- 1College of Agronomy and Biotechnology, Yunnan Agricultural University, Kunming, China
- 2Key Laboratory of Medicinal Plant Biology of Yunnan Province, Yunnan Agricultural University, Kunming, China
- 3National and Local Joint Engineering Research Center on Germplasm Innovation and Utilization of Chinese Medicinal Materials in Southwestern China, Yunnan Agricultural University, Kunming, China
Light is highly heterogeneous in natural conditions, and plants need to evolve a series of strategies to acclimate the dynamic light since it is immobile. The present study aimed to elucidate the response of light reaction of photosynthesis to dynamic sunflecks in a shade-tolerant species Panax notoginseng and to examine the regulatory mechanisms involved in an adaptation to the simulated sunflecks. When P. notoginseng was exposed to the simulated sunflecks, non-photochemical quenching (NPQ) increased rapidly to the maximum value. Moreover, in response to the simulated sunflecks, there was a rapid increase in light-dependent heat dissipation quantum efficiency of photosystem II (PSII) (ΦNPQ), while the maximum quantum yield of PSII under light (Fv′/Fm′) declined. The relatively high fluorescence and constitutive heat dissipation quantum efficiency of PSII (Φf,d) in the plants exposed to transient high light (400, 800, and 1,600 μmol m–2 s–1) was accompanied by the low effective photochemical quantum yield of PSII (ΦPSII) after the dark recovery for 15 min, whereas the plants exposed to transient low light (50 μmol m–2 s–1) has been shown to lead to significant elevation in ΦPSII after darkness recovery. Furthermore, PSII fluorescence and constitutive heat dissipation electron transfer rate (Jf,d) was increased with the intensity of the simulated sunflecks, the residual absorbed energy used for the non-net carboxylative processes (JNC) was decreased when the response of electron transfer rate of NPQ pathway of PSII (JNPQ) to transient low light is restricted. In addition, the acceptor-side limitation of PSI [Y(NA)] was increased, while the donor-side limitation of photosystems I (PSI) [Y(ND)] was decreased at transient high light conditions accompanied with active cyclic electron flow (CEF). Meanwhile, when the leaves were exposed to transient high light, the xanthophyll cycle (V cycle) was activated and subsequently, the JNPQ began to increase. The de-epoxidation state [(Z + A)/(V + A + Z)] was strongly correlated with NPQ in response to the sunflecks. In the present study, a rapid engagement of lutein epoxide (Lx) after the low intensity of sunfleck together with the lower NPQ contributed to an elevation in the maximum photochemical quantum efficiency of PSII under the light. The analysis based on the correlation between the CEF and electron flow devoted to Ribulose-1, 5-bisphosphate (RuBP) oxygenation (JO) indicated that at a high light intensity of sunflecks, the electron flow largely devoted to RuBP oxygenation would contribute to the operation of the CEF. Overall, photorespiration plays an important role in regulating the CEF of the shade-tolerant species, such as P. notoginseng in response to transient high light, whereas active Lx cycle together with the decelerated NPQ may be an effective mechanism of elevating the maximum photochemical quantum efficiency of PSII under light exposure to transient low light.
Introduction
Light is highly heterogeneous in natural conditions since it fluctuates over short (seconds) and long (hours, days, and seasons) timescales (Townsend et al., 2017, 2018a,b). The fluctuating light usually lasts only a few seconds to minutes, but it can contribute 20–80% of the total solar energy received by the leaves (Chazdon and Pearcy, 1991). On the other hand, plants (e.g., understory species) are commonly exposed to transient high light, which can readily exceed its requirement for photosynthesis (Ruban, 2016; Townsend et al., 2018b). The excessive light energy may induce photo-inhibition of photosystem II (PSII) and even cause photo-damage to the photosynthetic apparatus, and consequently reduce the photosynthetic carbon gain (Niyogi and Truong, 2013; Vialetchabrand et al., 2017). Photoprotection in the shade-tolerant species exposed to dynamic sunflecks is particularly important as the time to reach maximum photosynthetic rate lags dramatically behind the onset of sunflecks (Way and Pearcy, 2012; Mathur et al., 2018). The model plant Arabidopsis thaliana might optimize electron transport and PSI photoprotection mediated by phosphorylation of vesicle-like proteins in response to the dynamic sunflecks (Tikkanen et al., 2010; Grieco et al., 2012). It is, therefore, crucial to understand the mechanism underlying photoprotection in the shade-tolerant species under dynamic light conditions, however, it receive relatively little attention over the past decades (Tikkanen et al., 2012).
Non-photochemical quenching (NPQ) is one of the most effective ways for plants to dissipate excess light energy. The mechanism of NPQ associated with heat dissipation is not well understood, but PsbS proteins and the xanthophyll cycle play an important role in regulating the NPQ processes (Hubbart et al., 2012; Ikeuchi et al., 2014). When tobacco (Nicotiana tabacum) and A. thaliana are exposed to constant high light, violaxanthin (V) is de-epoxied to form antheraxanthin (A), which is further de-epoxied to form zeaxanthin (Z) (Garcia-Molina and Leister, 2020; Tan et al., 2020). Z is used as the quenching site of excess excitation energy to dissipate excess light energy (Jahns and Holzwarth, 2012). However, in N. tabacum and A. thaliana grown under constant low light, the epoxidation of Z to V may accelerate the biomass production by increasing the photosynthetic efficiency (Cao et al., 2018; Da et al., 2018). NPQ is positively correlated with V de-epoxidation state [(Z + A)/(V + A + Z)] under steady-state light, however, it is still unknown whether the xanthophyll cycle (V cycle) is activated and it relates to NPQ under the transient high light. In addition, lutein epoxide (Lx) cycle is widely found in the shade-tolerant plants (Bungard et al., 1999; Matsubara et al., 2005; Esteban et al., 2010; Förster et al., 2011; García-Plazaola et al., 2012). It has been reported that the Lx cycle is activated to quench the excess light energy and function as a photoprotector in the understory shade plants Virola elongata and Inga sapindoides in response to the dynamic sunflecks (Matsubara et al., 2007, 2009). However, it has not been extensively studied for the presence of the Lx cycle in the typically shade-tolerant species and the role of the Lx cycle in response to different intensities of dynamic light.
Absorbed light energy is managed through several competitive pathways, such as thermal processes fluorescence, and photochemistry (Hendrickson et al., 2004; Ishida et al., 2014). NPQ-associated thermal dissipation is a photoprotection mechanism of PSII (Demmig-Adams et al., 2012; Jahns and Holzwarth, 2012). Surprisingly, only a small proportion of leaf-absorbed light energy is finally used for photosynthetic carboxylation, with most of the energy lost through the regulatory thermal dissipation and fluorescence under high light conditions (Hendrickson et al., 2004; Chen et al., 2016; Huang et al., 2019). Thus, the excitation energy use and energy allocation in PSII complexes are important for resolving the response of photosynthetic organs to the environmental factors (Hendrickson et al., 2004; Kornyeyev and Hendrickson, 2007; Chen et al., 2016). The quantum yield of dissipation associated with NPQ (ΦNPQ) is high and the quantum yield of electron transport in PSII (ΦPSII) is low in rice (Oryza sativa) grown under constant high light (Ishida et al., 2014), suggesting that most of the light energy absorbed by the plants is safely dissipated in the form of NPQ. However, relatively little is known about the distribution and balance of light energy absorbed by PSII in the typically shade-tolerant plants in response to different intensities of transient light.
The cyclic electron flow around photosystem I (CEF-PSI) is thought to protect plants from the damages that occurs due to the over-reduction of the thylakoids under fluctuating light (Huang et al., 2011, 2012b; Kono et al., 2014). The CEF-dependent proton gradient not only promotes ATP synthesis but also binds the heat dissipation and oxygen evolving complex to protect PSII from photo-inhibition. Furthermore, the interception of PSI photo-inhibition is mainly due to the reduction of over-reduction and superoxide anion production on the PSI receptor side by CEF (Huang et al., 2012a). CEF activates the thermal dissipation process of NPQ by inducing the formation of ΔpH (proton gradient across the thylakoid membrane), regulates the redox state of P700, and controls the electron transport by the Cytb6f complex (Cytochrome b6f complex), thereby protecting PSII and PSI from photooxidation (Miyake et al., 2005; Huang et al., 2015b,c). This has also been confirmed by the performance observed in N. tabacum grown under high light (Endo et al., 1999). The evidence is accumulating that mitigation of PSI donor and acceptor side photoinhibition in Cerasus cerasoides, A. thaliana, and Bletilla striata grown under fluctuating light could be achieved by CEF initiation (Yang et al., 2019a,b). The plants increase the oxidation of P700 to inhibit the production of reactive oxygen species (ROS) through CEF in response to high light (Miyake et al., 2005; Huang et al., 2015b). Moreover, the expression level of NDH-dependent CEF genes was elevated, suggesting that CEF activation could meet the ATP/nicotinamide adenine dinucleotide phosphate (NADPH) requirements of increased photorespiration (Tikkanen et al., 2010; Suorsa et al., 2012). Thus, there is a tight connection between the CEF and photorespiration (Foyer et al., 2012; Sunil et al., 2019). Surprisingly, little is known about the role of the photorespiratory pathway in conjunction with the CEF in adapting to different intensities of transient light in the shade plants, which are often disturbed by sunflecks.
Panax notoginseng (Burkill) is a perennial herb belonging to the Araliaceae family, which is a typically shade-tolerant species (Chen et al., 2016; Xu et al., 2018). In agricultural production, P. notoginseng is planted in a shaded environment constructed by the shade nets. In our previous work, 9.6–11.5% of full sunlight (FL) is preferred for the growth of P. notoginseng (Zuo et al., 2014; Kuang et al., 2015; Xu et al., 2018). It has been demonstrated that more electrons in the high-light-grown P. notoginseng illuminated by constant high light were consumed by the non-net carboxylative processes to enhance the NPQ associated with heat dissipation; correspondingly, low-light-grown P. notoginseng protects the photosynthetic apparatus from photo-damage by decelerating the photochemical efficiency of PSII (Chen et al., 2016). The photosynthetic rate of P. notoginseng responds quickly to sunflecks and the response rate is more significantly limited by stomata in our previous study (Chen et al., 2014). However, fewer studies have previously investigated the light reaction of photosynthesis in the typically shade-tolerant species P. notoginseng exposed to sunflecks with different intensities. In the present study, we hypothesized that: (1) the greater increase in CEF activity in response to the transient low light may accommodate the electron flows; (2) Lx cycle exist in the typically shade-tolerant species in response to the transient low light, and Lx may play the role of improving light-harvesting efficiency; (3) NPQ coupled to the de-epoxidation in the V cycle might attribute to the energy dissipation in response to the transient high light; (4) the photorespiration pathways may play a role in regulating the photosynthetic electron flow under transient high light condition.
Materials and Methods
The Growth Condition
The experiments were conducted at the experimental farm of Yunnan Agricultural University in Kunming, Yunnan Province, China (altitude, 1,976 m; longitude 102°45′32″, latitude 25°8′2″; annual precipitation, 1,000 mm; annual average temperature, 15.10°C). There is plenty of rain from May to October and very little rain from November to April. A shade house was constructed using a special shade net for P. notoginseng. Light intensity in the shade house was collected every 10 s from 6:00 to 19:00 on a clear, cloudless day using a Li-1500 (Li-Cor, NE, United States) light quantum meter. FL intensity was measured simultaneously as a control. The light transmittance of the shade house was about 10% FL, which is a suitable light intensity for P. notoginseng growth. The soil was local raw soil (red soil). According to the method of Abedi-Koupai et al. (2006), the physical and chemical properties of the soil were analyzed: total nitrogen (N), 2.01 g kg–1, total phosphorus (P), 7.273 g kg–1, hydrolysable N, available P, 48.39 mg kg–1, available K, 1910.00 mg kg–1, pH, 6.42, and organic matter, 57.35 g kg–1.
Healthy 1-year-old P. notoginseng was disinfected with 0.1% potassium permanganate for 5 min. In January, the seedlings were planted in white pots contained with 10 kg disinfected raw soil, and there are three seedlings in each pot. Fertilization was conducted from April to July. Compound fertilizer, monopotassium phosphate, and potassium sulfate were applied with 0.4, 0.2, and 0.3 g/pot, respectively. During the growing period, insecticides (polyoxin, chlorothalonil) were sprayed to control the disease. In August (linear phase), P. notoginseng was used to determine the chlorophyll fluorescence and photosynthetic pigment content.
Chlorophyll Fluorescence
According to the method of Kramer et al. (2004), the chlorophyll fluorescence was measured using Dual-PAM-100 (Heinz Walz, Effeltrich, Germany). P. notoginseng was placed in an opaque incubator (MRC-1100E-LED, Gunning, Shanghai, China) for dark adaptation (more than 1 h), and the minimum fluorescence of PSII (Fo) and maximum fluorescence (Fm) were measured at 0 μmol m–2 s–1 light intensity. According to the previous studies, the light saturation point of P. notoginseng is 100–150 μmol m–2 s–1 (Chen et al., 2014). Thus, in the process of measuring the fluorescence induction curve, P. notoginseng was suddenly exposed to the simulated sunflecks for 30 min with the light intensity of 50 μmol m–2 s–1 (transient low light), 100 μmol m–2 s–1 (light saturation point), 400 μmol m–2 s–1 (transient moderate light), 800 μmol m–2 s–1 (transient high light), 1,600 μmol m–2 s–1 (transient high light), respectively, followed by the dark adaptation for 15 min, and the whole process lasted for 45 min.
Photosystem II chlorophyll minimum fluorescence (Fo′), maximum fluorescence (Fm′), and light-adapted steady-state fluorescence (Fs) after light adaptation were taken two times at 30 s intervals at the beginning, then at intervals of 1 min. Five replicates were randomly selected from each treatment for the analysis of chlorophyll fluorescence. The chlorophyll fluorescence parameters of PSII are calculated as follows (Genty et al., 1989; van Kooten and Snel, 1990; Demmig et al., 1996; Hendrickson et al., 2004). Maximum photochemical quantum efficiency of PSII under light (Fv′/Fm′) = (Fm′ − Fo′)/Fm; NPQ coefficient of PSII (NPQ) = (Fm − Fm′)/Fm′; photochemical quenching coefficient of PSII (qP) = (Fm′ − Fs)/(Fm′ − Fo′); effective quantum efficiency of PSII (ΦPSII) = Y(II) = (Fm′ − Fs)/Fm′; fluorescence and constitutive heat dissipation quantum efficiency of PSII (Φf,d) = Y(NO) = Fs/Fm; light-dependent heat dissipation quantum efficiency of PSII (ΦNPQ) = Y(NPQ) = Fs/Fm′ − Fs/Fm; electron transfer rate of photochemical quenching pathway of PSII (JPSII) = ΦPSII × PFD × 0.84 × 0.5; fluorescence and constitutive heat dissipation electron transfer rate of PSI (Jf,d) = Φf,d × PFD × 0.84 × 0.5; electron transfer rate of NPQ pathway of PSII (JNPQ) = ΦNPQ × PFD × 0.84 × 0.5; the electron transfer rate of PSII [ETR(II)] = Y(II) × PFD × 0.84 × 0.5.
Measurement of Light Absorption in Photosystem I
The light absorption of PSI was measured with reference to the measurement method of Huang et al. (2012b), and the redox state of P700 was measured using a Dual-PAM-100 measurement system (Heinz Walz, Effeltrich, Germany) with a dual wavelength (830/875 nm). After pre-illumination with far-red light, a saturating pulse of 10,000 μmol m–2 s–1 at 600 ms is applied and the signal of P700 is lowest when all of the P700 is in the reduced state. The determination of the highest P700 signal (Pm) is similar to that of the maximum chlorophyll fluorescence, except that 10 s of far-red light exposure was required before the determination of the highest P700 signal. PSI reaction center P700 maximum fluorescence (Pm′) was similar to Fm′, except that actinic light was used instead of far-red light. The calculation of PSI parameters was referred to the method of Miyake et al. (2005). Photochemical quantum yield of PSI [Y(I)] = (Pm′ − P)/Pm; donor terminal heat dissipation efficiency of PSI [Y(ND)] = 1 − P700red = P/Pm; receptor terminal heat dissipation efficiency of PSI [Y(NA)] = (Pm − Pm′)/Pm; the electron transfer rate of PSI [ETR(I)] = Y(I) × PFD × 0.84 × 0.5; cyclic electron transfer size of PSI (CEF) = JPSI – JPSII = ETR(I) – ETR(II).
Steady-State Gas Exchange Measurements
The steady-state gas exchange parameters were determined using the photosynthesis system (Li-6400XT, Li-Cor, NE, United States) with the 2 cm2 fluorescence leaf chamber. The gas exchange parameters of healthy 2-year-old P. notoginseng were measured from 9 to 11 a.m. on a sunny day, with five replicates per treatment (n = 5). The CO2 content in the chamber was maintained at 400 μmol mol–1 during the measurement of photosynthetic light response curves. The leaf was induced under the light intensity of 500 μmol m–2 s–1 (red light: blue light = 9:1) for 10 min. The automatic measurement procedure of the instrument was started after the data of all parameters were relatively stable. The gradient of light intensity was listed in the following order: 500, 300, 200, 150, 100, 80, 60, 40, 20, 0 μmol m–2 s–1. Induction is stabilized for 120–180 s at each light intensity, during which the gas exchange parameters are collected and the data are saved after the measurement. The relationship between the net photosynthetic rate (Pn) and photosynthetic photon flux density (PFD) was fitted, Pn = Pmax − PmaxC0e–α PFD/Pmax (Bassman and Zwier, 1991). In the formula, Pmax is the maximum net photosynthetic rate, α is the apparent quantum efficiency (AQY), and C0 is the index where the net photosynthetic rate level off to 0 under low light. According to the parameters in the formula, the following parameters can be calculated, respectively: dark respiration rate (Rd) = Pmax − PmaxC0; light compensation point (LCP) = Pmax ln(C0)/α; light saturation point (LSP) = Pmax ln(100C0)/α.
The light intensity in the chamber was maintained at 500 μmol m–2 s–1 and the leaf was induced under the CO2 intensity of 400 μmol mol–1 for 10 min. The gradient of reference cell CO2 concentration was set in the following order: 400, 300, 250, 200, 150, 100, 50, 400, 600, 800, 1,000, 1,200, 1,500 μmol mol–1. According to the method of Xu (2002), a linear regression was done for the points in the CO2 response curve where the internal leaf CO2 concentrations (Ci) were below 250 μmol mol–1 to obtain the carboxylation efficiency (CE), CO2 compensation point (Γ∗), and to calculate the rate of photorespiration (RL) = CE × I∗. The maximum carboxylation rate (Vcmax) and maximum electron transfer rate (Jmax) were calculated by using the method of Bernacchi et al. (2001).
Before measuring the light induction curve, P. notoginseng was moved into an opaque incubator (MRC-1100E-LED, Gunning, Shanghai, China) for dark adaptation, during which the plants were covered with a black bag. The CO2 concentration in the reference chamber was controlled to be 400 μmol mol–1. The leaves of P. notoginseng were put into the leaf chamber, and the light intensity in the leaf chamber was 0 μmol m–2 s–1. An automatic measurement was started after the data were stabilized. During measurement, the leaves were irradiated at 0 μmol m–2 s–1 light intensity for 2 min, and then induced with 400 μmol m–2 s–1 light intensity for 30 min. Data were collected every 30 s, and the gas exchange parameters were simultaneously collected.
According to the light response curve, the CO2 response curve, and light induction curve, the following relevant parameters can be obtained: net photosynthetic electron transfer rate (JCO2) = Pnet × 4; the residual absorbed energy used for the non-net carboxylative processes (JNC) = JPSII − JCO2 (Losciale et al., 2010). According to the method of Valentini et al. (1995), the rates of electron carboxylation (JC) and oxidation (JO) were calculated: JO = 2/3 × [JT – 4 × (Pn + Rd)], JC = 1/3 × [JT + 8 × (Pn + Rd)]; total photosynthetic electron flow through PSII (JT) = PFD × Y(II) × 0.84 × 0.5.
Determination of Photosynthetic Pigment Content
Panax notoginseng was placed in a dark incubator (MRC-1100E-LED, Gunning, Shanghai, China) for 12 h at a temperature of 25°C. After 12 h, P. notoginseng was placed under the light intensity of 50, 100, 400, 800, and 1,600 μmol m–2 s–1, respectively. The process lasted 90 min, with the first 30 min being light treatment and the last 60 min being dark treatment. The leaves were collected at 0, 5, 15, 30, 35, 45, 60, and 90 min, respectively, and the cleaned and dried leaves were wrapped in tin foil and quickly stored in liquid nitrogen. Afterward, the tin foil-wrapped leaves were stored in liquid nitrogen in a sealed tin box at −80°C in the refrigerator. To measure the photosynthetic pigment content, 0.2 g leaves were weighed in the dark condition, repeatedly extracted with cold acetone, and fixed to 10 ml. According to the method of Susan and Thayer (1990) and Zhao et al. (1995) with minor modifications, the content of V, anteraxantin (A), Z, lutein (L), and Lx were determined by high-performance liquid chromatography (HPLC) (Agilent 1260, CA, United States). Then, 1.2114 g Tris was dissolved in pure water and fixed into a 100 ml volumetric flask (0.1 mol L–1 Tris). 0.83 ml of 36–38% HCl solution was fixed into a 100 ml volumetric flask with pure water, (0.1 mol L–1 HCl). Further, 50 ml of 0.1 mol L–1 Tris solution and 40.3 ml of 0.1 mol L–1 HCl solution were mixed and the pH was adjusted to 7.5 with Tris mother liquor and HCl mother liquor to obtain 0.05 mol L–1 Tris–HCl buffer. Mobile phase A was prepared with acetonitrile and 0.05 mol L–1 Tris–Hc1 buffer at 70:3; mobile phase B was prepared with methanol and n-hexane at 5:1. The extracts were filtered through a 0.22 μm pore size membrane and sampled on a ZORBAX SB-C18 (5 μm, 4.6 mm × 250 mm) column with a column temperature of 25.0°C and a flow rate of 1 ml min–1 at a detection wavelength of 445 nm. The standards were purchased from Sigma and ChromaDex (CA, United States) with a purity of >98%.
Statistical Analysis
The data were counted using Microsoft Excel 2007 software (Microsoft Corp., WA, United States). The experimental site was divided into 15 plots, and 12 pots were placed per plot. We obtained five replicates (five healthy plants) randomly selected from each plot for the analysis of photosynthetic parameters. Also, the results were displayed as mean values of five independent plants. One-way ANOVA was performed using SPSS 19.0 software (IBM Corp., NY, United States), and Duncan’s test was applied for multiple comparisons of significant differences (P < 0.05), and the graphical data were presented as mean ± SE. GraphPad Prism 8.3.0 (CA, United States) software was used for graphing.
Results
Photosystem II Activity in Response to Simulated Sunflecks
The leaf exhibited a significant difference in PSII activity in response to the simulated sunflecks (Figure 1). The Fv′/Fm′ decreased rapidly at different intensities of transient light. In the process of dark recovery, the individuals exposed to transient light (50 and 400 μmol m–2 s–1) recovered to the higher levels with respect to Fv′/Fm′, and the others recovered to lower level. These results indicated that the transient high light leaded to lower PSII activity.
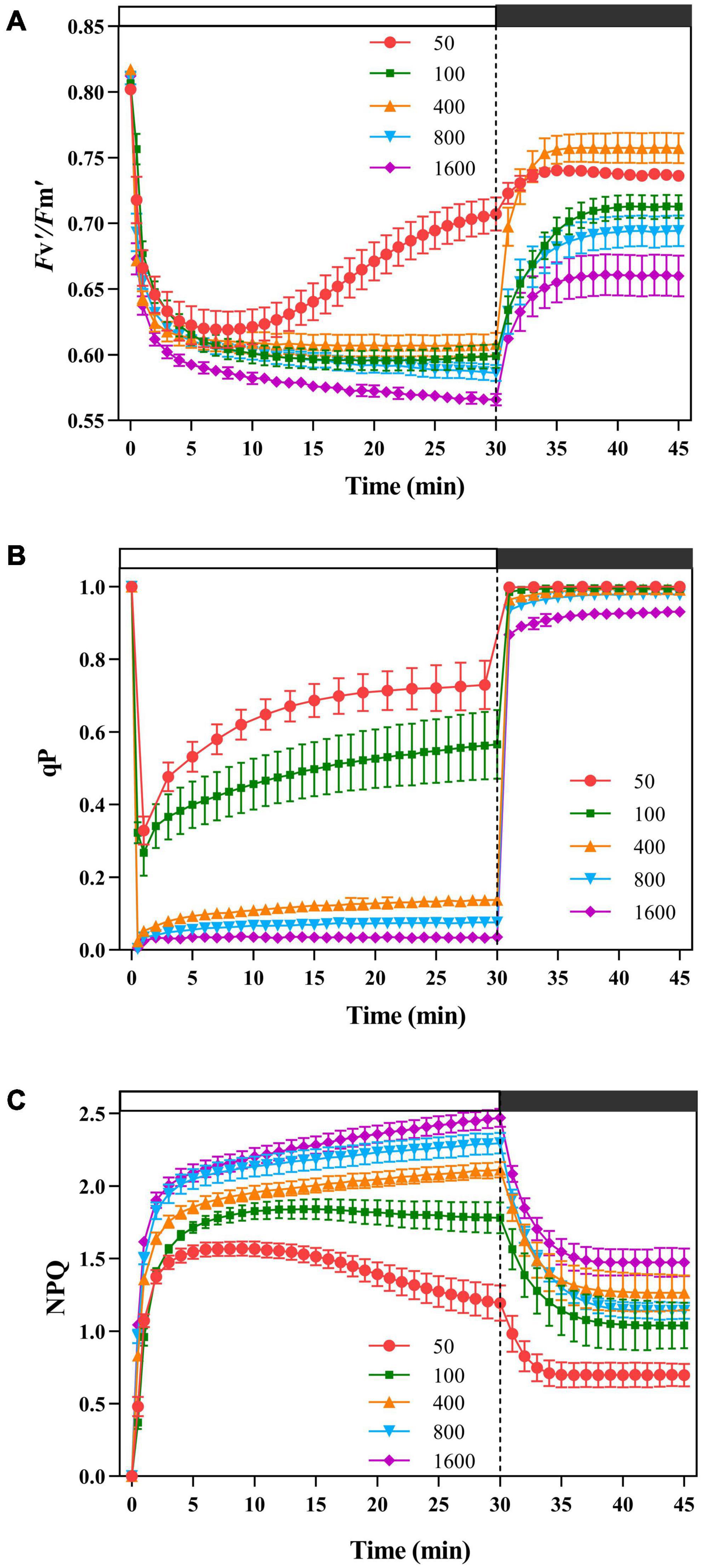
Figure 1. The effects of transient light on the maximum photochemical quantum efficiency of photosystem II (PSII) under light (Fv′/Fm′), photochemical quenching (qP), and non-photochemical quenching (NPQ). The simulated sunflecks of 50 (red), 100 (green), 400 (orange), 800 (blue), and 1,600 μmol m–2 s–1 (purple) was conducted for 30 min, respectively (the left of the dotted line), and then dark recovery was conducted for 15 min (the right of the dotted line). The Fv′/Fm′ (A), qP (B), and NPQ(C) were recorded every minute, the value is the means ± SE (n = 5).
Energy Dissipation Through Non-photochemical Quenching in Response to Transient High Light
Non-photochemical quenching responded quickly and increased rapidly at different transient light (Figure 1C). The plants exposed to the transient low light (50 μmol m–2 s–1) contributed most of the energy dissipation to the qP pathway (Figure 1B). When the plants were exposed to the transient light (below 400 μmol m–2 s–1), the plants dissipated more energy through NPQ in the early stages (Figure 1C) and increased their ability to dissipate energy through photochemical quenching in the later stages (Figure 1C), while at 800 and 1,600 μmol m–2 s–1 transient light, the energy dissipation through NPQ was dominant (Figure 1C). During the dark recovery period, qP recovered rapidly at all transient light, with no significant differences between the transient light; while NPQ remained at a high level at transient high light (400, 800, and 1,600 μmol m–2 s–1) (Figure 1).
Heat Dissipation in Response to Transient High Light
The plants were exposed to 50 μmol m–2 s–1 transient low light, the light energy received by PSII was mainly allocated to the photochemical reaction pathway (Figure 2A), while the others dissipated light energy via NPQ pathway (Figure 2C) and the fluorescence dissipation pathway (Figure 2B). When P. notoginseng was transformed from a dark environment to transient light, ΦPSII decreases with the increase of transient light (Figure 2A). As the simulated sunfleck proceeded, ΦPSII continuously dropped and ΦNPQ slowly increased under the transient light intensity of 400, 800, and 1,600 μmol m–2 s–1 (Figures 2A,C), indicating a slow reopening of available reaction centers. Φf,d at the simulated sunflecks of 400, 800, and 1,600 μmol m–2 s–1 was approximately higher than that at 100 μmol m–2 s–1. Furthermore, when P. notoginseng was switched from the simulated sunflecks to a dark environment, with the increase of transient light, the extent of recovery in ΦPSII decreased (Figure 2A).
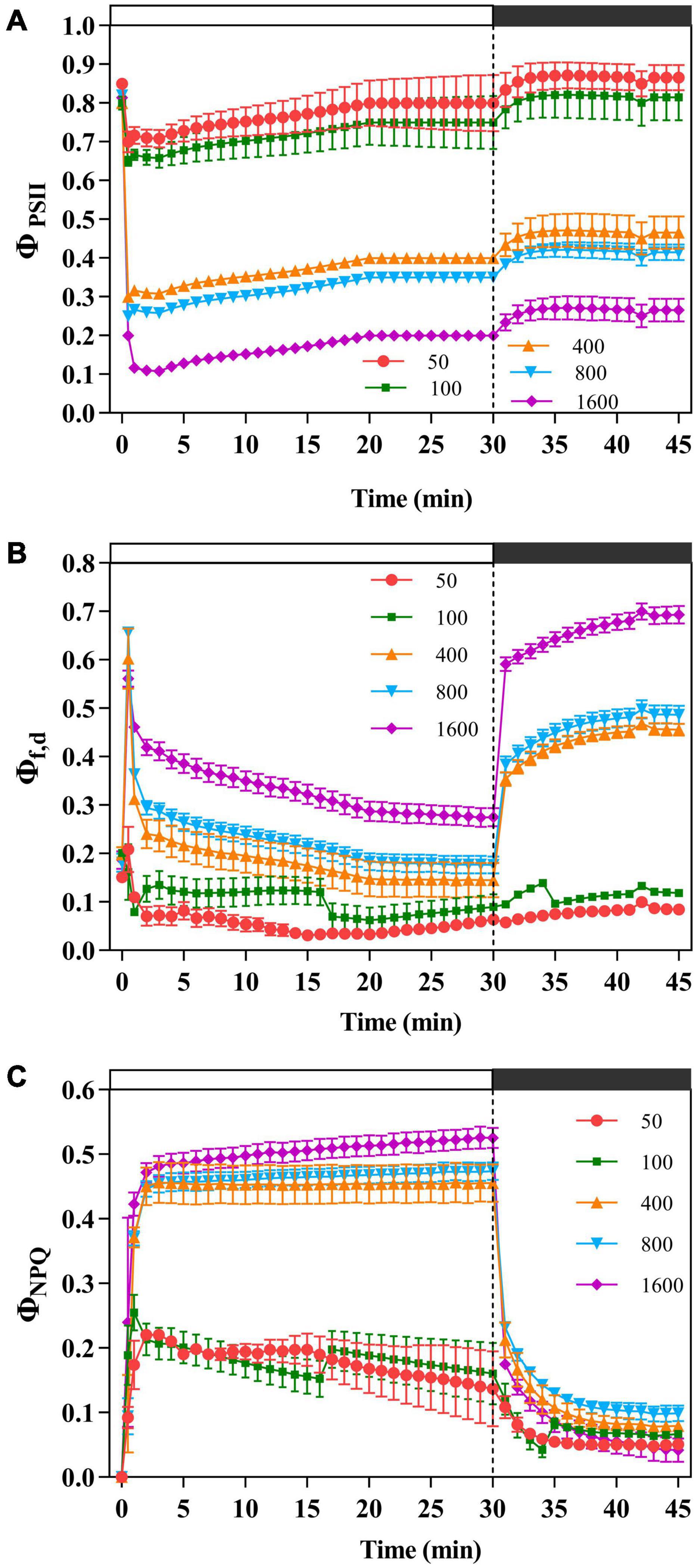
Figure 2. The effects of transient light on the effective photochemical quantum yield of PSII (ΦPSII), fluorescence and constitutive heat dissipation quantum efficiency of PSII (Φf,d), and light-dependent heat dissipation quantum efficiency of PSII (ΦNPQ). The Panax notoginseng was induced for 30 min in the simulated dynamic light of 50, 100, 400, 800, and 1,600 μmol m–2 s–1, respectively (the left of the dotted line), and then dark recovery was conducted for 15 min (the right of the dotted line). ΦPSII (A), Φf,d (B), and ΦNPQ (C) were recorded every minute, the value is the means ± SE (n = 5).
The Oxidation State of Photosystem I (P700+)
The light energy received by the PSI is mainly allocated to the PSI acceptor side for thermal dissipation [Y(NA)], except for the transient light of 50 and 100 μmol m–2 s–1, while the thermal dissipation at the PSI donor side [Y(ND)] is low, and the effective quantum efficiency of the PSI [Y(I)] decreases with the increase of transient light (Figure 3). When P. notoginseng was suddenly exposed to the simulated sunflecks, Y(ND) increased rapidly; while during darkness recovery, Y(ND) was close to the initial level (Figure 3B). Meanwhile, Y(I) decreased rapidly and then increased at transient light of 50, 100, and 400 μmol m–2 s–1 (Figure 3A). In contrast, Y(I) declined at transient light of 800, and 1,600 μmol m–2 s–1, and Y(I) rose rapidly during darkness recovery (Figure 3A).
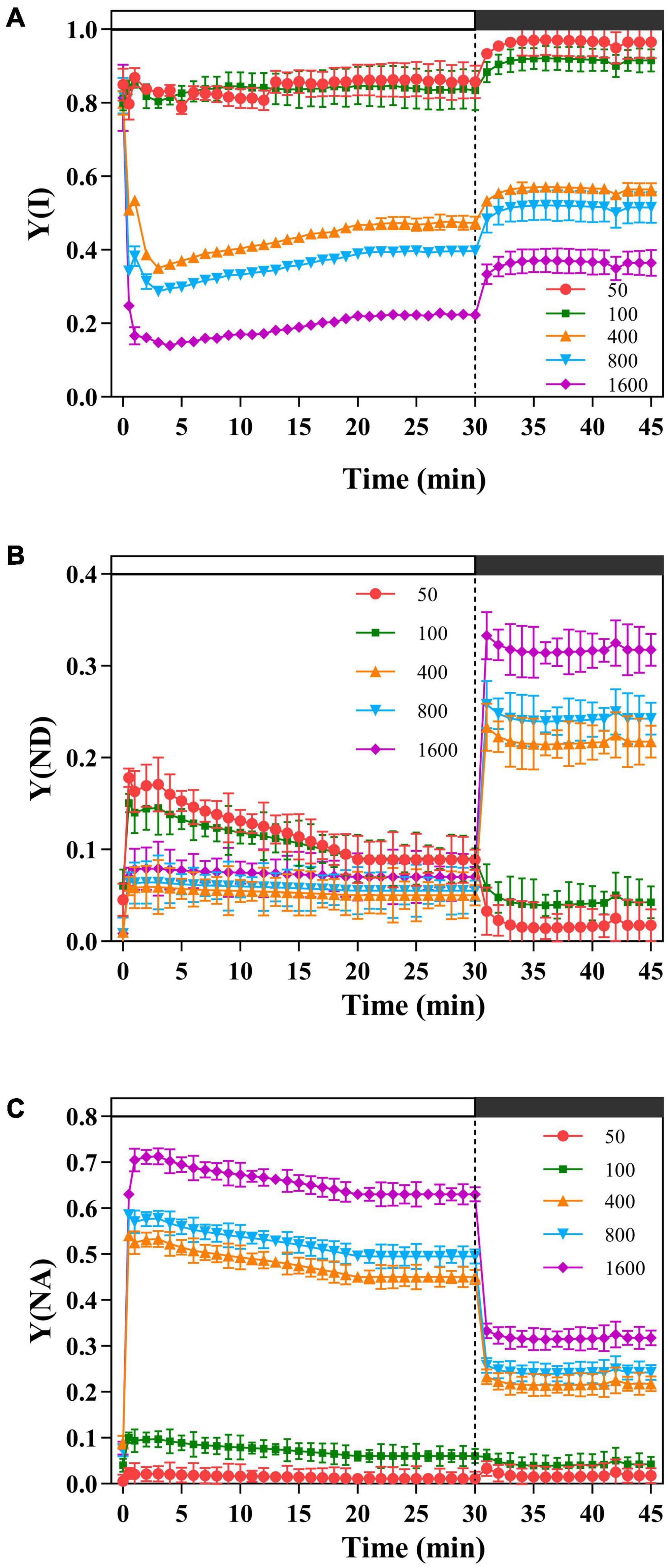
Figure 3. The effects of transient light on the quantum yield of photosystem I (PSI) [Y(I)], the donor-side limitation of PSI [Y(ND)], and the acceptor-side limitation of PSI [Y(NA)]. The P. notoginseng was induced for 30 min in the simulated dynamic light of 50, 100, 400, 800, and 1,600 μmol m–2 s–1, respectively (the left of the dotted line), and then dark recovery was conducted for 15 min (the right of the dotted line). [Y(I)] (A), [Y(ND)] (B), and [Y(NA)] (C) were recorded every min, the value is the means ± SE (n = 5).
Cyclic Electron Flow and Electron Low Devoted to RuBP Oxygenation
Electron transfer rate II [ETR(II)] increased rapidly when plants were exposed to transient high light (Figure 4B). The ETR(I) is at a low level at t transient light of 50 μmol m–2 s–1 (Figure 4A). The change of CEF was similar to that of ETR(I). In a short time, the transient high light excited high CEF to protect the photosystem from damage (Figure 4C). When plants in darkness were suddenly exposed to transient light, chlorophyll fluorescence rapidly reached a maximum in a short period of time and gradually stabilized over time (Figure 4D). The photochemical light was turned off, transient light resulted in different cyclic electron transfer activities. The cyclic electron transfer activity was 50 μmol m–2 s–1 (0.0027) > 1,600 μmol m–2 s–1 (0.0024) > 800 μmol m–2 s–1 (0.0023) > 400 μmol m–2 s–1 (0.0021) > 100 μmol m–2 s–1 (0.0014), suggesting that the high cyclic electron transfer activity plays an important role in photoprotection.
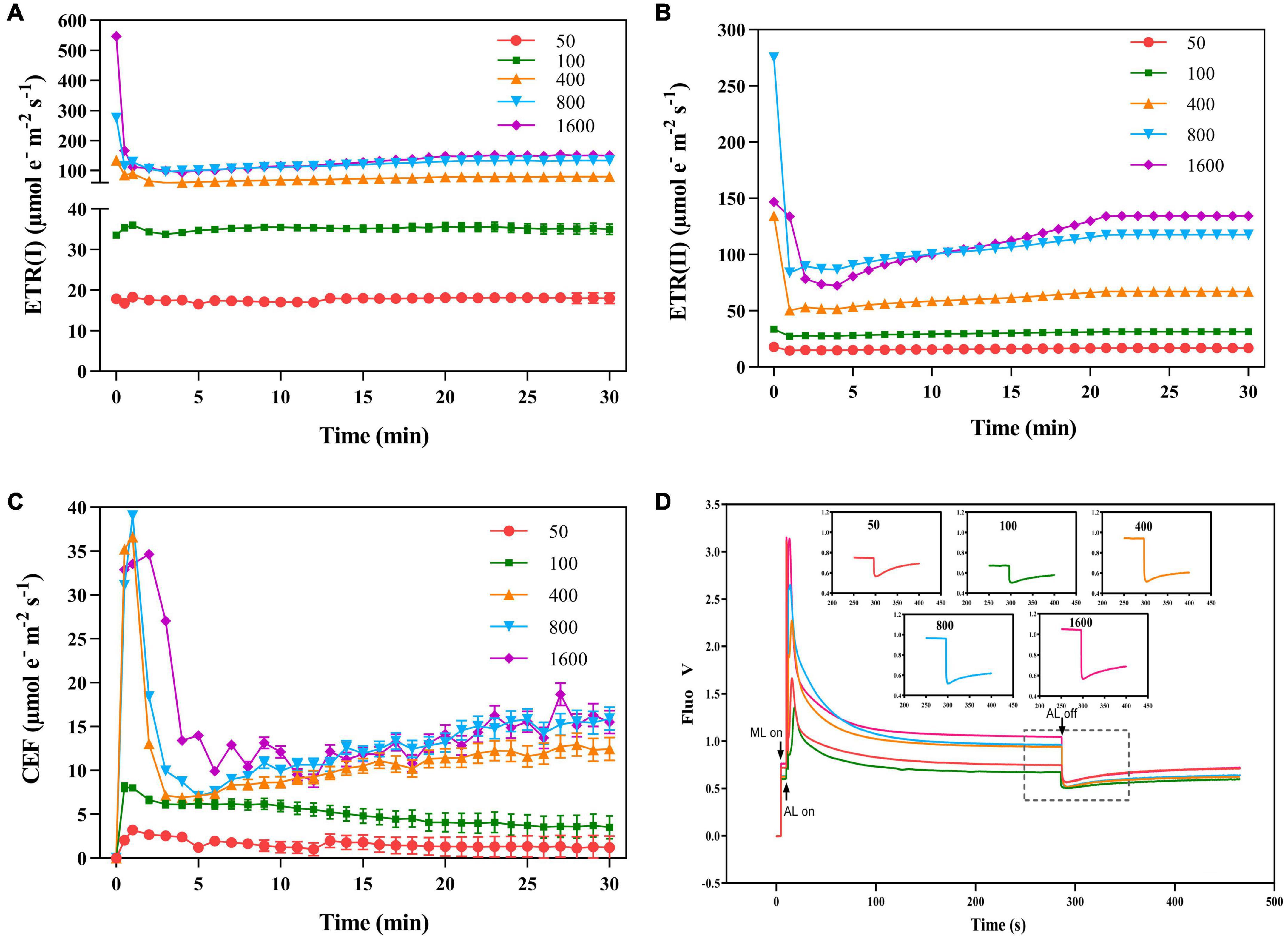
Figure 4. The effects of transient light on the electron transport rate of PS I [ETR(I)], electron transport rate of PSII [ETR(II)], and cyclic electron flow of PSI (CEF). The P. notoginseng was induced for 30 min in the simulated dynamic light of 50, 100, 400, 800, and 1,600 μmol m–2 s–1, respectively (the left of the dotted line), and then dark recovery was conducted for 15 min (the right of the dotted line). [ETR(I)] (A), [ETR(II)] (B), and (CEF) (C) were recorded every min, the value is the means ± SE (n = 5). (D) Effects of transient light on post-illumination. Vertical bars indicate the timing of on (upward) and off (downward) points of measuring light (ML), actinic light (AL) of 50 μmol m–2 s–1, and a saturating light flash (SF, 12,000 μmol m–2 s–1, 800 ms), respectively. The insets are magnified traces from the boxed area of the transient increase in chlorophyll fluorescence due to NDH activity on different light intensities for 280 s.
The value of JPSII increased rapidly when P. notoginseng was suddenly exposed to transient light (Figure 5A). A larger value of JPSII was exhibited at transient light of 1,600 μmol m–2 s–1 and a smaller value of JPSII was exhibited at transient low light (Figure 5A). There was a significant difference in JNPQ under the different levels of transient light (Figure 5C), indicating that the electron transfer rate through the NPQ of PSII is mainly influenced by the light intensity. The plants were suddenly exposed to transient light, Jf,d rises for a short time and falls over time. The value of Jf,d was increased with the intensity of the simulated sunflecks as presented by the elevation of Φf,d at the simulated sunflecks of 400, 800, and 1,600 μmol m–2 s–1 (Figures 2B, 5B). Moreover, the maximum values of JCO2 and JC were generally recorded in the transient low light (Figures 5D,F). At the high light intensity of sunflecks, a decrease in the electron flow devoted to Ribulose-1, 5-bisphosphate (RuBP) carboxylation indicated the reduction in the incident chloroplast CO2 concentration (Figure 5F). Electron flow was largely devoted to RuBP oxygenation in response to the high light intensity of sunflecks (Figure 5E). Based on the correlation between CEF and electron flow devoted to RuBP oxygenation (JO), the electron flow that was largely devoted to RuBP oxygenation would contribute to the operation of the CEF (Figure 5H).
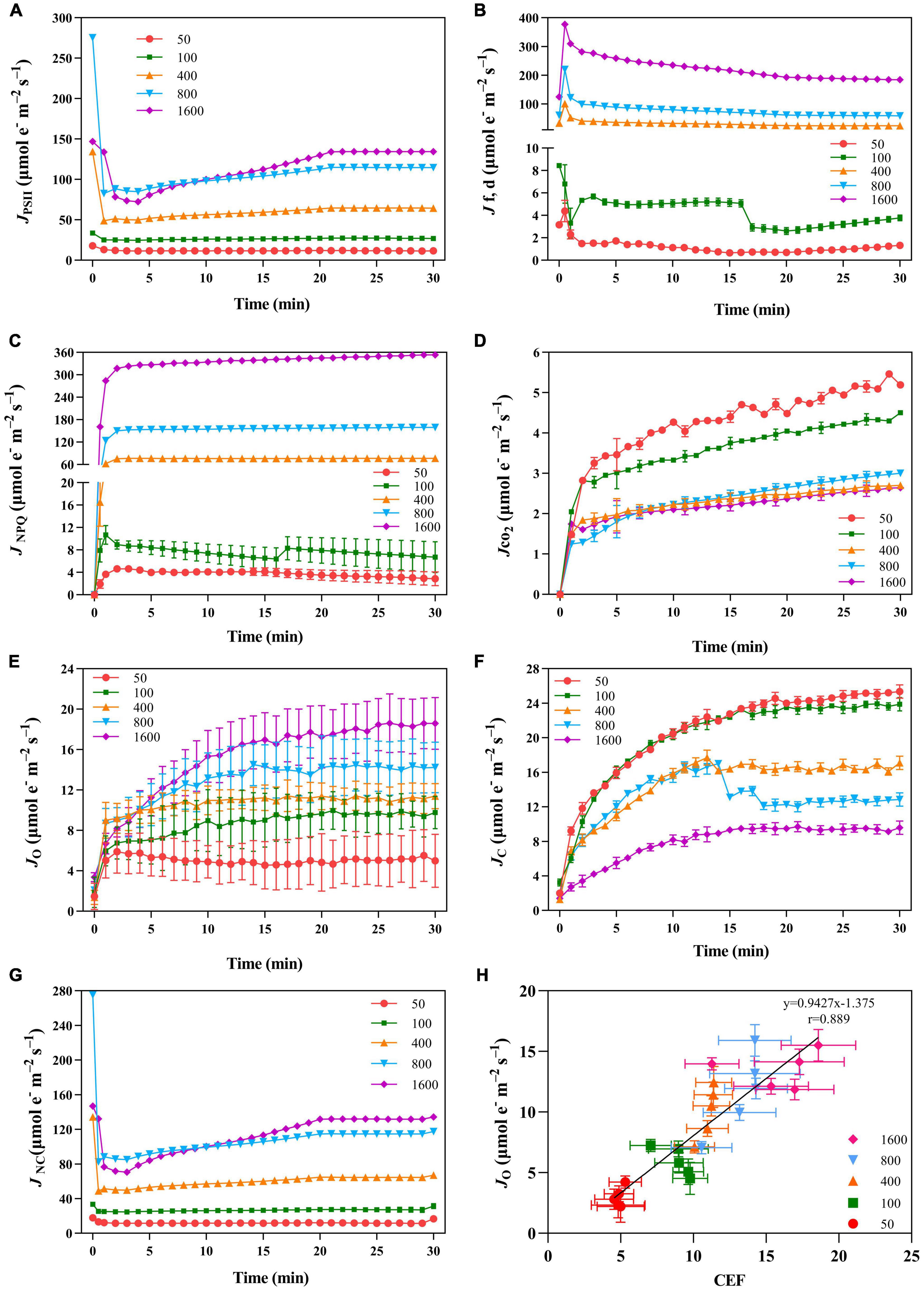
Figure 5. The effects of transient light on PSII photochemical quenching pathway ETR (JPSII, A), PSII fluorescence and constitutive heat dissipation ETR (Jf,d, B), PSII non-photochemical quenching electron transfer rate (JNPQ, C), net photosynthetic electron transport rate (JCO2, D), rate of electron transport for oxidation reaction (JO, E), carboxylation reaction (JC, F), and the residual absorbed energy used for the non-net carboxylative processes (JNC, G). (H) Correlation between CEF and JO under different transient light intensities. JPSII, JNPQ, Jf,d, JCO2, JO, and Jc were recorded every minute, the value is the means ± SE (n = 5).
Non-photochemical Quenching Attached to Xanthophyll Cycle
The xanthophylls de-epoxidation state (Z + A)/(V + A + Z) was higher in the plants exposed to transient high light (Figure 6A). The NPQ and V de-epoxidation state also showed a significant linear positive correlation (Figure 6B). On the other hand, under the transient high light conditions, the amount of Lx de-epoxidized to L was relatively reduced (Figure 6D), rapid engagement of Lx content after the low intensity of sunfleck contributes together with the lower NPQ to an elevation in the maximum photochemical quantum efficiency of PSII under light (Figures 1A,C, 6C).
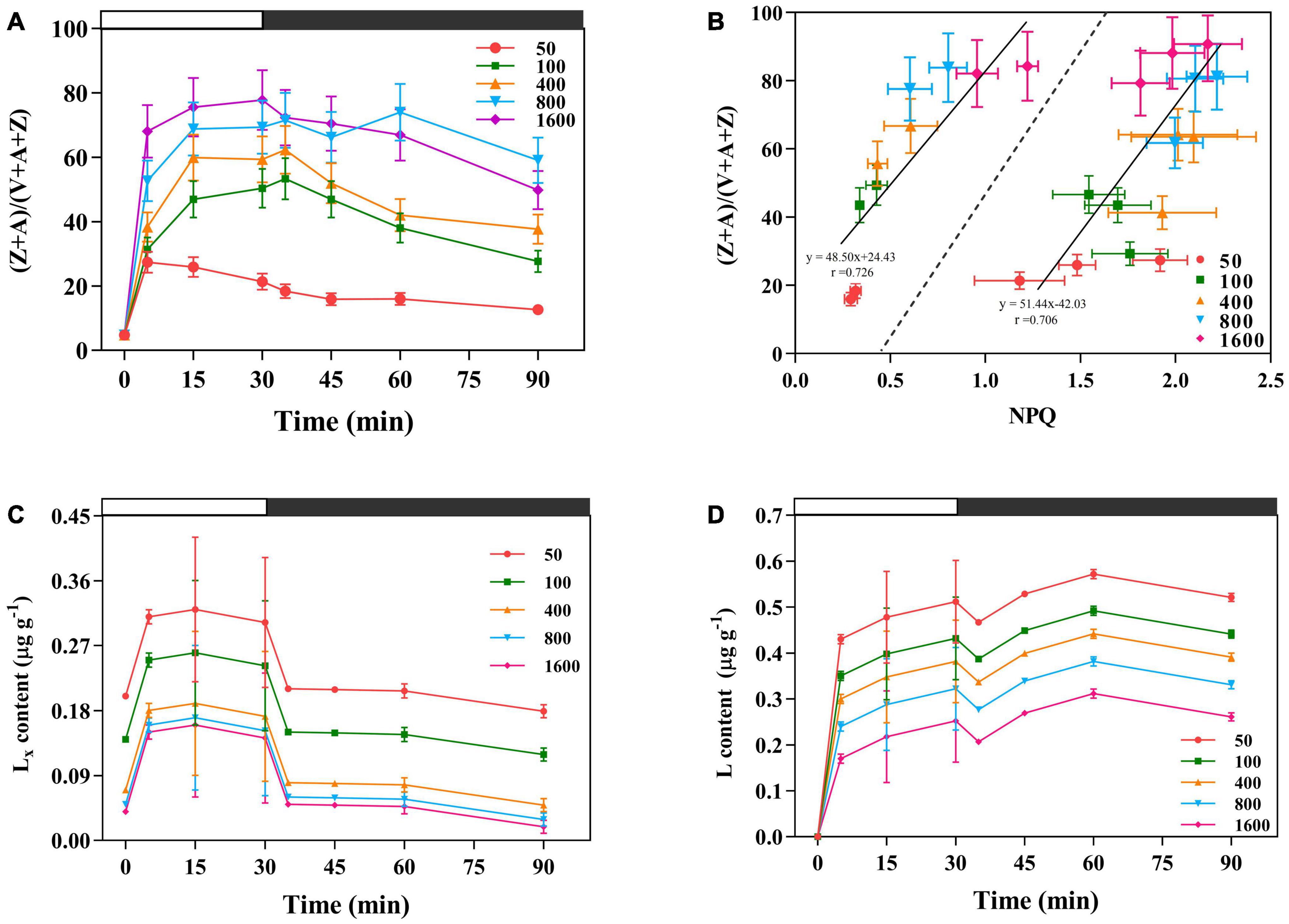
Figure 6. The effects of transient light on xanthophylls de-epoxidation state xanthophyll cycle (V cycle) (A). V is violaxanthin, A is anteraxantin, Z is zeaxanthin. (B) Correlation between NPQ and V cycle under different transient light intensities. (Z + A)/(V + A + Z) is the xanthophylls de-epoxidation state. The data to the left of the dashed line are the NPQ and (Z + A)/(V + A + Z) obtained at different transient light intensities for 35 and 45 min, respectively. The data to the right of the dashed line are the NPQ and (Z + A)/(V + A + Z) obtained at different transient light intensities for 5, 15, and 30 min, respectively. (C,D) The effects of transient light on the lutein epoxide (Lx) cycle. Lx: lutein epoxide, L: lutein (L), the amount of Lx de-epoxidized to L. The dark-adapted lutein level (time 0) was subtracted from the lutein content measured during the experiment. The value is the means ± SE (n = 5).
Non-photochemical Quenching in Response to Simulated Fluctuating Light
In the simulated sunflecks environment with alternating fluctuations between 4 min low light (50 μmol m–2 s–1) and 3 min high light (100, 400, 800, and 1,600 μmol m–2⋅s–1), Fv′/Fm′ and ΦPSII gradually increased with the extension of alternating time under the light intensity of 100 μmol m–2 s–1 (Figures 7A,B). Fv′/Fm′ in high light (800 and 1,600 μmol m–2 s–1) did not change significantly in the alternation of fluctuating light, but Fv′/Fm′ in low light gradually increased with the alternation of fluctuating light ΦPSII, indicating that the fluctuating light has an important effect on the recovery of PSII activity in the plants. Moreover, during alternating fluctuating light, ΦPSII at the light intensities of 400, 800, and 1,600 μmol m–2 s–1 was at a low level. The increase in ΦPSII becomes greater when the plants were transferring from high light (800 and 1,600 μmol m–2 s–1) to low light (50 μmol m–2 s–1), suggesting that in the process of alternating fluctuations, ΦPSII was inhibited under high light, and ΦPSII is recovered and electron-replenished under low light.
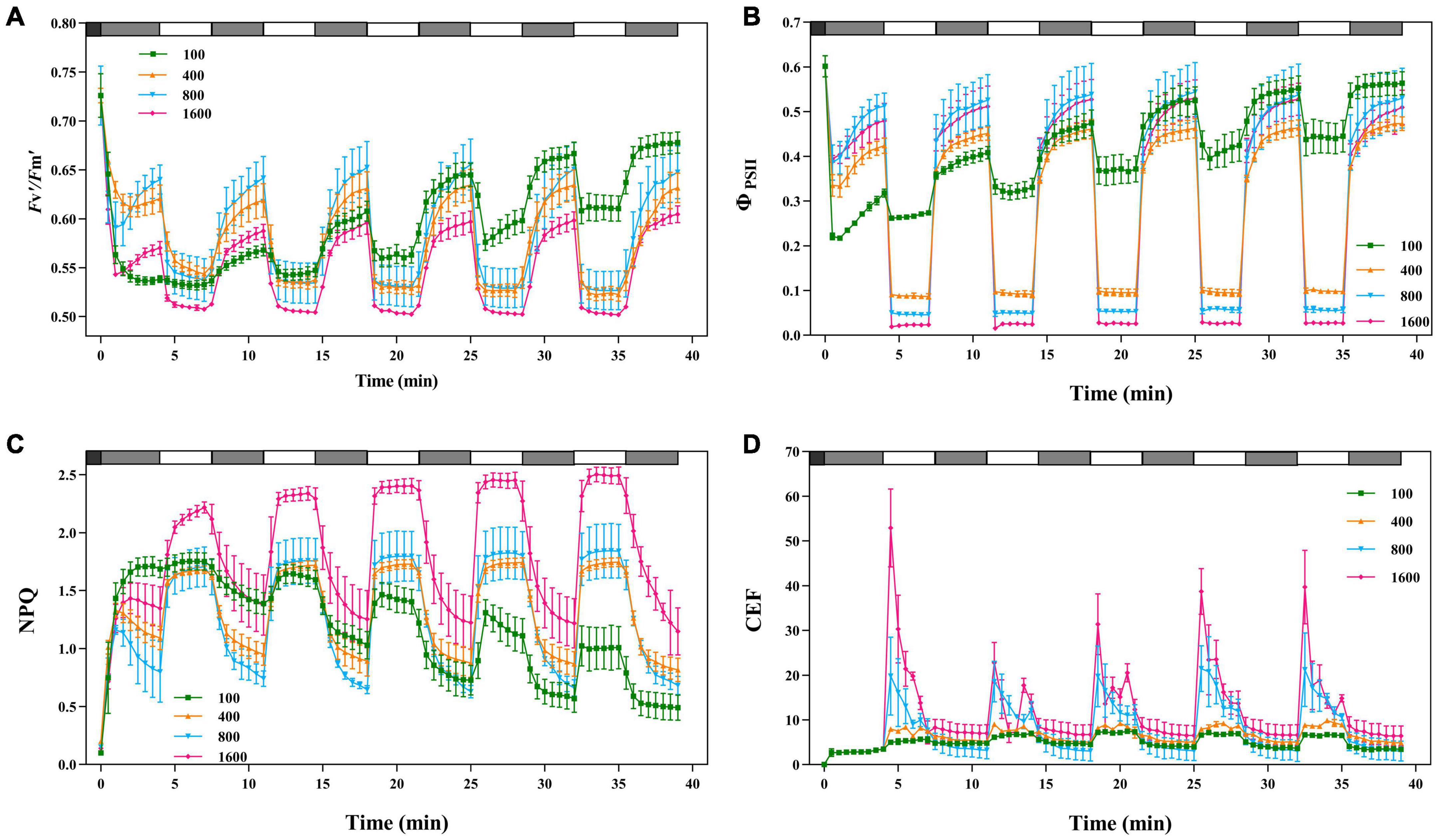
Figure 7. The effects of fluctuating light on Fv′/Fm′, ΦPSII, NPQ, and CEF. The black bars indicate dark, the gray bars indicate low light (50 μmol m–2 s–1) for 4 min and the white bars indicate transient light (100, 400, 800, and 1,600 μmol m–2 s–1) for 3 min. Fv′/Fm′ (A), ΦPSII (B), NPQ(C), and CEF (D) were recorded every 30 s, the value is the means ± SE (n = 5).
When P. notoginseng in darkness was transferred to 4 min of low light (50 μmol m–2 s–1), the NPQ gradually decreased, followed by a rapid increase when P. notoginseng was exposed to high light (800 and 1,600 μmol m–2 s–1; Figure 7C). As the alternating fluctuating light continued, NPQ was greater at light intensities of 400, 800, and 1,600 μmol m–2 s–1, and NPQ was smaller at light intensities of 100 μmol m–2 s–1. NPQ had “memory” in response to the simulated fluctuating light. When the plants were switched from darkness to 4 min of low light (50 μmol m–2 s–1), CEF was activated (Figure 7D). The CEF was greatly excited when P. notoginseng was exposed to high light (800 and 1,600 μmol m–2 s–1), but gradually decreased over time. CEF at light intensities of 100 and 400 μmol m–2 s–1 increased over time.
Discussion
Light is the ultimate resource for photosynthesis, and its intensity and spectral composition considerably vary in nature. These traits compel plants to maintain the balance between light absorption needed for photosynthesis and excess light dissipation for photo-protection (Wagner et al., 2006). Excess light may adversely affect the plants. If the photochemical capacity exceeds the input energy, the balance of energy absorption/utilization cannot be achieved (Jin et al., 2016; Crisp et al., 2017). This process leads to photo-inhibition and/or irreversible photo-oxidative damage due to the presence of ROS (Krieger-Liszkay et al., 2008; Szymańska et al., 2017). The plants evolve various mechanisms to adjust their response to dynamic sunflecks. Some mechanisms occur very quickly, such as NPQ (Carraretto et al., 2016) and cycle electron flow (Yamori et al., 2016; Kono et al., 2017, 2019).
Energy Dissipation Through Non-photochemical Quenching Was Dominant in Response to Transient High Light
Non-photochemical quenching responds very rapidly to the sunflecks, which have been documented in the tropical rainforest plants (Maxwell and Johnson, 2000; Tausz et al., 2005). The plants were exposed to the simulated sunflecks, NPQ increased rapidly to the maximum (Figure 1C). Moreover, in response to the simulated sunflecks, there was also a rapid increase in ΦNPQ (Figure 2C), while the Fv′/Fm′ declined (Figure 1A), confirming the previous studies (Tausz et al., 2005). Meanwhile, the plants exposed to the transient high light appeared to increase more rapidly and largely in NPQ than the ones exposed to the transient low light (Figure 1C), suggesting the importance of the NPQ upon exposure to the transient high light. Energy quenching (qE) characterized as a dominant part of thermal dissipation is caused by the excess light-induced proton gradients (ΔpH) across the vesicle-like membrane (Müller et al., 2001; Cazzaniga et al., 2013), and PSII subunit S (PsbS) is involved in this quenching process (Niyogi et al., 2005; Pawlak et al., 2020). However, drastic changes in the qE process are induced depending on the amount of PsbS (Johnson and Ruban, 2010, 2011). This suggests that PsbS may result in the acceleration of NPQ induction to adapt to transient light. On the other hand, Fv′/Fm′ and qP increased significantly while NPQ diminished after the light-to-dark transition (Figure 1), however, Fv′/Fm′ was difficult to return to the initial state with the increase of transient light intensity, indicating an impaired PSII (Figure 1A). A possible explanation is that the relaxation rate of NPQ lags the induction rate and is exacerbated by prolonged exposure to the excessive light conditions (Pérez-Bueno et al., 2008) so that NPQ inhibits photosynthetic quantum yield after the light-to-dark transition (Murchie and Niyogi, 2011). Interestingly, the recovery of Fv′/Fm′ was more pronounced at transient light of 400 μmol m–2 s–1 than at transient light intensity of 100 μmol m–2 s–1, which may be due to the higher CEF activity of the PSI at transient moderate light, where light energy mediates a balance between the photo-protection and light energy utilization (Figures 1A, 4D; Sato et al., 2014; Kromdijk et al., 2016).
The Greater Increase in Cyclic Electron Flow Activity in Responses to Transient Low Light May Accommodate the Electron Flows
The value of Φf,d at the simulated sunflecks of 400, 800, and 1,600 μmol m–2 s–1 was higher than that at 100 μmol m–2 s–1 (Figure 2B). A photo-damage was observed in A. thaliana exposed to transient high light (Li et al., 2002; Ikeuchi et al., 2014). Thus, the relatively high Φf,d in plants exposed to the transient high light (400, 800, and 1,600 μmol m–2 s–1) can be explained by a relatively high photo-damage as estimated from the low ΦPSII after dark recovery for 15 min (Figures 2A,B). The photo-damage rate is positively correlated with light intensity (Allakhverdiev and Murata, 2004), and the repair rate of the PSII depends on the intensity of the incident light, but it is maximized at relatively low light intensities (Takahashi and Murata, 2008). This interpretation was verified in the present study that the plants exposed to the simulated sunflecks of 50 μmol m–2 s–1 have been shown to lead to significant elevation in ΦPSII after darkness recovery, indicating a healthy PSII. A similar result was also shown when Haberlea rhodopensis was subjected to weak light (Durgud et al., 2018). When P. notoginseng was exposed to the simulated sunflecks close to the light saturation point, the light energy absorbed by the plants coincided with the need for photosynthesis, so that the value of ΦPSII significantly increased after darkness recovery (Figure 2A). The photochemical quantum yield of PSI [Y(I)] decreased more drastically after the start of simulated sunflecks of 400, 800, and 1,600 μmol m–2 s–1 (Figure 3A), it should be noted that this was the result of transient high light. This was also supported by the fact that the impairment on PSI was presented in A. thaliana exposed to high light (Munekage et al., 2002, 2008). In this study, the simulated sunflecks of 400, 800, and 1,600 μmol m–2 s–1 increased Y(NA), while Y(ND) was decreased in P. notoginseng (Figures 3B,C), this indicated that when being exposed to transient high light, the acceptor-side reactions restrict PSI activity. When the plants are suddenly exposed to the transient light above their light saturation point, the acidification of the thylakoid lumen is not able to sufficiently downregulate the linear electron flow, and the relaxed state of the thylakoid causes an electron rush to PSI, which leads to a reduction in the electron acceptors, oxygen photoreduction, and ROS formation (Tikkanen et al., 2010; Kono et al., 2014; Wang et al., 2015). By contrast, Y(NA) of PSI is decreased while Y(ND) is increased in P. notoginseng exposed to the simulated sunflecks of 50 μmol m–2 s–1 (Figures 3B,C), suggesting that the enhanced oxidation state of PSI (P700+) is also a mechanism to ensure the integrity of PSI. PSI photo-inhibition with large differences between the transient high light and transient low light can be explained by the photosynthetic alternative electron flows interacting with PSI. The greater increase in CEF activity in responses to the transient low light may accommodate the electron flows, consequently avoiding the response of photo-oxidative damage (Figure 4D; Kono et al., 2014).
Non-photochemical Quenching Coupled to the De-epoxidation in the V Cycle Might Attribute to Energy Dissipation in Response to Transient High Light
Photosystem II fluorescence and constitutive heat dissipation electron transfer rate (Jf,d) was increased with the intensity of the simulated sunflecks as presented by the elevation of Φf,d at simulated sunflecks of 400, 800, and 1,600 μmol m–2 s–1 (Figures 2B, 5B). This increased thermal and fluorescence dissipation at the high intensity of the simulated sunflecks may be the result of an imbalance in the exciton-radical pairs and relatively rapid electron transfer from the pheophytin to the primary quinone acceptor (Losciale et al., 2008). The residual absorbed energy used for non-net carboxylative processes (JNC) predominated at the simulated sunflecks of 1,600 μmol m–2 s–1 (Figure 5G). Even though JNC usually confers more potentially detrimental effects than NPQ because of the generation of ROS during non-net carboxylative processes, these mechanisms are preferentially activated when the response of JNPQ to high light is elevated (Losciale et al., 2008; Ishida et al., 2014). The trans-thylakoid pH-dependent V cycle is the main mechanism driving the heat dissipation operation, with a lumen pH (5.7–7.5) enabling optimal V de-epoxidase activity (Takizawa et al., 2007). The present study clearly showed that the simulated sunflecks of 50 μmol m–2 s–1 suppressed the (Z + A)/(V + A + Z) (Figure 6A), this could be explained by the fact that the acidity of thylakoid lumen does not activate the violaxanthin de-epoxidase (VDE) sufficiently at low intensity of sunflecks, so the plants dissipate the excess energy by alternative ways. It has been reported that the cyclic transport around PSI can create an optimal pH for VDE by elevating the trans-thylakoid pH (Cruz et al., 2005), CEF was increased in the plants exposed to the transient high light (400, 800, and 1,600 μmol m–2 s–1) (Figure 4C), the optimum lumen pH may activate the V cycle and subsequently the JNPQ begins to increase (Figures 5C, 6).
Lx Cycle Exists in the Typically Shade-Tolerant Species in Response to Transient Low Light
The operation of the Lx cycle is an additional mechanism to regulate light harvesting by antenna complex, allowing leaves with high (Z + A)/(V + A + Z) to exhibit rapid and enhanced NPQ (Matsubara et al., 2008; Esteban et al., 2010). Under the transient high light conditions, the amount of Lx de-epoxidized to L was relatively reduced (Figure 6D). A similar result was found in the earlier studies, where shaded young avocado leaves showed a decreasing trend in the total L pool after sudden exposure to sunlight, despite the deep oxidation of Lx, which may be related to photo-oxidation of L (Förster et al., 2009, 2011). The slow operation of the Lx cycle in some species may “lock in” photoprotection (Matsubara et al., 2005; Förster et al., 2011). The sudden exposure of P. notoginseng to transient high light was accompanied by a slow operation of the Lx cycle, therefore, based on the correlation between NPQ and the degree of de-epoxidation in the V cycle, the de-epoxidation in the V cycle might primarily attribute to energy dissipation (Figure 6B; Esteban et al., 2007; García-Plazaola et al., 2007). In particular, Lx plays a central role in light-harvesting in response to low light. Recombinant Lhcb5 reconstituted with Lx has a high fluorescence yield (Matsubara et al., 2007). Reduced Lx content results in a slower reduction in PSII electron acceptor and rapid formation of NPQ (García-Plazaola et al., 2012). In the present study, a rapid engagement of Lx content after the low intensity of sunfleck contributes together with the lower NPQ to an elevation in the maximum photochemical quantum efficiency of PSII under light (Figures 1A,C, 6C). The present study combined with the results of previous studies suggests that the presence of Lx in the transient low light may improve the maximum photochemical quantum efficiency of PSII under the light.
Photorespiration Plays a Role in Regulating the Cyclic Electron Flow in Response to Transient High Light
The regulation of photosynthetic electron flow in A. thaliana under the simulated sunflecks has been preliminarily investigated by previous work on CEF and O2-dependent alternative electron sinks (Kono et al., 2014; Allahverdiyeva et al., 2015; Tiwari et al., 2016). The adaptation of PSI to the sunflecks is mainly due to CEF, while photorespiration in Arabidopsis leaves contributes little to the reduction of photo-damage under the low intensity of sunflecks (Suorsa et al., 2012; Kono et al., 2014). The previous study with N. tabacum has demonstrated that the light intensity facilitates the capacity of the photorespiratory pathway, and high-light-grown leaves regulate the CO2 uptake and photosynthetic electron flow through the active photorespiratory pathway (Huang et al., 2014, 2015a). However, the importance of photorespiration exerted by the shade-growth plants suddenly exposed to sunflecks is unclear. In the present study, at the high light intensity of sunflecks, a decrease in the electron flow devoted to RuBP carboxylation indicated the reduction in the incident chloroplast CO2 concentration (Figure 5F). A. thaliana produces a photorespiratory response when moving from an environment with high CO2 concentration to ambient air, due to the reduced availability of CO2 (Foyer et al., 2012; Eisenhut et al., 2017), the fact is confirmed by the present study that electron flow was largely devoted to RuBP oxygenation in response to the high light intensity of sunflecks (Figure 5E). Diminished glycine/serine (Gly/Ser) in Arabidopsis CEF mutants grown under high light conditions indicates impaired photorespiration, suggesting a strong link between CEF and photorespiration (Florez-Sarasa et al., 2016). Similarly, based on the correlation between CEF and electron flow devoted to RuBP oxygenation (JO), the electron flow that was largely devoted to RuBP oxygenation would contribute to the operation of the CEF (Figure 5H; von Caemmerer, 2020; Marçal et al., 2021). Thus, for plants growing in the shade environment, the photorespiration pathway regulates the photosynthetic electron flow under transient high light.
Conclusion
Energy dissipation through NPQ predominates in response to the dynamic sunflecks, the V cycle plays an important role in regulating the NPQ processes, leading to the dissipation of excess light energy. Meanwhile, CEF was highly stimulated to protect PSI from photo-inhibition in response to the dynamic sunflecks. Additionally, photorespiration plays a role in regulating the CEF in response to the transient high light, whereas the Lx cycle together with the decelerated NPQ may be an effective mechanism of elevating the maximum photochemical quantum efficiency of PSII under the light in response to the transient low light. Overall, our results indicate that NPQ and CEF may protect the photosynthesis apparatus from dynamic sunfleck in a typically shade-tolerant species.
Data Availability Statement
The original contributions presented in the study are included in the article/supplementary material, further inquiries can be directed to the corresponding author.
Author Contributions
J-WC directed the whole process of the experiment and made suggestions for the writing of the manuscript. J-YZ participated in the whole experiment, analyzed the relevant experimental data, and wrote the manuscript. Q-HZ and S-PS measured the light absorption in photosystem I and chlorophyll fluorescence. ZC and H-MW participated in the determination of photosynthetic pigment content and steady-state gas exchange measurements. All authors contributed to the article and approved the submitted version.
Funding
This research was funded by the National Natural Science Foundation of China (81860676 and 32160248) and the Major Special Science and Technology Project of Yunnan Province, China (202102AA310048).
Conflict of Interest
The authors declare that the research was conducted in the absence of any commercial or financial relationships that could be construed as a potential conflict of interest.
Publisher’s Note
All claims expressed in this article are solely those of the authors and do not necessarily represent those of their affiliated organizations, or those of the publisher, the editors and the reviewers. Any product that may be evaluated in this article, or claim that may be made by its manufacturer, is not guaranteed or endorsed by the publisher.
Abbreviations
CEF, cyclic electron flow; ETR(I), electron transport rate of PSI; ETR(II), electron transport rate of PSII; Fm, maximum fluorescence after dark-adaptation; Fm′, maximum fluorescence after light adaptation; Fo, minimum fluorescence after dark-adaptation; Fo′, minimum fluorescence after light adaptation; Fs, light-adapted steady-state fluorescence; Fv′/Fm′, maximum photochemical quantum efficiency of PSII under light; Jf,d, PSII fluorescence and constitutive heat dissipation electron transfer rate; JNC, the residual absorbed energy used for non-net carboxylative processes; JNPQ, electron transfer rate of non-photochemical quenching pathway of PSII; JO, electron flow devoted to RuBP oxygenation; Lx, lutein epoxide; NPQ, non-photochemical quenching; [(Z + A)/(V + A + Z)], the de-epoxidation state; Y(I), quantum yield of PSI; Y(II), effective quantum yield of PSII photochemistry; Y(NA), the acceptor-side limitation of PSI; Y(ND), the donor-side limitation of PSI; Φf,d, the relatively high fluorescence and constitutive heat dissipation quantum efficiency of PSII; ΦNPQ, light-dependent heat dissipation quantum efficiency of PSII; ΦPSII, effective photochemical quantum yield of PSII.
References
Abedi-Koupai, J., Mostafazadeh-Fard, B., Afyuni, M., and Bagheri, M. R. (2006). Effect of treated wastewater on soil chemical and physical properties in an arid region. Plant Soil Environ. 52, 335–334. doi: 10.17221/3450-PSE
Allahverdiyeva, Y., Suorsa, M., Tikkanen, M., and Aro, E. M. (2015). Photoprotection of photosystems in fluctuating light intensities. J. Exp. Bot. 66, 2427–2436. doi: 10.1093/jxb/eru463
Allakhverdiev, S. I., and Murata, N. (2004). Environmental stress inhibits the synthesis de novo of proteins involved in the photodamage-repair cycle of photosystem II in Synechocystis sp. PCC 6803. Biochim. Biophys. Acta 1657, 23–32. doi: 10.1016/j.bbabio.2004.03.003
Bassman, J. B., and Zwier, J. C. (1991). Gas exchange characteristics of Populus trichocarpa Populus deltoides and Populus trichocarpa x P. deltoides clones. Tree Physiol. 8, 145–159. doi: 10.1093/treephys/8.2.145
Bernacchi, C. J., Singsaas, E. L., Pimentel, C., Portis, A. R., and Long, S. P. (2001). Improved temperature response functions for models of Rubisco-limited photosynthesis. Plant Cell Environ. 24, 253–259. doi: 10.1111/j.1365-3040.2001.00668.x
Bungard, R. A., Ruban, A. V., Hibberd, J. M., Press, M. C., Horton, P., and Scholes, J. D. (1999). Unusual carotenoid composition and a new type of xanthophyll cycle in plants. Proc. Natl. Acad. Sci. 96, 1135–1139. doi: 10.1073/pnas.96.3.1135
Cao, Y. M., Zhang, Z. Q., Zhang, T., You, Z., Geng, J. C., Wang, Y. F., et al. (2018). Overexpression of a zeaxanthin epoxidase gene from Medicago sativa enhances the tolerance to low light in transgenic tobacco. Acta Biochim. Pol. 65, 431–435. doi: 10.18388/abp.2018_2551
Carraretto, L., Teardo, E., Checchetto, V., Finazzi, G., Uozumi, N., and Szabo, I. (2016). Ion channels in plant bioenergetic organelles chloroplasts and mitochondria: from molecular identification to function. Mol. Plant 9, 371–395. doi: 10.1016/j.molp.2015.12.004
Cazzaniga, S., Dall’Osto, L., Kong, S. G., Wada, M., and Bassi, R. (2013). Interaction between avoidance of photon absorption excess energy dissipation and zeaxanthin synthesis against photooxidative stress in a rabidopsis. Plant J. 76, 568–579. doi: 10.1111/tpj.12314
Chazdon, R. L., and Pearcy, C. R. W. (1991). The importance of sunflecks for forest understory plants. Bioscience 41, 760–766. doi: 10.2307/1311725
Chen, J. W., Kuang, S. B., Long, G. Q., Meng, Z. G., Li, L. G., Chen, Z. J., et al. (2014). Steady-state and dynamic photosynthetic performance and nitrogen partitioning in the shade-demanding plant Panax notoginseng under different levels of growth irradiance. Acta Physiol. Plant. 36, 2409–2420. doi: 10.1007/s11738-014-1614-9
Chen, J. W., Kuang, S. B., Long, G. Q., Yang, S. C., Meng, Z. G., Li, L. G., et al. (2016). Photosynthesis light energy partitioning and photoprotection in the shade-demanding species Panax notoginseng under high and low level of growth irradiance. Funct. Plant Biol. 43, 479–493. doi: 10.1071/FP15283
Crisp, P. A., Ganguly, D. R., Smith, A. B., Murray, K. D., Estavillo, G. M., Searle, I., et al. (2017). Rapid recovery gene downregulation during excess-light stress and recovery in Arabidopsis. Plant Cell 29, 1836–1863. doi: 10.1105/tpc.16.00828
Cruz, J. A., Avenson, T. J., Kanazawa, A., Takizawa, K., Edwards, G. E., and Kramer, D. M. (2005). Plasticity in light reactions of photosynthesis for energy production and photoprotection. J. Exp. Bot. 56, 395–406. doi: 10.1093/jxb/eri022
Da, Q., Sun, T., Wang, M., Jin, H., Li, M., Feng, D., et al. (2018). M-type thioredoxins are involved in the xanthophyll cycle and proton motive force to alter NPQ under low-light conditions in Arabidopsis. Plant Cell Rep. 37, 279–291. doi: 10.1007/s00299-017-2229-6
Demmig, A. B., Adams, W. W. I. I. I., Barker, D. H., Logan, B. A., Bowling, D. R., and Verhoeven, A. S. (1996). Using chlorophyll fluorescence to assess the fraction of absorbed light allocated to thermal dissipation of excess excitation. Physiol. Plant. 98, 253–264. doi: 10.1034/j.1399-3054.1996.980206.x
Demmig-Adams, B., Cohu, C. M., Muller, O., and Lii, W. W. A. (2012). Modulation of photosynthetic energy conversion efficiency in nature: from seconds to season. Photosynth. Res. 113, 75–88. doi: 10.1007/s11120-012-9761-6
Durgud, M., Gupta, S., Ivanov, I., Omidbakhshfard, M. A., Benina, M., Alseekh, S., et al. (2018). Molecular mechanisms preventing senescence in response to prolonged darkness in a desiccation-tolerant plant. Plant Physiol. 177, 1319–1338. doi: 10.1104/pp.18.00055
Eisenhut, M., Bräutigam, A., Timm, S., Florian, A., Tohge, T., Fernie, A. R., et al. (2017). Photorespiration is crucial for dynamic response of photosynthetic metabolism and stomatal movement to altered CO2 availability. Mol. Plant 10, 47–61. doi: 10.1016/j.molp.2016.09.011
Endo, T., Shikanai, T., Takabayashi, A., Asada, K., and Sato, F. (1999). The role of chloroplastic NAD(P)H dehydrogenase in photoprotection. FEBS Lett. 457, 5–8. doi: 10.1016/S0014-5793(99)00989-8
Esteban, R., Jiménez, E. T., Jiménez, M. S., Morales, D., Hormaetxe, K., Becerril, J. M., et al. (2007). Dynamics of violaxanthin and lutein epoxide xanthophyll cycles in Lauraceae tree species under field conditions. Tree Physiol. 27, 1407–1414. doi: 10.1093/treephys/27.10.1407
Esteban, R., Matsubara, S., Soledad-Jiménez, M., Morales, D., Brito, P., Lorenzo, R., et al. (2010). Operation and regulation of the lutein epoxide cycle in seedlings of Ocotea foetans. Funct. Plant Biol. 37, 859–869. doi: 10.1071/FP10014
Florez-Sarasa, I., Noguchi, K., Araújo, W. L., Garcia-Nogales, A., Fernie, A. R., Flexas, J., et al. (2016). Impaired cyclic electron flow around photosystem I disturbs high-light respiratory metabolism. Plant Physiol. 172, 2176–2189. doi: 10.1104/pp.16.01025
Förster, B., Osmond, C. B., and Pogson, B. J. (2009). De novo synthesis and degradation of Lx and V cycle pigments during shade and sun acclimation in avocado leaves. Plant Physiol. 149, 1179–1195. doi: 10.1104/pp.108.131417
Förster, B., Pogson, B. J., and Osmond, C. B. (2011). Lutein from deepoxidation of lutein epoxide replaces zeaxanthin to sustain an enhanced capacity for nonphotochemical chlorophyll fluorescence quenching in avocado shade leaves in the dark. Plant Physiol. 156, 393–403. doi: 10.1104/pp.111.173369
Foyer, C. H., Neukermans, J., Queval, G., Noctor, G., and Harbinson, J. (2012). Photosynthetic control of electron transport and the regulation of gene expression. J. Exp. Bot. 63, 1637–1661. doi: 10.1093/jxb/ers013
Garcia-Molina, A., and Leister, D. (2020). Accelerated relaxation of photoprotection impairs biomass accumulation in Arabidopsis. Nat. Plants 6, 9–12. doi: 10.1038/s41477-019-0572-z
García-Plazaola, J. I., Esteban, R., Fernández-Marín, B., Kranner, I., and Porcar-Castell, A. (2012). Thermal energy dissipation and xanthophyll cycles beyond the Arabidopsis model. Photosynth. Res. 113, 89–103. doi: 10.1007/s11120-012-9760-7
García-Plazaola, J. I., Matsubara, S., and Osmond, C. B. (2007). The lutein epoxide cycle in higher plants: its relationships to other xanthophyll cycles and possible functions. Funct. Plant Biol. 34, 759–773. doi: 10.1071/FP07095
Genty, B., Briantais, J. M., and Baker, N. R. (1989). The relationship between the quantum of photosynthetic electron transport and quenching of chlorophyll fluorescence. BBA Gen Subj. 990, 87–92. doi: 10.1016/S0304-4165(89)80016-9
Grieco, M., Tikkanen, M., Paakkarinen, V., Kangasjärvi, S., and Aro, E. M. (2012). Steady-state phosphorylation of light-harvesting complex II proteins preserves photosystem I under fluctuating white light. Plant Physiol. 160, 1896–1910. doi: 10.1104/pp.112.206466
Hendrickson, L., Furbank, R. T., and Chow, W. S. (2004). A simple alternative approach to assessing the fate of absorbed light energy using chlorophyll fluorescence. Photosynth. Res. 82, 73–81. doi: 10.1023/B:PRES.0000040446.87305.f4
Huang, J., Zhao, X., and Chory, J. (2019). The Arabidopsis transcriptome responds specifically and dynamically to high light stress. Cell Rep. 29, 4186–4199.e3. doi: 10.1016/j.celrep.2019.11.051
Huang, W., Zhang, S. B., and Cao, K. F. (2012b). Physiological role of cyclic electron floe in higher plants. Plant Sci. J. 30, 100–106. doi: 10.3389/fpls.2015.00621
Huang, W., Yang, S. J., Zhang, S. B., Zhang, J. L., and Cao, K. F. (2012a). Cyclic electron flow plays an important role in photoprotection for the resurrection plant Paraboea rufescens under drought stress. Planta 235, 819–828. doi: 10.1007/s00425-011-1544-3
Huang, W., Yang, Y. J., Hu, H., and Zhang, S. B. (2015b). Different roles of cyclic electron flow around photosystem I under sub-saturating and saturating light intensities in tobacco leaves. Front. Plant Sci. 6, 923–932. doi: 10.3389/fpls.2015.00923
Huang, W., Zhang, S. B., Zhang, J. L., and Hu, H. (2015c). Photoinhibition of photosystem I under high light in the shade-established tropical tree species Psychotria rubra. Front. Plant Sci. 6:801. doi: 10.3389/fpls.2015.00801
Huang, W., Hu, H., and Zhang, S. B. (2015a). Photorespiration plays an important role in the regulation of photosynthetic electron flow under fluctuating light in tobacco plants grown under full sunlight. Front. Plant Sci. 6:621.
Huang, W., Zhang, S. B., and Cao, K. F. (2011). Cyclic electron flow plays an important role in photoprotection of tropical trees illuminated at temporal chilling temperature. Plant Cell Physiol. 52, 297–305. doi: 10.1093/pcp/pcq166
Huang, W., Zhang, S. B., and Hu, H. (2014). Sun leaves up-regulate the photorespiratory pathway to maintain a high rate of CO2 assimilation in tobacco. Front. Plant Sci. 5:688. doi: 10.3389/fpls.2014.00688
Hubbart, S., Ajigboye, O. O., Horton, P., and Murchie, E. H. (2012). The photoprotective protein PsbS exerts control over CO2 assimilation rate in fluctuating light in rice. Plant J. 71, 402–412. doi: 10.1111/j.1365-313X.2012.04995.x
Ikeuchi, M., Uebayashi, N., Sato, F., and Endo, T. (2014). Physiological functions of PsbS-dependent and PsbS-independent NPQ under naturally fluctuating light conditions. Plant Cell Physiol. 55, 1286–1295. doi: 10.1093/pcp/pcu069
Ishida, S., Uebayashi, N., Tazoe, Y., Ikeuchi, M., Homma, K., Sato, F., et al. (2014). Diurnal and developmental changes in energy allocation of absorbed light at PSII in field-grown rice. Plant Cell Physiol. 55, 171–182. doi: 10.1093/pcp/pct169
Jahns, P., and Holzwarth, A. R. (2012). The role of the xanthophyll cycle and of lutein in photoprotection of photosystem II. BBA Bioenerg. 1817, 182–193. doi: 10.1016/j.bbabio.2011.04.012
Jin, H., Li, M., Duan, S., Fu, M., Dong, X., Liu, B., et al. (2016). Optimization of light-harvesting pigment improves photosynthetic efficiency. Plant Physiol. 172, 1720–1731. doi: 10.1104/pp.16.00698
Johnson, M. P., and Ruban, A. V. (2010). Arabidopsis plants lacking PsbS protein possess photoprotective energy dissipation. Plant J. 61, 283–289. doi: 10.1111/j.1365-313X.2009.04051.x
Johnson, M. P., and Ruban, A. V. (2011). Restoration of rapidly reversible photoprotective energy dissipation in the absence of PsbS protein by enhanced delta ΔpH. J. Biol. Chem. 286, 19973–19981. doi: 10.1074/jbc.M111.237255
Kono, M., Kawaguchi, H., Mizusawa, N., Yamori, W., Suzuki, Y., and Terashima, I. (2019). Far-red light accelerates photosynthesis in the low-light phases of fluctuating light. Plant Cell Physiol. 61, 192–202. doi: 10.1093/pcp/pcz191
Kono, M., Noguchi, K., and Terashima, I. (2014). Roles of the cyclic electron flow around PSI (CEF-PSI) and O2-dependent alternative pathways in regulation of the photosynthetic electron flow in short-term fluctuating light in Arabidopsis thaliana. Plant Cell Physiol. 55, 990–1004. doi: 10.1093/pcp/pcu033
Kono, M., Yamori, W., Suzuki, Y., and Terashima, I. (2017). Photoprotection of PSI by far-red light against the fluctuating light-induced Photoinhibition in Arabidopsis thaliana and field-grown plants. Plant Cell Physiol. 58, 35–45. doi: 10.1093/pcp/pcw215
Kornyeyev, D., and Hendrickson, L. (2007). Energy partitioning in photosystem II complexes subjected to photoinhibitory treatment. Funct. Plant Biol. 34, 214–220. doi: 10.1071/FP06327
Kramer, D. M., Johnson, G., Kiirats, O., and Edwards, G. E. (2004). New fluorescence parameters for the determination of QA redox state and excitation energy fluxes. Photosynth. Res. 79, 209–218. doi: 10.1023/B:PRES.0000015391.99477.0d
Krieger-Liszkay, A., Fufezan, C., and Trebst, A. (2008). Singlet oxygen production in photosystem II and related protection mechanism. Photosynth. Res. 98, 551–564. doi: 10.1007/s11120-008-9349-3
Kromdijk, J., Glowacka, K., Leonelli, L., Gabilly, S. T., Twai, M., Niyogi, K. K., et al. (2016). Improving photosynthesis and crop productivity by accelerating recovery from photoprotection. Science 354, 857–861. doi: 10.1126/science.aai8878
Kuang, S. B., Xu, X. Z., Meng, Z. G., Zhang, G. H., Yang, S. C., Chen, Z. J., et al. (2015). Effects of light transmittance on plant growth and root ginsenoside content of Panax notoginseng. Chin. J. Appl. Environ. Biol. 21, 279–286.
Li, X. P., Müller-Moulé, P., Gilmore, A. M., and Niyogi, K. K. (2002). PsbS-dependent enhancement of feedback de-excitation protects photosystem II from photoinhibition. Proc. Natl. Acad. Sci. USA 99, 15222–15227. doi: 10.1073/pnas.232447699
Losciale, P., Chow, W. S., and Grappadelli, L. C. (2010). Modulating the light environment with the peach ‘asymmetric orchard’: effects on gas exchange performances photoprotection and photoinhibition. J. Exp. Bot. 61, 1177–1192. doi: 10.1093/jxb/erp387
Losciale, P., Oguchi, R., Hendrickson, L., Hope, A. B., Corelli-Grappadelli, L., and Chow, W. S. (2008). A rapid whole-tissue determination of the functional fraction of PSII after photoinhibition of leaves based on flash-induced P700 redox kinetics. Physiol. Plant 132, 23–32. doi: 10.1111/j.1399-3054.2007.01000.x
Marçal, D. M., Avila, R. T., Quiroga-Rojas, L. F., de Souza, R. P., Junior, C. C. G., Ponte, L. R., et al. (2021). Elevated [CO2] benefits coffee growth and photosynthetic performance regardless of light availability. Plant Physiol. Biochem. 158, 524–535. doi: 10.1016/j.plaphy.2020.11.042
Mathur, S., Jain, L., and Jajoo, A. (2018). Photosynthetic efficiency in sun and shade plants. Photosynthetica 56, 354–365. doi: 10.1007/s11099-018-0767-y
Matsubara, S., Krause, G. H., Aranda, J., Virgo, A., Beisel, K. G., Jahns, P., et al. (2009). Sun-shade patterns of leaf carotenoid composition in 86 species of neotropical forest plants. Funct. Plant Biol. 36, 20–36. doi: 10.1071/FP08214
Matsubara, S., Krause, G. H., Seltmann, M., Virgo, A., Kursar, T. A., Jahns, P., et al. (2008). Lutein epoxide cycle light harvesting and photoprotection in species of the tropical tree genus Inga. Plant Cell Environ. 31, 548–561. doi: 10.1111/j.1365-3040.2008.01788.x
Matsubara, S., Morosinotto, T., Osmond, C. B., and Bassi, R. (2007). Short-and long-term operation of the lutein-epoxide cycle in light-harvesting antenna complexes. Plant Physiol. 144, 926–941. doi: 10.1104/pp.107.099077
Matsubara, S., Naumann, M., Martin, R., Nichol, C., Rascher, U., Morosinotto, T., et al. (2005). Slowly reversible de-epoxidation of luteinepoxide in deep shade leaves of a tropical tree legume may ‘lock-in’ lutein-based photoprotection during acclimation to strong light. J. Exp. Bot. 56, 461–468. doi: 10.1093/jxb/eri012
Maxwell, K., and Johnson, G. N. (2000). Chlorophyll fluorescence-a practical guide. J. Exp. Bot. 51, 659–668. doi: 10.1093/jexbot/51.345.659
Miyake, C., Miyata, M., Shinzaki, Y., and Tomizawa, K. I. (2005). CO2 response of cyclic electron flow around PSI (CEF-PSI) in tobacco leaves-relative electron fluxes through PSI and PSII determine the magnitude of non-photochemical quenching (NPQ) of Chl fluorescence. Plant Cell Physiol. 46, 629–637. doi: 10.1093/pcp/pci067
Müller, P., Li, X. P., and Niyogi, K. K. (2001). Non-photochemical quenching. a response to excess light energy. Plant Physiol. 125, 1558–1566. doi: 10.1104/pp.125.4.1558
Munekage, Y. N., Genty, B., and Peltier, G. (2008). Effect of PGR5 impairment on photosynthesis and growth in Arabidopsis thaliana. Plant Cell Physiol. 49, 1688–1698. doi: 10.1093/pcp/pcn140
Munekage, Y., Hojo, M., Meurer, J., Endo, T., Tasaka, M., and Shikanai, T. (2002). PGR5 is involved in cyclic electron flow around photosystem I and is essential for photoprotection in Arabidopsis. Cell 110, 361–371. doi: 10.1016/S0092-8674(02)00867-X
Murchie, E. H., and Niyogi, K. K. (2011). Manipulation of photoprotection to improve plant photosynthesis. Plant Physiol. 155, 86–92. doi: 10.1104/pp.110.168831
Niyogi, K. K., and Truong, T. B. (2013). Evolution of flexible non-photochemical quenching mechanisms that regulate light harvesting in oxygenic photosynthesis. Curr. Opin. Plant Biol. 16, 307–314. doi: 10.1016/j.pbi.2013.03.011
Niyogi, K. K., Li, X. P., Rosenberg, V., and Jung, H. S. (2005). Is PsbS the site of non-photochemical quenching in photosynthesis? J. Exp. Bot. 56, 375–382. doi: 10.1093/jxb/eri056
Pawlak, K., Paul, S., Liu, C., Reus, M., Yang, C., and Holzwarth, A. R. (2020). On the PsbS-induced quenching in the plant major light-harvesting complex LHCII studied in proteoliposomes. Photosynth. Res. 144, 195–208. doi: 10.1007/s11120-020-00740-z
Pérez-Bueno, M. L., Johnson, M. P., Zia, A., Ruban, A. V., and Horton, P. (2008). The Lhcb protein and xanthophyll composition of the light harvesting antenna controls the ΔpH-dependency of non-photochemical quenching in Arabidopsis thaliana. FEBS Lett. 582, 1477–1482. doi: 10.1016/j.febslet.2008.03.040
Ruban, A. V. (2016). Nonphotochemical chlorophyll fluorescence quenching: mechanism and effectiveness in protecting plants from photodamage. Plant Physiol. 170, 1903–1916. doi: 10.1104/pp.15.01935
Sato, R., Ohta, H., and Masuda, S. (2014). Prediction of respective contribution of linear electron flow and PGR5-dependent cyclic electron flow to nonphotochemical quenching induction. Plant Physiol. Biochem. 81, 190–196. doi: 10.1016/j.plaphy.2014.03.017
Sunil, B., Saini, D., Bapatla, R. B., Aswani, V., and Raghavendra, A. S. (2019). Photorespiration is complemented by cyclic electron flow and the alternative oxidase pathway to optimize photosynthesis and protect against abiotic stress. Photosynth. Res. 139, 67–79. doi: 10.1007/s11120-018-0577-x
Suorsa, M., Jarvi, S., Grieco, M., Nurmi, M., Pietrzykowska, M., Rantala, M., et al. (2012). Proton gradient regulation5 is essential for proper acclimation of arabidopsis photosystem I to naturally and artificially fluctuating light conditions. Plant Cell 24, 2934–2948. doi: 10.1105/tpc.112.097162
Susan, A., and Thayer, O. B. (1990). Leaf xanthophyll content and composition in san and shade determined by HPLC. Photosynth. Res. 23, 331–343. doi: 10.1007/BF00034864
Szymańska, R., Ślesak, I., Orzechowska, A., and Kruk, J. (2017). Physiological and biochemical responses to high light and temperature stress in plants. Environ. Exp. Bot. 139, 165–177. doi: 10.1016/j.envexpbot.2017.05.002
Takahashi, S., and Murata, N. (2008). How do environmental stresses accelerate photoinhibition? Trends Plant Sci. 13, 178–182. doi: 10.1016/j.tplants.2008.01.005
Takizawa, K., Cruz, J. A., Kanazawa, A., and Kramer, D. M. (2007). The thylakoid proton motive force in vivo. quantitative, non-invasive probes, energetics, and regulatory consequences of light-induced pmf. BBA Bioenerg. 1767, 1233–1244. doi: 10.1016/j.bbabio.2007.07.006
Tan, S. L., Liu, T., Zhang, S. B., and Huang, W. (2020). Balancing light use efficiency and photoprotection in tobacco leaves grown at different light regimes. Environ. Exp. Bot. 175:104046. doi: 10.1016/j.envexpbot.2020.104046
Tausz, M., Warren, C. R., and Adams, M. A. (2005). Dynamic light use and protection from excess light in upper canopy and coppice leaves of Nothofagus cunninghamii in an old growth cool temperate rainforest in Victoria Australia. New Phytol. 165, 143–156. doi: 10.1111/j.1469-8137.2004.01232.x
Tikkanen, M., Grieco, M., Kangasjärvi, S., and Aro, E. M. (2010). Thylakoid protein phosphorylation in higher plant chloroplasts optimizes electron transfer under fluctuating light. Plant Physiol. 152, 723–735. doi: 10.1104/pp.109.150250
Tikkanen, M., Grieco, M., Nurmi, M., Rantala, M., Suorsa, M., and Aro, E. M. (2012). Regulation of the photosynthetic apparatus under fluctuating growth light. Philos. Trans. R. Soc. B Biol. Sci. 367, 3480–3493. doi: 10.1098/rstb.2012.0067
Tiwari, A., Mamedov, F., Grieco, M., Suorsa, M., Jajoo, A., Styring, S., et al. (2016). Photodamage of iron-sulphur clusters in photosystem i induces non-photochemical energy dissipation. Nat. Plants 2:16035. doi: 10.1038/nplants.2016.35
Townsend, A. J., Retkute, R., Chinnathambi, K., Randall, J. W. P., Foulkes, J., Carmo-Silva, E., et al. (2017). Suboptimal acclimation of photosynthesis to light in wheat canopies. Plant Physiol. 176, 1233–1246. doi: 10.1104/pp.17.01213
Townsend, A. J., Saccon, F., Giovagnetti, V., Wilson, S., Ungerer, P., and Ruban, A. V. (2018a). The causes of altered chlorophyll fluorescence quenching induction in the Arabidopsis mutant lacking all minor antenna complexes. Biochim. Biophys. Acta Bioenery 1859, 666–675. doi: 10.1016/j.bbabio.2018.03.005
Townsend, A. J., Ware, M. A., and Ruban, A. V. (2018b). Dynamic interplay between photodamage and photoprotection in photosystem II. Plant Cell Environ. 41, 1098–1112. doi: 10.1111/pce.13107
Valentini, R., Epron, D., Angeli, D., Matteucci, G., and Dreyer, E. (1995). In situestimation of net CO2 assimilation photosynthetic electron flow and photorespiration in Turkey oak (Q cerris L) leaves: diurnal cycles under different levels of water supply. Plant Cell Environ. 18, 631–640. doi: 10.1111/j.1365-3040.1995.tb00564.x
van Kooten, O., and Snel, J. F. H. (1990). The use of chlorophyll fluorescence nomenclature in plant stress physiology. Photosynth. Res. 25, 147–150. doi: 10.1007/BF00033156
Vialetchabrand, S., Matthews, J. S., Simkin, A. J., Raines, C. A., and Lawson, T. (2017). Importance of fluctuations in light on plant photosynthetic acclimation. Plant Physiol. 173, 2163–2179. doi: 10.1104/pp.16.01767
von Caemmerer, S. (2020). Rubisco carboxylase/oxygenase: from the enzyme to the globe: a gas exchange perspective. J. Plant Physiol. 252:153240. doi: 10.1016/j.jplph.2020.153240
Wagner, H., Jakob, T., and Wilhelm, C. (2006). Balancing the energy flow from captured light to biomass under fluctuating light conditions. New Phytol. 169, 95–108. doi: 10.1111/j.1469-8137.2005.01550.x
Wang, C., Yamamoto, H., and Shikanai, T. (2015). Role of cyclic electron transport around photosystem I in regulating proton motive force. BBA Bioenerg. 1847, 931–938. doi: 10.1016/j.bbabio.2014.11.013
Way, D. A., and Pearcy, R. W. (2012). Sunflecks in trees and forests: from photosynthetic physiology to global change biology. Tree Physiol. 32, 1066–1081. doi: 10.1093/treephys/tps064
Xu, X. Z., Zhang, J. Y., Zhang, G. H., Long, G. Q., Yang, S. C., Chen, Z. J., et al. (2018). Effects of light intensity on photosynthetic capacity an light energy allocation in Panax notoginseng. Chin. J. Appl. Ecol. 29, 193–204.
Yamori, W., Makino, A., and Shikanai, T. (2016). A physiological role of cyclic electron transport around photosystem I in sustaining photosynthesis under fluctuating light in rice. Sci. Rep. 6:20147. doi: 10.1038/srep20147
Yang, Y. J., Ding, X. X., and Huang, W. (2019a). Stimulation of cyclic electron flow around photosystem I upon a sudden transition from low to high light in two angiosperms Arabidopsis thaliana and Bletilla striata. Plant Sci. 287:110166.
Yang, Y. J., Zhang, S. B., Wang, J. H., and Huang, W. (2019b). Photosynthetic regulation under fluctuating light in field-grown Cerasus cerasoides: a comparison of young and mature leaves. Biochim. Biophys. Acta Bioenery 1860:148073. doi: 10.1016/j.bbabio.2019.148073
Zhao, S. J., Meng, Q. W., Xu, C. C., Han, H. Y., and Zou, Q. (1995). Analysis of the xanthophyll cycle components in plant tissues by high performance liquid chromatography. Plant Physiol. Commun. 31, 438–442.
Keywords: non-photochemical quenching, cyclic electron flow, lutein epoxide cycle, photorespiration, dynamic sunflecks, Panax notoginseng
Citation: Zhang J-Y, Zhang Q-H, Shuang S-P, Cun Z, Wu H-M and Chen J-W (2021) The Responses of Light Reaction of Photosynthesis to Dynamic Sunflecks in a Typically Shade-Tolerant Species Panax notoginseng. Front. Plant Sci. 12:718981. doi: 10.3389/fpls.2021.718981
Received: 01 June 2021; Accepted: 20 September 2021;
Published: 13 October 2021.
Edited by:
Laura De Gara, Campus Bio-Medico University, ItalyReviewed by:
Eva Kotabová, Centre of Algal Biotechnology, Institute of Microbiology, Academy of Sciences of the Czech Republic, CzechiaDavid Kramer, Michigan State University, United States
Copyright © 2021 Zhang, Zhang, Shuang, Cun, Wu and Chen. This is an open-access article distributed under the terms of the Creative Commons Attribution License (CC BY). The use, distribution or reproduction in other forums is permitted, provided the original author(s) and the copyright owner(s) are credited and that the original publication in this journal is cited, in accordance with accepted academic practice. No use, distribution or reproduction is permitted which does not comply with these terms.
*Correspondence: Jun-Wen Chen, Y2p3MzE0MTJAMTYzLmNvbQ==