- 1World Vegetable Center Korea Office, Wanju-gun, Jeollabuk-do, South Korea
- 2Organic Agricultural Division, National Institute of Agricultural Sciences, RDA, Wanju-gun, Jeollabuk-do, South Korea
Modern agriculture has become heavily dependent on chemical fertilizers, which have caused environmental pollution and the loss of soil fertility and sustainability. Microalgae and plant growth-promoting bacteria (PGPB) have been identified as alternatives to chemical fertilizers for improving soil fertility. This is because of their biofertilizing properties, through the production of bioactive compounds (e.g., phytohormones, amino acids, and carotenoids) and their ability to inhibit plant pathogens. Although treatment based on a single species of microalgae or bacteria is commonly used in agriculture, there is growing experimental evidence suggesting that a symbiotic relationship between microalgae and bacteria synergistically affects each other’s physiological and metabolomic processes. Moreover, the co-culture/combination treatment of microalgae and bacteria is considered a promising approach in biotechnology for wastewater treatment and efficient biomass production, based on the advantage of the resulting synergistic effects. However, much remains unexplored regarding the microalgal–bacterial interactions for agricultural applications. In this review, we summarize the effects of microalgae and PGPB as biofertilizing agents on vegetable cultivation. Furthermore, we present the potential of the microalgae–PGPB co-culture/combination system for the environmentally compatible production of vegetables with improved quality.
Introduction
Ecological Problems Caused by Chemical Fertilizers in Agriculture
High amounts of chemical fertilizers have been used to obtain high product yields with increased cultivation efficiency in agriculture. However, the excessive use of chemical fertilizers frequently causes severe environmental damage, such as water, soil, and air pollution (Savci, 2012). Moreover, the excessive use of chemical fertilizers leads to soil acidification and hardening, which decrease root vigor with reduced respiration. The population of beneficial microorganisms is also reduced by this practice, resulting in a loss of soil fertility and a high incidence of root diseases (Chandini et al., 2019). In particular, nitrogen (N) fertilizers are absorbed by crops in reactive forms such as nitrate (NO3), ammonia (NH3), and nitrogen oxides (NOx). These reactive forms can be sustainably produced by soil microbes, but with chemical fertilizers, excessive amounts remain in the soil, flow to the groundwater, and even contribute to the production of greenhouse gases such as nitrous oxide (N2O) (Choudhury and Kennedy, 2005; Giles, 2005).
The Advantage of Biofertilizers in Agriculture
Biofertilizers have been recommended as an alternative to chemical fertilizers in order to avoid the problems caused by chemical fertilizers in agriculture (Mahanty et al., 2017). Biofertilizers are preparations containing living or dormant cells, which have the advantage of growth-promoting functions in crops. This is performed through the production of phytohormones and/or useful substances/biochemicals, thereby enabling the development of ecofriendly and sustainable agriculture (Kumar, 2018). In general, biofertilizers play a significant role in the decomposition of organic matter, which aids mineralization within the soil, consequently increasing the availability of nutrients for plants and improving crop yield (Rodrìguez and Fraga, 1999).
Moreover, the application of biofertilizers can increase the quantity and biodiversity of useful bacteria, such as plant growth-promoting rhizobacteria (PGPR) belonging to Azotobacter, Bacillus, Burkholderia, Pantoea, Pseudomonas, Serratia, and Streptomyces (Verma et al., 2019; Gou et al., 2020). Currently, it is believed that the co-evolution of plant–microbe interactions has allowed some of the bacteria to be facultative intracellular endophytes (Bulgarelli et al., 2013), among which are PGPR that have beneficial effects on plants through direct or indirect pathways. For example, some PGPR strains affect plant growth by synthesizing phytohormones, metabolizing them, and/or acting on hormone biosynthesis in plants, while others produce substances that work against soil-borne pathogens (Beneduzi et al., 2012). In the last few decades, as the interest of consumers toward safe agricultural products grew, it became important to exploit beneficial microbes as biofertilizers for use in food safety practices and sustainable crop production (Shi et al., 2011).
The Role of Microorganisms in Plant Growth
Different microbial species coexist in the soil and have a variety of beneficial effects on plant growth promotion and biological control. These microbes usually improve soil fertility by providing nutrients, such as carbon, nitrogen, phosphorus, potassium, trace elements, vitamins, and amino acids, and making them accessible for plants. In doing so, they promote growth by mediating various activities, such as nitrogen fixation and phosphate and potassium solubilization (Güneş et al., 2014; Bagyalakshmi et al., 2017). These microbes are able to release plant growth-regulating substances, such as phytohormones, and also have a suppressive effect on plant diseases by producing/secreting antibiotics and/or secondary metabolites that work against pathogens (Yoshihisa et al., 1989; Sessitsch et al., 2004; Jung et al., 2006). These microorganisms are known as plant growth-promoting bacteria (PGPB), which generally belong to Pseudomonas, Azospirillum, Rhizobium, and Bacillus (Walsh et al., 2001; Esitken et al., 2010; Ahemad and Kibret, 2014).
Characteristics of Microalgae
In addition to PGPB, some microalgae species have also been used to promote plant growth, yield, and fruit quality (Guo et al., 2020). Microalgae are typically microscopic algae, which range in sizes between micrometers and tens of micrometers, depending on the species. It has been estimated that 200,000–800,000 species exist (Ebenezer et al., 2011), but only around 40,000–50,000 species have been described thus far (Suganya et al., 2016). They are photosynthetic eukaryotes, which do not have roots, stems, or leaves, unlike higher plants, and range from unicellular to multicellular species (Singh and Saxena, 2015). Many species of microalgae have been utilized for the removal of contaminants from wastewater or sewage, as well as the conversion of said sewage into an effluent that can be reused for various purposes. This is because of their ability to absorb and metabolize nutrients and heavy metals in the water such as cadmium, lead, zinc, and copper (Rajamani et al., 2007). Under certain growth conditions, microalgae produce and accumulate large amounts of lipids, proteins, and carbohydrates in the cell. The lipid content of microalgae is higher than that of other biofuels, usually between 20% and 50% of the cell’s dry weight but can reach up to 70% (Duan et al., 2012; Sun et al., 2018). Because of this, they have also been regarded as a suitable candidate for third-generation biofuel feedstock. A variety of studies have been conducted to maximize the potential of microalgae as biofuels, including the applications of genetically modified microalgae; high-density mass culturing; and efficient processes for cultivation, harvest, and extraction (Ghasemi et al., 2012).
In recent years, an increasing amount of research has been conducted to study the effects of microalgal–bacterial co-culture/combination systems for wastewater treatment and biomass production (Ramanan et al., 2016; Qi et al., 2018). However, investigations into microalgal–bacterial co-culture/combination systems for crop production remain largely unexplored. Hence, the current review describes the effects of microalgae and bacteria as biofertilizer agents in vegetable cultivation. Furthermore, it aims to propose the potential of the microalgae–PGPB co-culture/combination system to improve the production and the quality of vegetables.
Evaluation of Microalgae as Biofertilizers for Vegetable Production
Effect of Microalgae as Biofertilizers on Crop Cultivation
Many studies have indicated that microalgae are increasingly being employed, not only in bioremediation and biofuel production but also in agriculture. This is because a wide range of bioactive compounds, including plant growth-promoting substances (such as phytohormones), amino acids, carotenoids, and phycobilins, can be produced from microalgae. These compounds contribute to high productivity in agricultural crops by promoting plant growth and conferring resistance against pathogens with minimal environmental costs (Stirk et al., 2013b; Michalak and Chojnacka, 2014).
Microalgal extracts contain phytohormones such as auxin, cytokinin, abscisic acid, ethylene, and gibberellin, which play key roles in the regulation of growth and development. Accordingly, microalgal extracts can be used as renewable sources of plant biostimulation (Stirk et al., 2013a; Romanenko et al., 2015). Auxin is an essential regulator of various plant developmental processes, such as cell division and elongation. Indole-3-acetic acid (IAA) and indole-3-butanoic acid (IBA), the two dominant types of auxins in microalgae, can both stimulate and inhibit the growth and metabolism of higher plants (Hashtroudi et al., 2013). Cytokinins are involved in many physiological processes in plants, including root and shoot development, leaf senescence, nutrient mobilization, and seed germination (Ha et al., 2012), while gibberellins are able to promote cell division, trigger the accumulation of pigments and proteins, and stimulate cell elongation and expansion (Sponsel and Hedden, 2010). Ethylene is a gaseous plant hormone that renders tolerance to abiotic stresses such as drought, low temperatures, and high salinity, as well as biotic stresses such as the penetration of pathogens (Pierik et al., 2006). Thus, phytohormones not only affect plant growth and development but also activate plant defense systems against plant pathogens via interacting/cross-talking networks among them (Checker et al., 2018).
Various Microalgae Species Used for Important Vegetable Cultivation
Based on the increasing number of studies demonstrating that microalgae have the ability to promote plant growth and defend against plant pathogens, several microalgae species are being used in the cultivation of important vegetables (Table 1). In general, Chlorella vulgaris, Chlorella fusca, and Spirulina platensis have been used for tomato, cucumber, onion, lettuce, and pepper cultivation with a view to promote their production with marketable quality (Kim et al., 2018; Bumandalai, 2019; Rachidi et al., 2020).
Tomato (Solanum lycopersicum L.)
Tomato is the most popular home garden vegetable. It is a rich source of vitamins, minerals, and flavonoids such as quercetin (Nicola et al., 2009). Strategies for improving the productivity and nutritional quality of tomatoes are of great interest to producers (Dorais et al., 2008). In tomato fruits, treatment with Nannochloropsis oculata has been found to induce 33% and 36% higher levels of sugar and carotenoid content, respectively, compared to those treated with inorganic fertilizer under greenhouse conditions (Coppens et al., 2016). It was also found that among young tomato plants grown in phytotrons, the number of nodes, dry weight, and length of shoots had significantly increased from treatment with polysaccharide extracts from Arthrospira platensis, Dunaliella salina, and Porphyridium sp. when compared to the untreated control. Tomato plants treated with polysaccharide extracts also showed an increase in the activities of nitrate reductase (NR) and NAD-glutamate dehydrogenase (NAD-GDH), key enzymes for nitrogen assimilation and amino acid synthesis, as well as phenylalanine ammonia lyase (PAL) and β-1,3-glucanase, which activate plant defenses against pathogens (Kobayashi et al., 1995; Vera et al., 2011; Chen et al., 2017; Farid et al., 2019; Rachidi et al., 2020). Microalgal polysaccharides can elevate the activity of NADPH-synthesizing enzymes, shifting conditions to be more conducive to reduction in the intracellular redox state, which may favor photosynthesis and cell division. In addition, the levels of ascorbate (AsA) content and ascorbate peroxidase (APX) activity, which play central roles in photosynthesis and abiotic stress tolerance, have been shown to increase in polysaccharide-treated plants (Castro et al., 2012; Smirnoff, 2018; Chanda et al., 2019; Figure 1). Moreover, Garcia-Gonzalez and Sommerfeld (2016) assessed the properties of the microalgae Acutodesmus dimorphus as a biofertilizer and/or biostimulant. Under greenhouse conditions, foliar application of the algal extract at a concentration of 3.75 g ml–1 on tomato plants caused an increase in the number of branches and flowers per plant (Garcia-Gonzalez and Sommerfeld, 2016).
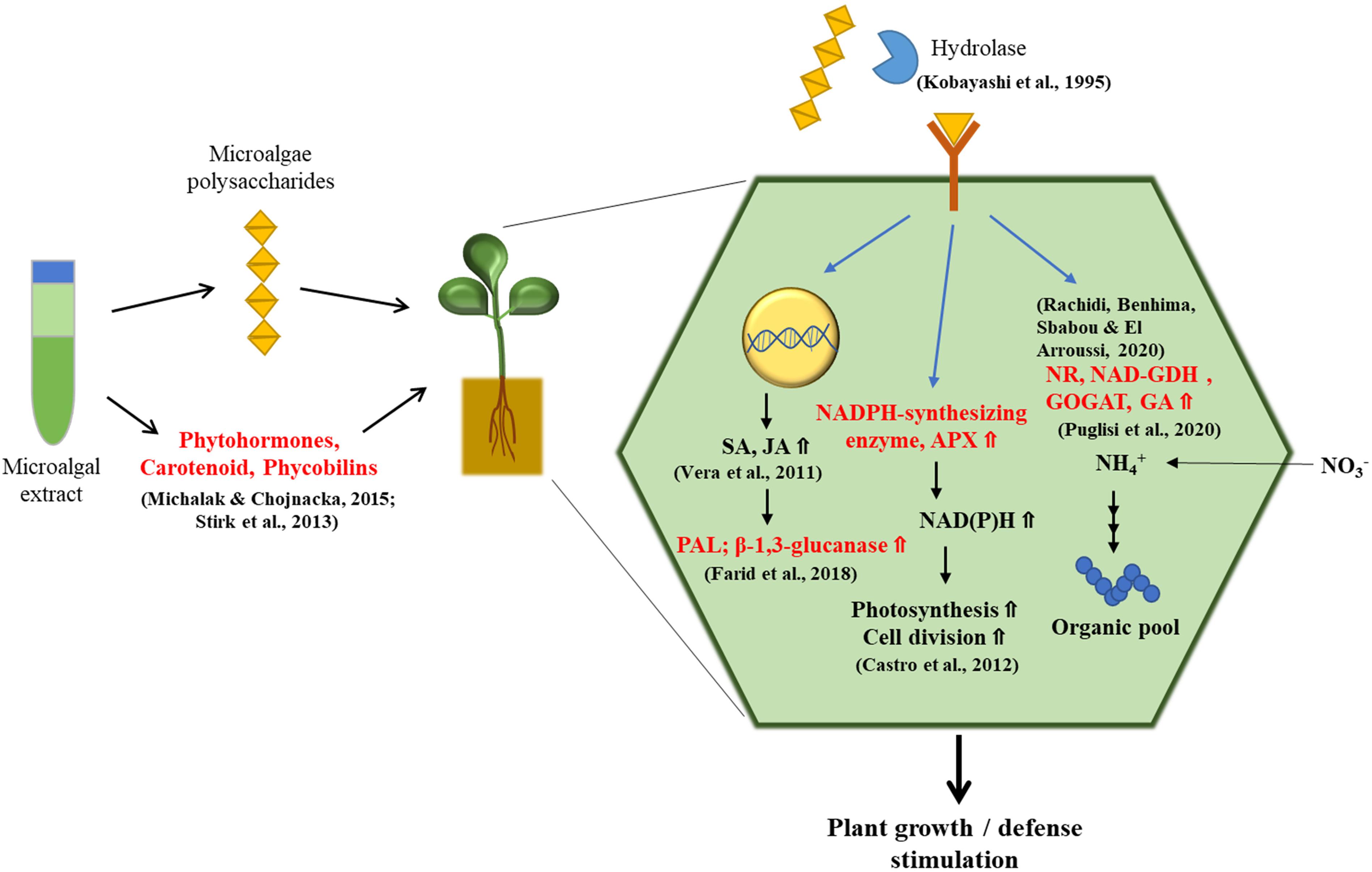
Figure 1. The treatment of microalgae extracts stimulates plant growth and defense system. Microalgal extracts contain many plant growth-promoting compounds such as polysaccharides, phytohormones, carotenoids, and phycobilins, which have the ability to stimulate the plant growth and defense system. Large polysaccharides from microalgal extracts are broken down into smaller fragments of oligosaccharides by hydrolytic enzymes. Oligosaccharides are perceived by plant’s membrane receptor and have a significant stimulatory effect on the plant growth by regulating activities of enzymes such as nitrate reductase (NR) and NAD-glutamate dehydrogenase (NAD-GDH) related with nitrate assimilation. Moreover, polysaccharides can increase activity of NADPH-synthesizing enzymes, ascorbate peroxidase (APX), and the amount of ascorbate (AsA), which are associated with photosynthesis, fundamental cellular metabolism, and cell cycle. Defense pathways are also stimulated by polysaccharides in plant cells: polysaccharide treatment upregulates the expression of genes involved in salicylic acid (SA) and jasmonic acid (JA) signaling pathways, resulting in increased activities of β-1,3-glucanase and phenylalanine ammonia lyase (PAL) linked to plant defense system.
Onion (Allium cepa L.)
Onion is one of the most economically important vegetable crops consumed primarily because of its ability to enhance the flavor of other foods (Kandoliya et al., 2015). Field experiments were performed at the experimental farm of Annamalai University, India, in 2016–2017 to determine the efficacy of microalgae, such as C. vulgaris and S. platensis, as biofertilizers on onion plants. Treatment comprising of cow dung with S. platensis on onion plants resulted in higher amounts of micro- and macronutrients available in the soil, including nitrogen, phosphate, potassium, zinc, and manganese. There was also an increase in the levels of biochemicals in the onion, such as total soluble sugars, total phenols, and free amino acids, along with improved growth parameters, when compared to the untreated control. In addition to this, higher amounts of minerals were observed in onion plants treated with “cow dung + S. platensis” and “cow dung + C. vulgaris” (Dineshkumar et al., 2020). Furthermore, compared to the untreated control, treatment with a mixture of Scenedesmus subspicatus and humic acid synergistically increased the onion root length by 39% and the concentration of soluble proteins by 37% (Gemin et al., 2019).
Cucumber (Cucumis sativus L.)
Cucumber is also an important vegetable crop (Huang et al., 2009) that is cultivated in many countries within both temperate and tropical zones (Tatlioglu, 1993). When vegetable crops such as cucumber, tomato, and squash were treated with Anabaena vaginicola and Nostoc calcicola, they showed increased growth factors when compared to the untreated control. These growth factors included root length, fresh and dry weight of roots, and plant height. Auxins such as IBA, which are involved in root development in plants, were also shown to be available with this treatment in the range of 1.275–2.958 μg g–1 dry weight with a trace amount of IAA in microalgal cells (Shariatmadari et al., 2013).
Eggplant (Solanum melongena L.)
Eggplant is ranked among the top 10 vegetables in terms of oxygen radical absorbance capacity due to its high content of total phenolics (Cao et al., 1996). Dias et al. (2016) conducted field and laboratory experiments to evaluate the growth, yield, and postharvest quality of eggplants with different concentrations of S. platensis solutions for foliar application at the Centro de Ciências e Tecnologia de Alimentos (CCTA), Brazil. This experiment was performed between October 2014 and January 2015. The results revealed that the number of fruits significantly increased in plants treated with low concentrations of this treatment (10, 15, 25, and 35 g l–1). This is likely due to the greater abundance of polypeptides, amino acids, and hormones in the microalgal species acting as plant growth promoters when compared to the levels in the untreated control (Dias et al., 2016).
Pepper (Capsicum annuum L.)
Pepper is a popular commercial vegetable and spice crop that is valued for its fruit color, flavor, pungency, and nutrient content (Kumar et al., 2006). Treatment with extracts of D. salina increased the germination rate by 69% and the root length of bell peppers by 24% in 25 mM NaCl. Meanwhile, Phaeodactylum tricornutum treatment was found to reduce the production of superoxide radicals and lipid peroxidation triggered by salt stress, when compared to the untreated control (Guzmán-Murillo et al., 2013).
Lettuce (Lactuca sativa L.)
Treatment of lettuce seedlings with Scenedesmus quadricauda extract promoted plant growth and induced the accumulation of chlorophyll, carotenoids, and total protein content in the plant cell. In addition, the leaf dry weights were positively affected by the treatment with S. quadricauda extract, reaching an increase of approximately 26% when compared to the untreated control. From a metabolic point of view, the treated leaves revealed increased enzyme activity levels of glutamate synthase (GOGAT), glutamine synthase (GS), citrate synthase (CS), malate dehydrogenase (MDH), and PAL, which are key enzymes associated with nitrogen (Gupta et al., 2012), carbon (Schiavon et al., 2008), and phenylpropanoid metabolism (Hyun et al., 2011). This suggested that the positive effect on the growth of lettuce most likely occurs through the stimulation of the metabolic pathways of carbon, nitrogen, and phenylpropanoid (Puglisi et al., 2020).
Evaluation of Bacteria as Biofertilizers for Vegetable Production
The Effect of Bacteria as Biofertilizers on Crop Cultivation
Bacteria are a major class of microorganisms that function as decomposers and recyclers in the soil. In doing so, these microbes contribute to the processes of nutrient cycling, energy flow, and bioconversion in the ecosystem. Most agricultural production systems are dependent on soil bacterial biomass pools, which facilitate quick responses to diverse environmental changes (Pankhurst et al., 1996). Microbial inoculums called effective microorganisms (EM), containing mixed cultures of beneficial and naturally occurring microorganisms, can increase the microbial diversity of the soil ecosystem. They consist mainly of lactic acid bacteria, photosynthetic bacteria, yeast, Actinomyces, and fermenting fungi (Balogun et al., 2016). Among these effective microorganisms, PGPB form specific symbiotic relationships with plants and directly promote plant growth by facilitating resource acquisition and/or modulating plant hormone levels (Glick, 1995). The application of PGPB to vegetable cultivations can prevent the excessive use of chemical fertilizers by up to 30%, thereby reducing production costs and pollution (Geries and Elsadany, 2021). PGPB treatments also have the ability to improve host plant defenses against soil-borne pathogens by producing antibiotics such as 2,4-diacetylphloroglucinol (2,4-DAPG), pyoluteorin (PLT), pyrrolnitrin, and phenazine-1-carboxylate (Bangera and Thomashow, 1999; Duffy and Défago, 1999).
Various Bacterial Species Used for Vegetable Cultivation
It has been adequately demonstrated that many species of bacteria are able to promote the growth and development of vegetables and control pathogens through various mechanisms, one of which include the production/release of inhibitory substances, allowing target crops to be disease resistant (Table 2). Representative commercially available bacterial strains are of the genus Bacillus spp.; these include B. amyloliquefaciens, B. subtilis, B. cereus, B. licheniformis, and B. pumilus, which produce various compounds for the biocontrol of plant pathogens and the growth promotion of vegetables such as tomato, cucumber, onion, lettuce, and pepper (Gutiérrez-Mañero et al., 2001; Compant et al., 2005; Cawoy et al., 2011; Yuan et al., 2012; Nie et al., 2017). Silo-Suh et al. (1994) reported that B. cereus UW85 suppresses the damping-off disease caused by Phytophthora medicaginis in alfalfa through the production of two fungistatic antibiotics, zwittermicin A and kanosamine (Silo-Suh et al., 1994).
In addition, Serratia liquefaciens and Pseudomonas putida are known to generate N-acyl-L-homoserine lactone (AHL) signaling molecules, which enhance the systemic resistance of tomato plants against the leaf fungal pathogen, Alternaria alternata (Schuhegger et al., 2006). Recently, it was shown that two phosphate-solubilizing bacteria (PSB), Pantoea agglomerans and Burkholderia anthina, contributed to improved growth traits of tomato plants with a higher level of phosphorous content in the soil, when compared to the untreated control, under greenhouse conditions (Walpola and Yoon, 2013). The ability of Azotobacter chroococcum and Pseudomonas fluorescens to improve vegetative growth and yield in onion production through the production of IAA, siderophores, and the solubilization of tricalcium phosphate (TCP) has also been demonstrated (Tarakhovskaya et al., 2007; Čolo et al., 2014).
Relationship Between Microalgae and Bacteria
Microalgae–Bacteria Interactions
In natural environments, microalgae and bacteria coexist and interact with each other. As a result, they demonstrate both beneficial (Unnithan et al., 2014) and harmful/toxic relationships (Doucette, 1995). The relationship between microalgae and bacteria is greatly dependent on the species and the environmental conditions (Doucette, 1995; Croft et al., 2005; Mujtaba and Lee, 2016). In actuality, both microalgae and bacteria can produce growth factors, and/or exotoxins, that promote and/or inhibit growth and development. In the beneficial relationship, microalgae enhance bacterial growth by providing photosynthetic oxygen and dissolved organic matter such as organic carbon, calcium carbonate, and 2,3-dihydroxypropane-1-sulfonate (DHPS) (Wolfaardt et al., 1994; Borde et al., 2003; Cooper and Smith, 2015). Generally speaking, the photosynthetic oxygen produced by microalgae or cyanobacteria (blue-green algae) is used as an electron acceptor in the bacterial degradation of organic matter. In turn, bacteria support photoautotrophic growth of their partners by providing carbon dioxide and other stimulatory means (Subashchandrabose et al., 2011). In a similar way, bacteria are also able to offer a selective advantage to microalgae for enhanced growth by providing micronutrients such as B-vitamins. These vitamins act as co-factors that are required for enzyme activity in the central cellular metabolism (Croft et al., 2005). Moreover, microalgae can acquire nutrients such as inorganic carbon, nitrogen, phosphorus, and sulfate generated from organic matter, through the activities of extracellular bacterial enzymes. However, in harmful/toxic relationships, microalgae can inhibit bacterial activity by releasing antibacterial metabolites and increasing the pH, the dissolved oxygen concentration, and the temperature of the culture medium (Naviner et al., 1999; Schumacher et al., 2003; Ribalet et al., 2008).
Both microalgae and PGPB have the ability to promote plant growth by producing polysaccharides and phytohormones, such as auxin and cytokinin. Furthermore, they can prevent plant diseases by stimulating defense systems and secreting antifungal enzymes and antibiotics (Figure 2; Najdenski et al., 2013; Stirk et al., 2013b; Walpola and Yoon, 2013; Michalak and Chojnacka, 2014; Cordero et al., 2016).
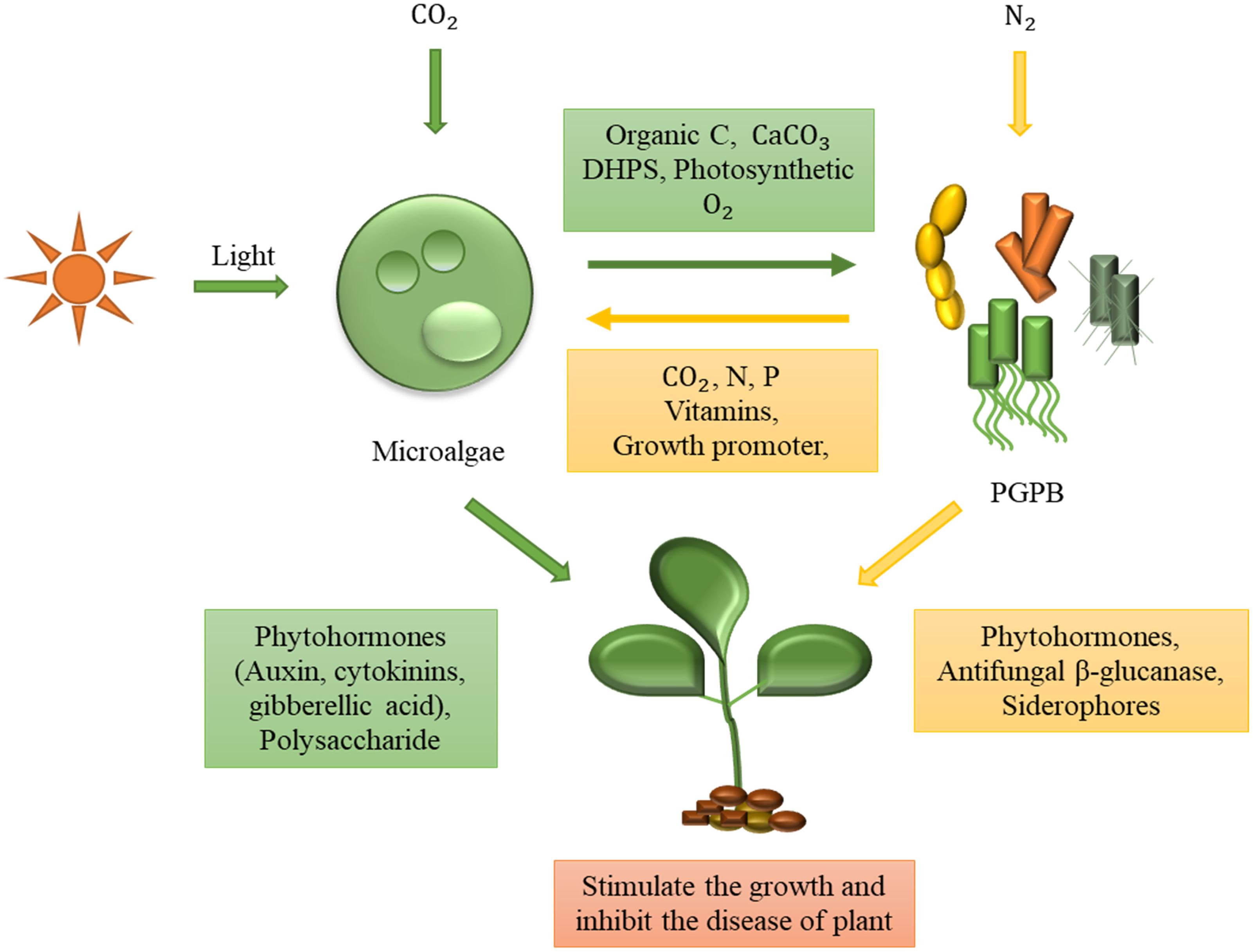
Figure 2. Symbiotic interactions between microalgae and plant growth-promoting bacteria (PGPB) for sustainable cultivation of plants. Microalgae and PGPB in the symbiotic relationship cooperate with each other by efficient exchange of nutrients. Microalgae supply photosynthetic oxygen, organic carbon, calcium carbonate, and 2 3-dihydroxypropane-1-sulfonate (DHPS) to bacteria in exchange for micronutrients (i.e., vitamins) and macronutrients (i.e., nitrogen and phosphorus). Both microalgae and bacteria can enhance plant growth by producing phytohormones and other growth stimulants. Moreover, they are also able to inhibit plant diseases by using their distinct disease-suppressive mechanisms.
Reciprocal Influence During the Co-culture/Combination of Microalgae and Bacteria
As microalgae have been widely used in various industries, extensive studies have shown that when microalgae and bacteria are co-cultured, there is an increase in microalgal productivity in the production of useful substances such as total lipids, carbohydrates, and chlorophylls (Table 3).
Amavizca et al. (2017) demonstrated that two PGPB strains, Azospirillum brasilense Cd and B. pumilus ES4, have similar effects on the growth of the green microalga Chlorella sorokiniana UTEX 2714, without any form of physical contact between them. The two PGPB remotely enhanced the growth rate of microalgae up to six-fold and induced an increase in the total amounts of lipids, carbohydrates, and chlorophyll a in the microalgal cells. These beneficial effects have been ascribed to the volatile compounds produced by the bacteria, which include CO2 (Amavizca et al., 2017). In addition, plant growth-promoting bacteria, such as A. brasilense, have the potential to significantly increase C. sorokiniana growth rates through a variety of mechanisms, including the production of IAA (Peng et al., 2020). Croft et al. (2005) reported that bacterial strains of Halomonas sp. have a growth-enhancing effect on the microalga Amphidinium operculatum through the provision of vitamin B12. In addition to this, the presence of algal extracts also leads to the promotion of bacterial growth with upregulated vitamin B12 biosynthesis, displaying positive reciprocity (Croft et al., 2005). Sometimes, a single bacterial strain can induce different effects on the growth and proliferation of distinct microalgae. For example, Muricauda sp. was found to promote the growth of Tetraselmis chuii and Cylindrotheca fusiformis, but drastically inhibited the growth of Nannochloropsis gaditana (Han et al., 2016).
Co-Culturing/Combination of Microalgae–Bacteria
Microalgae–Bacteria Co-culture for Wastewater Treatment and Biomass Production
The co-culture system of bacteria and microalgae has been mainly used for wastewater treatment and biomass production. Mujtaba et al. (2017) investigated the efficiency of nutrient removal (i.e., ammonium, phosphate, etc.) and the reduction of chemical oxygen demand (COD) from wastewater, using the symbiotic co-culture of P. putida and immobilized C. vulgaris. In general, symbiotic co-culture systems facilitate the simultaneous removal of a greater variety of nutrients from wastewater compared to monocultures (Mujtaba et al., 2017). Ogbonna et al. (2000) found that it is impossible to remove all nutrients such as acetate, propionate, ammonia, nitrate, and phosphorus from wastewater at once using monocultures of Rhodobacter sphaeroides, C. sorokiniana, and S. platensis, whereas these nutrients can all be removed simultaneously by using the co-culture system (Ogbonna et al., 2000). Moreover, the symbiotic co-culture of A. brasilense and Scenedesmus sp. has been successfully applied to biofuel production with higher biomass volume. This indicates that the symbiotic co-culture has the potential to increase microalgal colony size, and the fatty acid content inside biofuels, in nitrogen-deficient media (Contreras et al., 2019). The combination of cyanobacteria/microalgae and bacteria can more efficiently detoxify organic and inorganic pollutants and remove nutrients from wastewater compared to using either of them alone.
Soils and aquatic systems contaminated by heavy metals have also become a serious issue in crop production because of the risks associated with food contamination. Microalgae have the ability to detoxify and volatilize heavy metals via microalgal metabolism and high levels of metal binding (Yu et al., 1999). Microalgae form metal-binding peptides (organometallic complexes) such as class III metallothionein (MtIII) (Perales-Vela et al., 2006), which facilitate appropriate control of the cytoplasmic concentration of heavy metals, thereby preventing or neutralizing the potential toxicity caused by heavy metals (Kaplan, 2013; Priya et al., 2014). The application of microalgae, which can bioabsorb and biotransform arsenate (As) in rice fields, reduces the availability of As to plants, thereby rendering the food grains safe for human consumption (Debnath and Bhadury, 2016). In the case of the algal–bacterial synergistic interactions, a higher removal efficiency of heavy metals was achieved with the addition of bacterial inoculum, which enhanced algal growth with additional CO2 and organic compounds provided by the bacteria (Unnithan et al., 2014; Mubashar et al., 2020). Furthermore, algae can also be recycled as biofertilizing agents (Guo et al., 2020).
Potential of Microalgae–Bacteria Co-cultures/Combination for Vegetable Cultivation
There are two distinct application methods for the simultaneous use of microalgae and bacteria, one is co-culturing the microbes from the beginning and the other is preparing a mixture containing microalgae/microalgal extract and bacteria gained from each pure culture (combination).
The combined application of specific bacteria, which are growth promoters in plants and/or biocontrol agents against plant pathogens, can lead to the synergy necessary for ideal vegetable cultivation. When Pantoea ananatis and P. fluorescens (CPP-2) were co-cultured, IAA production and phosphate solubilization were higher than those from either strain alone. Additionally, the co-culture of CPP-2 showed promotion effects on root and shoot elongation of pea (Pisum sativum) plants when compared to the culture of an individual strain (Anwar et al., 2019). Moreover, the combination of P. putida WCS358 and RE8 was shown to enhance the suppression of Fusarium wilt in radish by approximately 50%, when compared to the untreated control, while that of the single-strain treatments was reduced by 30%. Even when one strain failed to suppress disease with a single application, the combination treatment still exhibited a suppressive effect against the disease. This implies that the reinforced resistance, brought on the combination application, is likely due to the additive/combinational effect of different disease-suppressive mechanisms (Boer et al., 2003).
Even though the application of microorganisms, via co-culture/combination treatment, is more effective for vegetable production (Minuto et al., 1993; Raupach and Kloepper, 1998), the use of a single microbial species for vegetable cultivation is commonly observed in practical situations. Although the activity of one microbial species is relatively narrow in scope from a practical point of view, it is easier to obtain related patents and/or safety certificates for the use of a single microbial species in agricultural and commercial sectors with obvious effects in a short period of time. In addition to this, the application of a single microbial species makes the processes of cultivation, harvesting, and extraction simpler and easier (Sylvia et al., 2005). However, in the case of co-culture/combination, the ability to suppress plant pathogens and promote plant growth is much more effective than that of the monoculture system because the microbes in the co-culture/combination system promote the growth of plants and/or prevent pathogens by different mechanisms (Spadaro and Gullino, 2005).
Several important practical examples of mixed treatments of cyanobacteria/microalgae–bacteria have been reported for crop cultivation (Table 4).
In 2012, the effects of combined treatments of cyanobacterial strains—CR1, CR2, and CR3 (Anabaena laxa, Anabaena sp., and Anabaena oscillarioides)—and bacterial strains—PR3, PR7, and PR10 (Providencia sp., Brevundimonas sp., and Ochrobactrum sp.)—on rice crop yield and C-N sequestration in soil were first reported in a pot experiment (Prasanna et al., 2012).
The synergistic efficacy of the combination of freshwater algae (C. vulgaris) and plant growth-promoting bacteria (B. licheniformis, Bacillus megatherium, Azotobacter sp., Azospirillum sp., and Herbaspirillum sp.) on yields and nutritional values of leaf and romaine lettuces has also been observed. The combined application of microalgal–bacterial preparation led to a significant increase of the romaine and leaf lettuce weight by 12.9% and 22.7%, respectively. Of note, total carotenoids in amounts 26.7% higher than in the controls were detected in the treated romaine lettuce under stress conditions during summer (Kopta et al., 2018).
Recently, Horácio et al. (2020) evaluated the effects of co-inoculation with a diazotrophic cyanobacterium (Anabaena cylindrica, Ana), Rhizobium (R. tropici + R. freirei, Riz), and A. brasilense (Azo) on the development of the common bean under greenhouse conditions. Grain production in the plants co-inoculated with Ana + Riz + Azo and fertilized with N (100 kg N ha–1) was 84.4% and 86.3% higher than that of untreated controls, respectively. This indicates that N fertilization can be replaced by co-inoculation with selected cyanobacterial–bacterial strains (Horácio et al., 2020). In addition, Gavilanes et al. (2020) conducted two field experiments to assess the efficacy of co-inoculation of A. cylindrica with A. brasilense on the yield performance of four maize cultivars in two locations (Londrina and Faxinal in Paraná, Brazil). They found that the co-inoculation of A. cylindrica and A. brasilense resulted in a yield that increased by 9% (967 kg ha–1) in Londrina and 23% (1,744 kg ha–1) in Faxinal, compared to the uninoculated control (Gavilanes et al., 2020).
It was also found that the growth and productivity of onion plants with the combination treatment were promoted when compared to those with either single-agent treatment. This supports the result of biochemical analyses that state that extracts of both S. platensis and Pseudomonas stutzeri possess bioactive compounds such as HCN, NH3, IAA, and amino acids that have stimulatory effects on plant growth and quality (Geries and Elsadany, 2021).
Additionally, after culturing C. fusca and B. amyloliquefaciens cc178 separately, they were combined in a ratio of 2:1 and used to irrigate tomato roots. It was found that rooting of fine roots was promoted and the plant growth, yield, and soluble liquid sugar content in fruits had significantly increased with the combination treatment when compared to each single treatment (unpublished data). Therefore, the combined application of microalgae and PGPB can exert beneficial effects on the yield and quality of vegetable crops.
Conclusion and Future Directions
Microalgae and bacteria have received great interest as biofertilizers in ecofriendly vegetable production. Until now, monoculture systems using certain agricultural microorganisms have been highlighted to improve the yield and quality of agricultural products. However, co-culture/combination systems of microorganisms can be more effective in enhancing microbial diversity in the soil, resistance to plant diseases, and productivity of vegetable crops. Therefore, further investigations to uncover the molecular mechanisms underlying the effect of microalgae–bacteria co-culture/combination on vegetable growth, and/or plant disease suppression, will be necessary for the extension of sustainable agriculture.
Author Contributions
All authors listed have made a substantial, direct and intellectual contribution to the work, and approved the final version of the manuscript for publication.
Funding
This research was financially supported in part by the World Vegetable Center Korea Office budget (WKO #10000379) and the long-term strategic donors to the World Vegetable Center: Republic of China (Taiwan), UK aid from the United Kingdom Government, United States Agency for International Development (USAID), Australian Centre for International Agricultural Research (ACIAR), Germany, Thailand, Philippines, South Korea, and Japan.
Conflict of Interest
The authors declare that the research was conducted in the absence of any commercial or financial relationships that could be construed as a potential conflict of interest.
Acknowledgments
The authors are grateful to Dr. Srinivasan Ramasamy for careful reading of the manuscript.
References
Ahemad, M., and Kibret, M. (2014). Mechanisms and applications of plant growth promoting rhizobacteria: current perspective. J. King Saud Univ. Sci. 26, 1–20. doi: 10.1016/j.jksus.2013.05.001
Albarracín Orio, A. G., Brücher, E., and Ducasse, D. A. (2016). A strain of Bacillus subtilis subsp. subtilis shows a specific antagonistic activity against the soil-borne pathogen of onion Setophoma terrestris. Eur. J. Plant Pathol. 144, 217–223. doi: 10.1007/s10658-015-0762-0
Amavizca, E., Bashan, Y., Ryu, C.-M., Farag, M. A., Bebout, B. M., and de-Bashan, L. E. (2017). Enhanced performance of the microalga Chlorella sorokiniana remotely induced by the plant growth-promoting bacteria Azospirillum brasilense and Bacillus pumilus. Sci. Rep. 7:41310. doi: 10.1038/srep41310
Anwar, M. S., Paliwal, A., Firdous, N., Verma, A., Kumar, A., and Pande, V. (2019). Co-culture development and bioformulation efficacy of psychrotrophic PGPRs to promote growth and development of Pea (Pisum sativum) plant. J. Gen. Appl. Microbiol. 65, 88–95. doi: 10.2323/jgam.2018.05.007
Bagyalakshmi, B., Ponmurugan, P., and Balamurugan, A. (2017). Potassium solubilization, plant growth promoting substances by potassium solubilizing bacteria (KSB) from southern Indian Tea plantation soil. Biocatal. Agricult. Biotechnol. 12, 116–124. doi: 10.1016/j.bcab.2017.09.011
Balogun, R. B., Ogbu, J. U., Umeokechukwu, E. C., and Kalejaiye-Matti, R. B. (2016). “Effective micro-organisms (EM) as sustainable components in organic farming: principles, applications and validity,” in Organic Farming for Sustainable Agriculture, ed. D. Nandwani (Cham: Springer), 259–291. doi: 10.1007/978-3-319-26803-3_12
Bangera, M. G., and Thomashow, L. S. (1999). Identification and characterization of a gene cluster for synthesis of the polyketide antibiotic 2,4-diacetylphloroglucinol from Pseudomonas fluorescens Q2-87. J. Bacteriol. 181, 3155–3163. doi: 10.1128/jb.181.10.3155-3163.1999
Beneduzi, A., Ambrosini, A., and Passaglia, L. M. (2012). Plant growth-promoting rhizobacteria (PGPR): their potential as antagonists and biocontrol agents. Genet. Mol. Biol. 35, 1044–1051. doi: 10.1590/s1415-47572012000600020
Boer, M., Bom, P., Kindt, F., Keurentjes, J., Sluis, I., Loon, L., et al. (2003). Control of Fusarium wilt of radish by combining Pseudomonas putida strains that have different disease-suppressive mechanisms. Phytopathology 93, 626–632. doi: 10.1094/PHYTO.2003.93.5.626
Borde, X., Guieysse, B., Delgado, O., Muñoz, R., Hatti-Kaul, R., Nugier-Chauvin, C., et al. (2003). Synergistic relationships in algal–bacterial microcosms for the treatment of aromatic pollutants. Bioresource Technol. 86, 293–300. doi: 10.1016/S0960-8524(02)00074-3
Bulgarelli, D., Schlaeppi, K., Spaepen, S., Van Themaat, E. V. L., and Schulze-Lefert, P. (2013). Structure and functions of the bacterial microbiota of plants. Annu. Rev. Plant Biol. 64, 807–838. doi: 10.1146/annurev-arplant-050312-120106
Bumandalai, O. (2019). Effect of Chlorella vulgaris as a biofertilizer on germination of tomato and cucumber seeds. Int. J. Aquat. Biol. 7, 95–99. doi: 10.22034/ijab.v7i2.582
Cao, G., Sofic, E., and Prior, R. L. (1996). Antioxidant capacity of tea and common vegetables. J. Agricult. Food Chem. 44, 3426–3431. doi: 10.1021/jf9602535
Castro, J., Vera, J., González, A., and Moenne, A. (2012). Oligo-carrageenans stimulate growth by enhancing photosynthesis, basal metabolism, and Cell Cycle in Tobacco Plants (var. Burley). J. Plant Growth Regulat. 31, 173–185. doi: 10.1007/s00344-011-9229-5
Cawoy, H., Bettiol, W., Fickers, P., and Ongena, M. (2011). “Bacillus-based biological control of plant diseases,” in Pesticides in the Modern World – Pesticides Use and Management, ed. M. Stoytcheva (Rijeka: InTech), 273–302. doi: 10.5772/17184
Chanda, M.-J., Merghoub, N., and El Arroussi, H. (2019). Microalgae polysaccharides: the new sustainable bioactive products for the development of plant bio-stimulants? World J. Microbiol. Biotechnol. 35:177. doi: 10.1007/s11274-019-2745-3
Chandini, Kumar, R., Kumar, R., and Prakash, O. (2019). “The impact of chemical fertilizers on our environment and ecosystem,” in Research Trends in Environmental Sciences, ed. P. Sharma (New Delhi: AkiNik Publications), 69–86.
Checker, V. G., Kushwaha, H. R., Kumari, P., and Yadav, S. (2018). “Role of phytohormones in plant defense: signaling and cross talk,” in Molecular Aspects of Plant-Pathogen Interaction, eds A. Singh and I. Singh (Singapore: Springer), 159–184. doi: 10.1007/978-981-10-7371-7_7
Chen, C., BÉLanger, R. R., Benhamou, N., and Paulitz, T. C. (2000). Defense enzymes induced in cucumber roots by treatment with plant growth-promoting rhizobacteria (PGPR) and Pythium aphanidermatum. Physiol. Mol. Plant Pathol. 56, 13–23. doi: 10.1006/pmpp.1999.0243
Chen, Y., Li, F., Tian, L., Huang, M., Deng, R., Li, X., et al. (2017). The phenylalanine ammonia lyase gene LjPAL1 is involved in plant defense responses to pathogens and plays diverse roles in lotus japonicus-rhizobium symbioses. Mol. Plant Microbe Interact. 30, 739–753. doi: 10.1094/mpmi-04-17-0080-r
Choudhury, A. T. M. A., and Kennedy, I. R. (2005). Nitrogen fertilizer losses from rice soils and control of environmental pollution problems. Commun. Soil Sci. Plant Anal. 36, 1625–1639. doi: 10.1081/CSS-200059104
Chowdhury, S. P., Dietel, K., Rändler, M., Schmid, M., Junge, H., Borriss, R., et al. (2013). Effects of Bacillus amyloliquefaciens FZB42 on lettuce growth and health under pathogen pressure and its impact on the rhizosphere bacterial community. PLoS One 8:e68818. doi: 10.1371/journal.pone.0068818
Èolo, J., Hajnal-Jafari, T., Duri, S., Stamenov, D., and Hamidovi, S. (2014). Plant growth promotion rhizobacteria in onion production. Polish J. Microbiol. 63, 83–88. doi: 10.33073/PJM-2014-012
Compant, S., Duffy, B., Nowak, J., Clément, C., and Barka, E. A. (2005). Use of plant growth-promoting bacteria for biocontrol of plant diseases: principles. Mech. Action Fut. Prospect. 71, 4951–4959. doi: 10.1128/AEM.71.9.4951-4959.2005
Contreras, J., Mata, T., Bermúdez, S., Caetano, N., Chandra, R., García, S., et al. (2019). Symbiotic co-culture of Scenedesmus sp. and Azospirillum brasilense on N-deficient media with biomass production for biofuels. Sustainability 11:707. doi: 10.3390/su11030707
Cooper, M. B., and Smith, A. G. (2015). Exploring mutualistic interactions between microalgae and bacteria in the omics age. Curr. Opin. Plant Biol. 26, 147–153. doi: 10.1016/j.pbi.2015.07.003
Coppens, J., Grunert, O., Van Den Hende, S., Vanhoutte, I., Boon, N., Haesaert, G., et al. (2016). The use of microalgae as a high-value organic slow-release fertilizer results in tomatoes with increased carotenoid and sugar levels. J. Appl. Phycol. 28, 2367–2377. doi: 10.1007/s10811-015-0775-2
Cordero, J., Garbayo, I., Cuaresma, M., Montero Lobato, Z., González-delValle, M. A., and Vílchez, C. (2016). Impact of microalgae-bacteria interactions on the production of algal biomass and associated compounds. Mar. Drugs 14:100. doi: 10.3390/md14050100
Croft, M. T., Lawrence, A. D., Raux-Deery, E., Warren, M. J., and Smith, A. G. (2005). Algae acquire vitamin B12 through a symbiotic relationship with bacteria. Nature 438, 90–93. doi: 10.1038/nature04056
de-Bashan, L. E., Bashan, Y., Moreno, M., Lebsky, V. K., and Bustillos, J. J. (2002). Increased pigment and lipid content, lipid variety, and cell and population size of the microalgae Chlorella spp. when co-immobilized in alginate beads with the microalgae-growth-promoting bacterium Azospirillum brasilense. Can. J. Microbiol. 48, 514–521. doi: 10.1139/w02-051
Debnath, M., and Bhadury, P. (2016). Adaptive responses and arsenic transformation potential of diazotrophic Cyanobacteria isolated from rice fields of arsenic affected Bengal Delta Plain. J. Appl. Phycol. 28, 2777–2792. doi: 10.1007/s10811-016-0820-9
Dias, G. A., Rocha, R. H. C., Araújo, J. L., Lima, J. F., and Guedes, W. A. (2016). Growth, yield, and postharvest quality in eggplant produced under different foliar fertilizer (Spirulina platensis) treatments. Semina Ciênc. Agrár. 37, 3893–3902. doi: 10.5433/1679-0359.2016v37n6p3893
Dineshkumar, R., Subramanian, J., Arumugam, A., Ahamed Rasheeq, A., and Sampathkumar, P. (2020). Exploring the microalgae biofertilizer effect on onion cultivation by field experiment. Waste Biomas. Valorizat. 11, 77–87. doi: 10.1007/s12649-018-0466-8
Dorais, M., Ehret, D., and Papadopoulos, A. (2008). Tomato (Solanum lycopersicum) health components: from the seed to the consumer. Phytochem. Rev. 7, 231–250. doi: 10.1007/s11101-007-9085-x
Doucette, G. J. (1995). Interactions between bacteria and harmful algae: a review. Nat. Tox. 3, 65–74. doi: 10.1002/nt.2620030202
Duan, X., Ren, G. Y., Liu, L. L., and Zhu, W. X. (2012). Salt-induced osmotic stress for lipid overproduction in batch culture of Chlorella vulgaris. Afr. J. Biotechnol. 11, 7072–7078. doi: 10.5897/AJB11.3670
Duffy, B. K., and Défago, G. (1999). Environmental factors modulating antibiotic and siderophore biosynthesis by Pseudomonas fluorescens biocontrol strains. Appl. Environ. Microbiol. 65, 2429–2438. doi: 10.1128/AEM.65.6.2429-2438.1999
Ebenezer, V., Medlin, L., and Ki, J.-S. (2011). Molecular detection quantification and diversity evaluation of microalgae. Mar. Biotechnol. 14, 129–142. doi: 10.1007/s10126-011-9427-y
Ergun, O., Dasgan, H., and Isık, O. (2020). Effects of microalgae Chlorella vulgaris on hydroponically grown lettuce. Acta Horticult. 2, 169–176. doi: 10.17660/ActaHortic.2020.1273.23
Esitken, A., Yildiz, H. E., Ercisli, S., Figen Donmez, M., Turan, M., and Gunes, A. (2010). Effects of plant growth promoting bacteria (PGPB) on yield, growth and nutrient contents of organically grown strawberry. Sci. Horticult. 124, 62–66. doi: 10.1016/j.scienta.2009.12.012
Farid, R., Mutale-Joan, C., Redouane, B., Mernissi Najib, E. L., Abderahime, A., Laila, S., et al. (2019). Effect of microalgae polysaccharides on biochemical and metabolomics pathways related to plant defense in Solanum lycopersicum. Appl. Biochem. Biotechnol. 188, 225–240. doi: 10.1007/s12010-018-2916-y
Garcia-Gonzalez, J., and Sommerfeld, M. (2016). Biofertilizer and biostimulant properties of the microalga Acutodesmus dimorphus. J. Appl. Phycol. 28, 1051–1061. doi: 10.1007/s10811-015-0625-2
Gavilanes, F. Z., Souza Andrade, D., Zucareli, C., Horácio, E. H., Sarkis Yunes, J., Barbosa, A. P., et al. (2020). Co-inoculation of Anabaena cylindrica with Azospirillum brasilense increases grain yield of maize hybrids. Rhizosphere 15:100224. doi: 10.1016/j.rhisph.2020.100224
Gemin, L. G., Mógor, ÁF., De Oliveira Amatussi, J., and Mógor, G. (2019). Microalgae associated to humic acid as a novel biostimulant improving onion growth and yield. Sci. Horticult. 256:108560. doi: 10.1016/j.scienta.2019.108560
Geries, L., and Elsadany, A. (2021). Maximizing growth and productivity of onion (Allium cepa L.) by Spirulina platensis extract and nitrogen-fixing endophyte Pseudomonas stutzeri. Arch. Microbiol. 203, 169–181. doi: 10.1007/s00203-020-01991-z
Ghasemi, Y., Rasoul-Amini, S., Naseri, A. T., Montazeri-Najafabady, N., Mobasher, M. A., and Dabbagh, F. (2012). Microalgae biofuel potentials (Review). Appl. Biochem. Microbiol. 48, 126–144. doi: 10.1134/S0003683812020068
Giles, J. (2005). Nitrogen study fertilizes fears of pollution. Nature 433:791. doi: 10.1038/433791a
Glick, B. R. (1995). The enhancement of plant growth by free-living bacteria. Canad. J. Microbiol. 41, 109–117. doi: 10.1139/m95-015
Gou, J.-Y., Suo, S.-Z., Shao, K.-Z., Zhao, Q., Yao, D., Li, H.-P., et al. (2020). Biofertilizers with beneficial rhizobacteria improved plant growth and yield in chili (Capsicum annuum L.). World J. Microbiol. Biotechnol. 36:86. doi: 10.1007/s11274-020-02863-w
Güneş, A., Turan, M., Güllüce, M., and Şahin, F. (2014). Nutritional content analysis of plant growth-promoting rhizobacteria species. Eur. J. Soil Biol. 60, 88–97. doi: 10.1016/j.ejsobi.2013.10.010
Guo, S., Wang, P., Wang, X., Zou, M., Liu, C., and Hao, J. (2020). “Microalgae as biofertilizer in modern agriculture,” in Microalgae Biotechnology for Food, Health and High Value Products, eds M. Alam, J. L. Xu, and Z. Wang (Singapore: Springer), 397–411. doi: 10.1007/978-981-15-0169-2_12
Gupta, N., Gupta, A. K., Gaur, V. S., and Kumar, A. (2012). Relationship of nitrogen use efficiency with the activities of enzymes involved in nitrogen uptake and assimilation of finger millet genotypes grown under different nitrogen inputs. ScientificWorldJournal 2012, 625731. doi: 10.1100/2012/625731
Gutiérrez-Mañero, F. J., Ramos-Solano, B., Probanza, A., Mehouachi, J., Tadeo, F., and Talon, M. (2001). The plant-growth-promoting rhizobacteria Bacillus pumilus and Bacillus licheniformis produce high amounts of physiologically active gibberellins. Physiol. Plant. 111, 206–211. doi: 10.1034/j.1399-3054.2001.1110211.x
Guzmán-Murillo, M. A., Ascencio, F., and Larrinaga-Mayoral, J. A. (2013). Germination and ROS detoxification in bell pepper (Capsicum annuum L.) under NaCl stress and treatment with microalgae extracts. Protoplasma 250, 33–42. doi: 10.1007/s00709-011-0369-z
Ha, S., Vanková, R., Yamaguchi-Shinozaki, K., Shinozaki, K., and Tran, L.-S. (2012). Cytokinins: metabolism and function in plant adaptation to environmental stresses. Trends Plant Sci. 17, 172–179. doi: 10.1016/j.tplants.2011.12.005
Han, J., Zhang, L., Wang, S., Yang, G., Zhao, L., and Pan, K. (2016). Co-culturing bacteria and microalgae in organic carbon containing medium. J. Biol. Res. 23:8. doi: 10.1186/s40709-016-0047-6
Hashtroudi, M. S., Ghassempour, A., Riahi, H., Shariatmadari, Z., and Khanjir, M. (2013). Endogenous auxins in plant growth-promoting Cyanobacteria—Anabaena vaginicola and Nostoc calcicola. J. Appl. Phycol. 25, 379–386. doi: 10.1007/s10811-012-9872-7
Horácio, E. H., Zucareli, C., Gavilanes, F. Z., Yunes, J. S., dos Santos, Sanzovo, A. W., et al. (2020). Co-inoculation of rhizobia, azospirilla and cyanobacteria for increasing common bean production Co-inoculação de rizobio, azospirillum e cianobactérias no aumento da produção de feijão comum Semina: Ciências Agrárias. Londrina 41, 2015–2028. doi: 10.5433/1679-0359.2020v41n5Supl1p2015
Huang, J., Wei, Z., Tan, S., Mei, X., Shen, Q., and Xu, Y. (2014). Suppression of bacterial wilt of tomato by bioorganic fertilizer made from the antibacterial compound producing strain Bacillus amyloliquefaciens HR62. J. Agricult. Food Chem. 62, 10708–10716. doi: 10.1021/jf503136a
Huang, S., Li, R., Zhang, Z., Li, L., Gu, X., Fan, W., et al. (2009). The genome of the cucumber Cucumis sativus L. Nat. Genet. 41, 1275–1281. doi: 10.1016/B978-0-08-040826-2.50017-5
Hyun, M. W., Yun, Y. H., Kim, J. Y., and Kim, S. H. (2011). Fungal and plant phenylalanine ammonia-lyase. Mycobiology 39, 257–265. doi: 10.5941/myco.2011.39.4.257
Jung, H. K., Kim, J. R., Woo, S. M., and Kim, S. D. (2006). An auxin producing plant growth promoting rhizobacterium Bacillus subtilis AH18 which has siderophore-producing biocontol activity. Kor. J. Microbiol. Biotechnol. 34, 94–100.
Kandoliya, U., Bodar, N., Bajaniya, V., Bhadja, N., and Golakiya, B. (2015). Determination of nutritional value and antioxidant from bulbs of different onion (Allium cepa) variety: a comparative study. Int. J. Curr. Microbiol. App. Sci 4, 635–641.
Kaplan, D. (2013). “Absorption and adsorption of heavy metals by microalgae,” in Handbook of Microalgal Culture, eds. A. Richmond and Q. Hu (Chichester, UK: John Wiley & Sons, Ltd), 602–611. doi: 10.1002/9781118567166.ch32
Kim, M.-J., Shim, C.-K., Kim, Y.-K., Ko, B.-G., Park, J.-H., Hwang, S.-G., et al. (2018). Effect of biostimulator Chlorella fusca on improving growth and qualities of chinese chives and spinach in organic farm. Plant Pathol. J. 34, 567–574. doi: 10.5423/PPJ.FT.11.2018.0254
Kobayashi, A., Tai, A., and Kawazu, K. (1995). Structural elucidation of an elicitor-active oligosaccharide, LN-3, prepared from Algal laminaran. J. Carbohydr. Chem. 14, 819–832. doi: 10.1080/07328309508005378
Kopta, T., Pavlikova, M., Sêkara, A., Pokluda, R., and Maršálek, B. (2018). Effect of bacterial-algal biostimulant on the yield and internal quality of lettuce (Lactuca sativa L.) produced for spring and summer crop. Not. Botan. Horti. Agrobot. Cluj Napoc. 46, 615–621. doi: 10.15835/nbha46211110
Kumar, S., Kumar, R., and Singh, J. (2006). “16 - Cayenne/American pepper,” in Handbook of Herbs and Spices, ed. K. V. Peter (Abington, PA: Woodhead Publishing), 299–312. doi: 10.1533/9781845691717.3.299
Kumar, V. V. (2018). “Biofertilizers and biopesticides in sustainable agriculture,” in Role of Rhizospheric Microbes in Soil, ed. V. Meena (Singapore: Springer), 377–398.
Lim, J.-H., and Kim, S.-D. (2009). Synergistic plant growth promotion by the indigenous auxins-producing PGPR Bacillus subtilis AH18 and Bacillus licheniforims K11. J. Kor. Soc. Appl. Biol. Chem. 52, 531–538. doi: 10.3839/jksabc.2009.090
Mahanty, T., Bhattacharjee, S., Goswami, M., Bhattacharyya, P., Das, B., Ghosh, A., et al. (2017). Biofertilizers: a potential approach for sustainable agriculture development. Environ. Sci. Pollut. Res. 24, 3315–3335. doi: 10.1007/s11356-016-8104-0
Mehta, P., Walia, A., Kulshrestha, S., Chauhan, A., and Shirkot, C. K. (2015). Efficiency of plant growth-promoting P-solubilizing Bacillus circulans CB7 for enhancement of tomato growth under net house conditions. J. Basic Microbiol. 55, 33–44. doi: 10.1002/jobm.201300562
Michalak, I., and Chojnacka, K. (2014). Algae as production systems of bioactive compounds. Eng. Life Sci. 15:191. doi: 10.1002/elsc.201400191
Minuto, A., Migheli, Q., and Garibaldi, A. (1993). “Integrated control of soil-borne plant pathogens by solar heating and antagonistic microorganisms,” in Proceedings of the IV International Symposium on Soil and Substrate Infestation and Disinfestation, Vol. 382, Leuven, 138–144. doi: 10.17660/actahortic.1995.382.14
Mubashar, M., Naveed, M., Mustafa, A., Ashraf, S., Shehzad Baig, K., Alamri, S., et al. (2020). Experimental investigation of chlorella vulgaris and Enterobacter sp. MN17 for decolorization and removal of heavy metals from textile wastewater. Water 12:3034. doi: 10.3390/w12113034
Mujtaba, G., and Lee, K. (2016). Advanced treatment of wastewater using symbiotic co-culture of microalgae and bacteria. Appl. Chem. Eng 27, 1–9. doi: 10.14478/ace.2016.1002
Mujtaba, G., Rizwan, M., and Lee, K. (2017). Removal of nutrients and COD from wastewater using symbiotic co-culture of bacterium Pseudomonas putida and immobilized microalga Chlorella vulgaris. J. Industr. Eng. Chem. 49, 145–151. doi: 10.1016/j.jiec.2017.01.021
Najdenski, H. M., Gigova, L. G., Iliev, I. I., Pilarski, P. S., Lukavský, J., Tsvetkova, I. V., et al. (2013). Antibacterial and antifungal activities of selected microalgae and cyanobacteria. Int. J. Food Sci. Technol. 48, 1533–1540. doi: 10.1111/ijfs.12122
Naviner, M., Bergé, J. P., Durand, P., and Le Bris, H. (1999). Antibacterial activity of the marine diatom Skeletonema costatum against aquacultural pathogens. Aquaculture 174, 15–24. doi: 10.1016/S0044-8486(98)00513-4
Nicola, S., Tibaldi, G., Fontana, E., Crops, A.-V., and Plants, A. (2009). Tomato production systems and their application to the tropics. Acta Horticult. 821, 27–34. doi: 10.17660/ActaHortic.2009.821.1
Nie, P., Li, X., Wang, S., Guo, J., Zhao, H., and Niu, D. (2017). Induced systemic resistance against Botrytis cinerea by Bacillus cereus AR156 through a JA/ET- and NPR1-dependent signaling pathway and activates PAMP-triggered immunity in Arabidopsis. Front. Plant Sci. 8:238. doi: 10.3389/fpls.2017.00238
Ogbonna, J. C., Yoshizawa, H., and Tanaka, H. (2000). Treatment of high strength organic wastewater by a mixed culture of photosynthetic microorganisms. J. Appl. Phycol. 12, 277–284. doi: 10.1023/A:1008188311681
Pankhurst, C. E., Ophel-Keller, K., Doube, B. M., and Gupta, V. V. S. R. (1996). Biodiversity of soil microbial communities in agricultural systems. Biodiver. Conserv. 5, 197–209. doi: 10.1007/BF00055830
Peng, H., de-Bashan, L. E., Bashan, Y., and Higgins, B. T. (2020). Indole-3-acetic acid from Azosprillum brasilense promotes growth in green algae at the expense of energy storage products. Algal Res. 47:101845. doi: 10.1016/j.algal.2020.101845
Perales-Vela, H. V., Peña-Castro, J. M., and Cañizares-Villanueva, R. O. (2006). Heavy metal detoxification in eukaryotic microalgae. Chemosphere 64, 1–10. doi: 10.1016/j.chemosphere.2005.11.024
Pierik, R., Tholen, D., Poorter, H., Visser, E. J. W., and Voesenek, L. A. C. J. (2006). The Janus face of ethylene: growth inhibition and stimulation. Trends Plant Sci. 11, 176–183. doi: 10.1016/j.tplants.2006.02.006
Prasanna, R., Joshi, M., Rana, A., Shivay, Y. S., and Nain, L. (2012). Influence of co-inoculation of bacteria-cyanobacteria on crop yield and C–N sequestration in soil under rice crop. World J. Microbiol. Biotechnol. 28, 1223–1235. doi: 10.1007/s11274-011-0926-9
Priya, M., Gurung, N., Mukherjee, K., and Bose, S. (2014). “23 – Microalgae in removal of heavy metal and organic pollutants from soil,” in Microbial Biodegradation and Bioremediation, ed. S. Das (Oxford: Elsevier), 519–537. doi: 10.1016/b978-0-12-800021-2.00023-6
Puglisi, I., La Bella, E., Rovetto, E. I., Lo Piero, A. R., and Baglieri, A. (2020). Biostimulant effect and biochemical response in lettuce seedlings treated with A Scenedesmus quadricauda extract. Plants (Basel Switzerland) 9:123. doi: 10.3390/plants9010123
Qi, W., Mei, S., Yuan, Y., Li, X., Tang, T., Zhao, Q., et al. (2018). Enhancing fermentation wastewater treatment by co-culture of microalgae with volatile fatty acid- and alcohol-degrading bacteria. Algal Res. 31, 31–39. doi: 10.1016/j.algal.2018.01.012
Rachidi, F., Benhima, R., Sbabou, L., and El Arroussi, H. (2020). Microalgae polysaccharides bio-stimulating effect on tomato plants: growth and metabolic distribution. Biotechnol. Rep. 25:e00426. doi: 10.1016/j.btre.2020.e00426
Rajamani, S., Siripornadulsil, S., Falcao, V., Torres, M., Colepicolo, P., and Sayre, R. (2007). “Phycoremediation of heavy metals using transgenic microalgae,” in Transgenic Microalgae as Green Cell Factories, eds R. León, A. Galván, and E. Fernández (New York, NY: Springer), 99–109. doi: 10.1007/978-0-387-75532-8_9
Ramanan, R., Kim, B.-H., Cho, D.-H., Oh, H.-M., and Kim, H.-S. (2016). Algae–bacteria interactions: evolution, ecology and emerging applications. Biotechnol. Adv. 34, 14–29. doi: 10.1016/j.biotechadv.2015.12.003
Raupach, G. S., and Kloepper, J. W. (1998). Mixtures of plant growth-promoting rhizobacteria enhance biological control of multiple cucumber pathogens. Phytopathology 88, 1158–1164. doi: 10.1094/PHYTO.1998.88.11.1158
Ribalet, F., Intertaglia, L., Lebaron, P., and Casotti, R. (2008). Differential effect of three polyunsaturated aldehydes on marine bacterial isolates. Aquat Toxicol. 86, 249–255. doi: 10.1016/j.aquatox.2007.11.005
Rodrìguez, H., and Fraga, R. (1999). Phosphate solubilizing bacteria and their role in plant growth promotion. Biotechnol. Adv. 17, 319–339. doi: 10.1016/S0734-9750(99)00014-2
Romanenko, E., Kosakovskaya, I., and Romanenko, P. (2015). Phytohormones of microalgae: biological role and involvement in the regulation of physiological processes. Pt I. auxins, abscisic acid, ethylene. Int. J. Algae 17, 179–201. doi: 10.1615/InterJAlgae.v18.i2.70
Savci, S. (2012). Investigation of effect of chemical fertilizers on environment. APCBEE Proc. 1, 287–292. doi: 10.1016/j.apcbee.2012.03.047
Schiavon, M., Ertani, A., and Nardi, S. (2008). Effects of an alfalfa protein hydrolysate on the gene expression and activity of enzymes of the tricarboxylic acid (TCA) cycle and nitrogen metabolism in Zea mays L. J. Agric. Food Chem. 56, 11800–11808. doi: 10.1021/jf802362g
Schuhegger, R., Ihring, A., Gantner, S., Bahnweg, G., Knappe, C., Vogg, G., et al. (2006). Induction of systemic resistance in tomato by N-acyl-L-homoserine lactone-producing rhizosphere bacteria. Plant Cell Environ. 29, 909–918. doi: 10.1111/j.1365-3040.2005.01471.x
Schumacher, G., Blume, T., and Sekoulov, I. (2003). Bacteria reduction and nutrient removal in small wastewater treatment plants by an algal biofilm. Water Sci. Technol. 47, 195–202. doi: 10.2166/wst.2003.0605
Sessitsch, A., Reiter, B., and Berg, G. (2004). Endophytic bacterial communities of field-grown potato plants and their plant-growth-promoting and antagonistic abilities. Can. J. Microbiol. 50, 239–249. doi: 10.1139/w03-118
Shariatmadari, Z., Riahi, H., Mehri, S., Seyed Hashtroudi, M., Ghassempour, A., and Aghashariatmadary, Z. (2013). Plant growth promoting cyanobacteria and their distribution in terrestrial habitats of Iran. Soil Sci. Plant Nutr. 59, 535–547. doi: 10.1080/00380768.2013.782253
Shi, Y., Cheng, C., Lei, P., Wen, T., and Merrifield, C. J. (2011). Safe food, green food, good food: chinese community supported agriculture and the rising middle class. Int. J. Agric. Sustain. 9, 551–558. doi: 10.1080/14735903.2011.619327
Silo-Suh, L. A., Lethbridge, B. J., Raffel, S. J., He, H., Clardy, J., and Handelsman, J. (1994). Biological activities of two fungistatic antibiotics produced by Bacillus cereus UW85. Appl. Environ. Microbiol. 60, 2023–2030. doi: 10.1128/AEM.60.6.2023-2030.1994
Singh, J., and Saxena, R. (2015). “An introduction to microalgae: diversity and significance. diversity and significance,” in Handbook of Marine Microalgae: Biotechnology Advances, ed. S. K. Kim (Amsterdam: Academic Press), 11–24. doi: 10.1016/B978-0-12-800776-1.00002-9
Smirnoff, N. (2018). Ascorbic acid metabolism and functions: a comparison of plants and mammals. Free Radic. Biol. Med. 122, 116–129. doi: 10.1016/j.freeradbiomed.2018.03.033
Spadaro, D., and Gullino, M. L. (2005). Improving the efficacy of biocontrol agents against soilborne pathogens. Crop Protect. 24, 601–613. doi: 10.1016/j.cropro.2004.11.003
Sponsel, V. M., and Hedden, P. (2010). “Gibberellin biosynthesis and inactivation,” in Plant Hormones, ed. P. J. Davies (Dordrecht: Springer), 63–94. doi: 10.1007/978-1-4020-2686-7_4
Stirk, W. A., Bálint, P., Tarkowská, D., Novák, O., Strnad, M., Ördög, V., et al. (2013a). Hormone profiles in microalgae: gibberellins and brassinosteroids. Plant Physiol. Biochem. 70, 348–353. doi: 10.1016/j.plaphy.2013.05.037
Stirk, W. A., Ördög, V., Novák, O., Rolčík, J., Strnad, M., Bálint, P., et al. (2013b). Auxin and cytokinin relationships in 24 microalgal strains(1). J. Phycol. 49, 459–467. doi: 10.1111/jpy.12061-
Subashchandrabose, S. R., Ramakrishnan, B., Megharaj, M., Venkateswarlu, K., and Naidu, R. (2011). Consortia of cyanobacteria/microalgae and bacteria: biotechnological potential. Biotechnol. Adv. 29, 896–907. doi: 10.1016/j.biotechadv.2011.07.009
Suganya, T., Varman, M., Masjuki, H. H., and Renganathan, S. (2016). Macroalgae and microalgae as a potential source for commercial applications along with biofuels production: a biorefinery approach. Renew. Sustain. Ener. Rev. 55, 909–941. doi: 10.1016/j.rser.2015.11.026
Sun, X.-M., Ren, L.-J., Zhao, Q.-Y., Ji, X.-J., and Huang, H. (2018). Microalgae for the production of lipid and carotenoids: a review with focus on stress regulation and adaptation. Biotechnol. Biof. 11, 272–272. doi: 10.1186/s13068-018-1275-9
Sylvia, D., Fuhrmann, J., Hartel, P., and Zuberer, D. (2005). Principles and Applications of Soil Microbiology. Upper Saddle River, NJ: Prentice Hall. 550.
Tarakhovskaya, E. R., Maslov, Y. I., and Shishova, M. F. (2007). Phytohormones in algae. Rus. J. Plant Physiol. 54, 163–170. doi: 10.1134/S1021443707020021
Tatlioglu, T. (1993). “13 – cucumber: Cucumis sativus L,” in Genetic Improvement of Vegetable Crops, eds G. Kalloo and B. O. Bergh (Amsterdam: Pergamon), 197–234.
Unnithan, V. V., Unc, A., and Smith, G. B. (2014). Mini-review: a priori considerations for bacteria–algae interactions in algal biofuel systems receiving municipal wastewaters. Algal Res. 4, 35–40. doi: 10.1016/j.algal.2013.11.009
Utkhede, R. S., and Koch, C. A. (1999). Rhizobacterial growth and yield promotion of cucumber plants inoculated with Pythium aphanidermatum. Can. J. Plant Pathol. 21, 265–271. doi: 10.1080/07060669909501189
Vera, J., Castro, J., Gonzalez, A., and Moenne, A. (2011). Seaweed polysaccharides and derived oligosaccharides stimulate defense responses and protection against pathogens in plants. Mar. Drugs 9, 2514–2525. doi: 10.3390/md9122514
Verma, M., Mishra, J., and Arora, N. (2019). “Plant growth-promoting rhizobacteria: diversity and applications,” in Environmental Biotechnology: For Sustainable Future, eds R. Sobti, N. Arora, and R. Kothari (Singapore: Springer), 129–173. doi: 10.1007/978-981-10-7284-0_6
Walpola, B. C., and Yoon, M.-H. (2013). Isolation and characterization of phosphate solubilizing bacteria and their co-inoculation efficiency on tomato plant growth and phosphorous uptake. Afr. J. Microbiol. Res. 7, 266–275. doi: 10.5897/AJMR12.2282
Walsh, U. F., Morrissey, J. P., and O’Gara, F. (2001). Pseudomonas for biocontrol of phytopathogens: from functional genomics to commercial exploitation. Curr. Opin. Biotechnol. 12, 289–295. doi: 10.1016/s0958-1669(00)00212-3
Watanabe, K., Takihana, N., Aoyagi, H., Hanada, S., Watanabe, Y., Ohmura, N., et al. (2005). Symbiotic association in Chlorella culture. FEMS Microbiol. Ecol. 51, 187–196. doi: 10.1016/j.femsec.2004.08.004
Wolfaardt, G. M., Lawrence, J. R., Robarts, R. D., and Caldwell, D. E. (1994). The role of interactions, sessile growth and nutrient amendments on the degradative efficiency of a microbial consortium. Can. J. Microbiol. 40, 331–340. doi: 10.1139/m94-055
Yoshihisa, H., Zenji, S., Fukushi, H., Katsuhiro, K., Haruhisa, S., and Takahito, S. (1989). Production of antibiotics by Pseudomonas cepacia as an agent for biological control of soilborne plant pathogens. Soil Biol. Biochem. 21, 723–728. doi: 10.1016/0038-0717(89)90070-9
Yu, Q., Matheickal, J. T., Yin, P., and Kaewsarn, P. (1999). Heavy metal uptake capacities of common marine macro algal biomass. Water Res. 33, 1534–1537. doi: 10.1016/S0043-1354(98)00363-7
Keywords: biofertilizers, combinational application, microalgae, mixed cultures, plant growth-promoting bacteria, vegetables
Citation: Kang Y, Kim M, Shim C, Bae S and Jang S (2021) Potential of Algae–Bacteria Synergistic Effects on Vegetable Production. Front. Plant Sci. 12:656662. doi: 10.3389/fpls.2021.656662
Received: 21 January 2021; Accepted: 12 March 2021;
Published: 12 April 2021.
Edited by:
Maurizio Ruzzi, University of Tuscia, ItalyReviewed by:
Narendra Singh, Southern University of Science and Technology, ChinaAna L. Gonçalves, University of Porto, Portugal
Copyright © 2021 Kang, Kim, Shim, Bae and Jang. This is an open-access article distributed under the terms of the Creative Commons Attribution License (CC BY). The use, distribution or reproduction in other forums is permitted, provided the original author(s) and the copyright owner(s) are credited and that the original publication in this journal is cited, in accordance with accepted academic practice. No use, distribution or reproduction is permitted which does not comply with these terms.
*Correspondence: Seonghoe Jang, c2Vvbmdob2UuamFuZ0B3b3JsZHZlZy5vcmc=