- 1Laboratoire de Recherche en Sciences Végétales, Université de Toulouse, CNRS, UPS, Toulouse, France
- 2Laboratoire de Recherche en Sciences Végétales, Université de Toulouse, CNRS, UPS, Toulouse INP, Auzeville-Tolosane, France
Introduction
Because of their motionless state, plants are exposed in their environment, often simultaneously, to multiple biotic (bacteria, fungi, oomycetes, viruses, insects) and abiotic (temperature, drought, water and nutrient availability) constraints. To adapt, plants have developed amazing abilities allowing the perception of their situation and the establishment of specific responses (Velásquez et al., 2018; Delplace et al., 2022). These capacities are even more important in the context of climate change for which various scenarios forecast, for the coming decades, an increase in the duration, intensity and frequency of extreme weather events, emergence of new pathogens and/or geographical expansion of their distribution and an increase in epidemics (McDonald & Stukenbrock, 2016; Chaloner et al., 2021; IPCC, 2021). Among climatic parameters, temperature is expected to fluctuate the most by the end of the century (IPCC, 2021). Worryingly, a growing number of studies describe that an elevation in ambient temperature has a negative impact on the majority of the resistance mechanisms deployed by plants (Desaint et al., 2021). It is therefore crucial to understand the effects of pathogens combined with elevated temperature on plant responses and to identify the mechanisms that contribute to robustness of resistance. However, our knowledge on the strategies developed by plants to face the combined constraints remains fragmented. Except for system involving the Arabidopsis plant model, the impact of temperature elevation combined with the induction of plant immunity through flagellin (flg22) treatment have mostly been investigated at the transcriptomic level (Huot et al., 2017; Velásquez et al., 2018; Kim et al., 2022; Yuan and Poovaiah, 2022). Indeed, immune mechanisms, recently demonstrate to be much more interconnected than the simplified “zig-zag model” previously proposed more than a decade ago, restrict pathogen proliferation (Jones and Dangl, 2006; Ngou et al., 2021; Yuan et al., 2021a). Early stages of perception and signaling are often decisive to elicit appropriate responses. Most external stimuli induce an increase in cytosolic calcium concentration that is central to initiate later responses (Liu et al., 2020). In eukaryotes, intracellular calcium variations are considered a universal second messenger, which must be decoded and relayed by calcium sensors to downstream targets allowing the implementation of finely-tuned, spatio-temporal responses, including transcriptome modifications (Ranty et al., 2016; Aldon et al., 2018; Liu et al., 2020; Luan and Wang, 2021). Numerous studies indicate that calcium sensors are involved in many processes such as plant development, biotic and abiotic responses (Zhu et al., 2015; Luan and Wang, 2021). Therefore, this opinion paper aims at providing evidence supporting the premise that proteins participating to the generation and decoding of calcium variations, would be central in shaping plant responses against combined biotic and elevated temperature constraints.
Calcium signaling in plant immunity
The generation of intracellular calcium variation in response to pests or PAMPs has been described for many years and the contribution of calcium signaling in the establishment of resistance to pathogens is supported by many studies (Atkinson et al., 1990; Knight et al., 1991; Blume et al., 2000; Lecourieux et al., 2006). To date, genetic approaches clearly established that the channels and pumps involved in calcium signal generation and dissipation as well as calcium sensors, contribute to the implementation of efficient responses against pathogens. Until 2019, the nature of calcium channels involved in immune responses remained largely unknown. Tian et al. (2019) and Wang et al. (2019) showed, in Arabidopsis and rice respectively, that Cyclic Nucleotide-Gated Channels (CNGCs), which are calcium permeable channels, are crucial for calcium increase upon flg22 or chitin perception. The perception of flg22 by the FLAGELLIN-SENSITIVE 2 (FLS2)/BRI1-ASSOCIATED RECEPTOR KINASE (BAK1) complex induce Pathogen Associated Molecular Patterns (PAMP)-Triggered Immunity (PTI) by leading to the phosphorylation and activation of the CNGC2/CNGC4 heterodimer by the BOTRYTIS-INDUCED KINASE1 (BIK1) (Tian et al., 2019) (Figure 1). CNGC’s activity is also regulated by calmodulin (CaM), as in the case of CaM7 in Arabidopsis, which prevents CNGC2/4 activity and calcium influx (Tian et al., 2019) (Figure 1). More recently, HOPZ-ACTIVATED RESISTANCE 1 (ZAR1), an intracellular Nucleotide-binding Leucine -rich Receptor (NLR), was shown to interact with bacterial effectors inducing Effector-Triggered Immunity (ETI) and to form pentameric complexes in vitro with protein kinases called resistosomes (Bi et al., 2021). This complex acts as a cation-selective channel engaged in the plasma membrane that induces calcium influx, reactive oxygen species production, cell death and finally immunity to plants (Bi et al., 2021) (Figure 1). Usually seen as two independent steps in plant immunity, PTI and ETI have been recently demonstrated to potentiate one another (Ngou et al., 2021; Yuan et al., 2021a) and calcium signaling is known to be a key player in both of them (Yuan et al., 2021b; Köster et al., 2022). In addition to the identification of the previous channels, strong evidence implicating CaM; the Calmodulin-like proteins (CMLs) (Zhu et al., 2015), Calcium-Dependent Protein Kinases (CDPKs) (Shi et al., 2018; Bredow and Monaghan, 2019) and Calcineurin B-Like (CBLs) (Ma et al., 2020; Tang et al., 2020) support that calcium sensors are essential in biotic stress responses (Figure 1). Increasing data report the contribution of CMLs to defense regulation against various pathogens such as oomycetes, bacteria or insects as shown for CML8, which contributes to defense against multiple pathogens varying in lifestyles and infection mode in Arabidopsis (Zhu et al., 2017; Zhu et al., 2021). In tomato, the silencing of CML55 suppresses infection by Phytophthora capsici (Zhang et al., 2022) whereas the silencing of CML13 in pepper enhances the plants’ susceptibility to Ralstonia solanacerarum (Shen et al., 2020). A. Mithöfer’s group also illustrated the contrasting effect of different CMLs in the plant-herbivore interaction. Loss of function of CML42 in Arabidopsis enhances resistance to the caterpillar Spodoptora littoralis, which correlates with the induction of jasmonic acid-responsive genes and to an accumulation of glucosinolates (Vadassery et al., 2012). In contrast, mutation of CML37 increases Arabidopsis susceptibility to this herbivore (Scholz et al., 2014). Other examples show contributions of CMLs in response to both biotic or abiotic constraints, but so far this has not been studied under combined stress conditions. For example, CML24 is a multifunctional CML involved in biotic and abiotic responses as well as in developmental processes (Delk et al., 2005; Tsai et al., 2007; Ma et al., 2008; Zhu et al., 2022) and CML37, described as a regulator of plant responses to S. littoralis, also positively contributes to drought responses in Arabidopsis (Scholz et al., 2015). Interestingly, CML37 and 42 also act antagonistically when plants are exposed to water deficit, herbivores or necrotrophic fungi (Heyer et al., 2021). Other CMLs may also contribute differently to the plant response depending on the constraint such as the Arabidopsis CML9 which is involved in both biotic and abiotic processes. CML9 loss of function results in a higher susceptibility to P. syringae (Leba et al., 2012) and to a lower sensitivity to water deficit (Magnan et al., 2008). Although this opinion paper mostly presents case studies on CMLs, excellent reviews highlight the importance of other calcium sensors, such as CDPKs and CBLs, in the calcium decoding steps related to plant immunity or responses to abiotic constraints and developmental processes (Bredow and Monaghan, 2019; Yip Delormel and Boudsocq, 2019; Ma et al., 2020; Tang et al., 2020).
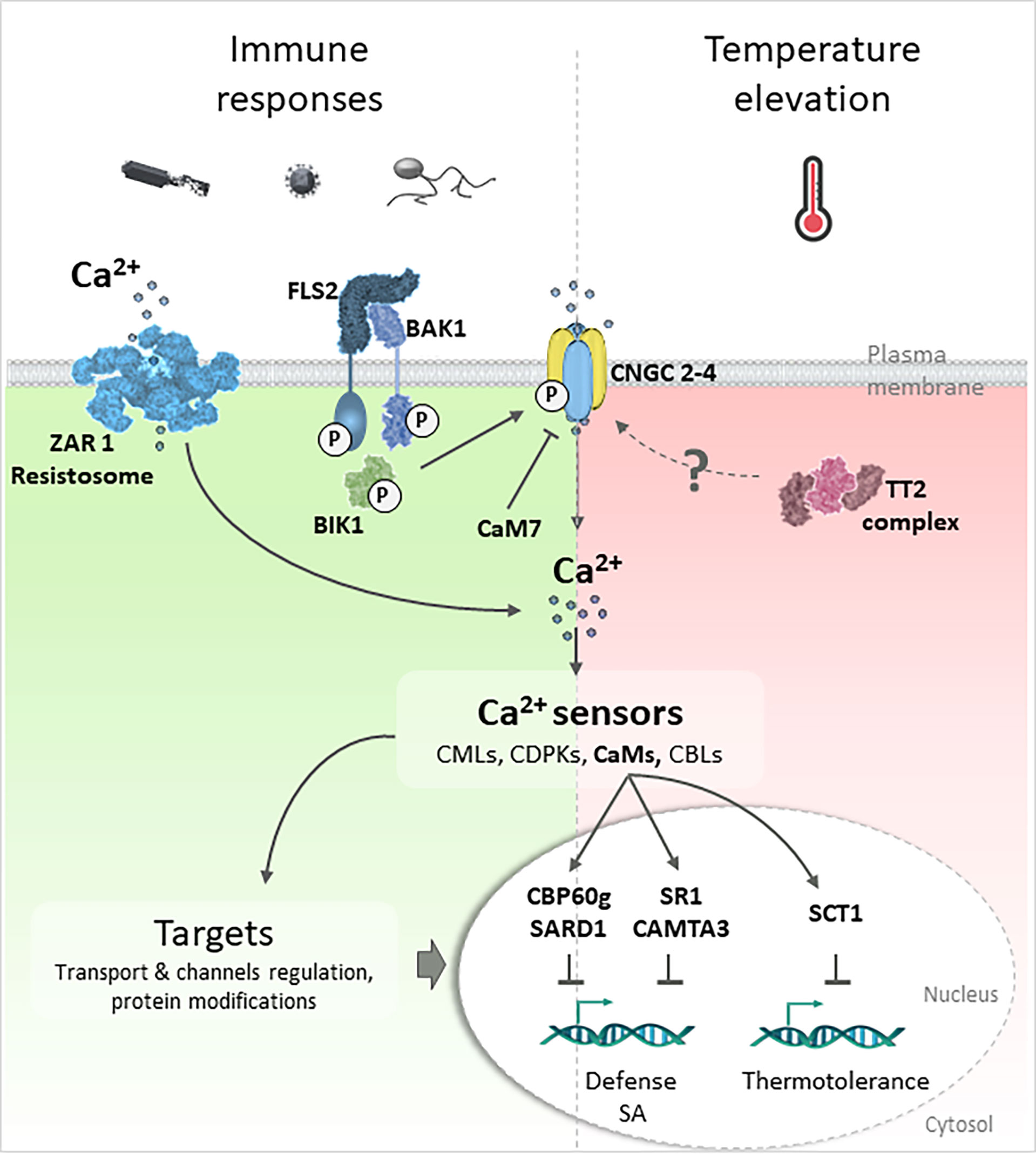
Figure 1 Involvement of calcium signaling in response to biotic and elevated temperature constraints. Plant defense responses to pathogens trigger calcium variations which are relayed by calcium sensors (CaM, CMLs, CDPKs or CBLs) to targets allowing the establishment of specific responses. The P. syringae’s flagellin is recognized by the Pathogen Recognition Receptors (PRR) complex FLS2/BAK1 and induce PTI leading to the kinase BIK1 phosphorylation which phosphorylates CNGC calcium channels and allows calcium entry. The NLR ZAR1 recognizes P. syringae effectors delivered in the cytosol inducing ETI and forms a pentameric structure integrating the plasmalemma (resistosome) (Bi et al., 2021) to act as a calcium-permeable channel. CNGC2-4 are also temperature-sensitive channels allowing calcium entry upon temperature elevation (Guihur et al., 2022b). Their activity is negatively regulated by the CaM7 (Tian et al., 2019). In response to elevated temperature, the rice TT2 complex, composed by a G-subunit, is involved in thermotolerance (Kan et al., 2022). TT2 signaling pathway is mediated by the transcription factor SCT1 that interact with CaM to repress genes required for thermotolerance. Under combined flagellin treatment and elevated temperature condition, SA production is repressed and PTI inhibited through CBP60g/SARD1 and CaM-binding SR1/CAMTA3 dependent pathways (Huot et al., 2017; Kim et al., 2022).
Calcium signaling in heat stress
Collectively, calcium signaling and decoding appear to significantly contribute to plant immunity and abiotic constraints (Patra et al., 2021). Regarding stress associated with temperature elevations, Gong et al. (1998) reported that heat-shock induces transient increases in calcium concentration and that exogenous calcium supply to tobacco seedlings promoted plant tolerance to subsequent transitory heat treatments, whereas the use of a calcium chelator had the opposite effect. It was therefore hypothesized that calcium signaling could significantly contribute to the thermotolerance of plants. Interestingly, the chloroplast has emerged during the last ten years (Navazio et al., 2020) to be important in calcium signaling at elevated temperatures as transient temperature elevations to 40°C were demonstrated to increase free calcium in the stroma but not in the cytosol (Lenzoni and Knight, 2019). Genetic approaches indicate that this calcium increase depends on the thylakoid Calcium-sensing receptor (CAS), already proposed to decode the calcium signal following perception of PAMPs (Nomura et al., 2012). Moreover, Finka et al. (2012) reported that two calcium channels, CNGCb from moss and CNGC2, its ortholog in Arabidopsis, act as thermosensors in plants. Disruption of CNGCb or CNGC2 produced a hyper-thermosensitive phenotype. Upon temperature elevation, the CNGC2/CNGC4 complex opens at the plasmalemma allowing the entry of calcium (Guihur et al., 2022b) (Figure 1). This complex shares features (localization, temperature increase opening, control of external calcium entry) with the animal thermosensor channel TRPV1 whose discovery was recognized by the award of the Nobel Prize in 2021 (Julius, 2013; Kefauver et al., 2020; Guihur et al., 2022a). Moreover, the recent identification of the Thermotolerance 2 (TT2) locus in rice encoding a G-subunit responsible for thermotolerance brings into light, albeit indirectly, the importance of calcium signaling in the plant response to temperature fluctuation (Kan et al., 2022). Indeed, among the downstream events linked to TT2, the sensing calcium transcription factor 1 (SCT1) interacts with calmodulin to repress target genes required for thermotolerance.
Calcium signaling actors in combined stress?
Recently, 14 common QTLs were identified among the 42 and 43 QTLs underlying the rice response to biotic or heat constraints respectively. Meta-expression analysis of the 1265 genes underlying the 14 QTLs showed that 24 genes were involved in calcium signaling, supporting its importance in plant response to both constraints (Kumar et al., 2022). However, common QTLs identified with constraints applied individually do not necessarily imply that they would also be detected when applying these constraints in combination. Indeed, transcriptome analyses, which constitute the majority of studies developed to understand the processes involved in the plant response to constraints applied in combination, showed that the plant response cannot simply result from the addition of mechanisms involved in responding to the same constraints applied individually (Suzuki et al., 2014; Pandey et al., 2015; Desaint et al., 2021). When constraints are applied in combination, few common genes are identified, their expression may be modulated differently (Rasmussen et al., 2013; Farjad et al., 2018) and depending on the severity or order of the applied constraints (Onaga et al., 2017). To date, there are only a few studies suggesting calcium signaling involvement in plant responses to combined constraints. Among these, Cao et al. (2017) showed that application of PAMPs together with salt altered calcium signal generation with an additive effect compared to the individual treatments. However, while the authors suggested this could result from activation of different calcium channels, it cannot be ruled out that this may also be associated with transcriptome changes or post-translational modifications of proteins involved in the formation of calcium-permeable channels, resulting in changed channel activity or other processes such as calcium release from other cellular compartments.
Recent studies present more convincing data that remains difficult to generalize, as they were obtained for a specific system implicating the Arabidopsis response to the combination of flg22 treatment and temperature elevation. Huot et al. (2017) showed a temperature elevation from 21°C to 28°C and 33°C increases the plants’ susceptibly and compromises PTI. Through transcriptome analysis, they proposed that the increase in plant susceptibility is associated with suppression of salicylic acid (SA) production. This inhibition appears to be independent of temperature-sensitive signaling pathways involving phytochrome B, the transcription regulators EARLY FLOWERING 3 and PHYTOCHROME INTERACTING FACTOR 4, which were previously proposed to participate in the integration of the effect of temperature on the plant and the coordination between growth and immunity (Gangappa and Kumar, 2018). In this case, it has been proposed the modulation of SA-mediated immunity relies on the CaM-binding transcription factor (TF) SR1/CAMTA3 dependent pathways, indirectly suggesting the contribution of calcium signaling (Huot et al., 2017) (Figure 1). These results, although very interesting, are surprising, since SR1/CAMTA3 is known to suppress plant immunity and promote plant development in non-stressful environments (Yuan et al., 2018). Its involvement in plant response to combined constraints therefore suggests different modes of action or regulation of this TF, such as putative modifications in its phosphorylation status. These results also support the central role of calcium in the combined responses to P. syringae and elevated temperature through suppression of SA production at 28°C. Indeed, Kim et al. (2022) demonstrated the involvement of the Calmodulin Binding Protein 60g (CBP60g) and Systemic Acquired Resistance Deficient 1 (SARD1) TF, already known to participate in PAMP-induced SA accumulation and in PTI response to P. syringae (Wang et al., 2011) (Figure 1).
Conclusions
To date, most research on plant stress physiology has been conducted by applying biotic or abiotic stresses separately, in simplified systems, under controlled conditions. By contrast, the impact of combined constraints is alarmingly poorly understood. Few studies have explored the contribution of calcium in plant responses to such condition but some results obtained by infecting plants at elevated temperature strongly support its central role in signaling following perception of multiple biotic and abiotic constraints. The scope of possible functions of calcium may arise from several molecular processes or actors such as CNGCs as calcium channels and thermosensors or calcium sensors. However, the associated mechanisms controlling its concentration fluctuation, subcellular accumulation and signaling remain to be deciphered. Therefore, understanding how calcium acts at the crossroad of biotic and abiotic constraints is relevant. Further information could be obtained by using a priori approaches (i.e genetics, comparative transcriptomics) and functional analyses of calcium toolbox players involved in combined stresses. Alternatively, genome-wide association mapping (GWA) analyses could uncover candidates, through the identification of the genetic basis underlying the natural diversity of the plants’ response to combined biotic and abiotic constraints. Indeed, such a strategy has already demonstrated its potential to identify genes underlying quantitative resistance to different pathogens (French et al., 2016; Bartoli and Roux, 2017) and in the response of plants to pathogens and temperature elevation (Aoun et al., 2017; Aoun et al., 2020; Bruessow et al., 2021). Such knowledge could provide solutions for plant breeding in order to preserve the agronomic and economic performance of crop species endangered by global change.
Author contributions
All authors listed have made a substantial, direct, and intellectual contribution to the work and approved it for publication.
Funding
SC is the recipient of a fellowship from the “École Universitaire de Recherche (EUR)” TULIP-GS (ANR-18-EURE-0019) and the Region Occitanie, Pyrénées-Méditerranée. This work was supported by the Université Paul Sabatier (Toulouse, France), the CNRS (France), the INRAE and by the Agence Nationale de la Recherche (ANR) (ANR-17-CE20-0017-01) thanks to the CaPPTure project. This study is set within the framework of the “Laboratoires d’Excellences (LABEX)” TULIP (ANR-10-LABX-41).
Acknowledgments
We thank Julie Cullimore for her comments on the manuscript and proofreading of the English text.
Conflict of interest
The authors declare that the research was conducted in the absence of any commercial or financial relationships that could be construed as a potential conflict of interest.
Publisher’s note
All claims expressed in this article are solely those of the authors and do not necessarily represent those of their affiliated organizations, or those of the publisher, the editors and the reviewers. Any product that may be evaluated in this article, or claim that may be made by its manufacturer, is not guaranteed or endorsed by the publisher.
References
Aldon, D., Mbengue, M., Mazars, C., Galaud, J. P. (2018). Calcium signaling in plant biotic interactions. Int. J. Mol. Sci. 19, 665. doi: 10.3390/IJMS19030665
Aoun, N., Desaint, H., Boyrie, L., Bonhomme, M., Deslandes, L., Berthomé, R., et al. (2020). A complex network of additive and epistatic quantitative trait loci underlies natural variation of arabidopsis thaliana quantitative disease resistance to ralstonia solanacearum under heat stress. Mol. Plant Pathol. 21, 1405–1420. doi: 10.1111/MPP.12964
Aoun, N., Tauleigne, L., Lonjon, F., Deslandes, L., Vailleau, F., Roux, F., et al. (2017). Quantitative disease resistance under elevated temperature: Genetic basis of new resistance mechanisms to ralstonia solanacearum. Front. Plant Sci. 8. doi: 10.3389/FPLS.2017.01387/BIBTEX
Atkinson, M. M., Keppler, L. D., Orlandi, E. W., Baker, C. J., Mischke, C. F. (1990). Involvement of plasma membrane calcium influx in bacterial induction of the K+/H+ and hypersensitive responses in tobacco. Plant Physiol. 92, 215–221. doi: 10.1104/PP.92.1.215
Bartoli, C., Roux, F. (2017). Genome-wide association studies in plant pathosystems: Toward an ecological genomics approach. Front. Plant Sci. 8. doi: 10.3389/FPLS.2017.00763/BIBTEX
Bi, G., Su, M., Li, N., Liang, Y., Dang, S., Xu, J., et al. (2021). The ZAR1 resistosome is a calcium-permeable channel triggering plant immune signaling. Cell 184, 3528–3541.e12. doi: 10.1016/J.CELL.2021.05.003
Blume, B., Nurnberger, T., Nass, N., Scheel, D. (2000). Receptor-mediated increase in cytoplasmic free calcium required for activation of pathogen defense in parsley. Plant Cell 12, 1425–1440. doi: 10.1105/TPC.12.8.1425
Bredow, M., Monaghan, J. (2019). Regulation of plant immune signaling by calcium-dependent protein kinases. Mol. Plant Microbe Interact. 32, 6–19. doi: 10.1094/MPMI-09-18-0267-FI
Bruessow, F., Bautor, J., Hoffmann, G., Yildiz, I., Zeier, J., Parker, J. E. (2021). Natural variation in temperature-modulated immunity uncovers transcription factor bHLH059 as a thermoresponsive regulator in arabidopsis thaliana. PloS Genet. 17, e1009290. doi: 10.1371/JOURNAL.PGEN.1009290
Cao, X. Q., Jiang, Z. H., Yi, Y. Y., Yang, Y., Ke, L. P., Pei, Z. M., et al. (2017). Biotic and abiotic stresses activate different Ca2+ permeable channels in arabidopsis. Front. Plant Sci. 8. doi: 10.3389/FPLS.2017.00083
Chaloner, T. M., Gurr, S. J., Bebber, D. P. (2021). Plant pathogen infection risk tracks global crop yields under climate change. Nat. Clim. Change 11, 710–715. doi: 10.1038/s41558-021-01104-8
Delk, N. A., Johnson, K. A., Chowdhury, N. I., Braam, J. (2005). CML24, regulated in expression by diverse stimuli, encodes a potential Ca2+ sensor that functions in responses to abscisic acid, daylength, and ion stress. Plant Physiol. 139, 240–253. doi: 10.1104/pp.105.062612
Delplace, F., Huard-Chauveau, C., Berthomé, R., Roby, D. (2022). Network organization of the plant immune system: from pathogen perception to robust defense induction. Plant J. 109, 447–470. doi: 10.1111/TPJ.15462
Desaint, H., Aoun, N., Deslandes, L., Vailleau, F., Roux, F., Berthomé, R. (2021). Fight hard or die trying: when plants face pathogens under heat stress. New Phytol. 229, 712–734. doi: 10.1111/NPH.16965
Farjad, M., Rigault, M., Pateyron, S., Martin-Magniette, M. L., Krapp, A., Meyer, C., et al. (2018). Nitrogen limitation alters the response of specific genes to biotic stress. Int. J. Mol. Sci. 19, 3364. doi: 10.3390/IJMS19113364
Finka, A., Cuendet, A. F. H., Maathuis, F. J. M., Saidi, Y., Goloubinoff, P. (2012). Plasma membrane cyclic nucleotide gated calcium channels control land plant thermal sensing and acquired thermotolerance. Plant Cell 24, 3333–3348. doi: 10.1105/TPC.112.095844
French, E., Kim, B. S., Iyer-Pascuzzi, A. S. (2016). Mechanisms of quantitative disease resistance in plants. Semin. Cell Dev. Biol. 56, 201–208. doi: 10.1016/J.SEMCDB.2016.05.015
Gangappa, S. N., Kumar, S. V. (2018). DET1 and COP1 modulate the coordination of growth and immunity in response to key seasonal signals in arabidopsis. Cell Rep. 25, 29–37. doi: 10.1016/J.CELREP.2018.08.096
Gong, M., van der Luit, A. H., Knight, M. R., Trewavas, A. J. (1998). Heat-shock-induced changes in intracellular Ca2+ level in tobacco seedlings in relation to thermotolerance. Plant Physiol. 116, 429–437. doi: 10.1104/PP.116.1.429
Guihur, A., Rebeaud, M. E., Bourgine, B., Goloubinoff, P. (2022a). How do humans and plants feel the heat? Trends Plant Sci. 27, 630–632. doi: 10.1016/j.tplants.2022.03.006
Guihur, A., Rebeaud, M. E., Goloubinoff, P. (2022b). How do plants feel the heat and survive? Trends Biochem. Sci. 47, 824–838. doi: 10.1016/J.TIBS.2022.05.004
Heyer, M., Scholz, S. S., Reichelt, M., Kunert, G., Oelmüller, R., Mithöfer, A. (2021). The Ca2+ sensor proteins CML37 and CML42 antagonistically regulate plant stress responses by altering phytohormone signals. Plant Mol. Biol. 109, 611–625. doi: 10.1007/S11103-021-01184-2
Huot, B., Castroverde, C. D. M., Velásquez, A. C., Hubbard, E., Pulman, J. A., Yao, J., et al. (2017). Dual impact of elevated temperature on plant defence and bacterial virulence in arabidopsis. Nat. Commun. 8, 1–12. doi: 10.1038/s41467-017-01674-2
IPCC (2021). “Summary for policymakers,” in Climate change 2021: The physical science basis. contribution of working group I to the sixth assessment report of the intergovernmental panel on climate change. Eds. Masson-Delmotte, V., Zhai, P., Pirani, A., Connors, S. L., Péan, C., Berger, S., Caud, N., Chen, Y., Goldfarb, L., Gomis, M. I., Huang, M., Leitzell, K., Lonnoy, E., Matthews, J. B. R., Maycock, T. K., Waterfield, T., Yelekçi, O., Yu, R., Zhou, B. (Cambridge University Press). Available at: https://www.ipcc.ch/report/ar6/wg1/.
Jones, J. D. G., Dangl, J. L. (2006). The plant immune system. Nature 444, 323–329. doi: 10.1038/nature05286
Julius, D. (2013). TRP channels and pain. Annu. Rev. Cell. Dev. Biol. 29, 355–384. doi: 10.1146/annurev-cellbio-101011-155833
Kan, Y., Mu, X. R., Zhang, H., Gao, J., Shan, J. X., Ye, W. W., et al. (2022). TT2 controls rice thermotolerance through SCT1-dependent alteration of wax biosynthesis. Nat. Plants 8, 53–67. doi: 10.1038/S41477-021-01039-0
Kefauver, J. M., Ward, A. Z. B., Patapoutian, A. (2020). Discoveries in structure and physiology of mechanically activated ion channels. Nature 587, 567–576. doi: 10.1038/s’1586-020-2933-1
Kim, J. H., Castroverde, C. D. M., Huang, S., Li, C., Hilleary, R., Seroka, A., et al. (2022). Increasing the resilience of plant immunity to a warming climate. Nature 607, 339–344. doi: 10.1038/s41586-022-04902-y
Knight, M. R., Campbell, A. K., Smith, S. M., Trewavas, A. J. (1991). Transgenic plant aequorin reports the effects of touch and cold-shock and elicitors on cytoplasmic calcium. Nature 352, 524–526. doi: 10.1038/352524a0
Köster, P., DeFalco, T. A., Zipfel, C. (2022). Ca2+ signals in plant immunity. EMBO J. 41, e110741. doi: 10.15252/EMBJ.2022110741
Kumar, R., Bahuguna, R. N., Tiwari, M., Pal, M., Chinnusamy, V., Sreeman, S., et al. (2022). Walking through crossroads–rice responses to heat and biotic stress interactions. Theor. Appl. Genet., 1–17. doi: 10.1007/S00122-022-04131-X
Leba, L. J., Cheval, C., Ortiz-Martín, I., Ranty, B., Beuzón, C. R., Galaud, J. P., et al. (2012). CML9, an arabidopsis calmodulin-like protein, contributes to plant innate immunity through a flagellin-dependent signaling pathway. Plant J. 71, 976–989. doi: 10.1111/J.1365-313X.2012.05045.X
Lecourieux, D., Ranjeva, R., Pugin, A. (2006). Calcium in plant defence-signalling pathways. New Phytol. 171, 249–269. doi: 10.1111/J.1469-8137.2006.01777.X
Lenzoni, G., Knight, M. R. (2019). Increases in absolute temperature stimulate free calcium concentration elevations in the chloroplast. Plant Cell Physiol. 60, 538–548. doi: 10.1093/pcp/pcpy227
Liu, J., Lenzoni, G., Knight, M. R. (2020). Design principle for decoding calcium signals to generate gene expression via transcription. Plant Physiol. 182, 1743–1761. doi: 10.1104//pp.19.01003
Luan, S., Wang, C. (2021). Calcium signaling mechanisms across kingdoms. Annu. Rev. Cell Dev. Biol. 37, 311–340. doi: 10.1146/annurev-cellbio-120219-035210
Magnan, F., Ranty, B., Charpenteau, M., Sotta, B., Galaud, J. P., Aldon, D. (2008). Mutations in AtCML9, a calmodulin-like protein from arabidopsis thaliana, alter plant responses to abiotic stress and abscisic acid. Plant J. 56, 575–589. doi: 10.1111/J.1365-313X.2008.03622.X
Ma, X., Li, Q. H., Yu, Y. N., Qiao, Y. M., Haq, S. U., Gong, Z. H. (2020). The CBL–CIPK pathway in plant response to stress signals. Int. J. Mol. Sci. 21, 5668. doi: 10.3390/IJMS21165668
Ma, W., Smigel, A., Tsai, Y. C., Braam, J., Berkowitz, G (2008). Innate immunity signaling: cytosolic Ca2+ elevation is linked to downstream nitric oxide generation through the action of calmodulin or a calmodulin-like protein A. Plant Physiol. 148, 818–828. doi: 10.1104/PP.108.125104
McDonald, B. A., Stukenbrock, E. V. (2016). Rapid emergence of pathogens in agro-ecosystems: global threats to agricultural sustainability and foss security. Philos. Trans. R. Soc B. Biol. Sci. 371, 20160026. doi: 10.1098/rstb.2016.0026
Navazio, L., Formentin, E., Cendron, L., Szabo, I. (2020). Chloroplast calcium signaling in the spotlight. Front. Plant Sci. 11. doi: 10.3389/fpls.2020.00186
Ngou, B. P. M., Ahn, H. K., Ding, P., Jones, J. D. G. (2021). Mutual potentiation of plant immunity by cell-surface and intracellular receptors. Nature 592, 110–115. doi: 10.1038/s41586-021-03315-7
Nomura, H., Komori, T., Uemura, S., Kanda, Y., Shimotani, K., Nakai, K., et al. (2012). Chloroplast-mediated activation of plant immune signaling in arabidopsis. Nat. Commun. 3, 926. doi: 10.1038/natcomms1926
Onaga, G., Wydra, K., Koopmann, B., Chebotarov, D., Séré, Y., Von Tiedemann, A. (2017). High temperature effects on Pi54 conferred resistance to magnaporthe oryzae in two genetic backgrounds of oryza sativa. J. Plant Physiol. 212, 80–93. doi: 10.1016/J.JPLPH.2017.02.004
Pandey, P., Ramegowda, V., Senthil-Kumar, M. (2015). Shared and unique responses of plants to multiple individual stresses and stress combinations: Physiological and molecular mechanisms. Front. Plant Sci. 6. doi: 10.3389/FPLS.2015.00723/BIBTEX
Patra, N., Hariharan, S., Gain, H., Maiti, M. K., Das, A., Banerjee, J. (2021). TypiCal but DeliCate ca++re: dissecting the essence of calcium signaling network as a robust response coordinator of versatile abiotic and biotic stimuli in plants. Front. Plant Sci. 12. doi: 10.3389/FPLS.2021.752246
Ranty, B., Aldon, D., Cotelle, V., Galaud, J. P., Thuleau, P., Mazars, C. (2016). Calcium sensors as key hubs in plant responses to biotic and abiotic stresses. Front. Plant Sci. 7. doi: 10.3389/FPLS.2016.00327
Rasmussen, S., Barah, P., Suarez-Rodriguez, M. C., Bressendorff, S., Friis, P., Costantino, P., et al. (2013). Transcriptome responses to combinations of stresses in arabidopsis. Plant Physiol. 161, 1783–1794. doi: 10.1104/PP.112.210773
Scholz, S. S., Reichelt, M., Vadassery, J., Mithöfer, A. (2015). Calmodulin-like protein CML37 is a positive regulator of ABA during drought stress in arabidopsis. Plant Signal Behav. 10, e1011951. doi: 10.1080/15592324.2015.1011951
Scholz, S. S., Vadassery, J., Heyer, M., Reichelt, M., Bender, K. W., Snedden, W. A., et al. (2014). Mutation of the arabidopsis calmodulin-like protein CML37 deregulates the jasmonate pathway and enhances susceptibility to herbivory. Mol. Plant 12, 1712–1726. doi: 10.1093/mp/ssu102
Shen, L., Yang, S., Guan, D., He, S. (2020). CaCML13 acts positively in pepper immunity against ralstonia solanacearum infection forming feedback loop with CabZIP63. Int. J. Mol. Sci. 21, 4186. doi: 10.3390/IJMS21114186
Shi, S., Li, S., Asim, M., Mao, J., Xu, D., Ullah, Z., et al. (2018). The arabidopsis calcium-dependent protein kinases (CDPKs) and their roles in plant growth regulation and abiotic stress responses. Int. J. Mol. Sci. 19, 1900. doi: 10.3390/IJMS19071900
Suzuki, N., Rivero, R. M., Shulaev, V., Blumwald, E., Mittler, R. (2014). Abiotic and biotic stress combinations. New Phytol. 203, 32–43. doi: 10.1111/NPH.12797
Tang, R. J., Wang, C., Li, K., Luan, S. (2020). The CBL-CIPK calcium signaling network: unified paradigm from 20 years of discoveries. Trends Plant Sci. 25, 604617. doi: 10.1016/j.tplants.2020.01.009
Tian, W., Hou, C., Ren, Z., Wang, C., Zhao, F., Dahlbeck, D., et al. (2019). A calmodulin-gated calcium channel links pathogen patterns to plant immunity. Nature 572, 131–135. doi: 10.1038/S41586-019-1413-Y
Tsai, Y. C., Delk, N. A., Chowdhury, N. I., Braam, J. (2007). Arabidopsis potential calcium sensors regulate nitric oxide levels and the transition to flowering. Plant Signal Behav. 2, 446–454. doi: 10.4161/psb.2.6.4695
Vadassery, J., Reichelt, M., Hause, B., Gershenzon, J., Boland, W., Mithöfer, A. (2012). CML42-mediated calcium signaling coordinates responses to spodoptera herbivory and abiotic stresses in arabidopsis. Plant Physiol. 159, 1159–1175. doi: 10.1104/PP.112.198150
Velásquez, A. C., Castroverde, C. D. M., He, S. Y. (2018). Plant pathogen warfare under changing climate conditions. Curr. Biol. 28, 619–634. doi: 10.1016/j.cub.2018.03.054
Wang, J., Liu, X., Zhang, A., Ren, Y., Wu, F., Wang, G., et al. (2019). A cyclic nucleotide-gated channel mediates cytoplasmic calcium elevation and disease resistance in rice. Cell Res. 29, 820–831. doi: 10.1038/S41422-019-0219-7
Wang, L., Tsuda, K., Truman, W., Sato, M., Nguyen, L. V., Katagiri, F., et al. (2011). CBP60g and SARD1 play partially redundant critical roles in salicylic acid signaling. Plant J. 67, 1029–1041. doi: 10.1111/J.1365-313X.2011.04655.X
Yip Delormel, T., Boudsocq, M. (2019). Properties and functions of calcium-dependent protein kinases and their relatives in arabidopsis thaliana. New Phytol. 224, 585–604. doi: 10.1111/NPH.16088
Yuan, P., Du, L., Poovaiah, B. W. (2018). Ca2+/Calmodulin-dependent AtSR1/CAMTA3 plays critical roles in balancing plant growth and immunity. Int. J. Mol. Sci. 19, 1764. doi: 10.3390/IJMS19061764
Yuan, M., Jiang, Z., Bi, G., Nomura, K., Liu, M., Wang, Y., et al. (2021a). Pattern-recognition receptors are required for NLR-mediated plant immunity. Nature 592, 105–109. doi: 10.1038/s41586-021-03316-6
Yuan, M., Ngou, B. P. M., Ding, P., Xin, X. F. (2021b). PTI-ETI crosstalk: an integrative view of plant immunity. Curr. Opin. Plant Biol. 62, 102030. doi: 10.1016/J.PBI.2021.102030
Yuan, P., Poovaiah, B. W. (2022). Interplay between Ca2+/Calmodulin-mediated signaling and AtSR1/CAMTA3 during increased temperature resulting in compromised immune response in plants. Int. J. Mol. Sci. 23, 2175. doi: 10.3390/IJMS23042175
Zhang, J., Zou, A., Wen, Y., Wei, X., Liu, C., Lu, X., et al. (2022). SlCML55, a novel solanum lycopersicum calmodulin-like gene, negatively regulates plant immunity to phytophthora pathogens. Sci. Hortic. (Amsterdam) 299, 111049. doi: 10.1016/J.SCIENTA.2022.111049
Zhu, X., Dunand, C., Snedden, W., Galaud, J. P. (2015). CaM and CML emergence in the green lineage. Trends Plant Sci. 20, 483–489. doi: 10.1016/j.tplants.2015.05.010
Zhu, X., Mazard, J., Robe, E., Pignoly, S., Aguilar, M., Clemente, H. S., et al. (2021). The same against many: AtCML8, a Ca2+ sensor acting as a positive regulator of defense responses against several plant pathogens. Int. J. Mol. Sci. 22, 10469. doi: 10.3390/IJMS221910469
Zhu, X., Robe, E., Jomat, L., Aldon, D., Mazars, C., Galaud, J. P. (2017). CML8, an arabidopsis calmodulin-like protein, plays a role in pseudomonas syringae plant immunity. Plant Cell Physiol. 58, 307–319. doi: 10.1093/PCP/PCW189
Keywords: biotic stress, calcium channels, calcium sensors, calcium signaling, combined stress, temperature elevation, thermosensors
Citation: Carpentier S, Aldon D, Berthomé R and Galaud J-P (2022) Is there a specific calcium signal out there to decode combined biotic stress and temperature elevation? Front. Plant Sci. 13:1004406. doi: 10.3389/fpls.2022.1004406
Received: 27 July 2022; Accepted: 20 October 2022;
Published: 03 November 2022.
Edited by:
Meral Tunc-Ozdemir, American College of Healthcare Sciences, United StatesReviewed by:
Mona F. A. Dawood, Assiut University, EgyptWusirika Ramakrishna, Central University of Punjab, India
Takuya Furuichi, Hagoromo International University, Japan
Junli Liu, Durham University, United Kingdom
Anna Coll, National Institute of Biology (NIB), Slovenia
Abdul Azeez, Washington State University, United States
Lorella Navazio, University of Padua, Italy
Copyright © 2022 Carpentier, Aldon, Berthomé and Galaud. This is an open-access article distributed under the terms of the Creative Commons Attribution License (CC BY). The use, distribution or reproduction in other forums is permitted, provided the original author(s) and the copyright owner(s) are credited and that the original publication in this journal is cited, in accordance with accepted academic practice. No use, distribution or reproduction is permitted which does not comply with these terms.
*Correspondence: Jean-Philippe Galaud, jean-philippe.galaud@univ-tlse3.fr; Richard Berthomé, richard.berthome@inrae.fr