- 1Department of Agronomy, University of Agriculture Faisalabad, Faisalabad, Pakistan
- 2College of Life Sciences, Yan’an University, Yan’an, China
- 3Department of Botany, University of Agriculture Faisalabad, Faisalabad, Pakistan
- 4Agronomic Research Institute, Ayub Agricultural Research Institute, Faisalabad, Pakistan
- 5College of Resources and Environmental Sciences, Gansu Agricultural University, Lanzhou, China
- 6Department of Soil and Environmental Science, College of Agriculture, University of Sargodha, Sargodha, Pakistan
- 7Institute of Soil and Environmental Science, University of Agriculture Faisalabad, Faisalabad, Pakistan
- 8Institute of Chemistry and Technology of Environmental Protection, Faculty of Chemistry, Brno University of Technology, Brno, Czechia
- 9Department of Agrochemistry, Soil Science, Microbiology and Plant Nutrition, Faculty of AgriSciences, Mendel University in Brno, Brno, Czechia
- 10Institute for Environmental Studies, Faculty of Science, Charles University in Prague, Prague, Czechia
Cadmium (Cd) is a major environmental contaminant due to its widespread industrial use. Cd contamination of soil and water is rather classical but has emerged as a recent problem. Cd toxicity causes a range of damages to plants ranging from germination to yield suppression. Plant physiological functions, i.e., water interactions, essential mineral uptake, and photosynthesis, are also harmed by Cd. Plants have also shown metabolic changes because of Cd exposure either as direct impact on enzymes or other metabolites, or because of its propensity to produce reactive oxygen species, which can induce oxidative stress. In recent years, there has been increased interest in the potential of plants with ability to accumulate or stabilize Cd compounds for bioremediation of Cd pollution. Here, we critically review the chemistry of Cd and its dynamics in soil and the rhizosphere, toxic effects on plant growth, and yield formation. To conserve the environment and resources, chemical/biological remediation processes for Cd and their efficacy have been summarized in this review. Modulation of plant growth regulators such as cytokinins, ethylene, gibberellins, auxins, abscisic acid, polyamines, jasmonic acid, brassinosteroids, and nitric oxide has been highlighted. Development of plant genotypes with restricted Cd uptake and reduced accumulation in edible portions by conventional and marker-assisted breeding are also presented. In this regard, use of molecular techniques including identification of QTLs, CRISPR/Cas9, and functional genomics to enhance the adverse impacts of Cd in plants may be quite helpful. The review’s results should aid in the development of novel and suitable solutions for limiting Cd bioavailability and toxicity, as well as the long-term management of Cd-polluted soils, therefore reducing environmental and human health hazards.
Introduction
The presence of organic and inorganic pollutants in the environment leads to its deterioration, which has become a grave issue and is threatening the global ecosystem (Zulfiqar et al., 2019; Zeeshan et al., 2021). Enrichment of soil with toxic heavy metals such as cadmium (Cd), lead (Pb), arsenic (As), nickel (Ni), mercury (Hg), and chromium (Cr) causes serious hazards to plant life and human health. These potentially toxic elements are present at low concentrations in the environment (Palansooriya et al., 2020). High levels of these toxic metals are harmful to humans, plants, and animals (but not exceptionally) because of their persistent nature in the environment (Afzal et al., 2019). Cd is one of the most toxic heavy metals to living organisms (Chellaiah, 2018; Zulfiqar et al., 2021). Cd is an element ranked 7th in the list of 20 most toxic metals and classified as group 1 carcinogen (Jaishankar et al., 2014). It is one of the most perilous metals owing to its high toxicity and serious extent of bioaccumulation (Singh et al., 2020; Qianqian et al., 2022). Cd toxicity adversely affects the human body, and it accumulates in the kidneys and causes emphysema, renal tubular damage, and kidney stones (Mahajan and Kaushal, 2018). In minerals, it replaces calcium owing to similar charge, ionic radius, and chemical behavior (Kubier et al., 2019). Therefore, it can easily be transferred and stored in the human body (Hajeb et al., 2014). Cd toxicity causes severe liver damage and reduces the supply of calcium in the body (Lata et al., 2019). Moreover, Cd directly influences the regulation of Zn and Fe via ZIP, and NRAMP (Tanveer and Shabala, 2022). It is released to the environment through natural as well as anthropogenic systems. Among natural systems, weathering of Cd-containing rocks, forest fires, volcanic eruptions, and wastewater are the principal means (Liu et al., 2013; Manzoor et al., 2019). Anthropogenic activities are a source of Cd contamination, mainly including metallurgical works, mining, electroplating, paints, combustion emissions, and excessive use of fertilizers and pesticides (Singh et al., 2020; Haider et al., 2021a; Figure 1). It is readily soluble, and it is mobile compared to other metals; therefore, it is quickly taken by plants (Song et al., 2015). After uptake, Cd is translocated and accumulated in edible parts of plants (Adil et al., 2020).
Cadmium toxicity adversely affects plants by inhibiting carbon fixation and reducing chlorophyll synthesis and photosynthetic activity (Liu et al., 2018). Exposure to Cd causes physiochemical, morphological, and structural changes in plants such as chlorosis and inhibition of lateral root formation and stomatal density (Bari et al., 2019; Huybrechts et al., 2020). It induces osmotic stress in plants by reducing leaf relative water content, stomatal conductance, and transpiration (Rizwan et al., 2016). It also has harmful effects on uptake and transportation of mineral elements, resulting in yield decline (Chen et al., 2018a,b,c). Cd toxicity causes overproduction of reactive oxygen species (ROS) and results in damage to plant membranes and destruction of cell organelles (Abbas et al., 2017). Cd is a very toxic heavy metal that adversely affects a variety of physiological functions leading to stunted growth with ultimate yield penalty on field crops.
The bioavailability and toxicity of Cd depend on physical and chemical properties of soil (Violante et al., 2010). With decrease in soil pH, Cd is transformed from a fixed form to a readily mobile form that enhances its availability for plant uptake (Mondal et al., 2020). Therefore, understanding the physical and chemical properties of soil and the dynamics of Cd in soil is essential for reducing the toxicity caused by Cd. This review presents an overview of the adverse effects of Cd toxicity on plants, ultrastructural and oxidative damage, carbon metabolism, and yield formation. The dynamics of Cd in the rhizosphere, and soil factors affecting soil uptake are also discussed. Moreover, potential remediation strategies such as physical, chemical, and biological methods to decontaminate Cd from polluted soils are also highlighted. Furthermore, the use of different forms of organic materials and molecular techniques to reduce Cd uptake and accumulation are described.
Cadmium Dynamics in Soil and the Rhizosphere
Biologically, Cd is not important for plants; however, it is easily acquired by plants because of micronutrients from the rhizosphere of soils (the soil-root interface) (Shahid et al., 2016). The presence of Cd has been observed in soils ranging between 0.07 to 1.1 mg kg–1 soil (World Health Organization [WHO], 2007). However, threshold level is approximately 100 mg kg–1 in agricultural soils (Asgher et al., 2015).
Cadmium (Cd) is primarily present as Cd ions or forming complexes, i.e., organic and inorganic in the soil solution. Both anionic and cationic forms of Cd exist in soils (Kabata-Pendias and Sadurski, 2004). Anionic forms are CdCl3–, Cd(OH)3–, Cd(OH)42–, and Cd(HS)42–, while cationic forms are CdCl+, CdOH+, CdHS+, and CdHCO3+. It has been found that 99% Cd is present in the soil solution as a free ionic form (Kabata-Pendias, 1993).
Several chemical reactions, namely, dissolution/precipitation, desorption/adsorption, and Cd ligand formation, affect the partitioning of Cd in soils. These processes are mainly influenced by ligands (organic and inorganic) (Shahid et al., 2014), redox conditions (Zhang et al., 2012), soil pH (Saeki and Kunito, 2012), metal contents, and temperature (Silber et al., 2012). Partitioning of Cd is vital in soil systems for regulation of Cd toxicity (Rizwan et al., 2017). Cd biogeochemical behavior depends on the concentration of free Cd ions in a soil medium (Shahid et al., 2016). Accumulation of Cd in plant root varies with Cd contents in the rhizosphere and plant type. Maize (Zea mays L.) showed more Cd accumulation in cell wall fraction than broad bean (Vicia faba L.) seedlings (Lozano-Rodriguez et al., 1997).
Factors Affecting Cadmium Dynamics
Several factors like soil pH, cation exchange capacity (CEC), organic matter, microbial activities in the soil, and root exudates influence the bioavailability of Cd (Jung, 2008; Shahid et al., 2016). One of the crucial factors in the regulation of Cd partitioning and its bioavailability is soil pH (Yu et al., 2016). Cd exists in various chemical forms at varying soil pH levels. It has been observed that Cd solubility in the soil solution is primarily affected by acidic soil conditions. A change in Cd from immobile forms like carbonates and Mn and Fe oxides to better exchangeable forms allow free Cd phytoavailability and mobility (Qi et al., 2018). For the solubility of Cd in soil, pH 6 acts as a threshold point because of complex formation with organic matter and its adsorption on mineral surfaces (Sullivan et al., 2013). On the other hand, rise in pH increases its alkalinity, affecting Cd adsorption into soil particles. Yu et al. (2016) described that soil pH played a key role in acclimatization of Cd in rice grains. Enhanced soil pH imparts a negative influence on phytoavailability, as adsorption and precipitation of Cd decrease free Cd availability in the soil solution (Meng et al., 2018).
The bioavailability of Cd is influenced by soil organic matter (SOM) because of formation of various complexes with Cd in the soil solution. The bioavailability of Cd depends on SOM source, concentration, and chemical forms. In addition, SOM has a direct influence on Cd binding and its acclimatization. Kirkham (2006) reported that higher SOM causes more sorption potential, which is 30 times more than mineral soil. Biochar application greater than 10% reduces the bioavailability of Cd in plants through its immobilization in soil (Xiao et al., 2019). In another study, the application of biochar decreased Cd stress in wheat (Triticum aestivum L.) by reducing its bioavailability (Abbas et al., 2018). On the contrary, Yousaf et al. (2016) depicted that SOM content and uptake of Cd increased in wheat predominantly because of application of poultry manure, sewage sludge, and farmyard manure.
Cation exchange capacity of the soil strongly influences the mobility and bioavailability of Cd. In a study, binding of Cd to exchangeable and acid-soluble fractions occurred in loamy and loamy sand soils having small Cd contents, and was found to be related to SOM. However, Cd was bound to a reducible fraction followed by an exchangeable acid-soluble fraction in silt-clay soil (Gusiatin and Klimiuk, 2012). Hong et al. (2002) reported less Cd mobility due to its strong affinity with clay mineral surface, Fe–Al oxides, and humus in clayey soils. The bioavailability Cd is directly influenced by the occurrence of mineral ions in the soil solution. This is directly related to ionic strength, competition, and complexation for root or soil exchange sites. Additionally, there is an inverse relationship between ionic strength and bioavailability, as Cd extraction by plants is enhanced because of less ionic strength in growth media (Gothberg et al., 2004).
Soil microbial activity is found to enhance the availability of Cd through organic acid secretion and succeeding solubilization of Cd-bearing minerals (Ahmad et al., 2015). Soil amendments having Cd-solubilizing microbes like plant growth-promoting rhizobacteria (PGPRs) play an essential role in enhancing the bioavailability of Cd (Wu et al., 2020). In a study, Sangthong et al. (2016) depicted the ability of Micrococcus sp. TISTR2221 to modulate more uptake of Cd in the root and stem parts of maize plant under Cd stress. On the other hand, to reduce the toxicity of Cd in plants, microbes like PGPRs and arbuscular mycorrhizal fungi play a crucial role in restricting the uptake of Cd in roots. Reduction in Cd phytoavailability was found by soil bioaugmentation, causing immobilized and free Cd-resistant bacteria and fungi in the rhizosphere (Sharma and Archana, 2016).
Root exudates also impart a role in sequestration and binding of Cd in soils and protect plant roots from Cd toxicity in soils (Liao and Xie, 2004). Furthermore, Cd uptake is minimized by root exudates in plants (Sarwar et al., 2010). Factors affecting Cd dynamics are presented in Figure 2.
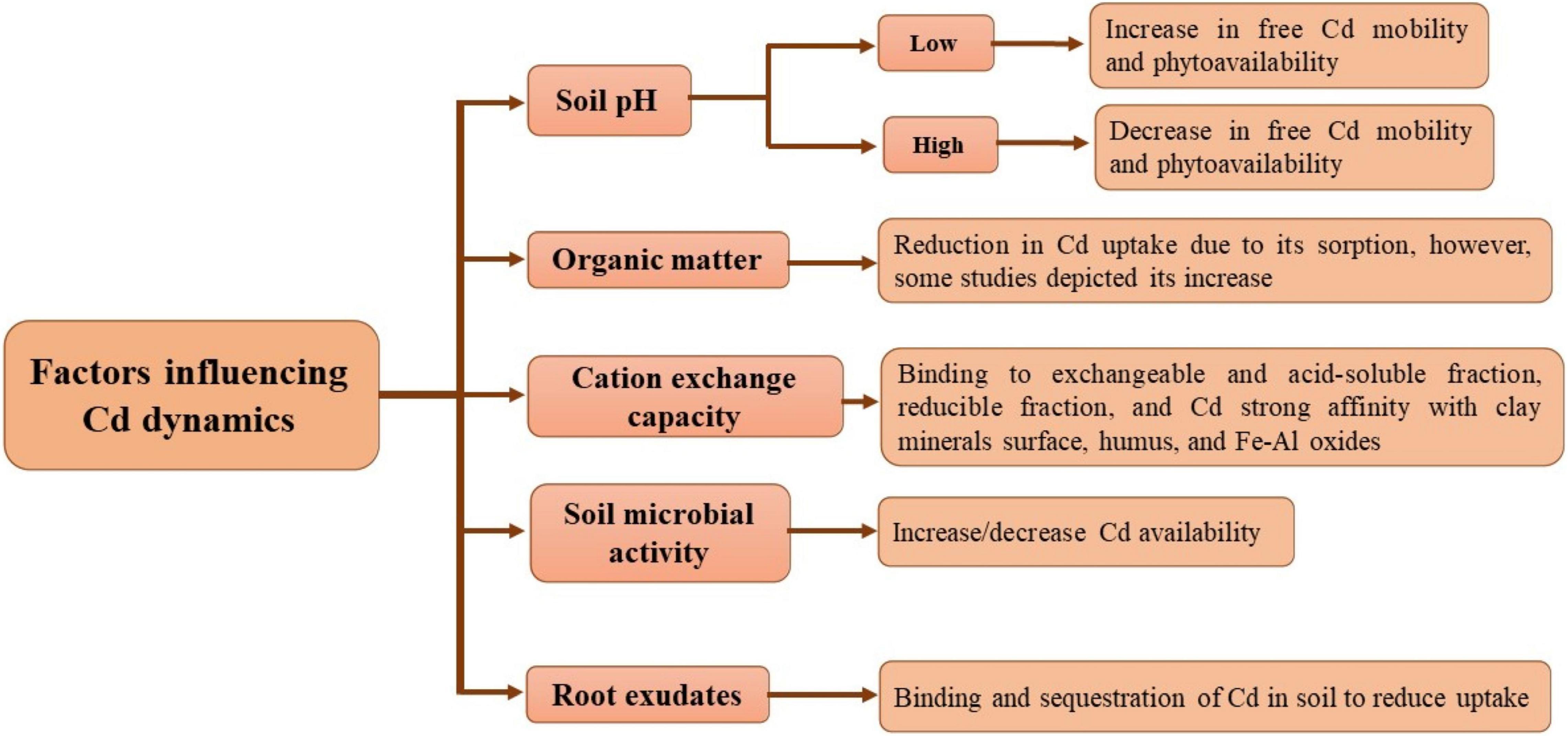
Figure 2. Factors affecting Cd dynamics in soils (conceived from Shahid et al., 2016).
Toxic Impacts of Cadmium on Plants
Impact of Cadmium Toxicity on Plant Growth and Yield Formation
Cadmium accumulation in agricultural soils has become a primary concern of scientific factions because of Cd’s increasing concentration, which substantially reduces plant growth and yield (Romero-Puertas et al., 2004; Goix et al., 2014; Zhang et al., 2014). Cd toxicity in soils causes several harmful impacts on plants, i.e., overproduction of oxidative markers like ROS, free radicals, and lipid peroxidation which induces oxidative stress and ultimately causes reduction in the yield of crops (Qayyum et al., 2017; Hussain et al., 2018). Therefore, it is a necessity for the current era to overcome Cd toxicity for better health of humans and plants. To overcome the toxic impacts of Cd, following strategies could be useful such as introduction of plant species that can phytoremediate heavy metals by sequestration of the metals in their vegetative parts and avoidance of the introduction of these heavy metals to plants by control of anthropogenic activities and implementation of lawful strategies of countries and governments.
Ultrastructural Changes Due to Cadmium Toxicity
Plants exhibit a different response when exposed to varying concentrations of Cd. Anatomical abnormalities mainly depend on plant species, exposure duration, uptake amount, sequestration, and localization in different parts (Shah et al., 2019). Cadmium is phloem-mobile and localized in any part of plants; it leads to reduction in biomass and yield; it causes chlorosis and even leaf fall that contradicts normal plant movements (Gallego et al., 2012).
Cadmium exposure causes considerable anatomical alterations in roots, stems, and leaves of Ceratopteris pteridoides. These alterations include closure of abaxial stomata, stomatal size reduction in leaves, scarification in tracheid walls, narrow xylem vessels, and disorganization in vascular bundles in roots and stems (Bora and Sarma, 2021). Trichome length, abaxial and adaxial density of stomata, and proportion of cortex were decreased in Trigonella foenum under Cd stress (Ahmad et al., 2005). Heterogeneity in vascular tissues of stems and leaves occurs in Arundo donax L. when exposed to Cd stress (Guo and Miao, 2010). Plants exposed to Cd cause severe impacts by reducing the size of parenchyma in leaves, disrupting the ultrastructure of chloroplasts, disorganizing vascular s organization, reducing epidermal tissue thickness, and exhibiting narrow xylem and phloem vessels. Heavy metal-tolerant plants can induce a plethora of mechanisms to reduce the noxious impacts of heavy metals by modification of microstructures. These modifications include an efficient vascular system by increasing a vascular bundle area for better translocation of water and food. These plants also develop a thick epidermis to conserve plenty of water in their bodies, which is covered with a waxy cuticle layer, and sequester a large amount of water in roots and shoots to prevent from translocation into the leaves. This prevention in uptake could modify the photosynthetic apparatus of plants. Some heavy metal-tolerant species also exhibit various avoidance mechanisms to prevent the entrance of heavy metals into roots. Effects of Cd on the ultrastructure of roots, stems, and leaves of different plant species are illustrated in Table 1.
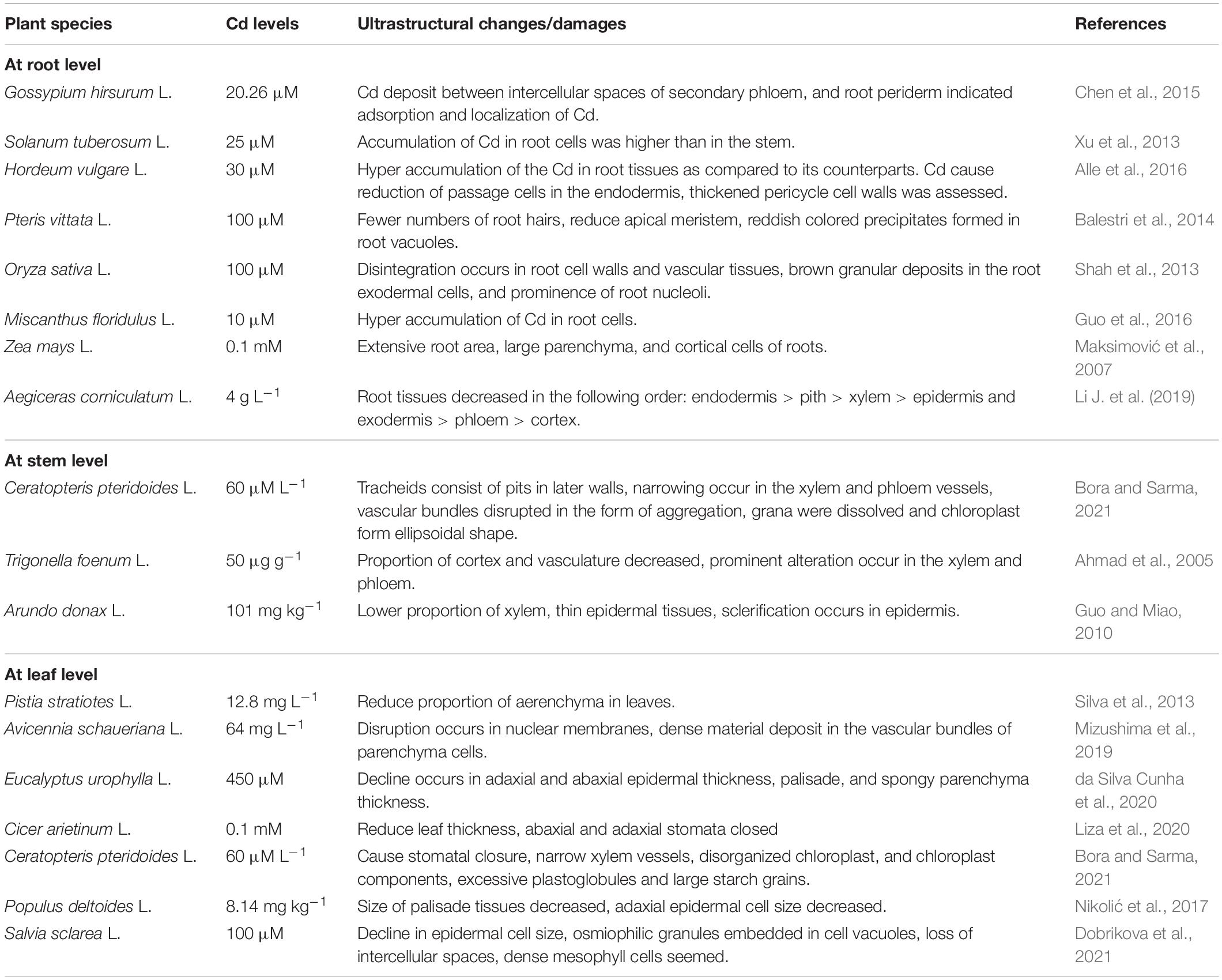
Table 1. Ultrastructural/anatomical damages in different plant species due to cadmium (Cd) toxicity.
Oxidative Damages Due to Cadmium Toxicity
Cadmium, like other HMs, induces oxidative damages by producing excessive H2O2 and lipid peroxidation in plants (Rizwan et al., 2019a; Shiyu et al., 2020; Yang et al., 2020; Figure 3). It is well-documented that Cd regimes induce the production of ROS (H2O2, O–2); these scavenge antioxidant enzymes (Kapoor et al., 2019; Hasanuzzaman et al., 2020; Unsal et al., 2020). Several studies suggest that Cd does not directly participate in ROS production but induces temporal oxidative damage to plants (Cuypers et al., 2010, 2016). Cellular ROS mainly comprise both free radicals and non-radicals (Hasanuzzaman et al., 2020). Free radicals include O2 •−, •OH, RO•, peroxyl radical (ROO•) and non-radicals, H2O2, 1O2, and ozone (O3) (Farooq et al., 2019; Maurya, 2020), while other non-radicals that exist in plants are excited carbonyls, hypochlorous acid (HOCl) and hydroperoxides (ROOH) (Kapoor et al., 2015). Because of oxidative damage, accumulation of thiobarbituric acid reactive substances (TBARSs) and malondialdehyde (MDA) occurs in, and results in electrolyte leakage under Cd stress (Younis et al., 2016).
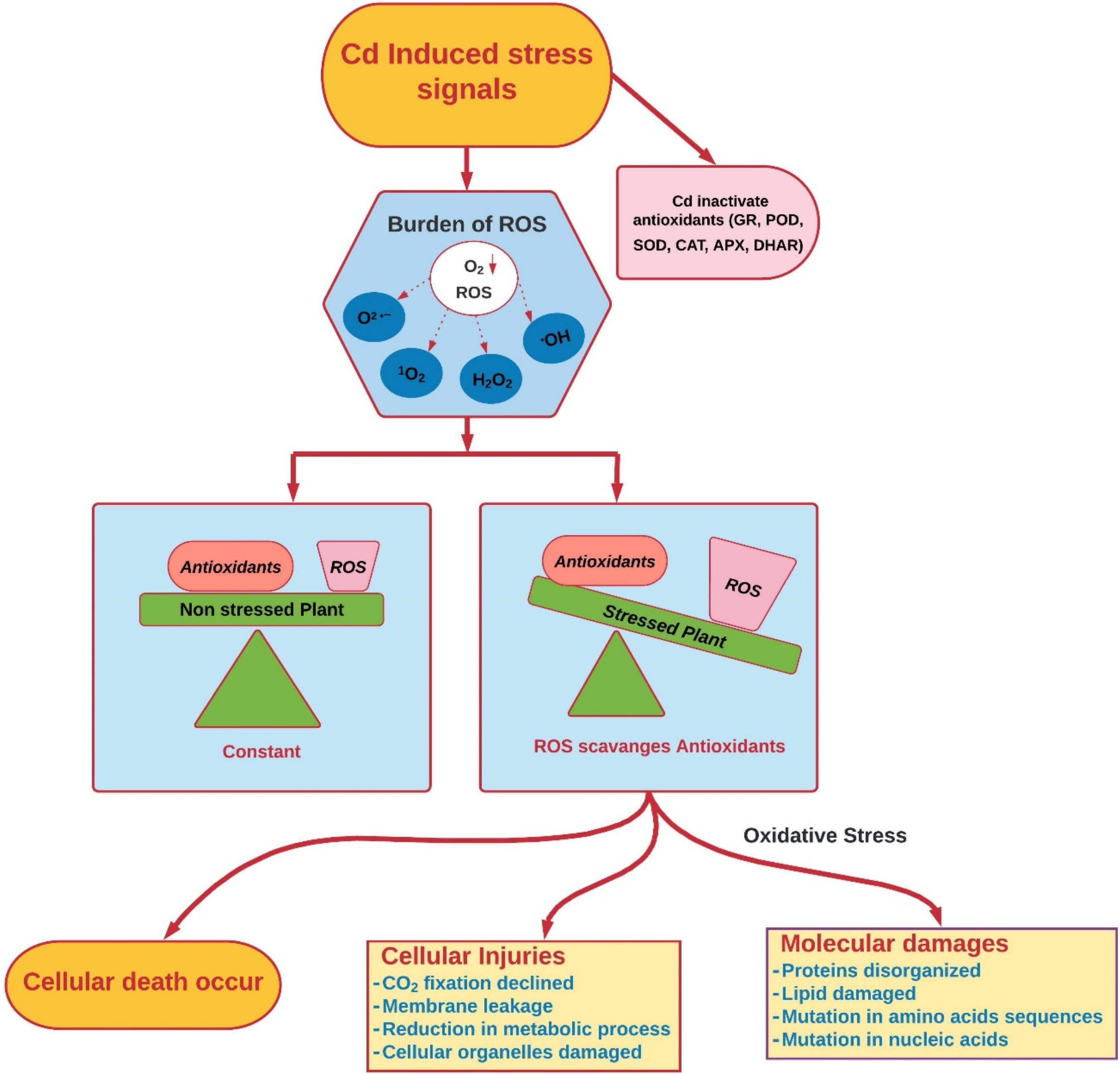
Figure 3. Cd-induced oxidative stress and damages in plants (conceived from Genchi et al., 2020; Shiyu et al., 2020). 1O2, singlet oxygen; ROS, reactive oxygen species; O2•–, superoxide anion; H2O2•, hydrogen peroxide; •OH, hydroxyl radical; APX, ascorbate peroxidase; GR, glutathione reductase; POD, peroxidase; CAT, catalase; SOD, superoxide dismutase; DHAR, dehydro-ascorbate reductase.
Accumulation of Cd in plants occurs because of some significant mechanisms like (i) structural similarity with nutrients taken by roots (phosphorous and zinc), (ii) direct Cd influence on the sulfhydryl (–SH) group, which impairs protein structure, (iii) dislocation of essential cations from binding sites, (iv) disturbance of normal level of ROS and antioxidants, which consequently damage nucleic acids, lipids, proteins, cellular pigments, and essential nutrients (Hossain et al., 2012; Choppala et al., 2014; Singh et al., 2016). Cd induces oxidative stress in several plant species, i.e., Phyllostachys pubescens (Li S. et al., 2016), Phoenix dactylifera L. (Zouari et al., 2016), Solanum lycopersicum (Medyńska-Juraszek et al., 2020), Triticum aestivum L. (Lin et al., 2007), Salvinia auriculata (Vestena et al., 2011), Spartina densiflora (Martínez Domínguez et al., 2010), and Phyllostachys pubescens (Li S. et al., 2016). Cd, in bivalent form, is unable to produce free radicals directly; however, after exposure to Cd, there is a significant increase in production of ROS (super oxide radicals, H2O2, and hydroxyl radicals). Cd induces oxidative stress by counteracting normal antioxidants, i.e., peroxidase (POD), dehydro-ascorbate reductase (DHAR), monodehydroascorbate reductase (MDHAR), ascorbate peroxidase (APX), catalase (CAT), and superoxide dismutase (SOD), and non-enzymatic antioxidants, i.e., vitamins C and E, tocopherols, carotenoids, ascorbic acid (ASA), and glutathione reductase (GR), which results in overproduction of ROS and causes damage to the biosynthetic machinery of cells. This xenobiotic-induced oxidative stress results in damage of biological membranes, macromolecules (proteins, lipids, and phospholipids), and biological membranes of cells. Cd also negatively influences the mitochondrial matrix by disrupting oxidative phosphorylation and ATP synthesis. Exposure to Cd also causes negative impacts on the cell repairing process of enzymatic proteins and damage to DNA and RNA, and reduces the proliferation and differentiation of cells (Akhter et al., 2021).
Carbon Metabolism and Yield Formation
Plants face toxic environmental constraints and mitigate these by dealing with carbon metabolism, constant supply of CO2, maintaining electron transport chain, and assimilation of CO2 at certain levels (Leegood, 1993). Disturbance in carbon metabolism occurs because of Cd toxicity and leads to decrease in photosynthetic efficiency (Gouia et al., 2003). Cd effectively causes alteration in photosynthesis by negative influence on its all aspects, including electron transport chain (ETC), photosystems (PSI and PSII), chl-proteins complexes, and CO2 reduction pathways in the stroma (Parmar et al., 2013). Cd-induced toxicity also convincingly results in alteration of chloroplast ultrastructure with inflated and disrupted thylakoids (Najeeb et al., 2011). Alteration in chloroplast ultrastructure was manifested by reduction in number of chloroplasts and size and number of grana, accumulation of plastoglobules, and excessive starch in leaves of Hordeum vulgare, Picris divarticata, and Brassica spp. (Ying et al., 2010; Wang et al., 2011; Elhiti et al., 2012). Moreover, the Willow plant showed aggregation of grana, disrupted thylakoids, and swallowed intrathylakoidal spaces owing to lipid peroxidation (LPX) (Hakmaoui et al., 2007). LPX is ensued by the activity of lipoxygenase (LOX) enzyme (Remans et al., 2010). This enzyme mediates the peroxidation of membrane fatty acids such as phosphatide glycerol (PG) and mono- and digalactosyldiacyl glycerol (MGDG, and DGDG. For instance, more accumulation of the activation of MGDG, DGDG, and PG results in the production of ROS and free radicals. Correlation of LOX with LPX reported in many plants like Lupine, Barely, Phaseolus, and Arabidopsis thaliana in response to Cd exposure (Maksymiec and Krupa, 2006; Tamás et al., 2009). In general, Cd causes a significant reduction in carbon metabolism, this reduction causes change in photosynthesis because of low supply of CO2, and low carbon levels counteract ETC and thylakoid membranes and photosynthetic enzymes. Ultrastructural changes in cell components like chloroplasts are also a major cause of reduction in photosynthesis efficiency of cells in leaves.
Disruption of photosynthetic pigments and biosynthetic inhibition in old and young leaves of plants have been reported as a primary cause of Cd toxicity (Xue et al., 2013; Anjum et al., 2016). Chlorophyll biosynthesis mainly depends on the aminolevulinate (ALA) compound, and Cd inhibits ALA at the site of glutamate availability and contradicts with the SH functional group of enzyme-like porphobilinogen deaminase and δ-aminolevulinic acid (Myśliwa-Kurdziel and Strzałka, 2002; Skrebsky et al., 2008). However, excessive concentration of ALA is responsible for the production of ROS, which can alter the redox potential of cells and alter cellular homeostatic functions, as reported in Cucumis sativus (Goncalves et al., 2009) and soybean (Noriega et al., 2007).
Cadmium toxicity alters the function of photosystems (PSI and PSII), interacts with PSII core complex and PSII supramolecular entities, and retards photoactivation (Quartacci et al., 2000; Sigfridsson et al., 2004). In contrast, PSI is considered more susceptible to Cd toxicity, and it might be because of Cd-induced Fe deficiency, which causes more damage to PSI (Timperio et al., 2007). Iron-deficit damage to PSI has been reported in Cucumis sativus L. under 10-μM Cd stress (Sárvári, 2008). In comparison to PSII, PSI is less explored in terms of Cd toxicity; however, photosynthetic yield was severely hindered because of Cd in Thlaspi caerulescens and Pisum sativum (Küpper et al., 2007; Wodala et al., 2012). Cd toxicity also hampers the Calvin cycle and shows inhibitory effects on various enzyme activities (Ying et al., 2010). Enzymes RUBP and PEP play a vital role during photosynthesis and are involved in CO2 fixation (Zhang et al., 2007). Excessive Cd ions decrease the activity of RUBP and PEP by causing alteration in their structures, and replace essential cofactors, such as Mg2+, which are involved in the process of carboxylation and shift to oxygenation reactions (Tran and Popova, 2013). Cd stress leads to disruption of photosynthetic traits, which results in damage of chloroplast components and hindrance in vital photosynthetic enzymes (Singh and Prasad, 2017).
Cadmium stress severely hinders plant growth, photosynthesis, and grain yield (Rizwan et al., 2016; Abbas et al., 2018). Several studies reported that Cd translocates to rice grains because of significant decline in grain yield and uptake of nutrients by roots (He et al., 2006; Liu et al., 2007; Rodda et al., 2011; Li and Zhou, 2012). However, in rice, the amplitude of yield reduction depends on genotype, concentration of Cd treatment, and duration of exposure (Song et al., 2017). Cadmium toxicity also severely reduces yield traits like number of spikelets per plant, grain number per ear, ear length, and weight in wheat crop (Khan et al., 2007). For instance, the threshold of Cd toxicity varies from genotype to genotype, exposure duration, and concentration of dose (Rizwan et al., 2016). Reduction in yield of crops has been reported previously on several plant species such as barley (Lentini et al., 2018), pea (Januškaitienė, 2010), tomato (Hayat et al., 2012), Phaseolus vulgaris (Rady, 2011), Zea mays L. (Anjum et al., 2015), and Cicer arietinum L. (Hayat et al., 2013; Liza et al., 2020). Cd toxicity severely reduces the growth and yield traits of plants; however, severity depends on plant species, Cd level, and exposure period. The impact of Cd on different plant crop yields is documented in Table 2.
Remediation of Cadmium-Polluted Soils
Physicochemical Remediation Methods
Remediation of Cd-contaminated soils could be achieved via organic chelate and surfactant application, and it is known as chelate-assisted phytoextraction or chelate-induced phytoextraction (Habiba et al., 2015; Sakouhi et al., 2016). Compared to traditional phytoremediation, chelate-induced phytoextraction is more beneficial, convenient, and environment frindly, because chelate-induced phytoextraction augments the extraction aptitude of hyperaccumulators (Clabeaux et al., 2011; Zhao et al., 2015; Wang et al., 2019). In chelate application strategy, different types of amino polycarboxylic acid chelates like S,S-ethylenediamine disuccinic acid (EDDS), iminodisuccinic acid (IDSA), [N, N]-bis glutamic acid (GLDA), ethylenediaminetetraacetate (EDTA), nitrilotriacetic acid (NTA), diethylenetriaminepentaacetic acid (DTPA), methylglycinediacetic acid (MGDA), ethylenebis (oxyethylenenitrilo) (EGTA) tetraacetic acid, and trans-1,2-diaminocyclohexane-N, N, N0, N0 -tetraacetic acid diethylenetriaminepentaacetic acid (CDTA) are applied in a Cd-contaminated growth medium to escalate Cd mobilization as well as phytoextraction capacity (Table 3; Zaheer et al., 2015; Hasan et al., 2019). Similarly, organic acids having low molecular weight, like oxalic acid (OA), citric acid CA), and tartaric acid (TA), have also been used as chelating agents. Having multi-ligand structures, these chelating agents form stable compounds with HMs and, resultantly, increase the mobility of soil HMs (Guo et al., 2019). According to Bian et al. (2016) and Moslehi et al. (2019), these chelating agents not only enhance the mobility of Cd in the soil solution but also change the form of Cd in soils, boost the availability of Cd for uptake and translocation, and, ultimately, upsurge Cd accumulation in aboveground biomass of plants grown in Cd-contaminated soils.
Among these chelating agents, EDTA has been most widely used because of its slower biodegradability and strong binding affinity toward Cd ions (Chen et al., 2004; Saifullah et al., 2009; Jiang et al., 2019). Structural characteristics of EDTA enable it to form a strong bond with Cd and increase Cd solubilization, translocation, and phytoextraction capacity of phytoremediation (Nowack, 2002; Hasan et al., 2019). However, remediation of Cd-polluted soils varies with different EDTA application rates, plant species, and soil types (Evangelou et al., 2007).
After EDTA, another widely used chelating agent for successful phytoextraction of Cd is EGTA (Pereira et al., 2010). Cheating agent EGTA enhances the uptake of Cd by plants efficiently (Hasan et al., 2019). Previous research studies have highlighted that application of EGTA increased the phytoextraction of Cd by 72% in Althaea rosea (Liu et al., 2008) by 43% in Mirabilis jalapa (Wang and Liu, 2014), and by 217% in Calendula officinalis (Jianv et al., 2010). Similarly, a surfactant named SDS is also being used to remediate organic and metal contaminations in soils (Pierattini et al., 2017). It was concluded that application of SDS not only increased the biomass but also increased Cd accumulation in roots and shoots of Althaea rosea (Liu et al., 2009, 2008) and Calendula officinalis (Jianv et al., 2010). GLDA is known as a green chelating agent, and it has better Cd extraction efficiency (Wu et al., 2015).
In terms of degradation, EDTA biodegrades slowly, while EDDS is a quick biodegradable chelating agent that can augment the mobility of HMs and their uptake, and translocation and accumulation of HMs in plant shoots in HM-contaminated soils (Li et al., 2013; Attinti et al., 2017; Zhu et al., 2017). However, EDDS phytoextraction efficacy depends on time of application, method of application, dose, and level of Cd contamination in soils.
Similar to EDDS and GLDA, NTA is also a quick biodegradable (approximately 7 days) and highly effective chelate for remediation of Cd-polluted soils (Hu X. et al., 2017). Similarly, application of some other chelating agents like DTPA and IDSA proved to be useful in Cd complexation, enhancing Cd solubility and its uptake by plants, e.g., hydroponically grown maize (Zhao et al., 2010). Saifullah et al. (2009) and Wang et al. (2019) highlighted that besides application of single chelating agents, when chelator complexes (a combination of two different chelates), e.g., GLDA + NTA and GLDA + CA were applied on Cd-contaminated soils, biomass production and Cd uptake by plants increased significantly.
Contrarily, where chemical amendments using chelating agents are conducted to phytoextract Cd from soil (Lambrechts et al., 2011), at the same time, these chemical amendments have a limitation of stunted plant growth, e.g., Pseudomonas brassicacearum (Krujatz, 2012), Lolium perenne, Brassica juncea, and Typha angustifolia (Muhammad et al., 2009; Goel and Gautam, 2010; Xu et al., 2010). Under such circumstances where the application of a single chelating agent resulted in stunted growth, application of a quick biodegradable chelate complex is a potential option for enhancing Cd phytoextraction (Wang et al., 2019). Similarly, combined application of chelating agents and plant growth regulators increased Cd uptake and biomass accumulation (Li Z. et al., 2018). Hence, in crux use of chelating agents is a viable option for remediation of Cd polluted soils. The application of chelating agents significantly enriched Cd uptake in plant biomass of many important plants (Table 3). Studies propose that application of chelates is a viable strategy for remediation of Cd-polluted soils. Soil contamination with heavy metals is a widespread environmental constraint. Therefore, it is very important to reduce the toxic impacts of HMs and their associated risks to plants and restoration of soils. The phytoremediation process includes phytostimulation, phytofiltration, phytotransformation, and phytoaccumulation, which extensively reduced the noxious effects of HMs in soils. The soil physicochemical remediation process includes soil washing, vitrification, solidification, stabilization, and use of metallophytes for phytoextraction. The process of phytoremediation of HM-contaminated soils is a reliable tool and necessary to make land resources accessible for crop production.
Plant Growth Regulators Assisted Remediation
Modulation of phytohormones or plant growth regulators (PGRs) not only mitigates the toxic effect of Cd stress on plants (Li Y. et al., 2018) but also enhances the tolerance of plants to Cd stress (Asgher et al., 2015) and efficacy of phytoextraction of Cd by plants in Cd-polluted soils (Sun et al., 2020). Research studies suggested the positive effect of PGRs on Cd translocation and accumulation, promotion of plant growth and nutritive value, and biomass accumulation under Cd stress (Asgher et al., 2015; Aderholt et al., 2017; Chen et al., 2019). Among key PGRs, cytokinins (CKs), ethylene, gibberellins (GAs), auxins, abscisic acid (ABA), polyamines (Pas), jasmonic acid (JA), brassinosteroids (BRs), and nitric oxide (NO) play substantial roles specifically in plant growth and developmental processes (Asgher et al., 2015). According to Piotrowska-Niczyporuk et al. (2012) and Sun et al. (2020), exogenous application of PGRs acts in diverse modes and enhances plant adaptability and tolerance to Cd stress in different ways. In addition, the role of CKs, indole-3-acetic acid (IAA), indole-3-butyric acid (IBA), and 1-naphthaleanecetic acid (NAA) in phytoextraction of Cd from Cd-polluted soils has been documented in many studies (Table 4; Bulak et al., 2014; Okem et al., 2015).
Gibberellins
Gibberellins (GA) can also protect plants from negative impacts of trace metals by reducing oxidative stress and increasing antioxidant mechanisms (Nguyen et al., 2020). GAs enhance sulfate assimilation, which promotes GSH/phytochelatin production, as S-containing metabolites are important for plant defense mechanisms. Application of GAs increased biomass accumulation, Cd uptake efficacy of Lolium perenne L. (He et al., 2014) and Helianthus annuus L. (Long et al., 2017), hampered MDA contents and oxidative stress of B. juncea under Cd toxicity (Meng et al., 2019), and in lupin plants and broad beans, it mitigated Cd toxicity by increasing soluble proteins under Cd stress (Sharaf et al., 2009). GAST1, a GA-stimulated transcript implicated in the control of ROS buildup, was also upregulated after an exogenous gibberellin was applied and subsequent transcriptomic techniques were employed (Sun et al., 2013). GA signaling boosted the expression of adenosine 50-phosphosulfate reductase, an enzyme crucial in sulfate assimilation, in A. thaliana under stress (Koprivova et al., 2008).
Abscisic Acid
Abscisic acid (ABA) is a plant hormone that regulates many aspects of plant development, growth, and stress responses (Nian et al., 2021). Reduced seed dormancy and wilty phenotypes are seen in ABA-deficient mutants from a variety of plant species, indicating that these important ABA activities are maintained across the plant kingdom (Haider et al., 2021b). In Bidens pilosa, the application of a stress hormone, e.g., ABA, enhanced plant tolerance to Cd stress and Cd extraction from Cd polluted-soil (Liu et al., 2017; Pompeu et al., 2017). Li et al. (2014) concluded that pretreatment of ABA decreased the activities of ascorbic acid, CAT, SOD, APX, POD, and GSH in roots of Vigna radiate L. under Cd stress. ABA, when used as a pretreatment before Cd treatment, did not result in increased contents of cysteine (CYS) and phytochelation (PC). This suggests the role of ABA in the regulation of PCS (Stroiński et al., 2013). The protective role of ABA against Cd stress has also been demonstrated by experiments comparing wild-type Arabidopsis plants and ABA-deficient plants, in which the mutants proved to be more sensitive to Cd metal stress (Sharma and Kumar, 2002). These findings strongly suggest that ABA may be involved in signal pathways during Cd stress.
Nitric Oxide
Nitric oxide is a free radical that reacts with oxygen molecules and controls the deposition of oxygen in plant tissues (Tran et al., 2011). NO is a signal molecule that activates cell defense mechanisms in response to a variety of stressors (Tran and Popova, 2013). Tran and Popova (2013) and Xu et al. (2015) documented that NO application diminished the structural modification of leaves, increased nutritional value, and improved antioxidant enzyme activities under Cd Stress. In other studies, application of NO on Cucumis sativus L. under Cd stress augmented chlorophyll contents and biomass accumulation, and decreased chlorosis symptoms and oxidative stress in plants (Yu et al., 2013).
Salicylic Acid
An endogenous phenolic PGR such as salicylic acid (SA) governs an imperative role in plant physiological processes (photosynthesis, growth, and development), specifically under abiotic stresses, including Cd toxicity (Tran and Popova, 2013; Gruznova et al., 2018). Ahmad et al. (2011) highlighted that SA in initial growth stages assisted plants in mitigating increased damage caused by Cd toxicity by expressing specific proteins and defense-related enzymes (Çanakci and Dursun, 2012; Roychoudhury et al., 2016). Pretreatment of SA abridged Cd accumulation, electrolyte leakage, and level of MDA in wheat shoots under Cd stress (Shakirova et al., 2016), enriched the level of lipids, upregulated the antioxidant system, and caused variations in fatty acid composition of vegetable seedlings (Tran and Popova, 2013; Semida et al., 2015; Rizwan et al., 2017). Increased levels of SA, in response to Cd stress, are reported in pea (Rodríguez-Serrano et al., 2006), maize (Krantev et al., 2008), Arabidopsis (Zawoznik et al., 2007), and halophyte Kosteletzkya virginica (Han et al., 2013). The role of SA in modulating the oxidative stress caused by Cd toxicity is evident by comparing the SA accumulating and deficient lines of Arabidopsis (Zawoznik et al., 2007; Tao et al., 2013). Mutants showed variable levels of H2O2 contents, lipid peroxidation, and antioxidant enzymes in comparison with wild plants. Increased levels of endogenous SA led to growth retardation while mutants having decreased endogenous SA showed least retardation in growth because of Cd stress. However, majority of studies disclose the protective role of SA in reduction of oxidative stress caused by Cd.
Jasmonic Acid
Jasmonates are oxylipins, which are oxygenated fatty acid derivatives (Ahmad et al., 2017). Methyl JA (MeJA) is a volatile molecule that may have a role in plant-to-plant communication. Exogenous application jasmonic acid (JA) resulted in reduced Cd, H2O2, and malondialdehyde accumulation in Vicia faba L. (Ahmad et al., 2017). Similarly, exogenous application of GB reduced the oxidative stress caused by Cd stress and increased the biomass of wheat (Rasheed et al., 2014). Application of ethylene on Arabidopsis thaliana under Cd stress increased root proliferation by modulating superoxide anion (Abozeid et al., 2017). At low levels (10−4 mol/L), it may incur a protective role in mitigation of Cd stress, but at high concentrations, JA might induce retardation in growth by degrading chlorophyll and photosynthetic enzymes (Maksymiec and Krupa, 2002).
Auxins
The auxin IAA is a well characterized hormone and is involved in growth regulation and physiological development of plant; however, it is still a less studied and less understood mystery in terms of response to Cd stress (Farooq et al., 2015; Teiri et al., 2018). Exogenous application of IAA on B. Juncea promoted uptake and accumulation of Cd that might be attributed to increased cell division, formation of vascular tissue and development of a broader root system that as a result, reduces the toxic effect of Cd (Teiri et al., 2018; Rostami and Azhdarpoor, 2019). Farooq et al. (2015) opined augmented growth and yield of rice when a precursor of an auxin was applied in Cd-contaminated soil. Elobeid et al. (2012), in their experiment, reported disturbed homeostasis of auxin in response to exogenously applied Cd. Yu et al. (2017) stated that an auxin transporter (OsAUX1) induced the extension of root hair and primary roots of rice under Cd stress. Ostrowski et al. (2016) reported that the application of an auxin conjugate (IAA-Asp) induced reduction of H2O2, and upregulated POD and CAT activity under Cd stress.
Cytokinins
Cytokinins (CKs) are a kind of plant hormone that promotes cytokinesis (cell division) in plant roots and shoots (Singh and Prasad, 2016). They have a role in cell proliferation and differentiation, as well as apical dominance, axillary bud development, and leaf senescence. Exogenous application of CKs inhibited Cd biosorption and augmented the activities of antioxidant enzymes in Chlorella vulgaris (Piotrowska-Niczyporuk et al., 2012) and tomato under Cd-contaminated soil conditions (Singh and Prasad, 2016).
Brassinosteroids
Brassinosteroids (BRs) are endogenous plant hormones that regulate a variety of physiological processes that are necessary for appropriate plant growth and development (Rehman et al., 2022a,b). Application of 28-homobrassinolide (homoBL) on the foliage of Brassica juncea improved Cd tolerance because of enhanced activity of antioxidant enzymes (CAT, POD, and SOD) (Hayat et al., 2007). The effect of 24-epibrassinolide (24-epiBL) was examined on Phaseolus vulgaris in response to Cd stress (Shahzad et al., 2018). 24-epiBL improved membrane stability index, proline content, and antioxidant system (Rady, 2011). Plants exposed to Cd stress have impaired electron transport (ETC) because of demolished photochemical reaction centers, whereas epi-brassinolide (EBL) reduced Cd toxicity and impaired the reaction centers of photosystems and ETC (Janeczko et al., 2005). Moreover, Cd stress helped older tissues more effectively and EBL improved the photosynthetic activity in radish leaves (Anuradha and Rao, 2009). Similarly, in tomatoes, BR application reduced the phytotoxic effects of Cd and improved fruit quality and yield (Hayat et al., 2012). Application of EBL in bean plants subjected to Cd stress increased the levels of antioxidant enzymes. Rise in an antioxidant system (superoxide dismutase, catalase, peroxidase and glutathione reductase, and proline) leads to increased tolerance, enhanced photosynthetic machinery, and growth. Similar kind of results were reported on other crops, i.e., mustard (Hayat et al., 2007) and chickpea (Rady, 2011), by exogenous application of EBL and HBL, respectively. The application of BRs as a shotgun approach (EBL and HBL) improved the chlorophyll content and photosynthesis efficiency of Cd- stressed tomato plants (Hayat et al., 2010). Besides this, BR treatment significantly increased the number of fruits, fruit yield, lycopene, and β-carotene contents in fruits of plants grown under Cd stress.
Polyamines
Polyamines are necessary for cell growth. Polyamine content is greater in quickly expanding tissues, and growth-promoting and regenerative hormone cues boost polyamine production and content (Hasanuzzaman et al., 2019). Rady and Hemida (2015) discussed that presoaking wheat seeds with polyamines, spermine or spermidine resulted in enhanced seedling growth, relative water contents, starch, ascorbic acid, membrane stability index, total glutathione, and concentration of protein, and that H2O2, total soluble sugars, concentration of proline, electrolyte leakage and MDA were decreased under Cd stress.
Some other compounds (paclobutrazol, daminozide, humic acid, and melatonin) are extensively used worldwide to decrease the devastating effects of Cd stress in cultivable plants (Khan et al., 2017; Hasan et al., 2019). In conclusion, the use of the above-mentioned PGRs might be an effective, eco-friendly strategy to enhance the growth and development of plants cultivated in a Cd-stressed environment.
Microbe-Assisted Remediation of Cadmium Stress
Soil microorganisms may not destroy or degrade HMs; however, they can affect physical and chemical characteristics that might help to migrate and transform them from highly toxic to less toxic forms and restrict their uptake by plants through a number of mechanisms including extracellular complexation, intracellular accumulation, and oxidation–reduction reaction. Furthermore, soil microorganisms could improve plant health by improving the uptake of nutrients that could upregulate plant growth and biomass production (Nejad et al., 2017; Rizwan et al., 2017). Thus, microbial symbioses have imperative ecological roles and can be used to increase the resilience and sustainability of ecosystems (French, 2017), especially in Cd-contaminated areas. Cd-induced polluted soil remediation can be mediated by two common types of mycorrhizae: (1) Ecto-mycorrhiza (ECM) and (2) Arbuscular mycorrhiza (AM).
In all ecosystems, AM and the other fungus, ECM, have made associations with almost all plant species (Lehmann et al., 2017). AM is a unique one, as it colonizes almost all types of plants to remediate HMs; however, unlike AM, ECM mostly colonizes woody plants. Phytoextraction of Cd mediated by Phragmites australis was observed under low Cd stress, and immobilization of Cd has in roots under high Cd stress has been reported (Huang et al., 2017). Sell et al. (2005) inoculated Populus canadensis and Salix viminalis with ECM strains including Hebeloma crustuliniforme, Pisolithus tinctorius, and Paxillus involutus, and observed an increase in Cd uptake and translocation from a sterilized Cd-contaminated soil. Hence, it can be stated that along with ECM inoculation, choice of the host plant is a determinant of better results. Arbuscular mycorrhizal fungi (AMFs) form a mycorrhizal symbiosis with almost 80% of higher plants and may increase the remediation of Cd-contaminated soils (Abdel-Latef et al., 2016; Table 5). The increasing attention on AMFs’ aptitude to retain HMs in the mycelium is due to a process known as “mycorrhizal-remediation,” which decreases the translocation of metals to other plant parts such as shoots and subsequently increases plant tolerance under such conditions (Moreira et al., 2015).
Inoculation of AMF improved the metal content in organic matter, electrical conductivity, soil pH, and the proportion of bioavailable Cd in a post-harvest soil (Wu et al., 2016). Nevertheless, Glomus versiforme significantly increased the translocation of Cd from roots to shoots relative to Fusarium caledonium. Furthermore, HMs cause oxidative stress in plants because of overproduction of ROS normally occurring during plant metabolism. There is always a balance between production and utilization of ROS in plant cells (Riaz et al., 2018; Yan et al., 2018; Kamran et al., 2019). AMF (Rhizoglomus intraradices, Glomus etunicatum, and Glomus versiforme) inoculation upregulated the activity of antioxidant enzymes in Cd-stressed plants that helped to increase plant growth and biomass (Tanwar et al., 2015; Sharma et al., 2017; Liu et al., 2018; Molina et al., 2020). Synthesis of sulfur-rich compounds such as phytochelatins and glutathione is important to mediate plant tolerance among numerous stress-induced detoxification pathways activated in plants (Mishra et al., 2009). AM’s role in regulation of thiol metabolism has recently been associated with Cd stress (Garg and Chandel, 2015). Thus, the defensive effect of AM is embodied in the mediation of the antioxidant enzyme system, alleviating the index of the lipid peroxidation process (Zhan et al., 2018; Janeeshma and Puthur, 2020; Luo et al., 2020), and AM inoculation improved phenol contents and proline and reduced H2O2 and lipid peroxidation (Hashem et al., 2016). Mycorrhizal plants show greater tolerance against metal stress through mechanisms such as chelation of metals in hyphae, immobilization, glomalin, root colonization, and compartmentalization in fungal cells (Yang et al., 2017). Inoculation of Anaerolineaceae in bioremediation of Cd stress is another possible method, because this regulates the shaping of microbial communities and mediates Cd solubility. AM (Rhodobacter sphaeroides) helped in P solubilization (Chen et al., 2019), Fe nutrition (Lehmann and Rillig, 2015), P and N uptake under different irrigation regimes (Liu et al., 2018), and enhanced the levels of K, P, and Ca in Euonymus japonica, and prominent levels of Zn, Mn, P, and K were sustained under stress (Bagheri et al., 2012). Chang et al. (2018) studied the putative role of Claroideoglomus etunicatum fungus that improved the uptake of N, P, and K by 20.1–76.8%, and by decreasing Cd uptake. AMF-induced glomalin accumulation acts as a defense system in plants against Cd-mediated oxidative stress in soils and plant tissues (Babadi et al., 2019). Bioaugmentation is a method that could be beneficial under low native AMF inoculum potential and involves the addition of microbial population for remediation of contaminated areas (Mongkhonsin et al., 2019). New stimulant formulations and techniques aimed at producing AMF inoculants might instigate the prevalent practice of AMF inoculation in the near future. Microbial flora such as fungi, algae, and photosynthetic flora effectively reduce HM contamination. Microbes showed various possible mechanisms to eliminate Cd toxicity including sequestering or accumulating metals in their cell walls and altering the composition of toxic compounds. Cadmium can be introduced to bacterial cells in the form of divalent cations by gene amplification, active efflux, and active influence on metallothionein genes. The major potential of remediation of metals by microbes is low operating costs, high capacity, metal recovery potential, and effective biosorbent regeneration.
Plant Growth-Promoting Rhizobacteria
Plant growth-promoting rhizobacteria (PGPRs) are rhizosphere inhabitants that enhance plant growth by improving plant nutrient availability, water relationship, and antioxidant activity to improve abiotic stress tolerance (Saeed et al., 2021). They are categorized based on (a) inherent characters (Kloepper, 1994): they (i) enhance root colonization, (ii) improve plant growth, (iii) acclimatize, survive, reproduce, and compete until expression of their potential in plant growth promotion/protection; (b) functional properties (Ahemad, 2014): (i) phytostimulators (phytohormones improve growth), (ii) biofertilizers (regulate nutrient uptake), (iii) rhizoremediators (solubilization of metals), and (iv) biopesticides (regulate plant diseases and pathogens by producing metabolic compounds and lytic enzymes). PGPRs help in phytoremediation; production of soluble minerals, siderophore, phytohormones, rhamnolipid, extracellular polymeric substances, osmo-protectants, 1-aminocyclopropane-1-carboxylate deaminase (ACCD), immobilization of metals (Mahajan and Kaushal, 2018), bioremediation; accumulation or transformation of contaminants, rhizoremediation; and remediation of contaminated soils by symbiotic relationship between plant roots and suitable microbial species. Inoculation of PGPRs has been reported to decrease Cd uptake and alleviate Cd-induced oxidative stress by producing phytohormones (Glick, 2014; Wang et al., 2015), ammonia, and siderophores that mediate nutrient availability, plant biomass accumulation, and plant water status (Ahmad et al., 2015; Hassan et al., 2015; Figure 4). However, Cd uptake may be improved by inoculation of Cd-tolerant bacteria in plants (Bojorquez et al., 2016; Sharma and Archana, 2016), which depicts the specificity of PGPRs and aim of experiment, i.e., phytostabilization vs. phytoextraction. Under heavy metal stress conditions, PGPR-induced IAA acts as a phytohormone to improve cell division and elongation to stimulate root growth, and enhances root nodulation, vascular bundle development, and plant growth (Goswami et al., 2016; Chen et al., 2017). Pseudomonas aeruginosa strains ZGKD5 and ZGKD2 augment to synthesize IAA in Solanum nigrum to improve tolerance against Cd stress (Shi et al., 2016). Huang et al. (2016) observed that Pse-w-MT induces Cd tolerance in Pisum sativum L. by improving the production of IAA. A Bacillus megaterium strain regulated cytokinin production by mediating the transcriptional level of the roots and shoots s receptor (AHK3/AHK4) that induced root morphogenesis in Arabidopsis thaliana (Jianfeng et al., 2017). Cd toxicity and uptake have been decreased by inoculation of Cd-resistant Micrococcus sp. TISTR2221 in maize (Suksabye et al., 2016) and Pseudomonas aeruginosa and Bacillus subtilis in rice (Sangthong et al., 2016).
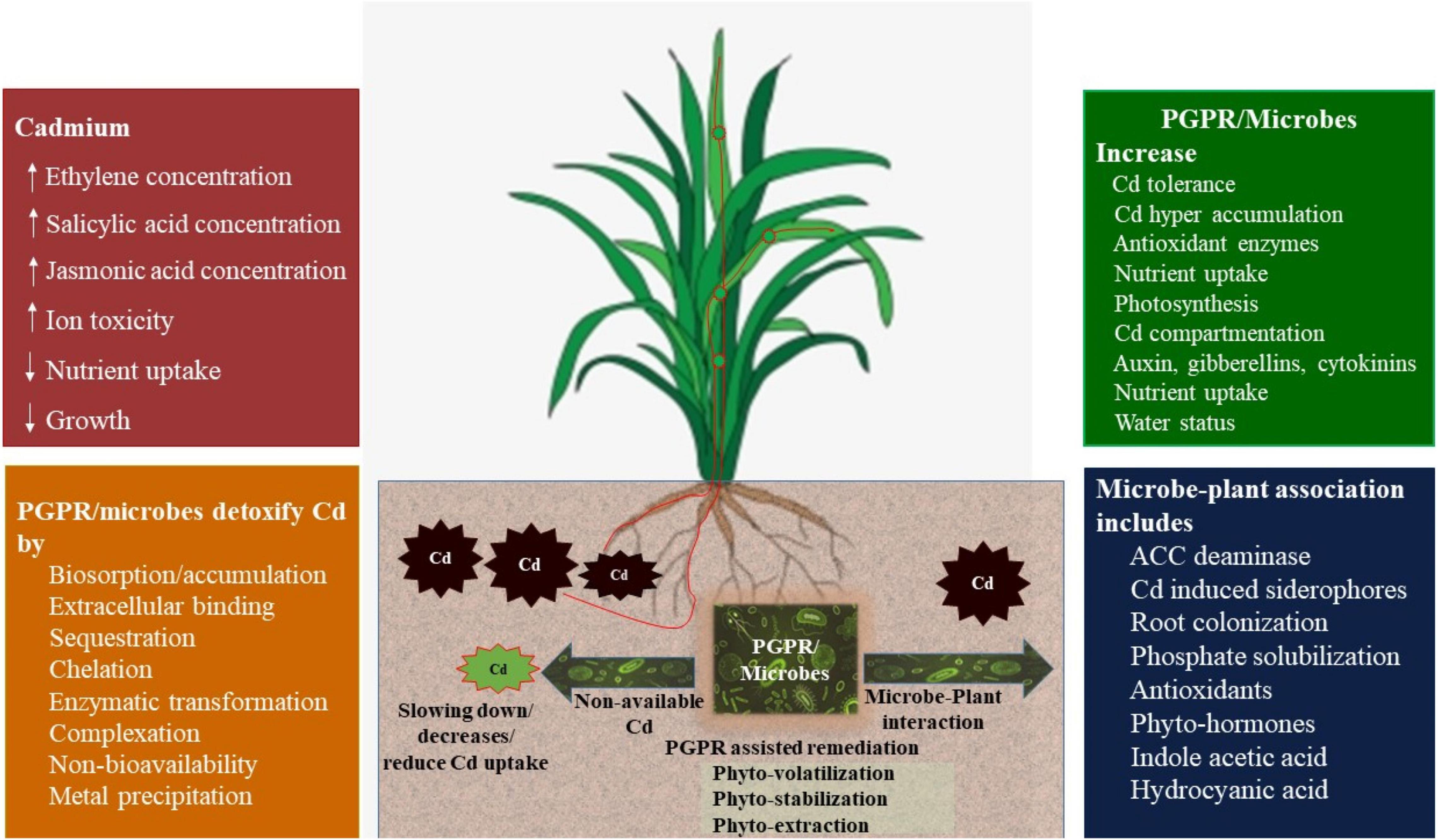
Figure 4. Impact of plant growth-promoting rhizobacteria (PGPRs) on remediation of Cd-contaminated soils (conceived from Ullah et al., 2015; Sharma and Archana, 2016).
Extracellular polymeric substances (EPSs), mucopolysaccharides, and proteins are produced by different PGPRs that help to bind toxic HMs for thriving plant growth (Upadhyay et al., 2011; Rajkumar et al., 2012). PGPR strains have been identified that produce EPS to counter the toxic effect of Cd contamination by decreasing the uptake of Cd in wheat (Joshi and Juwarkar, 2009). Pseudomonas aeruginosa inoculation with a PGPR strain helps to detoxify Cd stress based on extracellular biosorption, bioaccumulation, controlled siderophore production, formation of biofilm, enhanced respiration, and modified protein profile (Zivkovic et al., 2018). Recent studies have also shown the detoxification of Cd stress by P. aeruginosa (Zivkovic et al., 2018) and its adapted cells (Tang et al., 2018). Cd stress involved in the uptake of different nutrients influences metal solubility, and P solubilization, and improves nutrient mobility (chelation, precipitation, and immobilization) (Rafique et al., 2017; Saeid et al., 2018). PGPRs could be a promising option to enhance phyto-stabilization efficiency and bioleaching of Cu and Cd in heavily polluted soils owing to biosorption or bioaccumulation (Yang et al., 2018; Ke et al., 2021). Plants produce ROS-scavenging enzymes to detoxify ROS, which are produced under HM stress (Zainab et al., 2020). Exogenous applications of PGPR a strain, Streptomyces strain IT25, decreased catalase (CAT),and it is reported that PGPRs upregulated SOD (superoxide dismutase), POD (peroxidase), and PPO (plant polyphenol oxidases) genes to sequester Cd stress (Khanna et al., 2019; Abbasi et al., 2020) (Table 6). Azospirillum brasilense may ameliorate the negative effects of Cd stress by upregulation of lower Na/K ratio, TaSOS1 transcript level, proline, higher pigments, and antioxidant activities to improve dry weight (Ghassemi and Mostajeran, 2018). PGPR-mediated remediation of HMs and promotion of plant growth depend on PGPR strains coded by several genes and must be understood to build a multidimensional PGPR strain to perform multidimensional functions. Nevertheless, use of genetically engineered PGPR strains with hyper-accumulator plants to remove HMs is less common (Ullah et al., 2015). Several studies have also documented that genetic engineering mediated the improvement in remediation of HM stress in plants (Verma et al., 2017). Qiu et al. (2014) incorporated a bifunctional glutathione synthase gene (gcsgs) into Enterobacter sp. CBSB1 improved the efficiency of HM removal in B. juncea.
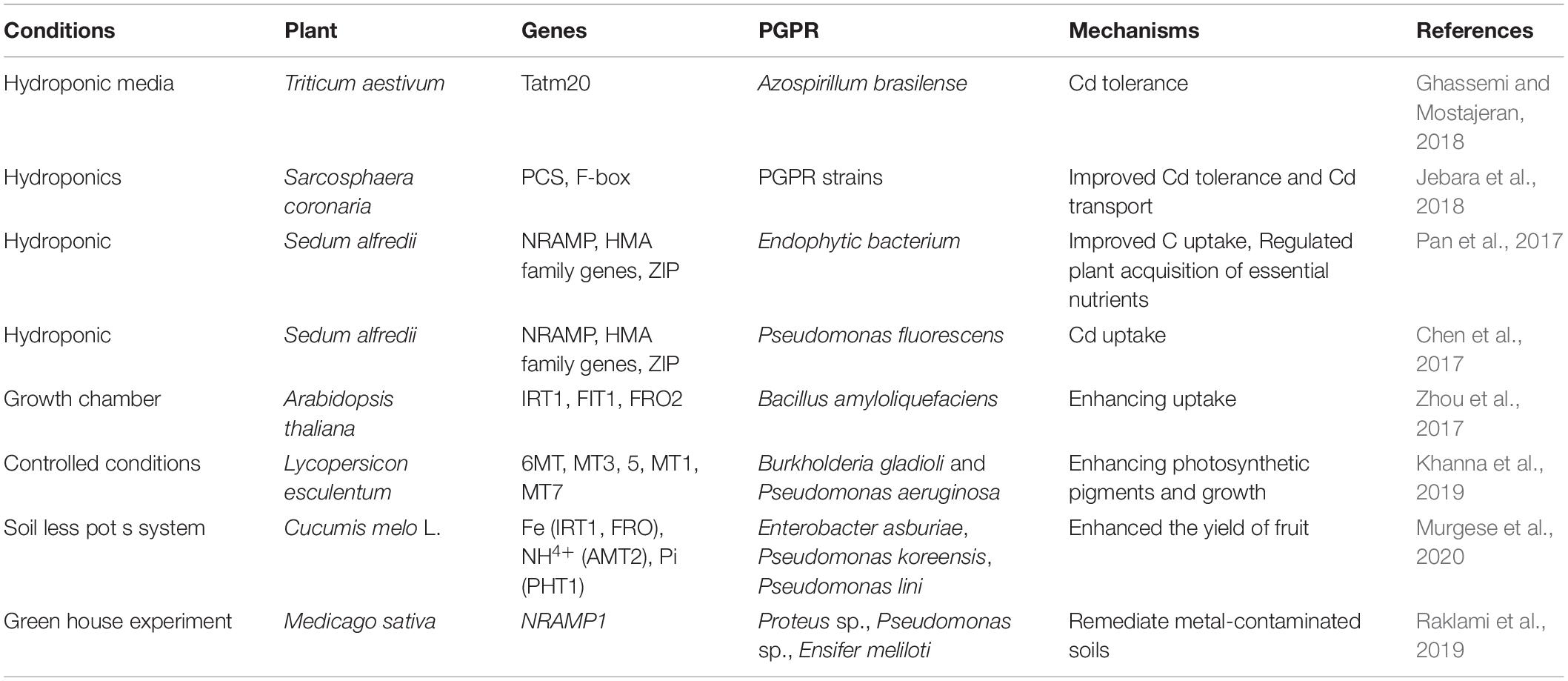
Table 6. Influence of inoculation with plant growth-promoting rhizobacteria (PGPRs) on Cd chelator and transporter gene expression (MT: metal transporter 1).
Cadmium Remediation Through Organic Amendments
To minimize the noxious effects of Cd in plants, use of soil additives is a promising method to fix Cd in soils because of its in situ assistance, low cost, and low energy needs (Yao et al., 2021). However, immobilization of Cd in soils has been performed using different inorganic and organic amendments. Various chemical and biological reactions are involved in Cd immobilization through these amendments (He et al., 2019). Two major sources of organic amendments are plants and animals that can increase soil fertility, as they are saturated with carbon, hydrogen, and oxygen (Hamid et al., 2020a). Use of organic amendments in agricultural soils have many benefits, but their most promising role is immobilization (Hu W. et al., 2017). Biochar, compost, and manure have been used as chief organic amendments for remediation of soil Cd (Hamid et al., 2019) through chelation, adsorption, and precipitation (Hamid et al., 2020a; Mondal et al., 2020).
Biochar
To overcome the deleterious effects of HMs, biochar addition to soil is a practical approach (Yuan et al., 2019). Soil productivity and growth of plants have been increased with biochar (Qayyum et al., 2017). In the past years, biochar (BC) has been used in HM-contaminated soils because of its higher adsorption capacity and ability to lower HM contents in soils (Boostani et al., 2019). Use of biochar is an efficient approach to minimize HMs in soils, but it is expensive compared to other amendments in terms of production (Sohail et al., 2020). Biochar, an organic soil additive, has been very helpful in immobilizing the in soils (Hamid et al., 2020b; Haider et al., 2022). Its role in Cd immobilization is auspicious because of its basic nature, porous texture, energetic functional groups, and higher CEC (Medynska-Juraszek and Cwielag-Piasecka, 2020). Biochar-amended soils showed lower Cd transport and accumulation (Abbas et al., 2018). Biochar can easily adsorb Cd, Pb, and Cu by forming complexes and cation exchange mechanisms in soils (Farooq et al., 2020). Breakdown of natural organic material under controlled temperature and limited or no oxygen resulted in biochar production (Hussain et al., 2021). It is well-reported in the published literature that the use of biochar in pots and field tests has significantly improved growth, biomass, and economic productivity in Cd-contaminated soils (Table 7).
Moreover, application of biochar enhances soil pH, water holding capacity, and porosity (Yuan et al., 2019). The role of biochar in growth enhancement is due to the presence of essential nutrients available for plants (Sohail et al., 2019). Rizwan et al. (2018) reported that application of biochar has a positive influence on plant growth. With application of biochar, the exchange portion of Cd in soils is reduced by up to 28% because of increase in soil pH (Bashir et al., 2018). Application of rice straw considerably reduced the concentration of extractable Cd and Cd in soils (Elyamine et al., 2019). Zia-ur-Rehman et al. (2020) reported that various types of biochar showed significant increase in wheat grain in addition to reduced Cd bioavailability. Different low-cost amendments enhanced the photosynthetic pigments and activity of enzymatic antioxidants (SOD, POD, CAT, and ASP) in maize (Shahkolaie et al., 2020).
Compost
In contrast to various fertilizers, utilization of compost is a valuable practice to increase soil fertility and crop yield (Abbas et al., 2020). Being a saturated organic carbon, compost enhances the HM-holding capacity of soils along with increase in soil absorption capacity (Medyńska-Juraszek et al., 2020). The bioavailability of HMs in soils has been decreased with the application of compost because of the mechanism of chelation, degradation of microbes, co-precipitation, and de-methylation (Hussain et al., 2021). Naturally, the process of composting helps to stabilize HMs and solid wastes from agricultural and municipal sources by degradation of various microbes (Awasthi et al., 2015). Addition of compost modifies soil aggregation, soil moisture content, and percentage of organic matter, and different nutrients resulted in higher crop growth and yield (Abd El-Mageed et al., 2018). This improved aggregation of soil resulted in altered soil physical and chemical properties, which helps to improve seed germination and roots of seedlings (Abd-El-Mageed et al., 2019). Addition of compost to soils showed 20, 19, and 10% decrease in shoot Cd, Cu, and Zn, respectively (Eissa, 2019). Application of vegetable waste compost reduced Cd concentration by up to 50 and 46% in maize shoots and roots, respectively, along with significant increase in plant growth and NPK contents (Bashir et al., 2021). An increase of 39–85 and 29–63% was observed in shoot and root fresh weight of pak choi cabbage, respectively, along with decrease in the concentration of Cd both in roots and shoots by up to 21–44 and 26–53%, respectively, when green waste compost was applied to HM-contaminated soils (Li et al., 2021).
Manure
Manure is used as a replacement for fertilizers, as it is a proper organic fertilizer for plant production (Zhen et al., 2020). Being an organic soil amendment, manure has been extensively used to mobilize HM-contaminated soils (Huang et al., 2018). Its application increased soil pH because of mineralization of carbon and excessive addition of basic cations (Hussain et al., 2021). Application of manure increased the organic matter in soils that resulted in reduced Cd mobilization and phytotoxicity (Liu et al., 2015). Higher accumulation of organic matter in soils stops Cd mobilization by forming organic compounds or, more importantly, adsorption (Halim et al., 2015). Long-term use of manure in uncontaminated soils introduced the availability of HMs as there is little or no information regarding the available or total HMs in the soil with the addition of manure in uncontaminated soils (Zhen et al., 2020). Li F. et al. (2019) stated that SOM had been increased in addition to reduced bioavailability of Cd with the addition of chicken manure in a Cd-contaminated paddy field. Farmyard manure application significantly decreased Cd bioavailability and increased wheat yield (Bashir et al., 2020). Treatment of contaminated soils with composted manure increased the growth of maize plants in addition to decreased HM uptake (Gul et al., 2016). Huang et al. (2020) observed a promising decrease in Cd and Zn bioavailability and enhanced biomass of B. juncea with the application of manure. Bashir et al. (2021) used animal manure to observe the growth of maize in polluted soil and found a prominent decrease of 58 and 52.4% in shoot and root Cd along with enhanced NPK contents.
Genetic Strategies for Cadmium Remediation
Genetic engineering has played a vital role in improving the phytoremediation abilities of plants in terms of removing or detoxifying hazardous heavy metals in the environment. Several molecular techniques are being widely used to reduce Cd accumulation. Molecular mechanisms underlying Cd interactions and remediation are shown and described in Figure 5.
Improvement in Cadmium Tolerance by Harnessing Genetic Variation
Screening of genotypes for low Cd accumulation is the primary step to develop Cd-tolerant genotypes. Utilization of these genotypes will help in the development of transgenic crops with enhanced tolerance. Rice has a main role in the supply of Cd to humans, which causes Cd toxicity in them. The risk of Cd toxicity in human beings can be reduced by identifying rice genotypes with low Cd-accumulating capacity. Considerable genetic variability has been found in polished rice and brown rice ranging from 0.14-1.43 and 0.06–0.99 mg kg–1, respectively (He et al., 2006; Liu et al., 2007). Indica rice displayed higher Cd accumulation in shoots and grains than japonica rice (He et al., 2006; Uraguchi et al., 2009).
In cereals, a substantial amount of genetic variability has been observed in grain legume crops, i.e., soybean (Arao et al., 2003; Sugiyama et al., 2011; Salazar et al., 2012; Vollmann et al., 2015). High or low Cd accumulating genotypes were identified in different crops by analyzing genetic variations present among them. In wheat, genotypes with low Cd accumulation capacity included durum lines 8982-TL-L, CDC-Verona, and Strongfield (Clarke et al., 2002; Pozniak et al., 2009). On the other hand, high Cd accumulating wheat genotypes were Joppa and Carpio (Elias et al., 2015; Elias and Manthey, 2016). Introgression of genes from one plant to another is also a promising genetic engineering technique, as many plants express genes that help in Cd tolerance (Lan et al., 2012; Yuan et al., 2012; Menguer et al., 2013). Aegilops tauschii accessions, i.e., AS623194, AS623402, and AS623402, were recommended to be rich sources of Cd-tolerant genes, as wild species have high genetic variability for Cd accumulation. Identification and inclusion of these genotypes in crop improvement programs will be helpful in developing new Cd-tolerant cultivars. Genes or QTLs controlling the accumulation of Cd can be identified using these lines in mapping populations (Qin et al., 2015).
Genetic Engineering Techniques for Cadmium Tolerance
Genetic engineering along with the development of transgenic plants may help in the cultivation of crops in soils contaminated with Cd. Various approaches in genetic engineering can help in the identification of desirable genes that can be used to develop plants with increased HM tolerance. These genes may assist in translocation and deposition of metals in tissues of transgenic plants along with other characteristics that help in tolerance of metals (Mosa et al., 2016; Sheoran et al., 2016). Recent research has displayed that several genes are involved in regulation of HM tolerance. This may be performed either by overexpression of one gene or by overexpression of multiple genes simultaneously (Bhargava et al., 2012). Therefore, genetic modifications involving transfer or regulation of genes should be studied thoroughly to develop crops tolerant to HM stress (Gerszberg and Hnatuszko-Konka, 2017). Metallothioneins and phytochelatins are key components that can be utilized to protect plants against toxic metals. Several studies have shown the importance of these components in phytoremediation, as HM tolerance was increased in transgenic plants developed using metallothionein genes and synthases (Sunitha et al., 2012).
Gene manipulation is one the most important strategies for producing Cd-tolerant plants. It involves complete understanding of molecular mechanisms involved in Cd stress and can be utilized to develop methods to reduce damages that are caused by toxic metals. This may also help reducing Cd concentration in edible plant parts by breeding crop varieties with low Cd accumulation and more agronomical importance. Most effective molecular techniques used worldwide for Cd tolerance in crop plants have been discussed.
Quantitative Trait Loci Related to Cadmium Uptake and Accumulation
The identification of chromosomal regions governing genes that control Cd tolerance has become an important genetic tool with the discovery of quantitative trait loci (QTLs) using family based method. This method has helped in the illustration of underlying genetic basis of Cd accumulation in various crops (Ishikawa et al., 2005, 2010; Knox et al., 2009; Ueno et al., 2009a,b; Jegadeesan et al., 2010; Jha and Bohra, 2016). In rice, QTLs are supposed to be located on LGs 3, 6, and 8 in chromosome segment substitution lines (CSSLs) developed from the cross between Kasalath (indica) and Koshihikari (japonica) (Ishikawa et al., 2005).
A QTL responsible for Cd accumulation in roots and shoots was found on LGs 6 and 7, while LG 3 consisted of a QTL for root or shoot Cd content. LGs 1, 3, 5, and 8 governed a total of six QTLs responsible for Cd tolerance (Xue et al., 2009). In F2 resulting from a cross between Badari Dhan and Shwe War, it was demonstrated that QTLs controlling 16.1% of total phenotypic variation (PV) related to Cd tolerance was mapped on LG 11 (Ueno et al., 2009a). QTL qLCdG11 for Cd tolerance was also mapped on LG 11 in an RIL population developed from a cross between Fukuhibiki 9 and LAC23. Major QTLs elucidating 85% of PV related to Cd accumulation were reported on LG 7 in rice (Ueno et al., 2009b). Afterward, QTL qGCd7 found on LG 7 explained 35.5% of phenotypic variation by analyzing backcross inbred lines developed by crossing Sasanishiki (japonica) with Habataki (indica). Major QTL qlGCd3 controlling Cd levels in rice grains was discovered by mapping CSSLs developed from a cross between LAC23 and Koshihikari (Abe et al., 2013). A total of 18 Cd-accumulating QTLs in milled rice and 14 in brown rice were reported to be located on LGs 2, 3, 4, 5, and 7 (Da-wei et al., 2018).
Luo et al. (2018) demonstrated CAL1 as a QTL in rice causing 13% change in Cd accumulation in leaves of a double haploid population. CAL1 is responsible for Cd translocation from root to shoot, and rice mutants with CAL1 knockout displayed decreased Cd concentration in leaves (Luo et al., 2018). Cd-accumulating QTLs were mapped in rice by growing an inbred population (743/Katy) derived from Xiang in soil contaminated with Cd revealing two QTLs, i.e., qCd-7 and qCd-2 involved in the process of Cd uptake and accumulation in rice (Li W. et al., 2016; McCouch et al., 2016). Sato et al. (2011) discovered two QTLs in brown rice affecting Cd accumulation, i.e., qLCdG11 and qLCdG3, explaining 9.4–12.9 and 8.3–13.9% of phenotypic variation, respectively. Five most important QTLs were discovered; three of them (gcc3, gcc9, and gcc1) were responsible for grain Cd concentration; scc10 was linked with Cd accumulation in shoots, and the QTL sgr5 was involved in supply of Cd to roots and shoots. Grain Cd accumulation was highly influenced by Srg5 in rice (Abe et al., 2013).
In durum wheat, the gene Cdu1 plays a role in Cd uptake, as reported by Knox et al. (2009), whereas Wiebe et al. (2010) illustrated the role of Cdu1 in accumulation of Cd in wheat grains. Recently, a major QTL on 5BL has been located in durum wheat controlling 54.3% PV for Cd uptake with the help of high-density genotyping (AbuHammad et al., 2016). In an RIL population developed by crossing Divide with D041735, another QTL, “QCdu. ndsu-5B,” has been discovered on 5B for Cd intake and tolerance. In soybean, a QTL for lower accumulation of Cd has been mapped on LGK controlling up to 57.3% of phenotypic variation (Jegadeesan et al., 2010).
Association Studies to Check Plant Response to Cadmium Stress
Advancement in methods used for genome mapping has led to revelation of various significant market trait associations (SMTAs) present in genomes for several traits including Cd intoxication (Huang and Han, 2014). Additionally, elucidation of crop genomes and other techniques, such as genome resequencing and genotyping by sequencing (GNB) to identify SNPs has helped in performing GWAS to study complex plant traits, i.e., HM toxicity and tolerance. Six SMTAs (such as pdil5-1, TaAP2-B, TaAP2-D, DME-5A, and Acc-1) related to genes controlling Cd tolerance were revealed in 235 accessions of Aegilops tauschii by GWAS using 7,185 SNPs.
A total of 17 QTLs for grain Cd accumulation were discovered in rice by GWAS conducted on 276 rice accessions using 416 K SNPs (Liu et al., 2019). A total of 312 out of 1,568 accessions of rice displaying more diversity were selected and evaluated to develop low Cd accumulating germplasm (Li W. et al., 2016; McCouch et al., 2016). Twenty-four rice accessions including 3 Indica accessions linked with reduced accumulation of Cd by up to 0.2 mg kg–1 were selected based on GWAS. A total of 312 accessions including subpopulations of Indica and Japonica rice were analyzed, leading to discovery of 28 QTLs related to Cd uptake and accumulation in plant tissues. Genes, i.e., OsNRAMP5, OsNRAMP1, OsLCD, and OsHMA3, that have been already discovered were also reported in recent GWASs (Li W. et al., 2016; McCouch et al., 2016).
A GWAS was carried out on 100 barley accessions and resulted in identification of several QTLs, i.e., 9 for root Cd accumulation, 21 for shoot Cd, 14 for transport of Cd from roots to shoots, and 15 for grain Cd amassment (Wu et al., 2015). Recently, 63 SMTAs on 5 LGs for leaf Cd were discovered in maize by GWAS using 43,737 SNPs (Zhao et al., 2018). This study also revealed several genes such as GRMZM2G45549 and GRMZM2G124103 coding for vacuolar ATPase, and GRMZM2G175576 for ATPase controlling Zn/Cd transport. Identification of specific DNA markers and accurate phenotyping techniques for study of Cd stress in crop plants will help in better understanding of Cd accumulation mechanism and improvement of genotypes for Cd tolerance.
Cadmium Phytoremediation by Overexpression of Genes
Introduction and overexpression of genes associated with metal uptake and translocation are the most effective and commonly used methods for phytoremediation using transgenics (Shukla et al., 2013; Mani and Kumar, 2014; Das et al., 2016). Various pathways including metal intake by roots, formation of metal-ligands and metal-chelator complexes, deposition of metals in vacuole, and long-distance translocation to shoots through symplast and apoplast can be exploited to enhance metal accumulation in plant tissues, as metal intake is a complex process (Nakamura et al., 2014; Das and Jayalekshmy, 2015). Genes coding for metal ion transporters and chelators are being used to manipulate plant metal uptake and translocation.
Metal transporters are also linked to gene families coding for ZIP proteins that are involved in Fe and Zn transport in cytoplasm. Conolly et al. (2002) reported 150% enhanced accumulation of Cd and Zn when gene coding for AtIRT1 was overexpressed in A. thaliana. However, some ZIP genes are highly specific, and their overexpression does not lead to improved accumulation of HMs like Cd (Tiong et al., 2014). Therefore, another strategy has been exploited in which genes coding for metal transporters and involved in microelements uptake and transport are transformed into plants. When absorption microelements are increased, HM uptake is reduced. These genes manipulate plant’s ability to collect HMs by enhancing or decreasing the effect of ZIP genes. This method may be utilized for phytoremediation of soil contaminated with HMs and for biofortification of crops (Siemianowski et al., 2014; Gong et al., 2015).
Nakamura et al. (2014) developed transgenic plants of Nicotine tabacum by overexpression of cysteine synthase (CS) and serine acetyltransferase (SAT) involved in cysteine biosynthesis. The plants produced by overexpression of these genes displayed enhanced Cd tolerance. He et al. (2016) inserted a homologous corn gene in Arabidopsis by activating methyltransferase gene (CIMT1 protein). Results displayed enhanced Cd tolerance in Arabidopsis by the overexpression of this gene. This gene could be utilized to enhance Cd tolerance in agricultural crops. Lee and Back (2017) overexpressed melatonin-related genes using transgenic OsSNAT1 in rice. The study revealed that transgenic OsSNAT1 provides tolerance to Cd. An expansin gene, TaEXPA2, was isolated from wheat and overexpressed in tobacco plants. This enhanced tolerance to Cd toxicity in tobacco plants and improved seed germination and growth of seedling and roots (Ren et al., 2018).
Expression of OXS3 (OXIDATIVE STRESS 3)-like gene fragments led to reduced accumulation of Cd in rice grains and other plant tissues without affecting yield and amount of minerals, i.e., Cu, Mn, Zn, and Fe (Blanvillain et al., 2009). Hence, this approach seems to be promising for production of Cd-tolerant genotypes without any loss in yield or minerals. OsHMA3 was involved in the transport of Zn to the vacuoles in root cells (Sasaki et al., 2012). The expression of OsHMA3 along with the OsHMA2 promoter had no effect on the growth of plants in vegetative stage. Moreover, when OsHMA3 was expressed under OsHMA2 control, the expression and localization of OsHMA3 in tissues were increased, and significant reduction in Cd concentration was observed because of increased Cd sequestration in roots, nodes, and vacuoles (Shao et al., 2018).
The functional gene OsHMA3 from rice was overexpressed in wheat for Cd sequestration in roots. Cd accumulation in roots was enhanced by up to 110–125%. Reduction in Cd accumulation in wheat grains was observed to be up to 40-folds, while translocation of Cd from roots to shoots was decreased by approximately 10-folds (Zhang L. et al., 2020). This technique offers an effective solution to reduce Cd accumulation in plants, leading to reduced health risks.
Clustered Regularly Interspaced Short Palindromic Repeats/Cas9-Based Genome Editing to Reduce Cadmium Accumulation
Genome editing tools like clustered regularly interspaced short palindromic repeats (CRISPR) and CRISPR-associated protein 9 (Cas9) systems (CRISPR/Cas9) can be used for Cd tolerance in plants, i.e., rice (Ishizaki, 2016; Jung et al., 2018). It is possible to develop genotypes with low Cd accumulation without affecting plant growth and yield by developing mutants with transporters having low Cd affinity, such as OsNramp5 and OsLCT1, using the CRISPR/Cas9 technology. Recently, another approach has been discovered that may be helpful in getting desired results, and it involve deletion of large DNA fragments using CRISPR/Cas9.
Conclusion
Higher uptake of Cd leads to toxicity in plants, and reduction of Cd uptake by plants is also imperative for ensuring food safety. As a result, plants that are confined in their habitat are more likely to be exposed to Cd toxicity, which negatively impacts all growth- and yield-related characteristics, resulting in substantial economic losses. In this review, we discussed that plants have an efficient biochemical defense mechanism and utilize numerous physiological and molecular processes to deal with increased Cd exposure to offset its inhibitory effects. Exclusion of excess Cd at the root level, vacuolar sequestration, enzymatic detoxification, and preservation of essential cations are some of the techniques utilized to reduce Cd2+, which are significant disadvantages of Cd toxicity. Cd toxicity can also be reduced using various remediation techniques such as phytoremediation, PGPR inoculation, microbial-assisted remediation, chemical remediation, nutrients utilization, and organic amendments. Moreover, through genetic engineering and molecular breeding, capacity for remediation and tolerance to Cd toxicity can be improved. This review covers all probable processes of Cd toxicity remediation approaches; however there are still some information gaps. As a result, further research is needed to investigate Cd toxicity at the grain level, as well as its impacts on other living biota and the mechanisms of its tolerance at the cell and organelle levels. Significant and consistent QTLs for Cd tolerance were discovered in mapping populations with various genetic origins, as well as chosen donors, which indicated a significant promise for application in improved breeding methods. Although many efforts have been made to reduce Cd toxicity in plants, further research should be carried out by keeping these points in focus. There is a need to identify Cd toxicity pathways at molecular levels for better understanding of Cd toxicity. More detailed studies are required to understand the mechanism of different amendments in reducing Cd toxicity in plants. There is a need for a multidisciplinary approach to identify key target tolerance traits with improved high-throughput screening techniques that can provide valuable insights into the underlying mechanisms of Cd tolerance and homeostasis, especially in agronomic crops, for a better understanding of the genetics of Cd tolerance. To draw a sound conclusion, long-term field trials are required for estimation of a risk and benefit analysis for various management strategies.
Author Contributions
UZ, WJ, and AM: conceptualization. WX and MN: methodology. JK and SH: software. MA: resources. MM and MK: data curation. UZ and FH: writing (original draft preparation). AM, JK, MB, MN, WX, and MI: writing (review and editing). NA and NF: visualization. MI and SH: supervision. MB and MK: project administration. All authors read and agreed to the published version of the manuscript.
Funding
This study was supported by a project of Ministry of Education, Youth and Sports of the Czech Republic (Grant No: FCH-S-21-7398).
Conflict of Interest
The authors declare that the research was conducted in the absence of any commercial or financial relationships that could be construed as a potential conflict of interest.
Publisher’s Note
All claims expressed in this article are solely those of the authors and do not necessarily represent those of their affiliated organizations, or those of the publisher, the editors and the reviewers. Any product that may be evaluated in this article, or claim that may be made by its manufacturer, is not guaranteed or endorsed by the publisher.
Acknowledgments
The authors highly acknowledge the Institute of Soil and Environmental Sciences, and the Department of Agronomy, University of Agriculture Faisalabad, Pakistan.
References
Abbas, A., Azeem, M., Naveed, M., Latif, A., Bashir, S., Ali, A., et al. (2020). Synergistic use of biochar and acidified manure for improving growth of maize in chromium contaminated soil. Int. J. Phytoremed. 22, 52–61. doi: 10.1080/15226514.2019.1644286
Abbas, T., Rizwan, M., Ali, S., Adrees, M., Zia-ur-Rehman, M., Qayyum, M. F., et al. (2017). Effect of biochar on alleviation of cadmium toxicity in wheat (Triticum aestivum L.) grown on Cd-contaminated saline soil. Environ. Sci. Pollut. Res. 25, 25668–25680. doi: 10.1007/s11356-017-8987-4
Abbas, T., Rizwan, M., Ali, S., Adrees, M., Mahmood, A., Zia-ur-Rehman, M., et al. (2018). Biochar application increased the growth and yield and reduced cadmium in drought stressed wheat grown in an aged contaminated soil. Ecotoxicol. Environ. Saf. 148, 825–833. doi: 10.1016/j.ecoenv.2017.11.063
Abbasi, S., Sadeghi, A., and Safaie, N. (2020). Streptomyces alleviate drought stress in tomato plants and modulate the expression of transcription factors ERF1 and WRKY70 genes. Sci. Hortic. 265:109206.
Abd El-Mageed, T. A., El- Samnoudi, I. M., Ibrahim, A. M., and Abd El Tawwab, A. R. (2018). Compost and mulching modulates morphological, physiological responses and water use efficiency in sorghum (bicolor L. Moench) under low moisture regime. Agric. Water Manage. 208, 431–439.
Abdel-Latef, A. A. H., Hashem, A., Rasool, S., Abdallah, E. F., Alqarawi, A., Egamberdieva, D., et al. (2016). Arbuscular mycorrhizal symbiosis and abiotic stress in plants: a review. J. Plant Biol. 59, 407–426.
Abd-El-Mageed, T. A., El-Sherif, A. M., Abd El-Mageed, S. A., and Abdou, N. M. (2019). A novel compost alleviate drought stress for sugar beet production grown in Cd-contaminated saline soil. Agric. Water Manag. 226:105831.
Abe, T., Nonoue, Y., Ono, N., Omoteno, M., Kuramata, M., Fukuoka, S., et al. (2013). Detection of QTLs to reduce cadmium content in rice grains using LAC23/Koshihikari chromosome segment substitution lines. Breed. Sci. 63, 284–291. doi: 10.1270/jsbbs.63.284
Abozeid, A., Ying, Z., Lin, Y., Liu, J., Zhang, Z., and Tang, Z. (2017). Ethylene improves root system development under cadmium stress by modulating superoxide anion concentration in Arabidopsis thaliana. Front. Plant Sci. 8:253.
AbuHammad, W. A., Mamidi, S., Kumar, A., Pirseyedi, S., Manthey, F. A., Kianian, S. F., et al. (2016). Identification and validation of a major cadmium accumulation locus and closely associated SNP markers in North Dakota durum wheat cultivars. Mol. Breed. 36:112.
Aderholt, M., Vogelien, D. L., Koether, M., and Greipsson, S. (2017). Phyto- extraction of contaminated urban soils by Panicum virgatum L. enhanced with application of a plant growth regulator (BAP) and citric acid. Chemosphere 175, 85–96. doi: 10.1016/j.chemosphere.2017.02.022
Adil, M. F., Sehar, S., Chen, G., Chen, Z. H., Jilani, G., Chaudhry, A. N., et al. (2020). Cadmium-zinc cross-talk delineates toxicity tolerance in rice via differential genes expression and physiological/ultrastructural adjustments. Ecotoxicol. Environ. Saf. 190:110076. doi: 10.1016/j.ecoenv.2019.110076
Afzal, M., Yu, M., Tang, C., Zhang, L., Muhammad, N., Zhao, H., et al. (2019). The negative impact of cadmium on nitrogen transformation processes in a paddy soil is greater under non-flooding than flooding conditions. Environ. Int. 129, 451–460. doi: 10.1016/j.envint.2019.05.058
Ahemad, M. (2014). Remediation of metalliferous soils through the heavy metal resistant plant growth promoting bacteria: paradigms and prospects. Arab. J. Chem. 12, 1365–1377. doi: 10.1016/j.arabjc.2014.11.020
Ahmad, J., Ali, A. A., Baig, M. A., Iqbal, M., Haq, I., and Qureshi, M. I. (2018). “Role of phytochelatins in cadmium stress tolerance in plants,” in Cadmium Toxicity and Tolerance in Plants, eds M. Hasanuzzaman, M. N. V. Prasad, and M. Fujita (Cambridge, MA: Academic Press).
Ahmad, M. T., Asghar, H. N., Saleem, M., Khan, M. Y., and Zahir, Z. A. (2015). Synergistic effect of rhizobia and biochar on growth and physiology of maize. Agron. J. 107, 1–8.
Ahmad, P., Alyemeni, M. N., Vijaya, L., Alam, P., Ahanger, M. A., and Alamri, S. A. (2017). Jasmonic acid alleviates negative impacts of cadmium stress by modifying osmolytes and antioxidants in faba bean (Vicia faba L.). Arch. Agron. Soil Sci. 63, 1889–1899. doi: 10.1080/03650340.2017.1313406
Ahmad, P., Nabi, G., and Ashraf, M. (2011). Cadmium-induced oxidative damage in mustard [Brassica juncea (L.) czern. &coss.] plants can be alleviated by salicylic acid. S. Afr. J. Bot. 77, 36–44. doi: 10.1016/j.sajb.2010.05.003
Ahmad, S. H., Reshi, Z., Ahmad, J., and Iqbal, M. (2005). Morpho-anatomical responses of Trigonellafoenum graecum Linn. to induced cadmium and lead stress. J. Plant Biol. 48, 64–84. doi: 10.1007/bf03030566
Akhter, Z., Bi, Z., Ali, K., Sun, C., Fiaz, S., Haider, F. U., et al. (2021). In response to abiotic stress, DNA methylation confers epi-genetic changes in plants. Plants 10:1096. doi: 10.3390/plants10061096
Ali, B., Gill, R. A., Yang, S., Gill, M. B., Farooq, M. A., Liu, D., et al. (2015). Regulation of cadmium-induced proteomic and metabolic changes by 5- aminolevulinic acid in leaves of Brassica napus L. PLoS One 10:e0123328. doi: 10.1371/journal.pone.0123328
Alle, V., Kondratovics, U., Osvalde, A., and Vikmane, M. (2016). Differences in cadmium accumulation and induced changes in root anatomical structures in plants used for food. Agron Res. 14, 1249–1260.
Alyemeni, M. N., Ahanger, M. A., Wijaya, L., Alam, P., Bhardwaj, R., and Ahmad, P. (2018). Selenium mitigates cadmium-induced oxidative stress in tomato (Solanum lycopersicum L.) plants by modulating chlorophyll fluorescence, osmolyte accumulation, and antioxidant system. Protoplasma 255, 459–469. doi: 10.1007/s00709-017-1162-4
Amirabad, S. A., Behtash, F., and Vafaee, Y. (2020). Selenium mitigates cadmium toxicity by preventing oxidative stress and enhancing photosynthesis and micronutrient availability on radish (Raphanus sativus L.) cv. Cherry Belle. Environ. Sci. Pollut. Res. 27, 12476–12490. doi: 10.1007/s11356-020-07751-2
Amirahmadi, E., Hojjati, S. M., Kammann, C., Ghorbani, M., and Biparva, P. (2020). The potential effectiveness of biochar application to reduce soil Cd bioavailability and encourage oak seedling growth. Appl. Sci. 10:3410. doi: 10.3390/app10103410
Anjum, S. A., Tanveer, M., Hussain, S., Bao, M., Wang, L., Khan, I., et al. (2015). Cadmium toxicity in Maize (Zea mays L.): consequences on antioxidative systems, reactive oxygen species and cadmium accumulation. Environ. Sci. Pollut. Res. 22, 17022–17030. doi: 10.1007/s11356-015-4882-z
Anjum, S. A., Tanveer, M., Hussain, S., Ullah, E., Wang, L., Khan, I., et al. (2016). Morpho-physiological growth and yield responses of two contrasting maize cultivars to cadmium exposure. CLEAN Soil Air Water 44, 29–36. doi: 10.1002/clen.201400905
Anuradha, S., and Rao, S. S. R. (2009). Effect of 24-epibrassinolide on the photosynthetic activity of radish plants under cadmium stress. Photosynthetica 47, 317–320. doi: 10.1007/s11099-009-0050-3
Arao, T., Ae, N., Sugiyama, M., and Takahashi, M. (2003). Genotypic differences in cadmium uptake and distribution in soybeans. Plant Soil 251, 247–253. doi: 10.1007/s00726-010-0809-7
Asgher, M., Khan, M. I. R., Anjum, N. A., and Khan, N. A. (2015). Minimising toxicity of cadmium in plants—role of plant growth regulators. Protoplasma 252, 399–413. doi: 10.1007/s00709-014-0710-4
Attinti, R., Barrett, K. R., Datta, R., and Sarkar, D. (2017). Ethylenediaminedisuccinic acid (EDDS) enhances phytoextraction of lead by vetiver grass from contaminated residential soils in a panel study in the field. Environ. Pollut. 225, 524–533. doi: 10.1016/j.envpol.2017.01.088
Awasthi, M. K., Pandey, A. K., Bundela, P. S., and Khan, J. (2015). Co-composting of organic fraction of municipal solid waste mixed with different bulking waste: characterization of physicochemical parameters and microbial enzymatic dynamic. Bioresour. Technol. 182, 200–207. doi: 10.1016/j.biortech.2015.01.104
Babadi, M., Zalaghi, R., and Taghavi, M. (2019). A non-toxic polymer enhances sorghum mycorrhiza symbiosis for bioremediation of Cd. Mycorrhiza 29, 375–387. doi: 10.1007/s00572-019-00902-5
Bagheri, V., Shamshiri, M. H., Shirani, H., and Roosta, H. R. (2012). Nutrient uptake and distribution in mycorrhizal pistachio seedlings under drought stress. J. Agric. Sci. Technol. 14, 1591–1604.
Balestri, M., Ceccarini, A., Forino, L. M. C., Zelko, I., Martinka, M., Lux, A., et al. (2014). Cadmium uptake, localization and stress-induced morphogenic response in the fern Pteris vittata. Planta 239, 1055–1064. doi: 10.1007/s00425-014-2036-z
Bari, M. A., Akther, M. S., Reza, M. A., and Kabir, A. H. (2019). Cadmium tolerance is associated with the root-driven coordination of cadmium sequestration, iron regulation, and ROS scavenging in rice. Plant Physiol. Biochem. 136, 22–33. doi: 10.1016/j.plaphy.2019.01.007
Bashir, A., Rizwan, M., Zia-ur-Rehman, M., Zubair, M., Riaz, M., Qayyum, M. F., et al. (2020). Application of co-composted farm manure and biochar increased the wheat growth and decreased cadmium accumulation in plants under different water regimes. Chemosphere 246, 1–10. doi: 10.1016/j.chemosphere.2019.125809
Bashir, S., Gulshan, A. B., Iqbal, J., Husain, A., Alwahibi, M. S., Alkahtani, J., et al. (2021). Comparative role of animal manure and vegetable waste induced compost for polluted soil restoration and maize growth. Saudi J. Biol. Sci. 28, 2534–2539. doi: 10.1016/j.sjbs.2021.01.057
Bashir, S., Rizwan, M. S., Salam, A., Fu, Q., Zhu, J., Shaaban, M., et al. (2018). Cadmium immobilization potential of rice straw-derived biochar, zeolite and rock phosphate: extraction techniques and adsorption mechanism. Bull. Environ. Contam. Toxicol. 100, 727–732. doi: 10.1007/s00128-018-2310-z
Bhargava, A., Carmona, F. F., Bhargava, M., and Srivastava, S. (2012). Approaches for enhanced phytoextraction of heavy metals. J. Environ. Manag. 105, 103–120. doi: 10.1016/j.jenvman.2012.04.002
Bian, R. J., Li, L. Q., Bao, D. D., Zheng, J. W., Zhang, X. H., Zheng, J. F., et al. (2016). Cd immobilization in a contaminated rice paddy by inorganic stabilizers of calcium hydroxide and silicon slag and by organic stabilizer of biochar. Environ. Sci. Pollut. Res. 23, 10028–10036. doi: 10.1007/s11356-016-6214-3
Blanvillain, R., Kim, J. H., Wu, S., Lima, A., and Ow, D. W. (2009). OXIDATIVE STRESS 3 is a chromatin-associated factor involved in tolerance to heavy metals and oxidative stress. Plant J. 57, 654–665.
Bloem, E., Haneklaus, S., Haensch, R., and Schnug, E. (2016). EDTA application on agricultural soils affects microelement uptake of plants. Sci. Total Environ. 577, 166–173. doi: 10.1016/j.scitotenv.2016.10.153
Bojorquez, C., Frias-Espericueta, M. G., and Voltolina, D. (2016). Removal of cadmium and lead by adapted strains of Pseudomonas aeruginosa and Enterobacter cloacae. Rev. Int. Contam. Ambient. 32, 407–412.
Boostani, H. R., Najafi-Ghiri, M., and Mirsoleimani, A. (2019). The effect of biochars application on reducing the toxic effects of nickel and growth indices of spinach (Spinacia oleracea L.) in a calcareous soil. Environ. Sci. Pollut. Res. 26, 1751–1760. doi: 10.1007/s11356-018-3760-x
Bora, M. S., and Sarma, K. P. (2021). Anatomical and ultrastructural alterations in Ceratopterispteridoides under cadmium stress: a mechanism of cadmium tolerance. Ecotoxicol. Environ. Saf. 218:112285. doi: 10.1016/j.ecoenv.2021.112285
Bulak, P., Walkiewicz, A., and Brzezińska, M. (2014). Plant growth regulators- assisted phytoextraction. Biol. Plant 58, 1–8. doi: 10.1007/s10535-013-0382-5
Çanakci, S., and Dursun, B. (2012). The effect of pre-application of salicylic acid on some physiological and biochemical characteristics of tomato seedling (Lycopersicon esculentum L) growing in cadmium containing media. Afr. J. Biotechnol. 11, 3173–3178. doi: 10.5897/AJB11.2364
Cao, B. I., Ma, Q., Zhao, Q., Wang, L., and Xu, K. (2015). Effects of silicon on absorbed light allocation, antioxidant enzymes and ultrastructure of chloroplasts in tomato leaves under simulated drought stress. Sci. Hort. 194, 53–62. doi: 10.1016/j.scienta.2015.07.037
Chang, Q., Wei, D. F., Fan, W. Q., Pan, L., Hua, D. Z., and Guo, W. (2018). Effects of arbuscular mycorrhizal symbiosis on growth, nutrient and metal uptake by maize seedlings (Zea mays L.) grown in soils spiked with lanthanum and cadmium. Environ. Pollut. 241, 607–615. doi: 10.1016/j.envpol.2018.06.003
Chellaiah, E. R. (2018). Cadmium (heavy metals) bioremediation by Pseudomonas aeruginosa: a mini-review. Appl. Water Sci. 8:154. doi: 10.1007/s13201-018-0796-5
Chen, B., Luo, S., Wu, Y., Ye, J., Wang, Q., Xu, X., et al. (2017). The effects of the endophytic bacterium Pseudomonas fluorescens sasm05 and IAA on the plant growth and cadmium uptake of Sedum alfredii Hance. Front. Microbiol. 8:253. doi: 10.3389/fmicb.2017.02538
Chen, C.-T., Chen, T.-H., Lo, K.-F., and Chiu, C.-Y. (2004). Effects of proline on copper transport in rice seedlings under excess copper stress. Plant Sci. 166, 103–111. doi: 10.1016/j.plantsci.2003.08.015
Chen, H., Yang, X., Gielen, G., Mandal, S., Xu, S., Guo, J., et al. (2019). Effect of biochars on the bioavailability of cadmium and di-(2-ethylhexyl) phthalate to Brassica chinensis L. in contaminated soils. Sci. Total Environ. 678, 43–52. doi: 10.1016/j.scitotenv.2019.04.417
Chen, L., Long, C., Wang, D., and Yang, J. (2020). Phytoremediation of cadmium (Cd) and uranium (U) contaminated soils by Brassica juncea L. enhanced with exogenous application of plant growth regulators. Chemosphere 242:125112. doi: 10.1016/j.chemosphere.2019.125112
Chen, Q., Lu, X., Guo, X., Pan, Y., Yu, B., Tang, Z., et al. (2018a). Differential responses to Cd stress induced by exogenous application of Cu, Zn or Ca in the medicinal plant Catharanthus roseus. Ecotoxicol. Environ. Saf. 157, 266–275. doi: 10.1016/j.ecoenv.2018.03.055
Chen, H., Yang, X., Wang, P., Wang, Z., Li, M., and Zhao, F. J. (2018b). Dietary cadmium intake from rice and vegetables and potential health risk: a case study in Xiangtan, southern China. Sci. Total Environ. 639, 271–277. doi: 10.1016/j.scitotenv.2018.05.050
Chen, H., Zhang, W., Yang, X., Wang, P., McGrath, S. P., and Zhao, F. J. (2018c). Effective methods to reduce cadmium accumulation in rice grain. Chemosphere 207, 699–707. doi: 10.1016/j.chemosphere.2018.05.143
Chen, Z., Zhao, Y., Fan, L., Xing, L., and Yang, Y. (2015). Cadmium (Cd) localization in tissues of cotton (Gossypium hirsutum L.), and its phytoremediation potential for Cd-contaminated soils. Bull. Environ. Contam. Toxicol. 95, 784–789. doi: 10.1007/s00128-015-1662-x
Choppala, G., Saifullah, Bolan, N., Bibi, S., Iqbal, M., Rengel, Z., et al. (2014). Cellular mechanisms in higher plants governing tolerance to cadmium toxicity. Crit. Rev. Plant Sci. 33, 374–391. doi: 10.1016/j.ecoenv.2015.06.003
Clabeaux, B. L., Navarro, D. A. G., Aga, D. S., and Bisson, M. A. (2011). Cd tolerance and accumulation in the aquatic macrophyte, Chara australis: potential use for charophytes in phytoremediation. Environ. Sci. Technol. 45, 5332–5338. doi: 10.1021/es200720u
Clarke, J. M., Norvell, W. A., Clarke, F. R., and Buckley, W. T. (2002). Concentration of cadmium and other elements in the grain of near-isogenic durum lines. Can. J. Plant Sci. 82, 27–33. doi: 10.1021/acs.jafc.7b01946
Conolly, E. L., Fett, J. P., and Guerinot, M. L. (2002). Expression of the IRT1 metal transporter is controlled by metals at the levels of transcript and protein accumulation. Plant Cell 14, 1347–1357. doi: 10.1105/tpc.001263
Cui, G., Ai, S., Chen, K., and Wang, X. (2019). Arbuscular mycorrhiza augments cadmium tolerance in soybean by altering accumulation and partitioning of nutrient elements, and related gene expression. Ecotoxicol. Environ. Saf. 171, 231–239. doi: 10.1016/j.ecoenv.2018.12.093
Cuypers, A., Hendrix, S., Amaral dos Reis, R., De Smet, S., Deckers, J., Gielen, H., et al. (2016). Hydrogen peroxide, signaling in disguise during metal phytotoxicity. Front. Plant Sci. 7:470. doi: 10.3389/fpls.2016.00470
Cuypers, A., Plusquin, M., Remans, T., Jozefczak, M., Keunen, E., Gielen, H., et al. (2010). Cadmium stress: an oxidative challenge. Biometals 23, 927–940. doi: 10.1007/s10534-010-9329-x
da Silva Cunha, L. F., de Oliveira, V. P., do Nascimento, A. W. S., da Silva, B. R. S., Batista, B. L., Alsahli, A. A., et al. (2020). Leaf application of 24-epibrassinolide mitigates cadmium toxicity in young Eucalyptus urophylla plants by modulating leaf anatomy and gas exchange. Physiol. Plant 173, 67–87. doi: 10.1111/ppl.13182
Dad, F. P., Khan, W. U. D., Tanveer, M., Ramzani, P. M. A., Shaukat, R., and Muktadir, A. (2020). Influence of iron-enriched biochar on Cd sorption, its ionic concentration and redox regulation of radish under cadmium toxicity. Agriculture 11:1. doi: 10.3390/agriculture11010001
Das, N., Bhattacharya, S., and Maiti, M. K. (2016). Enhanced cadmium accumulation and tolerance in transgenic tobacco overexpressing rice metal tolerance protein gene OsMTP1 is promising for phytore mediation. Plant Physiol. Biochem. 105, 297–309. doi: 10.1016/j.plaphy.2016.04.049
Das, R., and Jayalekshmy, V. G. (2015). Mechanism of heavy metal tolerance and improvement of tolerance in crop plants. J. Glob. Biosci. 4, 2678–2698.
Da-wei, H. Zhong-hua, S. Qian-long, L., Wei, C. Xiang-jin, W., et al., (2018). Identification of QTLs associated with cadmium concentration in rice grains. J. Integr. Agric. 17, 60345–60347.
Dobrikova, A. G., Apostolova, E. L., Hanć, A., Yotsova, E., Borisova, P., Sperdouli, I., et al. (2021). Cadmium toxicity in Salvia sclarea L.: an integrative response of element uptake, oxidative stress markers, leaf structure and photosynthesis. Ecotoxicol. Environ. Saf. 209:111851. doi: 10.1016/j.ecoenv.2020.111851
Eissa, M. A. (2019). Effect of compost and biochar on heavy metals phytostabilization by the halophytic plant old man saltbush [Atriplex nummularia Lindl]. Soil Sediment. Contam. 28, 135–147. doi: 10.1080/15320383.2018.1551325
Elhiti, M., Yang, C., Chan, A., Durnin, D. C., Belmonte, M. F., Ayele, B. T., et al. (2012). Altered seed oil and glucosinolate levels in transgenic plants overexpressing the Brassica napus SHOOTMERISTEMLESS gene. J. Exp. Bot. 63, 4447–4461. doi: 10.1093/jxb/ers125
Elias, E. M., and Manthey, F. (2016). Registration of ‘Joppa’ durum wheat. J. Plant Regist. 10, 139–144. doi: 10.3198/jpr2015.11.0071crc
Elias, E. M., Manthey, F., and AbuHammad, W. (2015). Registration of ‘Carpio’ durum wheat. J. Plant Regist. 9, 78–82. doi: 10.3198/jpr2014.05.0030crc
Elobeid, M., Göbel, C., Feussner, I., and Polle, A. (2012). Cadmium interferes with auxin physiology and lignification in poplar. J. Exp. Bot. 63, 1413–1421. doi: 10.1093/jxb/err384
Elyamine, A. M., Moussa, M. G., Afzal, J., Rana, M. S., Imran, M., Zhao, X., et al. (2019). Modified rice straw enhanced cadmium (ii) immobilization in soil and promoted the degradation of phenanthrene in co-contaminated soil. Int. J. Mol. Sci. 20:2189. doi: 10.3390/ijms20092189
Erdem, H. (2021). The effects of biochars produced in different pyrolysis temperatures from agricultural wastes on cadmium uptake of tobacco plant. Saudi J. Biol. Sci. 28, 3965–3971. doi: 10.1016/j.sjbs.2021.04.016
Evangelou, M. W. H., Ebel, M., and Schaeffer, A. (2007). Chelate assisted phytoextraction of heavy metals from soil: effect, mechanism, toxicity, and fate of chelating agents. Chemosphere 68, 989–1003. doi: 10.1016/j.chemosphere.2007.01.062
Farooq, H., Asghar, H. N., Khan, M. Y., Saleem, M., and Zahir, Z. A. (2015). Auxin-mediated growth of rice in cadmium-contaminated soil. Turk. J. Agric. For. 39, 272–276. doi: 10.3906/tar-1405-54
Farooq, M., Ullah, A., Usman, M., and Siddique, K. H. M. (2020). Application of zinc and biochar help to mitigate cadmium stress in bread wheat raised from seeds with high intrinsic zinc. Chemosphere 260:127652. doi: 10.1016/j.chemosphere.2020.127652
Farooq, M. A., Niazi, A. K., Akhtar, J., Farooq, M., Souri, Z., Karimi, N., et al. (2019). Acquiring control: the evolution of ROS-Induced oxidative stress and redox signaling pathways in plant stress responses. Plant Physiol. Biochem. 141, 353–369. doi: 10.1016/j.plaphy.2019.04.039
French, K. E. (2017). Engineering mycorrhizal symbioses to alter plant metabolism and improve crop health. Front. Microbiol. 8:1403. doi: 10.3389/fmicb.2017.01403
Gallego, S. M., Pena, L. B., Barcia, R. A., Azpilicueta, C. E., Iannone, M. F., Rosales, E. P., et al. (2012). Unravelling cadmium toxicity and tolerance in plants: insight into regulatory mechanisms. Environ. Exp. Bot. 83, 33–46. doi: 10.1016/j.jhazmat.2017.04.058
García, A. C., Tavares, O. C. H., and de Oliveira, D. F. (2020). Biochar as agricultural alternative to protect the rice plant growth in fragile sandy soil contaminated with cadmium. Biocatal. Agric. Biotechnol. 29:101829. doi: 10.1016/j.bcab.2020.101829
Garg, N., and Chandel, S. (2015). Role of arbuscular mycorrhiza in arresting reactive oxygen species (ROS) and strengthening antioxidant defense in Cajanus cajan (L.) Millsp. nodules under salinity (NaCl) and cadmium (Cd) stress. Plant Growth Regul. 75, 521–534. doi: 10.1007/s10725-014-0016-8
Genchi, G., Sinicropi, M. S., Lauria, G., Carocci, A., and Catalano, A. (2020). The effects of cadmium toxicity. Int. J. Environ. Res. Public Health 17:3782.
Gerszberg, A., and Hnatuszko-Konka, K. (2017). Tomato tolerance to abiotic stress: a review of most often engineered target sequences. Plant Growth Regul. 83, 175–198. doi: 10.1007/s10725-017-0251-x
Ghani, A. (2011). Varietal differences in canola (Brassica napus L.) for the growth, yield and yield components exposed to cadmium stress. J. Anim. Plant Sci. 21, 57–59.
Ghassemi, H. R., and Mostajeran, A. (2018). TASOS1 and TATM20 genes expression and nutrient uptake in wheat seedlings may be altered via excess cadmium exposure and inoculation with Azospirillumbrasilense sp7 under saline condition. Appl. Ecol. Environ. Res. 16, 1797–1817. doi: 10.15666/aeer/1602_17971817
Gill, S. S., Khan, N. A., and Tuteja, N. (2011). Differential cadmium stress tolerance in five Indian mustard (Brassica juncea L.) cultivars: an evaluation of the role of antioxidant machinery. Plant Signal. Behav. 6, 293–300. doi: 10.4161/psb.6.2.15049
Glick, B. (2014). Bacteria with ACC deaminase can promote plant growth and help to feed the world. Microbiol. Res. 169, 30–39. doi: 10.1016/j.micres.2013.09.009
Goel, S., and Gautam, A. (2010). Effect of chelating agents on mobilization of metal from waste catalyst. Hydrometallurgy 101, 120–125.
Goix, S., Lévêque, T., Xiong, T. T., Schreck, E., Baeza-Squiban, A., Geret, F., et al. (2014). Environmental and health impacts of fine and ultrafine metallic particles: assessment of threat scores. Environ. Res. 133, 185–194. doi: 10.1016/j.envres.2014.05.015
Goncalves, J. F., Nicoloso, F. T., Becker, A. G., Pereira, L. B., Tabaldi, L. A., Cargnelutti, D., et al. (2009). Photosynthetic pigments content, δ-aminolevulinic acid dehydratase and acid phosphatase activities and mineral nutrients concentration in cadmium-exposed Cucumis sativus L. J. Biol. 64, 310–318. doi: 10.2478/s11756-009-0034-6
Gondor, O. K., Pál, M., Darkó, É, Janda, T., and Szalai, G. (2016). Salicylic acid and sodium salicylate alleviate cadmium toxicity to different extents in maize (Zea mays L.). PLoS One 11:e0160157. doi: 10.1371/journal.pone.0160157
Gong, X., Yin, L., Chen, J., and Gu, C. (2015). Overexpression of the iron transporter NtPIC1 in tobacco mediates tolerance to cadmium. Plant Cell Rep. 34, 1963–1973. doi: 10.1007/s00299-015-1843-4
Goswami, D., Thakker, J. N., and Dhandhukia, P. C. (2016). Portraying mechanics of plant growth promoting rhizobacteria (PGPR): a review. Cogent. Food Agric. 2:1127500.
Gothberg, A., Greger, E., Holm, K., and Bengtsson, B. E. (2004). Influence of nutrient levels on uptake and effects of mercury, cadmium, and lead in water spinach. J. Environ. Qual. 33, 1247–1255. doi: 10.2134/jeq2004.1247
Gouia, H., Suzuki, A., Brulfert, J., and Ghorbal, M. H. (2003). Effects of cadmium on the co-ordination of nitrogen and carbon metabolism in bean seedlings. J. Plant Physiol. 160, 367–376. doi: 10.1078/0176-1617-00785
Gruznova, K. A., Bashmakov, D. I., Miliauskiene, J., Vastakaite, V., Duchovskis, P., and Lukatkin, A. S. (2018). The effect of a growth regulator Ribav-Extra on winter wheat seedlings exposed to heavy metals. Zemdirbyste 105, 227–234. doi: 10.13080/z-a.2018.105.029
Gul, S., Naz, A., Khan, A., Nisa, S., and Irshad, M. (2016). Phytoavailability and leachability of heavy metals from contaminated soil treated with composted livestock manure. Soil Sediment. Contam. 25, 181–194. doi: 10.1080/15320383.2016.1112361
Guo, B., Liu, C., Liang, Y., Li, N., and Fu, Q. (2019). Salicylic acid signals plant defence against cadmium toxicity. Int. J. Mol. Sci. 20:2960. doi: 10.3390/ijms20122960
Guo, H., Hong, C., Chen, X., Xu, Y., Liu, Y., Jiang, D., et al. (2016). Different growth and physiological responses to cadmium of the three Miscanthus species. PLoS One 11:e0153475. doi: 10.1371/journal.pone.0153475
Guo, Z. H., and Miao, X. F. (2010). Growth changes and tissues anatomical characteristics of giant reed (Arundo donax L.) in soil contaminated with arsenic, cadmium and lead. J. Central S. Univ. Technol. 17, 770–777. doi: 10.1007/s11771-010-0555-8
Gusiatin, Z. M., and Klimiuk, E. (2012). Metal (Cu, Cd and Zn) removal and stabilization during multiple soil washing by saponin. Chemosphere 86, 383–391. doi: 10.1016/j.chemosphere.2011.10.027
Habiba, U., Ali, S., Farid, M., Shakoor, M. B., Rizwan, M., Ibrahim, M., et al. (2015). EDTA enhanced plant growth, antioxidant defense system, and phytoextraction of copper by Brassica napus L. Environ. Sci. Pollut. Res. 22, 1534–1544. doi: 10.1007/s11356-014-3431-5
Hafeez, F., Rizwan, M., Saqib, M., Yasmeen, T., Ali, S., Abbas, T., et al. (2019). Residual effect of biochar on growth, antioxidant defense and cadmium (Cd) accumulation in rice in a Cd contaminated saline soil. Pak. J. Agric. Sci. 56, 197–204.
Haider, F. U., Coulter, J. A., Liqun, C., Hussain, S., Cheema, S. A., Wu, J., et al. (2022). An overview on biochar production, its implications, and mechanisms of biochar-induced amelioration of soil and plant characteristics. Pedosphere 32, 107–130. doi: 10.1016/S1002-0160(20)60094-7
Haider, F. U., Liqun, C., Coulter, J. A., Cheema, S. A., Wu, J., Zhang, R., et al. (2021a). Cadmium toxicity in plants: impacts and remediation strategies. Ecotoxicol. Environ. Saf. 211:111887. doi: 10.1016/j.ecoenv.2020.111887
Haider, F. U., Coulter, J. A., Cheema, S. A., Farooq, M., Wu, J., Zhang, R., et al. (2021b). Co-application of biochar and microorganisms improves soybean performance and remediate cadmium-contaminated soil. Ecotoxicol. Environ. Saf. 214:112112. doi: 10.1016/j.ecoenv.2021.112112
Hajeb, P., Sloth, J. J., Shakibazadeh, S., Mahyudin, N. A., and Afsah-Hejri, L. (2014). Toxic elements in food: occurrence, binding, and reduction approaches. Compr. Rev. Food Sci. Food Saf. 13, 457–472. doi: 10.1111/1541-4337.12068
Hakmaoui, A., Ater, M., Boka, K., and Baron, M. (2007). Copper and cadmium tolerance, uptake and effect on chloroplast ultrastructure. Studies on Salix purpurea and Phragmites australis. Z. Naturforschung C 62, 417–426. doi: 10.1515/znc-2007-5-616
Halim, M. A., Majumder, R. K., and Zaman, M. N. (2015). Paddy soil heavy metal contamination and uptake in rice plants from the adjacent area of Barapukuria coal mine, northwest Bangladesh. Arab. J. Geosci. 8, 3391–3401. doi: 10.1007/s12517-014-1480-1
Hamid, Y., Tang, L., Hussain, B., Usman, M., Gurajala, H. K., Rashid, M. S., et al. (2019). Efficiency of lime, biochar, Fe containing biochar and composite amendments for Cd and Pb immobilization in a co-contaminated alluvial soil. Environ. Pollut. 257:113609. doi: 10.1016/j.envpol.2019.113609
Hamid, Y., Tang, L., Hussain, B., Usman, M., Lin, Q., Rashid, M. S., et al. (2020a). Organic soil additives for the remediation of cadmium contaminated soil sand their impact on the soil-plant system: a review. Sci. Total Environ. 707:136121. doi: 10.1016/j.scitotenv.2019.136121
Hamid, Y., Tang, L., Hussain, B., Usman, M., Gurajala, H. K., Rashid, M. S., et al. (2020b). Efficiency of lime, biochar, Fe containing biochar and composite amendments for Cd and Pb immobilization in a co-contaminated alluvial soil. Environ. Pollut. 257:113609.
Han, R. M., Lefèvre, I., Albacete, A., Pérez-Alfocea, F., Barba-Espín, G., Díaz-Vivancos, P., et al. (2013). Antioxidant enzyme activities and hormonal status in response to Cd stress in the wetland halophyte Kosteletzkya virginica under saline conditions. Physiol. Plant. 147, 352–368. doi: 10.1111/j.1399-3054.2012.01667.x
Hasan, M., Uddin, M., Ara-Sharmeen, F. I, Alharby, H., Alzahrani, Y., Hakeem, K. R., et al. (2019). Assisting phytoremediation of heavy metals using chemical amendments. Plants 8:295. doi: 10.3390/plants8090295
Hasanuzzaman, M., Alhaithloul, H., Parvin, K., Bhuyan, M., Tanveer, M., Mohsin, S. M., et al. (2019). Polyamine action under metal/metalloid stress: regulation of biosynthesis, metabolism, and molecular interactions. Int. J. Mol. Sci. 20:3215. doi: 10.3390/ijms20133215
Hasanuzzaman, M., Bhuyan, M., Zulfiqar, F., Raza, A., Mohsin, S. M., Mahmud, J. A., et al. (2020). Reactive oxygen species and antioxidant defense in plants under abiotic stress: revisiting the crucial role of a universal defense regulator. Antioxidants 9:681. doi: 10.3390/antiox9080681
Hashem, A., Abdallah, E. F., Alqarawi, A. A., Al-Huqail, A. A., Egamberdieva, D., and Wirth, S. (2016). Alleviation of cadmium stress in Solanum lycopersicum L. by arbuscular mycorrhizal fungi via induction of acquired systemic tolerance. Saudi J. Biol. Sci. 23, 272–281. doi: 10.1016/j.sjbs.2015.11.002
Hassan, W., Bano, R., Bashir, S., and Aslam, Z. (2015). Cadmium toxicity and soil biological index under potato (Solanum tuberosum L.) cultivation. Soil Res. 54, 460–468.
Hayat, S., Ali, B., Hasan, S. A., and Ahmad, A. (2007). Brassinosteroid enhanced the level of antioxidants under cadmium stress in Brassica juncea. Environ. Exp. Bot. 60, 33–41. doi: 10.1016/j.envexpbot.2006.06.002
Hayat, S., Alyemeni, M. N., and Hasan, S. A. (2012). Foliar spray of brassinosteroid enhances yield and quality of Solanum lycopersicum under cadmium stress. Saudi J. Biol. Sci. 19, 325–335. doi: 10.1016/j.sjbs.2012.03.005
Hayat, S., Hasan, S. A., Hayat, Q., and Ahmad, A. (2010). Brassinosteroids protect Lycopersicon esculentum from cadmium toxicity applied as shotgun approach. Protoplasma 239, 3–14. doi: 10.1007/s00709-009-0075-2
Hayat, S., Hayat, Q., Alyemeni, M. N., and Ahmad, A. (2013). Proline enhances antioxidative enzyme activity, photosynthesis and yield of Cicer arietinum L. exposed to cadmium stress. Acta Bot. Croatica 72, 323–335. doi: 10.2478/v10184-012-0019-3
He, D., Cui, J., Gao, M., Wang, W., Zhou, J., Yang, J., et al. (2019). Effects of soil amendments applied on cadmium availability, soil enzyme activity, and plant uptake in contaminated purple soil. Sci. Total Environ. 654, 1364–1371. doi: 10.1016/j.scitotenv.2018.11.059
He, J., Zhu, C., Ren, Y., Yan, Y., and Jiang, D. (2006). Genotypic variation in grain cadmium concentration of lowland rice. J. Plant Nutr. Soil Sci. 169, 711–716. doi: 10.1002/jpln.200525101
He, L., Ma, X., and Li, Z. (2016). Maize oxidative stress 2 homologs enhance cadmium tolerance in Arabidopsis through activation of a putative SAM-dependent methyltransferase gene. Plant Physiol. 171, 1675–1685. doi: 10.1104/pp.16.00220
He, S., Wu, Q., and He, Z. (2014). Synergetic effects of DA-6/GA3 with EDTA on plant growth, extraction and detoxification of Cd by Lolium perenne. Chemosphere 117, 132–138. doi: 10.1016/j.chemosphere.2014.06.015
Hong, K., Tokunaga, S., and Kajiuchi, T. (2002). Evaluation of remediation process with plant derived biosurfactant for recovery of heavy metals from contaminated soils. Chemosphere 49, 379–387. doi: 10.1016/S0045-6535(02)00321-1
Hossain, M. A., Piyatida, P., da Silva, J. A. T., and Fujita, M. (2012). Molecular mechanism of heavy metal toxicity and tolerance in plants: central role of glutathione in detoxification of reactive oxygen species and methylglyoxal and in heavy metal chelation. J. Bot. 2012:872875.
Hu, W., Zhang, Y., Huang, B., and Teng, Y. (2017). Soil environmental quality in greenhouse vegetable production systems in eastern China: current status and management strategies. Chemosphere 170, 183–195. doi: 10.1016/j.chemosphere.2016.12.047
Hu, X., Liu, X., Zhang, X., Cao, L., Chen, J., and Yu, H. (2017). Increased accumulation of Pb and Cd from contaminated soil with Scirpustriqueter by the combined application of NTA and APG. Chemosphere 188, 397–402. doi: 10.1016/j.chemosphere.2017.08.173
Huang, X., and Han, B. (2014). Natural variations and genome-wide association studies in crop plants. Annu. Rev. Plant Biol. 65, 531–551. doi: 10.1146/annurev-arplant-050213-035715
Huang, D. F., Ling-Lin, X., Li-Nian, Y., Zhi-Qin, W., and Jian-Chang, Y. (2008). Comparison of agronomic and physiological traits of rice genotypes differing in cadmium-tolerance. Acta Agron. Sin. 34, 809–817. doi: 10.3724/sp.j.1006.2008.00809
Huang, H., Luo, L., Huang, L., Zhang, J., Gikas, P., and Zhou, Y. (2020). Effect of manure compost on distribution of Cu and Zn in rhizosphere soil and heavy metal accumulation by Brassica juncea. Water Air Soil Pollut. 231, 1–10.
Huang, J., Liu, Z., Li, S., Xu, B., Gong, Y., Yang, Y., et al. (2016). Isolation and engineering of plant growth promoting rhizobacteria Pseudomonas aeruginosa for enhanced cadmium bioremediation. J. Gen. Appl. Microbiol. 62, 258–265. doi: 10.2323/jgam.2016.04.007
Huang, Q., Yu, Y., Wan, Y., Wang, Q., Luo, Z., Qiao, Y., et al. (2018). Effects of continuous fertilization on bioavailability and fractionation of cadmium in soil and its uptake by rice (Oryza sativa L.). J. Environ. Manag. 215, 13–21. doi: 10.1016/j.jenvman.2018.03.036
Huang, X., Ho, S. H., Zhu, S., Ma, F., Wu, J., Yang, J., et al. (2017). Adaptive response of arbuscular mycorrhizal symbiosis to accumulation of elements and translocation in Phragmites australis affected by cadmium stress. J. Environ. Manag. 197, 448–455. doi: 10.1016/j.jenvman.2017.04.014
Hussain, A., Ali, S., Rizwan, M., ur Rehman, M. Z., Javed, M. R., Imran, M., et al. (2018). Zinc oxide nanoparticles alter the wheat physiological response and reduce the cadmium uptake by plants. Environ. Pollut. 242, 1518–1526.
Hussain, B., Ashraf, M. N., Abbas, A., Li, J., and Farooq, M. (2021). Cadmium stress in paddy fields: effects of soil conditions and remediation strategies. Sci. Total Environ. 754:142188. doi: 10.1016/j.scitotenv.2020.142188
Huybrechts, M., Hendrix, S., Bertels, J., Beemster, G. T. S., Vandamme, D., and Cuypers, A. (2020). Spatial analysis of the rice leaf growth zone under controlled and cadmium-exposed conditions. Environ. Exp. Bot. 177:104120. doi: 10.1016/j.envexpbot.2020.104120
Ibrahim, M., Li, G., Chan, F. K. S., Kay, P., Liu, X. X., Firbank, L., et al. (2019). Biochar effects potentially toxic elements and antioxidant enzymes in Lactuca sativa L. grown in multi-metals contaminated soil. Environ. Technol. Innov. 15:100427.
Ijaz, M., Rizwan, M. S., Sarfraz, M., Ul-Allah, S., Sher, A., Sattar, A., et al. (2020). Biochar reduced cadmium uptake and enhanced wheat productivity in alkaline contaminated soil. Int. J. Agric. Biol. 24, 1633–1640. doi: 10.17957/IJAB/15.1605
Ishikawa, S., Abe, T., Kuramata, M., Yamaguchi, M., Ando, T., Yamamoto, T., et al. (2010). A major quantitative trait locus for increasing cadmium-specific concentration in rice grain is located on the short arm of chromosome 7. J. Exp. Bot. 61, 923–934. doi: 10.1093/jxb/erp360
Ishikawa, S., Ae, N., and Yano, M. (2005). Chromosomal regions with quantitative trait loci controlling cadmium concentration in brown rice (Oryza sativa). N. Phytol. 168, 345–350. doi: 10.1111/j.1469-8137.2005.01516.x
Ishizaki, T. (2016). CRISPR/Cas9 in rice can induce new mutations in later generations, leading to chimerism and unpredicted segregation of the targeted mutation. Mol. Breed. 36:165.
Jaishankar, M., Tseten, T., Anbalagan, N., Mathew, B. B., and Beeregowda, K. N. (2014). Toxicity, mechanism and health effects of some heavy metals. Interdiscip. Toxicol. 7:60. doi: 10.2478/intox-2014-0009
Jan, S. U., Jamal, A., Sabar, M. A., Ortas, I., Isik, M., Aksahin, V., et al. (2020). Impact of Zea mays L. waste derived biochar on cadmium immobilization and wheat plant growth. Pak. J. Agri. Sci. 57, 1201–1210.
Janeczko, A., Koscielniak, J., Pilipowicz, M., Szarek-Lukaszewska, G., and Skoczowski, A. (2005). Protection of winter rape photosystem 2 by 24-epibrassinolide under cadmium stress. Photosynthetica 43, 293–298. doi: 10.1007/s11099-005-0048-4
Janeeshma, E., and Puthur, J. T. (2020). Direct and indirect influence of arbuscular mycorrhizae on enhancing metal tolerance of plants. Arch. Microbiol. 202, 1–16. doi: 10.1007/s00203-019-01730-z
Janoušková, M., Pavlíková, D., and Vosátka, M. (2006). Potential contribution of arbuscular mycorrhiza to cadmium immobilisation in soil. Chemosphere 65, 1959–1965. doi: 10.1016/j.chemosphere.2006.07.007
Januškaitienė, I. (2010). Impact of low concentration of cadmium on photosynthesis and growth of pea and barley. Environ. Res. Engg. Manage. 53, 24–29.
Jebara, S. H., Chiboub, M., and Jebara, M. (2018). “Antioxidant responses and gene level expressions of Sulla coronaria inoculated by heavy metals resistant plant growth promoting bacteria under cadmium stress,” in Recent Advances in Environmental Science from the Euro-Mediterranean and Surrounding Regions, Advances in Science, Technology & Innovation, eds A. Kallel, M. Ksibi, H. B. Dhia, and N. Khélifi (Cham: Springer International Publishing).
Jegadeesan, S., Yu, K., Povsa, V., Gawalko, E., Morrison, M. J., Shi, C., et al. (2010). Mapping and validation of simple sequence repeat markers linked to a major gene controlling seed cadmium accumulation in soybean [Glycine max (L.) Merr]. Theor. Appl. Genet. 121, 283–294. doi: 10.1007/s00122-010-1309-6
Jha, U. C., and Bohra, A. (2016). Genomics enabled breeding approaches for improving cadmium stress tolerance in plants. Euphytica 208, 1–31.
Jianfeng, W., Zhang, Y., Jin, J., Li, Q., Chenzhou, Z., Wenbin, N., et al. (2017). An intact cytokinin-signaling pathway is required for Bacillus sp. LZR216-promoted plant growth and root system architecture alteration in Arabidopsis thaliana seedlings. Plant Growth Regul. 84, 507–518. doi: 10.1007/s10725-017-0357-1
Jiang, M., Liu, S., Li, Y., Li, X., Luo, Z., Song, H., et al. (2019). EDTA-facilitated toxic tolerance, absorption and translocation and phytoremediation of lead by dwarf bamboos. Ecotoxicol. Environ. Saf. 170, 502–512.
Jiang, Q. Y., Zhuo, F., Long, S. H., Di-Zhao, H., Yang, D. J., Ye, Z. H., et al. (2016). Can arbuscular mycorrhizal fungi reduce Cd uptake and alleviate Cd toxicity of Lonicera japonica grown in Cd-added soils? Sci. Rep. 6:21805. doi: 10.1038/srep21805
Jianv, L., Qixing, Z., and Song, W. (2010). Evaluation of chemical enhancement on phytoremediation effect of Cd-contaminated soils with Calendula officinalis L. Int. J. Phytoremediat. 12, 503–515. doi: 10.1080/15226510903353112
Joshi, P. M., and Juwarkar, A. A. (2009). In vivo studies to elucidate the role of extracellular polymeric substances from Azotobacter in immobilization of heavy metals. Environ. Sci. Technol. 43, 5884–5889. doi: 10.1021/es900063b
Jung, M. C. (2008). Heavy metal concentration in soils and factors affecting metal uptake by plants in the vicinity of a Korean Cu–W mine. Sensors 8, 2413–2423. doi: 10.3390/s8042413
Jung, C., Capistrano-Gossmann, G., Braatz, J., Sashidhar, N., and Melzer, S. (2018). Recent developments in genome editing and applications in plant breeding. Plant Breed. 137, 1–9.
Kabata-Pendias, A. (1993). Behavioural properties of trace metals in soils. Appl. Geochem. 8, 3–9. doi: 10.1016/S0883-2927(09)80002-4
Kabata-Pendias, A., and Sadurski, W. (2004). “Trace elements and compounds in soil,” in Elements and Their Compounds in the Environment: Occurrence, Analysis and Biological Relevance, eds E. Merian, M. Anke, M. Ihnat, and M. Stoeppler (Hoboken, NJ: Wiley), 79–99.
Kamran, M., Malik, Z., Parveen, A., Huang, L., Riaz, M., Bashir, S., et al. (2020). Ameliorative effects of biochar on rapeseed (Brassica napus L.) growth and heavy metal immobilization in soil irrigated with untreated wastewater. J. Plant Growth Regul. 39, 266–281.
Kamran, M., Malik, Z., Parveen, A., Zong, Y., Abbasi, G. H., Rafiq, M. T., et al. (2019). Biochar alleviates Cd phytotoxicity by minimizing bioavailability and oxidative stress in pak choi (Brassica chinensis L.) cultivated in Cd-polluted soil. J. Environ. Manag. 250:109500. doi: 10.1016/j.jenvman.2019.109500
Kapoor, D., Sharma, R., Handa, N., Kaur, H., Rattan, A., Yadav, P., et al. (2015). Redox homeostasis in plants under abiotic stress: role of electron carriers, energy metabolism mediators and proteinaceous thiols. Front. Environ. Sci. 3:13. doi: 10.3389/fenvs.2015.00013
Kapoor, D., Singh, S., Kumar, V., Romero, R., Prasad, R., and Singh, J. (2019). Antioxidant enzymes regulation in plants in reference to reactive oxygen species (ROS) and reactive nitrogen species (RNS). Plant Gene 19:100182.
Ke, T., Guo, G., Liu, J., Zhang, C., Tao, Y., Wang, P., et al. (2021). Improvement of the Cu and Cd phytostabilization efficiency of perennial ryegrass through the inoculation of three metal-resistant PGPR strains. Environ. Pollut. 271:116314. doi: 10.1016/j.envpol.2020.116314
Khan, M. A., Khan, S., Khan, A., and Alam, M. (2017). Soil contamination with cadmium, consequences and remediation using organic amendments. Sci. Total Environ. 601, 1591–1605. doi: 10.1016/j.scitotenv.2017.06.030
Khan, N., Samiullah, Singh, S., and Nazar, R. (2007). Activities of antioxidative enzymes, sulphur assimilation, photosynthetic activity and growth of wheat (Triticum aestivum) cultivars differing in yield potential under cadmium stress. J. Agron. Crop Sci. 193, 435–444.
Khanna, K., Jamwal, V. L., Kohli, S. K., Gandhi, S. G., Ohri, P., Bhardwaj, R., et al. (2019). Plant growth promoting rhizobacteria induced Cd tolerance in Lycopersicon esculentum through altered antioxidative defense expression. Chemosphere 217, 463–474. doi: 10.1016/j.chemosphere.2018.11.005
Kirkham, M. B. (2006). Cadmium in plants on polluted soils: effects of soil factors, hyperaccumulation, and amendments. Geoderma 137, 19–32. doi: 10.1016/j.geoderma.2006.08.024
Kloepper, J. W. (1994). “Plant growth-promoting rhizobacteria,” in Plant Growth and Health Promoting Bacteria, ed. D. K. Maheshwari (Cham: Springer Science & Business Media), 137–166.
Knox, R. E., Pozniak, C. J., Clarke, F. R., Clarke, J. M., Houshmand, S., and Singh, A. K. (2009). Chromosomal location of the cadmium uptake gene (Cdu1) in durum wheat. Genome 52, 741–747. doi: 10.1139/g09-042
Koprivova, A., North, K. A., and Kopriva, S. (2008). Complex signaling network in regulation of adenosine 5′-phosphosulfate reductase by salt stress in Arabidopsis roots. Plant Physiol. 146, 1408–1420. doi: 10.1104/pp.107.113175
Krantev, A., Yordanova, R., Janda, T., Szalai, G., and Popova, L. (2008). Treatment with salicylic acid decreases the effect of cadmium on photosynthesis in maize plants. J. Plant Physiol. 165, 920–931. doi: 10.1016/j.jplph.2006.11.014
Krujatz, F. (2012). Assessing the toxic effects of nickel, cadmium and EDTA on growth of the plant growth-promoting rhizobacterium Pseudomonas brassicacearum. Water Air Soil Pollut. 223, 1281–1293.
Kubier, A., Wilkin, R. T., and Pichler, T. (2019). Cadmium in soils and groundwater: a review. Appl. Geochem. 108:104388. doi: 10.1016/j.apgeochem.2019.104388
Kumar, P., Edelstein, M., Cardarelli, M., Ferri, E., and Colla, G. (2015). Grafting affects growth, yield, nutrient uptake, and partitioning under cadmium stress in tomato. HortScience 50, 1654–1661.
Küpper, H., Parameswaran, A., Leitenmaier, B., Trtilek, M., and Šetlík, I. (2007). Cadmium-induced inhibition of photosynthesis and long-term acclimation to cadmium stress in the hyperaccumulator Thlaspicaerulescens. New Phytol. 175, 655–674. doi: 10.1111/j.1469-8137.2007.02139.x
Lambrechts, T., Gustot, Q., Couder, E., Houben, D., Iserentant, A., and Lutts, S. (2011). Comparison of EDTA-enhanced phytoextraction and phytostabilisation strategies with Lolium perenne on a heavy metal contaminated soil. Chemosphere 85, 1290–1298. doi: 10.1016/j.chemosphere.2011.07.034
Lan, H. X., Wang, Z. F., Wang, Q. H., Wang, M. M., Bao, Y. M., Huang, J., et al. (2012). Characterization of a vacuolar zinc transporter OZT1 in rice (Oryza sativa L.). Mol. Biol. Rep. 40, 1201–1210.
Lata, S., Kaur, H. P., and Mishra, T. (2019). Cadmium bioremediation: a review. Int. J. Pharm. Sci. Res. 10, 4120–4128. doi: 10.13040/IJPSR.0975-8232
Lee, K., and Back, K. (2017). Overexpression of rice serotonin N-acetyltransferase 1 in transgenic rice plants confers resistance to cadmium and senescence and increases grain yield. J. Pineal Res. 62, 1–14. doi: 10.1111/jpi.12392
Leegood, R. (1993). “Carbon metabolism,” in Photosynthesis and Production in a Changing Environment, eds D. O. Hall, J. M. O. Scurlock, H. R. Bolhar-Nordenkampf, R. C. Leegood, and S. P. Long (Cham: Springer), 247–267.
Lehmann, A., Leifheit, E., and Rillig, M. (2017). “Mycorrhizas and soil aggregation,” in Mycorrhizal Mediation of Soil: Fertility, Structure, and Carbon Storage, eds N. C. Johnson, C. Gehring, and J. Jansa (Amsterdan: Elsevier). doi: 10.1111/j.1469-8137.2004.01181.x
Lehmann, A., and Rillig, M. C. (2015). Arbuscular mycorrhizal contribution to copper, manganese and iron nutrient concentrations in crops - A meta-analysis. Soil Biol. Biochem. 81, 147–158. doi: 10.1016/j.soilbio.2014.11.013
Lentini, M., De Lillo, A., Paradisone, V., Liberti, D., Landi, S., and Esposito, S. (2018). Early responses to cadmium exposure in barley plants: effects on biometric and physiological parameters. Acta Physiol. Plant. 40, 1–11. doi: 10.1007/s11738-018-2752-2
Li, D. D., and Zhou, D. M. (2012). Acclimation of wheat to low-level cadmium or zinc generates its resistance to cadmium toxicity. Ecotoxicol. Environ. Saf. 79, 264–271.
Li, F., Li, Z., Mao, P., Li, Y., Li, Y., McBride, M. B., et al. (2019). Heavy metal availability, bioaccessibility, and leachability in contaminated soil: effects of pig manure and earthworms. Environ. Sci. Pollut. Res. 26, 20030–20039. doi: 10.1007/s11356-018-2080-5
Li, J., Yu, J., Du, D., Liu, J., Lu, H., and Yan, C. (2019). Analysis of anatomical changes and cadmium distribution in Aegicerascorniculatum (L.) Blanco roots under cadmium stress. Mar. Pollut. Bull. 149:110536. doi: 10.1016/j.marpolbul.2019.110536
Li, L., Chen, J., He, Q., Daud, M. K., and Zhu, S. (2012). Characterization of physiological traits, yield and fiber quality in three upland cotton cultivars grown under cadmium stress. Aust. J. Crop Sci. 6, 1527–1533.
Li, Q., Lu, Y., Shi, Y., Wang, T., Ni, K., Xu, L., et al. (2013). Combined effects of cadmium and fluoranthene on germination, growth and photosynthesis of soybean seedlings. J. Environ. Sci. 25, 1936–1946.
Li, S., Chen, J., Islam, E., Wang, Y., Wu, J., Ye, Z., et al. (2016). Cadmium-induced oxidative stress, response of antioxidants and detection of intracellular cadmium in organs of moso bamboo (Phyllostachys pubescens) seedlings. Chemosphere 153, 107–114. doi: 10.1016/j.chemosphere.2016.02.062
Li, S., Sun, X., Li, S., Liu, Y., Ma, Q., and Zhou, W. (2021). Effects of amendments on the bioavailability, transformation and accumulation of heavy metals by pakchoi cabbage in a multi-element contaminated soil. RSC Adv. 11, 4395–4405. doi: 10.1039/D0RA09358K
Li, W., Wu, S., Liu, Y., Jin, G., Zhao, H., Fan, L., et al. (2016). Genome-wide profiling of genetic variation in Agrobacterium-transformed rice plants. J. Zhejiang Univ. Sci. B 17, 992–996. doi: 10.1631/jzus.B1600301
Li, X., Gitau, M. M., Han, S., Fu, J., and Xie, Y. (2017a). Effects of cadmium-resistant fungi Aspergillus aculeatus on metabolic profiles of bermudagrass [Cynodondactylon (L.) Pers.] under Cd stress. Plant Physiol. Biochem. 114, 38–50. doi: 10.1016/j.plaphy.2017.02.014
Li, X., Han, S., Wang, G., Liu, X., Amombo, E., Xie, Y., et al. (2017b). The fungus Aspergillus aculeatus enhances salt-stress tolerance, metabolite accumulation, and improves forage quality in perennial ryegrass. Front. Microbiol. 8:1664. doi: 10.3389/fmicb.2017.01664
Li, Y., Luo, J., Yu, J., Xia, L., Zhou, C., Cai, L., et al. (2018). Improvement of the phytoremediation efficiency of Neyraudiareynaudiana for lead-zinc mine-contaminated soil under the interactive effect of earthworms and EDTA. Sci. Rep. 8:6417. doi: 10.1038/s41598-018-24715-2
Li, Y., Wang, L., Yang, L., and Li, H. (2014). Dynamics of rhizosphere properties and antioxidative responses in wheat (Triticum aestivum L.) under cadmium stress. Ecotoxicol. Environ. Saf. 102, 55–61. doi: 10.1016/j.ecoenv.2014.01.004
Li, Z., Zhang, R., and Zhang, H. (2018). Effects of plant growth regulators (DA-6 and 6-BA) and EDDS chelator on phytoextraction and detoxification of cadmium by Amaranthus hybridus Linn. Int. J. Phytoremed. 20, 1121–1128. doi: 10.1080/15226514.2017.1365348
Liao, M., and Xie, X. M. (2004). Cadmium release in contaminated soils due to organic acids. Pedosphere 14, 223–228.
Lin, H., Fang, C., Li, Y., Lin, W., He, J., Lin, R., et al. (2016). Effect of silicon on grain yield of rice under cadmium-stress. Acta Physiol. Plant. 38, 1–13. doi: 10.1007/s11738-016-2177-8
Lin, R., Wang, X., Luo, Y., Du, W., Guo, H., and Yin, D. (2007). Effects of soil cadmium on growth, oxidative stress and antioxidant system in wheat seedlings (Triticum aestivum L.). Chemosphere 69, 89–98. doi: 10.1016/j.chemosphere.2007.04.041
Liu, F., Liu, X., Ding, C., and Wu, L. (2015). The dynamic simulation of rice growth parameters under cadmium stress with the assimilation of multi-period spectral indices and crop model. Field Crops Res. 183, 225–234. doi: 10.1016/j.fcr.2015.08.004
Liu, J., Qian, M., Cai, G., Yang, J., and Zhu, Q. (2007). Uptake and translocation of Cd in different rice cultivars and the relation with Cd accumulation in rice grain. J. Hazard. Mater. 143, 443–447. doi: 10.1016/j.jhazmat.2006.09.057
Liu, J. N., Zhou, Q., Sun, T., Ma, L. Q., and Wang, S. (2008). Identification of and chemical enhancement of two ornamental plants for phytoremediation. Bull. Environ. Contam. Toxicol. 80, 260–265. doi: 10.1007/s00128-008-9357-1
Liu, J. N., Zhou, Q. X., Wang, S., and Sun, T. (2009). Cadmium tolerance and accumulation of Althaea rosea Cav. and its potential as a hyperaccumulation under chemical enhancement. Environ. Monit. Assess. 149, 419–427. doi: 10.1007/s10661-008-0218-5
Liu, L., Li, J. W., Yue, F. X., Yan, X. W., Wang, F. Y., Bloszies, S., et al. (2018). Effects of arbuscular mycorrhizal inoculation and biochar amendment on maize growth, cadmium uptake and soil cadmium speciation in Cd-contaminated soil. Chemosphere 194, 495–503. doi: 10.1016/j.chemosphere.2017.12.025
Liu, M., Sun, J., Li, Y., and Xiao, Y. (2017). Nitrogen fertilizer enhances growth and nutrient uptake of Medicago sativa inoculated with Glomus tortuosum grown in Cd-contaminated acidic soil. Chemosphere 167, 204–211.
Liu, X., Chen, S., Chen, M., Zheng, G., Peng, Y., Shi, X., et al. (2019). Association study reveals genetic loci responsible for arsenic, cadmium and lead accumulation in rice grain in contaminated farmlands. Front. Plant Sci. 10:61. doi: 10.3389/fpls.2019.00061
Liu, Y., Xiao, T., Ning, Z., Li, H., Tang, J., and Zhou, G. (2013). High cadmium concentration in soil in the Three Gorges region: geogenic source and potential bioavailability. Appl. Geochem. 37, 149–156. doi: 10.1016/j.apgeochem.2013.07.022
Liza, S. J., Shethi, K. J., and Rashid, P. (2020). Effects of cadmium on the anatomical structures of vegetative organs of chickpea (Cicer arientinum L.). Dhaka Univ. J. Biol. Sci. 29, 45–52. doi: 10.3329/dujbs.v29i1.46530
Long, C., Wang, D., Chen, L., Jiang, W. J., and Xiang, M. W. (2017). Effect of four kinds of phytohormones on U and Cd accumulation in Helianthus annuus. Chin. J. Environ. Eng. 11, 3251–3256.
Lozano-Rodriguez, E., Hernandez, L. E., Bonay, P., and Carpena-Ruiz, R. O. (1997). Distribution of cadmium in shoot and root tissues1. J. Exp. Bot. 48, 123–128. doi: 10.1093/jxb/48.1.123
Luo, J., Li, X., Jin, Y., Traore, I., Dong, L., Yang, G., et al. (2020). Effects of arbuscular mycorrhizal fungi glomus mosseae on the growth and medicinal components of Dysosma versipellis under copper stress. Bull. Environ. Contam. Toxicol. 107, 924–930. doi: 10.1007/s00128-019-02780-1
Luo, J. S., Huang, J., Zeng, D. L., Peng, J. S., Zhang, G. B., Ma, H. L., et al. (2018). A defensin-like protein drives cadmium efflux and allocation in rice. Nat. Commun. 9, 1–9. doi: 10.1038/s41467-018-03088-0
Ma, J., Ni, X., Huang, Q., Liu, D., and Ye, Z. (2021). Effect of bamboo biochar on reducing grain cadmium content in two contrasting wheat genotypes. Environ. Sci. Pollut. Res. 28, 17405–17416. doi: 10.1007/s11356-020-12007-0
Mahajan, P., and Kaushal, J. (2018). Role of phytoremediation in reducing cadmium toxicity in soil and water. J. Toxicol. 2018, 1–16. doi: 10.1155/2018/4864365
Maksimović, I., Kastori, R., Krstić, L., and Luković, J. (2007). Steady presence of cadmium and nickel affects root anatomy, accumulation and distribution of essential ions in maize seedlings. Biol. Plant. 51, 589–592. doi: 10.1007/s10535-007-0129-2
Maksymiec, W., and Krupa, Z. (2002). Jasmonic acid and heavy metals in Arabidopsis plants-a similar physiological response to both stressors? J. Plant Physiol. 159, 509–515. doi: 10.1078/0176-1617-00610
Maksymiec, W., and Krupa, Z. (2006). The effects of short-term exposition to Cd, excess Cu ions and jasmonate on oxidative stress appearing in Arabidopsis thaliana. Environ. Exp. Bot. 57, 187–194. doi: 10.1016/j.envexpbot.2005.05.006
Mani, D., and Kumar, C. (2014). Biotechnological advances in bioremediation of heavy metals contaminated ecosystems: an overview with special reference to phytoremediation. Int. J. Environ. Technol. 11, 843–872. doi: 10.1007/s13762-013-0299-8
Manzoor, M., Gul, I., Kallerhoff, J., and Arshad, M. (2019). Fungi-assisted phytoextraction of lead: tolerance, plant growth—promoting activities and phytoavailability. Environ. Sci. Pollut. Res. 26, 23788–23797. doi: 10.1007/s11356-019-05656-3
Martínez Domínguez, D., Córdoba García, F., Canalejo Raya, A., and Torronteras Santiago, R. (2010). Cadmium-induced oxidative stress and the response of the antioxidative defense system in Spartina densiflora. Physiol. Plant. 139, 289–302. doi: 10.1111/j.1399-3054.2010.01368.x
Maurya, A. K. (2020). “Oxidative stress in crop plants,” in Agronomic Crops, ed. M. Hasanuzzaman (Singapore: Springer).
McCouch, S. R., Wright, M. H., Tung, C. W., Maron, L. G., McNally, K. L., Fitzgerald, M., et al. (2016). Open access resources for genome-wide association mapping in rice. Nat. Commun. 7, 1–14. doi: 10.1038/ncomms10532
Medynska-Juraszek, A., and Cwielag-Piasecka, I. (2020). Effect of biochar application on heavy metal mobility in soils impacted by copper smelting processes. Pol. J. Environ. Stud. 29, 1749–1757. doi: 10.15244/pjoes/108928
Medyńska-Juraszek, A., Bednik, M., and Chohura, P. (2020). Assessing the influence of compost and biochar amendments on the mobility and uptake of heavy metals by green leafy vegetables. Int. J. Environ. Res. Public Health 17:7861.
Mehdizadeh, L., Farsaraei, S., and Moghaddam, M. (2021). Biochar application modified growth and physiological parameters of Ocimumciliatum L. and reduced human risk assessment under cadmium stress. J. Hazard. Mater. 409:124954. doi: 10.1016/j.jhazmat.2020.124954
Meng, D., Li, J., Liu, T., Liu, Y., Yan, M., Hu, J., et al. (2019). Effects of redox potential on soil cadmium solubility: insight into microbial community. J. Environ. Sci. 75, 224–232. doi: 10.1016/j.jes.2018.03.032
Meng, J., Zhong, L., Wang, L., Liu, X., Tang, C., Chen, H., et al. (2018). Contrasting effects of alkaline amendments on the bioavailability and uptake of Cd in rice plants in a Cd-contaminated acid paddy soil. Environ. Sci. Pollut. Res. 25, 8827–8835. doi: 10.1007/s11356-017-1148-y
Menguer, P. K., Farthing, E., Peaston, K. A., Ricachenevsky, F. K., Fett, J. P., and Williams, L. E. (2013). Functional analysis of the rice vacuolar zinc transporter OsMTP1. J. Exp. Bot. 64, 2871–2883. doi: 10.1093/jxb/ert136
Mishra, S., Tripathi, R. D., Srivastava, S., Dwivedi, S., Trivedi, P. K., Dhankher, O. P., et al. (2009). Thiol metabolism play significant role during cadmium detoxification by Ceratophyllumdemersum L. Bioresour. Technol. 100, 2155–2161. doi: 10.1016/j.biortech.2008.10.041
Mizushima, M., Ferreira, B., França, M., Almeida, A. A., Cortez, P., Silva, J., et al. (2019). Ultrastructural and metabolic disorders induced by short-term cadmium exposure in Avicenniaschaueriana plants and its excretion through leaf salt glands. Plant Biol. 21, 844–853. doi: 10.1111/plb.12992
Mokarram-Kashtiban, S., Hosseini, S. M., Kouchaksaraei, M. T., and Younesi, H. (2019). Biochar improves the morphological, physiological and biochemical properties of white willow seedlings in heavy metal-contaminated soil. Arch. Biol. Sci. 71, 281–291. doi: 10.2298/ABS180918010M
Molina, A. S., Lugo, M. A., PerezChaca, M. V., Vargas-Gil, S., Zirulnik, F., Leporati, J., et al. (2020). Effect of arbuscular mycorrhizal colonization on cadmium-mediated oxidative stress in Glycine max (L.) Merr. Plants 9:108. doi: 10.3390/plants9010108
Mondal, S. C., Sarma, B., Farooq, M., Nath, D. J., and Gogoi, N. (2020). Cadmium bioavailability in acidic soils under bean cultivation: role of soil additives. Int. J. Environ. Sci. Technol. 17, 153–160. doi: 10.1007/s13762-019-02263-0
Mongkhonsin, B., Nakbanpote, W., Meesungnoen, O., and Prasad, M. N. V. (2019). “Adaptive and tolerance mechanisms in herbaceous plants exposed to cadmium,” in Cadmium Toxicity and Tolerance in Plants, eds M. Hasanuzzaman, M. N. V. Prasad, and M. Fujita (Cambridge, MA: Academic Press), 73–109.
Moradi, R., Pourghasemian, N., and Naghizadeh, M. (2019). Effect of beeswax waste biochar on growth, physiology and cadmium uptake in saffron. J. Clean. Prod. 229, 1251–1261. doi: 10.1016/j.jclepro.2019.05.047
Moreira, F. M. D. S., Ferreira, P. A. A., Vilela, L. A. F., and Carneiro, M. A. C. (2015). “Symbioses of plants with rhizobia and mycorrhizal fungi in heavy metal-contaminated tropical soils,” in Heavy Metal Contamination of Soils, 1st Edn, eds I. Sherameti and A. Varma (Switzerland: Springer).
Mosa, K. A., Saadoun, I., and Kumar, K. (2016). Potential biotechnological strategies for the cleanup of heavy metals and metalloids. Front. Plant Sci. 7:303. doi: 10.3389/fpls.2016.00303
Moslehi, A., Feizian, M., Higueras, P., and Eisvand, H. R. (2019). Assessment of EDDS and vermicompost for the phytoextraction of Cd and Pb by sunflower (Helianthus annuus L.). Int. J. Phytoremed. 21, 191–199. doi: 10.1080/15226514.2018.1501336
Muhammad, D., Chen, F., Zhao, J., Zhang, G., and Wu, F. (2009). Comparison of EDTA- and citric acid-enhanced phytoextraction of heavy metals in artificially metal contaminated soil by Typha angustifolia. Int. J. Phytoremediat. 11, 558–574. doi: 10.1080/15226510902717580
Murgese, P., Santamaria, P., Leoni, B., and Crecchio, C. (2020). Ameliorative effects of PGPB on yield, physiological parameters, and nutrient transporter genes expression in barattiere (Cucumis melo L.). J. Soil Sci. Plant Nutr. 20, 784–793. doi: 10.1007/s42729-019-00165-1
Myśliwa-Kurdziel, B., and Strzałka, K. (2002). “Influence of metals on biosynthesis of photosynthetic pigments,” in Physiology and Biochemistry of Metal Toxicity and Tolerance in Plants, eds M. N. Prasad, K. Strzalka, and K. Strzałka (Cham: Springer Science & Business Media), 201–227.
Naeem, M. A., Shabbir, A., Amjad, M., Abbas, G., Imran, M., Murtaza, B., et al. (2020). Acid treated biochar enhances cadmium tolerance by restricting its uptake and improving physio-chemical attributes in quinoa (Chenopodium quinoa Willd.). Ecotoxicol. Environ. Saf. 191:110218. doi: 10.1016/j.ecoenv.2020.110218
Najeeb, U., Jilani, G., Ali, S., Sarwar, M., Xu, L., and Zhou, W. (2011). Insights into cadmium induced physiological and ultra-structural disorders in Juncus effusus L. and its remediation through exogenous citric acid. J. Hazard. Mater. 186, 565–574. doi: 10.1016/j.jhazmat.2010.11.037
Nakamura, M., Ochiai, T., Noji, M., Ogura, Y., Suzuki, K., Yoshimoto, N., et al. (2014). An improved tolerance to cadmium by overexpression of two genes for cysteine synthesis in tobacco. Plant Biotechnol. 31, 141–147. doi: 10.5511/plantbiotechnology.14.0130a
Nejad, Z. D., Jung, M. C., and Kim, K. H. (2017). Remediation of soils contaminated with heavy metals with an emphasis on immobilization technology. Environ. Geochem. Health 40, 927–953. doi: 10.1007/s10653-017-9964-z
Nguyen, T. Q., Sesin, V., Kisiala, A., and Emery, R. J. N. (2020). Phytohormonal roles in plant responses to heavy metal stress-implications for using macrophytes in phytoremediation of aquatic ecosystems. Environ. Toxicol. Chem. 40, 7–22. doi: 10.1002/etc.4909
Nian, L., Zhang, X., Yi, X., Liu, X., Ain, U. L., Yang, Y., et al. (2021). Genome-wide identification of ABA receptor PYL/RCAR gene family and their response to cold stress in Medicago sativa L. Physiol. Mol. Biol. Plants 27, 1979–1995. doi: 10.1007/s12298-021-01066-3
Nigam, N., Khare, P., Yadav, V., Mishra, D., Jain, S., Karak, T., et al. (2019). Biochar-mediated sequestration of Pb and Cd leads to enhanced productivity in Mentha arvensis. Ecotoxicol. Environ. Saf. 172, 411–422. doi: 10.1016/j.ecoenv.2019.02.006
Nikolić, N., Zorić, L., Cvetković, I., Pajević, S., Borišev, M., Orlović, S., et al. (2017). Assessment of cadmium tolerance and phytoextraction ability in young Populus deltoides L. and Populus× euramericana plants through morpho-anatomical and physiological responses to growth in cadmium enriched soil. iForest Biogeosci. For. 10:635. doi: 10.3832/ifor2165-010
Noriega, G. O., Balestrasse, K. B., Batlle, A., and Tomaro, M. L. (2007). Cadmium induced oxidative stress in soybean plants also by the accumulation of δ-aminolevulinic acid. Biometals 20, 841–851. doi: 10.1007/s10534-006-9077-0
Nowack, B. (2002). Environmental chemistry of aminopolycarboxylate chelating agents. Environ. Sci. Technol. 36, 4009–4016. doi: 10.1021/es025683s
Okem, A., Kulkarni, M. G., and Staden, J. V. (2015). Enhancing phytoremediation potential of Pennisetum clandestinumHochst in cadmium- contaminated coil using smoke-water and smoke-isolated karrikinolide. Int. J. Phytoremed. 17, 1046–1052. doi: 10.1080/15226514.2014.981245
Ostrowski, M., Ciarkowska, A., and Jakubowska, A. (2016). The auxin conjugate indole-3- acetyl-aspartate affects responses to cadmium and salt stress in Pisum sativum L. J. Plant Physiol. 191, 63–72. doi: 10.1016/j.jplph.2015.11.012
Palansooriya, K. N., Shaheen, S. M., Chen, S. S., Tsang, D. C. W., Hashimoto, Y., Hou, D., et al. (2020). Soil amendments for immobilization of potentially toxic elements in contaminated soils: a critical review. Environ. Int. 134:105046. doi: 10.1016/j.envint.2019.105046
Pan, F., Luo, S., Shen, J., Wang, Q., Ye, J., Meng, Q., et al. (2017). The effects of endophytic bacterium SaMR12 on Sedum alfredii Hance metal ion uptake and the expression of three transporter family genes after cadmium exposure. Environ. Sci. Pollut. Res. 24, 9350–9360. doi: 10.1007/s11356-017-8565-9
Parmar, P., Kumari, N., and Sharma, V. (2013). Structural and functional alterations in photosynthetic apparatus of plants under cadmium stress. Bot. Stud. 54, 1–6. doi: 10.1186/1999-3110-54-45
Pereira, B. F. F., de Abreu, C. A., Herpin, U., de Abreu, M. F., and Berton, R. S. (2010). Phytoremediation of lead by jack beans on a Rhodic Hapludox amended with EDTA. Sci. Agric. 67, 308–318. doi: 10.1590/S0103-90162010000300009
Pierattini, E. C., Francini, A., Raffaelli, A., and Sebastiani, L. (2017). Surfactant and heavy metal interaction in poplar: a focus on SDS and Zn uptake. Tree Physiol. 38, 109–118. doi: 10.1093/treephys/tpx155
Piotrowska-Niczyporuk, A., Bajguz, A., Zambrzycka, E., and Godlewska- Zylkiewicz, B. (2012). Phytohormones as regulators of heavy metal biosorption and toxicity in green alga Chlorella vulgaris (Chlorophyceae). Plant Physiol. Biochem. 52, 52–65. doi: 10.1016/j.plaphy.2011.11.009
Pompeu, G. B., Vilhena, M. B., and Gratão, P. L. (2017). Abscisic acid-deficient sit tomato mutant responses to cadmium-induced stress. Protoplasma 254, 771–783. doi: 10.1007/s00709-016-0989-4
Pozniak, C., Fox, S., and Knott, D. (2009). CDC Verona durum wheat. Can. J. Plant Sci. 89, 321–324. doi: 10.4141/CJPS08117
Qayyum, M. F., Liaquat, F., Rehman, R. A., Gul, M., Zia ul Hye, M., Rizwan, M., et al. (2017). Effects of co-composting of farm manure and biochar on plant growth and carbon mineralization in an alkaline soil. Environ. Sci. Pollut. Res. 24, 26060–26068. doi: 10.1007/s11356-017-0227-4
Qayyum, M. F., Rehman, R. A., Liaqat, S., Ikram, M., Ali, S., Rizwan, M., et al. (2019). Cadmium immobilization in the soil and accumulation by spinach (Spinacia oleracea) depend on biochar types under controlled and field conditions. Arab. J. Geosci. 12, 1–11. doi: 10.1007/s12517-019-4681-9
Qi, F., Lamb, D., Naidu, R., Bolan, N. S., Yan, Y., Ok, Y. S., et al. (2018). Cadmium solubility and bioavailability in soils amended with acidic and neutral biochar. Sci. Total Environ. 610, 1457–1466. doi: 10.1016/j.scitotenv.2017.08.228
Qianqian, M., Haider, F. U., Farooq, M., Adeel, M., Shakoor, N., Jun, W., et al. (2022). Selenium treated Foliage and biochar treated soil for improved lettuce (Lactuca sativa L.) growth in Cd-polluted soil. J. Cleaner Prod. 335:130267. doi: 10.1016/j.jclepro.2021.130267
Qin, P., Wang, L., Liu, K., Mao, S., Li, Z., Gao, S., et al. (2015). Genome wide association study of Aegilops tauschiitraits under seedling-stage cadmium stress. Crop J. 3, 405–415. doi: 10.1016/j.cj.2015.04.005
Qiu, Z., Tan, H., Zhou, S., and Cao, L. (2014). Enhanced phytoremediation of toxic metals by inoculating endophytic Enterobacter sp. CBSB1 expressing bifunctional glutathione synthase. J. Hazard Mater. 267, 17–20. doi: 10.1016/j.jhazmat.2013.12.043
Quartacci, M. F., Pinzino, C., Sgherri, C. L., Dalla Vecchia, F., and Navari-Izzo, F. (2000). Growth in excess copper induces changes in the lipid composition and fluidity of PSII-enriched membranes in wheat. Physiol. Plant. 108, 87–93. doi: 10.1034/j.1399-3054.2000.108001087.x
Rady, M. M. (2011). Effect of 24-epibrassinolide on growth, yield, antioxidant system and cadmium content of bean (Phaseolus vulgaris L.) plants under salinity and cadmium stress. Sci. Hort. 129, 232–237. doi: 10.1016/j.scienta.2011.03.035
Rady, M. M., and Hemida, K. A. (2015). Modulation of cadmium toxicity and enhancing cadmium-tolerance in wheat seedlings by exogenous application of poly- amines. Ecotoxicol. Environ. Saf. 119, 178–185. doi: 10.1016/j.ecoenv.2015.05.008
Rafique, M., Haque, K., Hussain, T., Amna, C., and Javed, H. (2017). Biochemical Talk in the Rhizoshperic Microbial Community for Phytoremediation. Hauppauge, NY: Nova Science Publishers.
Rafique, M., Ortas, I., Rizwan, M., Sultan, T., Chaudhary, H. J., Işik, M., et al. (2019). Effects of Rhizophagusclarus and biochar on growth, photosynthesis, nutrients, and cadmium (Cd) concentration of maize (Zea mays) grown in Cd-spiked soil. Environ. Sci. Pollut. Res. 26, 20689–20700. doi: 10.1007/s11356-019-05323-7
Rajkumar, M., Sandhya, S., Prasad, M. N. V., and Freitas, H. (2012). Perspectives of plant-associated microbes in heavy metal phytoremediation. Biotechnol. Adv. 30, 1562–1574. doi: 10.1016/j.biotechadv.2012.04.011
Raklami, A., Oufdou, K., Tahiri, A. I., Mateos-Naranjo, E., Navarro-Torre, S., Rodríguez-Llorente, I. D., et al. (2019). Safe cultivation of Medicago sativa in metal-polluted soils from semi-arid regions assisted by heat-and metallo-resistant PGPR. Microorganisms 7:212. doi: 10.3390/microorganisms7070212
Rasheed, R., Ashraf, M. A., Hussain, I., Haider, M. Z., Kanwal, U., and Iqbal, M. (2014). Exogenous proline and glycinebetaine mitigate cadmium stress in two genetically different spring wheat (Triticum aestivum L.) cultivars. Braz. J. Bot. 37, 399–406. doi: 10.1007/s40415-014-0089-7
Rehman, A., Shahzad, B., Haider, F. U., Ahmed, H. A. I., Lee, D.-J., Im, S. Y., et al. (2022a). “An introduction to brassinosteroids: history, biosynthesis, and chemical diversity,” in Brassinosteroids in Plant Developmental Biology and Stress Tolerance, eds J. Q. Yu, G. J. Ahammed, and P. Krishna (Cambridge, MA: Academic Press).
Rehman, A., Shahzad, B., Haider, F. U., Moeen-ud-din, M., Ullah, A., and Khan, I. (2022b). “Brassinosteroids in plant response to high temperature stress,” in Brassinosteroids in Plant Developmental Biology and Stress Tolerance, eds J. Q. Yu, G. J. Ahammed, and P. Krishna (Cambridge, MA: Academic Press).
Remans, T., Opdenakker, K., Smeets, K., Mathijsen, D., Vangronsveld, J., and Cuypers, A. (2010). Metal-specific and NADPH oxidase dependent changes in lipoxygenase and NADPH oxidase gene expression in Arabidopsis thaliana exposed to cadmium or excess copper. Funct. Plant Biol. 37, 532–544. doi: 10.1071/FP09194
Ren, T., Chen, N., Mahari, W. A. W., Xu, C., Feng, H., Ji, X., et al. (2021). Biochar for cadmium pollution mitigation and stress resistance in tobacco growth. Environ. Res. 192:110273. doi: 10.1016/j.envres.2020.110273
Ren, Y., Chen, Y., An, J., Zhao, Z., Zhang, G., Wang, Y., et al. (2018). Wheat expansin gene TaEXPA2 is involved in conferring plant tolerance to Cd toxicity. Plant Sci. 270, 245–256. doi: 10.1016/j.plantsci.2018.02.022
Riaz, M., Yan, L., Wu, X., Hussain, S., Aziz, O., Wang, Y., et al. (2018). Boron alleviates the aluminum toxicity in trifoliate orange by regulating antioxidant defense system and reducing root cell injury. J. Environ. Manage. 208, 149–158. doi: 10.1016/j.jenvman.2017.12.008
Rizwan, M., Ali, S., Abbas, T., Zia-Ur-Rehman, M., Hannan, F., Keller, C., et al. (2016). Cadmium minimization in wheat: a critical review. Ecotoxicol. Environ. Saf. 130, 43–53. doi: 10.1016/j.ecoenv.2016.04.001
Rizwan, M., Ali, S., Qayyum, M. F., Ok, Y. S., Zia-ur-Rehman, M., Abbas, Z., et al. (2017). Use of maize (Zea mays L.) for phytomanagement of Cd-contaminated soils: a critical review. Environ. Geochem. Health 39, 259–277. doi: 10.1007/s10653-016-9826-0
Rizwan, M., Ali, S., Ur Rehman, M. Z., and Maqbool, A. (2019a). A critical review on the effects of zinc at toxic levels of cadmium in plants. Environ. Sci. Pollut. Res. 26, 6279–6289. doi: 10.1007/s11356-019-04174-6
Rizwan, M., Ali, S., Ur Rehman, M. Z., Rinklebe, J., Tsang, D. C., Bashir, A., et al. (2018). Cadmium phytoremediation potential of Brassica crop species: a review. Sci. Total Environ. 631, 1175–1191. doi: 10.1016/j.scitotenv.2018.03.104
Rizwan, M., Noureen, S., Ali, S., Anwar, S., Zia-ur-Rehman, M., Qayyum, M. F., et al. (2019b). Influence of biochar amendment and foliar application of iron oxide nanoparticles on growth, photosynthesis, and cadmium accumulation in rice biomass. J. Soils Sediments 19, 3749–3759. doi: 10.1007/s11368-019-02327-1
Rodda, M. S., Li, G., and Reid, R. J. (2011). The timing of grain Cd accumulation in rice plants: the relative importance of remobilisation within the plant and root Cd uptake post-flowering. Plant Soil 347, 105–114. doi: 10.1007/s11104-011-0829-4
Rodríguez-Serrano, M., Romero-Puertas, M. C., Zabalza, A., Corpas, F. J., Gómez, M., Del Río, L. A., et al. (2006). Cadmium effect on oxidative metabolism of pea (Pisum sativum L.) roots. Imaging of reactive oxygen species and nitric oxide accumulation in vivo. Plant Cell Environ. 29, 1532–1544. doi: 10.1111/j.1365-3040.2006.01531.x
Romero-Puertas, M., Rodríguez-Serrano, M., Corpas, F., Gomez, M. D., Del Rio, L., and Sandalio, L. (2004). Cadmium-induced subcellular accumulation of O2•− and H2O2 in pea leaves. Plant Cell Environ. 27, 1122–1134. doi: 10.1111/j.1365-3040.2004.01217.x
Rostami, S., and Azhdarpoor, A. (2019). The application of plant growth regulators to improve phytoremediation of contaminated soils: a review. Chemosphere 220, 818–827. doi: 10.1016/j.chemosphere.2018.12.203
Roychoudhury, A., Ghosh, S., Paul, S., Mazumdar, S., Das, G., and Das, S. (2016). Pre-treatment of seeds with salicylic acid attenuates cadmium chloride-induced oxidative damages in the seedlings of mungbean (Vigna radiata L. wilczek). Acta Physiol. Plant. 38, 1–18. doi: 10.1007/s11738-015-2027-0
Saeed, Q., Xiukang, W., Haider, F. U., Kucerik, J., Mumtaz, M. Z., Holatko, J., et al. (2021). Rhizosphere bacteria in plant growth promotion, biocontrol, and bioremediation of contaminated sites: a comprehensive review of effects and mechanisms. Int. J. Mol. Sci. 21:10529. doi: 10.3390/ijms221910529
Saeid, A., Prochownik, E., and Dobrowolska-Iwanek, J. (2018). Phosphorus solubilization by Bacillus species. Molecules 23:2897. doi: 10.3390/molecules23112897
Saeki, K., and Kunito, T. (2012). Influence of chloride ions on cadmium adsorptions by oxides, hydroxides, oxyhydroxides, and phyllosilicates. Appl. Clay Sci. 62–63, 58–62. doi: 10.1016/j.clay.2012.04.018
Saifullah, M. E., Qadir, M., de Caritat, P., Tack, F. M. G., Du Laing, G., and Zia, M. (2009). H. EDTA-assisted Pb phytoextraction. Chemosphere 74, 1279–1291. doi: 10.1016/j.chemosphere.2008.11.007
Sakouhi, L., Rahoui, S., Massoud, M. B., Munemasa, S., Ferjani, E. E., Murata, Y., et al. (2016). Calcium and EGTA alleviate cadmium toxicity in germinating chickpea seeds. J. Plant Growth Regul. 35, 1064–1073. doi: 10.1007/s00344-016-9605-2
Salazar, M. J., Rodriguez, J. H., Nieto, G. L., and Pignata, M. L. (2012). Effects of heavy metal concentrations (Cd, Zn and Pb) in agricultural soils near different emission sources on quality, accumulation and food safety in soybean [Glycine max (L.) Merrill]. J. Hazard. Mater. 233, 244–253. doi: 10.1016/j.jhazmat.2012.07.026
Sangthong, C., Setkit, K., and Prapagdee, B. (2016). Improvement of cadmium phytoremediation after soil inoculation with a cadmium-resistant Micrococcus sp. Environ. Sci. Pollut. Res. 23, 756–764. doi: 10.1007/s11356-015-5318-5
Sárvári, É (2008). Effect of Cd on the iron re-supply-induced formation of chlorophyll-protein complexes in cucumber. Acta Biol. Szegediensis 52, 183–186.
Sarwar, N., Malhi, S. S., Zia, M. H., Naeem, A., Bibi, S., and Farid, G. (2010). Role of mineral nutrition in minimizing cadmium accumulation by plants. J. Sci. Food Agric. 90, 925–937. doi: 10.1002/jsfa.3916
Sasaki, A., Yamaji, N., Yokosho, K., and Ma, J. F. (2012). Nramp5 is a major transporter responsible for manganese and cadmium uptake in rice. Plant Cell 24, 2155–2167.
Sato, H., Shirasawa, S., Maeda, H., Nakagomi, K., Kaji, R., Ohta, H., et al. (2011). Analysis of QTL for lowering cadmium concentration in rice grains from ‘LAC23’. Breed. Sci. 61, 196–200. doi: 10.1270/jsbbs.61.196
Sell, J., Kayser, A., Schulin, R., and Brunner, I. (2005). Contribution of ectomycorrhizal fungi to cadmium uptake of poplars and willows from a heavily polluted soil. Plant Soil 277, 245–253. doi: 10.1007/s11104-005-7084-5
Semida, W. M., Rady, M. M., Abd El-Mageed, T. A., Howladar, S. M., and Abdelhamid, M. A. (2015). Alleviation of cadmium toxicity in common bean (Phaseolus vulgaris L.) plants by the exogenous application of salicylic acid. J. Hortic. Sci. Biotechnol. 90, 83–91. doi: 10.1080/14620316.2015.11513156
Shah, K., Nahakpam, S., Chaturvedi, V., and Singh, P. (2019). “Cadmium-induced anatomical abnormalities in plants,” in Cadmium Toxicity and Tolerance in Plants, eds M. Hasanuzzaman, M. N. V. Prasad, and M. Fujita (Amsterdam: Elsevier), 111–139. doi: 10.1016/b978-0-12-814864-8.00005-x
Shah, K., Singh, P., and Nahakpam, S. (2013). Effect of cadmium uptake and heat stress on root ultrastructure, membrane damage and antioxidative response in rice seedlings. J. Plant Biochem. Biotechnol. 22, 103–112. doi: 10.1007/s13562-012-0116-3
Shahid, M., Austruy, A., Echevarria, G., Arshad, M., Sanaullah, M., Aslam, M., et al. (2014). EDTA-enhanced phytoremediation of heavy metals: a review. Soil Sediment. Contam. 23, 389–416. doi: 10.1080/15320383.2014.831029
Shahid, M., Dumat, C., Khalid, S., Niazi, N. K., and Antunes, P. M. (2016). Cadmium bioavailability, uptake, toxicity and detoxification in soil plant system. Rev. Environ. Contam. Toxicol. 241, 73–137. doi: 10.1007/398_2016_8
Shahkolaie, S. S., Baranimotlagh, M., Dordipour, E., and Khormali, F. (2020). Effects of inorganic and organic amendments on physiological parameters and antioxidant enzymes activities in Zea mays L. from a cadmium-contaminated calcareous soil. S. Afr. J. Bot. 128, 132–140. doi: 10.1016/j.sajb.2019.10.007
Shahzad, B., Tanveer, M., Che, Z., Rehman, A., Cheema, S. A., Sharma, A., et al. (2018). Role of 24-epibrassinolide (EBL) in mediating heavy metal and pesticide induced oxidative stress in plants: a review. Ecotoxicol. Environ. Saf. 147, 935–944. doi: 10.1016/j.ecoenv.2017.09.066
Shakirova, F. M., Allagulova, C. R., Maslennikova, D. R., Klyuchnikova, E. O., Avalbaev, A. M., and Bezrukova, M. V. (2016). Salicylic acid-induced protection against cadmium toxicity in wheat plants. Environ. Exp. Bot. 122, 19–28. doi: 10.1016/j.envexpbot.2015.08.002
Sharaf, A. E. M. M., Farghal, I. I., and Sofy, M. R. (2009). Role of gibberellic acid in abolishing the detrimental effects of Cd and Pb on broad bean and lupin plants. Res. J. Agric. Biol. Sci. 5, 668–673.
Sharma, R. K., and Archana, G. (2016). Cadmium minimization in food crops by cadmium resistant plant growth promoting rhizobacteria. Appl. Soil Ecol. 107, 66–78. doi: 10.1016/j.apsoil.2016.05.009
Sharma, S., Anand, G., Singh, N., and Kapoor, R. (2017). Arbuscular mycorrhiza augments arsenic tolerance in wheat (Triticum aestivum L.) by strengthening antioxidant defense system and thiol metabolism. Front. Plant Sci. 8:906. doi: 10.3389/fpls.2017.00906
Sharma, S. S., and Kumar, V. (2002). Responses of wild type and abscisic acid mutants of Arabidopsis thaliana to cadmium. J. Plant Physiol. 159, 1323–1327. doi: 10.1078/0176-1617-00601
Shao, J. F., Xia, J., Yamaji, N., Shen, R. F., and Ma, J. F. (2018). Effective reduction of cadmium accumulation in rice grain by expressing OsHMA3 under the control of the OsHMA2 promoter. J. Exp. Bot. 69, 2743–2752.
Sheoran, V., Sheoran, A. S., and Poonia, P. (2016). Factors affecting phytoextraction: (a review). Pedosphere 26, 148–166. doi: 10.1016/S1002-0160(15)60032-7
Shi, P., Zhu, K., Zhang, Y., and Chai, T. (2016). Growth and cadmium accumulation of Solanum nigrum L. seedling were enhanced by heavy metal-tolerant strains of Pseudomonas aeruginosa. Water Air Soil Pollut. 227:459.
Shiyu, Q. I. N., Hongen, L. I. U., Zhaojun, N. I. E., Rengel, Z., Wei, G. A. O., Chang, L. I., et al. (2020). Toxicity of cadmium and its competition with mineral nutrients for uptake by plants: a review. Pedosphere 30, 168–180. doi: 10.1016/S1002-0160(20)60002-9
Shukla, D., Kesari, R., Tiwari, M., Dwivedi, S., Tripathi, R. D., Nath, P., et al. (2013). Expression of Ceratophyllumdemersumphytochelatin synthase, CdPCS1, in Escherichia coli and Arabidopsis enhances heavy metal(loid)s accumulation. Protoplasma 250, 1263–1272. doi: 10.1007/s00709-013-0508-9
Siemianowski, O., Barabasz, A., Kendziorek, M., Ruszczyńska, A., Bulska, E., Williams, L. E., et al. (2014). AtHMA4 expression in tobacco reduces Cd accumulation due to the induction of the apoplastic barrier. J. Exp. Bot. 65, 1125–1139. doi: 10.1093/jxb/ert471
Sigfridsson, K. G., Bernát, G., Mamedov, F., and Styring, S. (2004). Molecular interference of Cd2+ with Photosystem II. Biochim. Biophys. Acta Bioenerget. 1659, 19–31. doi: 10.1016/j.bbabio.2004.07.003
Silber, A., Bar-Yosef, B., Suryano, S., and Levkovitch, I. (2012). Zinc adsorption by perlite: effects of pH, ionic strength, temperature, and pre-use as growth substrate. Geoderma 170, 159–167.
Silva, S. A., Techio, V. H., de Castro, E. M., de Faria, M. R., and Palmieri, M. J. (2013). Reproductive, cellular, and anatomical alterations in Pistia stratiotes L. plants exposed to cadmium. Water Air Soil Pollut. 224, 1–8.
Singh, G., Pankaj, U., Chand, S., and Verma, R. (2019). Arbuscular mycorrhizal fungi assisted phytoextraction of toxic metals by Zea mays L. from tannery sludge. Soil Sediment. Contam. 28, 729–746. doi: 10.1080/15320383.2019.1657381
Singh, P., Singh, I., and Shah, K. (2020). Alterations in antioxidative machinery and growth parameters upon application of nitric oxide donor that reduces detrimental effects of cadmium in rice seedlings with increasing days of growth. S. Afr. J. Bot. 131, 283–294. doi: 10.1016/j.sajb.2020.02.022
Singh, S., Parihar, P., Singh, R., Singh, V. P., and Prasad, S. M. (2016). Heavy metal tolerance in plants: role of transcriptomics, proteomics, metabolomics, and ionomics. Front. Plant Sci. 6:1143. doi: 10.3389/fpls.2015.01143
Singh, S., and Prasad, S. M. (2016). Kinetin ameliorates cadmium induced toxicity on growth, pigments and photosynthesis by regulating antioxidant potential in tomato seedlings. Int. J. Sci. Eng. Appl. Sci. 2, 1–10.
Singh, S., and Prasad, S. M. (2017). Effects of 28-homobrassinoloid on key physiological attributes of Solanum lycopersicum seedlings under cadmium stress: photosynthesis and nitrogen metabolism. Plant Growth Reg. 82, 161–173. doi: 10.1007/s10725-017-0248-5
Singh, S., Singh, A., Srivastava, P. K., and Prasad, S. M. (2018). Cadmium toxicity and its amelioration by kinetin in tomato seedlings vis-à-vis ascorbate-glutathione cycle. J. Photochem. Photobiol. B Biol. 178, 76–84. doi: 10.1016/j.jphotobiol.2017.10.025
Skrebsky, E. C., Tabaldi, L. A., Pereira, L. B., Rauber, R., Maldaner, J., Cargnelutti, D., et al. (2008). Effect of cadmium on growth, micronutrient concentration, and δ-aminolevulinic acid dehydratase and acid phosphatase activities in plants of Pfaffia glomerata. Braz. J. Plant Physiol. 20, 285–294. doi: 10.1590/S1677-04202008000400004
Sohail, M. I., Rehman, M. Z., Rizwan, M., Yousaf, B., Ali, S., Haq, M. A., et al. (2020). Efficiency of various silicon rich amendments on growth and cadmium accumulation in field grown cereals and health risk assessment. Chemosphere 244, 1–12. doi: 10.1016/j.chemosphere.2019.125481
Sohail, M. I., Zia-ur-Rehman, M., Murtaza, G., and Wahid, M. A. (2019). Chemical investigations of Si-rich organic and inorganic amendments and correlation analysis between different chemical composition and Si contents in amendments. Arab. J. Geosci. 12:47. doi: 10.1007/s12517-018-4215-x
Song, W., Chen, S., Liu, J., Chen, L., Song, N., Li, N., et al. (2015). Variation of Cd concentration in various rice cultivars and derivation of cadmium toxicity thresholds for paddy soil by species-sensitivity distribution. J. Integr. Agric. 14, 1845–1854. doi: 10.1016/S2095-3119(14)60926-6
Song, Y., Jin, L., and Wang, X. (2017). Cadmium absorption and transportation pathways in plants. Int. J Phytoremed. 19, 133–141. doi: 10.1080/15226514.2016.1207598
Stroiński, A., Giżewska, K., and Zielezińska, M. (2013). Abscisic acid is required in transduction of cadmium signal to potato roots. Biol. Plant. 57, 121–127. doi: 10.1007/s10535-012-0135-x
Sugiyama, M., Ae, N., and Hajika, M. (2011). Developing of a simple method for screening soybean seedling cadmium accumulation to select soybean genotypes with low seed cadmium. Plant Soil 341, 413–422.
Suksabye, P., Pimthong, A., Dhurakit, P., Mekvichitsaeng, P., and Thiravetyan, P. (2016). Effect of biochars and microorganisms on cadmium accumulation in rice grains grown in Cd-contaminated soil. Environ. Sci. Pollut. Res. Int. 23, 962–973. doi: 10.1007/s11356-015-4590-8
Sullivan, T. S., McBride, M. B., and Thies, J. E. (2013). Soil bacterial and archaeal community composition reflects high spatial heterogeneity of pH, bioavailable Zn, and Cu in a metalliferous peat soil. Soil Biol. Biochem. 66, 102–109. doi: 10.1016/j.soilbio.2013.06.021
Sun, S., Wang, H., Yu, H., Zhong, C., Zhang, X., Peng, J., et al. (2013). GASA14 regulates leaf expansion and abiotic stress resistance by modulating reactive oxygen species accumulation. J. Exp. Bot. 64, 1637–1647. doi: 10.1093/jxb/ert021
Sun, S., Zhou, X., Cui, X., Liu, C., Fan, Y., McBride, M. B., et al. (2020). Exogenous plant growth regulators improved phytoextraction efficiency by Amaranths hypochondriacus L. in cadmium contaminated soil. Plant Growth Reg. 90, 29–40. doi: 10.1007/s10725-019-00548-5
Sunitha, M. S., Prashant, S., Kumar, S. A., Rao, S. R. I. N. A. T. H., Narasu, M. L., and Kishor, P. K. (2012). Cellular and molecular mechanisms of heavy metal tolerance in plants: a brief overview of transgenic plants over-expressing phytochelatin synthase and metallothionein genes. Plant Cell Biotechnol. Mol. Biol. 13, 99–104.
Tamás, L., Dudíková, J., Ďurčeková, K., Halušková, L’, Huttová, J., and Mistrík, I. (2009). Effect of cadmium and temperature on the lipoxygenase activity in barley root tip. Protoplasma 235:17. doi: 10.1007/s00709-008-0027-2
Tang, X., Zeng, G., Fan, C., Zhou, M., Tang, L., Zhu, J., et al. (2018). Chromosomal expression of CadR on Pseudomonas aeruginosa for the removal of Cd (II) from aqueous solutions. Sci. Total Environ. 636, 1355–1361. doi: 10.1016/j.scitotenv.2018.04.229
Tanveer, M., and Shabala, S. (2022). “Entangling the interaction between essential and nonessential nutrients: implications for global food security,” in Plant Nutrition and Food Security in the Era of Climate Change, ed. V. Kumar (Cambridge, MA: Academic Press), 1–25.
Tanwar, A., Aggarwal, A., Charaya, M. U., and Kumar, P. (2015). Cadmium remediation by arbuscular mycorrhizal fungus–colonized celery plants supplemented with ethylenediaminetetraacetic acid. Bioremediat. J. 19, 188–200. doi: 10.1080/10889868.2014.995371
Tao, S., Sun, L., Ma, C., Li, L., Li, G., and Hao, L. (2013). Reducing basal salicylic acid enhances Arabidopsis tolerance to lead or cadmium. Plant Soil 372, 309–318. doi: 10.1007/s11104-013-1749-2
Teiri, H., Pourzamani, H., and Hajizadeh, Y. (2018). Phytoremediation of VOCs from indoor air by ornamental potted plants: a pilot study using a palm species under the controlled environment. Chemosphere 197, 375–381.
Timperio, A. M., D’Amici, G. M., Barta, C., Loreto, F., and Zolla, L. (2007). Proteomics, pigment composition, and organization of thylakoid membranes in iron-deficient spinach leaves. J. Exp. Bot. 58, 3695–3710. doi: 10.1093/jxb/erm219
Tiong, J., McDonald, G. K., Genc, Y., Pedas, P., Hayes, J. E., Toubia, J., et al. (2014). HvZIP7 mediates zinc accumulation in barley (Hordeum vulgare) at moderately high zinc supply. New Phytol. 201, 131–143. doi: 10.1111/nph.12468
Tran, T. A., Paunova, S., Nedeva, D., and Popova, L. (2011). Nitric oxide alleviates cadmium toxicity on photosynthesis in pea plants. ComptesRendus de l’AcademieBulgare des Sci. 64, 1137–1142.
Tran, T. A., and Popova, L. P. (2013). Functions and toxicity of cadmium in plants: recent advances and future prospects. Turk. J. Bot. 37, 1–13. doi: 10.3906/bot-1112-16
Ueno, D., Kono, I., Yokosho, K., Ando, T., Yano, M., and Ma, J. F. (2009a). A major quantitative trait locus controlling cadmium translocation in rice (Oryza sativa). N. Phytol. 182, 644–653. doi: 10.1111/j.1469-8137.2009.02784.x
Ueno, D., Koyama, E., Kono, I., Ando, T., Yano, M., and Ma, J. F. (2009b). Identification of a novel major quantitative trait locus controlling distribution of Cd between roots and shoots in rice. Plant Cell Physiol. 50, 2223–2233. doi: 10.1093/pcp/pcp160
Ullah, A., Heng, S., Munis, M. F. H., Fahad, S., and Yang, X. (2015). Phytoremediation of heavy metals assisted by plant growth promoting (PGP) bacteria: a review. Environ. Exp. Bot. 117, 28–40. doi: 10.1016/j.envexpbot.2015.05.001
Unsal, V., Dalkıran, T., Çiçek, M., and Kölükçü, E. (2020). The role of natural antioxidants against reactive oxygen species produced by cadmium toxicity: a review. Adv. Pharm. Bull. 10:184. doi: 10.34172/apb.2020.023
Upadhyay, S. K., Singh, J. S., and Singh, D. P. (2011). Exopolysaccharide producing plant growth promoting rhizobacteria under salinity condition. Pedosphere 21, 214–222. doi: 10.1016/s1002-0160(11)60120-3
Uraguchi, S., Mori, S., Kuramata, M., Kawasaki, A., Arao, T., and Ishikawa, S. (2009). Root-to-shoot Cd translocation via the xylem is the major process determining shoot and grain cadmium accumulation in rice. J. Exp. Bot. 60:2677. doi: 10.1093/jxb/erp119
Varalakshmi, L. R., and Ganeshamurthy, A. (2013). Phytotoxicity of cadmium in radish and its effects on growth, yield, and cadmium uptake. Commun. Soil Sci. Plant Anal. 44, 1444–1456. doi: 10.1080/00103624.2013.767344
Verma, S., Verma, P. K., Meher, A. K., Bansiwal, A. K., Tripathi, R. D., and Chakrabarty, D. (2017). A novel fungal arsenic methyltransferase, WaarsM reduces grain arsenic accumulation in the transgenic rice plant. J. Hazard. Mater. 344, 626–634. doi: 10.1016/j.jhazmat.2017.10.037
Vestena, S., Cambraia, J., Ribeiro, C., Oliveira, J. A., and Oliva, M. A. (2011). Cadmium-induced oxidative stress and antioxidative enzyme response in water hyacinth and salvinia. Braz. J. Plant Physiol. 23, 131–139. doi: 10.1590/S1677-04202011000200005
Violante, A., Cozzolino, V., Perelomov, L., Caporale, A., and Pigna, M. (2010). Mobility and bioavailability of heavy metals and metalloids in soil environments. J. Soil Sci. Plant Nutr. 10, 268–292. doi: 10.4067/S0718-95162010000100005
Vollmann, J., Losak, T., Pachner, M., Watanabe, D., Musilova, L., and Hlusek, J. (2015). Soybean cadmium concentration: validation of a QTL affecting seed cadmium accumulation for improved food safety. Euphytica 203, 177–184. doi: 10.1007/s10681-014-1297-8
Wahid, A., and Ghani, A. (2008). Varietal differences in mungbean (Vigna radiata) for growth, yield, toxicity symptoms and cadmium accumulation. Ann. Appl. Biol. 152, 59–69. doi: 10.1111/j.1744-7348.2007.00192.x
Wang, F., Chen, F., Cai, Y., Zhang, G., and Wu, F. (2011). Modulation of exogenous glutathione in ultrastructure and photosynthetic performance against Cd stress in the two barley genotypes differing in Cd tolerance. Biol. Trace Elem. Res. 144, 1275–1288.
Wang, H., Gao, B., Wang, S., Fang, J., Xue, Y., and Yang, K. (2015). Removal of Pb(II), Cu(II), and Cd(II) from aqueous solutions by biochar derived from KMnO4 treated hickory wood. Bioresour. Technol. 197, 356–362.
Wang, K., Liu, Y., Song, Z., Wang, D., and Qiu, W. (2019). Chelator complexes enhanced Amaranthus hypochondriacus L. phytoremediation efficiency in Cd-contaminated soils. Chemosphere 237:124480. doi: 10.1016/j.chemosphere.2019.124480
Wang, S., and Liu, J. (2014). The effectiveness and risk comparison of EDTA with EGTA in enhancing Cd phytoextraction by Mirabilis jalapa L. Environ. Monit. Assess. 186, 751–759. doi: 10.1007/s10661-013-3414-x
Wen, E., Yang, X., Chen, H., Shaheen, S. M., Sarkar, B., Xu, S., et al. (2020). Iron-modified biochar and water management regime-induced changes in plant growth, enzyme activities, and phytoavailability of arsenic, cadmium and lead in a paddy soil. J. Hazard. Mater. 407:124344. doi: 10.1016/j.jhazmat.2020.124344
World Health Organization [WHO] (2007). Health Risks of Heavy Metals from Long-Range Transboundary Air Pollution. Copenhagen: World Health Organization.
Wu, F., Zhang, G., Dominy, P., Wu, H., and Bachir, D. M. (2007). Differences in yield components and kernel Cd accumulation in response to Cd toxicity in four barley genotypes. Chemosphere 70, 83–92.
Wiebe, K., Harris, N., Faris, J., Clarke, J., Knox, R. E., Taylor, G. J., et al. (2010). Targeted mapping of Cdu1-B, a major locus regulating grain cadmium concentration in durum wheat (Triticum turgidum L. var durum). Theor. Appl. Genet. 121, 1047–1058. doi: 10.1007/s00122-010-1370-1
Wodala, B., Eitel, G., Gyula, T., Ördög, A., and Horváth, F. (2012). Monitoring moderate Cu and Cd toxicity by chlorophyll fluorescence and P 700 absorbance in pea leaves. Photosynthetica 50, 380–386. doi: 10.1007/s11099-012-0045-3
Wu, B., He, T., Wang, Z., Qiao, S., Wang, Y., Xu, F., et al. (2020). Insight into the mechanisms of plant growth promoting strain SNB6 on enhancing the phytoextraction in cadmium contaminated soil. J. Hazard Mater. 385:121587. doi: 10.1016/j.jhazmat.2019.121587
Wu, D., Sato, K., and Ma, J. F. (2015). Genome-wide association mapping of cadmium accumulation in different organs of barley. New Phytol. 208, 817–829. doi: 10.1111/nph.13512
Wu, Z., Wu, W., Zhou, S., and Wu, S. (2016). Mycorrhizal inoculation affects Pb and Cd accumulation and translocation in pakchoi (Brassica chinensis L.). Pedosphere 26, 13–26. doi: 10.1016/S1002-0160(15)60018-2
Xiao, R., Wang, P., Mi, S., Ali, A., Liu, X., Li, Y., et al. (2019). Effects of crop straw and its derived biochar on the mobility and bioavailability in Cd and Zn in two smelter-contaminated alkaline soils. Ecotoxicol. Environ. Saf. 181, 155–163. doi: 10.1016/j.ecoenv.2019.06.005
Xie, Y., Su, L., He, Z., Zhang, J., and Tang, Y. (2021). Selenium inhibits cadmium absorption and improves yield and quality of cherry tomato (Lycopersicon esculentum) under cadmium stress. J. Soil Sci. Plant Nutr. 27, 12476–12490. doi: 10.1007/s42729-021-00427-x
Xu, D., Chen, Z., Sun, K., Yan, D., Kang, M., and Zhao, Y. (2013). Effect of cadmium on the physiological parameters and the subcellular cadmium localization in the potato (Solanum tuberosum L.). Ecotoxicol. Environ. Saf. 97, 147–153. doi: 10.1016/j.ecoenv.2013.07.021
Xu, S. S., Lin, S. Z., and Lai, Z. X. (2015). Cadmium impairs iron homeostasis in Arabidopsis thaliana by increasing the polysaccharide contents and the iron-binding capacity of root cell walls. Plant Soil 392, 71–85. doi: 10.1007/s11104-015-2443-3
Xu, W., Li, Y., He, J., Ma, Q., Zhang, X., Chen, G., et al. (2010). Cd uptake in rice cultivars treated with organic acids and EDTA. J. Environ. Sci. 22, 441–447.
Xue, D., Chen, M., and Zhang, G. (2009). Mapping of QTLs associated with cadmium tolerance and accumulation during seedling stage in rice (Oryza sativa L.). Euphytica 165, 587–596. doi: 10.1007/s10681-008-9785-3
Xue, Z. C., Gao, H. Y., and Zhang, L. T. (2013). Effects of cadmium on growth, photosynthetic rate and chlorophyll content in leaves of soybean seedlings. Biol. Plant. 57, 587–590. doi: 10.1007/s10535-013-0318-0
Yang, P., Zhou, X. F., Wang, L. L., Li, Q. S., Zhou, T., Chen, Y. K., et al. (2018). Effect of phosphate-solubilizing bacteria on the mobility of insoluble cadmium and metabolic analysis. Int. J. Environ. Res. Public Health 15:1330. doi: 10.3390/ijerph15071330
Yang, Y., Ge, Y., Zeng, H., Zhou, X., Peng, L., and Zeng, Q. (2017). Phytoextraction of cadmium contaminated soil and potential of regenerated tobacco biomass for recovery of cadmium. Sci. Rep. 7:7210. doi: 10.1038/s41598-017-05834-8
Yang, Y., Xiong, J., Tao, L., Cao, Z., Tang, W., Zhang, J., et al. (2020). Regulatory mechanisms of nitrogen (N) on cadmium (Cd) uptake and accumulation in plants: a review. Sci. Total Environ. 708:135186. doi: 10.1016/j.scitotenv.2019.135186
Yan, L., Riaz, M., Wu, X., Du, C., Liu, Y., Lv, B., et al. (2018). Boron inhibits aluminuminduced toxicity to citrus by stimulating antioxidant enzyme activity. J. Environ. Sci. Health C Environ. Carcinog. Ecotoxicol. Rev. 36, 145–163. doi: 10.1080/10590501.2018.1490513
Yao, P., Zhou, H., Li, X., Wei, L., Wang, J., Zhang, S., et al. (2021). Effect of biochar on the accumulation and distribution of cadmium in tobacco (Yunyan 87) at different developmental stages. Ecotoxicol. Environ. Saf. 207:111295. doi: 10.1016/j.ecoenv.2020.111295
Ying, R. R., Qiu, R. L., Tang, Y. T., Hu, P. J., Qiu, H., Chen, H. R., et al. (2010). Cadmium tolerance of carbon assimilation enzymes and chloroplast in Zn/Cd hyperaccumulator Picris divaricata. J. Plant Physiol. 167, 81–87. doi: 10.1016/j.jplph.2009.07.005
Younis, U., Malik, S. A., Rizwan, M., Qayyum, M. F., Ok, Y. S., Shah, M. H. R., et al. (2016). Biochar enhances the cadmium tolerance in spinach (Spinacia oleracea) through modification of Cd uptake and physiological and biochemical attributes. Environ. Sci. Pollut. Res. 23, 21385–21394. doi: 10.1007/s11356-016-7344-3
Yousaf, B., Liu, G., Wang, R., Zia-ur-Rehman, M., Rizwan, M. S., Imtiaz, M., et al. (2016). Investigating the potential influence of biochar and traditional organic amendments on the bioavailability and transfer of Cd in the soil–plant system. Environ Earth Sci. 75:374. doi: 10.1007/s12665-016-5285-2
Yousaf, M. T. B., Nawaz, M. F., Khawaja, H. F., Gul, S., Ali, S., Ahmad, I., et al. (2019). Ecophysiological response of early stage Albizia lebbeck to cadmium toxicity and biochar addition. Arab. J. Geosci. 12, 1–8. doi: 10.1007/s12517-019-4296-1
Yu, H. Y., Liu, C., Zhu, J., Li, F., Deng, D. M., Wang, Q., et al. (2016). Cadmium availability in rice paddy fields from a mining area: the effects of soil properties highlighting iron fractions and pH value. Environ Pollut. 209, 38–45. doi: 10.1016/j.envpol.2015.11.021
Yu, L., Gao, R., Qinghua, S., Wang, X., Wei, M., and Yang, F. (2013). Exogenous application of sodium nitroprusside alleviated cadmium induced chlorosis, photosynthesis inhibition and oxidative stress in cucumber. Pak. J. Bot. 45, 813–819.
Yu, R., Li, D., Du, X., Xia, S., Liu, C., and Shi, G. (2017). Comparative transcriptome analysis reveals key cadmium transport-related genes in roots of two pakchoi (Brassica rapa L. ssp. chinensis) cultivars. BMC Genom. 18:587. doi: 10.1186/s12864-017-3973-2
Yuan, L., Yang, S., Liu, B., Zhang, M., and Wu, K. (2012). Molecular characterization of a rice metal tolerance protein, OsMTP1. Plant Cell Rep. 31, 67–79. doi: 10.1007/s00299-011-1140-9
Yuan, P., Wang, J., Pan, Y., Shen, B., and Wu, C. (2019). Review of biochar for the management of contaminated soil: preparation, application and prospect. Sci. Total Environ. 659, 473–490. doi: 10.1016/j.scitotenv.2018.12.400
Zaheer, I. E., Ali, S., Muhammad, R., Farid, M., Shakoor, M. B., Gill, R. A., et al. (2015). Citric acid assisted phytoremediation of copper by Brassica napus L. Ecotoxicol. Environ. Saf. 120, 310–317. doi: 10.1016/j.ecoenv.2014.03.007
Zainab, N., Din, B. U., Javed, M. T., Afridi, M. S., Mukhtar, T., Kamran, M. A., et al. (2020). Deciphering metal toxicity responses of flax (Linumusitatissimum L.) with exopolysaccharide and ACC-deaminase producing bacteria in industrially contaminated soils. Plant Physiol. Biochem. 152, 90–99. doi: 10.1016/j.plaphy.2020.04.039
Zawoznik, M. S., Groppa, M. D., Tomaro, M. L., and Benavides, M. P. (2007). Endogenous salicylic acid potentiates cadmium-induced oxidative stress in Arabidopsis thaliana. Plant Sci. 173, 190–197. doi: 10.1016/j.plantsci.2007.05.004
Zeeshan, N., Nasir, A. A., Haider, F. U., Naveed, K., Naseer, S., and Murtaza, G. (2021). Risk assessment of trace metals deposition and growth of Abelmochus esculentus L. on industrially polluted soils ofFaisalabad, Pakistan. Pak. J. Agri. Sci. 58, 881–889. doi: 10.21162/PAKJAS/21.409
Zhan, F., Li, B., Jiang, M., Yue, X., He, Y., Xia, Y., et al. (2018). Arbuscular mycorrhizal fungi enhance antioxidant defense in the leaves and the retention of heavy metals in the roots of maize. Environ. Sci. Pollut. Res. 25, 24338–24347. doi: 10.1007/s11356-018-2487-z
Zhang, C., Yu, Z. G., Zeng, G. M., Jiang, M., Yang, Z. Z., Cui, F., et al. (2014). Effects of sediment geochemical properties on heavy metal bioavailability. Environ. Int. 73, 270–281. doi: 10.1016/j.envint.2014.08.010
Zhang, C. J., Chen, L., Shi, D. W., Chen, G. X., Lu, C. G., Wang, P., et al. (2007). Characteristics of ribulose-1, 5-bisphosphate carboxylase and C4 pathway key enzymes in flag leaves of a super-high-yield hybrid rice and its parents during the reproductive stage. S. Afric. J. Bot. 73, 22–28. doi: 10.1016/j.sajb.2006.05.002
Zhang, F., Liu, M., Li, Y., Che, Y., and Xiao, Y. (2019). Effects of arbuscular mycorrhizal fungi, biochar and cadmium on the yield and element uptake of Medicago sativa. Sci. Total Environ. 655, 1150–1158. doi: 10.1016/j.scitotenv.2018.11.317
Zhang, G., Fukami, M., and Sekimoto, H. (2002). Influence of cadmium on mineral concentrations and yield components in wheat genotypes differing in Cd tolerance at seedling stage. Field Crops Res. 77, 93–98. doi: 10.1016/S0378-4290(02)00061-8
Zhang, L., Gao, C., Chen, C., Zhang, W., Huang, X. Y., and Zhao, F. J. (2020). Overexpression of rice OsHMA3 in wheat greatly decreases cadmium accumulation in wheat grains. Environ. Sci. Technol. 54, 10100–10108. doi: 10.1021/acs.est.0c02877
Zhang, M., Liu, X., Yuan, L., Wu, K., Duan, J., Wang, X., et al. (2012). Transcriptional profiling in cadmium-treated rice seedling roots using suppressive subtractive hybridization. Plant Physiol. Biochem. 50, 79–86. doi: 10.1016/j.plaphy.2011.07.015
Zhang, S., Quan, L., Zhu, Y., Yan, J., He, X., Zhang, J., et al. (2020). Differential effects of three amendments on the immobilisation of cadmium and lead for Triticum aestivum grown on polluted soil. Environ. Sci. Pollut. Res. 27, 40434–40442. doi: 10.1007/s11356-020-10079-6
Zhao, F. J., Ma, Y., Zhu, Y. G., Tang, Z., and McGrath, S. P. (2015). Soil contamination in China: current status and mitigation strategies. Environ. Sci. Technol. 49, 750–759. doi: 10.1021/es5047099
Zhao, J., Yang, W., Zhang, S., Yang, T., Liu, Q., Dong, J., et al. (2018). Genome-wide association study and candidate gene analysis of rice cadmium accumulation in grain in a diverse rice collection. Rice 11:61. doi: 10.1186/s12284-018-0254-x
Zhao, K., Liu, X., Xu, J., and Selim, H. M. (2010). Heavy metal contaminations in a soil–rice system: identification of spatial dependence in relation to soil properties of paddy fields. J. Hazard. Mater. 181, 778–787. doi: 10.1016/j.jhazmat.2010.05.081
Zhen, H., Jia, L., Huang, C., Qiao, Y., Li, J., Li, H., et al. (2020). Long-term effects of intensive application of manure on heavy metal pollution risk in protected-field vegetable production. Environ. Pollut. 263:114552. doi: 10.1016/j.envpol.2020.114552
Zhou, C., Zhu, L., Ma, Z., and Wang, J. (2017). Bacillus amyloliquefaciens SAY09 increases cadmium resistance in plants by activation of auxin-mediated signaling pathways. Genes 8:173. doi: 10.3390/genes8070173
Zhu, W., Du, W., Shen, X., Zhang, H., and Ding, Y. (2017). Comparative adsorption of Pb and Cd by cow manure and its vermicompost. Environ. Pollut. 227, 89–97. doi: 10.1016/j.envpol.2017.04.048
Zhu, Y., Wang, H., Lv, X., Zhang, Y., and Wang, W. (2020). Effects of biochar and biofertilizer on cadmium-contaminated cotton growth and the ant oxidative defense system. Sci. Rep. 10, 1–12. doi: 10.1038/s41598-020-77142-7
Zhuo, F., Zhang, X. F., Lei, L. L., Yan, T. X., Lu, R. R., Hu, Z. H., et al. (2020). The effect of arbuscular mycorrhizal fungi and biochar on the growth and Cd/Pb accumulation in Zea mays. Int. J. Phytoremediation 22, 1009–1018. doi: 10.1080/15226514.2020.1725867
Zia-ur-Rehman, M., Zafar, M., Waris, A. A., Rizwan, M., Ali, S., Sabir, M., et al. (2020). Residual effects of frequently available organic amendments on cadmium bioavailability and accumulation in wheat. Chemosphere 244:125548. doi: 10.1016/j.chemosphere.2019.125548
Zivkovic, L. I., Rikalovic, M., Cvijovic, G. G., Kazazic, S., Vrvic, M., Brceski, I., et al. (2018). Cadmium specific proteomic responses of a highly resistant Pseudomonas aeruginosasan ai. RSC Adv. 8, 10541– 10549.
Zouari, M., Elloumi, N., Ahmed, C. B., Delmail, D., Rouina, B. B., Abdallah, F. B., et al. (2016). Exogenous proline enhances growth, mineral uptake, antioxidant defense, and reduces cadmium-induced oxidative damage in young date palm (Phoenix dactylifera L.). Ecol. Engg. 86, 202–209. doi: 10.1016/j.ecoleng.2015.11.016
Zulfiqar, U., Ayub, A., Hussain, S., Waraich, E. A., El-Esawi, M. A., Ishfaq, M., et al. (2021). Cadmium toxicity in plants: recent progress on morpho-physiological effects and remediation strategies. J. Soil Sci. Plant Nutr. [Epub ahead of print]. doi: 10.1007/s42729-021-00645-3
Keywords: cadmium, contamination, abiotic stress, plant physiology and growth, remediation
Citation: Zulfiqar U, Jiang W, Xiukang W, Hussain S, Ahmad M, Maqsood MF, Ali N, Ishfaq M, Kaleem M, Haider FU, Farooq N, Naveed M, Kucerik J, Brtnicky M and Mustafa A (2022) Cadmium Phytotoxicity, Tolerance, and Advanced Remediation Approaches in Agricultural Soils; A Comprehensive Review. Front. Plant Sci. 13:773815. doi: 10.3389/fpls.2022.773815
Received: 10 September 2021; Accepted: 02 February 2022;
Published: 09 March 2022.
Edited by:
Ann Cuypers, Hasselt University, BelgiumReviewed by:
Bhumi Nath Tripathi, Indira Gandhi National Tribal University, IndiaMohsin Tanveer, University of Tasmania, Australia
Copyright © 2022 Zulfiqar, Jiang, Xiukang, Hussain, Ahmad, Maqsood, Ali, Ishfaq, Kaleem, Haider, Farooq, Naveed, Kucerik, Brtnicky and Mustafa. This is an open-access article distributed under the terms of the Creative Commons Attribution License (CC BY). The use, distribution or reproduction in other forums is permitted, provided the original author(s) and the copyright owner(s) are credited and that the original publication in this journal is cited, in accordance with accepted academic practice. No use, distribution or reproduction is permitted which does not comply with these terms.
*Correspondence: Wang Xiukang, d2FuZ3hpdWthbmdAeWF1LmVkdS5jbg==; Adnan Mustafa, YWRuYW5tdXN0YWZhNzgwQGdtYWlsLmNvbQ==
†These authors have contributed equally to this work