct interaction of ligand-receptor pairs specifying stomatal patterning. Genes Dev. 26 (2), 126–136. doi: 10.1101/gad.179895.111
Lee, L. R., Bergmann, D. C. (2019). The plant stomatal lineage at a glance. J. Cell Sci. 132 (8), jcs228551. doi: 10.1242/jcs.228551
Lemontey, C., Mousset-Déclas, C., Munier-Jolain, N., Boutin, J. P. (2000). Maternal genotype influences pea seed size by controlling both mitotic activity during early embryogenesis and final endoreduplication level/cotyledon cell size in mature seed. J. Exp. Bot. 51 (343), 167–175. doi: 10.1093/jexbot/51.343.167
MacAlister, C. A., Ohashi-Ito, K., Bergmann, D. C. (2007). Transcription factor control of asymmetric cell divisions that establish the stomatal lineage. Nat. 445 (7127), 537–540. doi: 10.1038/nature05491
Masle, J., Gilmore, S. R., Farquhar, G. D. (2005). The ERECTA gene regulates plant transpiration efficiency in Arabidopsis. Nat. 436 (7052), 866–870. doi: 10.1038/nature03835
Medrano, H., Tomás, M., Martorell, S., Flexas, J., Hernández, E., Rosselló, J., et al. (2015). From leaf to whole-plant water use efficiency (WUE) in complex canopies: limitations of leaf WUE as a selection target. Crop J. 3, 220–228. doi: 10.1016/J.CJ.2015.04.002
Murata, Y., Mori, I. C., Munemasa, S. (2015). Diverse stomatal signaling and the signal integration mechanism. Annu. Rev. Plant Biol. 66, 369–392. doi: 10.1146/annurev-arplant-043014-114707
Nadeau, J. A., Sack, F. D. (2002). Control of stomatal distribution on the Arabidopsis leaf surface. Sci. 296 (5573), 1697–1700. doi: 10.1126/science.1069596
Nordborg, M., Hu, T. T., Ishino, Y., Jhaveri, J., Toomajian, C., Zheng, H., et al. (2005). The pattern of polymorphism in Arabidopsis thaliana. PLoS Biol. 3 (7), e196. doi: 10.1371/journal.pbio.0030196
Ohashi-Ito, K., Bergmann, D. C. (2006). Arabidopsis FAMA controls the final proliferation/differentiation switch during stomatal development. Plant Cell 18 (10), 2493–2505. doi: 10.1105/tpc.106.046136
Pillitteri, L. J., Sloan, D. B., Bogenschutz, N. L., Torii, K. U. (2007). Termination of asymmetric cell division and differentiation of stomata. Nat. 445 (7127), 501–505. doi: 10.1038/nature05467
Qi, X., Han, S. K., Dang, J. H., Garrick, J. M., Ito, M., Hofstetter, A. K., et al. (2017). Autocrine regulation of stomatal differentiation potential by EPF1 and ERECTA-LIKE1 ligand-receptor signaling. eLife 6, e24102. doi: 10.7554/eLife.24102
Qi, X., Torii, K. U. (2018). Hormonal and environmental signals guiding stomatal development. BMC Biol. 16 (1), 21. doi: 10.1186/s12915-018-0488-5
Qian, P., Song, W., Yokoo, T., Minobe, A., Wang, G., Ishida, T., et al. (2018). The CLE9/10 secretory peptide regulates stomatal and vascular development through distinct receptors. Nat. Plants 4 (12), 1071–1081. doi: 10.1038/s41477-018-0317-4
Raven, J. A. (2002). Selection pressures on stomatal evolution. New Phytol. 153 (3), 371–386. doi: 10.1046/j.0028-646X.2001.00334.x
Robinson, S., Barbier de Reuille, P., Chan, J., Bergmann, D., Prusinkiewicz, P., Coen, E. (2011). Generation of spatial patterns through cell polarity switching. Sci. 333 (6048), 1436–1440. doi: 10.1126/science.1202185
Sablowski, R. (2016). Coordination of plant cell growth and division: collective control or mutual agreement? Curr. Opin. Plant Biol. 34, 54–60. doi: 10.1016/j.pbi.2016.09.004
Schroeder, J. I., Allen, G. J., Hugouvieux, V., Kwak, J. M., Waner, D. (2001). GUARD CELL SIGNAL TRANSDUCTION. Annu. Rev. Plant Physiol. Plant Mol. Biol. 52, 627–658. doi: 10.1146/annurev.arplant.52.1.627
Shpak, E. D., McAbee, J. M., Pillitteri, L. J., Torii, K. U. (2005). Stomatal patterning and differentiation by synergistic interactions of receptor kinases. Sci. 309 (5732), 290–293. doi: 10.1126/science.1109710
Sánchez-Bermejo, E., Méndez-Vigo, B., Picó, F. X., Martínez-Zapater, J. M., Alonso-Blanco, C. (2012). Novel natural alleles at FLC and LVR loci account for enhanced vernalization responses in Arabidopsis thaliana. Plant Cell Environ. 35 (9), 1672–1684. doi: 10.1111/j.1365-3040.2012.02518.x
The 1001 Genomes Consortium. (2016). 1,135 Genomes reveal the global pattern of polymorphism in Arabidopsis thaliana. Cell 166 (2), 481–491. doi: 10.1016/j.cell.2016.05.063
Tisné, S., Barbier, F., Granier, C. (2011). The ERECTA gene controls spatial and temporal patterns of epidermal cell number and size in successive developing leaves of Arabidopsis thaliana. Ann. Bo. 108 (1), 159–168. doi: 10.1093/aob/mcr091
Tisné, S., Reymond, M., Vile, D., Fabre, J., Dauzat, M., Koornneef, M., et al. (2008). Combined genetic and modeling approaches reveal that epidermal cell area and number in leaves are controlled by leaf and plant developmental processes in Arabidopsis. Plant Physiol. 148 (2), 1117–1127. doi: 10.1104/pp.108.124271
Torii, K. U. (2012). Two-dimensional spatial patterning in developmental systems. Trends Cell Biol. 22 (8), 438–446. doi: 10.1016/j.tcb.2012.06.002
Torii, K. U. (2015). Stomatal differentiation: the beginning and the end. Curr. Opin. Plant. Biol. 28, 16–22. doi: 10.1016/j.pbi.2015.08.005
Torii, K. U., Mitsukawa, N., Oosumi, T., Matsuura, Y., Yokoyama, R., Whittier, R. F., et al. (1996). The Arabidopsis ERECTA gene encodes a putative receptor protein kinase with extracellular leucine-rich repeats. Plant Cell 8 (4), 735–746. doi: 10.1105/tpc.8.4.735
Tsukaya, H. (2008). Controlling size in multicellular organs: focus on the leaf. PLoS Biol. 6 (7), e174. doi: 10.1371/journal.pbio.0060174
Van Ooijen, J. W. (2000). MapQTL® v. 4.0 [computer program]: user friendly power in QTL mapping: addendum to the manual of version 3.0. -l. Netherlands: Plant Research International, Wageningen.
van Zanten, M., Snoek, L. B., Proveniers, M. C., Peeters, A. J. (2009). The many functions of ERECTA. Trends Plant Sci. 14 (4), 214–218. doi: 10.1016/j.tplants.2009.01.010
Vatén, A., Soyars, C. L., Tarr, P. T., Nimchuk, Z. L., Bergmann, D. C. (2018). Modulation of asymmetric division diversity through Cytokinin and SPEECHLESS regulatory interactions in the Arabidopsis stomatal lineage. Dev. Cell 47 (1), 53–66.e55. doi: 10.1016/j.devcel.2018.08.007
Wang, H., Ngwenyama, N., Liu, Y., Walker, J. C., Zhang, S. (2007). Stomatal development and patterning are regulated by environmentally responsive mitogen-activated protein kinases in Arabidopsis. Plant Cell 19 (1), 63–73. doi: 10.1105/tpc.106.048298
Weigel, D., Nordborg, M. (2015). Population genomics for understanding adaptation in wild plant species. Annu. Rev. Genet. 49, 315–338. doi: 10.1146/annurev-genet-120213-092110
Wijnen, C. L., Keurentjes, J. J. (2014). Genetic resources for quantitative trait analysis: novelty and efficiency in design from an Arabidopsis perspective. Curr. Opin. Plant. Biol. 18, 103–109. doi: 10.1016/j.pbi.2014.02.011
Woodward, F. I., Lake, J. A., Quick, W. P. (2002). Stomatal development and CO2: ecological consequences. New Phytol. 153, 477–484. doi: 10.1046/j.0028-646X.2001.00338.x
Yang, K., Jiang, M., Le, J. (2014). A new loss-of-function allele 28y reveals a role of ARGONAUTE1 in limiting asymmetric division of stomatal lineage ground cell. J. Integr. Plant Biol. 56 (6), 539–549. doi: 10.1111/jipb.12154.
Zeiger, E., Fraquhar, G. D., Cowan, I. D., (1987). Stomatal function. Stanford, CA, USA: Stanford University Press.
Zhang, L., Hu, G., Cheng, Y., Huang, J. (2008). Heterotrimeric G protein alpha and beta subunits antagonistically modulate stomatal density in Arabidopsis thaliana. Dev. Biol. 324 (1), 68–75. doi: 10.1016/j.ydbio.2008.09.008
Zhang, Y., Guo, X., Dong, J. (2016). Phosphorylation of the polarity protein BASL differentiates asymmetric cell fate through MAPKs and SPCH. Curr. Biol. 26 (21), 2957–2965. doi: 10.1016/j.cub.2016.08.066
Zhang, Y., Wang, P., Shao, W., Zhu, J. K., Dong, J. (2015). The BASL polarity protein controls a MAPK signaling feedback loop in asymmetric cell division. Dev. Cell 33 (2), 136–149. doi: 10.1016/j.devcel.2015.02.022
Keywords: stomatal abundance, stomatal development, Arabidopsis thaliana, natural variation, quantitative trait locus, stomatal lineage, stomatal satellite lineages
Citation: Delgado D, Sánchez-Bermejo E, de Marcos A, Martín-Jimenez C, Fenoll C, Alonso-Blanco C and Mena M (2019) A Genetic Dissection of Natural Variation for Stomatal Abundance Traits in Arabidopsis. Front. Plant Sci. 10:1392. doi: 10.3389/fpls.2019.01392
Received: 22 May 2019; Accepted: 09 October 2019;
Published: 11 November 2019.
Edited by:
Oscar Lorenzo, University of Salamanca, SpainReviewed by:
Jie Le, Institute of Botany (CAS), ChinaJosé Manuel Pérez-Pérez, Universidad Miguel Hernández de Elche, Spain
Copyright © 2019 Delgado, Sánchez-Bermejo, de Marcos, Martín-Jimenez, Fenoll, Alonso-Blanco and Mena. This is an open-access article distributed under the terms of the Creative Commons Attribution License (CC BY). The use, distribution or reproduction in other forums is permitted, provided the original author(s) and the copyright owner(s) are credited and that the original publication in this journal is cited, in accordance with accepted academic practice. No use, distribution or reproduction is permitted which does not comply with these terms.
*Correspondence: Montaña Mena, Montana.mena@uclm.es