- 1College of Life and Environmental Sciences, Hangzhou Normal University, Hangzhou, China
- 2Zhejiang Provincial Key Laboratory for Genetic Improvement and Quality Control of Medicinal Plants, Hangzhou Normal University, Hangzhou, China
- 3School of Public Health, Hangzhou Normal University, Hangzhou, China
Plant specialized metabolites (PSMs) play essential roles in the adaptation to harsh environments and function in plant defense responses. PSMs act as key components of defense-related signaling pathways and trigger the extensive expression of defense-related genes. In addition, PSMs serve as antioxidants, participating in the scavenging of rapidly rising reactive oxygen species, and as chelators, participating in the chelation of toxins under stress conditions. PSMs include nitrogen-containing chemical compounds, terpenoids/isoprenoids, and phenolics. Each category of secondary metabolites has a specific biosynthetic pathway, including precursors, intermediates, and end products. The basic biosynthetic pathways of representative PSMs are summarized, providing potential target enzymes of stress-mediated regulation and responses. Multiple metabolic pathways share the same origin, and the common enzymes are frequently to be the targets of metabolic regulation. Most biosynthetic pathways are controlled by different environmental and genetic factors. Here, we summarized the effects of environmental factors, including abiotic and biotic stresses, on PSM biosynthesis in various plants. We also discuss the positive and negative transcription factors involved in various PSM biosynthetic pathways. The potential target genes of the stress-related transcription factors were also summarized. We further found that the downstream targets of these Transcription factors (TFs) are frequently enriched in the synthesis pathway of precursors, suggesting an effective role of precursors in enhancing of terminal products. The present review provides valuable insights regarding screening targets and regulators involved in PSM-mediated plant protection in non-model plants.
Introduction
The ability of plants to synthesize an extremely wide arsenal of diverse metabolites makes them preeminent chemists (Fernie et al., 2004). Traditionally, plant metabolites are classified into two groups: primary and secondary (Patra et al., 2013). Primary metabolites are ubiquitous in all plants and play crucial housekeeping roles in plant growth and development (Fabregas and Fernie, 2021). Secondary metabolites, also called plant specialized metabolites (PSMs), are involved in various physiological and biochemical processes, such as defense and adaptation to adverse environments (Chapman et al., 2019; Sic Zlabur et al., 2021). With the development of detection technology, more PSMs have been identified and characterized in plants.
On the basis of their core structures, PSMs form three major categories: nitrogen-containing chemical compounds, terpenoids/isoprenoids, and phenolics (Marone et al., 2022). Nitrogen-containing compounds, consisting of cyanogenic glycosides, alkaloids, and glucosinolates, have been widely identified in natural plant products and synthetic compounds (Aharoni and Galili, 2011). Terpenoids/isoprenoids can be divided into five subgroups: monoterpenes, sesquiterpenes, diterpenes, triterpenes, and tetraterpenes, on the basis of the number of isoprene structural units (Bohlmann et al., 1998). Phenolics, containing at least one aromatic ring and one hydroxyl group, can be divided into four functional classes: phenolic acids, flavonoids, tannins, and stilbenes (Aharoni and Galili, 2011; Rasouli et al., 2016). Most of PSMs are produced by individual metabolic pathways and unequally accumulate in different tissues and organs. The structural complexity and uneven distribution ensure different biological functions of PSMs under changing environmental conditions (Desmet et al., 2021).
The roles of PSMs in human health and their potential as pharmaceutical drugs have been studied extensively (Hamilton, 2004; Tungmunnithum et al., 2018). Medicinal plants produce valuable PSM-derived drugs, such as, taxol from Taxus media, quinine from Cinchona officinalis, withanolide from Physalis angulate, and artemisinin from Artemisia annua, are widely applied in the treatment of a variety of serious diseases (Ravishankara et al., 2001; Zhan et al., 2018; Shi et al., 2021; Wani et al., 2021; Yu et al., 2021). In plants, PSMs are essential for several physiological processes, such as plant protection, pollinator attraction, signal transduction, and seed germination, which are required for their survival in harsh environments (Chae et al., 2014; Liu et al., 2021d; Singh et al., 2021; Wari et al., 2021).
Plant specialized metabolites contribute to plant protection against different types of biotic and abiotic stresses, similar to the adaptive immune system in animals (Castro-Moretti et al., 2020; Desmet et al., 2021). To adapt to stress conditions, plants gear their metabolism toward the biosynthesis of PSMs, which, although energy-costly, is beneficial for their survival (Abdala-Roberts et al., 2016). PSMs serve in various parts of a complete plant defense system, acting as message molecules and/or antioxidants (Kumar and Pandey, 2013). Some PSMs act as key components of complex signaling pathways and trigger both the extensive expression of defense-related genes and the accumulation of other metabolites (Maag et al., 2015). Other PSMs serve as antioxidants, participating in the scavenging of rapidly rising reactive oxygen species (ROS) and in the chelation of heavy-metal ions under stress conditions (Pacifico et al., 2021). They not only act as powerful antioxidants, they may also be toxic to herbivores, microbial pathogens, and competing plant species (Glas et al., 2012). Overall, some of the credit for a plant’s capability to tolerate or adapt to a changing environment goes to PSMs. The purpose of this review is to summarize the basic biosynthetic pathways of protection-related PSMs on a limited scale. Furthermore, the environmental and genetic factors involved in the biosynthesis of PSMs are also briefly summarized.
Basic Biosynthetic Pathway of Plant Specialized Metabolites
There are more than 400,000 vascular plants with up to one million metabolites on the Earth (Fang et al., 2019). Although there are many PSMs, their chemical structures are not random (Fernie et al., 2004). The vast majority of PSMs are variations on a core derived from several typical backbones having structural modifications, such as glycosylation, acylation, methylation, hydroxylation, and prenylation (Wang et al., 2019c). On the basis of the representative structures, PSMs can be grouped into major classes, such as nitrogen-containing compounds, terpenoids, and phenolics (D’Auria and Gershenzon, 2005). Here, we summarize the basic biosynthetic pathways of representative PSMs and attempt to better understand the potential targets of stress-mediated regulation.
Key Enzymes Involved in the Biosynthesis of Alkaloids
Alkaloids originally consisted of a large class of heterocyclic nitrogen-containing organic compounds (Zhang et al., 2021b). The nitrogen atom in the heterocyclic ring generally originates from an amino acid. On the basis of their amino acid precursors and chemical structures, alkaloids are classified into five subgroups: terpenoid indole alkaloids (TIAs), benzylisoquinoline alkaloids (BIAs), tropine alkaloids, purine alkaloids, and pyrrolizidine alkaloids (Bhambhani et al., 2021). From precursors to final products, a series of biochemical modification reactions occur during the different alkaloidal conversion steps, ensuring diverse arrays of chemical structures and biological activities (Lichman, 2021; Zhang et al., 2021c). The present review uses TIA and BIA as examples to investigate the complexity of alkaloid biosynthesis.
Terpenoid indole alkaloids are a class of PSMs found in various non-model medicinal plants, such as Catharanthus roseus, Rauvolfia serpentina, Ophiorrhiza pumila, and Vinca minor (Luijendijk et al., 1996; Schlager and Drager, 2016; Shi et al., 2020a; Zhan et al., 2020; Yang et al., 2021a; Vrabec et al., 2022). Catharanthus roseus is frequently used as the model plant to reveal a complete TIA biosynthetic pathway (O’Connor and Maresh, 2006). Strictosidine, a key skeleton unique to TIAs, is synthesized by strictosidine synthase with tryptamine, an indole ring donor derived from decarboxylated tryptophan, and secologanin, a terpenoid donor from the methylerythritol 4-phosphate (MEP) pathway (Moreno et al., 1993; Rai et al., 2013; Kumar et al., 2015). A series of key enzymes, including geraniol synthase, geraniol 10-hydroxylase, 10-hydroxygeraniol oxidoreductase, iridoid synthase, iridoid oxidase, 7-deoxyloganetic acid glucosyltransferase, 7-deoxyloganic acid, loganic acid-O-methyltransferase, and secologanin synthase, are involved in TIA skeleton biosynthesis (Geu-Flores et al., 2012; Simkin et al., 2013; Shen et al., 2017; Sandholu et al., 2020; Jeena et al., 2021). Then, the intermediate strictosidine is modified by different enzymes to produce species-specific TIAs (Qu et al., 2018; Williams et al., 2019). As detection technology progresses, more novel TIAs and TIA-related enzymes are being identified in different plant species.
Benzylisoquinoline alkaloids are also members of a structurally diverse class of PSMs that mainly exist in the Ranunculales order (Ziegler and Facchini, 2008). The biosynthesis of BIAs starts with dopamine and 4-hydroxyphenylacetaldehyde, a tyrosine derivative, to produce a fundamental precursor trihydroxylated alkaloid (S)-norcoclaurine by norcoclaurine synthase (Sheng and Himo, 2019). O-methylation, N-methylation, and hydroxylation successively occur on 4-hydroxyphenylacetaldehyde to synthetize (S)-3′-hydroxy-N-methylcoclaurine (Liu et al., 2021c). The conversion of (S)-3′-hydroxy-N-methylcoclaurine to (S)-reticuline, a branch-point product, in the production of morphine, tetrahydropalmatine, sanguinarine, and noscapine, is conducted by 3′-hydroxy-N-methylcoclaurine 4′-hydroxylase (He et al., 2018). Finally, cytochrome P450 superfamily proteins are responsible for several modification reactions, such as hydroxylation, isomerization, and coupling, on the BIA backbone that produce species-specific BIAs (Hori et al., 2018; Menendez-Perdomo and Facchini, 2018).
Key Enzymes Involved in the Biosynthesis of Glucosinolates
As a class of wound-induced PSMs, glucosinolates highly accumulate in the Brassicaceae family of plants (Sanchez-Pujante et al., 2017). Most of glucosinolates can be grouped into three major subgroups, aliphatic, indole-, and aromatic glucosinolates, on the basis of their amino acid features (Ishida et al., 2014). The complete biosynthetic pathway of glucosinolates in Brassica genus consists of three steps: side-chain elongation, core structure formation, and side-chain secondary modifications (Sotelo et al., 2016).
During side-chain elongation, aliphatic and aromatic amino acids are utilized to produce 2-oxo acids by branched-chain amino acid aminotransferase family enzymes. Then, 2-oxo acid and acetyl-CoA are condensed by methylthioalkylmalate synthase (Kochevenko et al., 2012). Side-chain elongation ends with an isomerization process and an oxidative decarboxylation by isopropylmalate isomerase and isopropylmalate dehydrogenase, respectively (Sanchez-Pujante et al., 2017). To produce the core structure of glucosinolates, the conversion of side-chain-elongated amino acids to aldoximes is catalyzed by cytochrome P450 mono-oxygenases, such as CYP79 and CYP83, to produce S-alkyl-thiohydroximate and thiohydroximate (Robin et al., 2016). Then, thiohydroximate is catalyzed to form the glucosinolate core structure by two key enzymes, uridine diphosphate glycosyltransferase 74 and sulfotransferases (Sonderby et al., 2010; Robin et al., 2016). Finally, side-chain modifications, such as oxidation, hydroxylation, methoxylation, alkenylation, and benzoylation, are required for the formation of the terminal glucosinolate products (Halkier and Gershenzon, 2006; Nguyen et al., 2020).
Key Enzymes Involved in the Biosynthesis of Terpenoids
Terpenoids are a structurally diverse group of PSMs in which each member has a core isoprene unit. The central core of the terpenoids is synthesized by one-unit dimethylallyl diphosphate (DMAPP) and three-units isopentenyl diphosphates (IPP) (Yu et al., 2017). Both DMAPP and IPP originate from the MEP pathway, occurring in the plastids, and from the mevalonate pathway, occurring in the cytoplasm, endoplasmic reticulum, and peroxisomes (Mahmoud et al., 2021).
Here, we take model plant Arabidopsis as an example. Isopentenyl phosphate kinase, common to most plants, catalyzes the conversion of isopentenyl monophosphate and dimethylallyl monophosphate to IPP and DMAPP (Henry et al., 2015). In plants, there is a classic upstream pathway that forms prenyl diphosphates having varied chain lengths, such as geranyl diphosphate (GPP), having 10 isoprene units, farnesyl diphosphate (FPP), having 15 isoprene units, and geranylgeranyl diphosphate (GGPP), having 20 isoprene units (Tholl, 2015; Jia and Chen, 2016). Next, terpene synthases participate in the conversion of FPP, GGPP, and GPP into mono-/sesqui-terpenes (Pichersky and Raguso, 2018; Zhou and Pichersky, 2020). Two units of FPP and one unit of GGPP can be condensed by squalene synthase to produce squalene and by phytoene synthase to produce phytoene, which are the precursors of sterols and carotenoids, respectively (Christianson, 2017). Thousands of different terpenoids having the same core skeleton are produced by various modifications, such as hydroxylation, dehydrogenation, reduction, glycosylation, methylation, and acylation (Liu et al., 2022a).
Key Enzymes Involved in the Biosynthesis of Phenolics
Phenolic Acids
Phenolic acids are important active ingredients in numerous medicinal plants (Chen et al., 2021b). Bioactivities, biosynthesis and biotechnological production of phenolic acids have been well revealed in Salvia miltiorrhiza (Shi et al., 2019). On the basis of the number aromatic ring structures, phenolic acids can be classified into different groups (Choi et al., 2021). Taking S. miltiorrhiza as an example, most phenolic acids are synthesized through the phenylpropanoid and tyrosine metabolic pathways (Wang et al., 2015). In the phenylpropanoid pathway, phenylalanine is treated as a substrate to produce cinnamic acid by phenylalanine ammonia-lyase (PAL) (Reyes Jara et al., 2022). Then, cinnamic acid is catalyzed to p-coumaroyl-CoA by two enzymes, cinnamic acid 4-hydroxylase (C4H), and 4-coumarate: CoA ligase (Huang et al., 2008). In the tyrosine pathway, tyrosine aminotransferase and hydroxyphenylpyruvate reductase are involved in the conversion of tyrosine to 3,4-dihydroxyphenyllactic acid (Rizi et al., 2021).
Subsequently, rosmarinic acid, an important precursor for downstream species-specific phenolic acids, is synthetized by rosmarinic acid synthase and cytochrome P450-dependent monooxygenase CYP98A14 (Deng et al., 2020b; Chen et al., 2021b). Over-expression of rosmarinic acid synthase and CYP98A14 resulted in higher content of phenolic acids in S. miltiorrhiza hairy roots (Fu et al., 2020).
Flavonoids
Flavonoids are a class of water-soluble pigments stored in the cell vacuoles (Dong and Lin, 2021). In plants, more than 9,000 flavonoids have been identified and classified into different groups on the basis of the number of hydroxyl/methyl groups on their heterocyclic or benzene ring (Noda et al., 2017). As phenolics, flavonoids also originated from the phenylpropanoid pathway (Wang et al., 2018b). Specific flavonoid biosynthesis starts with the conversion of p-coumaroyl-CoA, together with malonyl-CoA and acetyl-CoA, to naringenin chalcone by chalcone synthase, which is the first rate-limiting enzyme in the flavonoid biosynthetic pathway (Zhang et al., 2017). Naringenin chalcone, a basic skeleton for the downstream pathway, is converted to naringenin by the catalysis of chalcone isomerase, or it is converted to naringenin chalcone 2’-glucoside by the catalysis of chalcone 2’-glucosyltransferase (Miyahara et al., 2018).
Chalcone is a central intermediate product in different branch pathways, such as the flavanone biosynthesis, flavone biosynthesis, isoflavone biosynthesis, and flavonol biosynthesis (Liu et al., 2021b). In the cytoplasm, chalcone isomerase participates in the cyclization of chalcones to produce flavanones, opening a route to the heterocyclic C-ring-containing flavonoids (Nabavi et al., 2020). In addition, naringenin is the precursor for eriodictyol biosynthesis by flavanone 3′-hydroxylase catalysis, and for pentahydroxyflavanone biosynthesis by flavanone 3′,5′-hydroxylase catalysis (Grotewold, 2006). Flavone biosynthesis is another branch of the flavonoid biosynthetic pathway. Flavone synthase catalyzes the conversion of flavanones to flavones, such as apigenin, dihydroxyflavone, luteolin, and tricetin (Zuk et al., 2019). Flavanones can also be converted to apigenin C-glycosides and luteolin C-glycosides by flavanone-2-hydroxylase (Lam et al., 2019).
Multiple metabolic pathways have the same origin, and the common enzymes are frequently to be the targets of metabolic regulation. The MEP pathway provides common precursors for the TIA biosynthesis and terpenoid biosynthesis pathways. The phenylalanine pathway provided common precursors for the phenolic acid biosynthesis and flavonoid biosynthesis pathways. Manipulation of these common enzymes affects multiple metabolic pathways to response to environmental stresses.
Effects of Environmental Factors on Plant Specialized Metabolites Biosynthesis
The synthesis and tissue-specific accumulation of PSMs are strictly controlled in spatio-temporal mode and affected by various biotic and abiotic factors (Yang et al., 2012). Environmental stresses influence the formation and accumulation of PSMs in plants (Gupta and Dutta, 2011).
Effects of Environmental Factors on Alkaloid Biosynthesis
Over a hundred TIAs, such as bisindole alkaloids, have been detected in the medicinal plant C. roseus (Shukla et al., 2006). Catharanthus roseus seedlings under drought- and salinity-stress conditions exhibit a greatly higher alkaloid content compared with under control conditions (Hassan et al., 2021; Yahyazadeh et al., 2021). Furthermore, the impact of drought and salt stresses on the biosynthesis and accumulation of alkaloids, such as dihydrocoptisine, has also been revealed in Chelidonium majus (Yahyazadeh et al., 2018). Cadmium chloride elicitation increases the yields of reserpine and ajmalicine, two important MIAs, in the endangered medicinal plant Rauvolfia serpentina (Zafar et al., 2020).
Under herbivore attack, the biosynthesis of physostigmine, an approved antiherbivore alkaloid, rapidly increases in the damaged area (Rivero et al., 2021). Various stresses elevate the content of a mixture of toxic pyrrolizidine alkaloids in Echium plantagineum plants, protecting them from insect and livestock herbivory (Skoneczny et al., 2019). Aphid predation induces the biosynthesis of quinolizidine alkaloids, a type of toxic secondary metabolites produced in lupin species (Frick et al., 2019). As a type of PSM, both of biotic and abiotic stresses up-regulate the content of alkaloids, suggesting their important roles of in resistance to environmental stress.
Effects of Environmental Factors on Glucosinolate Biosynthesis
Glucosinolates are important precursors to various active ingredients in the Brassicaceae family of plants (Moreno et al., 2006). In pak choi (Brassica rapa), strong light, high-temperature, and drought increase the accumulation of glucosinolates (Park et al., 2021; Rao et al., 2021). Brassica oleracea has a powerful tolerance to chilling and freezing, and the low temperature-induced content of glucosinolates is hypothesized to be involved in the protective mechanism that enables this tolerance (Ljubej et al., 2021). In addition to low temperature, other postharvest stresses, such as wounding, also induce the biosynthesis of glucosinolates in B. oleracea (Villarreal-Garcia et al., 2016). In Broccoli sprouts, both of UV-A and UV-B light doses affect the tailored glucosinolate and phenolic profiles, suggesting an important role for light stress in glucosinolate biosynthesis (Moreira-Rodriguez et al., 2017).
Biotic stresses also can influence the glucosinolate composition in plants. The aphid-induced expression of CYP79B2, CYP79B3, and PAD33 leads to the accumulation of indolyl glucosinolates (Mewis et al., 2012). A gain-of-function Arabidopsis mutant, cml42, with a higher aliphatic glucosinolate content than the wild type, shows a strong resistance to herbivory (Vadassery et al., 2012).
Effects of Environmental Factors on Terpenoid Biosynthesis
Terpenoids play frequent roles in plant protection in the form of phytohormones, particularly as diterpene gibberellins, triterpene brassinosteroids (BRs), and sesquiterpene abscisic acid (ABA) (Patra et al., 2013; Chen et al., 2021a; Liu et al., 2021a). In lettuce, long-term high temperature expose facilitates the accumulation of gibberellin to accelerate bolting (Liu et al., 2020). Under drought-stress conditions, significant accumulations of ABA occur in wheat guard cells (Wang et al., 2021). In maize sprouts, NaCl stress greatly increases the content of carotenoid, which is a typical tetraterpenoid with an intense antioxidant capacity, by up-regulating the expression of several carotenoid biosynthetic pathway genes (He et al., 2021). In winter wheat, cold treatments elevate the endogenous BR content, indicating a role of triterpene BR in improving the cold tolerance of winter cereals (Janeczko et al., 2019).
Biotic stresses can also influence the terpenoid contents of plants. In various Iranian cultivars of basil, water-deficit stress enhances the accumulations of linalool, germacrene D, and γ-cadinene, three important aromatic terpenes with verified cytotoxic activities (Khakdan et al., 2021). The ability to synthesize specialized antimicrobial avenacins, belonging to the triterpenoids, is likely to have allowed oats (Avena spp.) to combat various diseases (Qi et al., 2004). Terpenoids are rich in Euphorbia peplus latex and function as defensive chemical substances against insect herbivores and various agricultural phytopathogenic fungi (Hua et al., 2017).
A large number of works showed that terpenoids are involved in the resistance to environmental stress in the form of phytohormones. Phytohormones, as signal molecules, transmit environmental signals to plant cells.
Effects of Environmental Factors on Phenolic Acid Biosynthesis
The biosynthesis of phenolic compounds is significantly affected by various abiotic stress conditions (Sharma et al., 2019). In many inbred maize lines, long-term drought treatments cause significant reductions in various phenolic acids, such as protocatechuic, caffeic, and sinapic (Kravic et al., 2021). In Chinese cabbage, salt stress leads to great decreases in phenolic compounds, such as sinapic acid, salicylic acid, and ferulic acid (Linic et al., 2021). Under alkaline conditions, rice enhances phenolic acid secretions in the root epidermis and stele, which effectively increases ion uptake and alleviates the Fe-deficiency responses (Li et al., 2021b). In Achillea pachycephala, drought stress dramatically increases the contents of phenolic acids, such as chlorogenic and caffeic (Gharibi et al., 2019).
Rhizobacteria-mediated systemic resistance helps protect plants from pathogens and insects (Singh et al., 2002). Phenolic acid-induced systemic resistance provides bio-protection to plants under pathogenic stress conditions (Nicholson and Hammerschmidt, 2003). In rice, the correlation between phenolics and seedling protection from Rhizobium solani has been revealed. An High Performance Liquid Chromatography analysis showed that the biosynthesis of phenolic acids is more enhanced in Rhizobium-infected seedlings compared with uninfected controls (Mishra et al., 2006). In the orchid D. officinale, a Dendrobium viroid infection increases the total phenolic acid content, which may play an important role in the activation of pathogen defense responses (Li et al., 2022).
Phenolic acid has complex biological functions. Some stresses inhibit phenolic acid synthesis, and some other stresses promote phenolic acid contents, suggesting that phenolic acids may play both positive and negative roles in the process of resisting environmental stress.
Effects of Environmental Factors on Flavonoid Biosynthesis
Flavonoids, common polyphenols, are antioxidants required in plant stress resistance (Laoue et al., 2022). Plants with high flavonoid contents have potential cellular antioxidant capacities under environmental stress conditions (Hidayat and Wulandari, 2021). The over-accumulation of several flavonoids, such as kaempferol, quercetin, and cyanidin, has been well documented in model plants (Nakabayashi et al., 2014). In Chinese liquorice, the contents of some ortho-dehydroxylated B-ring flavonoids, effective scavengers of ROS, increase under UV-B exposure (Zhang et al., 2018). In rice, salt and heat stresses enhance flavonoid accumulation, which is crucial for stress tolerance (Jan et al., 2021). In some plant species, abiotic stresses play negative roles in flavonoid accumulation. For example, accumulated Na2+ in Apocynum venetum leaves reduces the flavonoid concentration and decreases salt tolerance under salt-stress conditions (Xu et al., 2021).
The protective roles of hesperidin and hesperetin, the major flavonoids in citrus fruit, against invading microbes and toxins have been well investigated (Iranshahi et al., 2015). Several flavonol glycosides, such as quercetin and kaempferol glycosides, increase under short-wavelength radiation, which enhances plant defenses against various herbivorous insects (Rechner et al., 2017).
Anthocyanins, another subgroup of flavonoids, are frequently induced in plants by biotic and abiotic stresses (Li et al., 2021a). Various environmental factors play distinct roles in anthocyanin biosynthesis and tissue-specific accumulation in plants (Li et al., 2012; An et al., 2020). In apple, drought, low temperature, UV-B, and light exposure significantly up-regulate the accumulation of anthocyanins in fruit, and high temperature and increased nitrogen fertilizer significantly down-regulate the accumulation of anthocyanins in fruit (Gao et al., 2021).
Effects of Genetic Factors on the Biosynthesis of Plant Specialized Metabolites
Accumulations of PSMs under stressful environment conditions is controlled by an intricate network containing a large number of Transcription factors (TFs). Many key enzyme-encoding genes involved in PSM biosynthesis are the downstream targets of different TFs (Patra et al., 2013).
Transcription Factors Involved in the Biosynthesis of Alkaloids
Previous studies have identified several TFs that control specific steps and branches of the TIA and BIA biosynthetic pathways. In C. roseus, an ORCA3 TF regulates the expression of TIA biosynthetic pathway-related genes, such as GEISSOSCHIZINE SYNTHASE, STRICTOSIDINE SYNTHASE, and DEACETYLVINDOLINE ACETYLTRANSFERASE (Khataee et al., 2020). The interaction of MYC2 and GBFs governs TIA biosynthesis by modulating the TIA pathway genes in C. roseus (Sui et al., 2018). WRKY1 is a positive regulator of the TIA biosynthetic pathway (Suttipanta et al., 2011). A MAP kinase cascade modulates the TIA biosynthetic pathway by activating its downstream target AP2/ERF TF genes (Paul et al., 2017). In addition, the zinc-finger TF ZCT1 acts as a transcriptional repressor in the TIA biosynthetic pathway (Mortensen et al., 2019). In Ophiorrhiza pumila, OpWRKY2 and OpWRKY3 were identified as two positive regulators in the biosynthesis of camptothecin (Wang et al., 2019a; Hao et al., 2021).
In lotus (Nelumbo nucifera), WRKY40a and WRKY40b participate in the BIA biosynthetic pathway by regulating the TYDC, NCS, CYP80G, and 7OMT genes (Meelaph et al., 2018; Li et al., 2019a). In narrow-leafed lupin, the TF RAP2-7 is involved in the regulation of the quinolizidine alkaloid biosynthetic pathway (Czepiel et al., 2021). Two jasmonate-responsive TFs, ERF189 and ERF199, are involved in the biosynthesis of nicotine, the predominant alkaloid in tobacco leaves (Kato et al., 2014; Kajikawa et al., 2017). Under high temperature-stress conditions, MYC2 enhances the nicotine content by regulating the expression of the PMT1 gene, which encodes a putrescine N-methyl transferase involved in the key step of the pyridine alkaloid pathway (Yang et al., 2016). In Coptis japonica, isoquinoline alkaloid biosynthesis is controlled by CjbHLH1 homologs (Yamada et al., 2011).
Transcription Factors Involved in the Biosynthesis of Glucosinolates
Arabidopsis is a model plant used to reveal the transcriptional regulation of glucosinolate biosynthesis (Hirai et al., 2004). An analysis of the R2R3-MYB family in Arabidopsis showed that MYB34, MYB51, and MYB122 control the biosynthesis of indolic glucosinolates, whereas MYB28, MYB29, and MYB76 control the biosynthesis of aliphatic glucosinolates (Frerigmann and Gigolashvili, 2014; Baskar and Park, 2015). Another two Arabidopsis TFs, FRS7 and FRS12, are transcriptional repressors in the glucosinolate biosynthetic pathway (Fernandez-Calvo et al., 2020). In addition, a well-identified central circadian clock regulator, CCA1, participates in the host resistance of plants to the caterpillar Trichoplusia ni by enhancing basal indole glucosinolate biosynthesis (Lei et al., 2019). A bHLH TF, IAA-LEUCINE RESISTANT3, modulates the accumulation of glucosinolates under iron deficiency conditions and during pathogen infection (Samira et al., 2018). A proteomic analysis identified a jasmonate-responsive MYC2 TF that has opposite effects on the indolic and aliphatic glucosinolate pathways (Guo et al., 2012).
Short-term temperature treatments can enhance the accumulation of glucosinolates in B. rapa. A co-expression analysis identified a MYB family member, MYB51, that regulates the biosynthesis of glucosinolates after a short-term high temperature treatment (Rao et al., 2021). In addition, MYB28.3, MYB29.1, and MYB122.2, which are highly responsive to various abiotic and biotic stresses, are positive regulators of aliphatic glucosinolate biosynthesis in B. rapa (Baskar and Park, 2015; Seo et al., 2017).
Transcription Factors Involved in the Biosynthesis of Terpenoids
A number of TFs are involved in the terpenoid biosynthetic pathway (Patra et al., 2013). Artemisinin is an important sesquiterpene lactone in sweet wormwood, and several artemisinin biosynthesis-related TFs have been identified (Efferth, 2017). In sweet wormwood, two JA responsive TFs, ERF1 and ERF2, affect artemisinin biosynthesis by regulating the expression of AMORPHA-4,11-DIENE SYNTHASE and CYP SEQUITERPENE OXIDASE genes (Yu et al., 2012). AaWRKY1 controls the expression of 3-HYDROXY 3-METHYLGLUTARYL-COA REDUCTASE and ARTEMISINIC ALDEHYDEΔ11(13) REDUCTASE, which are key genes in the artemisinin biosynthetic pathway (Jiang et al., 2016).
Several stress-related TFs are involved in the biosynthesis of terpenoids. In cotton (Gossypium arboretum), GaWRKY1 regulates the conversion of sesquiterpenes to gossypol, which plays a role in responses to fungal infection (Xu et al., 2004). Terpenoids are enriched in the latex products from the rubber tree (Hevea brasiliensis). HbWRKY1 and HbEREBP1 are positive and negative regulators, respectively, of latex biosynthesis induced by wounding (Chen et al., 2012; Wang et al., 2013). Clade Iva bHLH TFs in the JA-signaling pathway participate in the regulation of bioactive terpenoid biosynthesis (Mertens et al., 2016b). In Medicago truncatula, two bHLH TFs, TSAR1 and TSAR2, affect triterpene saponin biosynthesis by regulating the expression of HMGR1, which encodes the rate-limiting enzyme for triterpene biosynthesis, under stress conditions (Mertens et al., 2016a). In roses, the over-expression of the PAP1 TF gene significantly activates the terpenoid biosynthetic pathway to enhance the production of terpenoid scent compounds (Zvi et al., 2012). The JA-responsive TF WRKY24 promotes the biosynthesis of saponin by increasing the expression of terpenoid biosynthetic pathway genes in Conyza blini (Sun et al., 2018). In Taxus media, a phloem-specific MYB3 affects the transcriptional regulation of paclitaxel biosynthesis, a classic diterpenoid compound, by activating the expression of TBT, DBTNBT, and TS genes (Yu et al., 2020).
Transcription Factors Involved in the Biosynthesis of Phenolic Acids
Several TFs act as regulators of the phenolic acid pathway in the Chinese medicinal plant S. miltiorrhiza (Sun et al., 2019a). A large number of TFs, including two ERF family members (SmERF115 and SmERF1L1), three MYB family members (SmMYB2a, SmMYB2b, and SmMYB52), four bHLH family members (SmbHLH3, SmbHLH37, SmbHLH51, and SmbHLH148), one ZIP family member (SmZIP1), and two GRAS family members (SmGRAS1 and SmGRAS2), are involved in the regulation of the phenolic acid biosynthetic pathway (Zhou et al., 2016; Du et al., 2018; Li et al., 2019b; Deng et al., 2020a; Zhang et al., 2020). Furthermore, the corresponding downstream targets of the above TFs also have been identified in S. miltiorrhiza. SmMYC2a/b binds to the E-boxes in the promoter regions of SmHCT6 and SmCYP98A14, which are key genes involved in the synthesis of 4-coumaroyl-3′,4′-dihydroxyphenyllactic acid and rosmarinic acid, respectively (Zhou et al., 2016). SmGRAS1, together with SmGRSA2, binds to the GARE box in the promoter region of SmKSL1, which catalyzes the biosynthesis of tanshinones from GGPP (Li et al., 2019b). SmbZIP1, an ABA-responsive TF, binds to the G-Box-like1 motif in the promoter region of SmC4H1, which is involved in the biosynthesis of phenolic acid precursors (Deng et al., 2020a). SmbHLH148 and SmMYB1 regulate phenolic acid biosynthesis by activating the expression of downstream genes, such as PAL1, C4H1, TAT, HPPR, RAS, and CYP98A14 (Xing et al., 2018; Zhou et al., 2021b). SmMYB52 simultaneously affects the production of phenolic acids by binding to the MBE elements in promoter regions of SmTAT1, Sm4CL9, SmC4H1, and SmHPPR1 (Yang et al., 2021b). Furthermore, SmbHLH3 acts a repressor in the biosynthesis of phenolic acids in S. miltiorrhiza hairy roots by reducing the expression of DXS3, DXR, HMGR1, KSL1, CPS1 and CYP76AH1 (Zhang et al., 2020). In S. miltiorrhiza hairy roots, tanshinone and salvianolic acid biosynthesis are controlled by SmMYB09 (Hao et al., 2020). Interestingly, over-expression of Arabidopsis MYC2 simultaneous promotes the biosynthesis of tanshinone and phenolic acid in S. miltiorrhiza hairy roots (Shi et al., 2020b). Additionally, SmJRB1 was identified as a positive regulator in regulation of phenolic acid biosynthesis (Zhou et al., 2021a).
Transcription Factors Involved in the Biosynthesis of Flavonoids
The complete flavonoid biosynthetic pathway, consisting of three major branches, has been well-studied in plants. Recently, TFs from different families, such as MYB, bHLH, and WRKY, have also been characterized (Lloyd et al., 2017; Yan et al., 2021).
In M. truncatula, MtMYB134 activates flavonol biosynthesis by binding the promoters of MtFLS1, MtFLS2, and MtCHS2 (Naik et al., 2021). In apple, MdNAC52 regulates the biosynthesis of anthocyanin and proanthocyanidin by activating the promoters of MdMYB9 and MdMYB11 genes (Sun et al., 2019b). In buckwheat, MYBF1 regulates the flavonol biosynthetic pathway by up-regulating the DFR and LDOX genes (Matsui et al., 2018). In Fagopyrum tataricum, light-induced FtMYB116 promotes the accumulation of rutin by binding directly to the promoter region of F3’H (Zhang et al., 2019). In pear (Pyrus pyrifolia), PpMYB17 positively controls the flavonoid biosynthetic pathway by activating the promoters of PpCHS, PpCHI, PpF3H, PpFLS, and PpUFGT (Premathilake et al., 2020). Another pear (Pyrus × bretschneideri) TF, PbWRKY75 affects flavonoid biosynthesis by regulating the expression of PbDFR, PbUFGT, and PbMYB10b (Cong et al., 2021). In Populus tomentosa, PtMYB6 promotes anthocyanin and proanthocyanidin biosynthesis by interacting physically with KNAT7 (Wang et al., 2019b). In potato (Solanum tuberosum), StWRKY13 promotes anthocyanin biosynthesis in tubers by activating the promoters of StCHS, StF3H, StDFR, and StANS (Zhang et al., 2021a). The over-expression of MdWRKY11 in apple calli revealed its novel function in promoting the accumulation of flavonoids and anthocyanin by binding to the promoter of MdHY5 (Wang et al., 2018a; Liu et al., 2019).
Furthermore, many negative regulators of the flavonoid biosynthetic pathway have been identified in different plant species. AtMYB4 and its close homologs AtMYB7 and AtMYB32 inhibit flavonoid accumulation by down-regulating the expression of ADT6, which catalyzes the key step that supplies phenylalanine (Wang et al., 2020). The over-expression of MYB15L in red-fleshed apple calli represses anthocyanin accumulation and cold tolerance (Xu et al., 2018). Homodimers of MdMYB16 inhibit anthocyanin synthesis through their C-terminal EARs, which are weakened by interactions with the TF MdbHLH33 (Xu et al., 2017). The over-expression of Arabidopsis MYB60 in lettuce plants significantly reduces the production and accumulation of anthocyanin pigments by inhibiting the expression of DIHYDROFLAVONOL-4-REDUCTASE gene (Park et al., 2008). The loss of MYB2-1 expression causes the purple color in cabbage leaves, suggesting that it encodes a potential negative regulator of the flavonoid biosynthetic pathway (Song et al., 2018). In Ginkgo biloba, a negative regulator, GbMYBF2, affects flavonoid biosynthesis by down-regulating several key genes, such as GbPAL, GbANS, GbFLS, and GbCHS2 (Xu et al., 2014). A Brassica napus WRKY TF, BnWRKY41-1, acts as a repressor of anthocyanin biosynthesis (Duan et al., 2018). In S. miltiorrhiza, SmbHLH60 was identified as a negative regulator in anthocyanin biosynthesis mainly via SmDRF gene (Liu et al., 2022b).
Environmental signals pass through cell membrane through a large number of TFs to activate downstream functional genes. To date, a number of TF involved in metabolic pathway have been identified in different plants. Our review summarizes a network that is involved in the transcriptional regulation of PSM biosynthesis under environmental stresses (Figure 1). To date, a large number of positive TFs have been identified, but the number of negative TFs is still limited. Negative regulatory TFs are also the key factors in the establishment of dynamic balance of plant secondary metabolism. In the future research, cloning and identification of negative regulatory TFs has become an urgent research hotspot.
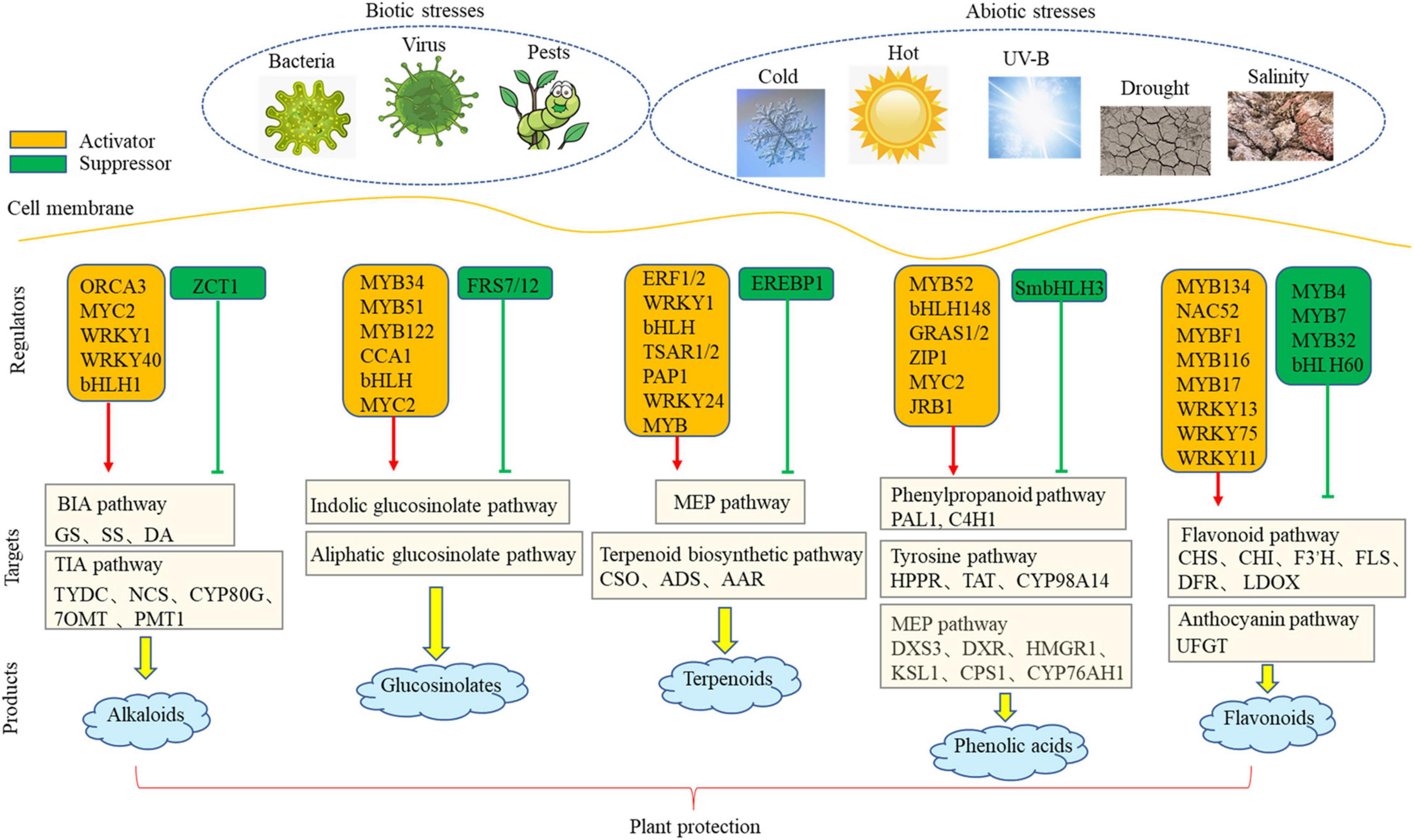
Figure 1. The network of transcriptional regulation of plant under biotic and abiotic stresses. Red arrow indicated activation pathways and green arrow indicated inhibition pathways.
Conclusion and Future Perspectives
Plants produce a large number of PSMs having diversified structures, and they play important physiological and ecological roles in stress tolerance. The biosynthesis of stress-related PSMs is controlled by environmental and genetic factors. Artificial regulation of PSM biosynthesis is helpful to enhance plant resistance to environmental stresses. We summarized potential genetic and environmental factors and their targets, particularly in MYB, bHLH, and WRKY families. For plant genetic improvement, overexpression of activating TFs or inhibition of expression of inhibitory TFs can increase the yield of PSM and enhance the resistance of plants to environmental stress. We further found that the downstream targets of these TFs are frequently enriched in the synthesis pathway of precursors, suggesting an effective role of precursors in enhancing of terminal products. Although most of PSM-related TFs have been identified in different plant species, including the model plant Arabidopsis, medicinal plant C. roseus, and woody plant poplar, these results also provide good guides for the regulation in other plants. This review summarizes the key enzymes and TFs involved in PSM biosynthetic pathways, providing valuable insights for screening targets and regulators in non-model plants.
Author Contributions
RC was involved in the review writing. RC and ZC were involved in manuscript refinement. XZ and CS initiated the idea of the review and were involved in the manuscript writing. All authors contributed to the article and approved the submitted version.
Funding
This work was supported by the National Natural Science Foundation of China (32000255 and 82173919) and the Natural Science Foundation of Zhejiang Province (LY21C050004 and LY19C150005).
Conflict of Interest
The authors declare that the research was conducted in the absence of any commercial or financial relationships that could be construed as a potential conflict of interest.
Publisher’s Note
All claims expressed in this article are solely those of the authors and do not necessarily represent those of their affiliated organizations, or those of the publisher, the editors and the reviewers. Any product that may be evaluated in this article, or claim that may be made by its manufacturer, is not guaranteed or endorsed by the publisher.
References
Abdala-Roberts, L., Rasmann, S., Berny-Mier, Y. T. J. C., Covelo, F., Glauser, G., and Moreira, X. (2016). Biotic and abiotic factors associated with altitudinal variation in plant traits and herbivory in a dominant oak species. Am. J. Bot. 103, 2070–2078. doi: 10.3732/ajb.1600310
Aharoni, A., and Galili, G. (2011). Metabolic engineering of the plant primary-secondary metabolism interface. Curr. Opin. Biotechnol. 22, 239–244. doi: 10.1016/j.copbio.2010.11.004
An, J. P., Liu, Y. J., Zhang, X. W., Bi, S. Q., Wang, X. F., You, C. X., et al. (2020). Dynamic regulation of anthocyanin biosynthesis at different light intensities by the BT2-TCP46-MYB1 module in apple. J. Exp. Bot. 71, 3094–3109. doi: 10.1093/jxb/eraa056
Baskar, V., and Park, S. W. (2015). Molecular characterization of BRMYB28 and BRMYB29 paralogous transcription factors involved in the regulation of aliphatic glucosinolate profiles in Brassica rapa ssp. pekinensis. C. R. Biol. 338, 434–442. doi: 10.1016/j.crvi.2015.04.001
Bhambhani, S., Kondhare, K. R., and Giri, A. P. (2021). Diversity in chemical structures and biological properties of plant alkaloids. Molecules 26:3374. doi: 10.3390/molecules26113374
Bohlmann, J., Meyer-Gauen, G., and Croteau, R. (1998). Plant terpenoid synthases: molecular biology and phylogenetic analysis. Proc. Natl. Acad. Sci. U.S.A. 95, 4126–4133. doi: 10.1073/pnas.95.8.4126
Castro-Moretti, F. R., Gentzel, I. N., Mackey, D., and Alonso, A. P. (2020). Metabolomics as an emerging tool for the study of plant–pathogen interactions. Metabolites 10:52.
Chae, L., Kim, T., Nilo-Poyanco, R., and Rhee, S. Y. (2014). Genomic signatures of specialized metabolism in plants. Science 344, 510–513. doi: 10.1126/science.1252076
Chapman, J. M., Muhlemann, J. K., Gayomba, S. R., and Muday, G. K. (2019). RBOH-dependent ROS synthesis and ROS scavenging by plant specialized metabolites to modulate plant development and stress responses. Chem. Res. Toxicol. 32, 370–396. doi: 10.1021/acs.chemrestox.9b00028
Chen, R., Cao, Y., Wang, W., Li, Y., Wang, D., Wang, S., et al. (2021b). Transcription factor SmSPL7 promotes anthocyanin accumulation and negatively regulates phenolic acid biosynthesis in Salvia miltiorrhiza. Plant Sci. 310, 110993. doi: 10.1016/j.plantsci.2021.110993
Chen, L., Lu, B., Liu, L., Duan, W., Jiang, D., Li, J., et al. (2021a). Melatonin promotes seed germination under salt stress by regulating ABA and GA3 in cotton (Gossypium hirsutum L.). Plant Physiol. Biochem. 162, 506–516. doi: 10.1016/j.plaphy.2021.03.029
Chen, Y. Y., Wang, L. F., Dai, L. J., Yang, S. G., and Tian, W. M. (2012). Characterization of HBEREBP1, a wound-responsive transcription factor gene in laticifers of Hevea brasiliensis muell. Arg. Mol. Biol. Rep. 39, 3713–3719. doi: 10.1007/s11033-011-1146-y
Choi, M., Sathasivam, R., Nguyen, B. V., Park, N. I., Woo, S. H., and Park, S. U. (2021). Expression analysis of phenylpropanoid pathway genes and metabolomic analysis of phenylpropanoid compounds in adventitious, hairy, and seedling roots of tartary buckwheat. Plants 11:90. doi: 10.3390/plants11010090
Christianson, D. W. (2017). Structural and chemical biology of terpenoid cyclases. Chem. Rev. 117, 11570–11648. doi: 10.1021/acs.chemrev.7b00287
Cong, L., Qu, Y., Sha, G., Zhang, S., Ma, Y., Chen, M., et al. (2021). PBWRKY75 promotes anthocyanin synthesis by activating PBDFR, PbUFGT, and PbMYB10b in pear. Physiol. Plant 173, 1841–1849. doi: 10.1111/ppl.13525
Czepiel, K., Krajewski, P., Wilczura, P., Bielecka, P., Swiecicki, W., and Kroc, M. (2021). Expression profiles of alkaloid-related genes across the organs of narrow-leafed lupin (Lupinus angustifolius L.) and in response to anthracnose infection. Int. J. Mol. Sci. 22:2676. doi: 10.3390/ijms22052676
D’Auria, J. C., and Gershenzon, J. (2005). The secondary metabolism of Arabidopsis thaliana: growing like a weed. Curr. Opin. Plant Biol. 8, 308–316. doi: 10.1016/j.pbi.2005.03.012
Deng, C., Wang, Y., Huang, F., Lu, S., Zhao, L., Ma, X., et al. (2020b). SMMYB2 promotes salvianolic acid biosynthesis in the medicinal herb Salvia miltiorrhiza. J. Integr. Plant Biol. 62, 1688–1702. doi: 10.1111/jipb.12943
Deng, C., Shi, M., Fu, R., Zhang, Y., Wang, Q., Zhou, Y., et al. (2020a). ABA-responsive transcription factor bZIP1 is involved in modulating biosynthesis of phenolic acids and tanshinones in Salvia miltiorrhiza. J. Exp. Bot. 71, 5948–5962. doi: 10.1093/jxb/eraa295
Desmet, S., Morreel, K., and Dauwe, R. (2021). Origin and function of structural diversity in the plant specialized metabolome. Plants 10:2393. doi: 10.3390/plants10112393
Dong, N. Q., and Lin, H. X. (2021). Contribution of phenylpropanoid metabolism to plant development and plant-environment interactions. J. Integr. Plant Biol. 63, 180–209. doi: 10.1111/jipb.13054
Du, T., Niu, J., Su, J., Li, S., Guo, X., Li, L., et al. (2018). SmbHLH37 Functions Antagonistically With SMMYC2 in Regulating Jasmonate-mediated biosynthesis of phenolic acids in Salvia miltiorrhiza. Front. Plant Sci. 9:1720. doi: 10.3389/fpls.2018.01720
Duan, S., Wang, J., Gao, C., Jin, C., Li, D., Peng, D., et al. (2018). Functional characterization of a heterologously expressed Brassica napus WRKY41-1 transcription factor in regulating anthocyanin biosynthesis in Arabidopsis thaliana. Plant Sci. 268, 47–53. doi: 10.1016/j.plantsci.2017.12.010
Efferth, T. (2017). From ancient herb to modern drug: Artemisia annua and artemisinin for cancer therapy. Semin. Cancer Biol. 46, 65–83. doi: 10.1016/j.semcancer.2017.02.009
Fabregas, N., and Fernie, A. R. (2021). The interface of central metabolism with hormone signaling in plants. Curr. Biol. 31, R1535–R1548. doi: 10.1016/j.cub.2021.09.070
Fang, C., Fernie, A. R., and Luo, J. (2019). Exploring the diversity of plant metabolism. Trends Plant Sci. 24, 83–98. doi: 10.1016/j.tplants.2018.09.006
Fernandez-Calvo, P., Inigo, S., Glauser, G., Vanden Bossche, R., Tang, M., Li, B., et al. (2020). FRS7 and FRS12 recruit NINJA to regulate expression of glucosinolate biosynthesis genes. New Phytol. 227, 1124–1137. doi: 10.1111/nph.16586
Fernie, A. R., Trethewey, R. N., Krotzky, A. J., and Willmitzer, L. (2004). Metabolite profiling: from diagnostics to systems biology. Nat. Rev. Mol. Cell. Biol. 5, 763–769. doi: 10.1038/nrm1451
Frerigmann, H., and Gigolashvili, T. (2014). Update on the role of R2R3-MYBs in the regulation of glucosinolates upon sulfur deficiency. Front. Plant Sci. 5:626. doi: 10.3389/fpls.2014.00626
Frick, K. M., Foley, R. C., Siddique, K. H. M., Singh, K. B., and Kamphuis, L. G. (2019). The role of jasmonate signalling in quinolizidine alkaloid biosynthesis, wounding and aphid predation response in narrow-leafed lupin. Funct. Plant Biol. 46, 443–454. doi: 10.1071/FP18278
Fu, R., Shi, M., Deng, C., Zhang, Y., Zhang, X., Wang, Y., et al. (2020). Improved phenolic acid content and bioactivities of Salvia miltiorrhiza hairy roots by genetic manipulation of RAS and CYP98A14. Food Chem. 331:127365. doi: 10.1016/j.foodchem.2020.127365
Gao, H. N., Jiang, H., Cui, J. Y., You, C. X., and Li, Y. Y. (2021). Review: The effects of hormones and environmental factors on anthocyanin biosynthesis in apple. Plant Sci. 312:111024. doi: 10.1016/j.plantsci.2021.111024
Geu-Flores, F., Sherden, N. H., Courdavault, V., Burlat, V., Glenn, W. S., Wu, C., et al. (2012). An alternative route to cyclic terpenes by reductive cyclization in iridoid biosynthesis. Nature 492, 138–142. doi: 10.1038/nature11692
Gharibi, S., Sayed Tabatabaei, B. E., Saeidi, G., Talebi, M., and Matkowski, A. (2019). The effect of drought stress on polyphenolic compounds and expression of flavonoid biosynthesis related genes in Achillea pachycephala Rech.f. Phytochemistry 162, 90–98. doi: 10.1016/j.phytochem.2019.03.004
Glas, J. J., Schimmel, B. C., Alba, J. M., Escobar-Bravo, R., Schuurink, R. C., and Kant, M. R. (2012). Plant glandular trichomes as targets for breeding or engineering of resistance to herbivores. Int. J. Mol. Sci. 13, 17077–17103. doi: 10.3390/ijms131217077
Grotewold, E. (2006). The genetics and biochemistry of floral pigments. Annu. Rev. Plant Biol. 57, 761–780. doi: 10.1146/annurev.arplant.57.032905.105248
Guo, J., Pang, Q., Wang, L., Yu, P., Li, N., and Yan, X. (2012). Proteomic identification of MYC2-dependent jasmonate-regulated proteins in Arabidopsis thaliana. Proteome Sci. 10:57. doi: 10.1186/1477-5956-10-57
Gupta, M. L., and Dutta, A. (2011). Stress-mediated adaptive response leading to genetic diversity and instability in metabolite contents of high medicinal value: an overview on Podophyllum hexandrum. OMICS 15, 873–882. doi: 10.1089/omi.2011.0096
Halkier, B. A., and Gershenzon, J. (2006). Biology and biochemistry of glucosinolates. Annu. Rev. Plant Biol. 57, 303–333. doi: 10.1146/annurev.arplant.57.032905.105228
Hamilton, A. (2004). Medicinal plants, conservation and livelihhods. Biodivers. Conserv. 13, 1477–1517.
Hao, X., Pu, Z., Cao, G., You, D., Zhou, Y., Deng, C., et al. (2020). Tanshinone and salvianolic acid biosynthesis are regulated by SmMYB98 in Salvia miltiorrhiza hairy roots. J. Adv. Res. 23, 1–12. doi: 10.1016/j.jare.2020.01.012
Hao, X., Xie, C., Ruan, Q., Zhang, X., Wu, C., Han, B., et al. (2021). The transcription factor OpWRKY2 positively regulates the biosynthesis of the anticancer drug camptothecin in Ophiorrhiza pumila. Hortic. Res. 8:7. doi: 10.1038/s41438-020-00437-3
Hassan, F. A. S., Ali, E., Gaber, A., Fetouh, M. I., and Mazrou, R. (2021). Chitosan nanoparticles effectively combat salinity stress by enhancing antioxidant activity and alkaloid biosynthesis in Catharanthus roseus (L.) G. Don. Plant Physiol. Biochem. 162, 291–300. doi: 10.1016/j.plaphy.2021.03.004
He, S. M., Liang, Y. L., Cong, K., Chen, G., Zhao, X., Zhao, Q. M., et al. (2018). Identification and characterization of genes involved in benzylisoquinoline alkaloid biosynthesis in coptis species. Front. Plant Sci. 9:731. doi: 10.3389/fpls.2018.00731
He, W., Luo, H., Xu, H., Zhou, Z., Li, D., Bao, Y., et al. (2021). Effect of exogenous methyl jasmonate on physiological and carotenoid composition of yellow maize sprouts under NaCl stress. Food Chem. 361:130177. doi: 10.1016/j.foodchem.2021.130177
Henry, L. K., Gutensohn, M., Thomas, S. T., Noel, J. P., and Dudareva, N. (2015). Orthologs of the archaeal isopentenyl phosphate kinase regulate terpenoid production in plants. Proc Natl Acad. Sci. U.S.A. 112, 10050–10055. doi: 10.1073/pnas.1504798112
Hidayat, R., and Wulandari, P. (2021). Effects of Andrographis paniculata (Burm. F.). Rep. Biochem. Mol. Biol. 10, 445–454. doi: 10.52547/rbmb.10.3.445
Hirai, M. Y., Yano, M., Goodenowe, D. B., Kanaya, S., Kimura, T., Awazuhara, M., et al. (2004). Integration of transcriptomics and metabolomics for understanding of global responses to nutritional stresses in Arabidopsis thaliana. Proc. Natl. Acad. Sci. U.S.A. 101, 10205–10210. doi: 10.1073/pnas.0403218101
Hori, K., Yamada, Y., Purwanto, R., Minakuchi, Y., Toyoda, A., Hirakawa, H., et al. (2018). Mining of the uncharacterized cytochrome P450 genes involved in alkaloid biosynthesis in california poppy using a draft genome sequence. Plant Cell Physiol. 59, 222–233. doi: 10.1093/pcp/pcx210
Hua, J., Liu, Y., Xiao, C. J., Jing, S. X., Luo, S. H., and Li, S. H. (2017). Chemical profile and defensive function of the latex of Euphorbia peplus. Phytochemistry 136, 56–64. doi: 10.1016/j.phytochem.2016.12.021
Huang, B., Yi, B., Duan, Y., Sun, L., Yu, X., Guo, J., et al. (2008). Characterization and expression profiling of tyrosine aminotransferase gene from Salvia miltiorrhiza (Dan-shen) in rosmarinic acid biosynthesis pathway. Mol. Biol. Rep. 35, 601–612. doi: 10.1007/s11033-007-9130-2
Iranshahi, M., Rezaee, R., Parhiz, H., Roohbakhsh, A., and Soltani, F. (2015). Protective effects of flavonoids against microbes and toxins: the cases of hesperidin and hesperetin. Life Sci. 137, 125–132. doi: 10.1016/j.lfs.2015.07.014
Ishida, M., Hara, M., Fukino, N., Kakizaki, T., and Morimitsu, Y. (2014). Glucosinolate metabolism, functionality and breeding for the improvement of Brassicaceae vegetables. Breed Sci. 64, 48–59. doi: 10.1270/jsbbs.64.48
Jan, R., Kim, N., Lee, S. H., Khan, M. A., Asaf, S., and Lubna, et al. (2021). Enhanced flavonoid accumulation reduces combined salt and heat stress through regulation of transcriptional and hormonal mechanisms. Front. Plant Sci. 12:796956. doi: 10.3389/fpls.2021.796956
Janeczko, A., Pociecha, E., Dziurka, M., Jurczyk, B., Libik-Konieczny, M., Oklestkova, J., et al. (2019). Changes in content of steroid regulators during cold hardening of winter wheat - Steroid physiological/biochemical activity and impact on frost tolerance. Plant Physiol. Biochem. 139, 215–228. doi: 10.1016/j.plaphy.2019.03.020
Jeena, G. S., Kumar, S., and Shukla, R. K. (2021). Characterization of MYB35 regulated methyl jasmonate and wound responsive geraniol 10-hydroxylase-1 gene from Bacopa monnieric. Planta 253:89. doi: 10.1007/s00425-021-03614-3
Jia, Q., and Chen, F. (2016). Catalytic functions of the isoprenyl diphosphate synthase superfamily in plants: a growing repertoire. Mol. Plant 9, 189–191. doi: 10.1016/j.molp.2015.12.020
Jiang, W., Fu, X., Pan, Q., Tang, Y., Shen, Q., Lv, Z., et al. (2016). Overexpression of AaWRKY1 leads to an enhanced content of artemisinin in Artemisia annua. Biomed. Res. Int. 2016:7314971. doi: 10.1155/2016/7314971
Kajikawa, M., Sierro, N., Kawaguchi, H., Bakaher, N., Ivanov, N. V., Hashimoto, T., et al. (2017). Genomic insights into the evolution of the nicotine biosynthesis pathway in tobacco. Plant Physiol. 174, 999–1011. doi: 10.1104/pp.17.00070
Kato, K., Shoji, T., and Hashimoto, T. (2014). Tobacco nicotine uptake permease regulates the expression of a key transcription factor gene in the nicotine biosynthesis pathway. Plant Physiol. 166, 2195–2204. doi: 10.1104/pp.114.251645
Khakdan, F., Govahi, M., Mohebi, Z., and Ranjbar, M. (2021). Water deficit stress responses of monoterpenes and sesquiterpenes in different Iranian cultivars of basil. Physiol. Plant 173, 896–910. doi: 10.1111/ppl.13485
Khataee, E., Karimi, F., and Razavi, K. (2020). Different carbon sources and their concentrations change alkaloid production and gene expression in Catharanthus roseus shoots in vitro. Funct. Plant Biol. 48, 40–53. doi: 10.1071/FP19254
Kochevenko, A., Klee, H. J., Fernie, A. R., and Araújo, W. L. (2012). Molecular identification of a further branched-chain aminotransferase 7 (BCAT7) in tomato plants. J. Plant Physiol. 169, 437–443. doi: 10.1016/j.jplph.2011.12.002
Kravic, N., Babic, V., Vukadinovic, J., Ristic, D., Dragicevic, V., Mladenovic Drinic, S., et al. (2021). Alteration of metabolites accumulation in maize inbreds leaf tissue under long-term water deficit. Biology 10:694. doi: 10.3390/biology10080694
Kumar, K., Kumar, S. R., Dwivedi, V., Rai, A., Shukla, A. K., Shanker, K., et al. (2015). Precursor feeding studies and molecular characterization of geraniol synthase establish the limiting role of geraniol in monoterpene indole alkaloid biosynthesis in Catharanthus roseus leaves. Plant Sci. 239, 56–66. doi: 10.1016/j.plantsci.2015.07.007
Kumar, S., and Pandey, A. K. (2013). Chemistry and biological activities of flavonoids: an overview. ScientificWorldJournal 2013:162750. doi: 10.1155/2013/162750
Lam, P. Y., Lui, A. C. W., Yamamura, M., Wang, L., Takeda, Y., Suzuki, S., et al. (2019). Recruitment of specific flavonoid B-ring hydroxylases for two independent biosynthesis pathways of flavone-derived metabolites in grasses. New Phytol 223, 204–219. doi: 10.1111/nph.15795
Laoue, J., Fernandez, C., and Ormeno, E. (2022). Plant flavonoids in mediterranean species: a focus on flavonols as protective metabolites under climate stress. Plants 11:172. doi: 10.3390/plants11020172
Lei, J., Jayaprakasha, G. K., Singh, J., Uckoo, R., Borrego, E. J., Finlayson, S., et al. (2019). CIRCADIAN CLOCK-ASSOCIATED1 controls resistance to aphids by altering indole glucosinolate production. Plant Physiol. 181, 1344–1359. doi: 10.1104/pp.19.00676
Li, C., Shi, L., Li, X., Wang, Y., Bi, Y., Li, W., et al. (2021a). ECAP is a key negative regulator mediating different pathways to modulate salt stress-induced anthocyanin biosynthesis in Arabidopsis. New Phytol. 233, 2216–2231. doi: 10.1111/nph.17937
Li, H., Chen, H., Deng, S., Cai, H., Shi, L., Xu, F., et al. (2021b). Inhibition of nitric oxide production under alkaline conditions regulates iron homeostasis in rice. Physiol. Plant 172, 1465–1476. doi: 10.1111/ppl.13333
Li, S., Wu, Z. G., Zhou, Y., Dong, Z. F., Fei, X., Zhou, C. Y., et al. (2022). Changes in metabolism modulate induced by viroid infection in the orchid Dendrobium officinale. Virus Res. 308:198626. doi: 10.1016/j.virusres.2021.198626
Li, W., Bai, Z., Pei, T., Yang, D., Mao, R., Zhang, B., et al. (2019b). SmGRAS1 and SmGRAS2 regulate the biosynthesis of tanshinones and phenolic acids in Salvia miltiorrhiza. Front. Plant Sci. 10:1367. doi: 10.3389/fpls.2019.01367
Li, J., Xiong, Y., Li, Y., Ye, S., Yin, Q., Gao, S., et al. (2019a). Comprehensive Analysis and Functional Studies of WRKY Transcription Factors in Nelumbo nucifera. Int J Mol Sci 20, 5006. doi: 10.3390/ijms20205006
Li, Y. Y., Mao, K., Zhao, C., Zhao, X. Y., Zhang, H. L., Shu, H. R., et al. (2012). MdCOP1 ubiquitin E3 ligases interact with MdMYB1 to regulate light-induced anthocyanin biosynthesis and red fruit coloration in apple. Plant Physiol. 160, 1011–1022. doi: 10.1104/pp.112.199703
Lichman, B. R. (2021). The scaffold-forming steps of plant alkaloid biosynthesis. Nat. Prod. Rep. 38, 103–129. doi: 10.1039/d0np00031k
Linic, I., Mlinaric, S., Brkljacic, L., Pavlovic, I., Smolko, A., and Salopek-Sondi, B. (2021). Ferulic acid and salicylic acid foliar treatments reduce short-term salt stress in chinese cabbage by increasing phenolic compounds accumulation and photosynthetic performance. Plants 10:2346. doi: 10.3390/plants10112346
Liu, Y., Watanabe, M., Yasukawa, S., Kawamura, Y., Aneklaphakij, C., Fernie, A. R., et al. (2021d). Cross-species metabolic profiling of floral specialized metabolism facilitates understanding of evolutional aspects of metabolism among Brassicaceae species. Front. Plant Sci. 12:640141. doi: 10.3389/fpls.2021.640141
Liu, X., Bu, J., Ma, Y., Chen, Y., Li, Q., Jiao, X., et al. (2021c). Functional characterization of (S)-N-methylcoclaurine 3′-hydroxylase (NMCH) involved in the biosynthesis of benzylisoquinoline alkaloids in Corydalis yanhusuo. Plant Physiol. Biochem. 168, 507–515. doi: 10.1016/j.plaphy.2021.09.042
Liu, W., Feng, Y., Yu, S., Fan, Z., Li, X., Li, J., et al. (2021b). The flavonoid biosynthesis network in plants. Int. J. Mol. Sci. 22:12824. doi: 10.3390/ijms222312824
Liu, C., Wang, H., Zhang, X., Ma, F., Guo, T., and Li, C. (2021a). Activation of the ABA signal pathway mediated by GABA improves the drought resistance of apple seedlings. Int. J. Mol. Sci. 22:12676. doi: 10.3390/ijms222312676
Liu, C. L., Xue, K., Yang, Y., Liu, X., Li, Y., Lee, T. S., et al. (2022a). Metabolic engineering strategies for sesquiterpene production in microorganism. Crit. Rev. Biotechnol. 42, 73–92. doi: 10.1080/07388551.2021.1924112
Liu, R., Su, Z., Zhou, H., Huang, Q., Fan, S., Liu, C., et al. (2020). LsHSP70 is induced by high temperature to interact with calmodulin, leading to higher bolting resistance in lettuce. Sci. Rep. 10:15155. doi: 10.1038/s41598-020-72443-3
Liu, S., Wang, Y., Shi, M., Maoz, I., Gao, X., Sun, M., et al. (2022b). SmbHLH60 and SmMYC2 antagonistically regulate phenolic acids and anthocyanins biosynthesis in Salvia miltiorrhiza. J. Adv. Res. doi: 10.1016/j.jare.2022.02.005
Liu, W., Wang, Y., Yu, L., Jiang, H., Guo, Z., Xu, H., et al. (2019). MdWRKY11 participates in anthocyanin accumulation in red-fleshed apples by affecting MYB transcription factors and the photoresponse factor MdHY5. J. Agric. Food Chem. 67, 8783–8793. doi: 10.1021/acs.jafc.9b02920
Ljubej, V., Radojcic Redovnikovic, I., Salopek-Sondi, B., Smolko, A., Roje, S., and Samec, D. (2021). Chilling and freezing temperature stress differently influence glucosinolates content in Brassica oleracea var. acephala. Plants 10:1305. doi: 10.3390/plants10071305
Lloyd, A., Brockman, A., Aguirre, L., Campbell, A., Bean, A., Cantero, A., et al. (2017). Advances in the MYB-bHLH-WD Repeat (MBW) pigment regulatory model: addition of a WRKY factor and co-option of an anthocyanin MYB for betalain regulation. Plant Cell Physiol. 58, 1431–1441. doi: 10.1093/pcp/pcx075
Luijendijk, T. J., van der Meijden, E., and Verpoorte, R. (1996). Involvement of strictosidine as a defensive chemical in Catharanthus roseus. J. Chem. Ecol. 22, 1355–1366. doi: 10.1007/BF02027718
Maag, D., Erb, M., Kollner, T. G., and Gershenzon, J. (2015). Defensive weapons and defense signals in plants: some metabolites serve both roles. Bioessays 37, 167–174. doi: 10.1002/bies.201400124
Mahmoud, S. S., Maddock, S., and Adal, A. M. (2021). Isoprenoid metabolism and engineering in glandular trichomes of Lamiaceae. Front. Plant Sci. 12:699157. doi: 10.3389/fpls.2021.699157
Marone, D., Mastrangelo, A. M., Borrelli, G. M., Mores, A., Laido, G., Russo, M. A., et al. (2022). Specialized metabolites: physiological and biochemical role in stress resistance, strategies to improve their accumulation, and new applications in crop breeding and management. Plant Physiol. Biochem. 172, 48–55. doi: 10.1016/j.plaphy.2021.12.037
Matsui, K., Oshima, Y., Mitsuda, N., Sakamoto, S., Nishiba, Y., Walker, A. R., et al. (2018). Buckwheat R2R3 MYB transcription factor FeMYBF1 regulates flavonol biosynthesis. Plant Sci. 274, 466–475. doi: 10.1016/j.plantsci.2018.06.025
Meelaph, T., Kobtrakul, K., Chansilpa, N. N., Han, Y., Rani, D., De-Eknamkul, W., et al. (2018). Coregulation of biosynthetic genes and transcription factors for aporphine-type alkaloid production in wounded lotus provides insight into the biosynthetic pathway of nuciferine. ACS Omega 3, 8794–8802. doi: 10.1021/acsomega.8b00827
Menendez-Perdomo, I. M., and Facchini, P. J. (2018). Benzylisoquinoline alkaloids biosynthesis in sacred lotus. Molecules 23:2899. doi: 10.3390/molecules23112899
Mertens, J., Van Moerkercke, A., Vanden Bossche, R., Pollier, J., and Goossens, A. (2016b). Clade IVa basic helix-loop-helix transcription factors form part of a conserved jasmonate signaling circuit for the regulation of bioactive plant terpenoid biosynthesis. Plant Cell Physiol. 57, 2564–2575. doi: 10.1093/pcp/pcw168
Mertens, J., Pollier, J., Vanden Bossche, R., Lopez-Vidriero, I., Franco-Zorrilla, J. M., and Goossens, A. (2016a). The bHLH transcription factors TSAR1 and TSAR2 regulate Triterpene saponin biosynthesis in Medicago truncatula. Plant Physiol. 170, 194–210. doi: 10.1104/pp.15.01645
Mewis, I., Khan, M. A., Glawischnig, E., Schreiner, M., and Ulrichs, C. (2012). Water stress and aphid feeding differentially influence metabolite composition in Arabidopsis thaliana (L.). PLoS One 7:e48661. doi: 10.1371/journal.pone.0048661
Mishra, R. P., Singh, R. K., Jaiswal, H. K., Kumar, V., and Maurya, S. (2006). Rhizobium-mediated induction of phenolics and plant growth promotion in rice (Oryza sativa L.). Curr. Microbiol. 52, 383–389. doi: 10.1007/s00284-005-0296-3
Miyahara, T., Sugishita, N., Ishida-Dei, M., Okamoto, E., Kouno, T., Cano, E. A., et al. (2018). Carnation I locus contains two chalcone isomerase genes involved in orange flower coloration. Breed Sci. 68, 481–487. doi: 10.1270/jsbbs.18029
Moreira-Rodriguez, M., Nair, V., Benavides, J., Cisneros-Zevallos, L., and Jacobo-Velazquez, D. A. (2017). UVA, UVB light doses and harvesting time differentially tailor glucosinolate and phenolic profiles in broccoli sprouts. Molecules 22:1065. doi: 10.3390/molecules22071065
Moreno, D. A., Carvajal, M., Lopez-Berenguer, C., and Garcia-Viguera, C. (2006). Chemical and biological characterisation of nutraceutical compounds of broccoli. J. Pharm. Biomed. Anal. 41, 1508–1522. doi: 10.1016/j.jpba.2006.04.003
Moreno, P. R., Schlatmann, J. E., Van der Heijden, R., van Gulik, W. M., Ten Hoopen, H. J., Verpoorte, R., et al. (1993). Induction of ajmalicine formation and related enzyme activities in Catharanthus roseus cells: effect of inoculum density. Appl. Microbiol. Biotechnol. 39, 42–47. doi: 10.1007/BF00166846
Mortensen, S., Weaver, J. D., Sathitloetsakun, S., Cole, L. F., Rizvi, N. F., Cram, E. J., et al. (2019). The regulation of ZCT1, a transcriptional repressor of monoterpenoid indole alkaloid biosynthetic genes in Catharanthus roseus. Plant Direct 3:e00193. doi: 10.1002/pld3.193
Nabavi, S. M., Samec, D., Tomczyk, M., Milella, L., Russo, D., Habtemariam, S., et al. (2020). Flavonoid biosynthetic pathways in plants: versatile targets for metabolic engineering. Biotechnol. Adv. 38:107316. doi: 10.1016/j.biotechadv.2018.11.005
Naik, J., Rajput, R., Pucker, B., Stracke, R., and Pandey, A. (2021). The R2R3-MYB transcription factor MtMYB134 orchestrates flavonol biosynthesis in Medicago truncatula. Plant Mol. Biol. 106, 157–172. doi: 10.1007/s11103-021-01135-x
Nakabayashi, R., Mori, T., and Saito, K. (2014). Alternation of flavonoid accumulation under drought stress in Arabidopsis thaliana. Plant Signal Behav. 9:e29518. doi: 10.4161/psb.29518
Nguyen, V. P. T., Stewart, J., Lopez, M., Ioannou, I., and Allais, F. (2020). Glucosinolates: natural occurrence, biosynthesis, accessibility, isolation, structures, and biological activities. Molecules 25:4537. doi: 10.3390/molecules25194537
Nicholson, R. L., and Hammerschmidt, R. E. (2003). Phenolic compounds and their role in disease resistance. Ann. Rev. Phytopathol. 30, 369–389.
Noda, N., Yoshioka, S., Kishimoto, S., Nakayama, M., Douzono, M., Tanaka, Y., et al. (2017). Generation of blue chrysanthemums by anthocyanin B-ring hydroxylation and glucosylation and its coloration mechanism. Sci. Adv. 3:e1602785. doi: 10.1126/sciadv.1602785
O’Connor, S. E., and Maresh, J. J. (2006). Chemistry and biology of monoterpene indole alkaloid biosynthesis. Nat. Prod. Rep. 23, 532–547. doi: 10.1039/b512615k
Pacifico, D., Lanzanova, C., Pagnotta, E., Bassolino, L., Mastrangelo, A. M., Marone, D., et al. (2021). sustainable use of bioactive compounds from solanum tuberosum and Brassicaceae wastes and by-products for crop protection-a review. Molecules 26:2174. doi: 10.3390/molecules26082174
Park, J. E., Kim, J., Purevdorj, E., Son, Y. J., Nho, C. W., and Yoo, G. (2021). Effects of long light exposure and drought stress on plant growth and glucosinolate production in pak choi (Brassica rapa subsp. Chinensis). Food Chem. 340:128167. doi: 10.1016/j.foodchem.2020.128167
Park, J. S., Kim, J. B., Cho, K. J., Cheon, C. I., Sung, M. K., Choung, M. G., et al. (2008). Arabidopsis R2R3-MYB transcription factor AtMYB60 functions as a transcriptional repressor of anthocyanin biosynthesis in lettuce (Lactuca sativa). Plant Cell Rep. 27, 985–994. doi: 10.1007/s00299-008-0521-1
Patra, B., Schluttenhofer, C., Wu, Y., Pattanaik, S., and Yuan, L. (2013). Transcriptional regulation of secondary metabolite biosynthesis in plants. Biochim. Biophys. Acta 1829, 1236–1247. doi: 10.1016/j.bbagrm.2013.09.006
Paul, P., Singh, S. K., Patra, B., Sui, X., Pattanaik, S., and Yuan, L. (2017). A differentially regulated AP2/ERF transcription factor gene cluster acts downstream of a MAP kinase cascade to modulate terpenoid indole alkaloid biosynthesis in Catharanthus roseus. New Phytol. 213, 1107–1123. doi: 10.1111/nph.14252
Pichersky, E., and Raguso, R. A. (2018). Why do plants produce so many terpenoid compounds? New Phytol. 220, 692–702. doi: 10.1111/nph.14178
Premathilake, A. T., Ni, J., Bai, S., Tao, R., Ahmad, M., and Teng, Y. (2020). R2R3-MYB transcription factor PpMYB17 positively regulates flavonoid biosynthesis in pear fruit. Planta 252:59. doi: 10.1007/s00425-020-03473-4
Qi, X., Bakht, S., Leggett, M., Maxwell, C., Melton, R., and Osbourn, A. (2004). A gene cluster for secondary metabolism in oat: implications for the evolution of metabolic diversity in plants. Proc. Natl. Acad. Sci. U.S.A. 101, 8233–8238. doi: 10.1073/pnas.0401301101
Qu, Y., Thamm, A. M. K., Czerwinski, M., Masada, S., Kim, K. H., Jones, G., et al. (2018). Geissoschizine synthase controls flux in the formation of monoterpenoid indole alkaloids in a Catharanthus roseus mutant. Planta 247, 625–634. doi: 10.1007/s00425-017-2812-7
Rai, A., Smita, S. S., Singh, A. K., Shanker, K., and Nagegowda, D. A. (2013). Heteromeric and homomeric geranyl diphosphate synthases from Catharanthus roseus and their role in monoterpene indole alkaloid biosynthesis. Mol. Plant 6, 1531–1549. doi: 10.1093/mp/sst058
Rao, S. Q., Chen, X. Q., Wang, K. H., Zhu, Z. J., Yang, J., and Zhu, B. (2021). Effect of short-term high temperature on the accumulation of glucosinolates in Brassica rapa. Plant Physiol. Biochem. 161, 222–233. doi: 10.1016/j.plaphy.2021.02.013
Rasouli, H., Farzaei, M. H., Mansouri, K., Mohammadzadeh, S., and Khodarahmi, R. (2016). Plant cell cancer: may natural phenolic compounds prevent onset and development of plant cell malignancy? A literature review. Molecules 21:1104. doi: 10.3390/molecules21091104
Ravishankara, M. N., Shrivastava, N., Padh, H., and Rajani, M. (2001). HPTLC method for the estimation of alkaloids of Cinchona officinalis stem bark and its marketed formulations. Planta Med. 67, 294–296. doi: 10.1055/s-2001-11995
Rechner, O., Neugart, S., Schreiner, M., Wu, S., and Poehling, H. M. (2017). Can narrow-bandwidth light from UV-A to green alter secondary plant metabolism and increase Brassica plant defenses against aphids? PLoS One 12:e0188522. doi: 10.1371/journal.pone.0188522
Reyes Jara, A. M., Gomez Lobato, M. E., Civello, P. M., and Martinez, G. A. (2022). Phenylalanine ammonia lyase is more relevant than Chalcone synthase and Chalcone isomerase in the biosynthesis of flavonoids during postharvest senescence of broccoli. J. Food Biochem. 46:e14054. doi: 10.1111/jfbc.14054
Rivero, J., Lidoy, J., Llopis-Gimenez, A., Herrero, S., Flors, V., and Pozo, M. J. (2021). Mycorrhizal symbiosis primes the accumulation of antiherbivore compounds and enhances herbivore mortality in tomato. J. Exp. Bot. 72, 5038–5050. doi: 10.1093/jxb/erab171
Rizi, M. R., Azizi, A., Sayyari, M., Mirzaie-Asl, A., and Conti, L. (2021). Increased phenylpropanoids production in UV-B irradiated Salvia verticillata as a consequence of altered genes expression in young leaves. Plant Physiol. Biochem. 167, 174–184. doi: 10.1016/j.plaphy.2021.07.037
Robin, A. H., Yi, G. E., Laila, R., Yang, K., Park, J. I., Kim, H. R., et al. (2016). Expression Profiling of glucosinolate biosynthetic genes in Brassica oleracea L. var. Capitata inbred lines reveals their association with glucosinolate content. Molecules 21:787. doi: 10.3390/molecules21060787
Samira, R., Li, B., Kliebenstein, D., Li, C., Davis, E., Gillikin, J. W., et al. (2018). The bHLH transcription factor ILR3 modulates multiple stress responses in Arabidopsis. Plant Mol Biol 97, 297–309. doi: 10.1007/s11103-018-0735-8
Sanchez-Pujante, P. J., Borja-Martinez, M., Pedreno, M. A., and Almagro, L. (2017). Biosynthesis and bioactivity of glucosinolates and their production in plant in vitro cultures. Planta 246, 19–32. doi: 10.1007/s00425-017-2705-9
Sandholu, A. S., Mujawar, S. P., Ramakrishnan, K., Thulasiram, H. V., and Kulkarni, K. (2020). Structural studies on 10-hydroxygeraniol dehydrogenase: a novel linear substrate-specific dehydrogenase from Catharanthus roseus. Proteins 88, 1197–1206. doi: 10.1002/prot.25891
Schlager, S., and Drager, B. (2016). Exploiting plant alkaloids. Curr. Opin Biotechnol. 37, 155–164. doi: 10.1016/j.copbio.2015.12.003
Seo, M. S., Jin, M., Sohn, S. H., and Kim, J. S. (2017). Expression profiles of BrMYB transcription factors related to glucosinolate biosynthesis and stress response in eight subspecies of Brassica rapa. FEBS Open Biol. 7, 1646–1659. doi: 10.1002/2211-5463.12231
Sharma, A., Shahzad, B., Rehman, A., Bhardwaj, R., Landi, M., and Zheng, B. (2019). Response of phenylpropanoid pathway and the role of polyphenols in plants under abiotic stress. Molecules 24:2452. doi: 10.3390/molecules24132452
Shen, C., Guo, H., Chen, H., Shi, Y., Meng, Y., Lu, J., et al. (2017). Identification and analysis of genes associated with the synthesis of bioactive constituents in Dendrobium officinale using RNA-Seq. Sci. Rep. 7:187. doi: 10.1038/s41598-017-00292-8
Sheng, X., and Himo, F. (2019). Enzymatic pictet-spengler reaction: computational study of the mechanism and enantioselectivity of norcoclaurine synthase. J. Am. Chem. Soc. 141, 11230–11238. doi: 10.1021/jacs.9b04591
Shi, M., Gong, H., Cui, L., Wang, Q., Wang, C., Wang, Y., et al. (2020a). Targeted metabolic engineering of committed steps improves anti-cancer drug camptothecin production in Ophiorrhiza pumila hairy roots. Ind. Crops Prod. 148:112277. doi: 10.1016/j.indcrop.2020.112277
Shi, M., Huang, F., Deng, C., Wang, Y., and Kai, G. (2019). Bioactivities, biosynthesis and biotechnological production of phenolic acids in Salvia miltiorrhiza. Crit. Rev. Food Sci. Nutr. 59, 953–964. doi: 10.1080/10408398.2018.1474170
Shi, M., Liao, P., Nile, S. H., Georgiev, M. I., and Kai, G. (2021). Biotechnological exploration of transformed root culture for value-added products. Trends Biotechnol. 39, 137–149. doi: 10.1016/j.tibtech.2020.06.012
Shi, M., Wang, Y., Wang, X., Deng, C., Cao, W., Hua, Q., et al. (2020b). Simultaneous promotion of tanshinone and phenolic acid biosynthesis in Salvia miltiorrhiza hairy roots by overexpressing Arabidopsis MYC2. Ind. Crops Prod. 155:112826. doi: 10.1016/j.indcrop.2020.112826
Shukla, A. K., Shasany, A. K., Gupta, M. M., and Khanuja, S. P. (2006). Transcriptome analysis in Catharanthus roseus leaves and roots for comparative terpenoid indole alkaloid profiles. J. Exp. Bot. 57, 3921–3932. doi: 10.1093/jxb/erl146
Sic Zlabur, J., Radman, S., Fabek Uher, S., Opacic, N., Benko, B., Galic, A., et al. (2021). Plant response to mechanically-induced stress: a case study on specialized metabolites of leafy vegetables. Plants 10:2650. doi: 10.3390/plants10122650
Simkin, A. J., Miettinen, K., Claudel, P., Burlat, V., Guirimand, G., Courdavault, V., et al. (2013). Characterization of the plastidial geraniol synthase from Madagascar periwinkle which initiates the monoterpenoid branch of the alkaloid pathway in internal phloem associated parenchyma. Phytochemistry 85, 36–43. doi: 10.1016/j.phytochem.2012.09.014
Singh, P., Arif, Y., Bajguz, A., and Hayat, S. (2021). The role of quercetin in plants. Plant Physiol. Biochem. 166, 10–19. doi: 10.1016/j.plaphy.2021.05.023
Singh, U. P., Sarma, B. K., Singh, D. P., and Bahadur, A. (2002). Plant growth-promoting rhizobacteria-mediated induction of phenolics in pea (Pisum sativum) after infection with Erysiphe pisi. Curr. Microbiol. 44, 396–400. doi: 10.1007/s00284-001-0007-7
Skoneczny, D., Zhu, X., Weston, P. A., Gurr, G. M., Callaway, R. M., and Weston, L. A. (2019). Production of pyrrolizidine alkaloids and shikonins in Echium plantagineum L. in response to various plant stressors. Pest Manag. Sci. 75, 2530–2541. doi: 10.1002/ps.5540
Sonderby, I. E., Geu-Flores, F., and Halkier, B. A. (2010). Biosynthesis of glucosinolates–gene discovery and beyond. Trends Plant Sci. 15, 283–290. doi: 10.1016/j.tplants.2010.02.005
Song, H., Yi, H., Lee, M., Han, C. T., Lee, J., Kim, H., et al. (2018). Purple Brassica oleracea var. capitata F. rubra is due to the loss of BoMYBL2-1 expression. BMC Plant Biol. 18:82. doi: 10.1186/s12870-018-1290-9
Sotelo, T., Velasco, P., Soengas, P., Rodriguez, V. M., and Cartea, M. E. (2016). Modification of leaf glucosinolate contents in brassica oleracea by divergent selection and effect on expression of genes controlling glucosinolate pathway. Front. Plant Sci. 7:1012. doi: 10.3389/fpls.2016.01012
Sui, X., Singh, S. K., Patra, B., Schluttenhofer, C., Guo, W., Pattanaik, S., et al. (2018). Cross-family transcription factor interaction between MYC2 and GBFs modulates terpenoid indole alkaloid biosynthesis. J. Exp. Bot. 69, 4267–4281. doi: 10.1093/jxb/ery229
Sun, M., Shi, M., Wang, Y., Huang, Q., Yuan, T., Wang, Q., et al. (2019a). The biosynthesis of phenolic acids is positively regulated by the JA-responsive transcription factor ERF115 in Salvia miltiorrhiza. J. Exp. Bot. 70, 243–254. doi: 10.1093/jxb/ery349
Sun, Q., Jiang, S., Zhang, T., Xu, H., Fang, H., Zhang, J., et al. (2019b). Apple NAC transcription factor MdNAC52 regulates biosynthesis of anthocyanin and proanthocyanidin through MdMYB9 and MdMYB11. Plant Sci. 289:110286. doi: 10.1016/j.plantsci.2019.110286
Sun, W. J., Zhan, J. Y., Zheng, T. R., Sun, R., Wang, T., Tang, Z. Z., et al. (2018). The jasmonate-responsive transcription factor CbWRKY24 regulates terpenoid biosynthetic genes to promote saponin biosynthesis in Conyza blinii H. Lev. J. Genet 97, 1379–1388.
Suttipanta, N., Pattanaik, S., Kulshrestha, M., Patra, B., Singh, S. K., and Yuan, L. (2011). The transcription factor CrWRKY1 positively regulates the terpenoid indole alkaloid biosynthesis in Catharanthus roseus. Plant Physiol. 157, 2081–2093. doi: 10.1104/pp.111.181834
Tholl, D. (2015). Biosynthesis and biological functions of terpenoids in plants. Adv. Biochem. Eng. Biotechnol. 148, 63–106. doi: 10.1007/10_2014_295
Tungmunnithum, D., Thongboonyou, A., Pholboon, A., and Yangsabai, A. (2018). Flavonoids and other phenolic compounds from medicinal plants for pharmaceutical and medical aspects: an overview. Medicines 5:93. doi: 10.3390/medicines5030093
Vadassery, J., Reichelt, M., Hause, B., Gershenzon, J., Boland, W., and Mithofer, A. (2012). CML42-mediated calcium signaling coordinates responses to Spodoptera herbivory and abiotic stresses in Arabidopsis. Plant Physiol 159, 1159–1175. doi: 10.1104/pp.112.198150
Villarreal-Garcia, D., Nair, V., Cisneros-Zevallos, L., and Jacobo-Velazquez, D. A. (2016). Plants as biofactories: postharvest stress-induced accumulation of phenolic compounds and glucosinolates in broccoli subjected to wounding stress and exogenous phytohormones. Front. Plant Sci. 7:45. doi: 10.3389/fpls.2016.00045
Vrabec, R., Marikova, J., Locarek, M., Korabecny, J., Hulcova, D., Hostalkova, A., et al. (2022). Monoterpene indole alkaloids from Vinca minor L. (Apocynaceae): Identification of new structural scaffold for treatment of Alzheimer’s disease. Phytochemistry 194:113017. doi: 10.1016/j.phytochem.2021.113017
Wang, B., Sun, W., Li, Q., Li, Y., Luo, H., Song, J., et al. (2015). Genome-wide identification of phenolic acid biosynthetic genes in Salvia miltiorrhiza. Planta 241, 711–725. doi: 10.1007/s00425-014-2212-1
Wang, S., Alseekh, S., Fernie, A. R., and Luo, J. (2019c). The structure and function of major plant metabolite modifications. Mol. Plant 12, 899–919. doi: 10.1016/j.molp.2019.06.001
Wang, L., Lu, W., Ran, L., Dou, L., Yao, S., Hu, J., et al. (2019b). R2R3-MYB transcription factor MYB6 promotes anthocyanin and proanthocyanidin biosynthesis but inhibits secondary cell wall formation in Populus tomentosa. Plant J. 99, 733–751. doi: 10.1111/tpj.14364
Wang, C., Wu, C., Wang, Y., Xie, C., Shi, M., Nile, S., et al. (2019a). Transcription factor OpWRKY3 is involved in the development and biosynthesis of camptothecin and its precursors in Ophiorrhiza pumila hairy roots. Int. J. Mol. Sci. 20:3996. doi: 10.3390/ijms20163996
Wang, J., Li, Y., Wu, T., Miao, C., Xie, M., Ding, B., et al. (2021). Single-cell-type transcriptomic analysis reveals distinct gene expression profiles in wheat guard cells in response to abscisic acid. Funct. Plant Biol. 48, 1087–1099. doi: 10.1071/FP20368
Wang, Z. L., Wang, S., Kuang, Y., Hu, Z. M., Qiao, X., and Ye, M. (2018b). A comprehensive review on phytochemistry, pharmacology, and flavonoid biosynthesis of Scutellaria baicalensis. Pharm Biol. 56, 465–484. doi: 10.1080/13880209.2018.1492620
Wang, N., Liu, W., Zhang, T., Jiang, S., Xu, H., Wang, Y., et al. (2018a). Transcriptomic analysis of red-fleshed apples reveals the novel role of MdWRKY11 in flavonoid and anthocyanin biosynthesis. J. Agric. Food Chem. 66, 7076–7086. doi: 10.1021/acs.jafc.8b01273
Wang, X. C., Wu, J., Guan, M. L., Zhao, C. H., Geng, P., and Zhao, Q. (2020). Arabidopsis MYB4 plays dual roles in flavonoid biosynthesis. Plant J 101, 637–652. doi: 10.1111/tpj.14570
Wang, Y., Guo, D., Li, H. L., and Peng, S. Q. (2013). Characterization of HbWRKY1, a WRKY transcription factor from Hevea brasiliensis that negatively regulates HbSRPP. Plant Physiol. Biochem. 71, 283–289. doi: 10.1016/j.plaphy.2013.07.020
Wani, K. I., Choudhary, S., Zehra, A., Naeem, M., Weathers, P., and Aftab, T. (2021). Enhancing artemisinin content in and delivery from Artemisia annua: a review of alternative, classical, and transgenic approaches. Planta 254:29. doi: 10.1007/s00425-021-03676-3
Wari, D., Aboshi, T., Shinya, T., and Galis, I. (2021). Integrated view of plant metabolic defense with particular focus on chewing herbivores. J. Integr. Plant Biol. 64, 449–475. doi: 10.1111/jipb.13204
Williams, D., Qu, Y., Simionescu, R., and De Luca, V. (2019). The assembly of (+)-vincadifformine- and (-)-tabersonine-derived monoterpenoid indole alkaloids in Catharanthus roseus involves separate branch pathways. Plant J. 99, 626–636. doi: 10.1111/tpj.14346
Xing, B., Liang, L., Liu, L., Hou, Z., Yang, D., Yan, K., et al. (2018). Overexpression of SmbHLH148 induced biosynthesis of tanshinones as well as phenolic acids in Salvia miltiorrhiza hairy roots. Plant Cell Rep. 37, 1681–1692. doi: 10.1007/s00299-018-2339-9
Xu, F., Ning, Y., Zhang, W., Liao, Y., Li, L., Cheng, H., et al. (2014). An R2R3-MYB transcription factor as a negative regulator of the flavonoid biosynthesis pathway in Ginkgo biloba. Funct. Integr. Genomics 14, 177–189. doi: 10.1007/s10142-013-0352-1
Xu, H., Wang, N., Liu, J., Qu, C., Wang, Y., Jiang, S., et al. (2017). The molecular mechanism underlying anthocyanin metabolism in apple using the MdMYB16 and MdbHLH33 genes. Plant Mol. Biol. 94, 149–165. doi: 10.1007/s11103-017-0601-0
Xu, H., Yang, G., Zhang, J., Wang, Y., Zhang, T., Wang, N., et al. (2018). Overexpression of a repressor MdMYB15L negatively regulates anthocyanin and cold tolerance in red-fleshed callus. Biochem. Biophys. Res. Commun. 500, 405–410. doi: 10.1016/j.bbrc.2018.04.088
Xu, Y. H., Wang, J. W., Wang, S., Wang, J. Y., and Chen, X. Y. (2004). Characterization of GaWRKY1, a cotton transcription factor that regulates the sesquiterpene synthase gene (+)-delta-cadinene synthase-A. Plant Physiol. 135, 507–515. doi: 10.1104/pp.104.038612
Xu, Z., Wang, M., Ren, T., Li, K., Li, Y., Marowa, P., et al. (2021). Comparative transcriptome analysis reveals the molecular mechanism of salt tolerance in Apocynum venetum. Plant Physiol. Biochem. 167, 816–830. doi: 10.1016/j.plaphy.2021.08.043
Yahyazadeh, M., Jerz, G., Winterhalter, P., and Selmar, D. (2021). The complexity of sound quantification of specialized metabolite biosynthesis: the stress related impact on the alkaloid content of Catharanthus roseus. Phytochemistry 187:112774. doi: 10.1016/j.phytochem.2021.112774
Yahyazadeh, M., Meinen, R., Hansch, R., Abouzeid, S., and Selmar, D. (2018). Impact of drought and salt stress on the biosynthesis of alkaloids in Chelidonium majus L. Phytochemistry 152, 204–212. doi: 10.1016/j.phytochem.2018.05.007
Yamada, Y., Kokabu, Y., Chaki, K., Yoshimoto, T., Ohgaki, M., Yoshida, S., et al. (2011). Isoquinoline alkaloid biosynthesis is regulated by a unique bHLH-type transcription factor in Coptis japonica. Plant Cell Physiol. 52, 1131–1141. doi: 10.1093/pcp/pcr062
Yan, H., Pei, X., Zhang, H., Li, X., Zhang, X., Zhao, M., et al. (2021). MYB-mediated regulation of anthocyanin biosynthesis. Int. J. Mol. Sci. 22:3103. doi: 10.3390/ijms22063103
Yang, C. Q., Fang, X., Wu, X. M., Mao, Y. B., Wang, L. J., and Chen, X. Y. (2012). Transcriptional regulation of plant secondary metabolism. J. Integr. Plant Biol. 54, 703–712. doi: 10.1111/j.1744-7909.2012.01161.x
Yang, L., Li, J., Ji, J., Li, P., Yu, L., Abd Allah, E. F., et al. (2016). High temperature induces expression of tobacco transcription factor NtMYC2a to regulate nicotine and JA biosynthesis. Front. Physiol. 7:465. doi: 10.3389/fphys.2016.00465
Yang, M., Wang, Q., Liu, Y., Hao, X., Wang, C., Liang, Y., et al. (2021a). Divergent camptothecin biosynthetic pathway in Ophiorrhiza pumila. BMC Biol. 19:122. doi: 10.1186/s12915-021-01051-y
Yang, R., Wang, S., Zou, H., Li, L., Li, Y., Wang, D., et al. (2021b). R2R3-MYB transcription factor SmMYB52 positively regulates biosynthesis of salvianolic acid B and inhibits root growth in Salvia miltiorrhiza. Int. J. Mol. Sci. 22:9538. doi: 10.3390/ijms22179538
Yu, C., Guo, H., Zhang, Y., Song, Y., Pi, E., Yu, C., et al. (2017). Identification of potential genes that contributed to the variation in the taxoid contents between two Taxus species (Taxus media and Taxus mairei). Tree Physiol. 37, 1659–1671. doi: 10.1093/treephys/tpx091
Yu, C., Luo, X., Zhang, C., Xu, X., Huang, J., Chen, Y., et al. (2020). Tissue-specific study across the stem of Taxus media identifies a phloem-specific TmMYB3 involved in the transcriptional regulation of paclitaxel biosynthesis. Plant J. 103, 95–110. doi: 10.1111/tpj.14710
Yu, C., Zhan, X., Zhang, C., Xu, X., Huang, J., Feng, S., et al. (2021). Comparative metabolomic analyses revealed the differential accumulation of taxoids, flavonoids and hormones among six Taxaceae trees. Sci. Hortic. 285:110196. doi: 10.1016/j.scienta.2021.110196
Yu, Z. X., Li, J. X., Yang, C. Q., Hu, W. L., Wang, L. J., and Chen, X. Y. (2012). The jasmonate-responsive AP2/ERF transcription factors AaERF1 and AaERF2 positively regulate artemisinin biosynthesis in Artemisia annua L. Mol. Plant 5, 353–365. doi: 10.1093/mp/ssr087
Zafar, N., Mujib, A., Ali, M., Tonk, D., Gulzar, B., Malik, M. Q., et al. (2020). Cadmium chloride (CdCl2) elicitation improves reserpine and ajmalicine yield in Rauvolfia serpentina as revealed by high-performance thin-layer chromatography (HPTLC). 3 Biotech 10:344. doi: 10.1007/s13205-020-02339-6
Zhan, G., Miao, R., Zhang, F., Chang, G., Zhang, L., Zhang, X., et al. (2020). Monoterpene indole alkaloids with acetylcholinesterase inhibitory activity from the leaves of Rauvolfia vomitoria. Bioorg. Chem. 102:104136. doi: 10.1016/j.bioorg.2020.104136
Zhan, X., Liao, X., Luo, X., Zhu, Y., Feng, S., Yu, C., et al. (2018). Comparative metabolomic and proteomic analyses reveal the regulation mechanism underlying MeJA-induced bioactive compound accumulation in cutleaf groundcherry (Physalis angulata L.) hairy roots. J. Agric. Food Chem. 66, 6336–6347. doi: 10.1021/acs.jafc.8b02502
Zhang, C., Xing, B., Yang, D., Ren, M., Guo, H., Yang, S., et al. (2020). SmbHLH3 acts as a transcription repressor for both phenolic acids and tanshinone biosynthesis in Salvia miltiorrhiza hairy roots. Phytochemistry 169:112183. doi: 10.1016/j.phytochem.2019.112183
Zhang, D., Jiang, C., Huang, C., Wen, D., Lu, J., Chen, S., et al. (2019). The light-induced transcription factor FtMYB116 promotes accumulation of rutin in Fagopyrum tataricum. Plant Cell Environ. 42, 1340–1351. doi: 10.1111/pce.13470
Zhang, J., Morris-Natschke, S. L., Ma, D., Shang, X. F., Yang, C. J., Liu, Y. Q., et al. (2021b). Biologically active indolizidine alkaloids. Med. Res. Rev. 41, 928–960. doi: 10.1002/med.21747
Zhang, S., Zhang, L., Zou, H., Qiu, L., Zheng, Y., Yang, D., et al. (2021c). Effects of light on secondary metabolite biosynthesis in medicinal plants. Front. Plant Sci. 12:781236. doi: 10.3389/fpls.2021.781236
Zhang, H., Zhang, Z., Zhao, Y., Guo, D., Zhao, X., Gao, W., et al. (2021a). StWRKY13 promotes anthocyanin biosynthesis in potato (Solanum tuberosum) tubers. Funct. Plant Biol. 49, 102–114. doi: 10.1071/FP21109
Zhang, X., Abrahan, C., Colquhoun, T. A., and Liu, C. J. (2017). A proteolytic regulator controlling chalcone synthase stability and flavonoid biosynthesis in Arabidopsis. Plant Cell 29, 1157–1174. doi: 10.1105/tpc.16.00855
Zhang, X., Ding, X., Ji, Y., Wang, S., Chen, Y., Luo, J., et al. (2018). Measurement of metabolite variations and analysis of related gene expression in Chinese liquorice (Glycyrrhiza uralensis) plants under UV-B irradiation. Sci. Rep. 8:6144. doi: 10.1038/s41598-018-24284-4
Zhou, F., and Pichersky, E. (2020). More is better: the diversity of terpene metabolism in plants. Curr. Opin. Plant Biol. 55, 1–10. doi: 10.1016/j.pbi.2020.01.005
Zhou, W., Shi, M., Deng, C., Lu, S., Huang, F., Wang, Y., et al. (2021b). The methyl jasmonate-responsive transcription factor SmMYB1 promotes phenolic acid biosynthesis in Salvia miltiorrhiza. Hortic. Res. 8:10. doi: 10.1038/s41438-020-00443-5
Zhou, W., Li, S., Maoz, I., Wang, Q., Xu, M., Feng, Y., et al. (2021a). SmJRB1 positively regulates the accumulation of phenolic acid in Salvia miltiorrhiza. Ind. Crops Prod. 164:113417. doi: 10.1016/j.indcrop.2021.113417
Zhou, Y., Sun, W., Chen, J., Tan, H., Xiao, Y., Li, Q., et al. (2016). SmMYC2a and SmMYC2b played similar but irreplaceable roles in regulating the biosynthesis of tanshinones and phenolic acids in Salvia miltiorrhiza. Sci Rep 6, 22852. doi: 10.1038/srep22852
Ziegler, J., and Facchini, P. J. (2008). Alkaloid biosynthesis: metabolism and trafficking. Annu. Rev. Plant Biol. 59, 735–769. doi: 10.1146/annurev.arplant.59.032607.092730
Zuk, M., Szperlik, J., Hnitecka, A., and Szopa, J. (2019). Temporal biosynthesis of flavone constituents in flax growth stages. Plant Physiol. Biochem. 142, 234–245. doi: 10.1016/j.plaphy.2019.07.009
Keywords: abiotic stress, biotic stress, plant specialized metabolites, plant protection, transcription factor
Citation: Zhan X, Chen Z, Chen R and Shen C (2022) Environmental and Genetic Factors Involved in Plant Protection-Associated Secondary Metabolite Biosynthesis Pathways. Front. Plant Sci. 13:877304. doi: 10.3389/fpls.2022.877304
Received: 16 February 2022; Accepted: 14 March 2022;
Published: 08 April 2022.
Edited by:
Zhihua Liao, Southwest University, ChinaReviewed by:
Qian Shen, Shanghai Jiao Tong University, ChinaGuoyin Kai, Zhejiang Chinese Medical University, China
Copyright © 2022 Zhan, Chen, Chen and Shen. This is an open-access article distributed under the terms of the Creative Commons Attribution License (CC BY). The use, distribution or reproduction in other forums is permitted, provided the original author(s) and the copyright owner(s) are credited and that the original publication in this journal is cited, in accordance with accepted academic practice. No use, distribution or reproduction is permitted which does not comply with these terms.
*Correspondence: Chenjia Shen, c2hlbmNqQGh6bnUuZWR1LmNu; Rong Chen, cm9uZ2NoZW4xOTg0QGh6bnUuZWR1LmNu