- School of Agricultural Biotechnology, Punjab Agricultural University, Ludhiana, India
Nitrogen is an essential nutrient required in large quantities for the proper growth and development of plants. Nitrogen is the most limiting macronutrient for crop production in most of the world’s agricultural areas. The dynamic nature of nitrogen and its tendency to lose soil and environment systems create a unique and challenging environment for its proper management. Exploiting genetic diversity, developing nutrient efficient novel varieties with better agronomy and crop management practices combined with improved crop genetics have been significant factors behind increased crop production. In this review, we highlight the various biochemical, genetic factors and the regulatory mechanisms controlling the plant nitrogen economy necessary for reducing fertilizer cost and improving nitrogen use efficiency while maintaining an acceptable grain yield.
Introduction
Cereal crops are highly cultivated in comparison to other crops worldwide. Among cereals, rice (Oryza sativa L.), wheat (Triticum aestivum L.), and maize (Zea mays L.) are most important in terms of human nutrition and represent 90% of cereal production worldwide. The value of cereal crops in world agriculture has significantly increased since the Green Revolution. The three major cereal crops are known to address the world protein and calorie demand either directly by human consumption or indirectly through livestock (Ladha et al., 2016; Guerrieri and Cavaletto, 2018). Many factors are known to influence the quality and quantity of cereal crops produced worldwide, and the most important among them is nitrogen availability. All plants depend on the external source of inorganic nitrogen (N), as it is the essential component of biomolecules, including proteins, nucleic acids, chlorophyll, and several secondary metabolites. In agricultural practices, nitrogen availability is a limiting factor to enhance the yield, and worldwide approximately 100 TgNyr–1 of reactive nitrogen is applied in the form of fertilizers to crop fields (Ladha et al., 2016). Globally, the total N fertilizer consumption has grown from 112.5 million tons in 2015 to 118.2 million tons in 2019 (see Figure 1A). Between 1970 and 2020, nitrogenous fertilizer consumption has increased at a higher rate across different countries (Figure 1B). It is observed that in cereals, yield can be directly correlated to nitrogen application (Ladha et al., 2016). Approximately 94 million tons of N fertilizer is applied to cereal crops every year, but less than 40% is utilized by the crops, while the remaining part dissipates in the environment, raising severe environmental issues such as water pollution and greenhouse gas emission (Plett et al., 2018). A total of 44 million tons of nitrogen accounts for biological fixation by the legumes and other plants, where 99 million tons accounts for other anthropogenic sources such as habitat destruction and fossil fuels (Matson et al., 2002). Natural sources such as soil bacteria, algae, and lightning account for 154 million tons. Among the cereal crops, barley has maximum nitrogen recovery (63%) followed by maize (37%), wheat (35–45%), and rice (30–50%). Nitrogen recovery not only changes with crop type it also depends on the environmental condition, type of fertilizers used, management strategy, and genotype to environment interactions.
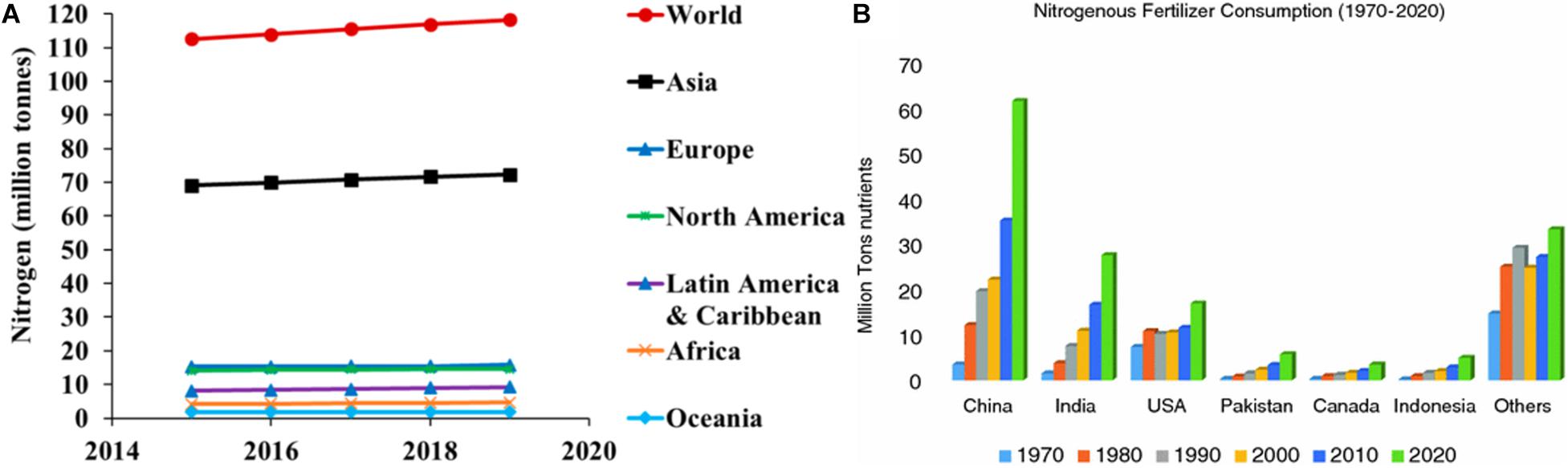
Figure 1. (A) The world and regional demand for nitrogen fertilizer forecasts, 2015–2019. Adapted from FAO (2016). (B) The consumption of nitrogenous fertilizer from 1970 to 2020 across different countries of the world.
In the post-Green Revolution era, traditional varieties were replaced by a few selected and widely adapted semi-dwarf, early-maturing, high-yielding, disease-resistant varieties that require high input conditions. The consumption of fertilizers is expected to double by 2050, i.e., from 112 Mt in 2015 to 236 Mt in 2050 (Tilman et al., 2011). Nevertheless, nitrogen fertilizer utilization is relatively inefficient. Around 50–70% of applied nitrogen always vanishes from the plant-soil system. The high input of commercially available fertilizers has led to the degradation of air, soil, and water quality with the exhaustion of natural resources such as nutrients and water. When the nitrogen supply is more than crop nitrogen demand, it leads to the accumulation of nitrogen in the soil, and the plants are susceptible to various loss pathways. Therefore, it is necessary to improve the resource use efficiency of cereal crops to minimize the negative impact of increasing yield on environments and natural resources. To reduce the effect of the increasing use of fertilizers on climate change and manage sustainable feeding to the growing world population, enhancing nitrogen use efficiency (NUE) in cereals must be a priority in breeding programs. It is essential to understand the underlying mechanism of nitrogen use efficiency to encounter the issues related to nitrogen application in fields. The use of N (nitrogen) in plants involves several stages, which can be divided into the primary N uptake phase, followed by reduction of nitrogen to useable forms, its assimilation into amino acids, translocation, and the last stage is remobilization of nitrogen to reproductive tissues (Figure 2; Masclaux-Daubresse et al., 2010). NUE in cereal crops is defined as the grain yield per unit of nitrogen available in the soil (Moll et al., 1982; Figure 2). The analysis of NUE gives details about plant response to different nitrogen availability conditions. Nitrogen use efficiency can be described by various formulas and definitions. Cereal NUE resulted from the combination between how effectively plants capture the nitrogen (uptake efficiency, NUpE) and how the plants use the taken-up nitrogen (utilization efficiency, NUtE) (Figure 2; Hansen et al., 2018). NUpE is calculated as the total amount of above-ground nitrogen content during harvest by available N in the soil, and NUtE is calculated as the nitrogen in grain tissues divided by N in above-ground plant biomass at harvest (Figure 2). So NUE is calculated at the time of harvest, i.e., end of the crop cycle. The usage index (UI) takes into account the absolute increase in the biomass and can be calculated as UI: shoot weight ∗(shoot weight/nitrogen content of the shoot) (Siddiqi and Glass, 1981). Craswell and Godwin (1984) described agronomic efficiency as differences between the grain weight with and without fertilizer divided by the total fertilizer applied; apparent nitrogen recovery as differences between the plant nitrogen uptake with and without fertilizer divided by the total fertilizer applied and multiplied by the factor 100; and the physiological efficiency as the differences between the grain weight with and without fertilizer divided by plant nitrogen uptake with and without fertilizer. The agronomic efficiency measures the efficiency of plants converting the applied nitrogen to the grain yield whereas the apparent nitrogen efficiency of plants captures the nitrogen from the soil (Craswell and Godwin, 1984). The physiological efficiency measures the efficiency of plants in terms of converting the capturing nitrogen to the grain yield.
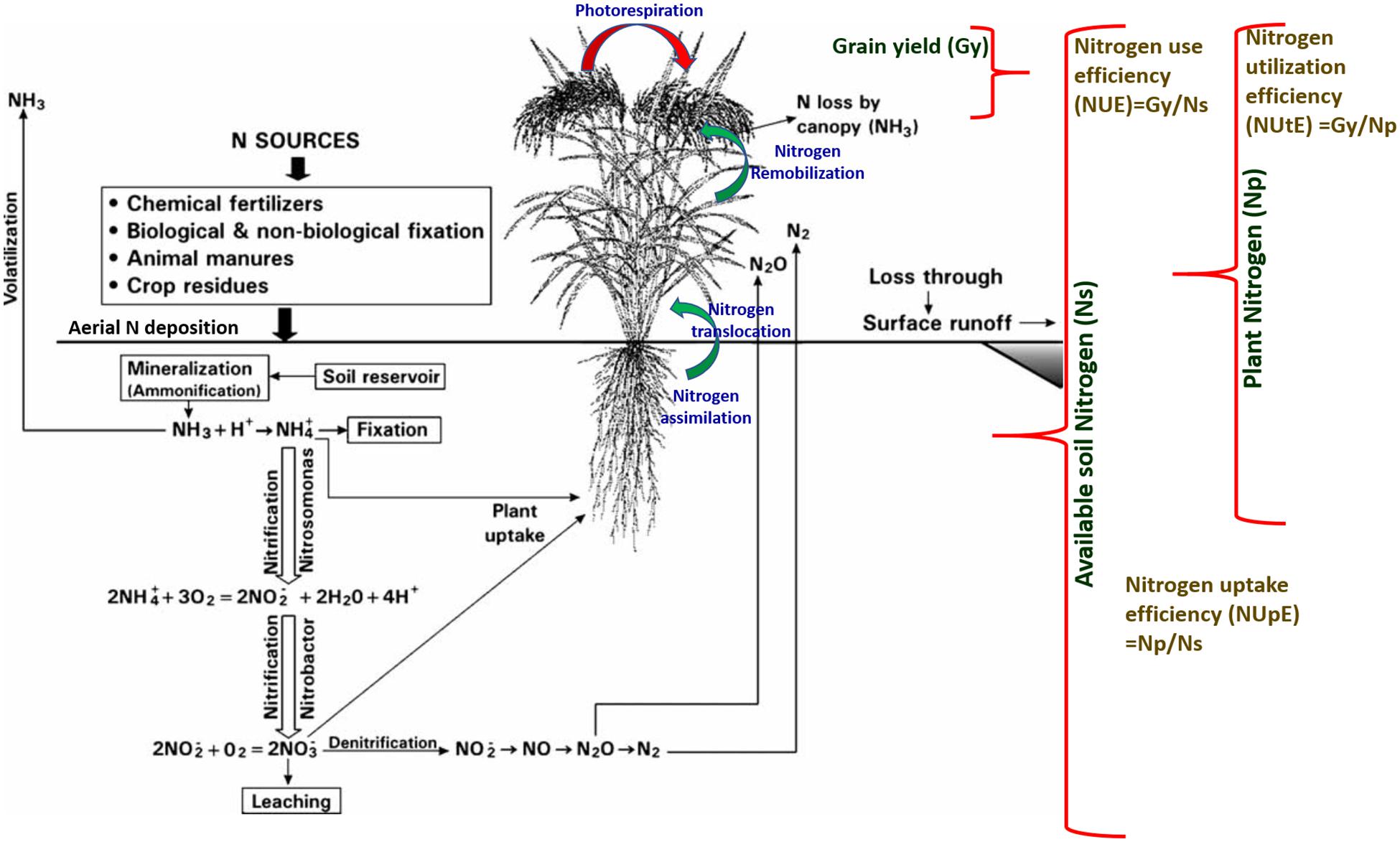
Figure 2. Schematic representation of the relationship between the nitrogen sources, key physiological processes for the nitrogen assimilation, translocation, remobilization, uptake, utilization, and conversion to the grain yield.
Improving resource use efficiency to minimize the negative impact of increasing yield with increasing input use on environments/natural resources is an urgent need for major cereal crops. The challenge here is to identify the specific and most responsive stage to the fertilizer application, having a plant that maximizes its early nitrogen uptake, and having traits such as early vegetative vigor and an extensive root system for efficient nutrient uptake considering above and below ground level factors. Later in growth development, a plant with the ability to assimilate and remobilize the available nitrogen and associated carbon to the grain is crucial. Another key challenge here includes appropriate root phenotyping, genotype x environmental interactions, soil characteristics, water-nutrient management, and nutrient dynamics balance. The key question, whether the improvement of nutrient uptake with reducing excessive input of fertilizers and safeguarding soil-health while maintaining the desired yield and grain protein content is feasible. Nanotechnology, including the use of nano fertilizers (1–100 nm in size) is beneficial and reported to have positive results, but still there is a need to specify the effect of nano fertilizers on specific crops (Cowling and Field, 2003).
Before understanding the biochemistry and genetics behind the improvement of nitrogen use efficiency in cereal crops, there is a need to understand the new potential source of nitrogen fertilizers, effect of nitrogen at different stages of growth, nitrogen status of the crop, and development and NUE in the effect of fertilizers (Cameron et al., 2013). The multiple fertilizer sources include anhydrous ammonia (82%N), urea (46%N), ammonium nitrate (34%), ammonium nitrate sulfate (26%), and aqua ammonia (25%N). Nitrogen fertilizers can be broadly classified into organic and inorganic fertilizers. Firstly, looking in terms of inorganic fertilizers, maximum nitrogen, i.e., more than 80%, is contributed by anhydrous ammonia application. Aqua ammonia or ammonium hydroxide is the second most important source of inorganic nitrogen fertilizers and it contains 25 to 29% ammonia by weight. Another form of nitrogen fertilizer is ammonium nitrate and its relevance from the agronomic aspect is a combination of two different forms of nitrogen (NH4NO3). This form of fertilizer is reported to enhance the baking quality of wheat (Dobermann and Cassman, 2002). Ammonium sulfate ((NH4)2SO4) is an important source of both nitrogen and sulfur that can be advantageous for crops that require acid such as rice and in high-pH soils. Another form of fertilizer that comes with a dual nutrient composition which acts as the source of nitrogen, phosphorous, and chloride include monoammonium (NH4H2PO4), diammonium (NH4)2HPO4) phosphates, ammonium chloride (NH4Cl), and ammonium sulfate (Inman et al., 2005). The organic form of fertilizer is urea [CO(NH2)2] (Franzen et al., 2002).
The cereal crop undergoes different stages of growth and development (Figure 3). The rate of nutrient uptake varies with the crop, crop growth stage, variety, and with growing conditions and environment. Proper understanding of the nutrient uptake patterns of cereal crops is required to determine the optimal timing and specific stage of fertilizer applications. Small amounts of nitrogen are important at an early stage for seedling vigor. About 50% of the required nitrogen is used up by the mid-tillering stage (Miller et al., 1993; Figure 3). However, a high dose of nitrogen may damage the seedlings and over-stimulate the vegetative growth early in the season and thus decrease the yields. Excess nitrogen may delay crop maturity. In the Montana study, more than 70% of the total above-ground N had been accumulated by the beginning of the grain filling stage (Figure 3).
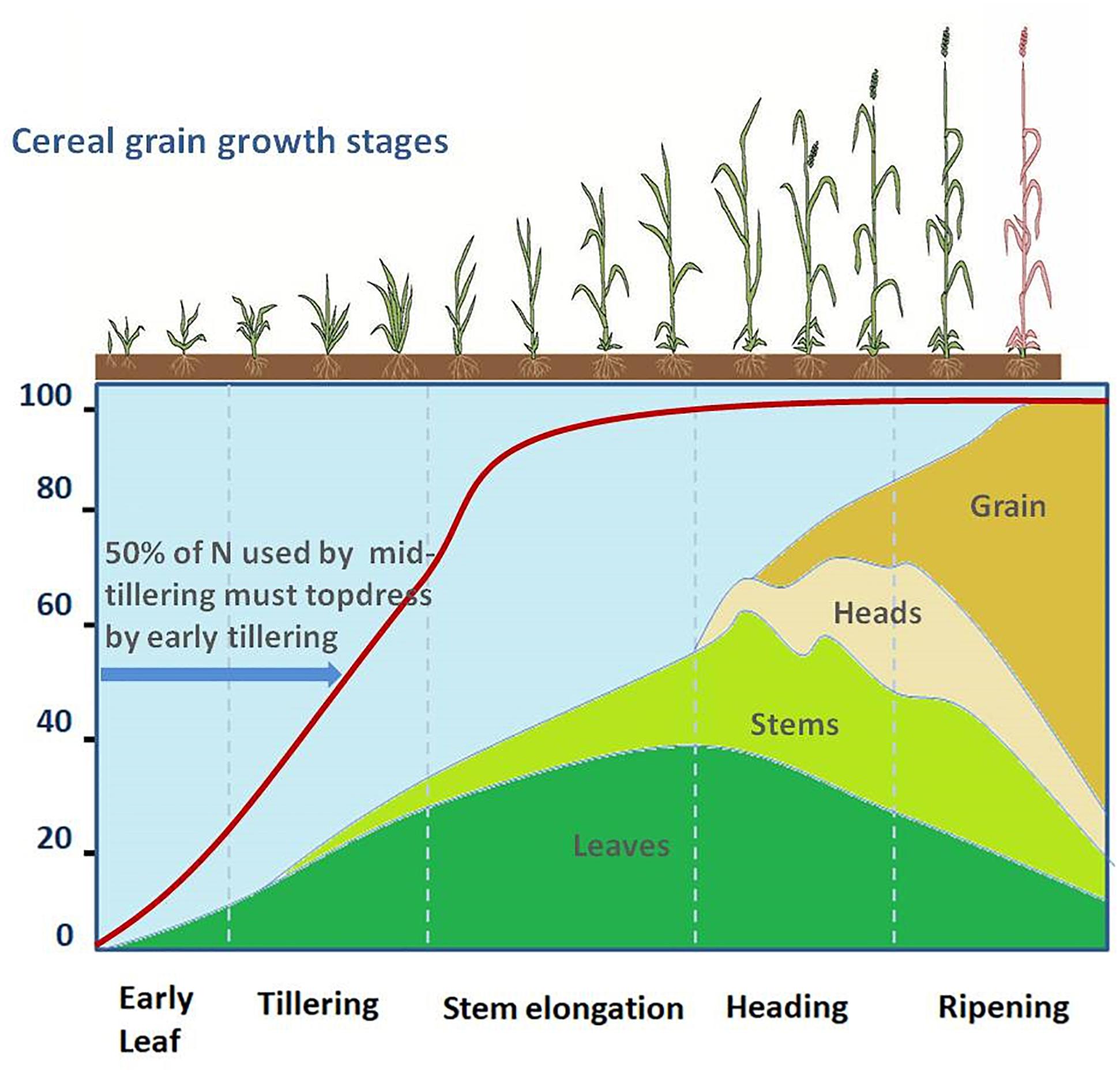
Figure 3. The different stages of growth and development in cereal crops. The red line indicates the requirement of nitrogen at different stages of growth and development, the colored areas under different curves show the accumulation of nitrogen in leaves, stem, head, and grain of spring wheat as a percent of maximum nitrogen. Source: Adapted and modified from Miller et al. (1993).
Several factors are reported to influence the requirement of nitrogen and it is observed that NUE decreases when nitrogen application exceeds the potential demand (Mullen et al., 2003). The most active area concerned with the increase in NUE in the crop system is the identification of the nitrogen status of the crop. It is described that there is no linear relation between nitrogen applied and crop yield, as NUE is regulated by several factors (Inman et al., 2007), such as soil type, availability of other macro and micronutrients (phosphorous, potassium, etc.) in soil, and crop rotation reported to regulate the nitrogen uptake and utilization (Hatfield et al., 2008). Nitrogen fertilization also depends on intensity, timing, and depth of tillage (Cassman et al., 2002; Osborne et al., 2002). There is a need to improve strategies to diagnose nitrogen status and this is the most active area of research to enhance the output of N fertilization. Among several N estimation approaches estimation of nitrate and ammonium forms in the soil, satellite imaging (Sharma et al., 2016), portable hyperspectral sensors (Shaver et al., 2014), drones, chlorophyll meters (SPAD), red edge optical reflectance (R750/R710) (Sharma and Franzen, 2013; Sharma et al., 2015), NDVI (normalized vegetation index), and RVI (ration vegetation index) (Sharma et al., 2016) offer the possibility of N estimation in less time.
The wild and primitive species of cereal crops are not fully recognized yet as an important source of novel variations for nutrient utilization efficiency. Association studies exploiting the best alleles to be assembled in superior varieties, identification and characterization of candidate genes with non-synonymous and regulatory SNPs will help breeders to choose specific donors to develop resource-efficient high yielding wheat varieties. Further, yield and grain protein content which represent nitrogen use efficiency are inversely related, so it very important for breeders to design cultivation programs to achieve comparatively successful NUE without compromising grain yield (Oury and Godin, 2007) and to develop such cultivars, it is very important to understand the details of various genetic, physiological, and biochemical factors affecting NUpE and NUtE.
Along-with-it agronomic practices and field management played a major role in combating loss of applied nitrogen to the environment (Karamanos et al., 2014). The present review focuses on the multiple biochemical and genetic factors affecting NUE directly and indirectly. The review gives a descriptive outline about the biochemistry involved in nitrogen uptake and utilization; genetic system influencing NUE among cereals and their phenotype outcomes positively affecting NUE. The related study among cereals is beneficial to design strategies for the combined increase in NUE without affecting other beneficial traits.
Biochemical and Biological Processes
Several metabolic processes in coordination influence the nitrogen use efficiency of higher plants. It is very important to understand the nitrogen use efficiency and its component before getting into the details of mechanisms affecting efficient NUE. The analysis of NUE gives details about plant response to different nitrogen availability conditions. Nitrogen use efficiency can be bifurcated into two components, i.e., nitrogen uptake efficiency (NUpE) and nitrogen utilization efficiency (NUtE) so to estimate NUE both of its components have to be calculated. NUpE is calculated as the total amount of above-ground nitrogen content during harvest by available N in the soil, and NUtE is calculated as the nitrogen in grain tissues divided by N in above-ground plant biomass at harvest. So NUE is calculated at the time of harvest, i.e., end of the crop cycle. However, yield and grain protein content which represent the nitrogen use efficiency are inversely related, so it very important for breeders to design cultivation programs to achieve comparatively successful NUE without compromising grain yield (Oury and Godin, 2007) and to develop such cultivars, it is very important to understand the details of various traits which affect NUpE and NUtE and keeping this in consideration, processes and traits related to NUE are discussed in detail.
Traits Affecting Nitrogen Uptake Efficiency
Root Architecture
Root development and root system architecture are highly responsive to nutrient availability. To date, the root architectural plasticity traits, genetic basis, mechanism, regulation, and function (Ford et al., 2006) associated with nutrient-uptake are not well understood. Root architecture is considered as a strong aspect for the improvement of NUE (Forde, 2014; Fan et al., 2017; Li et al., 2017). Broadly, root systems in cereal crops (wheat, rice, maize) can be divided into two parts a) embryonic (seminal roots), b) post-embryonic roots (crown roots). Nutrient absorption including nitrogen is well explained by “steep, cheap, and deep” root morphology (Lynch, 2013). It defines that the primary roots are involved in nitrogen acquisition from deeper horizons, whereas lateral roots with steep angles are involved in covering a greater volume of soil (Mandal et al., 2018). Lateral roots are reported to be more sensitive toward changing nitrogen content and biotic and abiotic stress. Low nitrogen content at the initial stages positively affects the lateral root initiation but severe nitrogen deficiency hampers root emergence and elongation. A high ratio of nitrate to ammonia in the soil showed a positive effect on lateral root length (Qin et al., 2017).
Addressing the challenge of efficient nutrient uptake by understanding the role of root traits in nutrient uptake and dissecting the genetic basis to maximize the potential to breed high yielding resource-efficient varieties of cereal crops using modern biotechnological and bioinformatic approaches is required. Dissecting the hidden potential of root traits for improving nutrient uptake and revealing the significant marker associations to be deployed in molecular breeding to breed resource-efficient varieties is mandatory. The exploitation of an appropriate root prototype and robust marker-trait associations/QTL/candidate genes may address the challenge of nutrient deficiency and low nutrient uptake. Efforts involving designing robust root system architecture providing a combination of different root traits (nodal root, root hair length, root hair density, root length density, root dry weight,% lateral root, root branching, root thickness, and root volume) may be a solution to the problem of efficient nutrient uptake especially nitrogen (N) (Figure 4). Various above and below ground factors are reported to play a significant role in the development of root architecture (Li et al., 2017). Different root traits are important for nutrient uptake at different stages of crop growth and development. Root size and morphological features are directly correlated with nitrogen uptake efficiency, as it is observed that among different forms of nitrogenous compounds present in soil especially nitrate easily escapes the soil system through leaching which initiates the need to enhance nitrogen uptake by improving root architecture including depth, density, and capacity of roots for post-anthesis N uptake (Foulkes et al., 2009). Primary studies to establish the molecular control of root architecture were carried out in Arabidopsis but several homologs were reported in rice and other cereal crops (Forde, 2014; Shahzad and Amtmann, 2017). Previous studies reported several genes/proteins associated with root architecture in different cereal crops. In rice, miR444a/ANR1 induces lateral root formation under low nitrate conditions (Yan et al., 2014). EL5, a plant-specific ATL Family E3 Ubiquitin ligase, maintains the viability of root apical meristem (Mochizuki et al., 2014; Nishizawa et al., 2015). OsMADS25 was reported to be involved in lateral and primary root development (Yu et al., 2015) and nitrate assimilation-related component 1 (OsNAR2.1) induced lateral root formation in rice (Huang and Schiefelbein, 2015). Similarly, in wheat, NAM, ATAF, and CUC transcription factor (TaNAC2-5A) promoted root growth (He et al., 2015) and the NUCLEAR FACTOR Y (TaNFYA-B1) accelerated root development (Qu et al., 2015).
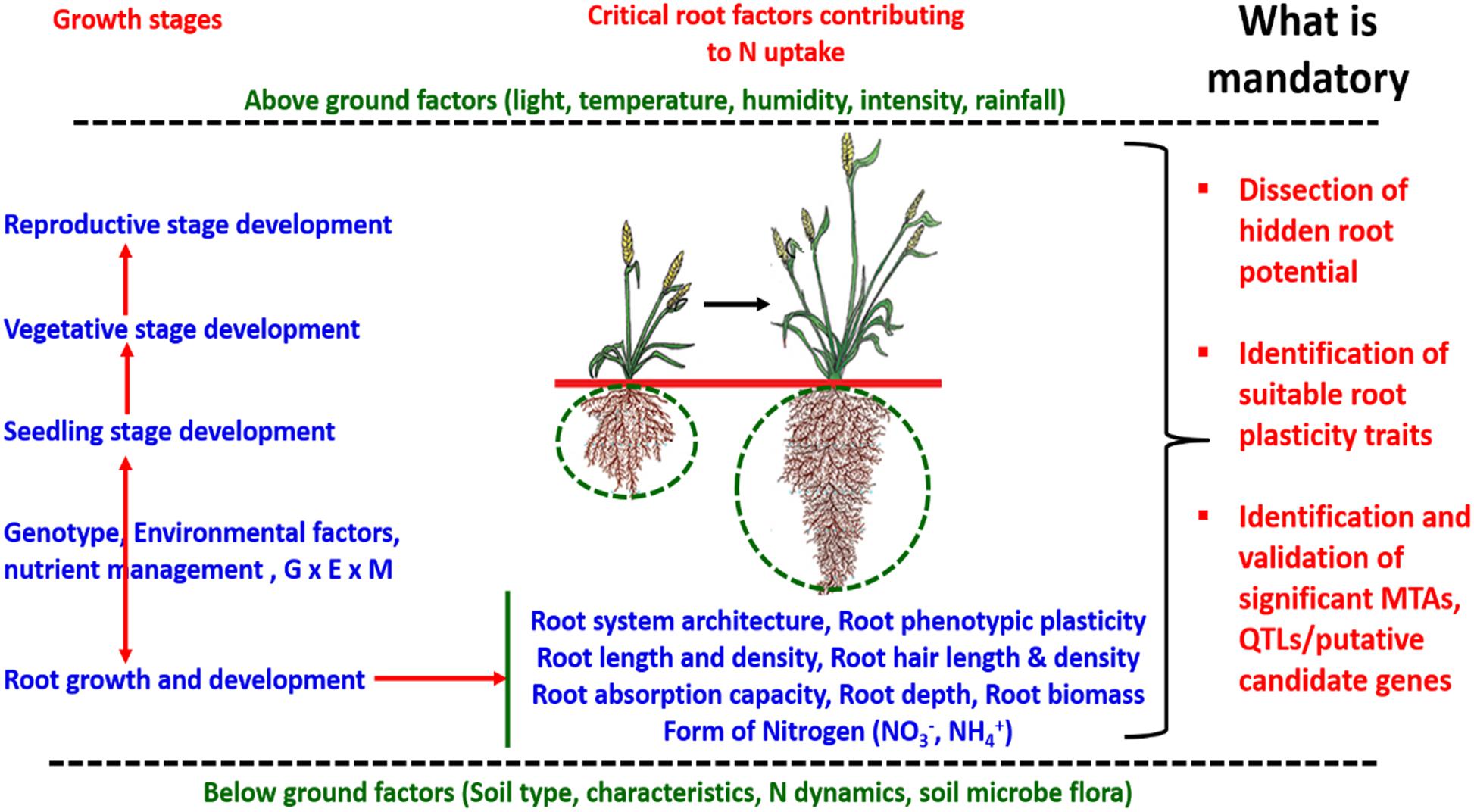
Figure 4. The schematic representation of the role of above and below ground factors, genotype-environment-nutrient interactions playing significant roles in developing root architecture at different stages of growth and development for efficient nitrogen uptake in cereal crops.
A specifically in-depth role of root proliferation to increase nitrogen uptake was reported in wheat (Carvalho and Foulkes, 2011). So, the rooting profile mandate for nitrate uptake at lower depths was analyzed by measuring root length density with a threshold value of 1 cm/cm3 (Gregory and Brown, 1989), where root length density is a measure of root length per unit volume of soil (Ford et al., 2006; Reynolds et al., 2006). Although these threshold values for nitrogen uptake are not absolute and are highly influenced by both genetic and environmental factors. A very high genetic variability in the root system was observed in wheat (Ehdaie et al., 2003; Ford et al., 2006). Further, several environmental factors including soil type and available nutrient resource majorly affects the root distribution characteristics. Aegilops tauschii (D genome), the wild relative of wheat, was reported to have deeper rooting systems (Reynolds et al., 2000). The identified candidate genes may be targeted in genomics-assisted breeding programs for the development of cultivars with relatively deep rooting systems. Under low nitrogen conditions, increase in the ratio of root biomass to total plant biomass (root dry weight ratio; RDWR) was observed to maintain the functional equilibrium between the roots and shoot growth (Robinson, 2002). An intricate relation between root and shoot development in higher plants was reported, viz., active shoot growth ensures carbohydrate supply to roots to enhance the root function, whereas active root growth improves shoot growth by xylem flow of the required amount of nutrients and phytohormones to the developing shoots. The simultaneous growth of root and shoot ensured enhanced crop productivity (Yang et al., 2004; Zhang et al., 2009). The increase in root-shoot biomass even at a low nitrogen supply ultimately enhanced the crop growth rate (CGR) contributing to higher grain yield and improved NUE (Ju et al., 2015).
Along with root length and density, another important trait under consideration for enhanced nitrogen uptake is root hairs which have a substantial role in increasing the surface area of roots to potentially increase the nitrogen uptake by active transport. Among several candidate genes, two genes, i.e., RTH1 and RTH3, for root hair elongation have been identified in maize (Hochholdinger and Tuberosa, 2009). Although root structure and function seem to be an outcome of the additive effect of multiple genes so it is difficult to target single genes for amplified nitrogen uptake (Hall and Richards, 2013). The approach to enhance nitrogen uptake includes pyramiding multiple beneficial traits marker-assisted selection. The quantitative trait loci (QTL) for traits including root length, root hair number, root density, root angle, and root-to-shoot ratio are well established in wheat (Bai et al., 2013; Atkinson et al., 2015), but there is need to understand the mechanism of orchestrated expression of multiple traits affecting root architecture to positively influence nitrogen uptake (Lynch, 2007).
Root N Transporter Systems
Substrate specific transporters are involved in nitrogen uptake in several forms including nitrate (NO3–), ammonium (NH4+), amino acids or peptides, and urea (Crawford and Glass, 1998; Kant, 2017). Nitrogen accumulation by root is an active process mediated by a specific type of transport protein for nitrogen uptake. The inorganic form of nitrogen which is most prominent in the rhizosphere is NO3–, along with it NH4+ is also present in the soil but its concentration is significantly less compared to NO3– concentration (Nieder et al., 2010). The uptake and transport of nitrate in plants is mediated by five transporter families including; the Nitrate Transporter 1/Peptide Transporter (NPF) family (Léran et al., 2014), the Nitrate Transporter 2 (NRT2) family, the Chloride Channel (CLC) family, the Slow Anion Associated Channel Homolog (SLC/SLAH) family, and aluminum-activated malate transporters (ALMT) (Li et al., 2017). Among the five families mentioned above NPF and NRT2 were reported to be associated with nitrate uptake and their localization in plants. The primary uptake of both NO3– and NH4+ is mediated by diffusion or mass flow, respectively, which ensures entry of both inorganic forms to root apoplast (Mandal et al., 2018). Active transport is the prominent mechanism that further ensures the transport of nitrogenous compounds through several layers of ground tissue leading to the plant vascular system (xylem). Several types of plasma membrane-associated transporter proteins were reported to be involved in active transport and classified as high- and low-affinity transporters (Loqué and Wirén, 2004; Glass, 2009; Dechorgnat et al., 2010). Based on affinity and NO3– concentration in the rhizosphere, three types of transport system including inducible high-affinity transport system (iHATS), constitutively expressed high-affinity transport system (cHATS), and non-saturable low-affinity transport system (LATS) are active in higher plants. iHATS is triggered at a low concentration of NO3– (1 to 200 lM) and its functioning varies with plant species and environmental condition (Siddiqi et al., 1991; Feng et al., 2011). In wheat, iHATS has a Michaelis constant (Km) approximately 27 lM and needs an induction period of 10 h before initiating the transport process (Goyal and Huffaker, 1986). cHATS as the name suggests is constitutively expressed and displayed on the plasma membrane even in the absence of NO3–. A common property of both cHATS and iHATS is that they are saturated after the external NO3– concentration reaches a certain threshold. The third one, LATS has low-affinity transporters and is activated at the higher concentration of NO3– in the soil (250 lM). Unlike cHATS and iHATS, LATS includes a non-saturable type of transporters (Siddiqi et al., 1991; Von Wirén et al., 1997). Two major gene families involved in NO3– transport in higher plants include NRT1 and NRT2. NRT1/PTR represents nitrate transporters, the peptide transporter family (NPF), and the NRT2 family known as the major facilitator superfamily (MFS) (Léran et al., 2014). The high-affinity transport system in wheat is reported to be regulated by five genes (TaNRT 2.1, TaNRT 2.2, TaNRT 2.3, TaNAR 2.1, and TaNAR2.2) and these transporters are activated by the plant growth hormone abscisic acid in the absence of NO3– (Cai et al., 2007). Among the three transporter systems discussed so far, LATS is involved in NH4+ uptake and LATS belongs to NH4+ permeases in the ammonium methylammonium permeases/transporter/Rhesus (MEP/AMT/Rh) family (Wirén and Merrick, 2004). The ammonium transporters (AMTs) are considered to improve NUE by generating the AMT mutant lines and analyzing the associated phenotypic effect. In rice, twelve different AMT-associated genes were broadly classified into two subfamilies: OsAMT1 and OsAMT2 (Li et al., 2017; Xuan et al., 2017). Post translational events such as phosphorylation controls the activity of these transporters which keeps in check the level of ammonia accumulated in the plant system (Li et al., 2017; Xuan et al., 2017).
Along with the above discussed inorganic forms, it is important to consider the mechanism of urea uptake by the root system as it is used as synthetic fertilizer in conventional agriculture (Andrews et al., 2013; Karamos et al., 2014). It is well established that the transporter for urea uptake is expressed in roots and leaves, which can mediate efficient uptake of urea followed by its hydrolysis to use it efficiently in anabolic processes (Witte, 2011). In rice, two types of transporters for urea uptake with linear Michaelis–Menten kinetics (Wang et al., 2011) was reported. In wheat, the urea uptake is very low as compared to other inorganic N sources making it difficult to measure the kinetics of urea uptake (Criddle et al., 1988). Among the five transporters present in the plant system; one is a high-affinity transporter and the rest are low-affinity transporters. High-affinity transporters come under the category of symporter which mediates the co-transport of urea and H+ ions, whereas low-affinity transporters are intrinsic proteins (tonoplast intrinsic protein, TIP) working as channel proteins in a pH independent manner. The expression of high-affinity urea transporters is generally regulated by ammonia, nitrate, and urea (Reddy and Ulaganathan, 2015). However, urea is majorly used as an N fertilizer in Asian agriculture but there is a need to further investigate the mechanism of urea uptake and its metabolic conversion to useful physiological components in plant systems.
Effect of Rhizospheric Associations
The rhizosphere is the region of soil that comes under direct contact with the root system and the organisms surviving in this region highly influence the mineral uptake including nitrogen uptake by roots (Richardson et al., 2009). Many higher plants including wheat are reported to secrete a variety of exudates mainly organic acids and certain sugars which directly influence the physiological processes of microorganisms living in association with the root system (Nguyen, 2003). Along with this, several environmental factors including climatic conditions, water level, soil type, and farming practices also affect these microbial communities (Mazzola et al., 2004). Rhizosphere microbial ecology is also reported to be varied concerning different wheat cultivars (Kapulnik et al., 1987; Germida and Siciliano, 2001; Wu et al., 2001). There are certain cultivars possessing the efficiency to positively influence root architecture which favors N availability in the rhizosphere, systemic plant metabolism, and microbial photoprotection. Along with beneficial or symbiotic organisms, there are certain microorganisms including bacteria and fungi, which compete with the plant root system for common nitrogen sources in the rhizosphere, i.e., ammonia and nitrate (Nelson and Mele, 2006). Along with competing for the common nitrogen source, certain microorganisms negatively influence the nitrogen uptake by channeling the available inorganic nitrates to gaseous nitrogen by process of denitrification (Herold et al., 2012). In higher plants, it is also observed that certain secondary metabolites released by roots have an inhibitory effect on the denitrification process. As discussed above, denitrification converts the nitrogen into an unavailable form, so inhibition of such processes positively influences the nitrogen uptake, but such a mechanism is not well elucidated in cultivated cereal crops (Bardon et al., 2014). There were several attempts made to transfer the beneficial traits influencing root-microbial from wild relatives of cultivated cereal crops. A chromosome of Leymus racemosus, a wild relative of wheat possessing the ability of nitrification inhibition in the root rhizosphere, was introduced into cultivated varieties (Subbarao et al., 2007; Ortiz et al., 2008).
The nitrogen uptake by root can be improved by improving nitrogen fixation. Unlike legumes, in cereals the nitrogen fixation is not dependent on symbiotic root-nodulating bacteria, whereas this process in cereals including wheat is entailed by other non-nodulating N-fixing bacteria contributing a subtle amount of fixed nitrogen to roots in the rhizosphere (Behl et al., 2012). Although, these N-fixing bacteria form the natural component of the root rhizosphere in wheat (Nelson and Mele, 2006; Venieraki et al., 2010), but the artificial introduction of N fixers may enhance nitrogen uptake which positively influences the yield (Behl et al., 2012; Neiverth et al., 2014). Genetic engineering is the major solution that can introduce the legume-like system of nitrogen fixation from bacteria to the cereal crops (Geddes et al., 2015). Previous studies reported several strains of bacteria that can be used as cereal seed inoculants to naturally fix nitrogen or can act as potential hosts to receive the gene clusters for nitrogen fixation. The most potent strains that can be targeted for nitrogen fixation are non-host-specific endophyte Pseudomonas stutzeri and epiphyte Klebsiella oxytoca known to colonize with the root system of rice and wheat (Triplett et al., 2008). Bacterial systems carry a diverse range of nif gene clusters ranging from 11 kb to 64 kb operons. The conserved region among these operons includes nitrogenase (nifHDK) and cofactor (FeMoCo) (Boyd et al., 2015) and the rest of the region in the operon specifies nitrogen fixation under different environmental conditions (Pascuan et al., 2015; Poudel et al., 2018). Ryu et al. (2020) compared diverse species, natural nif clusters, and engineering strategies to design bacteria capable of delivering fixed nitrogen to the cereal crop. Rhizosphere-associated increase in NUE is dependent on nitrogen-fixing microbial associations in cereals (Mus et al., 2016). Although there is less evidence on the occurrence of efficient diazotrophic associations in cereal crops (Van Deynze et al., 2018). Certain examples of fixed atmospheric N2 being transferred to cereals include associations between Azoarcus sp. strain BH72 and Kallar grass (Hurek et al., 2002), Herbaspirillum seropedicae and rice (Gyaneshwar et al., 2002), and Klebsiella pneumoniae and wheat (Iniguez et al., 2004). Several mechanisms controlled by microorganisms in the rhizosphere affecting the root architecture by increasing production of growth hormones including auxins (Ortíz-Castro et al., 2009), cytokinins (Cassan et al., 2009; Moubayidin et al., 2009) or gibberellins were detected. The gibberellins secreted by several bacteria and fungi (Bottini et al., 2004) in the rhizosphere enhanced the primary root elongation and lateral root extension in wheat (Upadhyay et al., 2009). The root-associated organisms produced a vast effect not only on nitrogen uptake but also on triggering plant defense systems against pathogenic organisms (Couillerot et al., 2011; Almario et al., 2013). In wheat, the pathogenic defense-related transcriptional accumulates increased in wheat when inoculated with the bacterium Pseudomonas fluorescens Q8r1-96 (Maketon et al., 2012). Overall, the microbial association in respect to nitrogen uptake is a broad subject that needs to be considered and explored for further improvement of nitrogen uptake efficiency in wheat and other cereal crops.
Traits Affecting Nitrogen Utilization Efficiency
Nitrate Assimilation
In higher plants, the major pathway for inorganic nitrogen assimilation into the carbon skeleton is nitrate reduction (Ali, 2020). The mechanism of nitrate assimilation involves reduction and its conversion into biologically active forms as described in detail in Figure 5. Nitrate, the primary form of nitrogen taken up by the roots, is reduced to nitrite by NAD(P)H-dependent enzyme nitrate reductase (NR) inside the cytoplasm (NR; EC 1.7.1.1). Nitrate reductase exists in homodimeric forms with subunits of about 900 amino acids (110kDa). Each subunit is associated with FAD, heme-Fe, and Mo-molybdopterin (Mo-MPT). Nitrate reductase along with a molybdenum cofactor (MoCo) is needed to catalyze the rate limiting step of nitrate reduction. As it is the major rate limiting step, it is highly regulated at the gene expression level by several factors (Campbell, 2002). NR activation is the very first step in the utilization of absorbed nitrogen for its conversion into biologically active molecules including amino acids, nucleic acid, and other nitrogen-containing biomolecules. Two genes encoding NADH-dependent nitrate reductase was reported in hexaploid wheat (Boisson et al., 2005).
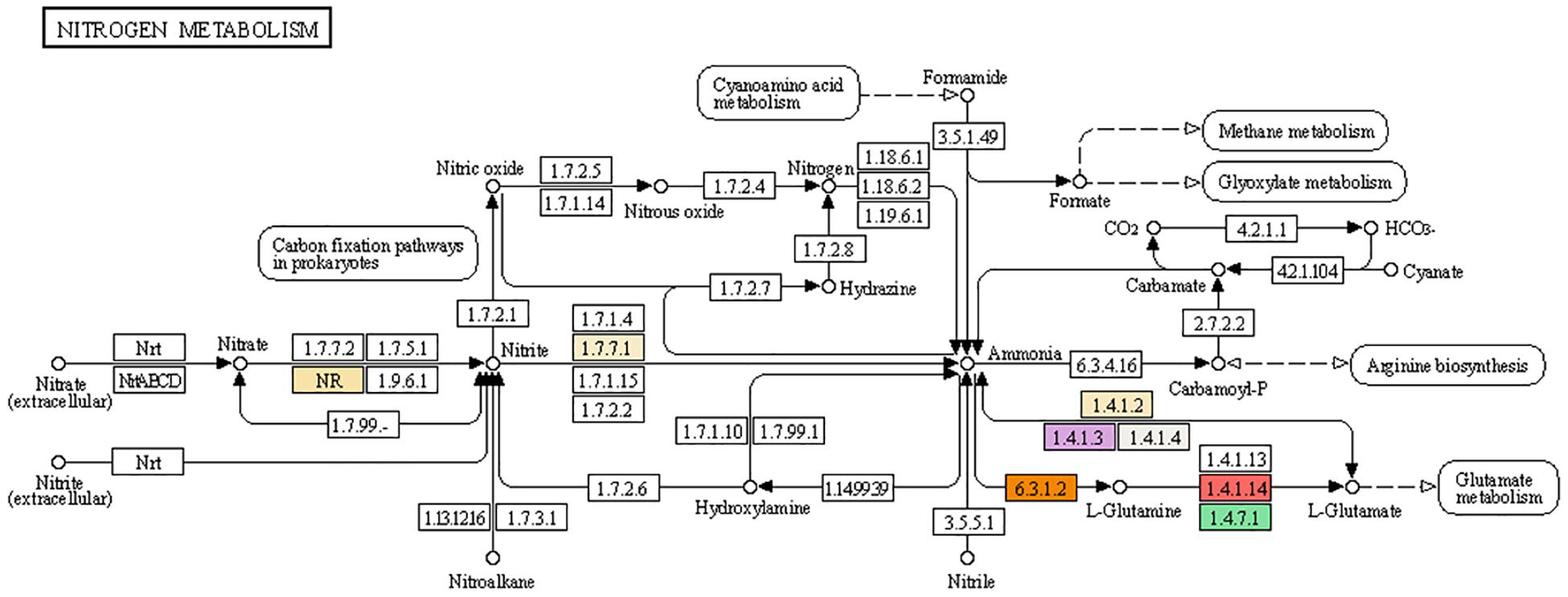
Figure 5. Mechanism of nitrogen assimilation and integrated pathways with different enzymes involved in channelizing nitrate toward amino acids and proteins. Nitrate and nitrite are the primary nitrogen source for cereal crops. Nitrate is converted to nitrite by regulatory enzyme: nitrate reductase (NR, highlighted in creamish color) (1.7.7.2). Nitrite is further reduced to ammonia by nitrite reductase (1.7.7.1, highlighted in creamish color). Ammonia is channelized for amino acid synthesis primarily by action of glutamate dehydrogenase (1.4.1.2, highlighted in creamish color¶and 1.4.1.3, highlighted in purple color). Glutamate is further converted to glutamine by glutamine synthetase (6.3.1.2, highlighted in orange color; 1.4.7.1, highlighted in green color and 1.4.1.14, highlighted in red color). Glutamine and glutamate are the primary amino acids routed for protein synthesis. Partial fraction of ammonia is involved in arginine metabolism using carbamoyl phosphate as C-skeleton. In addition to the primary nitrogen metabolism, secondary nitrogen sources such as nitric oxide, nitrous oxide, nitroalkane, nitrile, hydrazine, and formamide also contribute in nitrogen metabolism. Cyanoamino acid metabolism releases formamide which converts to formate for further used in methane and glyoxylate metabolism.
The second step catalyzed by nitrite reductase reduces the nitrate to ammonia (NO2– to NH4+) and this enzyme is compartmentalized in the plastids (NiR; EC 1.7.7.1; Sétif et al., 2009). The electron donor for the reduction of nitrite into ammonia is provided by ferredoxin by forming an enzyme-ferredoxin complex (Sakakibara et al., 2012). The incorporation of ammonium into the carbon skeleton is mediated by 2-oxoglutarate of the TCA cycle and amino acid transamination reactions to form glutamate and glutamine. Ammonia forms after two subsequent reactions are incorporated into an organic form. Glutamate is the amino acid that acts as a primary receiver of ammonia and this reaction is catalyzed by consecutive action of the two enzymes. Glutamine synthetase catalyzes the first reaction (GS; EC 6.3.1.2; Lea and Miflin, 2011) and is a major regulatory step in channeling the inorganic form of nitrogen to its organic form.
GS has two isoforms and works in different cellular compartments, the first isoform (GS1) is mainly expressed in the cytosol of several organs such as leaves, roots, and phloem cells, whereas the second isoform (GS2) is expressed in plastids of chloroplast, roots, and etiolated tissues (Lea and Miflin, 2011). In cereals including wheat the GS2 isoform is expressed majorly throughout the plant development cycle and its activity decreases post-anthesis, whereas cytosolic isoform GS1 isoenzymes show constitutive expression in the phloem and senescing leaves (Christiansen and Gregersen, 2014; Yamaya and Kusano, 2014). Another enzyme glutamate synthase (GOGAT; EC 1.4.7.1; Suzuki and Knaff, 2005) acts in conjunction with the primary enzyme and catalyzes glutamate synthesis (GS) by incorporating carbon skeletons in the 2-oxoglutarate form into the cycle. Further, these two amino acids act as principal donors of amino groups for the formation of other amino acids, nucleic acids, and other nitrogen-containing compounds (Lea and Miflin, 2011). GOGAT also exists in two isoforms, each with a role in N assimilation or N recycling. One isoform of GOGAT is ferredoxin-dependent isoenzyme (Fd-GOGAT) in reassimilation of photorespiratory ammonia, whereas the other isoenzyme of GOGAT is NADH dependent (NADH-GOGAT; EC 1.4.1.14) with its role in the synthesis of amino acids including glutamate for growth and development in photosynthetic and non-photosynthetic organs (Lea and Miflin, 2011). It is described from mutational studies that GS and GOGAT contribute to the assimilation of about 95% of the ammonia available in plant tissues (Lea and Miflin, 2011). The amino acid formed is utilized in protein formation and production of other metabolites important for growth and development and to ultimately increase productivity (Howarth et al., 2008).
Nitrogen assimilation and utilization is affected by several factors but carbon metabolism is the major player affecting NUtE. The effect of photosynthesis and carbon metabolism on nitrogen accumulation was studied in different plants to analyze the role of various carbon metabolites in nitrogen utilization. The change in photosynthetic rate was reported to affect nitrogen assimilation and vice versa. Carbon fixation depends on nitrogen assimilation, as it is important to provide enzymes and pigments for photosynthesis (Kant et al., 2012) that makes nitrogen an important component describing the photosynthetic activity and crop yield. Similarly, nitrogen assimilation depends on carbon metabolism which provides the electron donors for ammonium formation, the carbon skeleton (ketoglutarate) for ammonia assimilation in GS/GOGAT pathway, malate as a counter anion, carbon precursors for other amino acid pathways, and ATP for nitrate transport into the cell (Xu et al., 2012). Therefore, several factors were reported to regulate the nitrogen assimilation and utilization process which must be considered while describing NUE (Ali, 2020). Nitrogen use efficiency is related to nitrate acquisition which can further be enhanced by altering enzymes and proteins associated with nitrate assimilation utilizing different biotechnological approaches. There is strong need to target multiple mechanisms/enzymes/factors rather than approaching single-point rate-limiting regulation to enhance NUE. Therefore, future research is dependent on in-depth understanding of the regulatory mechanisms of N metabolism and the pathways linking C and N assimilation (Ali, 2020).
Canopy Architecture and Photosynthesis
Photosynthesis is an important physiological process occurring in higher plants. The most abundant protein in the biosphere is Rubisco. Rubisco is a major regulatory enzyme for the process of converting the inorganic form of energy to the organic form through the Calvin cycle. As Rubisco is a protein biomolecule, most of the nitrogen accumulated in the leaf is represented by the Rubisco content which in return represents the photosynthetic activity of mesophyll tissues. It is reported in wheat that approximately 75% of N in leaves is channeled for photosynthetic processes mediated by the Rubisco enzyme (Evans, 1983). So, it is observed that in nitrogen-limited conditions there is a reduction in Rubisco content which decreases the photosynthetic activity per unit area of leaf and ultimately decreases the organic matter production. Photosynthetic activity is correlated to leaf morphogenesis as it is the primary organ involved in carbon dioxide fixation, so leaf architecture directly affects yield in crop plants (Guo et al., 2019). It is reported earlier that leaf width affects grain- and panicle-related traits (Fu, 2019). A study on rice crop suggested that leaves were the major photosynthetic organ for plant morphological development, and spatial arrangement of leaves was reported to be strongly correlated to rice yield (Zhang et al., 2020). The reduced photosynthetic activity leads changes in canopy structure including reduced leaf expansion and decreased total leaf area (Sylvester-Bradley et al., 1990; Monneveux et al., 2005). The nitrogen uptake and utilization enhanced the source and sink capacities, thereby increasing the dry matter accumulation and ultimately improving the crop yield. Traits such as spike shape, plant height, and biomass accumulation in rice were reported to be associated with nitrogen uptake and utilization (Xu et al., 2020).
Grain yield in cereals is the outcome of coordinated regulation of multiple factors including photosynthesis, nitrogen uptake, and photorespiration (Sinclair et al., 2019). The correlation between yield and the absorption, uptake and utilization of nutrients played significant roles in improving rice yields. The complete understanding of the regulatory mechanisms and pathways involved in the transport of nutrients to the stems, sheaths, and leaves and then finally to the grains and how to improve the slow and ineffective filling of grains is mandatory (Li and Cui, 2014). It is reported that LWS5/D1-mediated GA signaling regulates the GPCR (G-protein coupled receptors) in rice (Miyako et al., 2000), ultimately improving nitrogen uptake, grain yield, and regulating leaf morphology (Zhu et al., 2020).
The optimization theory indicated that the equal coefficient of the light gradient (KL) and nitrogen (KN) positively increased canopy photosynthesis (Moreau et al., 2012). Further, the nitrogen gradient reported in wheat was less steep than in the optimization theory (Moreau et al., 2012). NUtE was affected by the photosynthesis rate per unit of nitrogen. In C3 crops such as wheat around 2 g N/m2 of the leaf N concentration increased the photosynthesis to 20–30 lmol CO2/m2/s in the light-saturated condition.
Exploiting the tendency of wheat cultivars to accumulate 2.0 g N/m2 under the favorable conditions may be another important aspect to increase NUtE. Genetic variability in specific leaf nitrogen (SLN, leaf nitrogen content per unit leaf area) was observed to be varied from 1.4 to 2.6 g/m2 for the 144 durum wheat genotypes (Araus et al., 1997), from 2.1 to 2.4 g/m2 for the 17 durum wheat cultivars (Giunta et al., 2002), and from 1.4 to 2.2 g/m2 for the 16 bread wheat cultivars (Moreau et al., 2012). The heritability for straw nitrogen including stem, leaf lamina, and leaf sheath at anthesis for winter wheat was > 0.60 under low nitrogen (Laperche et al., 2006), indicating the possibility for selection for this trait.
Photorespiration catalyzed by Rubisco (ribulose-1,5-bisphosphate carboxylase/oxygenase) activates the fixation of oxygen and release of previously fixed CO2 and NH3 at the cost of energy consumption. Consequently, the fixed nitrogen is lost from the metabolic cycle. Several components can be targeted to increase the photosynthetic activity by decreasing photorespiration through Rubisco, increasing carboxylase activity of Rubisco and by introducing mechanisms to increase carbon concentration in the vicinity of Rubisco. Increased photosynthetic activity will ultimately increase NUtE (Reynolds et al., 2000; Long et al., 2006; Murchie et al., 2008; Zhu et al., 2010). Genetic diversity can be exploited to strategize the required modification in photosynthetic components. The 30% improvement in photosynthetic activity was mediated through reduced photorespiration whereas other mechanisms hold the potential of 15–22% enhancement of photosynthetic activities (Long et al., 2006). Further study is required to better understand the molecular processes regulating the signaling pathways for leaf architecture, photosynthesis, and photorespiration. Gene editing technologies such as CRISPR-Cas9 or the expression of specific promoters can be used to alter the genes regulating signaling pathways in leaves, resulting in diverse germplasms with high yield potential (Zhu et al., 2020). Precision breeding techniques are required to improve the breeding efficiency among cereals for traits enhancing NUE.
Post-anthesis N Remobilization and Senescence Dynamics
Nitrogen uptake from roots is further mediated by its translocation from roots to leaves through the transpiration stream where roots act as source and growing tissues such as leaves and buds act as a sink. Although this source-sink relation changes with the developmental stage, during maturity the mature leaf acts as source, where proteins are degraded and release nitrogen which is remobilized to younger leaves and seeds (Lemaire et al., 2007).
Before discussing nitrogen remobilization, it is important to consider that plants are a better option for nitrogen storage as compared to soil. In soil, nitrogen is readily converted into a different reduced and oxidized form along with processes such as denitrification which decreases the availability of the biologically active form of nitrogen. Therefore, it is important to have crop plants which can efficiently store nitrogen in different tissues and maintain it in the biological system through accumulation in grains by remobilization (Hofman and Van Cleemput, 2004).
Nitrate remobilization from leaves to developing parts is a valuable determinant of NUE during the grain-filling stage. The crop yield depends on the remobilized nitrogen. Photosynthates stored in the old leaves act as a major source of recycled nitrogen for remobilization to developing seeds. Remobilization is dependent on the mechanism of autophagy which is basically regulated by several senescence-associated genes (ATG and metacaspases) that get induced during plant senescence (Havé et al., 2016). Nitrogen replenishment during the reproductive stage is mediated by tissue-specific transporters. The genes which code for nitrogen transporters such as AtNRT1.7 are further controlled by nitrogen limitation adaptation (NLA) regulators which are further controlled by miRNA827 (Liu W. et al., 2016). The strict control of nitrogen transporter expression suggests tight regulation of the remobilization process. Along with transporters, the enzymes such as ferredoxin-dependent glutamate synthase (OsFd-GOGAT) involved in ammonia recycling played a significant role in the remobilization process (Zeng et al., 2016).
In cereal crops, approximately 50–90% of nitrogen accumulated in grains were contributed to by remobilized nitrogen from leaves (Masclaux-Daubresse et al., 2010). Flag leaf senescence can be used as a phenotypic marker to estimate the stage of nitrogen remobilization to grains (Uauy et al., 2006). Although, delayed senescence of flag leaf led to higher grain yield, which persists with the results suggesting an inverse relationship between grain yield and grain protein content. As Rubisco is the most abundant protein present in the chloroplast of photosynthetically active tissues, i.e., leaves, during remobilization Rubisco is a major contributor of nitrogen for protein accumulation in grains. It is suggested that older leaf tissue chloroplasts degrade first in comparison to other organelles due to upregulation of proteases. Autophagy is the underlying mechanism for chloroplast and Rubisco degradation during senescence (Ishida et al., 2014; Li F. et al., 2015). The process of autophagy is mediated by exopeptidases and endopeptidases present in cell vacuoles (Ishida et al., 2014). The amino acids released after degrading the Rubisco protein transported via amino acid transporters belonged to the amino acid permeases (AAP) family (AAP1, AAP2, AAP3, AAP6, AAP7, AAP8 and AAP16) (Hunt et al., 2010; Taylor et al., 2015).
In wheat, nitrogen content in the above-ground tissue during anthesis is maximally in leaf lamina followed by the true stem, ear, and leaf sheath under optimal N supply (Barraclough et al., 2014; Gaju et al., 2014). However, under limiting nitrogen conditions the nitrogen content in the ear increases in comparison to other plant parts (Barraclough et al., 2014; Gaju et al., 2014).
The efficiency of post-anthesis nitrogen remobilization of true stem reserve N was low (48%) compared to the leaf sheath (61%) and leaf lamina (76%), but true stem acted as a major reservoir of nitrogen during harvest in well-fertilized crops. Theoretically, before anthesis true stem has a high capacity to store nitrogen which enhances nitrogen uptake and favors high NUpE (Foulkes et al., 2009). This condition of high nitrogen in stem (non-photosynthetic tissue) further facilitates the nitrogen translocation for grain filling without hampering photosynthetic capacity (Bertheloot et al., 2008), but to ensure benefits of storing nitrogen in non-photosynthetic tissue it is necessary to study the respiratory cost associated with it. Huge diversity among wheat germplasm for nitrogen storage and remobilization in non-photosynthetic tissues especially in stem during anthesis was reported (Kichey et al., 2007; Barraclough et al., 2014; Gaju et al., 2014). The shoot not only acts as the non-photosynthetic storage tissue for nitrogen but it also has regulatory control over N uptake from roots, and allocation to sink. The high accumulation of amino acid in phloem tissue positively affects nitrogen uptake from root followed by its assimilation in storage tissue (Zhang et al., 2015; Perchlik and Tegeder, 2017). Proper nitrogen partitioning in various tissues including shoot was reported to be important for C/N metabolism (Santiago and Tegeder, 2017).
Genetic diversity in terms of senescence and ‘stay-green’ phenotypes was reported in hexaploid wheat (Bogard et al., 2011; Gaju et al., 2011; Derkx et al., 2012; Naruoka et al., 2012). The stay-green phenotype acts as a mark for the capacity of a germplasm to stay green during the grain filling stage, i.e., retains its photosynthetic activity in comparison to other genotypes under consideration (Thomas and Smart, 1993). These factors including Rubisco degradation, stem nitrogen assimilation, and stay-green phenotypes provide major targets to ensure active remobilization of nitrogen to the grains post-anthesis. The molecular studies reported certain transcription factors such as NAM-B1 which efficiently increases nitrogen remobilization in wheat by accelerating the senescence of leaves (Uauy et al., 2006). The members of the WRKY and NAC transcription factors family acted as regulatory genes through their role in senescence under controlled environment conditions (Derkx et al., 2012). There was an association reported between QTL affecting leaf senescence, grain yield, grain protein content, and QTL for the anthesis period in a double-haploid mapping population of winter wheat. The post-anthesis nitrogen availability for grain filling depends upon leaf senescence and flowering time (Bogard et al., 2011). Grain yield and storage protein synthesis was reported to be highly correlated to nitrogen (N) uptake after anthesis and remobilization of nitrogen from pre-anthesis uptake synthesis (Dupont and Altenbach, 2003). Kichey et al. (2007) reported that a less significant fraction of grain storage protein was synthesized from post-anthesis nitrogen uptake. The aim of achieving both high grain yield and protein content in wheat depends on better understanding of the mechanisms behind post-anthesis nitrogen remobilization which can be exploited to achieve the desired outcomes under variable environmental conditions.
Grain Protein Content and Grain Yield
The endosperm is the major edible part of cereal grains and its nutritional value is defined by the composition of metabolic products accumulating in mature grain. Although, starch is the major constituent of the cereal endosperm, protein is also present in association with starch. Gluten storage protein forms the major fraction of protein accumulated in the endosperm, which is composed of an admixture of polymeric glutenins and monomeric gliadins. Overall, these storage proteins are from the 60–70% nitrogen store of seed endosperm. In wheat, the dough-making property essential for forming multiple consumable products including bread, pasta, and noodles is ensured by gluten protein and there is a need for the precise balance between the ratios of gliadin which ensure dough viscosity and glutenins responsible for dough elasticity. However, grain protein quality varies under different genetic backgrounds which affect the capacity of protein synthesis and nitrogen utilization (NUtE) (Shewry and Halford, 2002; Ravel et al., 2009). Environmental factors also affect several components of grain development including rate, duration of grain filling, and grain protein composition (Martre et al., 2003). Grain yield and grain protein content are two important components affecting NUtE but they are inversely related to each other (Oury and Godin, 2007; Bogard et al., 2010) which creates an obstacle in achieving both simultaneously. The metabolic competition between carbon and N fluxes for energy leads to the physiological basis of the inverse relation between grain yield and grain protein content (Munier-Jolain and Salon, 2005), so dilution in NUtE is in the effect of accumulated carbon-based compounds (Acreche and Slafer, 2009). The efficient nitrogen retained in grain can be calculated by considering grain protein deviation (GPD), which gives a measure of deviation from the regression between grain protein concentration (GPC) and grain yield. Identification of genotypes with higher GPC from an expected GY can be estimated by calculating GPD (Monaghan et al., 2001). Post-anthesis N uptake is mainly affected by genetic variability in cereal (Monaghan et al., 2001; Bogard et al., 2010, 2011). Along with it, another major consideration is that after anthesis the main source of nitrogen for grain is remobilization from other metabolically active tissues, rather than nitrogen uptake from the rhizosphere (Gaju et al., 2014), so there is a need to address the remobilization and efficient storage of nitrogen in photosynthetically active tissues to increase the GPD (Hawkesford, 2014). The increase of 16.6% grain yield in rice was reported with increasing nitrogen supply due to an increase in the productive tiller number (Liu X. et al., 2016). Similarly, yield improvement was observed in barley due to the improvement in the yield attributing components such as the number of productive tillers, grain size, and number of grains spike–1 (Beatty et al., 2010; Safina, 2010; Ghoneim et al., 2018).
Interactive Nitrogen Metabolism
Nutrient enrichment in plants depends upon interactive uptake, storage, mobilization, and translocation of micronutrients and macronutrients including nitrogen. These complex processes are regulated by coordinated interaction of multiple genes (Jin et al., 2013). Ionomic studies revealed variation in a given subset of elements in the rhizosphere leading to change in the macro- and micro-nutrient status of plants (Murgia and Vigani, 2015). Further, the nitrogen source in the rhizosphere affected the micro and macro-nutrient profile ultimately affecting core metabolic processes such as photosynthetic rate, NUE, growth, and yield (Na et al., 2014). Therefore, nitrogen use efficiency can be enhanced by synchronized increase in mineral uptake along with nitrogen. In a superficial view it was observed that decline in nitrogen content subsequently decreases uptake and utilization of other mineral nutrients including P, K, Mg, Ca, Cu, Fe, and Mn (Waters and Sankaran, 2011). Although nitrogen metabolism and its interaction with other nutrients varies with respect to environment, genotype, tissue, and nutrient. The K and P content in the roots were observed to be affected with varying nitrogen level as compared to Ca and Mg, whereas variation in K content was much smaller than Mg content in shoot (Shah et al., 2017). The low nutrient level was reported to elicit the expression of transporter proteins for coordinated uptake of nutrients such as nitrogen, phosphorous, and sulfur (Gojon et al., 2009). The synergetic effect of nitrogen fertilizer application led to cumulative uptake of nutrients such as P, K, Ca, Fe, Cu, and Mn in both leaves and roots (Shah et al., 2017).
As discussed in nitrate assimilation, nitrate reductase (NR) is essential for nitrate to nitrite conversion. NR activity is dependent on the presence of molybdenum cofactor (Moco) along with the availability of nitrite ions, growth condition, phosphorylation, and hormonal induction (Garg, 2013; Nemie-Feyissa et al., 2013). Molybdenum plays an essential role in nitrogen assimilation as it is a component of Mo-cofactor which is important for enzymes involved in plant growth and developmental processes. Mo act as cofactors for glutamate synthase enzymes involved in ammonia incorporation into amino acids (Liu et al., 2017). The deficiency of Mo led to poor nitrogen assimilation and plants showed symptom of nitrogen deficiencies indicating the key role of Mo in nitrogen metabolism (Kaiser et al., 2005). Mo was reported to significantly affect activities and expression of NR and other enzymes involved in nitrate assimilation (NiR, GS, GOGAT enzymes) (Imran et al., 2019). Remobilization from the older tissue was reported as a secondary mechanism to combat the nitrogen demand in case the uptake mechanism failed (Etienne et al., 2018). As most of the macro and micronutrients form part of the complex proteins including enzymes and pigments, degradation of these complex proteins channelizes the remobilization of nitrogen along with elements such as Zn, Cu, Mn, and Fe (Ono et al., 2013). A chromosomal locus in wild wheat was reported to regulate the early senescence and remobilization of protein associated with higher mobilization of N, Zn, Mn, and Fe from leaves to the seeds. Remobilization of Cu, Zn, and Fe was reported to be tightly linked with N catabolism during senescence (Waters and Sankaran, 2011). Similarly, delayed leaf senescence was observed to be associated with lower amounts of Fe and Zn in wheat seeds (Uauay et al., 2006). Nitrogen and other related nutrients (P, K, Ca, S, Mg, Fe, Zn, Cu, Mn) were reported to be negatively influenced by increased seed carbon concentration (Loladze, 2014). The seeds and leaves were reported to store large starch granules in chloroplasts under nitrogen-deficient conditions (Bhaskar and Syvertsen, 2005). Overall, the processes of macro and micronutrient assimilation, storage, and mobilization are interlinked, which provide multiple targets to enhance the NUE of cereal crops.
Biological Nitrification Inhibition
Nitrification is a key process, mediated by soil microorganisms, which converts reduced nitrogen (N) from ammonium (NH4+)/ammonia (NH3) (an immobile form of N) to nitrate (NO3–) (a mobile form of N) via nitrite (NO2–). The mobile nature of the nitrification product NO3– leads to the loss of N in the form of leaching causing groundwater pollution and leads to gaseous N2 via denitrification causing air pollution. Also, the nitrification process leads to the acidification of the soil facilitating the leaching of other important cations, i.e., Ca2+, Mg2+, and K+. The suppression of nitrification and denitrification minimizing the loss of ammonium fertilizer post-application are very critical steps to improve the retention of N fertilizer in soils, thus improving the N-use efficiency (NUE) of cereal crops with a view toward agricultural production and environmental sustainability. The use of synthetic inhibitors such as dicyandiamide (DCD), 3,4-dimethyl pyrazole phosphate (DMPP), 2-(N-3,4-dimethyl-1H-pyrazol-1-yl) succinic acid isomeric mixture (DMPSA), and nitrapyrin to reduce nitrification has been restricted because of the inconvenience of application, lack of availability, high cost, and their potential for environmental contamination. Considering these constraints, it is very much necessary to develop plant-derived environmentally friendly nitrification inhibitors to suppress soil-nitrifier activity which are referred to either as natural nitrification inhibitors (NNIs) or biological nitrification inhibitors (BNIs) (O’Sullivan et al., 2016). Recently, new methods have been developed to study soil N transformations to significantly reduce nitrification through root exudation. These compounds reportedly blocked the ammonia-monooxygenase and hydroxylamine-oxidoreductase enzymatic pathways in the soil microorganisms responsible for the oxidation of NH4+ to NO2–.
Genetic Factors Involved in Nue
QTL Related to NUE
NUE is a quantitative trait controlled by multiple genes (Yang et al., 2017). QTL mapping is a powerful tool to dissect the complexity of quantitative traits (Sun et al., 2012). Successful QTL mapping for complex traits including NUE is dependent on various factors such as the selection of suitable parents, appropriate population size, multi-location testing, and genome coverage. QTL can be affected by environmental variation. Constitutive QTL is consistent over environments, while adaptive QTL shows expression in a specific environment, or modulates their effect with changing environmental conditions. QTL analysis provides opportunities to identify the relationships between different traits. Co-localization of the QTL linked with different phenotypically correlated traits is good evidence that the traits might be genetically and functionally linked.
The NUE of cereal crops can be improved by employing classical genetics that involve both conventional breeding and QTL mapping in combination with MAS (marker-assisted selection). With the identification of agronomically relevant traits and the advances of next-generation sequencing, it is feasible to develop genomic knowledge for cereal crops even with complex genomes such as wheat (Guo et al., 2011). Identification of cheap, easy to use, widely distributed, co-dominant, trait-associated, and regulatory SNPs, candidate genes, and regulatory pathways could represent a significant milestone to accelerate the global hunt to improve wheat. Identification of genes with non-synonymous and regulatory SNPs could functionally differentiate accessions based on their distinct agronomic traits. Crop improvement programs can use association studies to access allelic diversity and to identify the best alleles to be assembled in superior varieties. The utilization of high-throughput genotyping techniques has the potential to increase marker density and may thus improve the accuracy of the identified QTL for nitrogen uptake and utilization-related traits. Several promising ways to improve NUE have been proposed such as focusing on root architecture (Foulkes et al., 2009) or senescence and remobilization (Gaju et al., 2011; Distelfeld et al., 2014). The ability to identify major and stable QTL controlling NUE-related traits and the use of this available information and knowledge in crop improvement breeding programs may condition part of the future cereal crop genetic gain.
Previous studies reported various QTL for NUE in the model crop plant, i.e., Arabidopsis and also in other cereals such as maize, rice, and wheat (Agrama et al., 1999; Gallais and Hirel, 2004; Ribaut et al., 2007; Li et al., 2017). Cho Y. I. et al. (2007) reported QTL for grain and shoot nitrogen content, harvest index, and physiological NUE under both low and the normal N on rice chromosomes 5, 7, 8, 9, and 10 using RILs. Similarly, Wei et al. (2012) identified QTL for nitrogen response, grain yield, and physiological NUE in rice. Further, significant QTL for grain yield, root NUE, and shoot dry weight have been detected in the wheat RIL population, i.e., TN18 × LM6 (Zhang et al., 2019). A total of 13 QTL including 7 QTL for nitrogen uptake and 6 for NUE were identified in rice grown under hydroponic culture (Zhou et al., 2017). The proportion of total phenotypic variation explained by QTL for NUP ranged from 3.16 to 13.99% and NUE QTL ranged from 3.76 to 12.34%. A major QTL on the short arm of chromosome 6B controlling grain protein content in wheat accounting for 66% of the phenotypic variation was reported and the functional gene named Gpc- B1 was cloned (Uauy et al., 2006). In winter wheat, the QTL associated with NUE on chr 1D, 6A, 7A, and 7D with LOD scores ranging from 2.63 to 8.33 and phenotypic variation up to 18.1% were identified (Brasier et al., 2020). Various novel NUE-related traits and alleles in adapted breeding materials (Fontaine et al., 2009), landraces (Pozzo et al., 2018; Van Deynze et al., 2018), and wheat wild relatives (Hu et al., 2015) were identified. QTL associated with NUE in rice were mapped using a recombinant inbred line (RIL) population on chromosome 6 (qNUEP-6; Shan et al., 2005) and on chromosome 9 (pnue9; Cho Y. I. et al., 2007). Thus, the research work carried out on cereal crops such as rice, maize, and wheat set a precedence for NUE research in other cereal crops such as barley (Cho Y. et al., 2007; Xu et al., 2014; Li P. et al., 2015; Lei et al., 2018; Mandolino et al., 2018).
Cormier et al. (2016) proposed that the identification of the genomic regions (QTL) associated with nitrogen response would enable more efficient cultivar selection. This approach allows breeders to efficiently screen germplasm and the genetic markers associated with nitrogen response, assisting in the development of high nitrogen use efficient cultivars. Previous studies have been conducted in rice and wheat to identify the novel traits, alleles, genes/QTL, adapted breeding lines, landraces, and wild relatives improving NUE differences in cereal crops. Genes/QTL influencing nitrogen uptake have been mapped in wheat under different doses of fertilizer application using bi-parental populations (An et al., 2006; Laperche et al., 2007b; Xu et al., 2013; Mahjourimajd et al., 2016). QTL for nutrient uptake was reported to be collocated with QTL for root hair length (Sandhu et al., 2015) and grain yield with root architectural plasticity traits (Sandhu et al., 2016) in rice. Several genetic loci for agronomic traits related to nitrogen use and grain yield have been mapped in the chromosomal regions containing GS2 in wheat and rice (Prasad et al., 1999; Obara et al., 2001; Yamaya et al., 2002; Habash et al., 2007; Laperche et al., 2007b; Fontaine et al., 2009; Yamaya, 2011), suggesting the role of the genomic region surrounding GS2 in breeding wheat and rice varieties with improved agronomic performance and nutrient use efficiency. Detailed descriptions of the QTL associated with nitrogen use efficiency-related traits and nitrogen use efficiency in rice are presented in Tables 1, 2, respectively and in wheat are presented in Tables 3, 4, respectively.
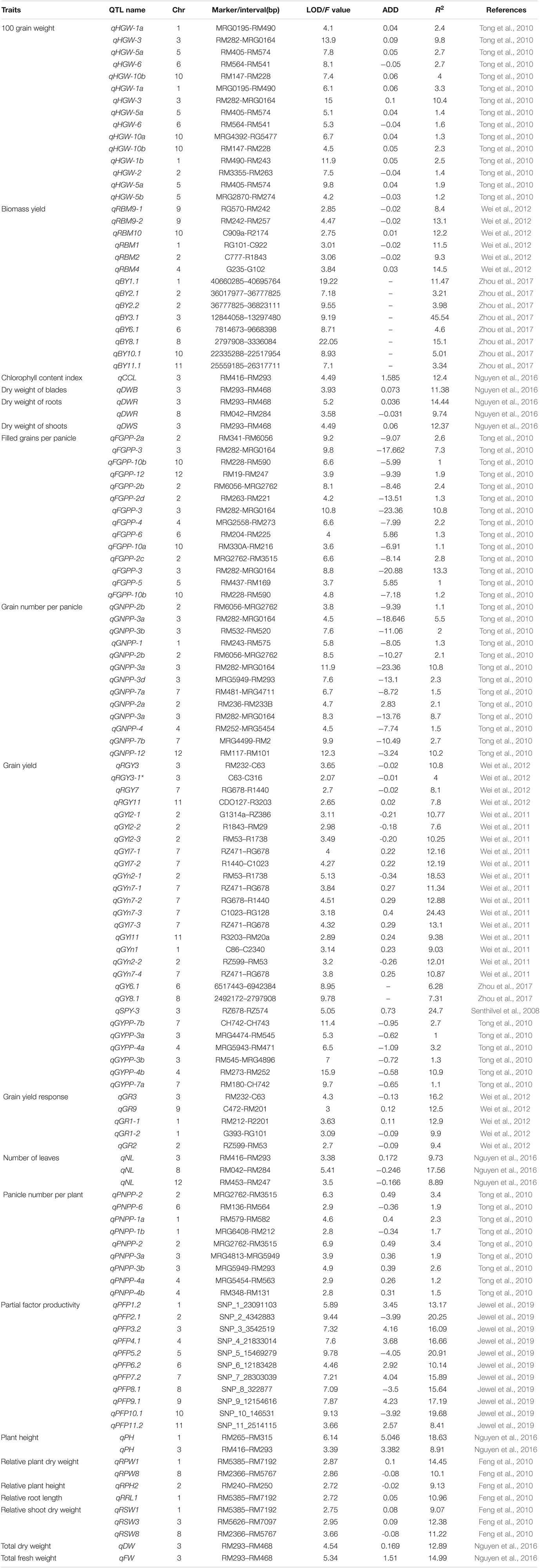
Table 1. Detailed description of QTL associated with nitrogen use efficiency-related traits in rice.

Table 3. Detailed description of QTL associated with nitrogen use efficiency-related traits in wheat.
Significant variability and marker-trait associations in genome-wide association studies for nitrogen uptake and use efficiency have been reported (Barraclough et al., 2010; Liu Z. et al., 2016; Monostori et al., 2017). Genome-wide association studies were conducted exploiting the phenotypic variability of the nested synthetic wheat introgression libraries developed at Punjab Agricultural University, Ludhiana (India). Several marker-trait associations associated with root and plant morphological traits, grain yield, and yield-related traits were identified (data unpublished). QTL associated with root-traits and nutrient-uptake (Sandhu et al., 2015, Sandhu et al., 2016) in rice have been reported. Several genetic regions associated with nutrient uptake have been detected in rice (Wissuwa et al., 1998; Ming et al., 2000), wheat (Su et al., 2006, 2009), maize (Zhu et al., 2005), common bean (Liao et al., 2004; Yan et al., 2004), and soybean (Li et al., 2005; Liang et al., 2010). Comparative mapping involving other cereal crops such as rice aims to identify highly conserved sequences, new genes, and regulatory elements to link genomes, genes, proteins, and traits controlling traits of interest across different species and genera. These inter-genome relational patterns can lead to new hypotheses, knowledge, and predictions about the related species.
Genes Related to NUE
Multiple sets of genes in crop plants are known to regulate the mechanisms associated with NUE such as nitrogen absorption, accumulation, and remobilization. Genes regulating NUE among different cereal crops such as wheat and rice are broadly divided into six categories including, transporters, signal molecules, amino acid biosynthesis, nitrate assimilation, transcription factors, and other genes (Figure 6). The detailed description of network genes associated with nitrogen use efficiency in rice crops is presented in Table 5 and in wheat in Table 6. Among these categories, transporters and nitrate assimilation genes are particularly involved in nitrogen uptake, and amino acid biosynthesis genes are involved in nitrogen utilization, whereas signaling molecules, transcription factors, and other genes have a passive role in both nitrogen uptake and nitrogen utilization (Zhou et al., 2009).
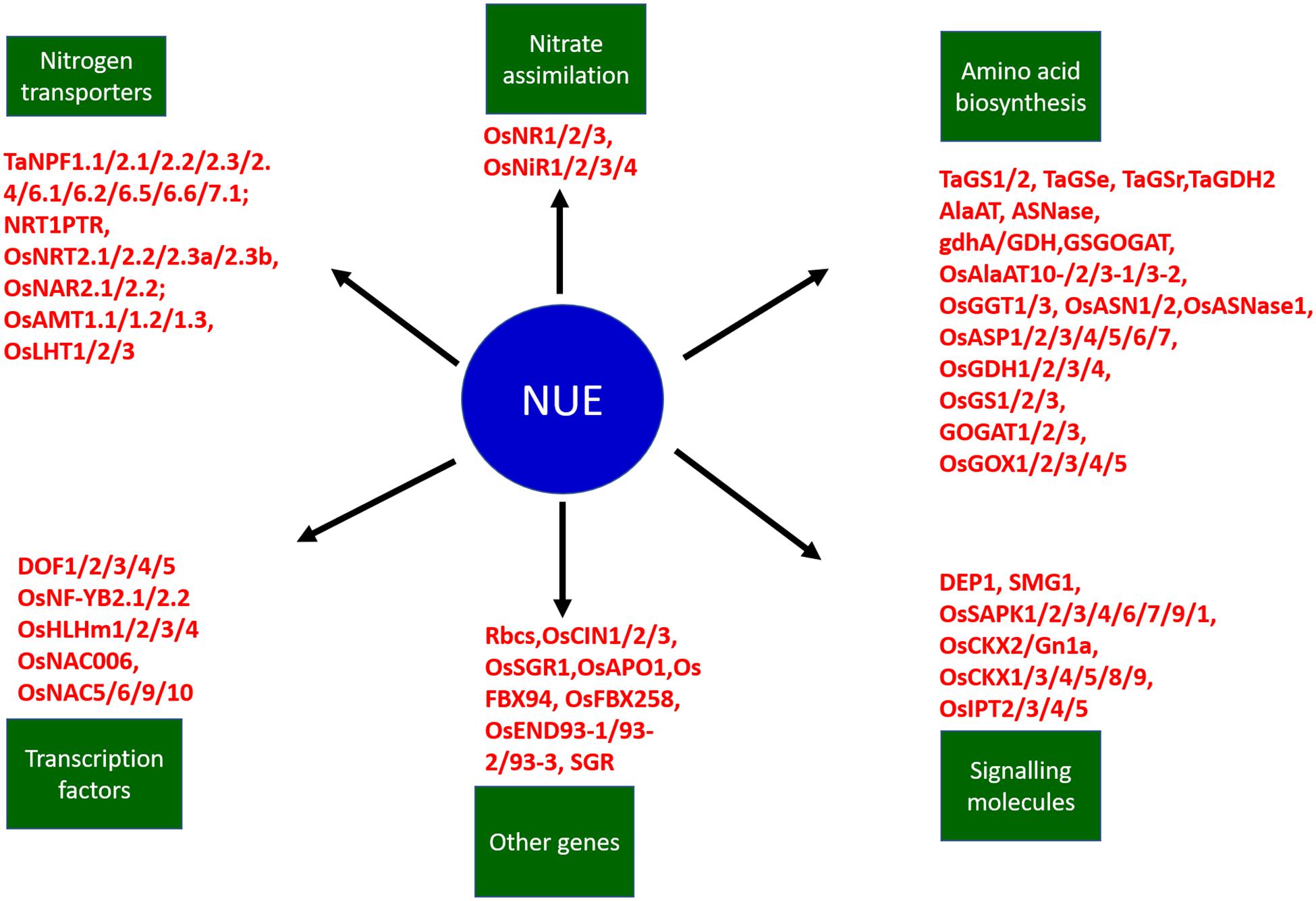
Figure 6. The schematic representation of the network of nitrogen transporters, genes, transcription factors, and signaling molecules involved in nitrogen use efficiency in cereal crops such as wheat and rice. NUE improvement involves multiple interconnected factors such as transporters, signal molecules, amino acid biosynthesis, nitrate assimilation, transcription factors, and other genes. The transporters and nitrate assimilation genes are particularly involved in nitrogen uptake, amino acid biosynthesis genes are involved in nitrogen utilization, and the signaling molecules, transcription factors, and other genes have passive roles in both nitrogen uptake and nitrogen utilization. Ta represents Triticum aestivum and Os represents Oryza sativa.

Table 5. Detailed description of network genes associated with nitrogen use efficiency in rice crop.
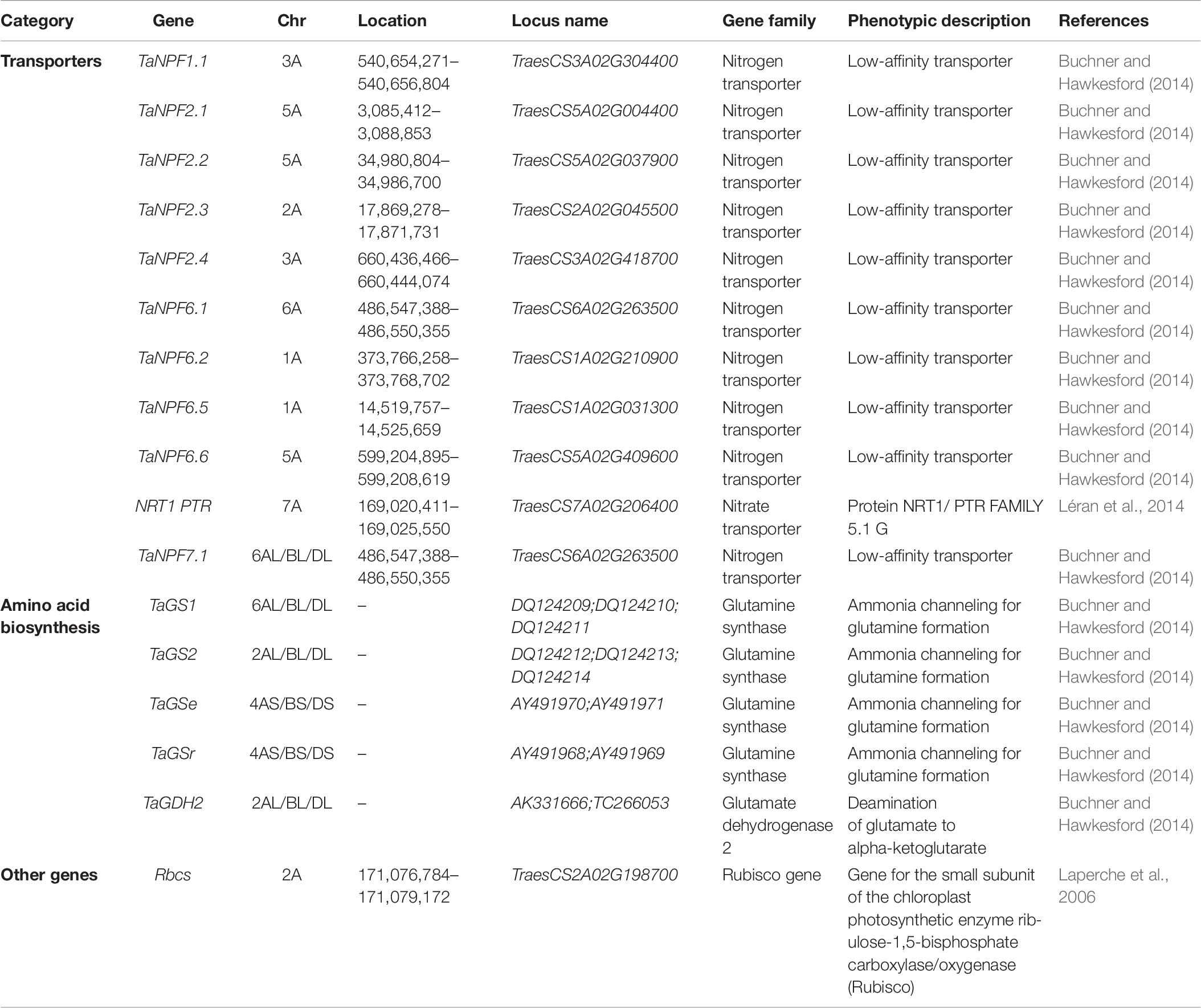
Table 6. Detailed description of network genes associated with nitrogen use efficiency in wheat crop.
Nitrate is the most common form of nitrogen present in soil which is transported in plants actively with the help of nitrate transporters. These nitrate transporters are encoded by NRT families. Firstly, these families were reported in Arabidopsis and were categorized into three subfamilies, i.e., NRT1 family, whose members are low-affinity transporters, and the NRT2/NRT family which primarily encodes high-affinity transporters (Plett et al., 2010). This information was used to find the orthologs of NRT transporter genes in cereal crops by using the reciprocal best hit (RBH) approach. It was observed that within cereals there is variability in gene number and family structure (Plett et al., 2010). Cereals express an additional NRT1.1 ortholog and devoid of NRT1.6/NRT1.7 in comparison to Arabidopsis. NRT2 family needs special focus in cereals for its functional analysis as this gene in grasses shows significant genetic distance. In rice, there are a total of four high-affinity NRT2 transporters (Table 5; Li et al., 2016). Among these four, two (OsNRT2.1 and Os-NRT2.2) genes have high similarities to NRTs in monocots, while OsNRT2.3 and OsNRT2.4 are more closely related to the Arabidopsis NRT2 (Cai et al., 2008). Rather than the above-described gene variants, OsNRT2.3 has further two subtypes, i.e., OsNRT2.3a and OsNRT2.3b. The overexpression of OsNRT2.3b is known to have a significant role in high grain yield and NUE in rice (Fan et al., 2016). It is established that in common wheat approximately 16 low-affinity nitrate transporter NPFs are expressed which are homologous to Arabidopsis NPFs (Buchner and Hawkesford, 2014). The expression of a particular transporter in wheat depends upon the nitrogen status of the plant and soil. NPF wheat genes have been reported to be regulated by plant nitrogen status, which suggests that nitrogen metabolism is the main regulator for genes involved in nitrate transport. Nitrate transporters are the main players in nitrogen uptake in most plants as nitrate is a precursor for N present in the soil, but in certain cases, ammonia is the predominant form in the soil. As in the case of rice when grown in paddy fields, ammonium ions (NH4+) are a major source of nitrogen. In such conditions, genes for ammonium transports, i.e., high-affinity transporter systems (HATS), for ammonia are expressed in roots (Tabuchi et al., 2007).
It is reported that HATS for ammonium transport in roots belong to the ammonium transporter/Rhesus-type/methylamine permease (AMT/Rh-type/MEP) protein family. The ammonium transporter system is well established in rice. It is known that there are 10 members of the AMT family, which are broadly classified as high and low-affinity transporters. Among the ten members, three OsAMT1 family members fall under the category of high-affinity transporters, whereas three OsAMT2, three OsAMT3 members, and one OsAMT4 member are components of low-affinity transporters (Loqué and Wirén, 2004). Although all sets of genes are present in rice, their expression varies, some are constitutively expressed in the roots and shoot while some are members of induced genes which are triggered after ammonium exposure or a decrease in plant N content (Kumar et al., 2003; Sonoda et al., 2003; Suenaga et al., 2003).
Nitrogen uptake is followed by nitrogen assimilation. It is a crucial metabolic step that regulates the grain yield and ultimately NUE. The glutamine synthetase (GS)/glutamate synthase (GOGAT) cycle is majorly involved in nitrate assimilation in the form of amino acids. The overexpression of genes encoding enzymes involved in the GS/GOGAT cycle is directly correlated with enhanced growth rate, biomass, and yield in rice. Especially overexpression of OsGS1 is reported to have a positive effect on grain yield under the influence of nitrate assimilation (Brauer et al., 2011). Three different forms of GS are reported in rice. Wheat is reported to have increased activity of GS1 especially in leaves which ultimately leads to accumulation of nitrogen in grains and also enhanced grain dry matter. Rice is known to have a small family of GS and GOGAT enzymes present in different cellular locations. Among variable isoforms of GS and GOGAT the cytosolic GS1;2 and the plastidic NADH-GOGAT1 are involved in ammonium ion assimilation in roots. It is reported that in conditions with high N content, overexpression of the GS1 gene enhances the nitrogen harvest index and NUE but no change in NUE was observed in a nitrogen-deficient environment.
Among cereals, maize has a C4 system so it has an enhanced capacity to assimilate and metabolize carbon and nitrogen. Expression of NAD-malic enzymes in C4 plants is responsible for enhanced nitrogen assimilation as compared to C3 plants. It is established from knock-out studies that overexpression of genes for two isoforms of enzymes, i.e., Gln1-3 and Gln1-4 genes in the maize leads to an increase in kernel number (Martin et al., 2006; Sun et al., 2018). Therefore, the gene for nitrogen assimilation plays a major role in kernel yield. Similar studies were conducted in barley where an extra copy of the HvGS1-1 gene was expressed which was reported to enhance the GS1 enzyme activity and such lines displayed high NUE and grain yield as compared to wild-type plants (Gao et al., 2018).
The last step in nitrogen use efficiency is the remobilization of nitrogen toward seeds during maturity. Monocots, dicots, C3, and C4 plants share a common mechanism for nitrogen remobilization (Masclaux-Daubresse et al., 2010). Among different amino acids, asparagine and glutamine are the common transport form and its concentration increase in phloem sap during senescence of leaves for nitrogen remobilization to reproductive tissue. In durum wheat asparagine synthetase encoding genes (AsnS1) are crucial for nitrogen remobilization from flag leaf to developing grains (Curci et al., 2018). Similarly, in rice, the growing panicle derives approximately 80% of the nitrogen from the senescing organs and reaches reproductive organs through the phloem. Nitrogen is majorly transported in the phloem sap in the form of glutamine. Two enzymes GS and GOGAT are essential for nitrogen remobilization and reutilization in senescing and developing organs, respectively (Tabuchi et al., 2007). In rice, it is observed that GS1-1 is crucial for the remobilization process, whereas NADH-GOGAT1 is involved in the reutilization of transported glutamine in growing tissues (Hayakawa et al., 1994; Tabuchi et al., 2007). In maize, wheat, and barley, the grain nitrogen content is correlated with flag leaf senescence, which seems to play an important role in nitrogen availability for grain filling (Martin et al., 2006; Uauy et al., 2006). High yield is reported to be affected by leaf senescence, as delayed leaf senescence is responsible for prolonged photosynthesis, which improved the grain yield. However, the delayed leaf senescence was reported to decrease nitrogen remobilization efficiency and grain protein content (Masclaux-Daubresse et al., 2010).
Transcription Factors Involved in NUE
The major switches in the plant regulatory networks are transcription factors and like several metabolic processes NUE is crucially dependent on coordinated transcription factors (Figure 6 and Tables 5, 6; Spitz and Furlong, 2012). Transcription factors involved in lateral root growth in response to nitrate belong to MADS-box and ANR1 is a member of the transcription factors reported in Arabidopsis (Zhang, 1998). These transcription factors initiate the signaling pathway of NRT1.1 (Remans et al., 2006). Another family of transcription factors involved in nitrogen metabolism is the NLP (NIN-like protein) family of transcription factors (Konishi and Yanagisawa, 2013; Marchive et al., 2013). These transcription factors are reported to interact with NLP genes, including TCP20 (teosinte branched1/cycloidea/proliferating cell factor1-20) (Guan et al., 2017). This interaction is important for lateral root growth in response to nitrate (Guan et al., 2014; Xu et al., 2016). BT1 and BT2 (bric-abrac/tramtrack/broad) form the third major family of transcription factors which act on multiple genes to form a network for nitrate assimilation. System biology approaches were used to discover the web of transcription factors involved in NUE. Functional analysis indicated that the transcription factor which actively regulates NUE in Arabidopsis have orthologs in cereals especially rice (Araus et al., 2016). The transcription factors belonging to the DOF (DNA-binding with one finger) and bHLH (helix loop helix) families are actively involved in NUE is rice (Table 5). These transcription factors are reported to be involved in various biological processes, such as tissue differentiation and hormone signaling (Noguero et al., 2013). A report suggests that enhanced expression of the DOF1 gene in rice increases N assimilation and plant growth under low-N conditions (Kurai et al., 2011). DOF family transcription factors are reported to control ammonium uptake by inducing genes of the ammonium transporter family in roots of rice (Wu et al., 2017). Alongside this, in wheat the DOF1.3 gene was overexpressed under stress conditions such as nitrogen starvation (Curci et al., 2017). A total of 170 unique genes encoding transcription factors belonging to the different families, including bHLH, MYB, bZIP, C2C2-Dof, TERF, WRKY, NF-Y, NAC, AUX/IAA, and the auxin-modulated ARF, etc., displayed differential expression between nitrogen-stressed and control durum wheat tissues.
miRNA Involved in Different Aspects of NUE
miRNAs are reported to play important roles in NUE along with several transcription factors. The miRNA169 family is reported to regulate the expression of genes for nitrogen transport under low nitrogen conditions. This family of miRNA is broadly studied among cereals, as it is reported in maize, miR169 expression decreases in N-deficient plants (Zhao et al., 2012). Furthermore, several new miR169 family members are reported to express in durum wheat which responds to nitrogen-deficient conditions (Zuluaga et al., 2017, 2018). The conserved ttu-miR169h and ttu-miR169c at the seedling and grain filling stages, respectively, and the newly identified ttu-novel-61 belonging to the miR169 family, were downregulated in both stages of durum wheat plants subjected to nitrogen starvation in both the roots and leaves. These miRNAs are known to negatively regulate the CCAAT box-binding transcription factors in several tissues which influence the NUE-related genes in durum wheat plants (Zuluaga et al., 2017). Through miRNA studies, several transcription factors and genes are revealed to have an important role in enhancing NUE, for example, degradome libraries and sequencing of miRNAs among maize seedlings revealed that there are 99 loci categorized into 47 miRNA families, 9 of which are paralog to miR169, miR171, and miR398 (Zhao et al., 2012). Besides, eight miRNA families showed differential expression under nitrogen-deficient conditions and the target analysis proposed a role of newly identified miRNA target genes in a wide range of metabolic processes and cellular responses (Zhao et al., 2012). Recently, a study involving degradome sequencing and small RNA together with target gene validation showed that two new putative miR169 species (miRC10 and miRC68) may play a key role in low nitrogen adaptation of maize seedlings (Zhao et al., 2013b). mir164a and mir164b are reported to have a specific role in nitrogen remobilization. The miR164 family is reported to influence NAC transcription factors and several studies were conducted to define the relation between miR164 and NAC regulation among cereals. The NAM-B1 gene in bread wheat was reported as a NAC transcription factor affecting the grain nutrient concentration (Waters et al., 2009) in addition to increasing the remobilization of nutrients from leaves to developing grains in wild wheat. Further, zma-miR164 in maize was downregulated in leaves after severe nitrogen stress treatment (Xu et al., 2011). The regulation of NAC genes by miR164 in cereals may maintain the nitrogen remobilization from leaves to seeds under low nitrogen conditions.
The expression of variable miRNAs in rice among low nitrogen tolerant and sensitive genotypes through a microarray showed differential expression of a total of 32 miRNAs between two genotypes including miR164 and another 7 miRNAs. Six miRNAs, viz., miR156, miR164, miR820, miR528, miR821, and miR1318 and four miRNAs, viz., miR164, miR528, miR167, and miR168 showed differential expression in leaves and roots, respectively (Nischal et al., 2012). The identified miRNAs were predicted to control genes encoding for the proteins and the transcription factors associated with stress responses or metabolic processes. Many miRNAs are reported to be involved in stress response in plants. Although they do not have a direct role in NUE, their involvement in stress response makes them important while considering several factors affecting NUE. In the roots of maize, under low NO3– conditions miR528a,b, and miR528a∗,b∗ were repressed suggesting their role in integrating NO3– signals into root developmental changes (Trevisan et al., 2012). Moreover, Zma-miR528a,b family members showed downregulation in maize roots and leaves of seedlings exposed to nitrogen deficiency (Zhao et al., 2012). It was reported that increased expression of rice miR528 was subsequently associated with an increase in total nitrogen accumulation, plant biomass, and chlorophyll synthesis (Yuan et al., 2015). miR528 in rice is known to be involved in enhancing N-mediated tillering by inhibiting auxin signaling in axillary buds. Along with it, Osa-miR393 is another class of miRNA expressed in rice acting as a regulator of OsTB1 and OsAFB2 genes (Li et al., 2016). Under nitrogen deficit conditions, TaMIR1129, TaMIR1118, and TaMIR1136 were reported to be upregulated, whereas TaMIR1133 was downregulated in roots in wheat. The expression of some of these miRNAs was inversely correlated with the concentration and duration of nitrogen application (Zhao et al., 2013a). TaMIR2275, another common wheat miRNA, showed gradual upregulation during nitrogen starvation, while the expression of miRNA was progressively restored upon nitrogen recovery treatment. Overexpression of TaMIR2275 produced plants with increased nitrogen accumulation and biomass, while the reverse was observed in the knockdown mutants. Consequently, several classes of miRNAs are involved in nitrogen metabolism by affecting multiple processes associated directly or indirectly with NUE. Overall, it is essential to understand the precise network of miRNA expression and interaction to completely channelize the mechanism underlying NUE.
The identification of suitable traits, QTL, and candidate genes underlying QTL may provide new opportunities for the introgression of these QTL and genes into elite genetic backgrounds contributing to the development of nutrient efficient varieties (Figure 7).
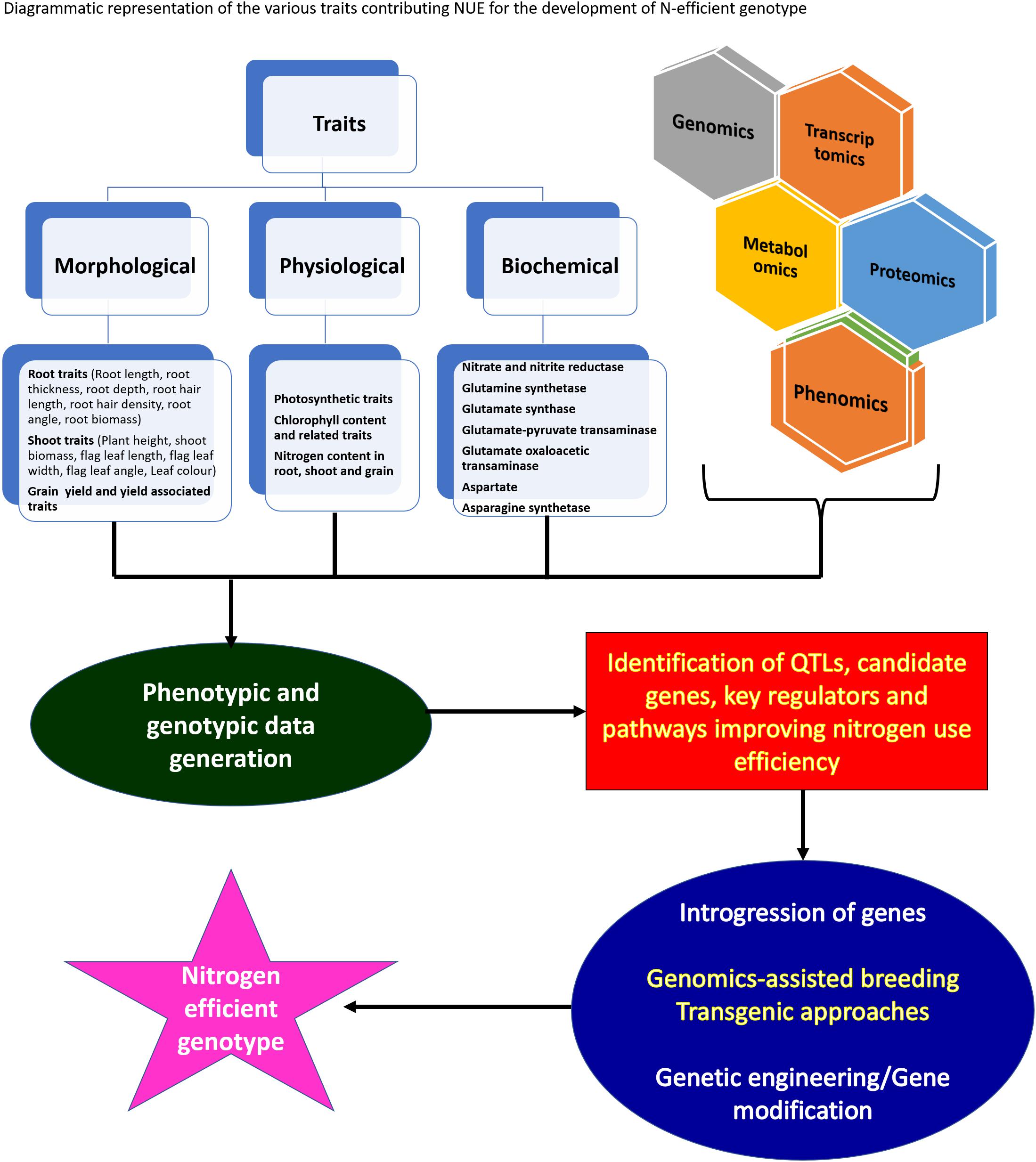
Figure 7. Schematic representation of the various traits, QTL, and candidate genes contributing to the development of nutrient efficient genotypes. The pipeline for identification and deployment of traits/QTL/gene/regulatory factors associated with nitrogen use efficiency. The identified traits and donors can be used to develop suitable mapping populations to be further used in mapping of genomic regions and identification of candidate genes or key regulators associated with trait of interest using recent biotechnological approaches such as phenomics, genomics, transcriptomics, metabolomics, and proteomics. The deployment of identified QTL/gene/regulatory factors in genomics-assisted breeding programs and their functional characterization employing transgenic and genetic engineering approaches.
Conclusion
Excessive use of nitrogen fertilizers to boost the grain yields of cereal crops is a major cause of water, soil, and air pollution as well as greenhouse gas emissions. It has an economic impact globally due to the high production costs of nitrogen fertilizer. The challenge in improving NUE in cereal crops is achieving both high yield and high nitrogen use efficiency (NUE) simultaneously. Therefore, improving nitrogen use efficiency is very important for environmentally friendly and profitable crop production. The ultimate goal of improving our understanding of agronomic management, suitable traits, QTL, genes, and the mechanisms and functions of genes associated with nitrogen use efficiency is to enhance crop production and productivity. The careful selection of diverse genotypes, exploitation of natural variation, exploring root architecture, high-throughput precise phenotyping, standardized field trials, new techniques for efficient fertilizer application, appropriate field management practices, and identification of new QTL/genes/nitrogen transporters and signaling molecules could be helpful to reduce fertilizer consumption in the near future. The challenge here is to identify consistent genomic regions and molecular regulators interacting at several nodes in the gene network to act as the key component in nitrogen metabolism. The improvement in basic research in combination with agronomical, marker-aided molecular breeding and biotechnological strategies will help to achieve higher nitrogen use efficiency in cereal crops. The compiled information on QTL in the present review can be used further in metaQTL analysis to study the congruency of the identified regions associated with particular traits of interest.
Author Contributions
NS: conceptualization, funding acquisition, resources, and supervision. NS, MS, AK, DD, and JS: literature search. NS and MS: writing–original draft. NS and PC: critical review and editing. All authors contributed to manuscript revision, read, and approved the final version of the manuscript.
Funding
The work was compiled under projects funded by the Department of Biotechnology, Government of India (Grant No. BT/IN/UK-VNC/42/RG/2015-16 and BT/PR30871/BIC/101/1159/2018).
Conflict of Interest
The authors declare that the research was conducted in the absence of any commercial or financial relationships that could be construed as a potential conflict of interest.
Acknowledgments
We are thankful to the Department of Biotechnology, Government of India for providing grants.
References
Abiko, T., Wakayama, M., Kawakami, A., Obara, M., Kisaka, H., Miwa, T., et al. (2010). Changes in nitrogen assimilation, metabolism, and growth in transgenic rice plants expressing a fungal NADP (H)-dependent glutamate dehydrogenase (gdhA). Planta 232, 299–311.
Acreche, M. M., and Slafer, G. A. (2009). Variation of grain nitrogen content in relation with grain yield in old and modern Spanish wheats grown under a wide range of agronomic conditions in a Mediterranean region. J. Agr. Sci. 147, 657–667. doi: 10.1017/s0021859609990190
Agrama, H., Zakaria, A., Said, F., and Tuinstra, M. (1999). Identification of quantitative trait loci for nitrogen use efficiency in maize. Mol. Breed. 5, 187–195. doi: 10.1023/a:1009669507144
Ali, A. (2020). Nitrate assimilation pathway in higher plants: critical role in nitrogen signalling and utilization. Plant Sci. Today 7, 182–192.
Almario, J., Moënne-Loccoz, Y., and Muller, D. (2013). Monitoring of the relation between 2,4-diacetylphloroglucinol-producing pseudomonas and Thielaviopsis basicola populations by real-time PCR in tobacco Black root-rot suppressive and conducive soils. Soil Bio. Biochem. 57, 144–155. doi: 10.1016/j.soilbio.2012.09.003
An, D., Su, J., Liu, Q., Zhu, Y., Tong, Y., Li, J., et al. (2006). Mapping QTLs for nitrogen uptake in relation to the early growth of wheat (Triticum aestivum L.). Plant Soil 284, 73–84. doi: 10.1007/s11104-006-0030-3
Andrews, M., Raven, J., and Lea, P. (2013). Do plants need nitrate? The mechanisms by which nitrogen form affects plants. Ann. Appl. Biol. 163, 174–199. doi: 10.1111/aab.12045
Araus, J. L., Amaro, T., Zuhair, Y., and Nachit, M. M. (1997). Effect of leaf structure and water status on carbon isotope discrimination in field-grown durum wheat. Plant Cell Environ. 20, 1484–1494. doi: 10.1046/j.1365-3040.1997.d01-43.x
Araus, V., Vidal, E. A., Puelma, T., Alamos, S., Mieulet, D., Guiderdoni, E., et al. (2016). Members of BTB gene family regulate negatively nitrate uptake and nitrogen use efficiency in Arabidopsis thaliana and Oryza sativa. Plant Physiol. 171:01731.2015. doi: 10.1104/pp.15.01731
Atkinson, J. A., Wingen, L. U., Griffiths, M., Pound, M. P., Gaju, O., Foulkes, M. J., et al. (2015). Phenotyping pipeline reveals major seedling root growth QTL in hexaploid wheat. J. Exp. Bot. 66, 2283–2292. doi: 10.1093/jxb/erv006
Bai, C., Liang, Y., and Hawkesford, M. J. (2013). Identification of QTLs associated with seedling root traits and their correlation with plant height in wheat. J Exp. Bot. 64, 1745–1753. doi: 10.1093/jxb/ert041
Bardon, C., Piola, F., Bellvert, F., Haichar, F. E., Comte, G., Meiffren, G., et al. (2014). Evidence for biological denitrification inhibition (BDI) by plant secondary metabolites. New Phytol. 204, 620–630. doi: 10.1111/nph.12944
Barraclough, P. B., Howarth, J. R., Jones, J., Lopez-Bellido, R., Parmar, S., Shepherd, C. E., et al. (2010). Nitrogen efficiency of wheat: genotypic and environmental variation and prospects for improvement. Eur. J. Agro. 33, 1–11.
Barraclough, P. B., Lopez-Bellido, R., and Hawkesford, M. J. (2014). Genotypic variation in the uptake, partitioning and remobilisation of nitrogen during grain-filling in wheat. Field Crops Res. 156, 242–248. doi: 10.1016/j.fcr.2013.10.004
Beatty, P. H., Anbessa, Y., Juskiw, P., Carroll, R. T., Wang, J., and Good, A. G. (2010). Nitrogen use efficiencies of spring Barley grown under varying nitrogen conditions in the field and growth chamber. Ann. Bot. 105, 1171–1182. doi: 10.1093/aob/mcq025
Behl, R. K., Ruppel, S., Kothe, E., and Narula, N. (2012). Wheat x Azotobacter x VA Mycorrhiza interactions towards plant nutrition and growth–a review. J. Appl. Bot. Food Qual. 81, 95–109.
Bertheloot, J., Martre, P., and Andrieu, B. (2008). Dynamics of light and nitrogen distribution during grain filling within wheat canopy. Plant Physiol. 148, 1707–1720. doi: 10.1104/pp.108.124156
Bhaskar, R., and Syvertsen, P. J. (2005). Concurrent changes in net CO2 assimilation and chloroplast structure in nitrogen deficient citrus leaves. Env. Exp. Bot. 54, 41–48.
Bogard, M., Allard, V., Brancourt-Hulmel, M., Heumez, E., Machet, J., Jeuffroy, M., et al. (2010). Deviation from the grain protein concentration–grain yield negative relationship is highly correlated to post-anthesis N uptake in winter wheat. J. Exp. Bot. 61, 4303–4312. doi: 10.1093/jxb/erq238
Bogard, M., Jourdan, M., Allard, V., Martre, P., Perretant, M. R., Ravel, C., et al. (2011). Anthesis date mainly explained correlations between post-anthesis leaf senescence, grain yield, and grain protein concentration in a winter wheat population segregating for flowering time QTLs. J. Exp. Bot. 62, 3621–3636. doi: 10.1093/jxb/err061
Boisson, M., Mondon, K., Torney, V., Nicot, N., Laine, A., Bahrman, N., et al. (2005). Partial sequences of nitrogen metabolism genes in hexaploid wheat. Theo. App. Gene 110, 932–940. doi: 10.1007/s00122-004-1913-4
Bottini, R., Cassian, F., and Piccoli, P. (2004). Gibberellin production by bacteria and its involvement in plant growth promotion and yield increase. App. Microbio. Biotech. 65, 497–503. doi: 10.1007/s00253-004-1696-1
Boyd, E. S., Costas, A. M. G., Hamilton, T. L., Mus, F., and Peters, J. W. (2015). Evolution of molybdenum nitrogenase during the transition from anaerobic to aerobic metabolism. J. Bacteriol. 197, 1690–1699.
Brasier, K., Ward, B., Smith, J., Seago, J., Oakes, J., Balota, M., et al. (2020). Identification of quantitative trait loci associated with nitrogen use efficiency in winter wheat. PLoS One 15:e0228775.
Brauer, E. K., Rochon, A., Bi, Y. M., Bozzo, G. G., Rothstein, S. J., and Shelp, B. J. (2011). Reappraisal of nitrogen use efficiency in rice overexpressing glutamine synthetase1. Physiol. Plant. 141, 361–372.
Buchner, P., and Hawkesford, M. J. (2014). Complex phylogeny and gene expression patterns of members of the nitrate transporter 1/Peptide transporter family (NPF) in wheat. J. Exp. Bot. 65, 5697–5710. doi: 10.1093/jxb/eru231
Cai, C., Wang, J., Zhu, Y., Shen, Q., Li, B., Tong, Y., et al. (2008). Gene structure and expression of the high-affinity nitrate transport system in rice roots. J. Int. Plant Bio. 50, 443–451. doi: 10.1111/j.1744-7909.2008.00642.x
Cai, C., Zhao, X., Zhu, Y., Li, B., Tong, Y., and Li, Z. (2007). Regulation of the high-affinity nitrate transport system in wheat roots by exogenous Abscisic acid and glutamine. J. Int. Plant Bio. 49, 1719–1725. doi: 10.1111/j.1744-7909.2007.00485.x
Cameron, K., Di, H., and Moir, J. (2013). Nitrogen losses from the soil/plant system: a review. Ann. App. Bio. 162, 145–173. doi: 10.1111/aab.12014
Campbell, W. H. (2002). “Molecular control of nitrate reductase and other enzymes involved in nitrate assimilation,” in Photosynthetic Nitrogen Assimilation and Associated Carbon and Respiratory Metabolism, eds C. H. Foyer and G. Noctor (Dordecht: Kluwer Academic Publishers), 35–48. doi: 10.1007/0-306-48138-3_3
Carvalho, P., and Foulkes, M. J. (2011). “Chapter 195: roots and the uptake of water and nutrients,” in Encyclopedia of Sustainability Science and Technology, ed. R. A. Meyers (Heidelberg: Springer), 1390–1404.
Cassan, F., Perrig, D., Sgroy, V., Masciarelli, O., Penna, C., and Luna, V. (2009). Azospirillum brasilense Az39 and Bradyrhizobium japonicum E109, inoculated singly or in combination, promote seed germination and early seedling growth in corn (Zea mays L.) and soybean (Glycine Max L.). Eur. J. Soil Bio. 45, 28–35. doi: 10.1016/j.ejsobi.2008.08.005
Cassman, K. G., Dobermann, A., and Walters, D. T. (2002). Agroecosystems, nitrogen-use efficiency, and nitrogen management. AMBIO Am. J. Hum. Environ. 31, 132–140.
Cho, Y., Kang, H., Lee, J., Lee, Y., Lim, S., Gauch, H., et al. (2007). Identification of quantitative trait loci in rice for yield, yield components, and agronomic traits across years and locations. Crop Sci. 47, 2403–2417. doi: 10.2135/cropsci2006.08.0509
Cho, Y. I., Jiang, W. Z., Chin, J. H., Piao, Z. Z., Cho, Y. G., McCouch, S. R., et al. (2007). Identification of QTLs associated with physiological nitrogen use efficiency in rice. Mol. Cells 23, 72–79.
Christiansen, M. W., and Gregersen, P. L. (2014). Members of the Barley NAC transcription factor gene family show differential Co-regulation with senescence-associated genes during senescence of flag leaves. J. Exp. Bot. 65, 4009–4022. doi: 10.1093/jxb/eru046
Cormier, F., Foulkes, J., Hirel, B., Gouache, D., Moenne-Loccoz, Y., and Le Gouis, J. (2016). Breeding for increased nitrogen-use efficiency: a review for wheat (T. aestivum L.). Plant Breed. 135, 255–278. doi: 10.1111/pbr.12371
Couillerot, O., Combes-Meynet, E., Pothier, J. F., Bellvert, F., Challita, E., Poirier, M., et al. (2011). The role of the antimicrobial compound 2,4-diacetylphloroglucinol in the impact of biocontrol Pseudomonas fluorescens F113 on Azospirillum brasilense phytostimulators. Microbio 157, 1694–1705. doi: 10.1099/mic.0.043943-0
Cowling, S. A., and Field, C. B. (2003). Environmental control of leaf area production: Implications for vegetation and land-surface modelling. Glob. Biogeo. Cycles 17, 7–14. doi: 10.1029/2002gb001915
Craswell, E. T., and Godwin, D. C. (1984). “The efficiency of nitrogen fertilizers applied to cereals grown in different climates,” in Advances in Plant Nutrition, Vol. 1, eds P. B. Tinker and A. Lauchli (Westport, CT: Praeger Publishers), 1–55.
Crawford, N. M., and Glass, A. D. (1998). Molecular and physiological aspects of nitrate uptake in plants. Tre. Plant Sci. 3, 389–395. doi: 10.1016/s1360-1385(98)01311-9
Criddle, R. S., Ward, M. R., and Huffaker, R. C. (1988). Nitrogen uptake by wheat seedlings, interactive effects of four nitrogen sources: NO3-, NO2-, NH4+, and urea. Plant Physiol. 86, 166–175. doi: 10.1104/pp.86.1.166
Curci, P. L., Aiese Cigliano, R., Zuluaga, D. L., Janni, M., Sanseverino, W., and Sonnante, G. (2017). Transcriptomic response of durum wheat to nitrogen starvation. Sci. Rep. 7:1176. doi: 10.1038/s41598-017-01377-0
Curci, P. L., Bergès, H., Marande, W., Maccaferri, M., Tuberosa, R., and Sonnante, G. (2018). Asparagine synthetase genes (AsnS1 and AsnS2) in durum wheat: Structural analysis and expression under nitrogen stress. Euphytica 214:36. doi: 10.1007/s10681-017-2105-z
Dai, G., Cheng, S., Hua, Z., Zhang, M., Jiang, H., Feng, Y., et al. (2015). Mapping quantitative trait loci for nitrogen uptake and utilization efficiency in rice (Oryza sativa L.) at different nitrogen fertilizer levels. Genet. Mol. Res. 14, 10404–10414. doi: 10.4238/2015.september.8.1
Dechorgnat, J., Nguyen, C. T., Armengaud, P., Jossier, M., Diatloff, E., Filleur, S., et al. (2010). From the soil to the seeds: the long journey of nitrate in plants. J. Exp. Bot. 62, 1349–1359. doi: 10.1093/jxb/erq409
Deng, Z., Cui, Y., Han, Q., Fang, W., Li, J., and Tian, J. (2017). Discovery of consistent QTLs of wheat spike-related traits under nitrogen treatment at different development stages. Front. Plant Sci. 8:2120. doi: 10.3389/fpls.2017.02120
Derkx, A. P., Orford, S., Griffiths, S., Foulkes, M. J., and Hawkesford, M. J. (2012). Identification of differentially Senescing mutants of wheat and impacts on yield, biomass and nitrogen Partitioning F. J. Int. Plant Biol. 54, 555–566. doi: 10.1111/j.1744-7909.2012.01144.x
Distelfeld, A., Avni, R., and Fischer, A. M. (2014). Senescence, nutrient remobilization, and yield in wheat and Barley. J. Exp. Bot. 65, 3783–3798. doi: 10.1093/jxb/ert477
Dobermann, A., and Cassman, K. G. (2002). “Plant nutrient management for enhanced productivity in intensive grain production systems of the United States and Asia,” in Progress in Plant Nutrition: Plenary Lectures of the XIV International Plant Nutrition Colloquium, Netherlands, 153–175. doi: 10.1007/978-94-017-2789-1_12
Duan, P., Rao, Y., Zeng, D., Yang, Y., Xu, R., Zhang, B., et al. (2014). SMALL GRAIN 1, which encodes a mitogen−activated protein kinase kinase 4, influences grain size in rice. Plant J. 77, 547–557.
Dupont, F. M., and Altenbach, S. B. (2003). Molecular and biochemical impacts of environmental factors on wheat grain development and protein synthesis. J. Cereal Sci. 38, 133–146.
Ehdaie, B., Whitkus, R. W., and Waines, J. G. (2003). Root biomass, water-use efficiency, and performance of wheat–rye translocations of chromosomes 1 and 2 in spring bread wheat ‘Pavon’. Crop Sci. 43:710. doi: 10.2135/cropsci2003.0710
Etienne, P., Diquelou, S., Prudent, M., Salon, C., Maillard, A., and Ourry, A. (2018). Macro and micronutrient storage in plants and their remobilization when facing scarcity: the case of drought. Agri. 8:14.
Evans, J. R. (1983). Nitrogen and photosynthesis in the flag leaf of wheat (Triticum aestivum L.). Plant Physiol. 72, 297–302. doi: 10.1104/pp.72.2.297
Fan, X., Naz, M., Fan, X., Xuan, W., Millerl, A. J., and Xu, G. (2017). Plant nitrate transporters: from gene function to application. J. Exp. Bot. 68, 2463–2475.
Fan, X., Tang, Z., Tan, Y., Zhang, Y., Luo, B., Yang, M., et al. (2016). Overexpression of a pH-sensitive nitrate transporter in rice increases crop yields. Proc. Natl. Acad. Sci. U.S.A. 113, 7118–7123. doi: 10.1073/pnas.1525184113
Fan, X., Zhang, W., Zhang, N., Chen, M., Zheng, S., Zhao, C., et al. (2018). Identification of QTL regions for seedling root traits and their effect on nitrogen use efficiency in wheat (Triticum aestivum L.). Theo. App. Gene 131, 2677–2698. doi: 10.1007/s00122-018-3183-6
FAO (2016). World Fertilizer Trends and Outlook to 2019. Rome: Food and Agriculture Organization of the United Nations (FAO).
Feng, H., Yan, M., Fan, X., Li, B., Shen, Q., Miller, A. J., et al. (2011). Spatial expression and regulation of rice high-affinity nitrate transporters by nitrogen and carbon status. J. Exp. Bot. 62, 2319–2332. doi: 10.1093/jxb/erq403
Feng, Y., Cao, L. Y., Wu, W. M., Shen, X. H., Zhan, X. D., Zhai, R. R., et al. (2010). Mapping QTLs for nitrogen-deficiency tolerance at seedling stage in rice (Oryza sativa L.). Plant Breed. 129, 652–656. doi: 10.1111/j.1439-0523.2009.01728.x
Fontaine, J. X., Ravel, C., Pageau, K., Heumez, E., Dubois, F., Hirel, B., et al. (2009). A quantitative genetic study for elucidating the contribution of glutamine synthetase, glutamate dehydrogenase and other nitrogen-related physiological traits to the agronomic performance of common wheat. Theo. App. Gene. 119, 645–662.
Ford, K. E., Gregory, P. J., Gooding, M. J., and Pepler, S. (2006). Genotype and fungicide effects on late-season root growth of winter wheat. Plant Soil 284, 33–44. doi: 10.1007/s11104-006-0028-x
Forde, B. G. (2014). Nitrogen signalling pathways shaping root system architecture: an update. Curr. Opin. Plant Biol. 21, 30–36.
Foulkes, M., Hawkesford, M., Barraclough, P., Holdsworth, M., Kerr, S., Kightley, S., et al. (2009). Identifying traits to improve the nitrogen economy of wheat: Recent advances and future prospects. Field Crops Res. 114, 329–342. doi: 10.1016/j.fcr.2009.09.005
Franzen, D. W., Hopkins, D. H., Sweeney, M. D., Ulmer, M. K., and Halvorson, A. D. (2002). Evaluation of soil survey scale for zone development of site-specific nitrogen management. Agro. J. 94:381. doi: 10.2134/agronj2002.0381
Fu, X. (2019). Study on the Cloning of the Rice Leaf Shape Gene NL7 and its Molecular Regulation Mechanism. Master dissertation. Beijing: Chinese Academy of Agricultural Sciences.
Gaju, O., Allard, V., Martre, P., Le Gouis, J., Moreau, D., Bogard, M., et al. (2014). Nitrogen partitioning and remobilization in relation to leaf senescence, grain yield and grain nitrogen concentration in wheat cultivars. Field Crop Res. 155, 213–223. doi: 10.1016/j.fcr.2013.09.003
Gaju, O., Allard, V., Martre, P., Snape, J., Heumez, E., LeGouis, J., et al. (2011). Identification of traits to improve the nitrogen-use efficiency of wheat genotypes. Field Crop Res. 123, 139–152. doi: 10.1016/j.fcr.2011.05.010
Gallais, A., and Hirel, B. (2004). An approach to the genetics of nitrogen use efficiency in maize. J. Exp. Bot. 55, 295–306. doi: 10.1093/jxb/erh006
Gao, Y., Bang, T. C., and Schjoerring, J. K. (2018). Cisgenic overexpression of cytosolic glutamine synthetase improves nitrogen utilization efficiency in Barley and prevents grain protein decline under elevated CO 2. Plant Biotech. J. 17, 1209–1221. doi: 10.1111/pbi.13046
Garg, S. (2013). Role and hormonal regulation of nitrate reductase activity in higher plants: a review. Plant Sci. Feed. 3, 13–20.
Geddes, B. A., Ryu, M.-H., Mus, F., Costas, A. G., Peteres, J. W., Voigt, C. A., et al. (2015). Use of plant colonizing bacteria as chassis for transfer of N2-fixation to cereals. Curr. Opin. Biotechnol. 32, 216–222.
Germida, J., and Siciliano, S. (2001). Taxonomic diversity of bacteria associated with the roots of modern, recent and ancient wheat cultivars. Bio. Fert. Soils 33, 410–415. doi: 10.1007/s003740100343
Ghoneim, A. M., Gewaily, E. E., and Osman, M. M. (2018). Effects of nitrogen levels on growth, yield and nitrogen use efficiency of some newly released Egyptian rice genotypes. Open Agri. 3, 310–318. doi: 10.1515/opag-2018-0034
Giunta, F., Motzo, R., and Deidda, M. (2002). SPAD readings and associ- ated leaf traits in durum wheat, barley and triticale cultivars. Euphy 125, 197–205. doi: 10.1023/a:1015878719389
Gojon, A., Nacry, P., and Davidian, J. C. (2009). Root uptake regulation: A central process for NPS homeostasis in plants. Curr. Opin. Plant Biol. 12, 328–338.
Goyal, S. S., and Huffaker, R. C. (1986). The uptake of NO3-, NO2-, and NH4+ by intact wheat (Triticum aestivum) seedlings. Plant Physiol. 82, 1051–1056. doi: 10.1104/pp.82.4.1051
Gregory, P. J., and Brown, S. C. (1989). Root growth, water use and yield of crops in dry environments: what characteristics are desirable? Asp. App. Biol. 22, 234–243.
Guan, P., Ripoll, J., Wang, R., Vuong, L., Bailey-Steinitz, L. J., Ye, D., et al. (2017). Interacting TCP and NLP transcription factors control plant responses to nitrate availability. Proc. Natl. Acad. Sci. U.S.A. 114, 2419–2424. doi: 10.1073/pnas.1615676114
Guan, P., Wang, R., Nacry, P., Breton, G., Kay, S. A., Pruneda-Paz, J. L., et al. (2014). Nitrate foraging by Arabidopsis roots is mediated by the transcription factor TCP20 through the systemic signaling pathway. Proc. Natl. Acad. Sci. U.S.A. 111, 15267–15272. doi: 10.1073/pnas.1411375111
Guerrieri, N., and Cavaletto, M. (2018). “Cereals proteins,” in Proteins in Food Processing. A volume n Wood head Publishing Series in Food Science, Technology and Nutrition, 2nd Edn, ed. R. Y. Yada (Kidlington: Elsevier), 223–244.
Guo, T. T., Wang, D. F., Fang, J., Zhao, J. F., Yuan, S. J., Xiao, L. T., et al. (2019). Mutations in the rice OsCHR4 gene, encoding a CHD3 family chromatin remodeler, induce narrow and rolled leaves with increased cuticular wax. Int. J. Mol. Sci. 20:2567. doi: 10.3390/ijms20102567
Guo, Y., Kong, F., Xu, Y., Zhao, Y., Liang, X., Wang, Y., et al. (2011). QTL mapping for seedling traits in wheat grown under varying concentrations of N. P and K nutrients. Theo. Appl. Gene 124, 851–865. doi: 10.1007/s00122-011-1749-7
Gyaneshwar, P., James, E., Reddy, P., and Ladha, J. (2002). Herbaspirillum colonization increases growth and nitrogen accumulation in aluminium-tolerant rice varieties. New Phytol. 154, 131–145. doi: 10.1046/j.1469-8137.2002.00371.x
Habash, D. Z., Bernard, S., Schondelmaier, J., Weyen, J., and Quarrie, S. A. (2007). The genetics of nitrogen use in hexaploid wheat: N utilisation, development and yield. Theo. Appl. Genet. 114, 403–419.
Hall, A. J., and Richards, R. A. (2013). Prognosis for genetic improvement of yield potential and water-limited yield of major grain crops. Field Crop Res. 143, 18–33. doi: 10.1016/j.fcr.2012.05.014
Han, M., Wong, J., Su, T., Beatty, P. H., and Good, A. G. (2016). Identification of nitrogen use efficiency genes in barley: searching for QTLs controlling complex physiological traits. Front. Plant Sci. 7:1587.
Hansen, N. J. S., Plett, D., Berger, B., and Garnett, T. (2018). “Tackling nitrogen use E?ciency in cereal crops using high-throughput phenotyping,” in Engineering Nitrogen Utilization in Crop Plants, eds A. Shrawat, A. Zayed, and D. Lightfoot (Cham: Springer Nature), 121–139.
Hatfield, J. L., Gitelson, A. A., Schepers, J. S., and Walthall, C. L. (2008). Application of spectral remote sensing for agronomic decisions. Agro. J. 100, 117–131. doi: 10.2134/agronj2006.0370c
Havé, M., Marmagne, A., Chardon, F., and Masclaux-Daubresse, C. (2016). Nitrogen remobilisation during leaf senescence: lessons from Arabidopsis to crops. J. Exp. Bot. 68, 2513–2529.
Hawkesford, M. J. (2014). Reducing the reliance on nitrogen fertilizer for wheat production. J. Cereal Sci. 59, 276–283. doi: 10.1016/j.jcs.2013.12.001
Hayakawa, T., Nakamura, T., Hattori, F., Mae, T., Ojima, K., and Yamaya, T. (1994). Cellular localization of NADH-dependent glutamate-synthase protein in vascular bundles of unexpanded leaf blades and young grains of rice plants. Planta 193, 455–460. doi: 10.1007/bf00201826
He, X., Qu, B., Li, W., Zhao, X., Teng, W., Ma, W., et al. (2015). The nitrate-inducible NAC transcription factor TaNAC2-5A controls nitrate response and increases wheat yield. Plant Physiol. 169, 1991–2005.
Herold, M. B., Baggs, E. M., and Daniell, T. J. (2012). Fungal and bacterial denitrification are differently affected by long-term pH amendment and cultivation of arable soil. Soil Bio. Biochem. 54, 25–35. doi: 10.1016/j.soilbio.2012.04.031
Hochholdinger, F., and Tuberosa, R. (2009). Genetic and genomic dissection of maize root development and architecture. Curr. Opinion Plant Bio. 12, 172–177. doi: 10.1016/j.pbi.2008.12.002
Hofman, G., and Van Cleemput, O. (2004). Soil and Plant Nitrogen. Paris: International Fertilizer Industry Association.
Howarth, J. R., Parmar, S., Jones, J., Shepherd, C. E., Corol, D., Galster, A. M., et al. (2008). Co-ordinated expression of amino acid metabolism in response to N and S deficiency during wheat grain filling. J. Exp. Bot. 59, 3675–3689. doi: 10.1093/jxb/ern218
Hu, B., Wang, W., Ou, S., Tang, J., Li, H., Che, R., et al. (2015). Variation in NRT1.1B contributes to nitrate-use divergence between rice subspecies. Nat. Genet. 47:834. pmid:26053497.
Huang, L., and Schiefelbein, J. (2015). Conserved gene expression programs in developing roots from diverse plants. Plant Cell 27, 2119–2132.
Hunt, E., Gattolin, S., Newbury, H. J., Bale, J. S., Tseng, H. M., Barrett, D. A., et al. (2010). A mutation in amino acid permease AAP6 reduces the amino acidcontent of the Arabidopsis sieve elements but leaves aphid herbivoresunaffected. J. Exp. Bot. 61, 55–64.
Hurek, T., Handley, L., Reinhold-Hurek, B., and Piche, Y. (2002). Azoarcus grass endophytes contribute fixed nitrogen to the plant in an unculturable state. Mol. Plant Microbe Int. 15, 233–242.
Imran, M., Sun, X., Hussain, S., Ali, U., Rana, M. S., Rasul, F., et al. (2019). Molybdenum-induced effects on nitrogen metabolism enzymes and elemental profile of winter wheat (Triticum aestivum L.) under different nitrogen sources. Int. J. Mol. Sci. 20:3.
Iniguez, A., Dong, Y., and Triplett, E. (2004). Nitrogen fixation in wheat provided by Klebsiella pneumoniae 342. Mol. Plant Microbe Interact. 17, 1078–1085. doi: 10.1094/MPMI.2004.17.10.1078
Inman, D., Khosla, R., Reich, R. M., and Westfall, D. G. (2007). Active remote sensing and grain yield in irrigated maize. Prec. Agri. 8, 241–252. doi: 10.1007/s11119-007-9043-z
Inman, D., Khosla, R., Westfall, D. G., and Reich, R. (2005). Nitrogen uptake across site specific management zones in irrigated corn production systems. Agro. J. 97, 169–176. doi: 10.2134/agronj2005.0169
Ishida, H., Izumi, M., Wada, S., and Makino, A. (2014). Roles of autophagy in chloroplastrecycling. Biochim. Biophys. Acta 1837, 512–521.
Jewel, Z., Ali, J., Mahender, A., Hernandez, J., Pang, Y., and Li, Z. (2019). Identification of quantitative trait loci associated with nutrient use efficiency traits, using SNP markers in an early backcross population of rice (Oryza sativa L.). Int. J. Mol. Sci. 20:900. doi: 10.3390/ijms20040900
Jin, T., Zhou, J., Chen, J., Zhu, L., Zhao, Y., and Huang, Y. (2013). The genetic architecture of zinc and iron content in maize grains as revealed by QTL mapping and meta-analysis. Breed. Sci. 63, 317–324.
Ju, C., Buresh, R. J., Wang, Z., Zhang, H., Liu, L., Yang, J., et al. (2015). Root and shoot traits for rice varieties with higher grain yield and higher nitrogen use efficiency at lower nitrogen rates application. Field Crop Res. 175, 47–55.
Kaiser, B. N., Gridley, K. L., Ngaire-Brady, J., Phillips, T., and Tyerman, S. D. (2005). The role of molybdenum in agricultural plant production. Ann. Bot. 96, 745–754.
Kant, S. (2017). Understanding nitrate uptake, signaling and remobilisation for improving plant nitrogen use efficiency. Cell Dev. Biol.
Kant, S., Seneweera, S., Rodin, J., Materne, M., Burch, D., Rothstein, S. J., et al. (2012). Improving yield potential in crops under elevated CO2: integrating the photosynthetic and nitrogen utilization efficiencies. Front. Plant Sci. 3:162. doi: 10.3389/fpls.2012.00162
Kapulnik, Y., Okon, Y., and Henis, Y. (1987). Yield response of spring wheat cultivars (Triticum aestivum and T. turgidum) to inoculation with Azospirillum brasilense under field conditions. Biol. Fertility Soil 4, 27–35.
Karamanos, R. E., Hanson, K., and Stevenson, F. C. (2014). Nitrogen form, time and rate of application, and nitrification inhibitor effects on crop production. Can. J. Plant Sci. 94, 425–432. doi: 10.4141/cjps2013-205
Karamos, R. E., Hanson, K., and Stevenson, F. C. (2014). Nitrogen form, time and rate of application, and nitrification inhibitor effects on crop production. Can. J. Plant Sci. 94, 425–432.
Kichey, T., Hirel, B., Heumez, E., Dubois, F., and Le Gouis, J. (2007). In winter wheat (Triticum aestivum L.), post-anthesis nitrogen uptake and remobilisation to the grain correlates with agronomic traits and nitrogen physiological markers. Field Crop Res. 102, 22–32. doi: 10.1016/j.fcr.2007.01.002
Konishi, M., and Yanagisawa, S. (2013). Arabidopsis NIN-like transcription factors have a central role in nitrate signalling. Nat. Commun. 4:1617. doi: 10.1038/ncomms2621
Kumar, A., Silim, S. N., Okamoto, M., Siddiqi, M. Y., and Glass, A. D. (2003). Differential expression of three members of the AMT1 gene family encoding putative high-affinity NH4 + transporters in roots of Oryza sativa subspecies indica. Plant Cell Env. 26, 907–914. doi: 10.1046/j.1365-3040.2003.01023.x
Kurai, T., Wakayama, M., Abiko, T., Yanagisawa, S., Aoki, N., and Ohsugi, R. (2011). Introduction of the ZmDof1 gene into rice enhances carbon and nitrogen assimilation under low-nitrogen conditions. Plant Biotec. J. 9, 826–837. doi: 10.1111/j.1467-7652.2011.00592.x
Ladha, J. K., Tirol-Padre, A., Reddy, C. K., Cassman, K. G., Verma, S., Powlson, D. S., et al. (2016). Global nitrogen budgets in cereals: A 50-year assessment for maize, rice and wheat production systems. Sci. Rep. 6:19355. doi: 10.1038/srep19355
Laperche, A., Brancourt-Hulmel, M., Heumez, E., Gardet, O., Hanocq, E., Devienne-Barret, F., et al. (2007a). Using genotype × nitrogen interaction variables to evaluate the QTL involved in wheat tolerance to nitrogen constraints. Theor. Appl. Genet. 115, 399–415. doi: 10.1007/s00122-007-0575-4
Laperche, A., Devienne-Barret, F., Maury, O., Le Gouis, J., and Ney, B. (2006). A simplified conceptual model of carbon/nitrogen functioning for QTL analysis of winter wheat adaptation to nitrogen deficiency. Theor. App. Gene. 112, 797–807. doi: 10.1007/s00122-005-0176-z
Laperche, A., Le Gouis, J., Hanocq, E., and Brancourt-Hulmel, M. (2007b). Modelling nitrogen stress with probe genotypes to assess genetic parameters and genetic determinism of winter wheat tolerance to nitrogen constraint. Euphy 161, 259–271. doi: 10.1007/s10681-007-9433-3
Lea, P. J., and Miflin, B. J. (2011). “Nitrogen assimilation and its relevance to crop improvement,” in Annual Plant Reviews, Nitrogen Metabolism in Plants in the Post-Genomic Era, ed. H. Zhang (Chichester: Wiley-Blackwell), 1–40.
Lei, L., Li, G., Zhang, H., Powers, C., Fang, T., Chen, Y., et al. (2018). Nitrogen use efficiency is regulated by interacting proteins relevant to development in wheat. Plant Biotec. J. 16, 1214–1226. doi: 10.1111/pbi.12864
Lemaire, G., van Oosterom, E., Sheehy, J., Jeuffroy, M. H., Massignam, A., and Rossato, L. (2007). Is crop N demand more closely related to dry matter accumulation or leaf area expansion during vegetative growth? Field Crops Res. 100, 91–106.
Léran, S., Varala, K., Boyer, J. C., Chiurazzi, M., Crawford, N., Daniel-Vedele, F., et al. (2014). A unified nomenclature of NITRATE TRANSPORTER 1/PEPTIDE TRANSPORTER family members in plants. T. Plant Sci. 19, 5–9.
Li, F., Chung, T., Pennington, J. G., Federico, M. L., Kaeppler, H. F., Kaeppler, S. M., et al. (2015). Autophagic recycling plays a central role in maizenitrogen remobilization. Plant Cell 7, 1389–1408.
Li, G., and Cui, K. (2014). Sucrose translocation and its relationship with grain yield formation in rice. Plant Physiol. J. 50, 735–740.
Li, H., Hu, B., and Chu, C. (2017). Nitrogen use efficiency in crops: Lessons from Arabidopsis and rice. J. Exp. Bot. 68, 2477–2488. doi: 10.1093/jxb/erx101
Li, P., Chen, F., Cai, H., Liu, J., Pan, Q., Liu, Z., et al. (2015). A genetic relationship between nitrogen use efficiency and seedling root traits in maize as revealed by QTL analysis. J. Exp. Bot. 66, 3175–3188. doi: 10.1093/jxb/erv127
Li, S., Zhao, B., Yuan, D., Duan, M., Qian, Q., Tang, L., et al. (2013). Rice zinc finger protein DST enhances grain production through controlling Gn1a/OsCKX2 expression. Proc. Natl. Acad. Sci. U.S.A. 110, 3167–3172.
Li, X., Xia, K., Liang, Z., Chen, K., Gao, C., and Zhang, M. (2016). MicroRNA393 is involved in nitrogen-promoted rice tillering through regulation of auxin signal transduction in axillary buds. Sci. Rep. 6:32158. doi: 10.1038/srep32158
Li, Y. D., Wang, Y. J., Tong, Y. P., Gao, J. G., Zhang, J. S., and Chen, S. Y. (2005). QTL mapping of phosphorus deficiency tolerance in soybean (Glycine max L. Merr.). Euphy 142, 137–142.
Liang, Q., Cheng, X., Mei, M., Yan, X., and Liao, H. (2010). QTL analysis of root traits as related to phosphorus efficiency in soybean. Ann. Bot. 106, 223–234.
Liao, H., Yan, X. L., Rubio, G., Beebe, S. E., and Blair, M. W. (2004). Genetic mapping of basal root gravitropism and phosphorus acquisition efficiency in common bean. Func. Plant Bio. 31, 959–970.
Liu, L., Xiao, W., Li, L., Li, D.-M., Gao, D.-S., Zhu, C.-Y., et al. (2017). Effect of exogenously applied molybdenum on its absorption and nitrate metabolism in strawberry seedlings. Plant Physiol. Biochem. 115, 200–211.
Liu, W., Sun, Q., Wang, K., Du, Q., and Li, W.-X. (2016). Nitrogen limitation adaptation (NLA) is involved in source-to-sink remobilization of nitrate by mediating the degradation of NRT1.7 in Arabidopsis. New Phytol. 214, 734–744.
Liu, X., Wang, H., Zhou, J., Hu, F., Zhu, D., Chen, Z., et al. (2016). Effect of N fertilization pattern on rice yield, N use efficiency and Fertilizer–N fate in the Yangtze River basin, China. PLoS One 11:e0166002. doi: 10.1371/journal.pone.0166002
Liu, Z., Zhu, C., Jiang, Y., Tian, Y., Yu, J., An, H., et al. (2016). Association mapping and genetic dissection of nitrogen use efficiency-related traits in rice (Oryza sativa L.). Func. Integ. Geno. 16, 323–333. doi: 10.1007/s10142-016-0486-z
Loladze, I. (2014). Hidden shift of the ionome of plants exposed to elevated CO2 depletes minerals at the base of human nutrition. eLife 3:e02245.
Long, S. P., Zhu, X., Naidu, S. L., and Ort, D. R. (2006). Can improvement in photosynthesis increase crop yields? Plant Cell Env. 29, 315–330. doi: 10.1111/j.1365-3040.2005.01493.x
Loqué, D., and von Wirén, N. (2004). Regulatory levels for the transport of ammonium in plant roots. J. Exp. Bot. 55, 1293–1305.
Lynch, J. P. (2007). Roots of the second Green Revolution. Aust. J. Bot. 55:493. doi: 10.1071/bt06118
Lynch, J. P. (2013). Steep, cheap and deep: an ideotype to optimize water and N acquisition by maize root systems. Ann. Bot. 112, 347–357.
Mahjourimajd, S., Kuchel, H., Langridge, P., and Okamoto, M. (2016). Evaluation of Australian wheat genotypes for response to variable nitrogen application. Plant Soil 399, 247–255. doi: 10.1007/s11104-015-2694-z
Maketon, C., Fortuna, A., and Okubara, P. A. (2012). Cultivar-dependent transcript accumulation in wheat roots colonized by Pseudomonas fluorescens q8r1-96 wild type and mutant strains. Bio. Cont. 60, 216–224. doi: 10.1016/j.biocontrol.2011.11.002
Mandal, V. K., Sharma, N., and Raghuram, N. (2018). “Molecular targets for improvement of crop nitrogen use efficiency: current and emerging options,” in Engineering Nitrogen Utilization in Crop Plants, eds A. Shrawat, A. Zayed, and D. Lightfoot (Cham: Springer). doi: 10.1007/978-3-319-92958-3_577
Mandolino, C. I., D’Andrea, K. E., Olmos, S. E., Otegui, M. E., and Eyhérabide, G. H. (2018). Maize nitrogen use efficiency: QTL mapping in a US Dent x Argentine-Caribbean flint RILs population. Mayd 63:17.
Marchive, C., Roudier, F., Castaings, L., Bréhaut, V., Blondet, E., Colot, V., et al. (2013). Nuclear retention of the transcription factor NLP7 orchestrates the early response to nitrate in plants. Nat. Commun. 4:1713. doi: 10.1038/ncomms2650
Martin, A., Lee, J., Kichey, T., Gerentes, D., Zivy, M., Tatout, C., et al. (2006). Two Cytosolic glutamine Synthetase Isoforms of maize are specifically involved in the control of grain production. Plant Cell 18, 3252–3274. doi: 10.1105/tpc.106.042689
Martre, P., Porter, J. R., Jamieson, P. D., and Triboï, E. (2003). Modeling grain nitrogen accumulation and protein composition to understand the sink/Source regulations of nitrogen Remobilization for wheat. Plant Physiol. 133, 1959–1967. doi: 10.1104/pp.103.030585
Masclaux-Daubresse, C., Daniel-Vedele, F., Dechorgnat, J., Chardon, F., Gaufichon, L., and Suzuki, A. (2010). Nitrogen uptake, assimilation and remobilization in plants: Challenges for sustainable and productive agriculture. Ann. Bot. 105, 1141–1157. doi: 10.1093/aob/mcq028
Matson, P., Lohse, K. A., and Hall, S. J. (2002). The globalization of nitrogen deposition: Consequences for terrestrial ecosystems. AMBIO Am J. Hu. Environ. 31, 113–119. doi: 10.1579/0044-7447-31.2.113
Mazzola, M., Funnell, D., and Raaijmakers, J. (2004). Wheat cultivar-specific selection of 2,4-Diacetylphloroglucinol-Producing fluorescent Pseudomonas species from resident soil populations. Micro. Ecol. 48, 338–348. doi: 10.1007/s00248-003-1067-y
Miller, R. O., Jacobsen, J. S., and Skogley, E. O. (1993). Aerial accumulation and partitioning of nutrients by hard red spring wheat. Comm. Soil Sci. Plant Anal. 24, 2389–2407. doi: 10.1080/00103629309368963
Ming, F., Zheng, X., Mi, G., He, P., Zhu, L., and Zhang, F. (2000). Identification of quantitative trait loci affecting tolerance to low phosphorus in rice (Oryza Sativa L.). Chinese Sci. Bull. 45:520.
Miyako, U., Yukiko, F., Masatomo, K., Motoyuki, A., Yukimoto, I., Hidemi, K., et al. (2000). Rice dwarf mutant d1, which is defective in the α subunit of the heterotrimeric G protein, affects gibberellin signal transduction. Proc. Natl. Acad. Sci. U.S.A. 97, 11638–11643.
Mochizuki, S., Jikumaru, Y., Nakamura, H., Koiwai, H., Sasaki, K., Kamiya, Y., et al. (2014). Ubiquitin ligase EL5 maintains the viability of root meristems by influencing cytokinin-mediated nitrogen effects in rice. J. Exp. Bot. 65, 2307–2318.
Moll, R. H., Kamprath, E. J., and Jackson, W. A. (1982). Analysis and interpretation of factors which contribute to efficiency of nitrogen utilization 1. Agro. J. 74, 562–564. doi: 10.2134/agronj1982.00021962007400030037x
Monaghan, J. M., Snape, J. W., Chojecki, A. J., and Kettlewell, P. S. (2001). The use of grain protein deviation for identifying wheat cultivars with high grain protein concentration and yield. Euphy 122, 309–317. doi: 10.1023/a:1012961703208
Monneveux, P., Zaidi, P. H., and Sanchez, C. (2005). Population density and low nitrogen affects yield-associated traits in tropical maize. Crop Sci. 45, 535–545. doi: 10.2135/cropsci2005.0535
Monostori, I., Szira, F., Tondelli, A., Arendas, T., Gierczik, K., Cattivelli, L., et al. (2017). Genome-wide association study and genetic diversity analysis on nitrogen use efficiency in a Central European winter wheat (Triticum aestivum L.) collection. PLoS One 12:e0189265.
Moreau, D., Allard, V., Gaju, O., Le Gouis, J., Foulkes, M. J., and Martre, P. (2012). Acclimation of leaf nitrogen to vertical light gradient at anthesis in wheat is a whole-plant process that scales with the size of the canopy. Plant Physiol. 160, 1479–1490. doi: 10.1104/pp.112.199935
Moubayidin, L., Di Mambro, R., and Sabatini, S. (2009). Cytokinin–auxin crosstalk. Tree Plant Sci. 14, 557–562. doi: 10.1016/j.tplants.2009.06.010
Mullen, R. W., Freeman, K. W., Raun, W. R., Johnson, G. V., Stone, M. L., and Solie, J. B. (2003). Identifying an in-season response index and the potential to increase wheat yield with nitrogen. Agro. J. 95, 347–351. doi: 10.2134/agronj2003.3470
Munier-Jolain, N. G., and Salon, C. (2005). Are the carbon costs of seed production related to the quantitative and qualitative performance? An appraisal for legumes and other crops. Plant Cell Environ. 28, 1388–1395. doi: 10.1111/j.1365-3040.2005.01371.x
Murchie, E. H., Pinto, M., and Horton, P. (2008). Agriculture and the new challenges for photosynthesis research. New Phytol. 181, 532–552. doi: 10.1111/j.1469-8137.2008.02705.x
Murgia, I., and Vigani, G. (2015). Analysis of arabidopsis thaliana after 4-1, atfh and after 4-1/atfh mutants uncovers frataxin and ferritin contributions to leaf ionome homeostasis. Plant Physiol. Biochem. 94, 65–72.
Mus, F., Crook, M., Garcia, K., Costas, A., Geddes, B., Kouri, E., et al. (2016). Symbiotic nitrogen fixation and the challenges to its extension to nonlegumes. Appl. Environ. Microbiol. 82, 3698–3710. doi: 10.1128/AEM.01055-16
Na, L., Li, Z., Xiangxiang, M., Ara, N., Jinghua, Y., and Mingfang, Z. (2014). E?ect of nitrate/ammonium ratios on growth, root morphology and nutrient elements uptake of watermelon (Citrullus lanatus) seedlings. J. Plant Nutr. 37, 1859–1872.
Naruoka, Y., Sherman, J. D., Lanning, S. P., Blake, N. K., Martin, J. M., and Talbert, L. E. (2012). Genetic analysis of green leaf duration in spring wheat. Crop Sci. 52, 99–109. doi: 10.2135/cropsci2011.05.0269
Neiverth, A., Delai, S., Garcia, D. M., Saatkamp, K., de Souza, E. M., de Oliveira Pedrosa, F., et al. (2014). Performance of different wheat genotypes inoculated with the plant growth promoting bacterium Herbaspirillum seropedicae. Eur. J. Soil Bio. 64, 1–5.
Nelson, D. R., and Mele, P. M. (2006). The impact of crop residue amendments and lime on microbial community structure and nitrogen- fixing bacteria in the wheat rhizosphere. Soil Rese. 44:319. doi: 10.1071/sr06022
Nemie-Feyissa, D., Królicka, A., Førland, N., Hansen, M., Heidari, B., and Lillo, C. (2013). Post-translational control of nitrate reductase activity responding to light and photosynthesis evolved already in the early vascular plants. J. Plant Physiol. 170, 662–667.
Nguyen, C. (2003). Rhizodeposition of organic C by plants: mechanisms and controls. Agro 23, 375–396. doi: 10.1051/agro:2003011
Nguyen, H. T., Dang, D. T., Van Pham, C., and Bertin, P. (2016). QTL mapping for nitrogen use efficiency and related physiological and agronomical traits during the vegetative phase in rice under hydroponics. Euphy 212, 473–500. doi: 10.1007/s10681-016-1778-z
Nieder, R., Benbi, D. K., and Scherer, H. W. (2010). Fixation and defixation of ammonium in soils: a review. Biol. Fert. Soils 47, 1–14. doi: 10.1007/s00374-010-0506-4
Nischal, L., Mohsin, M., Khan, I., Kardam, H., Wadhwa, A., Abrol, Y. P., et al. (2012). Identification and comparative analysis of MicroRNAs associated with Low-N tolerance in rice genotypes. PLoS One 7:e50261. doi: 10.1371/journal.pone.0050261
Nishizawa, Y., Mochizuki, S., Koiwai, H., Kondo, K., Kishimoto, K., Katoh, E., et al. (2015). Rice ubiquitin ligase EL5 prevents root meristematic cell death under high nitrogen conditions and interacts with a cytosolic GAPDH. Plant Signal. Behav. 10:e990801.
Noguero, M., Atif, R. M., Ochatt, S., and Thompson, R. D. (2013). The role of the DNA-binding one zinc finger (DOF) transcription factor family in plants. Plant Sci. 209, 32–45. doi: 10.1016/j.plantsci.2013.03.016
Obara, M., Kajiura, M., Fukuta, Y., Yano, M., Hayashi, M., Yamaya, T., et al. (2001). Mapping of QTLs associated with cytosolic glutamine synthetase and NADH-glutamate synthase in rice (Oryza sativa L.). J. Exp. Bot. 52, 1209–1217.
Ono, Y., Wada, S., Izumi, M., Makino, A., and Ishida, H. (2013). Evidence for contribution of autophagy to Rubisco degradation during leaf senescence in Arabidopsis thaliana. Plant Cell Environ. 36, 1147–1159.
Ortiz, R., Braun, H., Crossa, J., Crouch, J. H., Davenport, G., Dixon, J., et al. (2008). Wheat genetic resources enhancement by the international maize and wheat improvement center (CIMMYT). Gen. Res. Crop Evol. 55, 1095–1140. doi: 10.1007/s10722-008-9372-4
Ortíz-Castro, R., Contreras-Cornejo, H. A., Macías-Rodríguez, L., and López-Bucio, J. (2009). The role of microbial signals in plant growth and development. Plant Sig. Beh. 4, 701–712. doi: 10.4161/psb.4.8.9047
Osborne, S. L., Schepers, J. S., Francis, D. D., and Schlemmer, M. R. (2002). Use of spectral radiance to estimate in-season biomass and grain yield in nitrogen- and water-stressed corn. Crop Sci. 42:165. doi: 10.2135/cropsci2002.0165
O’Sullivan, C. A., Fillery, I. R., Roper, M. M., and Richards, R. A. (2016). Identification of several wheat landraces with biological nitrification inhibition capacity. Plant Soil 404, 61–74. doi: 10.1007/s11104-016-2822-4
Oury, F., and Godin, C. (2007). Yield and grain protein concentration in bread wheat: how to use the negative relationship between the two characters to identify favourable genotypes? Euphy 157, 45–57. doi: 10.1007/s10681-007-9395-5
Pascuan, C., Fox, A. R., Soto, G., and Ayub, N. D. (2015). Exploring the ancestral mechanisms of regulation of horizontally acquired nitrogenases. J. Mol. Evol. 81, 84–89.
Park, S. Y., Yu, J. W., Park, J. S., Li, J., Yoo, S. C., Lee, N. Y., et al. (2007). The senescence-induced staygreen protein regulates chlorophyll degradation. Plant Cell 19, 1649–1664.
Perchlik, M., and Tegeder, M. (2017). Improving plant nitrogen use efficiency through alteration of amino acid transport processes. Plant Physiol. 175, 235–247.
Plett, D., Toubia, J., Garnett, T., Tester, M., Kaiser, B. N., and Baumann, U. (2010). Dichotomy in the NRT gene families of dicots and grass species. PLoS One 5:e15289. doi: 10.1371/journal.pone.0015289
Plett, D. C., Holtham, L. R., Okamoto, M., and Garnett, T. P. (2018). Nitrate uptake and its regulation in relation to improving nitrogen use efficiency in cereals. Semin. Cell Dev. Biol. 74, 97–104. doi: 10.1016/j.semcdb.2017.08.027
Poudel, S., Colman, D. R., Fixen, K. R., Ledbetter, R. N., Zheng, Y., Pence, N., et al. (2018). Electron transfer to nitrogenase in different genomic and metabolic backgrounds. J. Bacteriol. 200, 757–717.
Pozzo, T., Higdon, S. M., Pattathil, S., Hahn, M. G., and Bennett, A. B. (2018). Characterization of novel glycosyl hydrolases discovered by cell wall glycan directed monoclonal antibody screening and metagenome analysis of maize aerial root mucilage. PLoS One 13:e0204525.
Prasad, M., Varshney, R. K., Kumar, A., Balyan, H. S., Sharma, P. C., Edwards, K. J., et al. (1999). A microsatellite marker associated with a QTL for grain protein content on chromosome arm 2DL of bread wheat. Theo. App. Genet. 99, 341–345.
Qin, S., Sun, X., Hu, C., Tan, Q., Zhao, X., Xin, J., et al. (2017). Effect of NO3 -:NH4+ ratios on growth, root morphology and leaf metabolism of oilseed rape (Brassica napus L.) seedlings. Acta Physiol Plant 39:198.
Qu, B., He, X., Wang, J., Zhao, Y., Teng, W., Shao, A., et al. (2015). A wheat CCAAT box-binding transcription factor increases the grain yield of wheat with less fertilizer input. Plant Physiol. 167, 411–423.
Ravel, C., Martre, P., Romeuf, I., Dardevet, M., El-Malki, R., Bordes, J., et al. (2009). Nucleotide polymorphism in the wheat transcriptional activator spa influences its pattern of expression and has pleiotropic effects on grain protein composition, dough viscoelasticity, and grain hardness. Plant Physiol. 151, 2133–2144. doi: 10.1104/pp.109.146076
Reddy, M. M., and Ulaganathan, K. (2015). Nitrogen nutrition, its regulation and biotechnological approaches to improve crop productivity. Am. J. Plant Sci. 6, 2745–2798.
Remans, T., Nacry, P., Pervent, M., Filleur, S., Diatloff, E., Mounier, E., et al. (2006). The Arabidopsis NRT1.1 transporter participates in the signaling pathway triggering root colonization of nitrate-rich patches. Proce. Natl. Acad. Sci. U.S.A. 103, 19206–19211. doi: 10.1073/pnas.0605275103
Ren, Y., Qian, Y., Xu, Y., Zou, C., Liu, D., Zhao, X., et al. (2017). Characterization of QTLs for root traits of wheat grown under different nitrogen and phosphorus supply levels. Front. Plant Sci. 8:2096. doi: 10.3389/fpls.2017.02096
Reynolds, M., Dreccer, F., and Trethowan, R. (2006). Drought-adaptive traits derived from wheat wild relatives and landraces. J. Exp. Bot. 58, 177–186. doi: 10.1093/jxb/erl250
Reynolds, M. P., Van Ginkel, M., and Ribaut, J. (2000). Avenues for genetic modification of radiation use efficiency in wheat. J. Exp. Bot. 51, 459–473. doi: 10.1093/jexbot/51.suppl_1.459
Ribaut, J., Fracheboud, Y., Monneveux, P., Banziger, M., Vargas, M., and Jiang, C. (2007). Quantitative trait loci for yield and correlated traits under high and low soil nitrogen conditions in tropical maize. Mol. Breed. 20, 15–29. doi: 10.1007/s11032-006-9041-2
Richardson, A. E., Barea, J., McNeill, A. M., and Prigent-Combaret, C. (2009). Acquisition of phosphorus and nitrogen in the rhizosphere and plant growth promotion by microorganisms. Plant Soil 321, 305–339. doi: 10.1007/s11104-009-9895-2
Robinson, D. (2002). Root proliferation, nitrate inflow and their carbon costs during nitrogen capture by competing plants in patchy soil. Int. Root Env: Int. Appr. 41–50. doi: 10.1007/978-94-010-0566-1_5
Ryu, M.-H., Zhang, J., Toth, T., Khokhani, D., Geddes, B. A., Mus, F., et al. (2020). Control of nitrogen fixation in bacteria that associate with cereals. Nat. Microbio 5, 314–330.
Safina, S. A. (2010). Effect of nitrogen levels on grain yield and quality of some barley genotypes grown on sandy soil and salinity irrigation. Egypt. J. Agro. 32, 207–222.
Sakakibara, Y., Kimura, H., Iwamura, A., Saitoh, T., Ikegami, T., Kurisu, G., et al. (2012). A new structural insight into differential interaction of cyanobacterial and plant ferredoxins with nitrite reductase as revealed by NMR and X-ray crystallographic studies. J. Biochem. 151, 483–492. doi: 10.1093/jb/mvs028
Sandhu, N., Raman, K. A., Torres, R. O., Audebert, A., Dardou, A., Kumar, A., et al. (2016). Rice root architectural plasticity traits and genetic regions for adaptability to variable cultivation and stress conditions. Plant Physiol. 171, 2562–2576.
Sandhu, N., Torres, R. O., Sta Cruz, M. T., et al. (2015). Traits and QTLs for development of dry direct-seeded rainfed rice varieties. J. Exp. Bot. 66, 225–244. doi: 10.1093/jxb/eru413
Santiago, J. P., and Tegeder, M. (2017). Implications of nitrogen phloem loading for carbon metabolism and transport during Arabidopsis development. J. Int. Plant Bio. 59, 409–421.
Senthilvel, S., Vinod, K. K., Malarvizhi, P., and Maheswaran, M. (2008). QTL and QTL × Environment effects on agronomic and nitrogen acquisition traits in rice. J. Int. Plant Bio. 50, 1108–1117. doi: 10.1111/j.1744-7909.2008.00713.x
Sétif, P., Hirasawa, M., Cassan, N., Lagoutte, B., Tripathy, J. N., and Knaff, D. B. (2009). New insights into the catalytic cycle of plant nitrite reductase. Electron transfer kinetics and charge storage. Biochem. 48, 2828–2838.
Shah, J. M., Bukhari, S. A. H., Zeng, J. B., QuAN, X. Y., Ali, E., Muhammad, N., et al. (2017). Nitrogen (N) metabolism related enzyme activities, cell ultrastructure and nutrient contents as affected by N level and barley genotype. J. Int. Agri. 16, 190–198.
Shahzad, Z., and Amtmann, A. (2017). Food for thought: how nutrients regulate root system architecture. Curr. Opin. Plant Biol. 39, 80–87.
Shan, Y. H., Wang, Y. L., and Pan, X. B. (2005). Mapping of QTLs for nitrogen use efficiency and related traits in rice (Oryza sativa L.). Agric. Sci. Chin. 4, 721–727. doi: 10.1007/s10142-016-0486-z
Sharma, L., Bu, H., Franzen, D., and Denton, A. (2016). Use of corn height measured with an acoustic sensor improves yield estimation with ground based active optical sensors. Comp. Elect. Agri. 124, 254–262. doi: 10.1016/j.compag.2016.04.016
Sharma, L. K., Bu, H., and Franzen, D. W. (2015). Comparison of two ground-based active-optical sensors for in-season estimation of corn (Zea mays L.) yield. J. Plant Nutr. 39, 957–966. doi: 10.1080/01904167.2015.1109109
Sharma, L. K., and Franzen, D. W. (2013). Use of corn height to improve the relationship between active optical sensor readings and yield estimates. Prec. Agri. 15, 331–345. doi: 10.1007/s11119-013-9330-9
Shaver, T., Khosla, R., and Westfall, D. (2014). Evaluation of two crop canopy sensors for nitrogen recommendations in irrigated maize. J. Plant Nutr. 37, 406–419. doi: 10.1080/01904167.2013.860460
Shewry, P. R., and Halford, N. G. (2002). Cereal seed storage proteins: Structures, properties and role in grain utilization. J. Exp. Bot. 53, 947–958. doi: 10.1093/jexbot/53.370.947
Shrawat, A. K., Carroll, R. T., DePauw, M., Taylor, G. J., and Good, A. G. (2008). Genetic engineering of improved nitrogen use efficiency in rice by the tissue−specific expression of alanine aminotransferase. Plant Biotech. J. 6, 722–732.
Siddiqi, M. Y., and Glass, A. D. (1981). Utilization index: a modified approach to the estimation and comparison of nutrient utilization efficiency in plants. J. Plant Nutr. 4, 289–302. doi: 10.1080/01904168109362919
Siddiqi, M. Y., Glass, A. D., and Ruth, T. J. (1991). Studies of the uptake of nitrate in Barley. J. Exp. Bot. 42, 1455–1463. doi: 10.1093/jxb/42.11.1455
Sinclair, T. R., Rufty, T. W., and Lewis, R. S. (2019). Increasing Photosynthesis: unlikely solution for world food problem. Trends Plant Sci. 24, 1032–1039.
Sonoda, Y., Ikeda, A., Saiki, S., Wirén, N. V., Yamaya, T., and Yamaguchi, J. (2003). Distinct expression and function of three ammonium transporter genes (OsAMT1;1 – 1;3) in rice. Plant Cell Physiol. 44, 726–734. doi: 10.1093/pcp/pcg083
Spitz, F., and Furlong, E. E. (2012). Transcription factors: From enhancer binding to developmental control. Nat. Rev. Genet. 13, 613–626. doi: 10.1038/nrg3207
Su, J., Xiao, Y., Li, M., Liu, Q., Li, B., Tong, Y., et al. (2006). Mapping QTLs for phosphorus-deficiency tolerance at wheat seedling stage. Plant Soil 281, 25–36.
Su, J. Y., Zheng, Q., Li, H. W., Li, B., Jing, R. L., Tong, Y. P., et al. (2009). Detection of QTLs for phosphorus use efficiency in relation to agronomic performance of wheat grown under phosphorus sufficient and limited conditions. Plant Sci. 176, 824–836.
Sun, J. J., Guo, Y., Zhang, G. Z., Gao, M. G., Zhang, G. H., Kong, F. M., et al. (2013). QTL mapping for seedling traits under different nitrogen forms in wheat. Euphytica 191, 317–331. doi: 10.1007/s10681-012-0834-6
Subbarao, G. V., Tomohiro, B., Masahiro, K., Osamu, I., Samejima, H., Wang, H. Y., et al. (2007). Can biological nitrification inhibition (BNI) genes from perennial Leymus racemosus (Triticeae) combat nitrification in wheat farming? Plant Soil 299, 55–64. doi: 10.1007/s11104-007-9360-z
Suenaga, A., Moriya, K., Sonoda, Y., Ikeda, A., Von Wirén, N., Hayakawa, T., et al. (2003). Constitutive expression of a novel-type ammonium transporter OsAMT2 in rice plants. Plant Cell Physiol. 44, 206–211. doi: 10.1093/pcp/pcg017
Sun, H., Qian, Q., Wu, K., Luo, J., Wang, S., Zhang, C., et al. (2014). Heterotrimeric G proteins regulate nitrogen-use efficiency in rice. Nat. Genet. 46, 652–656.
Sun, J., Guo, Y., Zhang, G., Gao, M., Zhang, G., Kong, F., et al. (2012). QTL mapping for seedling traits under different nitrogen forms in wheat. Euphyt 191, 317–331. doi: 10.1007/s10681-012-0834-6
Sun, Q., Liu, X., Yang, J., Liu, W., Du, Q., Wang, H., et al. (2018). MicroRNA528 affects lodging resistance of maize by regulating lignin biosynthesis under nitrogen-luxury conditions. Mol. Plant 11, 806–814. doi: 10.1016/j.molp.2018.03.013
Suzuki, A., and Knaff, D. B. (2005). Glutamate synthase: structural, mechanistic and regulatory properties, and role in the amino acid metabolism. Photosynthesis Res. 83, 191–217. doi: 10.1007/s11120-004-3478-0
Sylvester-Bradley, R., Stokes, D. T., Scott, R. K., and Willington, V. B. A. (1990). A physiological analysis of the diminishing responses of winter wheat to applied nitrogen. 2. Evidence. Aspects Appl. Biol. 25, 289–300.
Tabuchi, M., Abiko, T., and Yamaya, T. (2007). Assimilation of ammonium ions and reutilization of nitrogen in rice (Oryza sativa L.). J. Exp. Bot. 58, 2319–2327. doi: 10.1093/jxb/erm016
Tamura, W., Kojima, S., Toyokawa, A., Watanabe, H., Tabuchi-Kobayashi, M., Hayakawa, T., et al. (2011). Disruption of a Novel NADH-Glutamate Synthase2 gene caused marked reduction in spikelet number of rice. Front. Plant Sci. 2:57. doi: 10.3389/fpls.2011.00057
Taylor, M. R., Reinders, A., and Ward, J. M. (2015). Transport function of rice amino acidpermeases (AAPs). Plant Cell Physiol. 56, 1355–1363.
Thomas, H., and Smart, C. M. (1993). Crops that stay green. Ann. Appl. Bio. 123, 193–219. doi: 10.1111/j.1744-7348.1993.tb04086.x
Tilman, D., Balzer, C., Hill, J., and Befort, B. L. (2011). Global food demand and the sustainable intensification of agriculture. Proc. Natl. Acad. Sci. U.S.A. 108, 20260–20264. doi: 10.1073/pnas.1116437108
Tong, H., Chen, L., Li, W., Mei, H., Xing, Y., Yu, X., et al. (2010). Identification and characterization of quantitative trait loci for grain yield and its components under different nitrogen fertilization levels in rice (Oryza sativa L.). Mol. Breed. 28, 495–509. doi: 10.1007/s11032-010-9499-9
Trevisan, S., Nonis, A., Begheldo, M., Manoli, A., Palme, K., Caporale, G., et al. (2012). Expression and tissue-specific localization of nitrate-responsive miRNAs in roots of maize seedlings. Plant Cell Environ. 35, 1137–1155. doi: 10.1111/j.1365-3040.2011.02478.x
Triplett, E. W., Kaeppler, S. M., and Chelius, M. K. (2008). Klebsiella pneumoniae inoculants for Enhancing Plant Growth. US patent 7,393,678. Madison, WI: Wisconsin Alumni Research.
Uauay, C., Brevis, J. C., and Dubcovsky, J. (2006). The high grain protein content gene Gpc-B1 accelerates senescence and has pleiotropic effects on protein content in wheat. J. Exp. Bot. 57, 2785–2794.
Uauy, C., Distelfeld, A., Fahima, T., Blechl, A., and Dubcovsky, J. (2006). A NAC gene regulating senescence improves grain protein, zinc, and iron content in wheat. Science 314, 1298–1301. doi: 10.1126/science.1133649
Upadhyay, S. K., Singh, D. P., and Saikia, R. (2009). Genetic diversity of plant growth promoting Rhizobacteria isolated from Rhizospheric soil of wheat under saline condition. Curr. Microbio. 59, 489–496. doi: 10.1007/s00284-009-9464-1
Van Deynze, A., Zamora, P., Delaux, P. M., Heitmann, C., Jayaraman, D., Rajasekar, S., et al. (2018). Nitrogen fixation in a landrace of maize is supported by a mucilage-associated diazotrophic microbiota. PLoS Boil 16:e2006352.
Venieraki, A., Dimou, M., Pergalis, P., Kefalogianni, I., Chatzipavlidis, I., and Katinakis, P. (2010). The genetic diversity of culturable nitrogen-fixing bacteria in the rhizosphere of wheat. Micro. Eco. 61, 277–285. doi: 10.1007/s00248-010-9747-x
Von Wirén, N., Gazzarrini, S., and Frommer, W. B. (1997). Regulation of mineral uptake in plants. Plant Soil 196, 191–199. doi: 10.1023/a:1004241722172
Wang, W., Köhler, B., Cao, F., Liu, G., Gong, Y., Sheng, S., et al. (2011). Rice DUR3 mediates high-affinity urea transport and plays an effective role in improvement of urea acquisition and utilization when expressed in Arabidopsis. New Phytol. 193, 432–444. doi: 10.1111/j.1469-8137.2011.03929.x
Waters, B., and Sankaran, R. P. (2011). Moving micronutrients from the soil to the seeds: Genes and physiological processes from a bioforti?cation perspective. Plant Sci. 180, 562–574.
Waters, B. M., Uauy, C., Dubcovsky, J., and Grusak, M. A. (2009). Wheat (Triticum aestivum) NAM proteins regulate the translocation of iron, zinc, and nitrogen compounds from vegetative tissues to grain. J. Exp. Bot. 60, 4263–4274. doi: 10.1093/jxb/erp257
Wei, D., Cui, K., Pan, J., Ye, G., Xiang, J., Nie, L., et al. (2011). Genetic dissection of grain nitrogen use efficiency and grain yield and their relationship in rice. Field Crops Res. 124, 340–346. doi: 10.1016/j.fcr.2011.07.003
Wei, D., Cui, K., Ye, G., Pan, J., Xiang, J., Huang, J., et al. (2012). QTL mapping for nitrogen-use efficiency and nitrogen-deficiency tolerance traits in rice. Plant Soil 359, 281–295.
Wirén, N. V., and Merrick, M. (2004). Regulation and function of ammonium carriers in bacteria, fungi, and plants. Mol. Mech. Cont. Transmem. Transport 95–120. doi: 10.1007/b95775
Wissuwa, M., Yano, M., and Ae, N. (1998). Mapping of QTLs for phosphorus-deficiency tolerance in rice (Oryza sativa L.). Theo. App. Genet. 97, 777–783.
Witte, C. (2011). Urea metabolism in plants. Plant Sci. 180, 431–438. doi: 10.1016/j.plantsci.2010.11.010
Wu, H., Haig, T., Pratley, J., Lemerle, D., and An, M. (2001). Allelochemicals in wheat (Triticum aestivum L.): Cultivar difference in the exudation of phenolic acids. J. Agri. Food Chem. 49, 3742–3745. doi: 10.1021/jf010111x
Wu, Y., Yang, W., Wei, J., Yoon, H., and An, G. (2017). Transcription factor OsDOF18 controls ammonium uptake by inducing ammonium transporters in rice roots. Mol. Cells 40, 178–185. doi: 10.14348/molcells.2017.2261
Xu, C., Chen, L., Chen, S., Chu, G., Wang, D. Y., and Zhang, X. F. (2020). Rhizosphere aeration improves nitrogen transformation in soil, and nitrogen absorption and accumulation in rice plants. Rice Sci. 27, 162–174.
Xu, G., Fan, X., and Miller, A. J. (2012). Plant nitrogen assimilation and use efficiency. Annu. Rev. Plant Biol. 63, 153–182. doi: 10.1146/annurev-arplant-042811-105532
Xu, N., Wang, R., Zhao, L., Zhang, C., Li, Z., Lei, Z., et al. (2016). The Arabidopsis NRG2 protein mediates nitrate signaling and interacts with and regulates key nitrate regulators. Plant Cell 28, 485–504. doi: 10.1105/tpc.15.00567
Xuan, W., Beeckman, T., and Xu, G. (2017). Plant nitrogen nutrition: sensing and signaling. Curr. Opin. Plant Biol. 39, 57–65.
Xu, Y., Wang, R., Tong, Y., Zhao, H., Xie, Q., Liu, D., et al. (2013). Mapping QTLs for yield and nitrogen-related traits in wheat: Influence of nitrogen and phosphorus fertilization on QTL expression. Theor. App. Gene. 127, 59–72. doi: 10.1007/s00122-013-2201-y
Xu, Y., Wang, R., Tong, Y., Zhao, H., Xie, Q., Liu, D., et al. (2014). Mapping QTLs for yield and nitrogen-related traits in wheat: influence of nitrogen and phosphorus fertilization on QTL expression. Theor. App. Genet. 127, 59–72.
Xu, Z., Zhong, S., Li, X., Li, W., Rothstein, S. J., Zhang, S., et al. (2011). Genome-wide identification of MicroRNAs in response to low nitrate availability in maize leaves and roots. PLoS One 6:e28009. doi: 10.1371/journal.pone.0028009
Yamaya, T. (2011). Disruption of a novel NADH-glutamate synthase2 gene caused marked reduction in spikelet number of rice. Front. Plant Sci. 2:57.
Yamaya, T., and Kusano, M. (2014). Evidence supporting distinct functions of three cytosolic glutamine synthetases and two NADH-glutamate synthases in rice. J. Exp. Bot. 65, 5519–5525. doi: 10.1093/jxb/eru103
Yamaya, T., Obara, M., Nakajima, H., Sasaki, S., Hayakawa, T., and Sato, T. (2002). Genetic manipulation and quantitative−trait loci mapping for nitrogen recycling in rice. J. Exp. Bot. 53, 917–925.
Yan, X., Liao, H., Beebe, S. E., Blair, M. W., and Lynch, J. P. (2004). QTL mapping of root hair and acid exudation traits and their relationship to phosphorus uptake in common bean. Plant Soil 265, 17–29.
Yan, Y., Wang, H., Hamera, S., Chen, X., and Fang, R. (2014). MiR444a has multiple functions in the rice nitrate-signaling pathway. Plant J. 78, 44–55.
Yang, C., Yang, L., Yang, Y., and Ouyang, Z. (2004). Rice root growth and nutrient uptakeas influenced by organic manure in continuously and alternately flooded paddysoils. Agric. Water Manag. 70, 67–81.
Yang, X., Xia, X., Zhang, Z., Nong, B., Zeng, Y., Xiong, F., et al. (2017). QTL mapping by whole genome re-sequencing and analysis of candidate genes for nitrogen use efficiency in rice. Front. Plant Sci. 8:1634. doi: 10.3389/fpls.2017.01634
Yu, C., Liu, Y., Zhang, A., Su, S., Yan, A., Huang, L., et al. (2015). MADS-box transcription factor OsMADS25 regulates root development through affection of nitrate accumulation in rice. PLoS One 10:e0135196.
Yuan, S., Li, Z., Li, D., Yuan, N., Hu, Q., and Luo, H. (2015). Constitutive expression of rice MicroRNA528 alters plant development and enhances tolerance to salinity stress and nitrogen starvation in creeping Bentgrass. Plant Physiol. 169, 576–593. doi: 10.1104/pp.15.00899
Zeng, D.-D., Qin, R., Li, M., Alamin, M., Jin, X.-L., Liu, Y., et al. (2016). The ferredoxin-dependent glutamate synthase (OsFd-GOGAT) participates in leaf senescence and the nitrogen remobilization in rice. Mol. Genet. Geno 292, 385–395. doi: 10.1007/s00438-016-1275-z
Zhang, G. H., Hou, X., Wang, L., Xu, J., Chen, J., Fu, X., et al. (2020). PHOTO-SENSITIVE LEAF ROLLING 1 encodes a polygalacturonase that modifies cell wall structure and drought tolerance in rice. New Phytol. 229, 890-901. doi: 10.1111/nph.16899
Zhang, H. (1998). An Arabidopsis MADS box gene that controls nutrient-induced changes in root architecture. Science 279, 407–409. doi: 10.1126/science.279.5349.407
Zhang, H., Xue, Y., Wang, Z., Yang, J., and Zhang, J. (2009). An alternate wetting and mod-erate soil drying regime improves root and shoot growth in rice. Crop Sci. 49, 2246–2260. doi: 10.2135/cropsci2009.02.0099
Zhang, L. Z., Garneau, M. G., Majumdar, R., Grant, J., and Tegeder, M. (2015). Improvement of pea biomass and seed productivity by simultaneous increase of phloem and embryo loading with amino acids. Plant J 81, 134–146. doi: 10.1111/tpj.12716
Zhang, M., Gao, M., Zheng, H., Yuan, Y., Zhou, X., Guo, Y., et al. (2019). QTL mapping for nitrogen use efficiency and agronomic traits at the seedling and maturity stages in wheat. Mol. Breed. 39:71. doi: 10.1007/s11032-019-0965-8
Zhao, M., Tai, H., Sun, S., Zhang, F., Xu, Y., and Li, W. (2012). Cloning and characterization of maize miRNAs involved in responses to nitrogen deficiency. PLoS One 7:e29669. doi: 10.1371/journal.pone.0029669
Zhao, Y., Guo, L., Lu, W., Li, X., Chen, H., Guo, C., et al. (2013a). Expression pattern analysis of microRNAs in root tissue of wheat (Triticum aestivum L.) under normal nitrogen and low nitrogen conditions. J. Plant Biochem. Biotech. 24, 143–153. doi: 10.1007/s13562-013-0246-2
Zhao, Y., Xu, Z., Mo, Q., Zou, C., Li, W., Xu, Y., et al. (2013b). Combined small RNA and degradome sequencing reveals novel miRNAs and their targets in response to low nitrate availability in maize. Ann. Bot. 112, 633–642. doi: 10.1093/aob/mct133
Zheng, B. S., Le Gouis, J., Leflon, M., Rong, W. Y., Laperche, A., and Brancourt-Hulmel, M. (2010). Using probe genotypes to dissect QTL × environment interactions for grain yield components in winter wheat. Theor. Appl. Genet. 121, 1501–1517. doi: 10.1007/s00122-010-1406-6
Zhou, Y., Cai, H., Xiao, J., Li, X., Zhang, Q., and Lian, X. (2009). Over-expression of aspartate aminotransferase genes in rice resulted in altered nitrogen metabolism and increased amino acid content in seeds. Theor. Appl. Genet. 118, 1381–1390. doi: 10.1007/s00122-009-0988-3
Zhou, Y., Tao, Y., Tang, D., Wang, J., Zhong, J., Wang, Y., et al. (2017). Identification of QTL associated with nitrogen uptake and nitrogen use efficiency using high throughput Genotyped CSSLs in rice (Oryza sativa L.). Front. Plant Sci. 8:1166. doi: 10.3389/fpls.2017.01166
Zhu, J., Kaeppler, S. M., and Lynch, J. P. (2005). Mapping of QTL controlling root hair length in maize (Zea mays L.) under phosphorus deficiency. Plant Soil 270, 299–310. doi: 10.1007/s11104-004-1697-y
Zhu, X., Long, S. P., and Ort, D. R. (2010). Improving photosynthetic efficiency for greater yield. Ann. Rev. Plant Bio. 61, 235–261. doi: 10.1146/annurev-arplant-042809-112206
Zhu, H., Li, C., and Gao, C. (2020). Author Correction: applications of CRISPR-Cas in agriculture and plant biotechnology. Nat. Rev. Mol. Cell Biol. 21:782. doi: 10.1038/s41580-020-00312-y
Zuluaga, D. L., De Paola, D., Janni, M., Curci, P. L., and Sonnante, G. (2017). Durum wheat miRNAs in response to nitrogen starvation at the grain filling stage. PLoS One 12:e0183253. doi: 10.1371/journal.pone.0183253
Keywords: biochemical, cereal, genetic, genes, nitrogen use efficiency, N fertilizer, QTL
Citation: Sandhu N, Sethi M, Kumar A, Dang D, Singh J and Chhuneja P (2021) Biochemical and Genetic Approaches Improving Nitrogen Use Efficiency in Cereal Crops: A Review. Front. Plant Sci. 12:657629. doi: 10.3389/fpls.2021.657629
Received: 23 January 2021; Accepted: 06 April 2021;
Published: 04 June 2021.
Edited by:
Carla S. Santos, Catholic University of Portugal, PortugalReviewed by:
Upendra Singh, International Fertilizer Development Center, United StatesMubshar Hussain, Bahauddin Zakariya University, Pakistan
Copyright © 2021 Sandhu, Sethi, Kumar, Dang, Singh and Chhuneja. This is an open-access article distributed under the terms of the Creative Commons Attribution License (CC BY). The use, distribution or reproduction in other forums is permitted, provided the original author(s) and the copyright owner(s) are credited and that the original publication in this journal is cited, in accordance with accepted academic practice. No use, distribution or reproduction is permitted which does not comply with these terms.
*Correspondence: Nitika Sandhu, bml0aWthc2FuZGh1QHBhdS5lZHU=
†These authors have contributed equally to this work