- 1The Laboratory of Seed Science and Technology, Guangdong Key Laboratory of Plant Molecular Breeding, Guangdong Laboratory of Lingnan Modern Agriculture, State Key Laboratory for Conservation and Utilization of Subtropical Agro-Bioresources, South China Agricultural University, Guangzhou, China
- 2Yuxi Zhongyan Tobacco Seed Co., Ltd., Yuxi, China
Reactive oxygen species (ROS) play an essential role in the regulation of seed dormancy, germination, and deterioration in plants. The low level of ROS as signaling particles promotes dormancy release and triggers seed germination. Excessive ROS accumulation causes seed deterioration during seed storage. Maintaining ROS homeostasis plays a central role in the regulation of seed dormancy, germination, and deterioration in crops. This study highlights the current advances in the regulation of ROS homeostasis in dry and hydrated seeds of crops. The research progress in the crosstalk between ROS and hormones involved in the regulation of seed dormancy and germination in crops is mainly summarized. The current understandings of ROS-induced seed deterioration are reviewed. These understandings of ROS-dependent regulation on seed dormancy, germination, and deterioration contribute to the improvement of seed quality of crops in the future.
Introduction
Reactive oxygen species (ROS) are known as a class of highly reactive and oxygen-bearing molecules including superoxide anion (O2⋅–), hydrogen peroxide (H2O2), hydroxyl radical (OH), and singlet oxygen (1O2) (Nathan and Ding, 2010). It has been well reported that ROS plays a pivotal function in the regulation of seed dormancy, germination, and deterioration (Kurek et al., 2019; Considine and Foyer, 2021). The low level of ROS as signaling particles promotes physiological dormancy release and triggers seed germination (Kumar et al., 2015; Considine and Foyer, 2021). However, the high level of ROS usually causes the orthodox seed deterioration under natural and artificial aging conditions by influencing lipid peroxidation, membrane permeability, defective proteins, antioxidant system, mitochondrial degradation, and DNA and RNA damages (Kurek et al., 2019). Therefore, keeping a balance in the ROS levels in seeds plays an important role in the regulation of seed dormancy, germination, and deterioration. In this study, the current advances in the regulation of ROS in seed dormancy, germination, and deterioration in crops are reviewed mainly considering three aspects: (1) the regulation of ROS homeostasis in seeds, (2) the crosstalk between ROS and hormones in seed dormancy and germination, (3) and ROS involving in seed deterioration.
Regulation of Reactive Oxygen Species Homeostasis in Seeds
Production of Reactive Oxygen Species in Seeds
In dry seeds, the ROS are generated by the non-enzymatic reaction, mainly the autooxidation of lipids (Bewley et al., 2012). Lipids are easily oxidized as the main source of free radicals under low humidity conditions in seeds during dry storage, while a weakening lipid oxidation occurs with the increase in humidity (Singh et al., 2014). When seed imbibition with the water content increased from 8–10 to 50% or more, the production of ROS begins to switch from non-enzymatic system to the enzymatic system in seeds (Kibinza et al., 2006; Bazin et al., 2011; Basbouss-Serhal et al., 2016; Bailly, 2019). The mitochondrion is an important site for the main source of cellular ROS in seeds (Bailly, 2004). In the matrix of mitochondria, the oxygen consumed during electron transport is reduced to superoxide through the respiratory electron transport chain (RETC), and then the produced superoxide is converted to H2O2 by Mn superoxide dismutase (Mn-SOD) or Cu/Zn-SOD (Figure 1; Imlay, 2003; Møller et al., 2020). Chloroplasts are another vital source of ROS in photosynthesizing cells. Illumination of photosystem I (PSI) and photosystem II (PS II) generates O2⋅–, OH, and 1O2 (Pospíšil et al., 2004; Pospíšil, 2009; Richards et al., 2015), and the O2⋅– is converted into H2O2 by Fe-SOD or Cu/Zn-SOD in chloroplasts (Waszczak et al., 2018). Meanwhile, the glycolates derived from chloroplast are converted into glyoxylate and H2O2 by glycolate oxidase (GOX) in peroxisomes (Considine and Foyer, 2021).
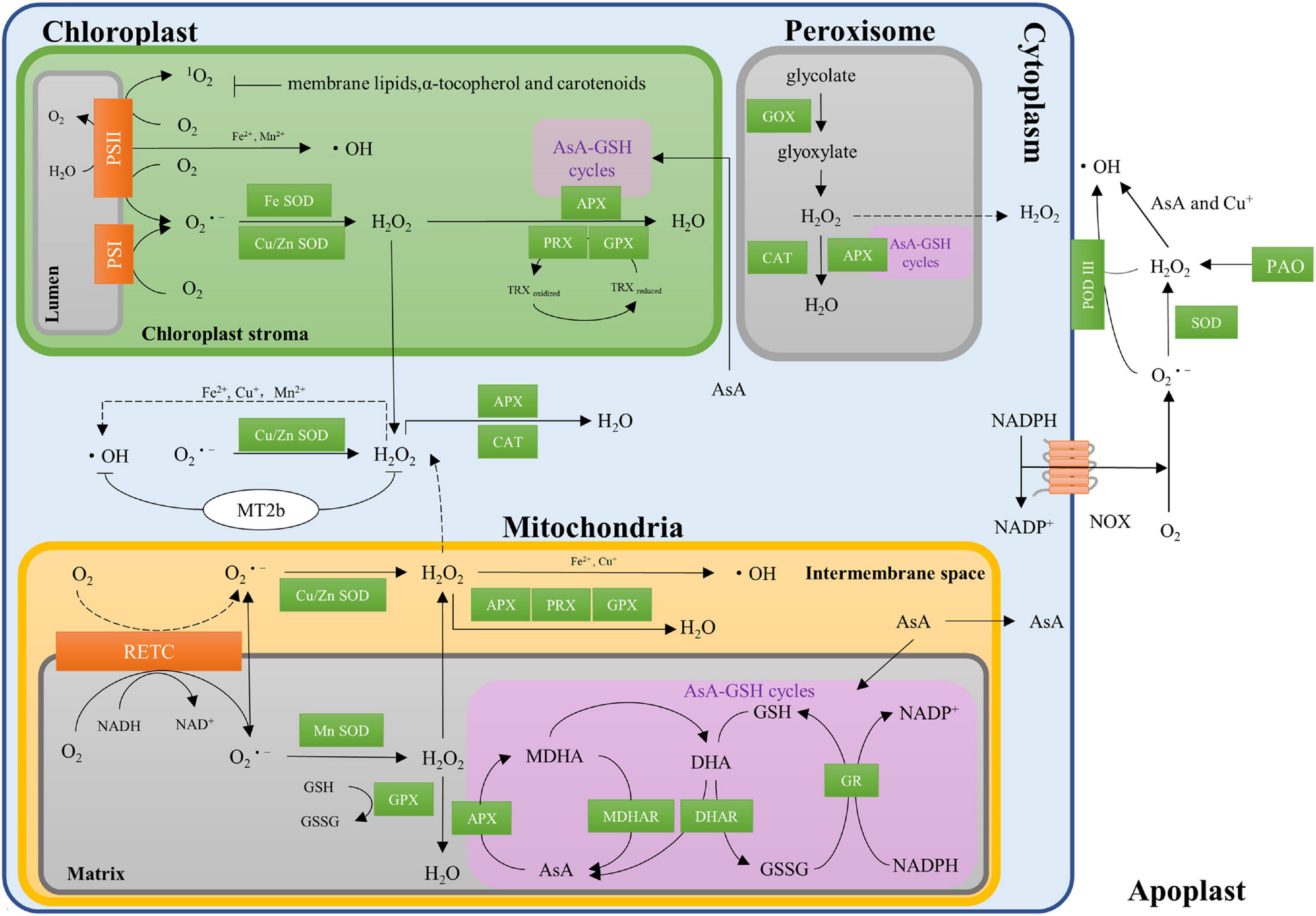
Figure 1. Regulation of reactive oxygen species (ROS) homeostasis in crop seeds. NADP+, nicotinamide adenine dinucleotide phosphate; NADPH, reduced form of NADP+; NAD+, nicotinamide adenine dinucleotide phosphate; NADH, nicotinamide adenine dinucleotide; NOXs, transmembrane NADPH oxidases; RETC, the respiratory electron transport chain; SOD, superoxide dismutase; GPX, glutathione peroxidase; AsA, ascorbate; GSH, glutathione; AsA-GSH cycles, the ascorbate-glutathione cycles; APX, ascorbate peroxidase; MDHAR, monodehydroascorbate reductase; DHAR, dehydroascorbate reductase; GR, glutathione reductase; MDHA, monodehydroascorbate; DHA, dehydroascorbate; GSSG, oxidized glutathione; PRX, peroxiredoxins; PSI/II, photosystem I/II; TRX, thioredoxin; MT2b, type 2 metallothioneins; CAT, catalase; GOX, glycolate oxidase; PAO, polyamine oxidase; POD III, secreted class III heme-containing peroxidases.
Transmembrane NADPH oxidases (NOXs) are well-studied cytosolic ROS-producing enzymes in plants (Ishibashi et al., 2015; Kai et al., 2016; Li et al., 2017). NOXs mediate the transfer of electrons from cytosolic NADPH, through flavin adenine dinucleotide (FAD) to penetrate the membrane, via hemes, to oxygen, leading to superoxide generation (Figure 1; Katerina and Cosa, 2016). The production of ROS also occurs in the apoplastic system (Richards et al., 2015; Waszczak et al., 2018). For example, H2O2 is generated by polyamine oxidase (PAO) during the catalytic synthesis between spermidine and spermine (Yoda et al., 2006; Moschou et al., 2008). The OH is converted from O2⋅– and H2O2 by ascorbate (Fry, 1998; Schopfer, 2001) and secreted class III heme-containing peroxidases (POD III) in the apoplastic system (Mika et al., 2008; Heyno et al., 2011; Miura, 2012). Interestingly, OH could be produced from H2O2 through metal-based (Fe2+, Cu+, or Mn2+) Haber-Weiss or Fenton reactions in all mitochondria, chloroplasts, and cytoplasm (Pospíšil et al., 2004; Pospíšil, 2009). The enzymatic reactions in dry seeds are inactive, while the ROS generation by non-enzymatic reactions remains poorly understood in crops. It is important to understand the contributions of each site such as mitochondrion, peroxisomes, chloroplasts, cytoplasm, and apoplastic systems on ROS generation in the future.
Scavenging System of Reactive Oxygen Species in Seeds
To keep ROS homeostasis in seeds, the internal antioxidant defense systems comprising of both enzymatic and non-enzymatic components are activated to relieve oxidative damages. Superoxide dismutase (SOD) including Mn-SOD, Fe-SOD, and Cu/Zn-SOD as important enzymatic components are widely distributed in the mitochondrial, chloroplasts, cytosol, and extracellular space of cells (Imlay, 2003). SODs can dismutate superoxide radicals into H2O2 (Figure 1). Then, H2O2 is converted into water and oxygen by catalase (CAT), glutathione peroxidase (GPX), peroxiredoxins (PRX), or by the ascorbate-glutathione (AsA-GSH) cycle (Figure 1). Several components such as ascorbate peroxidase (APX), monodehydroascorbate (MDHA), monodehydroascorbate reductase (MDHAR), dehydroascorbate reductase (DHAR), and glutathione reductase (GR) involve in the AsA-GSH cycle (Noctor and Foyer, 1998; Bailly, 2004), in which AsA is utilized as a specific electron donor to invert H2O2 to water by APX.
Several non-enzymatic components such as ascorbic acid (AsA, vitamin C), glutathione (GSH), hioredoxin (TRX), α-tocopherol (vitamin E), and carotenoids have been identified as the potent antioxidants in seeds (Figure 1). Of them, AsA and GSH have long been considered to function together in the AsA-GSH cycle (Foyer and Halliwell, 1976). Both GSH and TRX can be used as reducing substrates by GPX in the detoxification of H2O2 (Herbette et al., 2002). It has also been reported that membrane lipids, α-tocopherol (vitamin E), and carotenoids play important roles in clear 1O2 produced in the chloroplast (Krieger-Liszkay and Trebst, 2006; Ramel et al., 2012).
Metallothioneins (MTs) can bind metal ions through the thiol groups of their cysteine residues, which have been reported to be involved in the scavenging of ROS in the past decades. For example, the MTs can scavenge⋅OH and O2⋅– in seeds (Figure 1; Hassinen et al., 2011), and overexpression of OsMT2b can reduce the H2O2 production in rice (Wong et al., 2004). Altogether, ROS homeostasis is controlled through a complex network of ROS production and scavenging systems, while its molecular mechanisms such as MTs involved in ROS homeostasis remain unclear. Maintaining ROS homeostasis plays a central role in seed dormancy, germination, and deterioration, and whether MTs involved in seed dormancy, germination, and deterioration needs further investigation.
Reactive Oxygen Species Involved in Regulation of Seed Dormancy and Germination
Roles of Reactive Oxygen Species in Regulation of Seed Dormancy and Germination
The regulatory roles of ROS in dormancy release and seed germination in crops have been reported. For example, the non-enzymatic ROS generation frequently occurs in seeds contributing to dormancy release during desiccated seed storage (Finch-Savage and Leubner-Metzger, 2006). The accumulation of H2O2, OHs, and superoxide radicals has been widely observed during seed germination (Schopfer, 2001; Morohashi, 2002; Kranner et al., 2010; Li et al., 2017). Rice PAO OsPAO5 oxidizes PAs and releases H2O2, which is involved in coleorhiza-limited seed germination (Chen et al., 2016). The ROS produced by NOXs are involved in radical and root elongation during rice seed germination (Li et al., 2017). It has been reported that ROS-regulated dormancy release might be involved in mRNA oxidation (Bazin et al., 2011), protein carbonylation (Oracz et al., 2009), and oxidation (Bailly et al., 2008) in plants (Figure 2). For example, the oxidation of a specific subset of seed-stored mRNAs has been observed during dormancy alleviation by dry after-ripening. A total of 24 stored mRNAs, such as protein phosphatase 2C PPH1, mitogen-activated protein kinase phosphatase 1, and phenyl ammonia lyase 1, became highly oxidized during after-ripening in sunflower (Bazin et al., 2011). When seed germination, ROS can directly interact with polysaccharides of the cell wall that might promote cell elongation of the radical (Figure 2; Fry, 1998). A suitable ROS level will alleviate seed dormancy and trigger seed germination; however, the threshold of ROS level induced seed dormancy to germination, and its molecular mechanisms are understood poorly in most crops.
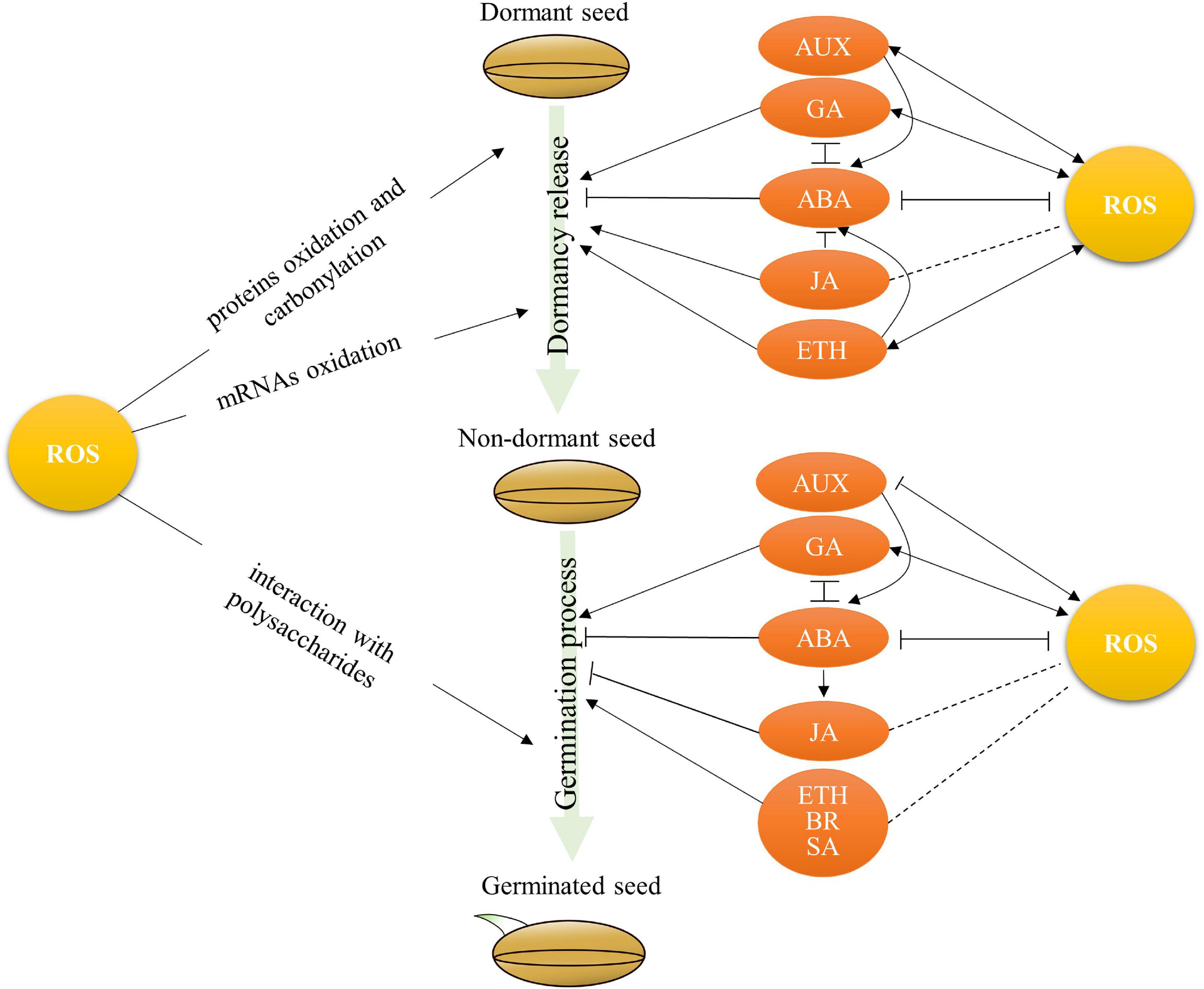
Figure 2. The crosstalk between ROS and hormones in regulation of seed dormancy and germination in crops. ABA, abscisic acid; GA, gibberellins; ETH, Ethylene; AUX, auxins; JA, jasmonates; SA, salicylic acid; BR, brassinosteroids. Arrows and lines with slanted dashes indicate positive and negative effects, respectively, and the dot lines indicate the putative effects.
Crosstalk Between Reactive Oxygen Species, Abscisic Acid, and Gibberellins in Regulation of Seed Dormancy and Germination
Abscisic acid (ABA) and gibberellins (GAs) are the main plant hormones that antagonistically mediate seed dormancy and germination (Finch-Savage and Leubner-Metzger, 2006). ABA induces seed dormancy and inhibits seed germination (Vaistij et al., 2013), while GA promotes dormancy release and facilitates seed germination (Gubler et al., 2008; Graeber et al., 2012). The involvement of ROS in seed dormancy and germination might be through the regulation of ABA and GA metabolisms in seeds (Figure 2). For instance, the biosynthesis of GA is stimulated by ROS through mitogen-activated protein kinase (MAPK) cascades (Kumar et al., 2015). The accumulation of H2O2 causes ABA degradation through influencing ABA catalytic enzyme (Ishibashi et al., 2015, 2017; Amooaghaie and Ahmadi, 2017; Li et al., 2018; Anand et al., 2019). The ascorbic acid and ROS involved in the inhibition of rice seed germination have been reported through influencing ABA levels (Ye et al., 2012). A recent study has shown that H2O2 enhances the germination capacity of primed tomato seeds due to the decrease in ABA/GA3 ratio by enhancing the expression of GA biosynthesis gene GA3ox1 and ABA catabolism gene ABA 8-hydroxylase (ABA-H) (Anand et al., 2019). These reports illustrate that ROS plays a positive role in GA synthesis and ABA degradation, which, in turn, facilitates dormancy release and seed germination.
Interestingly, the accumulation of ROS affected by GA and ABA has been observed in seeds (Figure 2). Exogenous GA treatments have been elucidated to induce ROS production. For example, the content of H2O2 and O2⋅– will be increased in caryopsis, embryo, and aleurone layer under GA3 treatment during the early imbibition stage in Avena fatua (Cembrowska-Lech et al., 2015). Similarly, exogenous GA3 and GA4+7 treatments could effectively promote the production of endogenous ROS during seed germination in Brassica parachinensis (Chen et al., 2021). However, the production of H2O2 and O2⋅– is suppressed by ABA treatment in both dormant and non-dormant seeds in sunflower (El-Maarouf-Bouteau et al., 2015). Therefore, the balance of ROS and ABA/GA levels plays an important role in seed dormancy and germination. For example, the changing of balance between ABA and ROS is active in barley seed embryos after imbibition and then regulates seed dormancy and germination (Ishibashi et al., 2017). One major QTL qSE3, which encodes a K+ transporter gene OsHAK21, positively regulates seed germination and seedling establishment by increasing ABA biosynthesis and activating ABA signaling responses, and then decreasing H2O2 level in germinating seeds under salinity stress in rice (He et al., 2019). Furthermore, the antagonism between ABA and GA partially mediated by ROS during seed germination has also been observed in rice (Ye and Zhang, 2012).
The molecular mechanism of the relationship between ROS homeostasis and the ABA signaling pathway has been conducted in Arabidopsis. It showed that Arabidopsis Abscisic Acid-Insensitive 5 (ABI5), a key component in ABA signaling, directly binds to the CAT1 promoter and activates CAT1 expression, and then ROS homeostasis is altered by ABI5 though affecting CATALASE expression and catalase activity (Finkelstein, 1994a,b; Bi et al., 2017). ABI4 directly combines with NADPH oxidase gene RbohD and Vitamin C Defective 2 (VTC2), the key genes involved in the ROS production and scavenging, to modulate ROS metabolism during seed germination under salinity stress (Luo et al., 2021). However, the molecular mechanisms of the crosstalk between ROS, ABA, and GA in the regulation of seed dormancy and germination are still poorly understood in crops.
Crosstalk Between Reactive Oxygen Species and Other Hormones in Regulation of Seed Dormancy and Germination
Other hormones such as ethylene (ETH), auxins, and jasmonates (JA), salicylic acid (SA), and brassinosteroids (BR) are also involved in the regulation of seed dormancy and germination (Figure 2). Several reports indicate that ROS might be also involved in the regulation of ETH and auxins on seed dormancy or germination in crops. For instance, the treatment of ROS-generated compound methylviologen increases the expression of ETH receptors ETR2 and ETH-responsive factors ERF1 in dormant sunflower embryos (Oracz et al., 2009). Exogenous ETH promotes dormancy release due to the ROS accumulation in dormant embryonic axes through activating NADPH oxidase by ETH in sunflower (El-Maarouf-Bouteau et al., 2015). The interaction of ROS, ABA, and ETH has been reported to regulate dormancy release in sunflowers (El-Maarouf-Bouteau et al., 2015). However, whether the crosstalk between ROS and ETH is involved in the regulation of seed germination remains unclear in crops. Biochemical analysis has revealed that the increase in H2O2 and the activation of peroxidases promote the oxidative degradation of IAA (Gazarian et al., 1998). In Arabidopsis, auxin promotes the production of superoxides such as NADPH oxidase and superoxide oxidase, while reducing the expression of antioxidant enzymes such as catalase and ascorbate oxidase (Iglesias et al., 2010; KrishnaMurthy and Rathinasabapathi, 2013). Similarly, the exogenous auxin regulates H2O2 metabolism by affecting the expression and activity of CuZn-superoxide dismutase, catalase, and peroxidase in tomatoes (Ja et al., 2009). The inhibition of auxin-stimulated NADH oxidase activity has been reported in the elongation growth of soybean hypocotyls (Morre et al., 1995). The crosstalk between ROS and auxin in the regulation of seed dormancy or germination might be through the influencing ROS homeostasis and auxin level.
It has been well reported that JA control seed dormancy and germination mainly through modulating ABA metabolism or signaling pathway. For example, JA promotes dormancy release through the suppression of ABA biosynthesis Ta9-cis-EPOXYCAROTENOID DIOXY-GENASE TaNCED1 and TaNCED2 in wheat (Xu et al., 2016), while ABA promotes JA biosynthesis to synergistically inhibit seed germination in rice (Wang et al., 2020). However, whether the crosstalk between ROS and JA is involved in the regulation of seed dormancy and seed germination needs further investigation in crops (Figure 2). Meanwhile, it has been reported that SA promotes seed germination under high salinity by modulating antioxidant activity in Arabidopsis (Lee et al., 2010). Spatiotemporal variations in SA and H2O2 have been observed in sunflower seeds during the transition from dormancy to germination (Vigliocco et al., 2020). Exogenous BRs increase seed germination under stress conditions in Brassica juncea (Soares et al., 2020). However, whether the crosstalk between ROS, SA, and BR is involved in the regulation of seed dormancy and germination needs further investigation in crops (Figure 2). It is an intriguing task to tackle the complicated network between ROS and hormones in regulating seed dormancy and germination in crops.
Reactive Oxygen Species Involved in Regulation of Seed Deterioration
Seed Deterioration and Reactive Oxygen Species Accumulation
Seed viability will be undermined due to deterioration under natural and artificial aging conditions in crops. For example, seed germination will be remarkably reduced after 18- and 24-months seed storage in sunflower in the dry airtight container under ambient temperature (Huang et al., 2021). In sweet corn, seed germination will be significantly decreased after 30 days of natural aging (17–28°C, 30–60% of RH) or after 0.5 h of artificial aging treatment (45°C, 100% of RH) (Zhang et al., 2022). Similarly, accelerated seed deterioration has been observed in rice and common bean under elevated temperature (45°C) and moisture (100% of RH) conditions (Dantas et al., 2019). When the seed experiences deterioration, the deleterious ROS will be largely accumulated in the seeds. In orthodox seeds, seed deterioration is caused by the reduction of antioxidant enzymes and the high accumulation of ROS (Ebone et al., 2019). Similarly, the higher accumulation of H2O2 could aggravate desiccation damage of recalcitrant seeds such as tea (Camellia sinensis) under chilling or drying stress (Chen et al., 2011). Thus, the instability of the intracellular ROS status causes the consequent oxidative damages to reduce seed viability in plants (Sano et al., 2016; Ebone et al., 2019).
Mechanisms of Reactive Oxygen Species in Regulation of Seed Deterioration
Seed deterioration caused by ROS is mainly involved in lipid peroxidation, protein oxidation, DNA and RNA damages, and repair system damage (Figure 3). Lipid peroxidation is the primary factor influencing seed deterioration (Zhao et al., 2021). The excessive ROS attack the membrane polysaturated fatty acids and divide the long-chain fatty acids into small compounds, which affects membrane permeability and ionic homeostasis (Oenel et al., 2017; Ebone et al., 2019). Lipid peroxidation disrupts many organelles, especially the mitochondrial damage influencing energy production for seed germination. Moreover, the end products formed from lipid peroxidation are also involved in seed deterioration. For example, the malondialdehyde (MDA) level is widely regarded as an indicator of lipid peroxidation and oxidative stress in seeds (Marnett, 1999). The 4-hydroxy-2,3-non-enal (HNE) influences the expression of genes by reacting with nucleic acids, proteins, and phospholipids during seed deterioration (Oenel et al., 2017).
Excessive ROS induces protein oxidation that is associated with seed deterioration in many crops (Figure 3). For instance, increased protein carbonylation has been observed in aged lettuce seeds (Adetunji et al., 2021) and Vigna unguiculata seeds (Boucelha et al., 2021). Several amino acids such as arginine, lysine, proline, and threonine residues with nucleophilic centers react with the reactive carbonyl species (RCS) derived from HNE and MDA (Smakowska et al., 2014; Satour et al., 2018; Biswas et al., 2020). However, Arabidopsis NADP-ME1 catalyzes the oxidative decarboxylation of malate to pyruvate that protects protein oxidation, especially carbonylation during seed deterioration (Yazdanpanah et al., 2019). The accumulation of carbonylated proteins results in the disruption of the tricarboxylic acid (TCA) cycle, electron transport chain (ETC) enzymes, and glycolysis in seeds (Yin et al., 2017; Chen et al., 2019; Zhang et al., 2021).
The disruption of DNA and RNA induced by ROS is also involved in seed deterioration (Figure 3; Sano et al., 2016). The nucleotide damage usually occurs in the hydroxylation at the C-8 position in guanine (G) to form 8-oxoguanine (8-oxoG) during seed deterioration, which results in transversion mutations (GC → TA) due to a mispair of 8-oxoG with adenine (A) or cytosine (C) during DNA replication (Johnston et al., 2010; Boesch et al., 2011; Sano et al., 2016; Ebone et al., 2019). The degradation of RNA is also observed in seed deterioration during storage in soybean (Fleming et al., 2017, 2018). A significant reduction in mean RNA integrity number (RIN) has been observed in soybean seeds after being stored dry at 5°C for 1–27 years, which is positively associated with seed germination (Fleming et al., 2017). The fragmented mRNA in dry-stored soybean seeds leads to inefficient translation and faulty proteins and then results in the loss of germination capacity (Fleming et al., 2018). To avoid seed deterioration, the repair system is induced during seed germination (Long et al., 2015). For example, the DNA and protein damages can be repaired by base excision repair (BER) (Sano et al., 2016) and L-isoaspartyl methyltransferase (PIMT), respectively (Mudgett et al., 1997; Bewley et al., 2012; Sano et al., 2016). Nevertheless, if the extent of seed deterioration is beyond the ability of the repair system, the loss of seed vigor will not be restored. Overall, the molecular mechanisms of seed deterioration are still poorly understood in crops.
Conclusion
In conclusion, ROS is mainly produced by lipid oxidation in dry seeds and enzymatic catalysis in hydrated seeds, respectively. The processes of ROS production occur in the mitochondrion, peroxisomes, chloroplasts, cytoplasm, and apoplastic systems in seeds. The antioxidant systems include the enzymatic and non-enzymatic systems involved in the scavenging ROS in seeds. Maintaining ROS homeostasis plays a central role in seed dormancy, germination, and deterioration in crops. The crosstalk between ROS, ABA, and GA in the regulation of seed dormancy and germination has been well investigated. However, the crosstalk between ROS and other hormones such as ETH, JA, SA, and BR involved in the regulation of seed dormancy and seed germination remains unclear in crops. The seed deterioration caused by excessive ROS accumulation is widely considered due to influencing lipid peroxidation, protein oxidation, DNA and RNA damages, and repair system damage in seeds under natural and artificial aging conditions in crops. Overall, the mechanisms of ROS regulation on seed dormancy, germination, and deterioration remain poorly understood in crops.
Author Contributions
ZW and YZ designed the manuscript. ZW, WL, YZ, and YN wrote the manuscript. All authors contributed to the article and approved the submitted version.
Funding
This research was funded by the National Natural Science Foundation of China (Grant Nos. 31971995, 31901601, and 31771889), the Natural Science Foundation of Guangdong Province (Grant No. 2020A1515011053), and startup funding from the South China Agricultural University.
Conflict of Interest
YN and YZ were employed in Yuxi Zhongyan Tobacco Seed Co., Ltd.
The remaining authors declare that the research was conducted in the absence of any commercial or financial relationships that could be construed as a potential conflict of interest.
Publisher’s Note
All claims expressed in this article are solely those of the authors and do not necessarily represent those of their affiliated organizations, or those of the publisher, the editors and the reviewers. Any product that may be evaluated in this article, or claim that may be made by its manufacturer, is not guaranteed or endorsed by the publisher.
References
Adetunji, A. E., Sershen Varghese, B., and Pammenter, N. (2021). Effects of exogenous application of five antioxidants on vigour, viability, oxidative metabolism and germination enzymes in aged cabbage and lettuce seeds. S. Afr. J. Bot. 137, 85–97. doi: 10.1016/j.sajb.2020.10.001
Amooaghaie, R., and Ahmadi, F. (2017). Triangular interplay between ROS, ABA and GA in dormancy alleviation of Bunium persicum seeds by cold stratification. Russ. J. Plant Physiol. 64, 588–599. doi: 10.1134/s1021443717040021
Anand, A., Kumari, A., Thakur, M., and Koul, A. (2019). Hydrogen peroxide signaling integrates with phytohormones during the germination of magnetoprimed tomato seeds. Sci. Rep. 9:8814 doi: 10.1038/s41598-019-45102-5
Bailly, C. (2004). Active oxygen species and antioxidants in seed biology. Seed. Sci. Res. 14, 93–107. doi: 10.1079/ssr2004159
Bailly, C. (2019). The signalling role of ROS in the regulation of seed germination and dormancy. Biochem. J. 476, 3019–3032. doi: 10.1042/bcj20190159
Bailly, C., El-Maarouf-Bouteau, H., and Corbineau, F. (2008). From intracellular signaling networks to cell death: the dual role of reactive oxygen species in seed physiology. C. R. Biol. 331, 806–814. doi: 10.1016/j.crvi.2008.07.022
Basbouss-Serhal, I., Leymarie, J., and Bailly, C. (2016). Fluctuation of Arabidopsis seed dormancy with relative humidity and temperature during dry storage. J. Exp. Bot. 67, 119–130. doi: 10.1093/jxb/erv439
Bazin, J., Langlade, N., Vincourt, P., Arribat, S., Balzergue, S., El-Maarouf-Bouteau, H., et al. (2011). Targeted mRNA oxidation regulates sunflower seed dormancy alleviation during dry after-ripening. Plant Cell 23, 2196–2208. doi: 10.1105/tpc.111.086694
Bewley, J. D., Bradford, K. J., Hilhorst, H. W. M., and Nonogaki, H. (2012). Seeds: Physiology of Development, Germination and Dormancy. Berlin: Springer Science & Business Media.
Bi, C., Ma, Y., Wu, Z., Yu, Y. T., Liang, S., Lu, K., et al. (2017). Arabidopsis ABI5 plays a role in regulating ROS homeostasis by activating CATALASE 1 transcription in seed germination. Plant Mol. Biol. 94, 197–213. doi: 10.1007/s11103-017-0603-y
Biswas, M. S., Terada, R., and Mano, J. I. (2020). Inactivation of carbonyl-detoxifying enzymes by H2O2 is a trigger to increase carbonyl load for initiating programmed cell death in plants. Antioxidants 9:141. doi: 10.3390/antiox9020141
Boesch, P., Weber-Lotfi, F., Ibrahim, N., Tarasenko, V., Cosset, A., Paulus, F., et al. (2011). DNA repair in organelles: pathways, organization, regulation, relevance in disease and aging. Biochim. Biophys. Acta Mol. Cell Res. 1813, 186–200. doi: 10.1016/j.bbamcr.2010.10.002
Boucelha, L., Abrous-Belbachir, O., and Djebbar, R. (2021). Is protein carbonylation a biomarker of seed priming and ageing? Funct. Plant Biol. 48, 611–623. doi: 10.1071/FP21001
Cembrowska-Lech, D., Koprowski, M., and Kepczynski, J. (2015). Germination induction of dormant Avena fatua caryopses by KAR1 and GA3 involving the control of reactive oxygen species (H2O2 and O2⋅–) and enzymatic antioxidants (superoxide dismutase and catalase) both in the embryo and the aleurone layers. J. Plant Physiol. 176, 169–179. doi: 10.1016/j.jplph.2014.11.010
Chen, B., Yin, G., Whelan, J., Zhang, Z., Xin, X., He, J., et al. (2019). Composition of mitochondrial complex I during the critical node of seed aging in Oryza sativa. J. Plant Physiol. 236, 7–14. doi: 10.1016/j.jplph.2019.02.008
Chen, B. X., Peng, Y. X., Yang, X. Q., et al. (2021). Delayed germination of Brassica parachinensis seeds by coumarin involves decreased GA4 production and a consequent reduction of ROS accumulation. Seed Sci. Res. 31, 224–235.
Chen, D., Li, Y., Fang, T., Shi, X., and Chen, X. (2016). Specific roles of tocopherols and tocotrienols in seed longevity and germination tolerance to abiotic stress in transgenic rice. Plant Sci. 244, 31–39. doi: 10.1016/j.plantsci.2015.12.005
Chen, Q., Yang, L., Ahmad, P., Wan, X., and Hu, X. (2011). Proteomic profiling and redox status alteration of recalcitrant tea (Camellia sinensis) seed in response to desiccation. Planta 233, 583–592. doi: 10.1007/s00425-010-1322-7
Considine, M. J., and Foyer, C. H. (2021). Stress effects on the reactive oxygen species-dependent regulation of plant growth and development. J. Exp. Bot. 72, 5795–5806. doi: 10.1093/jxb/erab265
Dantas, A. F., Fascineli, M. L., Jose, S. C. B. R., Padua, J. G., Gimenes, M. A., and Grisolia, C. K. (2019). Loss of genetic integrity in artificially aged seed lots of rice (Oryza sativa L.) and common bean (Phaseolus vulgaris L.). Mutat. Res. Genet. Toxicol. Environ. Mutagen. 846:403080. doi: 10.1016/j.mrgentox.2019.07.008
Ebone, L. A., Caverzan, A., and Chavarria, G. (2019). Physiologic alterations in orthodox seeds due to deterioration processes. Plant Physiol. Biochem. 145, 34–42. doi: 10.1016/j.plaphy.2019.10.028
El-Maarouf-Bouteau, H., Sajjad, Y., Bazin, J., Langlade, N., Cristescu, S. M., Balzergue, S., et al. (2015). Reactive oxygen species, abscisic acid and ethylene interact to regulate sunflower seed germination. Plant Cell Environ. 38, 364–374. doi: 10.1111/pce.12371
Finch-Savage, W. E., and Leubner-Metzger, G. (2006). Seed dormancy and the control of germination. New Phytol. 171, 501–523. doi: 10.1111/j.1469-8137.2006.01787.x
Finkelstein, R. R. (1994a). Maternal effects govern variable dominance of two abscisic acid response mutations in Arabidopsis thaliana. Plant Physiol. 105, 1203–1208. doi: 10.1104/pp.105.4.1203
Finkelstein, R. R. (1994b). Mutations at two new Arabidopsis ABA response loci are similar to the abi3 mutations. Plant J. 5, 765–771. doi: 10.1046/j.1365-313X.1994.5060765.x
Fleming, M. B., Patterson, E. L., Reeves, P. A., Richards, C. M., Gaines, T. A., and Walters, C. (2018). Exploring the fate of mRNA in aging seeds: protection, destruction, or slow decay? J. Exp. Bot. 69, 4309–4321. doi: 10.1093/jxb/ery215
Fleming, M. B., Richards, C. M., and Walters, C. (2017). Decline in RNA integrity of dry-stored soybean seeds correlates with loss of germination potential. J. Exp. Bot. 68, 2219–2230. doi: 10.1093/jxb/erx100
Foyer, C. H., and Halliwell, B. (1976). The presence of glutathione and glutathione reductase in chloroplasts: a proposed role in ascorbic acid metabolism. Planta 133, 21–25. doi: 10.1007/bf00386001
Fry, S. C. (1998). Oxidative scission of plant cell wall polysaccharides by ascorbate-induced hydroxyl radicals. Biochem. J. 332, 507–515. doi: 10.1042/bj3320507
Gazarian, I. G., Lagrimini, L. M., Mellons, F. A., Naldrett, M. J., Ashby, G. A., and Thorneley, R. N. F. (1998). Identification of skatolyl hydroperoxide and its role in the peroxidase-catalysed oxidation of indol-3-yl acetic acid. Biochem. J. 333, 223–232. doi: 10.1042/bj3330223
Graeber, K., Nakabayashi, K., Miatton, E., Leubner-Metzger, G., and Soppe, W. J. J. (2012). Molecular mechanisms of seed dormancy. Plant Cell Environ. 35, 1769–1786. doi: 10.1111/j.1365-3040.2012.02542.x
Gubler, F., Hughes, T., Waterhouse, P., and Jacobsen, J. (2008). Regulation of dormancy in barley by blue light and after-ripening: effects on abscisic acid and gibberellin metabolism. Plant Physiol. 147, 886–896. doi: 10.1104/pp.107.115469
Hassinen, V. H., Tervahauta, A. I., Schat, H., and Karenlampi, S. O. (2011). Plant metallothioneins - metal chelators with ROS scavenging activity? Plant Biol. 13, 225–232. doi: 10.1111/j.1438-8677.2010.00398.x
He, Y., Yang, B., He, Y., Zhan, C., Cheng, Y., Zhang, J., et al. (2019). A quantitative trait locus, qSE3, promotes seed germination and seedling establishment under salinity stress in rice. Plant J. 97, 1089–1104. doi: 10.1111/tpj.14181
Herbette, S., Lenne, C., Leblanc, N., Julien, J. L., Drevet, J. R., and Roeckel-Drevet, P. (2002). Two GPX-like proteins from Lycopersicon esculentum and Helianthus annuus are antioxidant enzymes with phospholipid hydroperoxide glutathione peroxidase and thioredoxin peroxidase activities. Eur. J. Med. Chem. 269, 2414–2420. doi: 10.1046/j.1432-1033.2002.02905.x
Heyno, E., Mary, V., Schopfer, P., and Krieger-Liszkay, A. (2011). Oxygen activation at the plasma membrane: relation between superoxide and hydroxyl radical production by isolated membranes. Planta 234, 35–45. doi: 10.1007/s00425-011-1379-y
Huang, Y., Cai, S., Ruan, X., Xu, J., and Cao, D. (2021). CSN improves seed vigor of aged sunflower seeds by regulating the fatty acid, glycometabolism, and abscisic acid metabolism. J. Adv. Res. 33, 1–13. doi: 10.1016/j.jare.2021.01.019
Iglesias, M. J., Terrile, M., Bartoli, C., D’Ippolito, S., and Casalongue, C. (2010). Auxin signaling participates in the adaptative response against oxidative stress and salinity by interacting with redox metabolism in Arabidopsis. Plant Mol. Biol. 74, 215–222. doi: 10.1007/s11103-010-9667-7
Imlay, J. A. (2003). Pathways of oxidative damage. Annu. Rev. Microbiol. 57, 395–418. doi: 10.1146/annurev.micro.57.030502.090938
Ishibashi, Y., Aoki, N., Kasa, S., Sakamoto, M., Kai, K., Tomokiyo, R., et al. (2017). The interrelationship between abscisic acid and reactive oxygen species plays a key role in barley seed dormancy and germination. Front. Plant Sci. 8:275. doi: 10.3389/fpls.2017.00275
Ishibashi, Y., Kasa, S., Sakamoto, M., Aoki, N., Kai, K., Yuasa, T., et al. (2015). A role for reactive oxygen species produced by NADPH oxidases in the embryo and aleurone cells in barley seed germination. PLoS One 10:e0143173. doi: 10.1371/journal.pone.0143173
Ja, T., Dunajska, K., Mazurek, P., and Piotrowska Ba Tretyn, A. (2009). Exogenous auxin regulates H2O2 metabolism in roots of tomato (Lycopersicon esculentum Mill.) seedlings affecting the expression and activity of CuZn-superoxide dismutase, catalase, and peroxidase. Acta Physiol. Plant 31, 249–260. doi: 10.1007/s11738-008-0225-8
Johnston, J. W., Pimbley, I., Harding, K., and Benson, E. E. (2010). Detection of 8-hydroxy-2′-deoxyguanosine as a marker of oxidative damage in DNA and germplasm exposed to cryogenic treatments. Cryo letters 31, 1–13.
Kai, K., Kasa, S., Sakamoto, M., Aoki, N., Watabe, G., Yuasa, T., et al. (2016). Role of reactive oxygen species produced by NADPH oxidase in gibberellin biosynthesis during barley seed germination. Plant Signal. Behav. 11:e1180492. doi: 10.1080/15592324.2016.1180492
Katerina, K., and Cosa, G. (2016). “Chapter 1: Overview of Reactive Oxygen Species,” in Singlet Oxygen: Applications in Biosciences and Nanosciences, eds N. Santi and C. Flors (London,UK: Royal Society of Chemistry), 1–21.
Kibinza, S., Vinel, D., Come, D., Bailly, C., and Corbineau, F. (2006). Sunflower seed deterioration as related to moisture content during ageing, energy metabolism and active oxygen species scavenging. Physiol. Plant 128, 496–506. doi: 10.1111/j.1399-3054.2006.00771.x
Kranner, I., Roach, T., Beckett, R. P., Whitaker, C., and Minibayeva, F. V. (2010). Extracellular production of reactive oxygen species during seed germination and early seedling growth in Pisum sativum. J. Plant Physiol. 167, 805–811. doi: 10.1016/j.jplph.2010.01.019
Krieger-Liszkay, A., and Trebst, A. (2006). Tocopherol is the scavenger of singlet oxygen produced by the triplet states of chlorophyll in the PSII reaction centre. J. Exp. Bot. 57, 1677–1684. doi: 10.1093/jxb/erl002
KrishnaMurthy, A., and Rathinasabapathi, B. (2013). Oxidative stress tolerance in plants Novel interplay between auxin and reactive oxygen species signaling. Plant Signal. Behav. 8:e25761. doi: 10.4161/psb.25761
Kumar, S. P. J., Prasad, S. R., Banerjee, R., and Thammineni, C. (2015). Seed birth to death: dual functions of reactive oxygen species in seed physiology. Ann. Bot. 116, 663–668. doi: 10.1093/aob/mcv098
Kurek, K., Plitta-Michalak, B., and Ratajczak, E. (2019). Reactive oxygen species as potential drivers of the seed aging process. Plants Basel 8:174. doi: 10.3390/plants8060174
Lee, S., Kim, S. G., and Park, C. M. (2010). Salicylic acid promotes seed germination under high salinity by modulating antioxidant activity in Arabidopsis. New Phytol. 188, 626–637. doi: 10.1111/j.1469-8137.2010.03378.x
Li, W. Y., Chen, B. X., Chen, Z.-J., Gao, Y. T., Chen, Z., and Liu, J. (2017). Reactive oxygen species generated by NADPH oxidases promote radicle protrusion and root elongation during rice seed germination. Int. J. Mol. Sci. 18:110. doi: 10.3390/ijms18010110
Li, Z., Gao, Y., Zhang, Y., Lin, C., Gong, D., Guan, Y., et al. (2018). Reactive oxygen species and gibberellin acid mutual induction to regulate tobacco seed germination. Front. Plant Sci. 9:1279. doi: 10.3389/fpls.2018.01279
Long, R. L., Gorecki, M. J., Renton, M., Scott, J. K., Colville, L., Goggin, D. E., et al. (2015). The ecophysiology of seed persistence: a mechanistic view of the journey to germination or demise. Biol. Rev. 90, 31–59. doi: 10.1111/brv.12095
Luo, X., Dai, Y., Zheng, C., Yang, Y., Chen, W., Wang, Q., et al. (2021). The ABI4-RbohD/VTC2 regulatory module promotes reactive oxygen species (ROS) accumulation to decrease seed germination under salinity stress. New Phytol. 229, 950–962. doi: 10.1111/nph.16921
Marnett, L. J. (1999). Lipid peroxidation-DNA damage by malondialdehyde. Mutat. Res. 424, 83–95. doi: 10.1016/S0027-5107(99)00010-X
Mika, A., Buck, F., and Luethje, S. (2008). Membrane-bound class III peroxidases: identification, biochemical properties and sequence analysis of isoenzymes purified from maize (Zea mays L.) roots. J. Proteom. 71, 412–424. doi: 10.1016/j.jprot.2008.06.006
Miura, T. (2012). A mechanistic study of the formation of hydroxyl radicals induced by horseradish peroxidase with NADH. J. Biochem. 152, 199–206. doi: 10.1093/jb/mvs068
Møller, I. M., Igamberdiev, A. U., Bykova, N. V., Finkemeier, I., Rasmusson, A. G., and Schwarzlaender, M. (2020). Matrix redox physiology governs the regulation of plant mitochondrial metabolism through posttranslational protein modifications. Plant Cell 32, 573–594. doi: 10.1105/tpc.19.00535
Morohashi, Y. (2002). Peroxidase activity develops in the micropylar endosperm of tomato seeds prior to radicle protrusion. J. Exp. Bot. 53, 1643–1650. doi: 10.1093/jxb/erf012
Morre, D. J., Brightman, A. O., Hidalgo, A., and Navas, P. (1995). Selective inhibition of auxin-stimulated NADH oxidase activity and elongation growth of soybean hypocotyls by thiol reagents. Plant Physiol. 107, 1285–1291. doi: 10.1104/pp.107.4.1285
Moschou, P. N., Paschalidis, K. A., Delis, I. D., Andriopoulou, A. H., Lagiotis, G. D., Yakoumakis, D. I., et al. (2008). Spermidine exodus and oxidation in the apoplast induced by abiotic stress is responsible for H2O2 signatures that direct tolerance responses in tobacco. Plant Cell 20, 1708–1724. doi: 10.1105/tpc.108.059733
Mudgett, M. B., Lowenson, J. D., and Clarke, S. (1997). Protein repair-L-isoaspartyl methyltransferase in plants. Phylogenetic distribution and the accumulation of substrate proteins in aged barley seeds. Plant Physiol. 115, 1481–1489. doi: 10.1104/pp.115.4.1481
Nathan, C., and Ding, A. H. (2010). SnapShot: reactive oxygen intermediates (ROI). Cell 140:951.e2. doi: 10.1016/j.cell.2010.03.008
Noctor, G., and Foyer, C. H. (1998). Ascorbate and glutathione: keeping active oxygen under control. Ann. Rev. Plant Biol. 49, 249–279. doi: 10.1146/annurev.arplant.49.1.249
Oenel, A., Fekete, A., Krischke, M., Faul, S. C., Gresser, G., Havaux, M., et al. (2017). Enzymatic and non-enzymatic mechanisms contribute to lipid oxidation during seed aging. Plant Cell Physiol. 58, 925–933. doi: 10.1093/pcp/pcx036
Oracz, K., El-Maarouf-Bouteau, H., Kranner, I., Bogatek, R., Corbineau, F., and Bailly, C. (2009). The mechanisms involved in seed dormancy alleviation by hydrogen cyanide unravel the role of reactive oxygen species as key factors of cellular signaling during germination. Plant Physiol. 150, 494–505. doi: 10.1104/pp.109.138107
Pospíšil, P. (2009). Production of reactive oxygen species by photosystem II. Biochim. Biophys. Acta Bioenerg. 1787, 1151–1160. doi: 10.1016/j.bbabio.2009.05.005
Pospíšil, P., Arato, A., Krieger-Liszkay, A., and Rutherford, A. W. (2004). Hydroxyl radical generation by photosystem II. Biochemistry 43, 6783–6792. doi: 10.1021/bi036219i
Ramel, F., Birtic, S., Cuine, S., Triantaphylides, C., Ravanat, J.-L., and Havaux, M. (2012). Chemical quenching of singlet oxygen by carotenoids in plants. Plant Physiol. 158, 1267–1278. doi: 10.1104/pp.111.182394
Richards, S. L., Wilkins, K. A., Swarbreck, S. M., Anderson, A. A., Habib, N., Smith, A. G., et al. (2015). The hydroxyl radical in plants: from seed to seed. J. Exp. Bot. 66, 37–46. doi: 10.1093/jxb/eru398
Sano, N., Rajjou, L., North, H. M., Debeaujon, I., Marion-Poll, A., and Seo, M. (2016). Staying alive: molecular aspects of seed longevity. Plant Cell Physiol. 57, 660–674. doi: 10.1093/pcp/pcv186
Satour, P., Youssef, C., Chatelain, E., Vu, B. L., Teulat, B., Job, C., et al. (2018). Patterns of protein carbonylation during Medicago truncatula seed maturation. Plant Cell Environ. 41, 2183–2194. doi: 10.1111/pce.13194
Schopfer, P. (2001). Hydroxyl radical-induced cell-wall loosening in vitro and in vivo: implications for the control of elongation growth. Plant J. 28, 679–688. doi: 10.1046/j.1365-313x.2001.01187.x
Singh, K. L., Chaudhuri, A., and Kar, R. K. (2014). Superoxide and its metabolism during germination and axis growth of Vigna radiata (L.) Wilczek seeds. Plant Signal. Behav. 9:e29278. doi: 10.4161/psb.29278
Smakowska, E., Czarna, M., and Janska, H. (2014). Mitochondrial ATP-dependent proteases in protection against accumulation of carbonylated proteins. Mitochondrion 19, 245–251. doi: 10.1016/j.mito.2014.03.005
Soares, T. F. S. N., Dias, D. C. F. D. S., Oliveira, A. M. S., Ribeiro, D. M., and Dias, L. A. D. S. (2020). Exogenous brassinosteroids increase lead stress tolerance in seed germination and seedling growth of Brassica juncea L. Ecotoxicol. Environ. Saf. 193:110296. doi: 10.1016/j.ecoenv.2020.110296
Vaistij, F. E., Gan, Y., Penfield, S., Gilday, A. D., Dave, A., He, Z., et al. (2013). Differential control of seed primary dormancy in Arabidopsis ecotypes by the transcription factor SPATULA. P. Natl. Acad. Sci. U.S.A. 110, 10866–10871. doi: 10.1073/pnas.1301647110
Vigliocco, A., Del Bel, Z., Veronica Perez-Chaca, M., Molina, A., Zirulnik, F., Maria Andrade, A., et al. (2020). Spatiotemporal variations in salicylic acid and hydrogen peroxide in sunflower seeds during transition from dormancy to germination. Physiol. Plant 169, 27–39. doi: 10.1111/ppl.13043
Wang, Y., Hou, Y., Qiu, J., Wang, H., Wang, S., Tang, L., et al. (2020). Abscisic acid promotes jasmonic acid biosynthesis via a ‘SAPK10-bZIP72-AOC’ pathway to synergistically inhibit seed germination in rice (Oryza sativa). New Phytol. 228, 1336–1353. doi: 10.1111/nph.16774
Waszczak, C., Carmody, M., and Kangasjarvi, J. (2018). Reactive oxygen species in plant signaling. Ann. Rev. Plant Biol. 69, 209-236. doi: 10.1146/annurev-arplant-042817-040322
Wong, H. L., Sakamoto, T., Kawasaki, T., Umemura, K., and Shimamoto, K. (2004). Down-regulation of metallothionein, a reactive oxygen scavenger, by the small GTPase OsRac1 in rice. Plant Physiol. 135, 1447–1456. doi: 10.1104/pp.103.036384
Xu, Q., Truong, T. T., Barrero, J. M., Jacobsen, J. V., Hocart, C. H., and Gubler, F. (2016). A role for jasmonates in the release of dormancy by cold stratification in wheat. J. Exp. Bot. 67, 3497–3508. doi: 10.1093/jxb/erw172
Yazdanpanah, F., Maurino, V. G., Mettler-Altmann, T., Buijs, G., Bailly, M., Jashni, M. K., et al. (2019). NADP-MALIC ENZYME 1 Affects germination after seed storage in Arabidopsis thaliana. Plant Cell Physiol. 60, 318–328. doi: 10.1093/pcp/pcy213
Ye, N., and Zhang, J. (2012). Antagonism between abscisic acid and gibberellins is partially mediated by ascorbic acid during seed germination in rice. Plant Signal. Behav. 7, 563–565. doi: 10.4161/psb.19919
Ye, N., Zhu, G., Liu, Y., Zhang, A., Li, Y., Liu, R., et al. (2012). Ascorbic acid and reactive oxygen species are involved in the inhibition of seed germination by abscisic acid in rice seeds. J. Exp. Bot. 63, 1809–1822. doi: 10.1093/jxb/err336
Yin, G., Xin, X., Fu, S., An, M., Wu, S., Chen, X., et al. (2017). Proteomic and carbonylation profile analysis at the critical node of seed ageing in Oryza sativa. Sci. Rep. 7:40611. doi: 10.1038/srep40611
Yoda, H., Hiroi, Y., and Sano, H. (2006). Polyamine oxidase is one of the key elements for oxidative burst to induce programmed cell death in tobacco cultured cells. Plant Physiol. 142, 193–206. doi: 10.1104/pp.106.080515
Zhang, K., Zhang, Y., Sun, J., Meng, J., and Tao, J. (2021). Deterioration of orthodox seeds during ageing: influencing factors, physiological alterations and the role of reactive oxygen species. Plant Physiol. Biochem. 158, 475–485. doi: 10.1016/j.plaphy.2020.11.031
Zhang, T., Ayed, C., Fisk, I. D., Pan, T., Wang, J., Yang, N., et al. (2022). Evaluation of volatile metabolites as potential markers to predict naturally-aged seed vigour by coupling rapid analytical profiling techniques with chemometrics. Food Chem. 367:130760. doi: 10.1016/j.foodchem.2021.130760
Keywords: reactive oxygen species, seed germination, seed dormancy, seed deterioration, crops
Citation: Li W, Niu Y, Zheng Y and Wang Z (2022) Advances in the Understanding of Reactive Oxygen Species-Dependent Regulation on Seed Dormancy, Germination, and Deterioration in Crops. Front. Plant Sci. 13:826809. doi: 10.3389/fpls.2022.826809
Received: 01 December 2021; Accepted: 25 January 2022;
Published: 23 February 2022.
Edited by:
María Serrano, Miguel Hernández University of Elche, SpainReviewed by:
Ganesh K. Jaganathan, University of Shanghai for Science and Technology, ChinaSven Krishnan Nelson, Heliponix, LLC, United States
Copyright © 2022 Li, Niu, Zheng and Wang. This is an open-access article distributed under the terms of the Creative Commons Attribution License (CC BY). The use, distribution or reproduction in other forums is permitted, provided the original author(s) and the copyright owner(s) are credited and that the original publication in this journal is cited, in accordance with accepted academic practice. No use, distribution or reproduction is permitted which does not comply with these terms.
*Correspondence: Yunye Zheng, zheng_yunye@126.com; Zhoufei Wang, wangzf@scau.edu.cn