- 1Clean Energy Research Center, Korea Institute of Science and Technology, Seoul, South Korea
- 2Department of Wood Science, University of British Columbia, Vancouver, BC, Canada
- 3Department of Paper and Bioprocess Engineering, State University of New York College of Environmental Science and Forestry, Syracuse, NY, United States
- 4Feedstocks Division, Joint BioEnergy Institute, Emeryville, CA, United States
- 5Environmental Genomics and Systems Biology Division, Lawrence Berkeley National Laboratory, Berkeley, CA, United States
The complex and heterogeneous polyphenolic structure of lignin confers recalcitrance to plant cell walls and challenges biomass processing for agroindustrial applications. Recently, significant efforts have been made to alter lignin composition to overcome its inherent intractability. In this work, to overcome technical difficulties related to biomass recalcitrance, we report an integrated strategy combining biomass genetic engineering with a pretreatment using a bio-derived deep eutectic solvent (DES). In particular, we employed biomass from an Arabidopsis line that expressed a bacterial hydroxycinnamoyl-CoA hydratase-lyase (HCHL) in lignifying tissues, which results in the accumulation of unusual C6C1 lignin monomers and a slight decrease in lignin molecular weight. The transgenic biomass was pretreated with renewable DES that can be synthesized from lignin-derived phenols. Biomass from the HCHL plant line containing C6C1 monomers showed increased pretreatment efficiency and released more fermentable sugars up to 34% compared to WT biomass. The enhanced biomass saccharification of the HCHL line is likely due to a reduction of lignin recalcitrance caused by the overproduction of C6C1 aromatics that act as degree of polymerization (DP) reducers and higher chemical reactivity of lignin structures with such C6C1 aromatics. Overall, our findings demonstrate that strategic plant genetic engineering, along with renewable DES pretreatment, could enable the development of sustainable biorefinery.
Introduction
Growing concerns over global warming and our over-dependence on fossil resources have forced society to demand sustainable and green products (Isikgor and Becer, 2015). Lignocellulosic biomass presents a promising source of renewable carbon, holding enormous potential for the production of chemicals and fuels. In order to utilize biomass as a source of renewable energy and chemicals, there have been significant efforts to develop an efficient process for biomass conversion (Demirbaş, 2001). Among several technologies developed within the biorefinery concept, the production of second-generation biofuels (e.g., bioethanol) from lignocellulosic biomass is well established and close to commercialization. Despite the successful demonstration of biomass conversion to biofuels, the production of cellulosic biofuels still encounters several technical challenges for achieving a sustainable energy future (Dale et al., 2014; Maity, 2015; Kim and Kim, 2018).
Lignin, an essential component of biomass that provides mechanical strength for upright growth and acts as a physical barrier against pathogens (Boudet, 2007), is often blamed for conferring recalcitrance to biomass against processing and utilization. In the typical biological conversion process, effective removal of lignin is crucial to maximize the utilization of carbohydrates to produce fuels and building block chemicals (Ding et al., 2012; Ragauskas et al., 2014). However, considering its complex and heterogeneous structure, hydrophobic character, and other intractable properties, lignin is one of the most challenging biomaterials to handle (Himmel, 2009).
During the last decades, researchers have developed several routes to overcome lignin-associated recalcitrance. For example, the lignin-first approach was introduced to extract the reactive lignin at the early stage of biomass fractionation, providing opportunities for the use of both lignin and carbohydrates (Renders et al., 2017). In contrast to the conventional carbohydrates-oriented pretreatment technologies of biomass, this new biorefinery scheme offers potential valorization routes for both lignin fractions and residual carbohydrates. While the above strategy focuses on the development of processes to overcome the technical difficulties related to lignin, another effort to reduce the biomass recalcitrance through genetic engineering has shown its effectiveness (Chen and Dixon, 2007; Bhagia et al., 2016; Thomas et al., 2017).
Previously, biomass cell wall engineering was directed to strategically reduce total lignin content by downregulating one or more enzymes in the monolignol pathway, which includes cinnamate 4-hydroxylase (C4H) (Schilmiller et al., 2009), 4-coumarate-CoA ligase (4CL) (Xu et al., 2011), and cinnamoyl-CoA reductase (CCR) (Chabannes et al., 2001). Although the decrease in the amount of lignin was proven to improve processing efficiency, this method often involves an agronomic penalty (Bonawitz and Chapple, 2013). Moreover, modification of lignin monomeric composition leading to structural modifications has been extensively studied to make biomass more amenable to processing without compromising biomass yield. In-planta expression of a bacterial 3-dehydroshikimate dehydratase resulted in the higher deposition of H-units and lower amounts of G- and S-units, resulting in more than a two-fold improvement in saccharification efficiency (Eudes et al., 2015). Incorporation of chemically labile ester linkages (zip-lignin) in the lignin backbone was proven to enhance biomass pretreatment efficiency (Wilkerson et al., 2014; Kim et al., 2017). Previous work also reported a strategy for the overproduction of uncommon lignin monomers through in-planta expression of a bacterial hydroxycinnamoyl-CoA hydratase-lyase (HCHL) in biomass (Mitra et al., 2002; Eudes et al., 2012). Figure 1 describes the enzymatic reactions catalyzed by HCHL which is expressed in lignifying tissues of engineered plants. HCHL cleaves the side-chain of coumaroyl-CoA and feruloyl-CoA, resulting in an increased amount of unusual C6C1 end-groups in lignin, including 4-hydroxybenzaldehyde, vanillin, syringylaldehyde, and 4-hydroxybenzoic acid (Eudes et al., 2012).
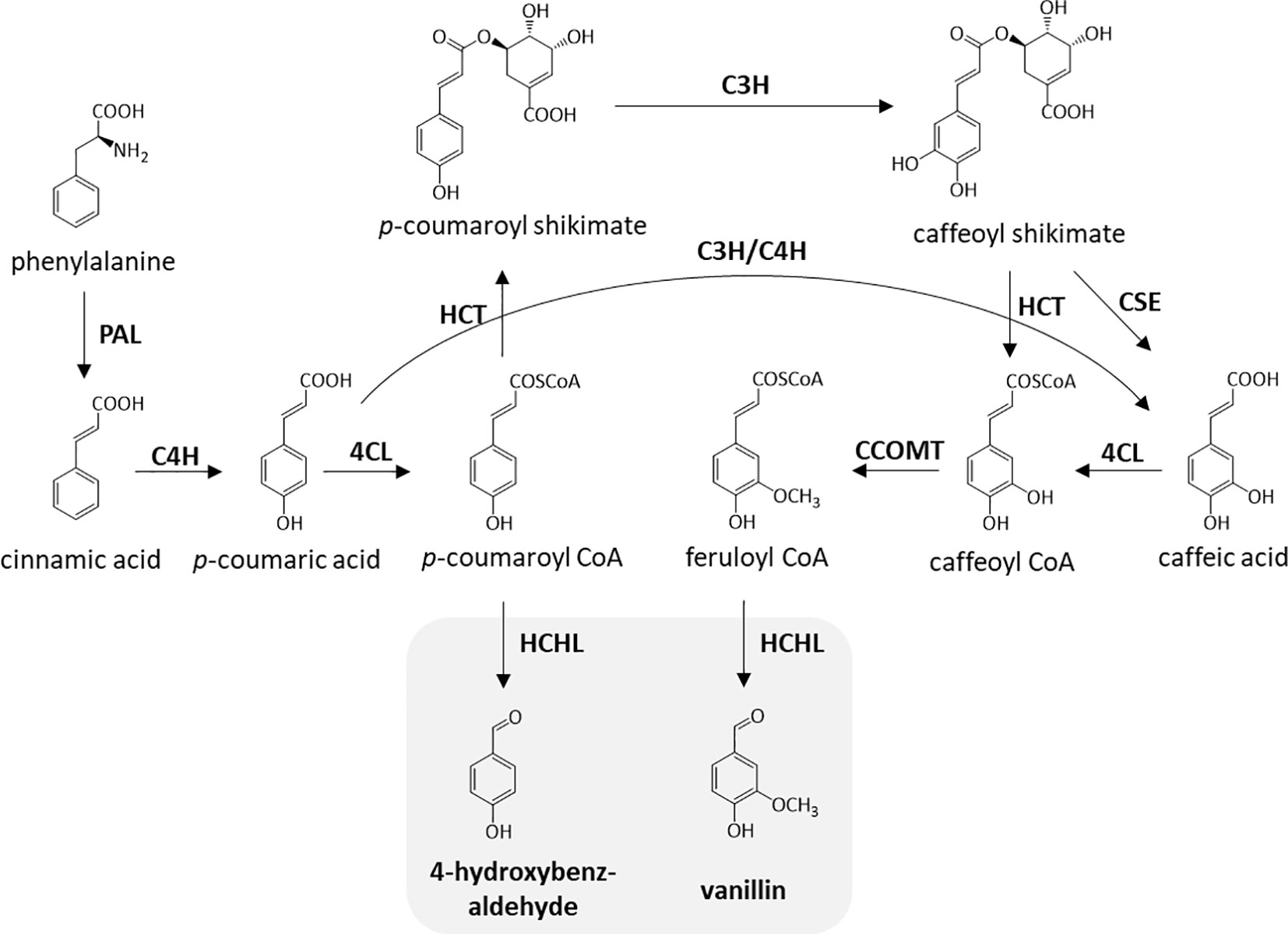
Figure 1 Enzymatic steps catalyzed by hydroxycinnamoyl-CoA hydratase-lyase (HCHL). The two products of HCHL activity (in gray) are converted into several other C6C1 aromatics in plant tissues. PAL, phenylalanine ammonia lyase; C4H, cinnamate 4-hydroxylase; 4CL, 4-coumarate-CoA ligase; HCT, hydroxycinnamoyl-CoA shikimate hydroxycinnamoyl transferase; C3H, coumarate 3-hydroxylase; CSE, caffeoyl shikimate esterase; CCOMT, caffeoyl-CoA O-methyltransferase.
Bio-derived deep eutectic solvents (DESs) have gained considerable attention because of their potential uses in biomass pretreatment and processing. DESs are mixtures of compounds formed by strong intermolecular hydrogen bonds, resulting in a lower melting point than that of any individual component (Dai et al., 2013). As an alternative to organic solvents for biomass pretreatment, DESs exhibit promising solvent properties including high dissolution capability, low vapor pressure, tunability, stabilization of carbohydrates through hydrogen-bond interactions, and compatibility with certain microorganisms (Vigier et al., 2015). Recently, DESs synthesized from lignin-derivable phenolic compounds were found to be effective in lignin removal, and thus resulted in enhanced biomass saccharification efficacy (Kim et al., 2018). Also, renewable DESs prepared from phenolic aldehydes (e.g., vanillin and 4-hydroxybenzaldehyde) with choline chloride (ChCl), integrated with the use of low-recalcitrant engineered biomass via down-regulation of cinnamyl alcohol dehydrogenase (CAD), demonstrated the potential of developing a closed-loop biorefinery process (Kim et al., 2019).
In this work, we employed, as a raw material for DES-assisted pretreatment, the biomass from a previously described plant genetic engineering approach that increases non-conventional C6C1 monomers in lignin via HCHL expression. We also demonstrate the use of renewable DES for pretreating such biomass designed for improved processability.
Materials and Methods
Biomass Material
Arabidopsis thaliana (ecotype Columbia, Col-0) wild type (WT) and line IRX5:HCHL-1 (HCHL) were used in this study (Eudes et al., 2012). Previously, five transgenic lines that express HCHL were isolated and characterized, of which two showed reduced biomass and/or height. Line IRX5:HCHL-1and one with no yield penalty was selected for in-depth characterization and grown on a larger scale for further research (Eudes et al., 2012). Seeds were germinated directly on soil. Growing conditions were: 14 h light/day at 100 µmol/m2/s, 22°C, and 55% humidity. For analyses, stems from mature senesced dried plants harvested without siliques and leaves were ball-milled to a fine powder using a Mixer Mill MM 400 (Retsch Inc., Newtown, PA) and stainless steel balls for 2 min.
Bio-Derived Deep Eutectic Solvent Preparation
All chemicals used for DES synthesis were purchased from Sigma-Aldrich (St. Louis, MO) and used without further purification. DES used in this work was synthesized using ChCl (purity: ≥98%) and vanillin (VAN, purity: 99%) as a hydrogen-bond acceptor and donor, respectively. ChCl and VAN were mixed at 1:2 molar ratio, and then heated to 100°C with continuous stirring until a homogeneous liquid was formed (Kim et al., 2018; Kim et al., 2019). The prepared DES (i.e., ChCl-VAN) was stored in a vacuum oven before use.
Pretreatment and Enzymatic Saccharification
For biomass pretreatment, 5 wt% biomass solution was prepared by mixing 0.2 g of biomass (WT and HCHL line) and 3.8 g ChCl-VAN in a 20 ml pressure tube (Ace Glass, Vineland, NJ). The tube was placed to the preheated oil bath at 80°C. A mild pretreatment temperature used in this work to examine the effect of engineered biomass on pretreatment efficiency (Kim et al., 2019). After 3 h, the pretreated slurry was transferred to a 15 ml centrifuge tube and washed with 50 ml (5 × 10 ml) ethanol and deionized water mixture (2:1, v/v) to separate lignin and remove any residual DES (Kim et al., 2019).
Enzymatic hydrolysis of the pretreated biomass was carried out in 5 ml of 50 mM citrate buffer supplemented with Cellic® CTec3 (266.3 mg/ml) and HTec (234.8 mg/ml) from Novozymes (9:1 ratio) at 10 mg protein per gram solid biomass (solid loading ≈2 wt%). The enzymatic saccharification was performed in an Incubator-Genie™ rotating hybridization oven (Scientific Industries, Bohemia, NY) at 50°C for 72 h.
Topochemical Analysis
Sections of intact stems of mature plants (5 cm from bottom) of unpretreated and DES-pretreated were gradually dehydrated with ethanol-water mixtures with increasing ethanol concentration up to 99.5%. After the ethanol was replaced by acetone, the samples were embedded in the epoxy resin. Ultrathin sections of 80 nm were prepared from embedded samples with a diamond knife mounted on ultramicrotome. Lignin was selectively stained with 1% KMnO4 and the stained section was mounted on copper grids, and observed with a Hitachi H7600 transmission electron microscope (TEM) at an acceleration voltage of 80 keV.
Analytical Methods
Compositional analysis of raw materials and pretreated samples was conducted according to the National Renewable Energy Laboratory protocol (Sluiter et al., 2008), and the results are given in Table 1.
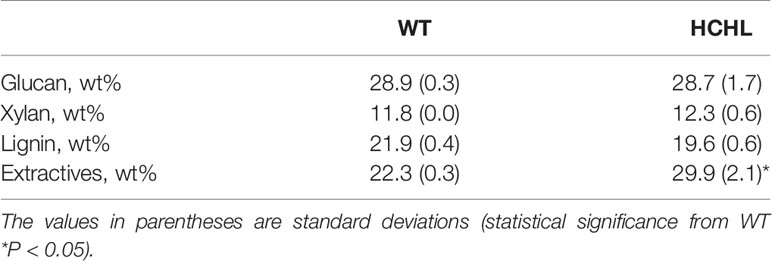
Table 1 Compositional analysis of wild-type (WT) and hydroxycinnamoyl-CoA hydratase-lyase (HCHL) Arabidopsis line.
After pretreatment and saccharification, the hydrolysate was filtered through a 0.45 μm polytetrafluoroethylene (PTFE) syringe filter (VWR, Radnor, PA). The amount of glucose and xylose released was quantified using a YL 9100 high performance liquid chromatography (Young-Lin) equipped with a refractive index detector and a Bio-Rad Aminex HPX-87H ion-exchange column. A solution of 5 mM H2SO4 was used as a mobile phase at a constant flow rate of 0.6 ml/min, and the temperature of column compartment was maintained at 60°C.
Structural information of lignin was analyzed by two-dimensional (2D) 1H-13C heteronuclear single-quantum coherence (HSQC) nuclear magnetic resonance (NMR) spectroscopy. Before the NMR analysis, cellulolytic enzyme lignin was isolated from each line as described elsewhere (Yoo et al., 2016). The prepared lignin dissolved with DMSO-d6 was placed in a 5 mm NMR tube. The NMR spectra were acquired using a Bruker Avance III HD 800 MHz NMR spectrometer equipped with a TCI Cryoprobe with the following acquisition parameters: spectra width 12 ppm in F2 (1H) dimension with 1024 time of domain (acquisition time, 53.2 ms), 200 ppm in F1 (13C) dimension with 512 time of domain (acquisition time, 6.4 ms), a 1.2-s relaxation delay, and 32 scans. Assignment of the HSQC spectra is described elsewhere (Kim and Ralph, 2010; Eudes et al., 2012).
Gel permeation chromatography (GPC) was used to determine the molecular weight distribution of the lignin from the WT control and HCHL transgenic. GPC measurement was conducted using a Waters 2489 GPC system equipped with a UV detector at 270 nm of wavelength. The eluent used for the analysis was tetrahydrofuran, and the three Waters Styragel columns (HR0.5, HR3, and HR4e) were used. All samples were acetylated using 1.0 ml acetic anhydride/pyridine (1:1, v/v) at room temperature for at least 24 h prior to GPC analysis.
Computational Analysis
Density functional theory (DFT) based computational analysis was performed to gain a mechanistic understanding of structurally altered lignin molecules. It is noted, considering the complex structure of lignin, that lignin model compounds with a representative interunit linkage are typically employed for computational studies (Sun et al., 2016). Figure 2 shows five representative β-O-4 dimeric model compounds from typical lignin (1) and from the C6C1-enriched lignin of the HCHL line (2, 3, 4, and 5) tested for the mechanistic study. The geometry optimizations of model compounds were conducted using DFT with the B3LYP and the 6-31+G(2d,2p) basis set. Frequency calculations were also carried out to verify that the optimized structures corresponded to energy minima. In this study, the electrophilicity index, a global reactivity descriptor, was calculated using the electronegativity and the chemical hardness to compare the chemical reactivity of each model structure (Shi et al., 2016; Kim et al., 2019).
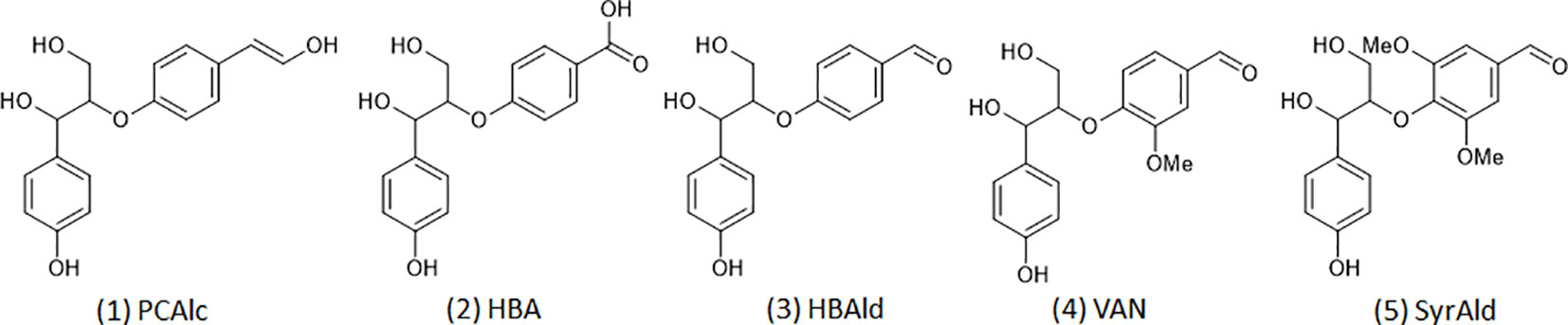
Figure 2 Dimeric model compounds: 1) 4-hydroxylphenylglycerol-β-p-coumaryl alcohol ether (PCAlc), 2) 4-hydroxylphenylglycerol-β-4-oxybenzoic acid ether (HBA), 3) 4-hydroxylphenylglycerol-β-4-oxybenzaldehyde ether (HBAld), 4) 4-hydroxylphenylglycerol-β-vanillin ether (VAN), and 5) 4-hydroxylphenylglycerol-β-syringaldehyde ether (SyrAld).
Statistical Analysis
Analysis of variance (ANOVA) was conducted using R (R Foundation for Statistical Computing, Vienna, Austria) to test the null hypothesis of no statistical difference in saccharification yield (glucose and xylose) between the WT and transgenic HCHL Arabidopsis. Three independent tests were conducted and each sample was analyzed. The null hypothesis was rejected at the 0.05 level.
Results
Structural Analysis of Lignin in Wild-Type and Hydroxycinnamoyl-Coa Hydratase-Lyase Arabidopsis
The isolated lignins were subjected to HSQC NMR analysis to elucidate the structural differences between Arabidopsis WT and the HCHL line. The HSQC spectra, respective peak assignments, and lignin substructures of WT and transgenic biomass are shown in Figure 3. The HSQC spectra were divided broadly into aromatic (δH/δC 6.0–8.0/90–150) and aliphatic (δH/δC 3.0–5.5/50–90) regions. In the aromatic regions of the WT lignin, typical lignin subunits, including p-hydroxyphenyl (H), guaiacyl (G), and syringyl (S) were observed. In the lignin isolated from the HCHL line, signal intensities of guaiacyl (G) and syringyl (S) units were relatively lower. Instead, the aromatic regions of the lignin obtained from the HCHL line had several new correlations compared with WT. For example, the H/C correlations from the oxidized S-unit (SA) at 7.3/107.2 ppm and oxidized H-unit (HA) at 8.0/130.1 ppm were observed, which is in agreement with the previous study (Eudes et al., 2012). It is also noted that the relative S/G levels of both lignins were similar (0.17 for the WT and 0.19 for the HCHL line), although the engineered lignin exhibited some structural changes.
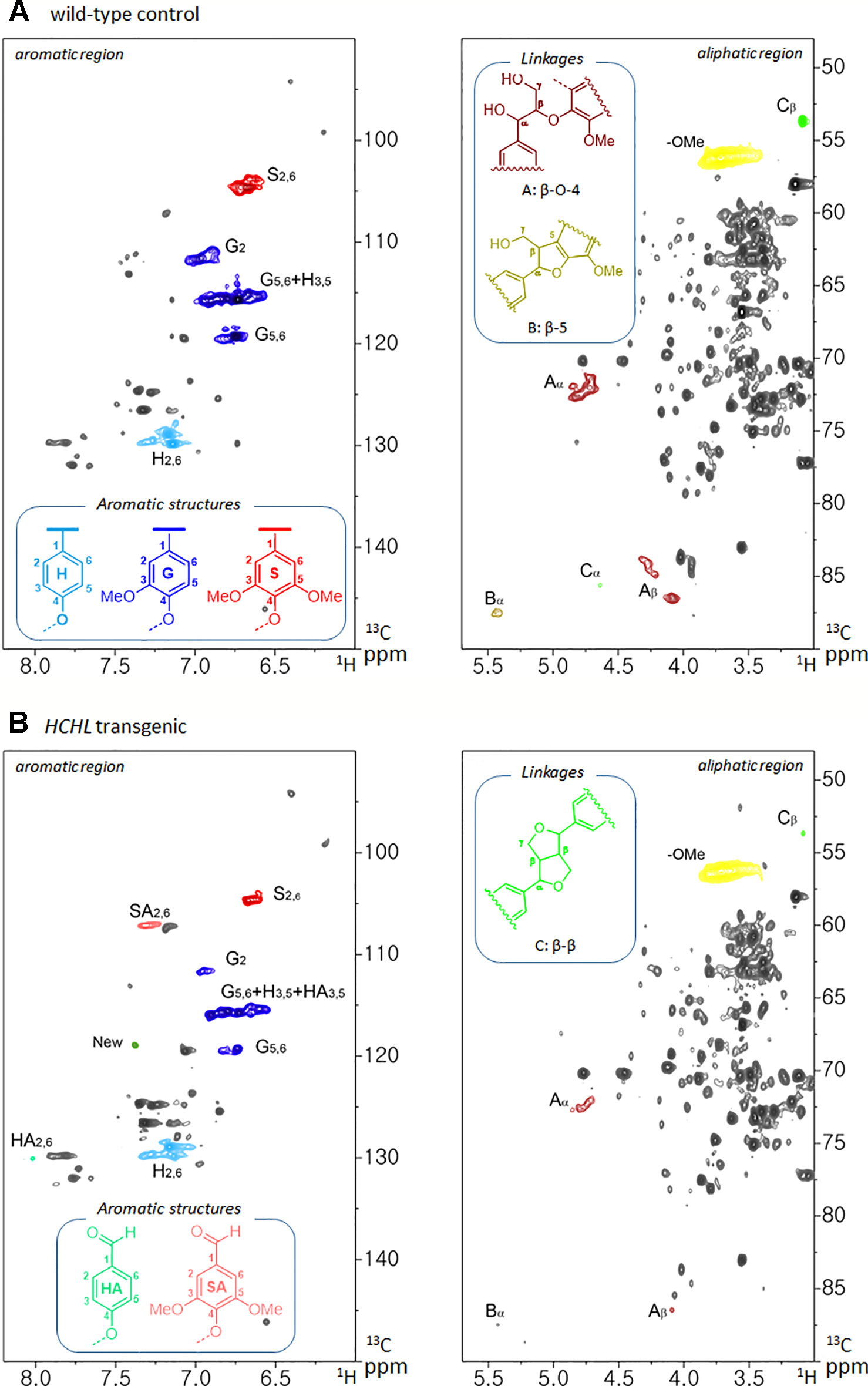
Figure 3 Two-dimensional 1H-13C heteronuclear single-quantum coherence (2D HSQC) nuclear magnetic resonance (NMR) spectra of isolated lignins from Arabidopsis wild-type (WT) (A) and hydroxycinnamoyl-CoA hydratase-lyase (HCHL) line (B) with main structures of lignin subunits (H, p-hydroxyphenyl; G, guaiacyl; S, syringyl; A, β-O-4; B, β-5; and C, β-β).
The aliphatic sidechain region provides information about the structural types and distribution of interunit linkages of the lignin fraction (Kim and Ralph, 2010). HSQC spectra of the WT and engineered lignin showed correlations corresponding to β-ether (β-O-4, substructure A), phenylcoumaran (β-5, substructure B), and resinol (β-β, substructure C) units. As shown in the figure, both lignins isolated from WT and HCHL line exhibited the typical lignin sidechain hydrogen and carbon resonances, although the signal intensities of the engineered lignin were marginally lower than that from WT lignin.
The molecular weight distribution of isolated lignins was measured, and the results are presented in Table 2. As seen, the molecular weight of lignins from WT and HCHL line are marginally different (2,853 Da for WT and 2,973 Da for HCHL). Together with the data from biomass compositional analysis, this result suggests that the expression of the HCHL gene did not significantly affect lignin content, although some non-traditional side-chain-truncated units were observed.

Table 2 Molecular weight distribution of isolated lignins from wild-type (WT) and hydroxycinnamoyl-CoA hydratase-lyase (HCHL) transgenic lines.
Biomass Pretreatment Using Bio-Derived Deep Eutectic Solvent and Enzymatic Saccharification
For biomass pretreatment, the DES (ChCl-VAN) was synthesized at a 1:2 molar ratio. As previously reported, the eutectic point of ChCl-VAN was found to be 55–60°C, which is significantly lower than that of ChCl (302°C) and VAN (81–83°C) (Kim et al., 2019). The pretreatment of WT and transgenic Arabidopsis with ChCl-VAN was conducted at 80°C for 3 h. It is noted that the pretreatment conditions used in this work are relatively milder compared with other conventional pretreatment methods (e.g., dilute acid- or organosolv pretreatment) that are typically conducted between 100 and 250°C with catalyst (Wyman et al., 2005; Agbor et al., 2011; Wyman et al., 2013). Indeed, it was hypothesized that reduced biomass recalcitrance due to the incorporation of side-chain-truncated monomers in lignin of engineered plants would facilitate the release of sugars under low-severity pretreatment conditions. After DES pretreatment, 58.0% (WT) and 49.0% (HCHL) of the biomass was recovered. Compositional analysis of the pretreated biomass was conducted and the amount of glucan, xylan, and lignin was as follows; 40.7% (WT) and 40.6% (HCHL) for glucan, 13.2% (WT) and 13.3% (HCHL) for xylan, and 25.0% (WT) and 18.2% (HCHL) for lignin. It also revealed that lignin removal was found to be 33.6%, and 54.7% from WT and HCHL line, respectively. The pretreated solid was enzymatically hydrolyzed for 72 h at 50°C.
Figure 4 illustrates the sugar yields after ChCl-VAN pretreatment of biomass from the WT and HCHL lines followed by enzymatic saccharification. Glucose release for WT was 102 μg/mg untreated biomass after ChCl-VAN pretreatment, whereas the amount of glucose released from the engineered biomass was significantly higher (+ 34.3%) and reached 137 μg/mg untreated biomass. This clearly indicates that a strategic expression of the HCHL gene in biomass reduced lignin-associated recalcitrance and resulted in enhanced cell wall digestibility. Similarly, xylose release from the HCHL line was 37 μg/mg untreated biomass, which is 68% higher than that from the WT. The enhanced saccharification was also reported from HCHL-engineered Arabidopsis (+78% reducing sugars after hot water pretreatment at 120°C, +31% reducing sugars after 1.2% H2SO4 pretreatment at 120°C, and +71% reducing sugars after 0.25% NaOH pretreatment at 120°C) (Eudes et al., 2012). Although more comprehensive research is required to optimize DES-assisted pretreatment to maximize the sugar release, this work demonstrates that pretreatment using lignin-derived DES is effective on lignocellulosic biomass.
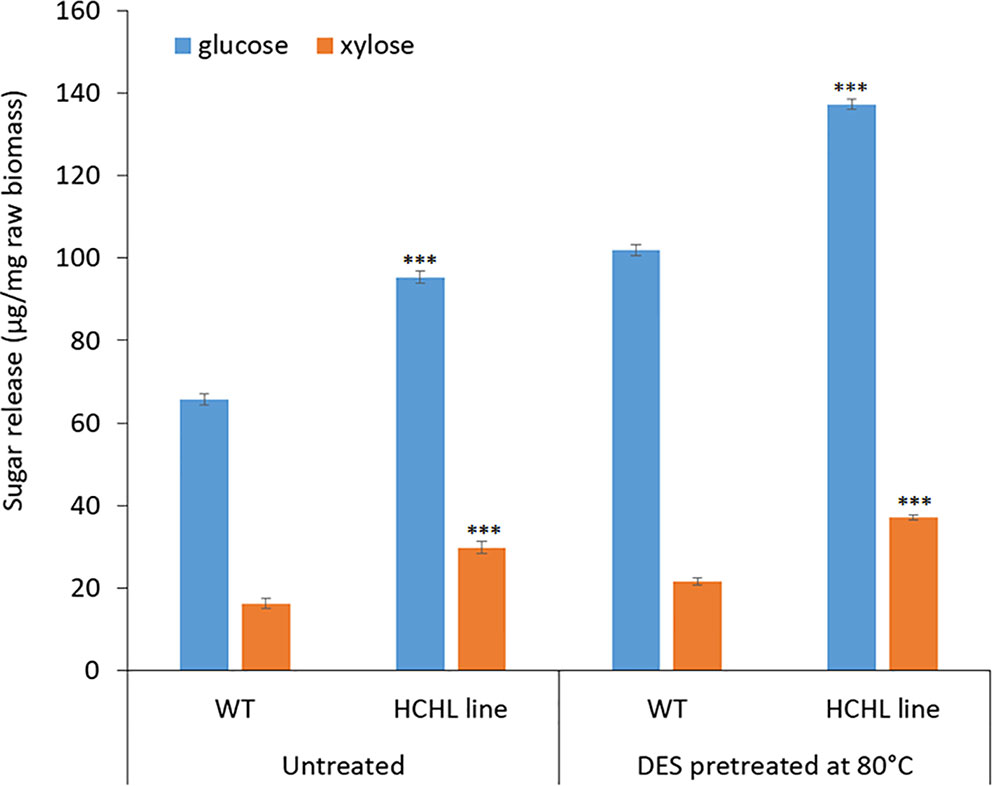
Figure 4 Sugar yield (mean ± standard deviation of three independent tests) from the wild type (WT) and hydroxycinnamoyl-CoA hydratase-lyase (HCHL) lines before and after bio-derived deep eutectic solvent (DES) pretreatment at 80°C (statistical significance from WT ***P < 0.001).
Cell Wall Ultrastructural Morphology
Electron microscopic analysis is widely used to obtain high-resolution information on the lignin distribution in biomass cell walls, which can be visualized by staining with potassium permanganate (KMnO4) (Koch and Schmitt, 2013). Figure 5 shows the TEM micrographs of intact stems from WT (Figure 5A) and HCHL lines (Figure 5D) before and after DES pretreatment at 80°C, in which lignin is selectively stained with 1% KMnO4 and darker areas indicate higher lignin concentration.
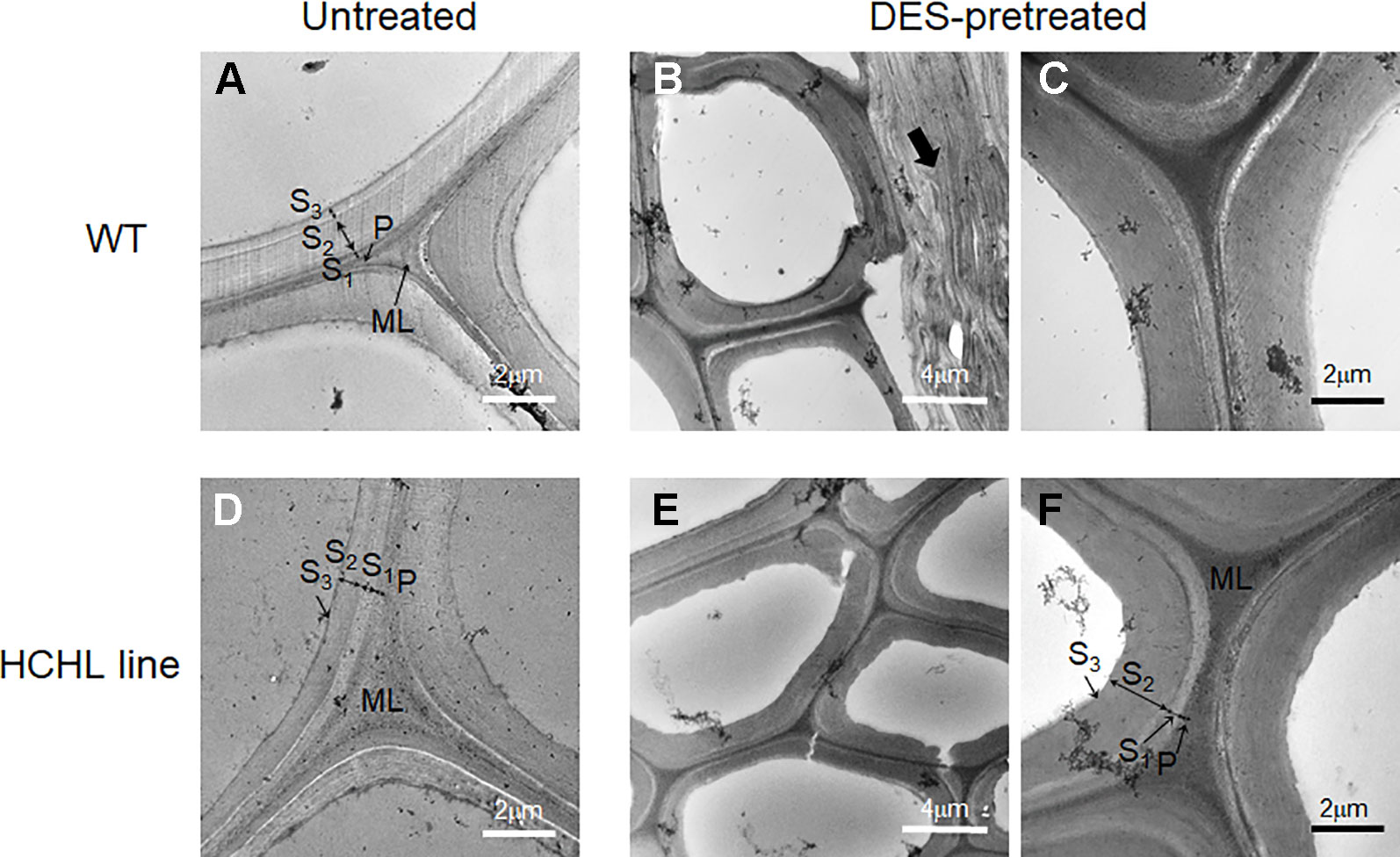
Figure 5 TEM micrographs of cross-sections of wild type (WT) (A–C) and hydroxycinnamoyl-CoA hydratase-lyase (HCHL) lines (D–F) before (A ,D) and after ChCl-VAN pretreatment at 80°C (B, C, E, and F).
Regarding the untreated samples, the different layers of fiber and vessel cell walls from both WT and HCHL lines exhibit general staining, including the middle lamella (ML), primary wall (P), and secondary wall (S1, S2, and S3 layers). Particularly, the middle lamella portion is highly reactive to the staining which indicates higher lignin concentration. Previously, it was reported that the HCHL transgenic has a cell wall structure similar to that of WT using light microscopy and stainings such as toluidine blue, Maule, and phloroglucinol-HCl (Eudes et al., 2012). After the DES pretreatment, TEM images of cell wall structures showed little difference for both stem samples. The middle lamella, primary wall, and secondary wall layers are clearly observed for both genotypes, and the middle lamella portion contains higher lignin content than the secondary wall. Considering that the middle lamella lignin is relatively tougher to be removed compared to that in the primary and secondary cell walls (Terashima and Fukushima, 1988; Doherty et al., 2010), the lignin removal after DES pretreatment likely occurs in the primary and secondary cell walls.
Interestingly, some parts of thin wall cells such as in parenchyma cells were compressed and delamination zones were observed for both samples after DES-assisted pretreatment (arrow in Figure 5B). This is quite surprising because common DESs have negligible vapor pressure, and the pretreatment conditions used in this work are relatively mild. It is speculated that the cell wall delamination during the pretreatment is likely due to lignin dissolution in the DES, resulting in a loosened fiber cell wall with delamination. Changes in cell wall architecture by DES pretreatment, such as delamination, and possibly an increase in the porosity are main factors for enhanced digestibility (Donohoe et al., 2011). Taken together, the TEM images did not show any distinct differences between unpretreated WT and HCHL lines at the cell wall ultrastructure scale, implying that the enhanced efficiency of pretreatment and digestibility in the case of HCHL-engineered plants should be discussed at the molecular scale.
Computational Study
As observed above, the strategic introduction of the HCHL gene into lignifying tissue reduced biomass recalcitrance via incorporation of side-chain-truncated C6C1 lignin monomers, which led to enhanced saccharification yields. It was hypothesized that incorporation of C6C1 monomers makes lignin structure chemically more amenable. To validate this hypothesis, we employed the DFT-based computational study to compute a kinetic quantity of different lignin structures (Socha et al., 2014). Model dimers with aryl-ether linkage found in the typical WT lignin structure and engineered line were used to understand chemical reactivity. Normal β-O-4 structure containing p-coumaryl alcohol end group and those with side-chain-truncated monomers (C6C1: HBA, HBAld, and SyrAld) were computed to find the optimized geometry. Figure 6 depicts the optimized structures and the Cβ-O bond length in the four lignin model dimers. In this work, the Cβ-O bond length was particularly investigated because it is the most frequent linkage found in the lignin macromolecule and also the weakest linkage that is easily cleaved under heated reaction (Kim et al., 2011). As shown in Figure 6, the DFT calculations show that the bond length of Cβ-O in PCAlc dimer is 1.4260 Å. In the case of model dimers with a shorter C1 side chain, the dissociating bond length noticeably elongated. For example, the Cβ-O bond length increased to 1.4297, 1.4340, 1.4426, and 1.4425 Å with HBA, HBAld, VAN, and SyrAld, respectively. This result indicates that it requires a lower bond dissociation energy for the ether linkage when the C6C1 monomers are incorporated into lignin structure.
The electrophilicity index was also calculated to assess the chemical reactivity of dimeric model compounds, and the results are given in Figure 7. The electrophilicity index of common lignin dimeric structure with p-coumaryl alcohol end group (PCAlc) was calculated to be 1.30 eV. Interestingly, the values of four dimeric model compounds with a shortened C1 side-chain monomer end group were considerably higher than that of a conventional lignin structure, representing 1.56 (+20.2%), 1.86 (+43.1%), 1.89 (+45.3%), and 1.83 (+40.8%) with HBA, HBAld, VAN, and SyrAld, respectively. It is evident that lignin dimers with C6C1 monomers have a higher electrophilicity index, suggesting that these structures are chemically more reactive than the typical β-O-4 structure (Shi et al., 2016). The results from DFT computational study support the hypothesis that lignin structure in the HCHL line is more susceptible to biomass pretreatment.
Discussion
Throughout the last decades, cell wall engineering has been a critical approach to reduce biomass recalcitrance by altering lignin structures. Perturbations of genes on the biosynthesis pathway of lignin result in significant structural changes, which allows designing readily tractable biomass structures for the production of biofuels and chemicals (Ralph et al., 2019). The strategic expression of HCHL in lignifying tissues of Arabidopsis resulted in the overproduction of side-chain-truncated (C6C1) lignin monomers incorporated in lignin as end-groups (Eudes et al., 2012). 2D HSQC NMR analysis confirms that isolated lignin from HCHL transgenic biomass contains a significant amount of oxidized C6C1 units. Considering the similar lignin content with a slight difference in molecular weight of isolated lignin, as well as comparable ultrastructural morphologies between the WT and HCHL lines, we propose that engineering the biosynthesis of C6C1 monomers in-planta is an effective approach to modify lignin without any agronomic penalty.
In this work, VAN, an oxidized C6C1 unit found in HCHL plants, was used to synthesize ChCl-VAN, a renewable DES that was previously shown to be effective for biomass pretreatment (Kim et al., 2018). Furthermore, integration of lignin-derived DES with HCHL-engineered biomass offers opportunities to move closer towards achieving a closed-loop biorefinery (Kim et al., 2019). The pretreatment of WT and HCHL biomass using ChCl-VAN showed that lignin removal from transgenic biomass was higher, resulting in enhanced saccharification efficiencies. Considering that lignin content in both samples is quite similar, the improvement of biomass digestibility for the HCHL line is associated with 1) significant structural alteration in lignin, and 2) the higher reactivity of short-side chain lignin monomers.
Regarding the chemical properties of lignin derived from HCHL plants, DFT-based calculation revealed higher reactivity for structures corresponding to C6C1 monomers linked to lignin. Moreover, lignin with side-chain-truncated monomers requires less energy for the cleavage of β-aryl ether bond, which is typically necessary for lignin fractionation during biomass pretreatment. Previously, lignins rich in H-units were computationally found to be more reactive than other types of lignins (G- and S-unit) (Shi et al., 2016), and transgenic biomass consisting of H-lignin yielded higher sugars upon saccharification (Bonawitz et al., 2014). As discussed above, the more reactive nature of C6C1 monomers with aldehyde or carboxylic acid functionalities that form lignin end-groups contributed to the increased pretreatment efficiency followed by higher saccharification yield.
It is noted that although both empirical and computational results undoubtedly support the hypothesis, more work is necessary to gain a far-reaching insight of structural modification resulting from the expression of HCHL. However, the pretreatment of transgenic biomass using a bio-derivable DES offers opportunities to operate reactors with reduced amount of energy and chemicals, which is highly desirable in developing a sustainable bioeconomy.
Conclusions
Plant cell wall engineering has been developed for the production of biofuels and renewable chemicals. In this study, we strategically expressed the HCHL gene in Arabidopsis to reduce the degree of lignin polymerization via incorporation of side-chain-truncated monomers in lignin polymer ends. The transgenic Arabidopsis yielded higher levels of fermentable sugars compared to WT plants when pretreated with the lignin-derived DES at mild conditions. The results of this work clearly indicate that interfering with the lignin biosynthetic pathway has the potential to improve the conversion of biomass into biofuels and other intermediate products. Together with the development of tailor-made biomass that is more amenable to chemical processes, biomass pretreatment using a renewable DES could make the biofuel industry more economically feasible in the future.
Data Availability Statement
The datasets analyzed in this article are not publicly available. Requests to access the datasets should be directed to KHK, a3dhbmdob2tpbUBraXN0LnJlLmty.
Author Contributions
KHK conceived and designed the experiments. KHK, YW, MT, and AE performed the experiments. KHK, CGY, CSK, and JS analyzed the data of the experiments. KHK, MT, AE, and CGY drafted manuscript. All authors read and approved the final manuscript.
Funding
This work is supported by the Korea Institute of Science and Technology–The University of British Columbia Biorefinery on-site laboratory project. NMR analysis is supported by SUNY ESF and NIH Shared Instrumentation Grant 1S10OD012254. This work was part of the DOE Joint BioEnergy Institute (http://www.jbei.org) supported by the U. S. Department of Energy, Office of Science, Office of Biological and Environmental Research, through contract DE-AC02-05CH11231 between Lawrence Berkeley National Laboratory and the U.S. Department of Energy.
Conflict of Interest
The authors declare that the research was conducted in the absence of any commercial or financial relationships that could be construed as a potential conflict of interest.
References
Agbor, V. B., Cicek, N., Sparling, R., Berlin, A., Levin, D. B. (2011). Biomass pretreatment: fundamentals toward application. Biotechnol. Adv. 29:6, 675–685. doi: 10.1016/j.biotechadv.2011.05.005
Bhagia, S., Muchero, W., Kumar, R., Tuskan, G. A., Wyman, C. E. (2016). Natural genetic variability reduces recalcitrance in poplar. Biotechnol. biofuels 9:(1), 106. doi: 10.1186/s13068-016-0521-2
Bonawitz, N. D., Chapple, C. (2013). Can genetic engineering of lignin deposition be accomplished without an unacceptable yield penalty? Curr. Opin. Biotechnol. 24:(2), 336–343. doi: 10.1016/j.copbio.2012.11.004
Bonawitz, N. D., Kim, J. I., Tobimatsu, Y., Ciesielski, P. N., Anderson, N. A., Ximenes, E., et al. (2014). Disruption of mediator rescues the stunted growth of a lignin-deficient Arabidopsis mutant. Nature 509:(7500), 376–380. doi: 10.1038/nature13084
Boudet, A. M. (2007). Evolution and current status of research in phenolic compounds. Phytochemistry 68:(22–24), 2722–2735. doi: 10.1016/j.phytochem.2007.06.012
Chabannes, M., Barakate, A., Lapierre, C., Marita, J. M., Ralph, J., Pean, M., et al. (2001). Strong decrease in lignin content without significant alteration of plant development is induced by simultaneous down-regulation of cinnamoyl CoA reductase (CCR) and cinnamyl alcohol dehydrogenase (CAD) in tobacco plants. Plant J. 28:(3), 257–270. doi: 10.1046/j.1365-313X.2001.01140.x
Chen, F., Dixon, R. A. (2007). Lignin modification improves fermentable sugar yields for biofuel production. Nat. Biotechnol. 25:7, 759. doi: 10.1038/nbt1316
Dai, Y. T., van Spronsen, J., Witkamp, G. J., Verpoorte, R., Choi, Y. H. (2013). Natural deep eutectic solvents as new potential media for green technology. Anal. Chim. Acta 766, 61–68. doi: 10.1016/j.aca.2012.12.019
Dale, B. E., Anderson, J. E., Brown, R. C., Csonka, S., Dale, V. H., Herwick, G., et al. (2014). Take a closer look: biofuels can support environmental, economic and social goals. Environ. Sci. Technol. 48:(13), 7200–7203. doi: 10.1021/es5025433
Demirbaş, A. (2001). Biomass resource facilities and biomass conversion processing for fuels and chemicals. Energy Convers. Manage. 42:(11), 1357–1378. doi: 10.1016/S0196-8904(00)00137-0
Ding, S.-Y., Liu, Y.-S., Zeng, Y., Himmel, M. E., Baker, J. O., Bayer, E. A. (2012). How does plant cell wall nanoscale architecture correlate with enzymatic digestibility? Science 338:(6110), 1055–1060. doi: 10.1126/science.1227491
Doherty, T. V., Mora-Pale, M., Foley, S. E., Linhardt, R. J., Dordick, J. S. (2010). Ionic liquid solvent properties as predictors of lignocellulose pretreatment efficacy. Green Chem. 12:11, 1967–1975. doi: 10.1039/c0gc00206b
Donohoe, B. S., Vinzant, T. B., Elander, R. T., Pallapolu, V. R., Lee, Y. Y., Garlock, R. J., et al. (2011). Surface and ultrastructural characterization of raw and pretreated switchgrass. Bioresour. Technol. 102:(24), 11097–11104. doi: 10.1016/j.biortech.2011.03.092
Eudes, A., George, A., Mukerjee, P., Kim, J. S., Pollet, B., Benke, P. I., et al. (2012). Biosynthesis and incorporation of side-chain-truncated lignin monomers to reduce lignin polymerization and enhance saccharification. Plant Biotechnol. J. 10:(5), 609–620. doi: 10.1111/j.1467-7652.2012.00692.x
Eudes, A., Sathitsuksanoh, N., Baidoo, E. E. K., George, A., Liang, Y., Yang, F., et al. (2015). Expression of a bacterial 3-dehydroshikimate dehydratase reduces lignin content and improves biomass saccharification efficiency. Plant Biotechnol. J. 13:(9), 1241–1250. doi: 10.1111/pbi.12310
Himmel, M. E. (2009). Biomass recalcitrance: deconstructing the plant cell wall for bioenergy (Oxford: Wiley-Blackwell).
Isikgor, F. H., Becer, C. R. (2015). Lignocellulosic biomass: a sustainable platform for the production of bio-based chemicals and polymers. Polym. Chem. 6:(25), 4497–4559. doi: 10.1039/c5py00263j
Kim, K. H., Kim, C. S. (2018). Recent Efforts to Prevent Undesirable Reactions From Fractionation to Depolymerization of Lignin: Toward Maximizing the Value From Lignin. Front. Energy Res. 6, 92. doi: 10.3389/fenrg.2018.00092
Kim, H., Ralph, J. (2010). Solution-state 2D NMR of ball-milled plant cell wall gels in DMSO-d(6)/pyridine-d(5). Org. Biomol. Chem. 8:(3), 576–591. doi: 10.1039/b916070a
Kim, S., Chmely, S. C., Nimos, M. R., Bomble, Y. J., Foust, T. D., Paton, R. S., et al. (2011). Computational study of bond dissociation enthalpies for a large range of native and modified lignins. J. Phys. Chem. Lett. 2:(22), 2846–2852. doi: 10.1021/jz201182w
Kim, K. H., Dutta, T., Ralph, J., Mansfield, S. D., Simmons, B. A., Singh, S. (2017). Impact of lignin polymer backbone esters on ionic liquid pretreatment of poplar. Biotechnol. Biofuels 10. doi: 10.1186/s13068-017-0784-2
Kim, K. H., Dutta, T., Sun, J., Simmons, B., Singh, S. (2018). Biomass pretreatment using deep eutectic solvents from lignin derived phenols. Green Chem. 20:(4), 809–815. doi: 10.1039/c7gc03029k
Kim, K. H., Eudes, A., Jeong, K., Yoo, C. G., Kim, C. S., Ragauskas, A. (2019). Integration of renewable deep eutectic solvents with engineered biomass to achieve a closed-loop biorefinery. Proc. Nat. Acad. Sci. U.S.A. 116:(28), 13816–13824. doi: 10.1073/pnas.1904636116
Koch, G., Schmitt, U. (2013). “Topochemical and electron microscopic analyses on the lignification of individual cell wall layers during wood formation and secondary changes” in Cellular aspects of wood formation. Ed. Fromm, J.(Heidelberg: Springer), 41–69. doi: 10.1007/978-3-642-36491-4_2
Maity, S. K. (2015). Opportunities, recent trends and challenges of integrated biorefinery: Part I. Renewable Sustainable Energy Rev. 43, 1427–1445. doi: 10.1016/j.rser.2014.11.092
Mitra, A., Mayer, M. J., Mellon, F. A., Michael, A. J., Narbard, A., Parr, A. J., et al. (2002). 4-Hydroxycinnamoyl-CoA hydratase/lyase, an enzyme of phenylpropanoid cleavage from Pseudomonas causes formation of C-6-C-1 acid and alcohol glucose conjugates when expressed in hairy roots of Datura stramonium L. Planta 215:(1), 79–89. doi: 10.1007/s00425-001-0712-2
Ragauskas, A. J., Beckham, G. T., Biddy, M. J., Chandra, R., Chen, F., Davis, M. F., et al. (2014). Lignin valorization: improving lignin processing in the biorefinery. Science 344:(6185), 1246843. doi: 10.1126/science.1246843
Ralph, J., Lapierre, C., Boerjan, W. (2019). Lignin structure and its engineering. Curr. Opin. Biotechnol. 56, 240–249. doi: 10.1016/j.copbio.2019.02.019
Renders, T., Van den Bosch, S., Koelewijn, S. F., Schutyser, W., Sels, B. F. (2017). Lignin-first biomass fractionation: the advent of active stabilisation strategies. Energy Environ. Sci. 10:(7), 1551–1557. doi: 10.1039/c7ee01298e
Schilmiller, A. L., Stout, J., Weng, J. K., Humphreys, J., Ruegger, M. O., Chapple, C. (2009). Mutations in the cinnamate 4-hydroxylase gene impact metabolism, growth and development in Arabidopsis. Plant J. 60:(5), 771–782. doi: 10.1111/j.1365-313X.2009.03996.x
Shi, J., Pattathil, S., Parthasarathi, R., Anderson, N. A., Kim, J. I., Venketachalam, S., et al. (2016). Impact of engineered lignin composition on biomass recalcitrance and ionic liquid pretreatment efficiency. Green Chem. 18:(18), 4884–4895. doi: 10.1039/c6gc01193d
Sluiter, A., Hames, B., Ruiz, R., Scarlata, C., Sluiter, J., Templeton, D., et al. (2008). “Determination of structural carbohydrates and lignin in biomass-laboratory analytical procedure (LAP)” (Colorado, USA: National Renewable Energy Laboratory). Report number TP-510-42618).
Socha, A. M., Parthasarathi, R., Shi, J., Pattathil, S., Whyte, D., Bergeron, M., et al. (2014). Efficient biomass pretreatment using ionic liquids derived from lignin and hemicellulose. Proc. Nat. Acad. Sci. U. S. A. 111:(35), E3587–E3595. doi: 10.1073/pnas.1405685111
Sun, J., Dutta, T., Parthasarathi, R., Kim, K. H., Tolic, N., Chu, R. K., et al. (2016). Rapid room temperature solubilization and depolymerization of polymeric lignin at high loadings. Green Chem. 18:(22), 6012–6020. doi: 10.1039/c6gc02258h
Terashima, N., Fukushima, K. (1988). Heterogeneity in Formation of Lignin.11. An Autoradiographic Study of the Heterogeneous Formation and Structure of Pine Lignin. Wood Sci. Technol. 22:(3), 259–270. doi: 10.1007/Bf00386021
Thomas, V. A., Kothari, N., Bhagia, S., Akinosho, H., Li, M., Pu, Y., et al. (2017). Comparative evaluation of Populus variants total sugar release and structural features following pretreatment and digestion by two distinct biological systems. Biotechnol. Biofuels 10:1, 292. doi: 10.1186/s13068-017-0975-x
Vigier, K. D., Chatel, G., Jerome, F. (2015). Contribution of deep eutectic solvents for biomass processing: opportunities, challenges, and limitations. Chemcatchem 7:8, 1250–1260. doi: 10.1002/cctc.201500134
Wilkerson, C. G., Mansfield, S. D., Lu, F., Withers, S., Park, J. Y., Karlen, S. D., et al. (2014). Monolignol ferulate transferase introduces chemically labile linkages into the lignin backbone. Science 344:(6179), 90–93. doi: 10.1126/science.1250161
Wyman, C. E., Dale, B. E., Elander, R. T., Holtzapple, M., Ladisch, M. R., Lee, Y. (2005). Comparative sugar recovery data from laboratory scale application of leading pretreatment technologies to corn stover. Bioresour. Technol. 96:(18), 2026–2032. doi: 10.1016/j.biortech.2005.01.018
Wyman, C. E., Dale, B. E., Balan, V., Elander, R. T., Holtzapple, M. T., Ramirez, R. S., et al. (2013). “Comparative performance of leading pretreatment technologies for biological conversion of corn stover, poplar wood, and switchgrass to sugars” in Aqueous pretreatment plant biomass for biological and chemical conversion to fuels and chemicals, Ed. Wyman, C. E. (Chichester: Wiley), 239–259. doi: 10.1002/9780470975831.ch12
Xu, B., Escamilla-Treviño, L. L., Sathitsuksanoh, N., Shen, Z., Shen, H., Percival Zhang, Y. H., et al. (2011). Silencing of 4-coumarate: coenzyme A ligase in switchgrass leads to reduced lignin content and improved fermentable sugar yields for biofuel production. New Phytol. 192:(3), 611–625. doi: 10.1111/j.1469-8137.2011.03830.x
Keywords: lignocellulosic biomass, lignin engineering, hydroxycinnamoyl-CoA hydratase-lyase, bioenergy, saccharification
Citation: Kim KH, Wang Y, Takada M, Eudes A, Yoo CG, Kim CS and Saddler J (2020) Deep Eutectic Solvent Pretreatment of Transgenic Biomass With Increased C6C1 Lignin Monomers. Front. Plant Sci. 10:1774. doi: 10.3389/fpls.2019.01774
Received: 18 October 2019; Accepted: 19 December 2019;
Published: 29 January 2020.
Edited by:
Jin Zhang, Oak Ridge National Laboratory (DOE), United StatesReviewed by:
Rajeev Kumar, University of California, Riverside, Unites StatesKewei Zhang, Zhejiang Normal University, China
Copyright © 2020 Kim, Wang, Takada, Eudes, Yoo, Kim and Saddler. This is an open-access article distributed under the terms of the Creative Commons Attribution License (CC BY). The use, distribution or reproduction in other forums is permitted, provided the original author(s) and the copyright owner(s) are credited and that the original publication in this journal is cited, in accordance with accepted academic practice. No use, distribution or reproduction is permitted which does not comply with these terms.
*Correspondence: Kwang Ho Kim, a3dhbmdob2tpbUBraXN0LnJlLmty